- 1Stem Cells and Regenerative Medicine Section, Developmental Biology and Cancer Department, UCL Great Ormond Street Institute of Child Health, University College London, London, United Kingdom
- 2Great Ormond Street Hospital (GOSH), London, United Kingdom
Lung organoids have emerged as powerful tools for studying lung distal diseases by recapitulating the cellular diversity and microenvironment of the lung tissue. This review article highlights the advancements in leveraging mechanobiology and biophysical cues in lung organoid engineering to improve their physiological relevance and disease modelling capabilities. We discuss the role of mechanobiology in lung development and homeostasis, as well as the integration of biophysical cues in the design and culture of lung organoids. Furthermore, we explore how these advancements have contributed to the understanding of lung distal diseases pathogenesis. We also discuss the challenges and future directions in harnessing mechanobiology and biophysical cues in lung organoid research. This review showcases the potential of lung organoids as a platform to investigate the underappreciated impacts of biophysical and biomechanical properties in enhancing lung organoids complexity and functionality, and ultimately provide new insight into embryonic lung development and pulmonary distal diseases pathogenesis.
1 Basics of lung organoids
3D organoids systems allow to re-create the architecture and physiology of human organs in remarkable detail. They also allow to better mimic the in vivo tissue by improving the structural complexity of cell arrangement in culture, including cell polarity. This make 3D organoids an ideal system to be dissected and interrogated for fundamental mechanistic studies on development, regeneration and repair in human tissues, and can also be used in diagnostics, disease modelling, drug discovery and personalized medicine (Kim et al., 2020; Zhao et al., 2022). Despite being powerful in vitro models, organoids present also some limitations, including low scalability, limited lifespan, difficulty in imposing the proper extracellular forces, incomplete matching of organ-level functionality, and lack of vascularization (Tang et al., 2022). To overcome these limitations engineering solutions are in constant development to gain control over self-organization and functionality of 3D organoids (Garreta et al., 2021).
Lung organoids represent a powerful in vitro model to study human lung development and disease modelling (Liberti and Morrisey, 2021). The human developing lung has a complex architecture that comprises up to 144 different cell types/states based on scRNA-seq, scATAC-seq, Visium Spatial Transcriptomics, and mRNA in situ hybridization using Hybridization Chain Reaction (HCR) (Trivedi et al., 2018; He et al., 2022). Various specialized cell types contribute to a normal functional lung for essential respiration. Perturbations of the extracellular environment during development can result in severe pulmonary diseases from birth to adulthood (H.-T. Kim et al., 2018). As high-throughput microscopic imaging is challenging due to the highly complex architecture of lungs, models to faithfully recapitulate lung development and disease are in urgent demand. While 2D cell culture will inevitably fail to fully mimic the spatial responses to the environmental biochemical and biophysical cues (Reviewed more thoroughly by Cunniff et al., 2021), airway epithelial cells-derived lung organoids emerge as a powerful in vitro 3D model that can faithfully recapitulate the pathophysiological aspects of human lungs with different extent of complexity in terms of structure and cell morphology, as well as interaction of different cell types (Huch and Koo, 2015; Sachs et al., 2019). Limitations of 3D organoids related to the difficulty for the inner cells to receive nutrients and exchange the waste with the extracellular environment while growing, could be overcome by culturing organoids in microfluidic devices to facilitate the nutrients and waste circulation and using engineered scaffolds to direct the morphological growth (De Santis et al., 2021). This highlights the importance of biophysical and biomechanical properties in lung organoids culture (Huch and Koo, 2015; Sachs et al., 2019).
Lung organoids can be classified as three major types: i) airway-organoids consisting of secretory club cells, goblet cells, ciliated cells, and bronchiolar epithelial cells; ii) alveolar organoids consisting of alveolar type one (AT1) and alveolar type two (AT2) cells; iii) complex organoids consisting of both proximal and distal airway epithelial cells (Demchenko et al., 2022). In addition, there are two main sources of lung organoids generation: primary lung epithelial progenitor-derived organoids and induced pluripotent stem cell (IPSCs)-derived organoids (Dye et al., 2015; Leeman et al., 2019; Rabata et al., 2020). The generation of primary lung progenitors-derived organoids requires biopsy sections which undergoes single cell suspension through enzymatic digestion. The obtained single cells can be selected against specific cell markers and seeded in Matrigel drops to be further cultured as a more homogenous cell population (Katsura et al., 2020). However, lung organoids generated from progenitors in primary tissues usually lack mesenchymal cells and stromal cells which supplies signalling and structural support (Schutgens and Clevers, 2020). IPSCs-derived organoids are generated from reprogrammed somatic cells, generally skin fibroblasts (Jacob et al., 2019). IPSCs-derived organoids have great advantages in terms of the involvement of both epithelial and mesenchymal cells in the system and retaining genetic information as they can be derived from patients (Choi et al., 2016; Chen et al., 2017). In fact, mesenchymal cells contribute to the maturation of organoids in alveolospheres (Barkauskas et al., 2013). By embedding the lung organoids in Matrigel and applying a cocktail of growth factors and adjusting extracellular matrix (ECM) compositions, different combinations of biochemical and biophysical signals can direct the lung organoids under self-expansion or further differentiation (Figure 1) (Barkauskas et al., 2013; Chen et al., 2017; Sachs et al., 2019).
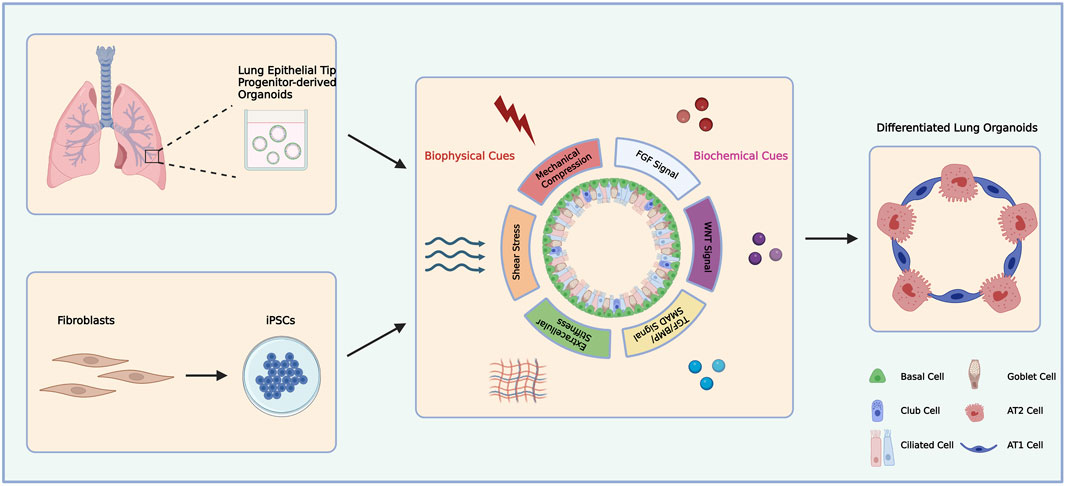
FIGURE 1. Schematic diagram of primary lung epithelial tip progenitor-derived and iPSCs-derived lung organoids differentiation.
Both human primary tissue and IPSCs-derived lung organoids allow studying cell-cell and cell-environment interactions during lung development and disease modelling due to their ability of mimicking in vivo pathophysiological conditions. Several studies used primary tissue and IPSCs-derived lung organoids to model pulmonary diseases, including Idiopathic pulmonary fibrosis (IPF), Chronic obstructive pulmonary disease (COPD), Cystic fibrosis (CF), and respiratory viral infections like COVID-19 (Y. Han et al., 2022; Mou et al., 2012; Strikoudis et al., 2019; Tan et al., 2017; Wilkinson et al., 2017; Wong et al., 2012). The addition of transforming growth factor beta 1 (TGF-β1) to 3D lung organoids containing mesenchymal cells resulted in morphological scarring typically found in IPF, but not in 2D cell culture (Wilkinson et al., 2017). In addition, alveolar organoids derived from human pluripotent stem cells have been used to study the pathogenesis of CF and drug screening. Treatment with TGF-β1 successfully induced fibrotic changes that is characterized in CF. This phenotype is greatly ameliorated by supressing ERK signalling, providing a potential target pathway for CF (Kim et al., 2021).
While the biochemical and genetic aspects of lung development and disease have been widely investigated, the role of mechanical aspects have been investigated in the last 20 years only. This review will shed light on the crucial role of different types of mechanical forces in regulating lung development and disease pathogenesis.
2 Mechanical forces shaping lung morphogenesis and regulating alveolar specification
In the developing lungs, a ramified network of pulmonary epithelium arises from the protrusion of lung bud into the surrounding mesenchyme (Grobstein, 1953; Hogan, 1999). The reciprocal biochemical interactions between lung epithelium and mesenchymal cells are thought to mediate branching morphogenesis. Non-canonical Wnt signalling has been shown to play a role in determining the direction and position of early lung branching. Specifically, Wnt5a-null mutant lungs showed defective cytoskeleton organization and focal adhesion which resulted in perturbed lung branching (Zhang et al., 2022). Peak and others showed that Fgf10-loaded bead can induce ectopic budding of lung epithelium which does not affect the focal adhesion and myosin light chain phosphorylation expression in the protruding area. The ectopic buddings are disrupted when applying epithelium tension by mimicking luminal fluid flow using pharmaceutical treatment, which highlights the crucial role of mechanical forces in lung morphogenesis (Unbekandt et al., 2008; Peak et al., 2022).
Lung epithelial cells are constantly exposed to various mechanical forces throughout development, which have been shown to shape airway tree branching morphogenesis and regulate alveolar specifications. The biochemical signals secreted by the surrounding mesenchymal tissue guide lung branching morphogenesis. Interestingly, this can be overridden if mechanical forces are applied. For instance, the physical instability occurring between the epithelium and the extracellular microenvironment resulted in local morphological protrusion and branching of embryonic airway epithelium independent of the surrounding mesenchyme (Varner et al., 2015). Smooth muscle cells surrounding the airway epithelium during development also contribute to airway morphogenesis. Mouse embryonic airway branching can be altered by manipulating smooth muscle cells differentiation surrounding the airway, likely as a consequence of the high stiffness of the smooth muscle outer layers. By promoting smooth muscle differentiation, lung buddings are inhibited, whereas by inhibiting the smooth muscle differentiation, ectopic branching appears (Goodwin et al., 2019; H. Y; Kim et al., 2015). Bioengineered ‘microfluidic chest cavities’ have been used to generate different levels of pressure, and this allowed regulating the interval of smooth muscle contraction. This confirmed that the physical contraction of smooth muscle directs the lung branching morphogenesis (Nelson et al., 2017). Another study conducted by the Nan Tang group shed light on the key role of mechanical forces in lung branching morphogenesis during early lung development. Using Cre-Shh reporter mice, they distinguished epithelial cells with two types of spindle fibres orientations, i.e., parallel to the long axis and randomly oriented spindle fibres. By embedding epithelial cells in stretchable gels, they found the ratio of normally oriented and randomly oriented spindle fibres are biased when mechanical forces are applied (Tang et al., 2018).
The mechanical forces-regulated cell division pattern contributes to the normal branching morphogenesis. The ablation of the mechanical-responsive transcriptomic activator Yes-associated protein (Yap) in developing mouse lungs has also been shown to affect epithelial cells proliferation and affect branching morphogenesis. There is an uncovered feedback regulation between Yap and myosin light chain phosphorylation which represents the mechanical force responsive signalling mediator. Deletion of Yap results in downregulated myosin light chain phosphorylation (Lin et al., 2017). The relationship between mechanical forces and regulated cell proliferation greatly impacted on normal lung branching morphogenesis. Despite the role of mechanical forces in early lung branching process, they also regulate the alveolar specification at later sacculation stage. In vivo and ex vivo stretching of whole lungs induces activation of Yap/Taz signalling which promotes AT1 differentiation. By using ROCK inhibitor, Y27632, AT1 cells significantly decreased, which suggests the regulation of AT1 differentiation through ROCK-YAP/Taz signalling pathway (Nguyen et al., 2021). The Nan Tang group showed the shear stress, the force exerted by the flowing amniotic fluid within the epithelial air tree to the surface of epithelium, regulates AT1 and AT2 fate decision. They suggested progenitors protrude from the epithelium due to the attraction of Fgf10 signalling from the surrounding stromal cells, and myosin accumulates at the apical surface of the protruding epithelium. The inhalation of amniotic fluid exerts shear stress to the epithelium, where the protruding cells are protected from the mechanical force by the accumulated myosin and become AT2 cell which remains cuboidal shape. In contrast, cells subjected to shear stress differentiate into flattened AT1 cells (Li et al., 2018). While further studies are needed to uncover the underlying molecular mechanisms of mechanical forces-regulated branching morphogenesis and alveolar specification, it is evident that mechanical forces have a key role in lung development. The reciprocal interactions between mechanical forces and biochemical signals may be the key to further decipher the process of lung branching morphogenesis and further alveolar specification.
3 Biomechanical signalling in lung homeostasis and diseases
The major function of the lungs is gas exchange. As such, the mechanical forces exerted to the lung epithelium by the constant breathing movement play an important role in maintaining tissue homeostasis and epithelial cell fate decision (Shiraishi et al., 2023). Perturbation of the mechanical forces can greatly impact on lung function, leading to pathogenesis. AT1 are flattened, non-proliferative alveolar cells, which are mainly responsible for gas exchange and account for 95% of alveolar surface. AT2 are cuboidal proliferative epithelial cells that secret surfactant proteins to prevent the collapse of the alveolar units during breathing. The proliferative ability of AT2 cells and the secretion of surfactant proteins are promoted by the mechanical stress generated by ventilation, which maintains the integrity of the alveolar epithelium (Arold et al., 2009; B; Han, 2005). The mechanical forces generated by ventilation and exerted to the airway epithelium by various environmental stiffness regulate the activity of Yap by manipulating the shape of nuclei to control the nuclear influx rate of Yap in airway epithelium (Elosegui-Artola et al., 2017). The activity of Yap is crucial for maintaining the balanced differentiation of club cells and goblet cells. Ablation of Yap in the proximal epithelium directs the trans-differentiation of goblet cells into club cells, therefore altering the epithelial homeostasis (Hicks-Berthet et al., 2021). Recent studies have shown surprising postnatal AT1 plasticity in reprogramming into AT2 upon inhibition of Yap signalling. Nuclear Yap activity is required to maintain the AT1 identity (Penkala et al., 2021). Another study suggests the reprogramming of AT1 into AT2 in response to mechanical cues through extracellular matrix-cytoskeleton, mediated by Cdc42 and Ptk2. The loss of mechanical constraint in AT1 cells results in rapid AT1-AT2 trans-differentiation. These findings suggest the crucial role of mechanical forces exerted by breathing in maintaining AT1 and AT2 homeostasis in postnatal lung (Shiraishi et al., 2023). In addition, it has been suggested that myeloid cells are responsive to the cyclic hydrostatic pressure through mechanical-responsive ion channel PIEZO1 which, in turn, regulates the innate immune system of the lung via c-Jun activation (Solis et al., 2019).
As epithelial cells along the airways have been shown to be mechano-sensitive and mechano-responsive, the disruption of the mechanical forces can result in altered tissue homeostasis and lung disease, such as COPD and emphysema, characterized by the accumulation of ectopic levels of elastin and collagen fibres, resulting in alveolar destruction (Kononov et al., 2001). Lung fibrosis is caused by over-proliferation of fibroblasts and, consequently, the accumulation of extracellular matrix proteins. IPF is the most common type of pulmonary fibrosis, characterized by impaired alveolar functions, mainly due to AT2 cells loss (Plantier et al., 2011). Extracellular matrix-cytoskeleton interactions regulated by Cdc42 in AT2 cells play an important role in IPF progression. Cdc42 ablation in AT2 cells results in failure of AT2-AT1 transition which exposes alveolar type cells to the constant mechanical forces from the extracellular matrix. The accumulated mechanical stress, in turn, activates TGF-β signalling that mediates further peripheral-central IPF progression (Wu et al., 2020). These findings, that highlight the connection between mechanical forces and pulmonary pathogenesis, are determinant for building up a patho-physiologically relevant disease model.
4 Scaffold materials promoting lung organoids structural organization and maturation
Although numerous studies have successfully reported the generation of lung organoids derived from pluripotent stem cells comprising multiple differentiated epithelial cells, the differentiated cells retain embryonic rather than mature characteristics, as revealed by transcriptomic analysis (Barkauskas et al., 2013; Firth et al., 2014; Dye et al., 2015; Konishi et al., 2016).
Different types of ECM provide mechanical, biophysical and signalling cues in a variety of developing organisms and tissues. The ECM helps to regulate tissue morphology during embryonic development by integrating cell genetic programs with chemical and mechanical input from the local microenvironment (Walma and Yamada, 2020). This implies that epithelial cells continually remodel their intercellular contacts throughout morphogenesis, which significantly rearranges cells within the epithelial sheet (H. Y. Kim et al., 2013). Interestingly, epithelial cells themselves contribute to producing ECM components which finally determines their differentiation towards specific cell types (Mamidi et al., 2018; Fernandez Vallone et al., 2020; Michielin et al., 2020). Lung alveolarization requires precise coordination of cell growth and differentiation with extracellular matrix (ECM) synthesis and deposition to allow alveolar septation. Laminin, which is a key component of the basement matrix in the developing lungs by supporting first epithelial branching and then capillary network formation, displays a simplified network along with the thinning of the alveolar septa. Conversely, elastin density is continuously increasing during alveologenesis as recently evidenced by 3D volumetric analysis of mouse and human lung tissues (Luo et al., 2018). This allows for proper maturation of elastin fibres to ensure elastic recoil of the lung during breathing. ECM deposition defects and alveolar simplification underlie many pulmonary diseases such as emphysema that results from the enzymatic destruction of ECM components leading to destruction of alveolar walls and airspace enlargement. Interestingly, alterations of the actomyosin network during development, for example, due to mutation in genes encoding cytoskeletal proteins, has been shown to repress metalloproteinase activity leading to disrupted ECM remodelling (H.-T. Kim et al., 2018).
Scaffold biomaterials can, therefore, provide physical and biochemical properties to promote maturation of lung organoids (De Santis et al., 2021), as previously reported (Xinaris et al., 2012; Watson et al., 2014; Finkbeiner et al., 2015). To enhance lung organoids engraftment, Dye et al. introduced an alternative niche consisting in microporous poly (lactide-co-glycolide) (PLG) scaffolds for the lung organoids to grow, which was previously utilized for the survival, and functionalization of human islet upon transplantation (Gibly et al., 2013; Hlavaty et al., 2014; Dye et al., 2016). These scaffolds provide a rigid surface for the epithelial cells to adhere with porous structures that allows further growth and vasculature of the epithelium (Figure 2B). Thanks to the scaffolds support, transplanted human lung organoids can form pseudostratified layers structurally similar to the adult airway. In addition, CC10+ secretory club cells and MUC5AC + goblet cells have been detected, which were not or rarely detected in foetal lung airway but found within adult airway epithelium. The retrieved scaffold after in vivo culturing also showed high level of vasculature with various types of mesenchymal tissues. The structure and cellular maturation of lung organoids are promoted upon the introduction of PLG scaffolds, whereas the lack of alveolar cells suggests further improvement is required for a full maturation (Dye et al., 2016).
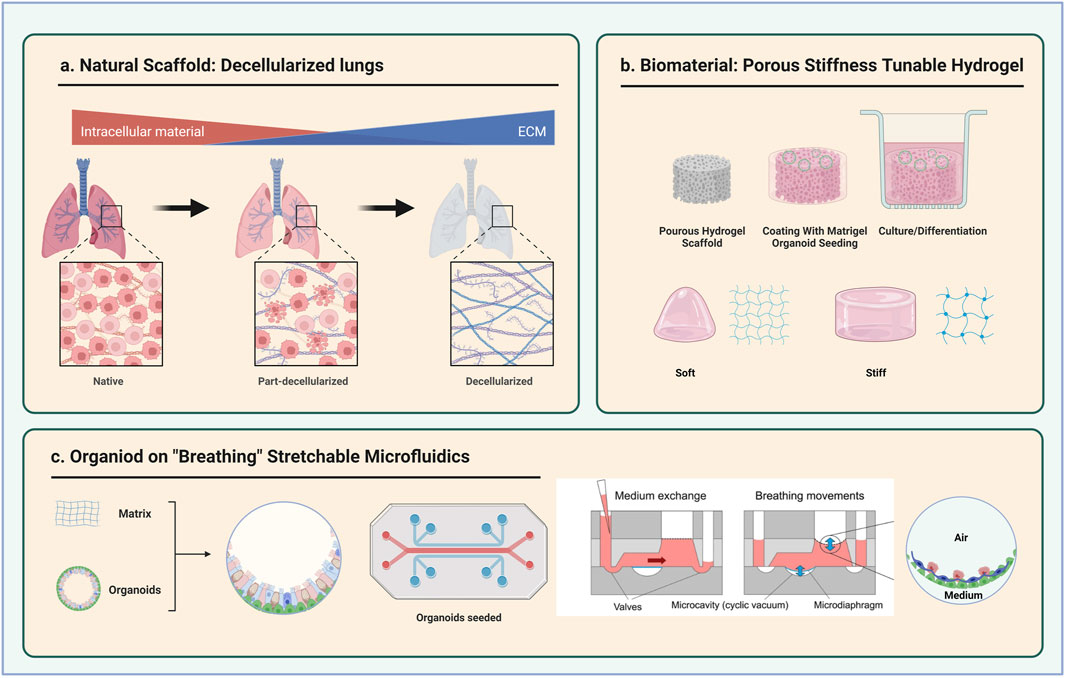
FIGURE 2. Schematic diagram of three types of scaffolds for lung organoids culture. (A) Decellularized lungs (B) Porous and stiffness tuneable hydrogel (C) Stretchable microfluidic devices. Partly taken from Stucki et al., 2018.
Another natural scaffold for lung epithelium culture consists in the decellularization of the whole lungs preserving its original acellular extracellular matrix (Gilbert et al., 2006; Tommasini et al., 2023) (Figure 2A). Recellularization of the decellularized lungs using lung progenitors derived from human IPSCs have shown successful expansion and alveolar specification of AT2 progenitors (Gilpin et al., 2014; Leiby et al., 2023). Of note, the seeding of human lung organoids on the decellularized lung airway showed minimal or no club cell and goblet cell differentiation (Dye et al., 2015). Further characterization of the acellular lungs in terms of mechanical properties and stiffness will provide better insight into the role of the mechanical properties in guiding the maintenance and differentiation of epithelial cells (Melo et al., 2014).
As organoids are usually spherical in shape with multiple layers of cells, the growth factors, nutrients and oxygen poorly diffuse into the deeper layers of the organoids. From this perspective, microfluidics-based organoid-on-chip devices provide a platform for organoids to grow with a fine control of chemical and physical parameters (Bhatia and Ingber, 2014; Giulitti et al., 2016). These devices are characterized by membranes-integrated multi-micro-channels and holes from multiple dimensions filled with fluids which allow continuous perfusion of cells in culture. The membranes can separate different compartments in the device with independent channels, thus allowing the co-culture of alveolar epithelial cells and endothelial cells (Huh et al., 2010). In addition, a “breathing” microfluidics-based model has been developed by the Guenat group to mimic the mechanical stretches applied to the alveolar epithelial cells and showed successful alveolar function (Stucki et al., 2018) (Figure 2C). The incorporation of such “breathing” lung model with scalable mechanical stimuli and adjustable architecture and the 3D lung organoids from different developmental stages has great potential for studying the role of mechanical forces in instructing lung architecture organization and epithelial differentiation.
In summary, the three types of scaffolds provide adhesion sites for lung organoids to grow and are capable of maintaining the survival of progenitors, as well as of leading to lineage specification with respective advantages and drawbacks (Table 1). This suggests the crucial role of physical environment in tissue organization and cellular differentiation.
5 Biophysical stimulation methods for enhancing lung organoids functionality
The crucial role of mechanical forces in embryonic lung development and adult lung homeostasis have been elucidated in the previous section. Mechanical stretching generates tensional force against the limited range of motion of the material due to its elastic physical property such as stiffness. The implementation of various biophysical stimulations, such as mechanical stretching mimicking fluid or air flow and environmental stiffness, is, therefore, likely to enhance the maturation and functionality of lung organoids. Previous studies showed significant upregulation of Surfactant protein b (SPB) and c (SPC) in isolated rat embryonic AT2 cells at late developmental stages in response to mechanical cyclic stretch (Sanchez-Esteban et al., 2001). The Tang group also showed the role of shear stress in regulating alveolar differentiation, where tip progenitor epithelial cells exposed to mechanical stress become flattened and differentiate into AT1 cells, whereas those protected from the stress by myosin accumulation differentiate into AT2 cells (Li et al., 2018). In contrast, lung alveolar organoids derived from tumour-free tissue from patients undergoing lung resection surgery for lung cancer or from emphysematous tissue cultured in a chip with stretchable polydimethylsiloxane (PDMS) membrane showed no variation of mRNA levels in SPB and SPC upon stretching (van Riet et al., 2022). It is, therefore, possible that the alveolar cells from postnatal tissue or from adult lung disease patients’ tissue are less responsive to the mechanical stretch in terms of surfactant protein production. Of note, the conventional in vitro AT2 cell culture tends to trans-differentiate into AT1 cells over time (Mao et al., 2015), whereas the culture of AT2 organoids in the stretchable chip maintains the AT2 phenotype for longer time without trans-differentiating to AT1 cells (van Riet et al., 2022). This study suggested a potential role of mechanical stretch in alveolar type cells maintenance. Further validation of the role of mechanical stretch in enhancing embryonic alveolar maturation by growing embryonic lung tip epithelial progenitors-derived organoids on the breathing device is needed. By applying constant cyclic stretch to the organoids derived from postanal day5 mouse lungs with co-culture of fibroblast and CD326-positive cells, expression of mesenchymal genes is significantly upregulated in lung organoids. Upon lateral stretching, the population of α-smooth muscle actin (αSMA)- and platelet-derived growth factor receptor-α (PDGFRα)-positive cells increased with highly activated transforming growth factor-beta (TGF-β) signalling (Joshi et al., 2022). The PDGFRα-positive mesenchymal cells have been shown to provide physical support to maintain the proliferative ability of AT2 cells in adult mouse lungs. A reduced number of PDGFRα-positive cells resulted in depletion of proliferative AT2 population with impaired alveologenesis (Gao et al., 2022; Riccetti et al., 2022). As the only surfactant protein-associated gene detected in the lung organoids derived by Joshi and others is surfactant protein D, which is secreted by the proximal airway epithelial cells, it is believed no alveolar cells are included in their organoid system. It would be interesting to investigate further if cyclic stretch increases the population of mesenchymal cells. This may promote alveologenesis or contribute to alveolar maintenance in embryonic lung development and adult lung homeostasis. This investigation can be done using alveolar cells-integrated organoids derived from embryonic and adult lung tissue.
Despite the importance of mechanical stretch in embryonic lung development and postnatal lung homeostasis, the impact of environmental stiffness in proximal and distal airway epithelial differentiation and alveolar maintenance is still understudied. Various pulmonary diseases, such as COPD and IPF, are characterized by pathologic stiffening of the environmental ECM. By seeding primary rat alveolar epithelial cells in polyacrylamide gel substrates of varying stiffness, no influence of substrate stiffness in alveolar differentiation, specifically in AT2-AT1 transition was detected (Jones, 2011). However, 2D primary cell culture cannot faithfully recapitulate the interactions between ECM and epithelial cells in vivo. The combination of 3D lung organoids model and stiffness-tuneable 3D synthetic hydrogels (Urciuolo et al., 2023) may fill the gap of the impacts of environmental stiffness in epithelial specification, especially alveolar differentiation and maintenance.
6 Recapitulating disease phenotypes and pathogenesis using biophysical cues
Many pulmonary disorders are associated with various forms of mechanical stimuli perceived by cells in the lung. These stimuli derive from the pathogenic alteration of ECM stiffness, like in IPF and COPD, or from direct mechanical compression, like in Congenital Diaphragmatic Hernia (CDH), where the ectopic protrusion of abdominal organs hinders the developing lungs in the thoracic cavity (Edel et al., 2021; Nho et al., 2022). IPF is characterized by the failure of AT2-AT1 transitioning and bronchiolization of the distal epithelial cells, along with accumulation of fibroblast and differentiated myofibroblast in the distal ECM, which results in local stiffening of the extracellular environment. The local accumulation of fibroblasts is associated with increased migration and proliferation via the activation of multiple signalling pathways such as TGF-β signalling, and deposition of ECM proteins (Selman and Pardo, 2002; Selman, 2006). Schruf and others successfully established an iPSC-derived AT2 organoid that recapitulates airway epithelial-like expression signature in IPF through the treatment of 9 IPF-relevant molecules cocktails (Schruf et al., 2020). Strikoudis and others modelled embryonic stem cell-derived fibrotic organoids using CRISPR-Cas9 gene editing, which showed high similarities in gene expression compared to IPF patients. They documented the important role of interleukin-11 in fibrosis during IPF pathogenesis (Strikoudis et al., 2019). Despite the success in recapitulating the gene expression profiles observed in IPF patients by gene editing or applying biochemical signals, the role of biophysical signals in IPF pathogenesis are still underappreciated. As such, can the disease phenotype and pathogenesis be better recapitulated by integrating biophysical cues to lung organoids models? A recent study conducted by the Magin group revealed the key role of environmental stiffness in IPF pathogenesis through the crosstalk between fibroblasts and AT2 cells (Caracena et al., 2022). A 3D co-culture of fibroblasts and AT2 cells were embedded in PEG-based hydrogels with tuneable stiffness, that recapitulates the mechanical properties of healthy and fibrotic lungs. In stiff conditions, the upregulation of profibrotic genes is highly promoted with upregulated ECM remodelling genes that has been shown to contribute to pathogenic fibrogenesis condition in IPF. Surprisingly, AT2 cells are also stiffness-responsive and their crosstalk with fibroblasts contributes to the myofibroblast differentiation through TGF-β activation. The presence of AT2 cells synergistically promote fibrosis with the high-stiffness microenvironment (Caracena et al., 2022). By manipulating mechanical properties alone, the pathogenesis of IPF was promoted. The emergence of a transient population of epithelial cells in the 3D model was identified in IPF condition in vivo. It would be, therefore, interesting to perform RNA-sequencing on the transient population of cells to compare the transcriptional profiles with the epithelial cells in IPF tissue to evaluate the level of recapitulation of IPF pathogenesis in addition to evaluating the level of fibrogenesis. Moreover, additional biochemical signals can be integrated into the 3D model together with the biophysical cue to study the conditions of fibroblasts activation.
Despite the cause of CDH remain elusive, growing evidence has suggested the key role of mechanical compression exerted to the developing lungs since the pseudoglandular stage from the herniated abdominal content in causing pulmonary hypoplasia (Nelson et al., 2017). Mechanical compression is recapitulated in the nitrofen-exposed rat lung to create an ex vivo CDH model. The transcriptomic profile of these lungs showed downregulation of Spc and Pdpn suggesting impaired alveolar functionality (Fox et al., 2018). A human CDH foetus-derived iPSCs lung organoids model including mesenchymal cells and epithelial cells was also generated to study the effects of mechanical compression in CDH pathogenesis. Interestingly, comparing lung organoids generated from CDH patients and healthy embryonic lungs without mechanical compression, the expression levels of SPB, SPC, NKX2.1, SOX2, SOX9 and PDGFRα were significantly downregulated in CDH organoids. This suggests an inherently impairment of epithelial progenitors and alveolarization in CDH-organoids development. By applying mechanical compression ex vivo to the CDH-organoids, the expression of SOX2, SOX9, NKX2.1, PDPN and Ki67 are remarkably downregulated, which suggests further disturbed progenitor identity, alveolar function and proliferative ability (Kunisaki et al., 2021). Although the iPSCs-derived lung organoids model with integrated mechanical compression recapitulate multiple aspects of CDH pathogenesis, a functional evaluation of the alveolar epithelial cells is still missing. Due to the time course of iPSC generation and organoids culture, the mechanical compression is only exerted to the organoid system at one time point which may not faithfully mimic the prolonged mechanical compression to the developing lungs in vivo. Thus, a model that can provide constant mechanical compression to the lung organoid, for example, using gels with tuneable stiffness, may create a more reliable in vitro CDH model to study its pathogenesis.
Overall, by integrating biophysical signals, the phenotypes and pathogenesis of pulmonary diseases are better recapitulated in 3D organoid cultures.
7 Conclusion and future perspectives
Organoids have been shown to be a robust model to successfully recapitulate key transcriptional signatures of healthy and diseased tissue, although they often turn to be functionally immature. A better understanding of cellular mechanisms is required and could be achieved by integrating biophysical cues in culture to develop a more reliable organoids-based in vitro model.
Alveoli are the functional units for gas exchange which is the major function of the lungs. Alterations in their development cause malfunctions and represent the basis of many pulmonary distal diseases. It is well recognized that mesenchymal cells can sense the mechanical forces and influence epithelial cells behaviour. However, how the epithelial progenitors and alveolar epithelial cells receive and respond to the mechanical signals remain poorly studied (Zepp et al., 2021). Emerging studies revealed the astonishing plasticity of AT1 and AT2 cells in response to the mechanical stress from various sources. Different types of scaffolds mimicking physical properties of the in vivo environment and the integration of biophysical cues have been developed to promote the maturation of lung organoids and showed different levels of success (van Riet et al., 2022). This is supported by the culture of AT2 cells in decellularized lung scaffolds with co-culture of endothelial, epithelial cells and fibroblast. It was found that mechanical stretch alone is unable to affect AT1 differentiation when high levels of Wnt and FGF agonists are present. However, a Wnt- and FGF-low environment synergizes with tidal-level mechanical strain to promote AT2 differentiation to AT1s within the engineered lung tissues (Leiby et al., 2023). Although challenging, in the future, the combination of biophysical and biochemical cues at different stages in culturing lung organoids should be performed and may be crucial to further promote their maturity.
Despite different levels of organoids maturation promoted by PLG scaffolds and decellularized lung scaffolds, there is an obvious limitation in both methods due to the uncontrollable physical and biochemical parameters in culture. Microfluidic devices may overcome these disadvantages thanks to the integration of sensors that can detect the alterations of these parameters. Mechanical inputs such as through membrane stretching and shear stress exerted by air or fluid flow have also some limitations related to the local control of the stimuli. Synthetic hydrogel scaffolds possess physical properties that mimics in vivo environment. The combination of hydrogels and microfluidics may maximise their individual advantages, to further promote organoids maturation.
IPSCs-derived lung organoids have been favoured as the model to study embryonic development and pathogenesis due to their availability, scalability and plasticity that allows to differentiate them into the desired state. Adult cells-derived organoids, although more difficult to be established in vitro, are valuable in studying disease pathology as well. For example, primary human nasopharyngeal and bronchial organoids are derived from COPD patient, consisting of goblet, club and ciliated cells. The COPD lung organoids exhibit hallmarks of COPD pathologic conditions including hyperplasic goblet cells and lowered beating frequency ciliated cells. The SARS-CoV-2 infection are more severe in the bronchial region with greater pro-inflammation response in COPD organoids. The COPD lung organoids provided a model to study COPD pathology and the pathogen-host interaction (Chan et al., 2022). The pathological characteristics retained in the patients’ adult cells-derived organoids make them invaluable as the model for disease pathology study and drug screening. Dysregulation of the mechanical forces or physical properties may result in malfunctioning of alveolar cells and lead to severe pulmonary diseases. Thus, it is crucial to understand the role of mechanical forces in lung development and epithelial maintenance to create more mature in vitro lung organoids to model lung development and disease pathogenesis. Different tissue compositions surrounding the airway epithelium potentially create a differential gradient of environmental stiffness in the adult lungs. Specifically, there is a gradual loss of cartilage and smooth muscles surrounding the proximal-distal airway axis. Both cartilage and smooth muscle cells are completely missing in the alveolar region and the tissue is enriched with soft capillaries for gas exchange (Badri et al., 2008; Nikolić et al., 2018). This suggests a potential spatio-temporal stiffness gradient change along the airway during lung development, which may also contribute to the different epithelial cell specification. The potential spatio-temporal stiffness gradient can be measured using Atomic Force Microscopy (AFM), which will give insight into how differential stiffness relates to epithelial commitments. A high environmental stiffness has been suggested to promote pathogenesis of IPF through the regulation of myofibroblast differentiation and fibroblast-AT2 cells crosstalk (Caracena et al., 2022). Fibroblasts and lung epithelial cells are stiffness-responsive, which confirms the importance of the fine-established environmental stiffness along the airway. Alteration of mechanical stiffness displayed by the surrounding tissues and the resulted dysregulated signalling likely affects IPF pathogenesis. In addition, the prolonged mechanical compression exerted to the lungs will result in alterations of mechanical-responsive signalling in epithelial progenitors and alveolar epithelial cells which may lead to changes in their fate decisions. Impaired alveolarization will greatly impact the postnatal lung function and is associated with high mortality. Therefore, in the next future researches should focus on the underlying molecular mechanisms of different mechanical forces in regulating epithelial commitment and maintenance during lung development and adulthood homeostasis. By manipulating the molecular pathways in the lung disease organoid model, the pathogenesis and potential therapeutic targets of the pulmonary diseases can be better investigated.
In conclusion, lung organoids represent a robust in vitro model to study lung development and disease pathogenesis. Mechanical forces are crucial in lung development in terms of epithelial commitment and maintenance. As such, combining biochemical and biophysical cues will possibly lead to the next level of organoids-based disease modelling.
Author contributions
FM: Writing–original draft, Writing–review and editing. ZS: Writing–original draft, Writing–review and editing. PD: Writing–review and editing.
Funding
This work is supported by the Longfonds Voorhen Astma Fonds BREATH Consortium (Award 552269 to PD), a Sparks-GOSH grant (to PD and FM) and the NIHR Great Ormond Street Hospital Biomedical Research Centre. FM is supported by a NIHR Great Ormond Street Hospital BRC Catalyst Fellowship. PD is supported by National Institute for Health Research (NIHR-RP-2014-04-046).
Acknowledgments
This is a short text to acknowledge the contributions of specific colleagues, institutions, or agencies that aided the efforts of the authors.
Conflict of interest
The authors declare that the research was conducted in the absence of any commercial or financial relationships that could be construed as a potential conflict of interest.
The author PDC declared that they were an editorial board member of Frontiers at the time of submission. This had no impact on the peer review process and the final decision.
Publisher’s note
All claims expressed in this article are solely those of the authors and do not necessarily represent those of their affiliated organizations, or those of the publisher, the editors and the reviewers. Any product that may be evaluated in this article, or claim that may be made by its manufacturer, is not guaranteed or endorsed by the publisher.
References
Arold, S. P., Bartolák-Suki, E., and Suki, B. (2009). Variable stretch pattern enhances surfactant secretion in alveolar type II cells in culture. Am. J. Physiology-Lung Cell. Mol. Physiology 296 (4), L574–L581. doi:10.1152/ajplung.90454.2008
Badri, K. R., Zhou, Y., and Schuger, L. (2008). Embryological origin of airway smooth muscle. Proc. Am. Thorac. Soc. 5 (1), 4–10. doi:10.1513/pats.200704-049VS
Barkauskas, C. E., Cronce, M. J., Rackley, C. R., Bowie, E. J., Keene, D. R., Stripp, B. R., et al. (2013). Type 2 alveolar cells are stem cells in adult lung. J. Clin. Investigation 123 (7), 3025–3036. doi:10.1172/JCI68782
Bhatia, S. N., and Ingber, D. E. (2014). Microfluidic organs-on-chips. Nat. Biotechnol. 32 (8), 760–772. doi:10.1038/nbt.2989
Caracena, T., Blomberg, R., Hewawasam, R. S., Fry, Z. E., Riches, D. W. H., and Magin, C. M. (2022). Alveolar epithelial cells and microenvironmental stiffness synergistically drive fibroblast activation in three-dimensional hydrogel lung models. Biomaterials Sci. 10 (24), 7133–7148. doi:10.1039/D2BM00827K
Chan, L. L., Anderson, D. E., Cheng, H. S., Ivan, F. X., Chen, S., Kang, A. E., et al. (2022). The establishment of COPD organoids to study host-pathogen interaction reveals enhanced viral fitness of SARS-CoV-2 in bronchi. Nat. Commun. 13 (1), 7635. doi:10.1038/s41467-022-35253-x
Chen, Y.-W., Huang, S. X., de Carvalho, A. L. R. T., Ho, S.-H., Islam, M. N., Volpi, S., et al. (2017). A three-dimensional model of human lung development and disease from pluripotent stem cells. Nat. Cell Biol. 19 (5), 542–549. doi:10.1038/ncb3510
Choi, J., Iich, E., and Lee, J.-H. (2016). Organogenesis of adult lung in a dish: differentiation, disease and therapy. Dev. Biol. 420 (2), 278–286. doi:10.1016/j.ydbio.2016.10.002
Cunniff, B., Druso, J. E., and van der Velden, J. L. (2021). Lung organoids: advances in generation and 3D-visualization. Histochem. Cell Biol. 155 (2), 301–308. doi:10.1007/s00418-020-01955-w
De Santis, M. M., Michielin, F., Shibuya, S., de Coppi, P., and Wagner, D. E. (2021). Lung tissue bioengineering for transplantation and modelling of development, disease and regeneration in Lung stem cells in development, health and disease. Editors M. Z. Nikolić, and B. L. M. Hogan European Respiratory Society, 248–272. doi:10.1183/2312508X.10011020
Demchenko, A., Lavrov, A., and Smirnikhina, S. (2022). Lung organoids: current strategies for generation and transplantation. Cell Tissue Res. 390 (3), 317–333. doi:10.1007/s00441-022-03686-x
Dye, B. R., Dedhia, P. H., Miller, A. J., Nagy, M. S., White, E. S., Shea, L. D., et al. (2016). A bioengineered niche promotes in vivo engraftment and maturation of pluripotent stem cell derived human lung organoids. ELife 5, e19732. doi:10.7554/eLife.19732
Dye, B. R., Hill, D. R., Ferguson, M. A., Tsai, Y.-H., Nagy, M. S., Dyal, R., et al. (2015a). In vitro generation of human pluripotent stem cell derived lung organoids. ELife 4, e05098. doi:10.7554/eLife.05098
Edel, G. G., Schaaf, G., Wijnen, R. M. H., Tibboel, D., Kardon, G., and Rottier, R. J. (2021). Cellular origin(s) of congenital diaphragmatic hernia. Front. Pediatr. 9, 804496. doi:10.3389/fped.2021.804496
Elosegui-Artola, A., Andreu, I., Beedle, A. E. M., Lezamiz, A., Uroz, M., Kosmalska, A. J., et al. (2017). Force triggers YAP nuclear entry by regulating transport across nuclear pores. Cell 171 (6), 1397–1410.e14. doi:10.1016/j.cell.2017.10.008
Fernandez Vallone, V., Leprovots, M., Ribatallada-Soriano, D., Gerbier, R., Lefort, A., Libert, F., et al. (2020). LGR 5 controls extracellular matrix production by stem cells in the developing intestine. EMBO Rep. 21 (7), e49224. doi:10.15252/embr.201949224
Finkbeiner, S. R., Hill, D. R., Altheim, C. H., Dedhia, P. H., Taylor, M. J., Tsai, Y.-H., et al. (2015). Transcriptome-wide analysis reveals hallmarks of human intestine development and maturation in vitro and in vivo. Stem Cell Rep. 4 (6), 1140–1155. doi:10.1016/j.stemcr.2015.04.010
Firth, A. L., Dargitz, C. T., Qualls, S. J., Menon, T., Wright, R., Singer, O., et al. (2014). Generation of multiciliated cells in functional airway epithelia from human induced pluripotent stem cells. Proc. Natl. Acad. Sci. 111 (17), E1723–E1730. doi:10.1073/pnas.1403470111
Fox, Z. D., Jiang, G., Ho, K. K. Y., Walker, K. A., Liu, A. P., and Kunisaki, S. M. (2018). Fetal lung transcriptome patterns in an ex vivo compression model of diaphragmatic hernia. J. Surg. Res. 231, 411–420. doi:10.1016/j.jss.2018.06.064
Gao, F., Li, C., Danopoulos, S., Al Alam, D., Peinado, N., Webster, S., et al. (2022). Hedgehog-responsive PDGFRa(+) fibroblasts maintain a unique pool of alveolar epithelial progenitor cells during alveologenesis. Cell Rep. 39 (1), 110608. doi:10.1016/j.celrep.2022.110608
Garreta, E., Kamm, R. D., Chuva de Sousa Lopes, S. M., Lancaster, M. A., Weiss, R., Trepat, X., et al. (2021). Rethinking organoid technology through bioengineering. Nat. Mater. 20 (2), 145–155. doi:10.1038/s41563-020-00804-4
Gibly, R. F., Zhang, X., Lowe, W. L., and Shea, L. D. (2013). Porous scaffolds support extrahepatic human islet transplantation, engraftment, and function in mice. Cell Transplant. 22 (5), 811–819. doi:10.3727/096368912X636966
Gilbert, T., Selloro, T., and Badylak, S. (2006). Decellularization of tissues and organs. Biomaterials 27, 3675–3683. doi:10.1016/j.biomaterials.2006.02.014
Gilpin, S. E., Ren, X., Okamoto, T., Guyette, J. P., Mou, H., Rajagopal, J., et al. (2014). Enhanced lung epithelial specification of human induced pluripotent stem cells on decellularized lung matrix. Ann. Thorac. Surg. 98 (5), 1721–1729. doi:10.1016/j.athoracsur.2014.05.080
Giulitti, S., Zambon, A., Michielin, F., and Elvassore, N. (2016). Mechanotransduction through substrates engineering and microfluidic devices. Curr. Opin. Chem. Eng. 11, 67–76. doi:10.1016/j.coche.2016.01.010
Goodwin, K., Mao, S., Guyomar, T., Miller, E., Radisky, D. C., Košmrlj, A., et al. (2019). Smooth muscle differentiation shapes domain branches during mouse lung development. Development 146, dev181172. doi:10.1242/dev.181172
Grobstein, C. (1953). Inductive epithelio-mesenchymal interaction in cultured organ rudiments of the mouse. Science 118 (3054), 52–55. doi:10.1126/science.118.3054.52
Han, B. (2005). Ventilator-induced lung injury: role of protein-protein interaction in mechanosensation. Proc. Am. Thorac. Soc. 2 (3), 181–187. doi:10.1513/pats.200501-008AC
Han, Y., Yang, L., Lacko, L. A., and Chen, S. (2022). Human organoid models to study SARS-CoV-2 infection. Nat. Methods 19 (4), 418–428. doi:10.1038/s41592-022-01453-y
He, P., Lim, K., Sun, D., Pett, J. P., Jeng, Q., Polanski, K., et al. (2022). A human fetal lung cell atlas uncovers proximal-distal gradients of differentiation and key regulators of epithelial fates. Cell 185 (25), 4841–4860.e25. doi:10.1016/j.cell.2022.11.005
Hicks-Berthet, J., Ning, B., Federico, A., Tilston-Lunel, A., Matschulat, A., Ai, X., et al. (2021). Yap/Taz inhibit goblet cell fate to maintain lung epithelial homeostasis. Cell Rep. 36 (2), 109347. doi:10.1016/j.celrep.2021.109347
Hlavaty, K. A., Gibly, R. F., Zhang, X., Rives, C. B., Graham, J. G., Lowe, W. L., et al. (2014). Enhancing human islet transplantation by localized release of trophic factors from PLG scaffolds. Am. J. Transplant. 14 (7), 1523–1532. doi:10.1111/ajt.12742
Huch, M., and Koo, B.-K. (2015). Modeling mouse and human development using organoid cultures. Development 142 (18), 3113–3125. doi:10.1242/dev.118570
Huh, D., Matthews, B. D., Mammoto, A., Montoya-Zavala, M., Hsin, H. Y., and Ingber, D. E. (2010). Reconstituting organ-level lung functions on a chip. Science 328 (5986), 1662–1668. doi:10.1126/science.1188302
Jacob, A., Vedaie, M., Roberts, D. A., Thomas, D. C., Villacorta-Martin, C., Alysandratos, K.-D., et al. (2019). Derivation of self-renewing lung alveolar epithelial type II cells from human pluripotent stem cells. Nat. Protoc. 14 (12), 3303–3332. doi:10.1038/s41596-019-0220-0
Jones, J., Safi, A., Wei, X., Espinosa, H. D., Budinger, G. S., Takawira, D., et al. (2011). Substrate stiffness regulates extracellular matrix deposition by alveolar epithelial cells. Res. Rep. Biol. 1, 1–12. doi:10.2147/RRB.S13178
Joshi, R., Batie, M. R., Fan, Q., and Varisco, B. M. (2022). Mouse lung organoid responses to reduced, increased, and cyclic stretch. Am. J. Physiology-Lung Cell. Mol. Physiology 322 (1), L162–L173. doi:10.1152/ajplung.00310.2020
Katsura, H., Sontake, V., Tata, A., Kobayashi, Y., Edwards, C. E., Heaton, B. E., et al. (2020). Human lung stem cell-based alveolospheres provide insights into SARS-CoV-2-mediated interferon responses and pneumocyte dysfunction. Cell Stem Cell 27 (6), 890–904.e8. doi:10.1016/j.stem.2020.10.005
Kim, H.-T., Yin, W., Jin, Y.-J., Panza, P., Gunawan, F., Grohmann, B., et al. (2018). Myh10 deficiency leads to defective extracellular matrix remodeling and pulmonary disease. Nat. Commun. 9 (1), 4600. doi:10.1038/s41467-018-06833-7
Kim, H. Y., Pang, M.-F., Varner, V. D., Kojima, L., Miller, E., Radisky, D. C., et al. (2015). Localized smooth muscle differentiation is essential for epithelial bifurcation during branching morphogenesis of the mammalian lung. Dev. Cell 34 (6), 719–726. doi:10.1016/j.devcel.2015.08.012
Kim, H. Y., Varner, V. D., and Nelson, C. M. (2013). Apical constriction initiates new bud formation during monopodial branching of the embryonic chicken lung. Development 140 (15), 3146–3155. doi:10.1242/dev.093682
Kim, J. H., An, G. H., Kim, J. Y., Rasaei, R., Kim, W. J., Jin, X., et al. (2021). Human pluripotent stem cell-derived alveolar organoids for modeling pulmonary fibrosis and drug testing. Cell Death Discov. 7 (1), 48. doi:10.1038/s41420-021-00439-7
Kim, J., Koo, B. K., and Knoblich, J. A. (2020). Human organoids: model systems for human biology and medicine. Nat. Rev. Mol. Cell Biol. 21 (10), 571–584. doi:10.1038/s41580-020-0259-3
Konishi, S., Gotoh, S., Tateishi, K., Yamamoto, Y., Korogi, Y., Nagasaki, T., et al. (2016). Directed induction of functional multi-ciliated cells in proximal airway epithelial spheroids from human pluripotent stem cells. Stem Cell Rep. 6 (1), 18–25. doi:10.1016/j.stemcr.2015.11.010
Kononov, S., Brewer, K., Sakai, H., Cavalcante, F. S. A., Sabayanagam, C. R., Ingenito, E. P., et al. (2001). Roles of mechanical forces and collagen failure in the development of elastase-induced emphysema. Am. J. Respir. Crit. Care Med. 164 (10), 1920–1926. doi:10.1164/ajrccm.164.10.2101083
Kunisaki, S. M., Jiang, G., Biancotti, J. C., Ho, K. K. Y., Dye, B. R., Liu, A. P., et al. (2021). Human induced pluripotent stem cell-derived lung organoids in an ex vivo model of the congenital diaphragmatic hernia fetal lung. Stem Cells Transl. Med. 10 (1), 98–114. doi:10.1002/sctm.20-0199
Leeman, K. T., Pessina, P., Lee, J.-H., and Kim, C. F. (2019). Mesenchymal stem cells increase alveolar differentiation in lung progenitor organoid cultures. Sci. Rep. 9 (1), 6479. doi:10.1038/s41598-019-42819-1
Leiby, K. L., Yuan, Y., Ng, R., Raredon, M. S. B., Adams, T. S., Baevova, P., et al. (2023). Rational engineering of lung alveolar epithelium. Npj Regen. Med. 8 (1), 22. doi:10.1038/s41536-023-00295-2
Li, J., Wang, Z., Chu, Q., Jiang, K., Li, J., and Tang, N. (2018). The strength of mechanical forces determines the differentiation of alveolar epithelial cells. Dev. Cell 44 (3), 297–312.e5. doi:10.1016/j.devcel.2018.01.008
Liberti, D. C., and Morrisey, E. E. (2021). Organoid models: assessing lung cell fate decisions and disease responses. Trends Mol. Med. 27 (12), 1159–1174. doi:10.1016/j.molmed.2021.09.008
Lin, C., Yao, E., Zhang, K., Jiang, X., Croll, S., Thompson-Peer, K., et al. (2017). YAP is essential for mechanical force production and epithelial cell proliferation during lung branching morphogenesis. ELife 6, e21130. doi:10.7554/eLife.21130
Luo, Y., Li, N., Chen, H., Fernandez, G. E., Warburton, D., Moats, R., et al. (2018). Spatial and temporal changes in extracellular elastin and laminin distribution during lung alveolar development. Sci. Rep. 8 (1), 8334. doi:10.1038/s41598-018-26673-1
Mamidi, A., Prawiro, C., Seymour, P. A., de Lichtenberg, K. H., Jackson, A., Serup, P., et al. (2018). Mechanosignalling via integrins directs fate decisions of pancreatic progenitors. Nature 564 (7734), 114–118. doi:10.1038/s41586-018-0762-2
Mao, P., Wu, S., Li, J., Fu, W., He, W., Liu, X., et al. (2015). Human alveolar epithelial type II cells in primary culture. Physiol. Rep. 3 (2), e12288. doi:10.14814/phy2.12288
Melo, E., Garreta, E., Luque, T., Cortiella, J., Nichols, J., Navajas, D., et al. (2014). Effects of the decellularization method on the local stiffness of acellular lungs. Tissue Eng. Part C. Methods 20 (5), 412–422. doi:10.1089/ten.tec.2013.0325
Michielin, F., Giobbe, G. G., Luni, C., Hu, Q., Maroni, I., Orford, M. R., et al. (2020). The microfluidic environment reveals a hidden role of self-organizing extracellular matrix in hepatic commitment and organoid formation of hiPSCs. Cell Rep. 33 (9), 108453. doi:10.1016/j.celrep.2020.108453
Mou, H., Zhao, R., Sherwood, R., Ahfeldt, T., Lapey, A., Wain, J., et al. (2012). Generation of multipotent lung and airway progenitors from mouse ESCs and patient-specific cystic fibrosis iPSCs. Cell Stem Cell 10 (4), 385–397. doi:10.1016/j.stem.2012.01.018
Nelson, C. M., Gleghorn, J. P., Pang, M.-F., Jaslove, J., Goodwin, K., Varner, V. D., et al. (2017). Microfluidic chest cavities reveal that transmural pressure controls the rate of lung development. Development 144, 4328–4335. doi:10.1242/dev.154823
Nguyen, T. M., van der Merwe, J., Elowsson Rendin, L., Larsson-Callerfelt, A.-K., Deprest, J., Westergren-Thorsson, G., et al. (2021). Stretch increases alveolar type 1 cell number in fetal lungs through ROCK-Yap/Taz pathway. Am. J. Physiology-Lung Cell. Mol. Physiology 321 (5), L814–L826. doi:10.1152/ajplung.00484.2020
Nho, R. S., Ballinger, M. N., Rojas, M. M., Ghadiali, S. N., and Horowitz, J. C. (2022). Biomechanical force and cellular stiffness in lung fibrosis. Am. J. Pathology 192 (5), 750–761. doi:10.1016/j.ajpath.2022.02.001
Nikolić, M. Z., Sun, D., and Rawlins, E. L. (2018). Human lung development: recent progress and new challenges. Development 145 (16), dev163485. doi:10.1242/dev.163485
Peak, K. E., Mohr-Allen, S. R., Gleghorn, J. P., and Varner, V. D. (2022). Focal sources of FGF-10 promote the buckling morphogenesis of the embryonic airway epithelium. Biol. Open 11 (9), bio059436. doi:10.1242/bio.059436
Penkala, I. J., Liberti, D. C., Pankin, J., Sivakumar, A., Kremp, M. M., Jayachandran, S., et al. (2021). Age-dependent alveolar epithelial plasticity orchestrates lung homeostasis and regeneration. Cell Stem Cell 28 (10), 1775–1789.e5. doi:10.1016/j.stem.2021.04.026
Plantier, L., Crestani, B., Wert, S. E., Dehoux, M., Zweytick, B., Guenther, A., et al. (2011). Ectopic respiratory epithelial cell differentiation in bronchiolised distal airspaces in idiopathic pulmonary fibrosis. Thorax 66 (8), 651–657. doi:10.1136/thx.2010.151555
Rabata, A., Fedr, R., Soucek, K., Hampl, A., and Koledova, Z. (2020). 3D cell culture models demonstrate a role for FGF and WNT signaling in regulation of lung epithelial cell fate and morphogenesis. Front. Cell Dev. Biol. 8, 574. doi:10.3389/fcell.2020.00574
Riccetti, M. R., Ushakumary, M. G., Waltamath, M., Green, J., Snowball, J., Dautel, S. E., et al. (2022). Maladaptive functional changes in alveolar fibroblasts due to perinatal hyperoxia impair epithelial differentiation. JCI Insight 7 (5), e152404. doi:10.1172/jci.insight.152404
Sachs, N., Papaspyropoulos, A., Zomer-van Ommen, D. D., Heo, I., Böttinger, L., Klay, D., et al. (2019). Long-term expanding human airway organoids for disease modeling. EMBO J. 38 (4), e100300. doi:10.15252/embj.2018100300
Sanchez-Esteban, J., Cicchiello, L. A., Wang, Y., Tsai, S.-W., Williams, L. K., Torday, J. S., et al. (2001). Mechanical stretch promotes alveolar epithelial type II cell differentiation. J. Appl. Physiology 91 (2), 589–595. doi:10.1152/jappl.2001.91.2.589
Schruf, E., Schroeder, V., Le, H. Q., Schönberger, T., Raedel, D., Stewart, E. L., et al. (2020). Recapitulating idiopathic pulmonary fibrosis related alveolar epithelial dysfunction in a human iPSC-derived air-liquid interface model. FASEB J. 34 (6), 7825–7846. doi:10.1096/fj.201902926R
Schutgens, F., and Clevers, H. (2020). Human organoids: tools for understanding biology and treating diseases. Annu. Rev. Pathology Mech. Dis. 15 (1), 211–234. doi:10.1146/annurev-pathmechdis-012419-032611
Selman, M., and Pardo, A. (2002). Idiopathic pulmonary fibrosis: an epithelial/fibroblastic cross-talk disorder. Respir. Res. 3 (1), 3. doi:10.1186/rr175
Selman, M. (2006). Role of epithelial cells in idiopathic pulmonary fibrosis: from innocent targets to serial killers. Proc. Am. Thorac. Soc. 3 (4), 364–372. doi:10.1513/pats.200601-003TK
Shiraishi, K., Shah, P. P., Morley, M. P., Loebel, C., Santini, G. T., Katzen, J., et al. (2023). Biophysical forces mediated by respiration maintain lung alveolar epithelial cell fate. Cell 186 (7), 1478–1492.e15. doi:10.1016/j.cell.2023.02.010
Solis, A. G., Bielecki, P., Steach, H. R., Sharma, L., Harman, C. C. D., Yun, S., et al. (2019). Mechanosensation of cyclical force by PIEZO1 is essential for innate immunity. Nature 573 (7772), 69–74. doi:10.1038/s41586-019-1485-8
Strikoudis, A., Cieślak, A., Loffredo, L., Chen, Y.-W., Patel, N., Saqi, A., et al. (2019). Modeling of fibrotic lung disease using 3D organoids derived from human pluripotent stem cells. Cell Rep. 27 (12), 3709–3723.e5. doi:10.1016/j.celrep.2019.05.077
Stucki, J. D., Hobi, N., Galimov, A., Stucki, A. O., Schneider-Daum, N., Lehr, C.-M., et al. (2018). Medium throughput breathing human primary cell alveolus-on-chip model. Sci. Rep. 8 (1), 14359. doi:10.1038/s41598-018-32523-x
Tan, Q., Choi, K. M., Sicard, D., and Tschumperlin, D. J. (2017). Human airway organoid engineering as a step toward lung regeneration and disease modeling. Biomaterials 113, 118–132. doi:10.1016/j.biomaterials.2016.10.046
Tang, X. Y., Wu, S., Wang, D., Chu, C., Hong, Y., Tao, M., et al. (2022). Human organoids in basic research and clinical applications. Signal Transduct. Target. Ther. 7 (1), 168. doi:10.1038/s41392-022-01024-9
Tang, Z., Hu, Y., Wang, Z., Jiang, K., Zhan, C., Marshall, W. F., et al. (2018). Mechanical forces program the orientation of cell division during airway tube morphogenesis. Dev. Cell 44 (3), 313–325.e5. doi:10.1016/j.devcel.2017.12.013
Tommasini, F., Benoist, T., Shibuya, S., Woodall, M. N. J., Naldi, E., Palor, M., et al. (2023). Lung viral infection modelling in a bioengineered whole-organ. Biomaterials 301, 122203. doi:10.1016/j.biomaterials.2023.122203
Trivedi, V., Choi, H. M. T., Fraser, S. E., and Pierce, N. A. (2018). Multidimensional quantitative analysis of mRNA expression within intact vertebrate embryos. Development 145 (1), dev156869. doi:10.1242/dev.156869
Unbekandt, M., del Moral, P.-M., Sala, F. G., Bellusci, S., Warburton, D., and Fleury, V. (2008). Tracheal occlusion increases the rate of epithelial branching of embryonic mouse lung via the FGF10-FGFR2b-Sprouty2 pathway. Mech. Dev. 125 (3–4), 314–324. doi:10.1016/j.mod.2007.10.013
Urciuolo, A., Giobbe, G. G., Dong, Y., Michielin, F., Brandolino, L., Magnussen, M., et al. (2023). Hydrogel-in-hydrogel live bioprinting for guidance and control of organoids and organotypic cultures. Nat. Commun. 14 (1), 3128. doi:10.1038/s41467-023-37953-4
van Riet, S., van Schadewijk, A., Khedoe, P. P. S. J., Limpens, R. W. A. L., Bárcena, M., Stolk, J., et al. (2022). Organoid-based expansion of patient-derived primary alveolar type 2 cells for establishment of alveolus epithelial Lung-Chip cultures. Am. J. Physiology-Lung Cell. Mol. Physiology 322 (4), L526–L538. doi:10.1152/ajplung.00153.2021
Varner, V. D., Gleghorn, J. P., Miller, E., Radisky, D. C., and Nelson, C. M. (2015). Mechanically patterning the embryonic airway epithelium. Proc. Natl. Acad. Sci. 112 (30), 9230–9235. doi:10.1073/pnas.1504102112
Walma, D. A. C., and Yamada, K. M. (2020). The extracellular matrix in development. Development 147 (10), dev175596. doi:10.1242/dev.175596
Watson, C. L., Mahe, M. M., Múnera, J., Howell, J. C., Sundaram, N., Poling, H. M., et al. (2014). An in vivo model of human small intestine using pluripotent stem cells. Nat. Med. 20 (11), 1310–1314. doi:10.1038/nm.3737
Wilkinson, D. C., Alva-Ornelas, J. A., Sucre, J. M. S., Vijayaraj, P., Durra, A., Richardson, W., et al. (2017). Development of a three-dimensional bioengineering technology to generate lung tissue for personalized disease modeling. Stem Cells Transl. Med. 6 (2), 622–633. doi:10.5966/sctm.2016-0192
Wong, A. P., Bear, C. E., Chin, S., Pasceri, P., Thompson, T. O., Huan, L.-J., et al. (2012). Directed differentiation of human pluripotent stem cells into mature airway epithelia expressing functional CFTR protein. Nat. Biotechnol. 30 (9), 876–882. doi:10.1038/nbt.2328
Wu, H., Yu, Y., Huang, H., Hu, Y., Fu, S., Wang, Z., et al. (2020). Progressive pulmonary fibrosis is caused by elevated mechanical tension on alveolar stem cells. Cell 180 (1), 107–121.e17. doi:10.1016/j.cell.2019.11.027
Xinaris, C., Benedetti, V., Rizzo, P., Abbate, M., Corna, D., Azzollini, N., et al. (2012). In vivo maturation of functional renal organoids formed from embryonic cell suspensions. J. Am. Soc. Nephrol. 23 (11), 1857–1868. doi:10.1681/ASN.2012050505
Zepp, J. A., Morley, M. P., Loebel, C., Kremp, M. M., Chaudhry, F. N., Basil, M. C., et al. (2021). Genomic, epigenomic, and biophysical cues controlling the emergence of the lung alveolus. Science 371 (6534), eabc3172. doi:10.1126/science.abc3172
Zhang, K., Yao, E., Chuang, E., Chen, B., Chuang, E. Y., Volk, R. F., et al. (2022). Wnt5a–Vangl1/2 signaling regulates the position and direction of lung branching through the cytoskeleton and focal adhesions. PLOS Biol. 20 (8), e3001759. doi:10.1371/journal.pbio.3001759
Keywords: lung organoids, mechanobiology, biophysical cues, lung distal diseases, disease modelling
Citation: Shao Z, De Coppi P and Michielin F (2023) Leveraging mechanobiology and biophysical cues in lung organoids for studying lung development and disease. Front. Chem. Eng. 5:1255783. doi: 10.3389/fceng.2023.1255783
Received: 09 July 2023; Accepted: 09 October 2023;
Published: 24 October 2023.
Edited by:
Yan Li, Florida State University, United StatesReviewed by:
Pedro M Baptista, Health Research Institute of Aragon (IIS Aragon), SpainMariaceleste Aragona, University of Copenhagen, Denmark
Copyright © 2023 Shao, De Coppi and Michielin. This is an open-access article distributed under the terms of the Creative Commons Attribution License (CC BY). The use, distribution or reproduction in other forums is permitted, provided the original author(s) and the copyright owner(s) are credited and that the original publication in this journal is cited, in accordance with accepted academic practice. No use, distribution or reproduction is permitted which does not comply with these terms.
*Correspondence: Federica Michielin, Zi5taWNoaWVsaW5AdWNsLmFjLnVr