- 1Department of Laboratory Medicine, Huashan Hospital, Shanghai Medical College, Fudan University, Shanghai, China
- 2State Key Laboratory for Microbial Metabolism and School of Life Sciences and Biotechnology, Shanghai Jiaotong University, Shanghai, China
Carbapenemase-producing Klebsiella pneumoniae (KPC) has emerged and spread throughout the world. A retrospective analysis was performed on carbapenem-resistant K. pneumoniae isolated at our teaching hospital during the period 2009–2010, when the initial outbreak occurred. To determine the mechanism(s) that underlies the increased infectivity exhibited by KPC, Multilocus Sequence Typing (MLST) was conducted. A series of plasmids was also extracted, sequenced and analyzed. Concurrently, the complete sequences of blaKPC−2-harboring plasmids deposited in GenBank were summarized and aligned. The blaKPC−2 and KlcAHS genes in the carbapenem-resistant K. pneumoniae isolates were examined. E. coli strains, carrying different Type I Restriction and Modification (RM) systems, were selected to study the interaction between RM systems, anti-RM systems and horizontal gene transfer (HGT). The ST11 clone predominated among 102 carbapenem-resistant K. pneumoniae isolates, all harbored the blaKPC−2 gene; 98% contained the KlcAHS gene. KlcAHS was one of the core genes in the backbone region of most blaKPC−2 carrying plasmids. Type I RM systems in the host bacteria reduced the rate of pHS10842 plasmid transformation by 30- to 40-fold. Presence of the anti-restriction protein, KlcAHS, on the other hand, increased transformation efficiency by 3- to 6-fold. These results indicate that RM systems can significantly restrict HGT. In contrast, KlcAHS can disrupt the RM systems and promote HGT by transformation. These findings suggest that the anti-restriction protein, KlcAHS, represents a novel mechanism that facilitates the increased transfer of blaKPC-2 and KlcAHS-carrying plasmids among K. pneumoniae strains.
Introduction
Since carbapenemase-producing Klebsiella pneumoniae (KPC) was first reported in the USA in 2001, it has emerged worldwide as a multidrug-resistant hospital pathogen and a significant problem in treating infectious diseases (Yigit et al., 2001). KPC infections often reach mortality rates between 23 and 75% due, in part, to the lack of effective antimicrobial agents (Koraimann and Wagner, 2014). Carbapenem antibiotics are ineffective in treating more than half the patients infected with K. pneumoniae due to drug resistance (Pitout et al., 2015). This is particularly problematic because carbapenems are the antibiotics of last resort in treating multidrug-resistant gram-negative bacteria. Carbapenemases in clinical, K. pneumoniae isolates are encoded mainly by the blaKPC gene, which is carried by many different plasmids (Naas et al., 2008; Nordmann et al., 2011). The horizontal transfer of resistance gene-containing plasmids is a crucial mechanism for disseminating antimicrobial resistance (Carattoli, 2013). The exchange of genetic material by mobile elements, e.g., plasmids and phages, between closely and distantly related bacterial species is influenced by three horizontal gene transfer (HGT) mechanisms: conjugation, transformation, and transduction (Brown-Jaque et al., 2015). Due to these mechanisms, bacteria can achieve significant genetic diversity by acquiring DNA from distantly-related species. This plays a key role in evolution (e.g., acquiring antibiotic resistance), contributing to the fitness and diversity of prokaryotes (Wiedenbeck and Cohan, 2011; San Millan et al., 2015).
Restriction and Modification (RM) systems, which are widespread among prokaryotes (>50% possess genes that encode at least one RM system type), are major barriers to HGT (Veiga and Pinho, 2009; Serfiotis-Mitsa et al., 2010; Vasu et al., 2012; Roberts et al., 2015). Four RM systems (I–IV) are classified dependent upon the complexity of their structure and function (Tock and Dryden, 2005). Type I RM systems, e.g., EcoKI, are composed of the products of three genes: hsdR (R, restriction), hsdM (M, methylation), and hsdS (S, sequence specificity). Type I RM systems can protect host bacteria from foreign DNA by recognizing specific DNA sequences and incising them with restriction endonuclease activity. Notably, Type I RM systems exhibit two seemingly inconsistent enzymatic activities: restriction endonucleases (REase) and methyltransferase (MTase) (Tock and Dryden, 2005). REase recognizes, cleaves and degrades invading foreign DNA, which usually lacks specific modification (e.g., methylation). Type I RM-associated MTase, on the other hand, can convert hemimethylated DNA to the completely methylated form, which resists REase activity. The evidence to date suggests that RM systems are an imperfect barrier to foreign DNA. Plasmids employ a number of strategies to avoid restriction (Wilkins, 2002; Tock and Dryden, 2005).
HGT is directly responsible for the spread of antibiotic resistance genes among pathogenic bacteria derived from both clinical and environment backgrounds (Thomas and Nielsen, 2005; McMahon et al., 2009). An exploration of the mechanism by which mobile genetic elements circumvent barriers such as RM systems during HGT is urgently needed. Multiple anti-RM genes within mobile elements is one likely explanation (Belogurov et al., 2000; McMahon et al., 2009; Serfiotis-Mitsa et al., 2010; Balabanov et al., 2012; Chen et al., 2014). Anti-RM gene products disrupt or negate the RM defense systems and, consequently, promote HGT (Webb et al., 1996; McMahon et al., 2009).
Here, we report that blaKPC−2 and KlcAHS genes coexisted in essentially all carbapenem-resistant K. pneumoniae isolated at Huashan Hospital. KlcAHS exhibits a high degree of homology with reported anti-restriction proteins KlcA136 (43% identity and 66% similarity) and ArdB (30% identity and 58% similarity); both these proteins possess anti-restriction activity and promote transduction (Kamachi et al., 2006). The present study was the first to demonstrate the ability of KlcAHS to facilitate transformation by disrupting RM systems. These findings suggest that KlcAHS is a novel contributor to the spread of carbapenem-resistant K. pneumoniae.
Methods and Materials
Rate of Carbapenem Resistance
The carbapenem resistance data for K. pneumoniae isolated between 2006 and the third quarter of 2016 were obtained using WHONET software, a computerized microbiology laboratory data management and analysis program that, among other things, informs drug-policy decisions and preventive measures (Agarwal et al., 2009). Antimicrobial susceptibility testing was performed using the K-B agar diffusion method. Briefly; all specimens were inoculated onto MHA agar plates and incubated at 35°C for 16–18 h. Ten micrograms imipenem and meropenem disks were used to detect carbapenem-resistant K. pneumoniae. Isolates were included in this study if the diameter of the inhibition zone was ≤19 mm. If more than one isolate of the same genus was obtained from a single patient, only one isolate was used. The results were interpreted according to the American Clinical and Laboratory Standards Institute standard (CLSI, 2012).
Retrospective Analyses of MLSTs
One hundred and two carbapenem-resistant K. pneumoniae isolates derived from 74 sputum, 20 urine, and 8 blood samples were collected by the microbiology section, Huashan Hospital, during the period 2009–2010, when the initial outbreak at our hospital occurred. In addition to meeting the criteria listed in the preceding paragraph, each isolate was determined to be carbapenem-resistant at imipenem or meropenem concentrations ≥4 μg/mL based upon the 2012 Clinical and Laboratory Standard Institutes standards [imipenem (S = ≤1; I = 2; R = ≥4 μg/mL), and/or meropenem (S = ≤1; I = 2; R = ≥4 μg/mL)]. MLST was performed on all isolates according to the literature using seven conserved housekeeping genes (gapA, infB, mdh, pgi, phoE, rpoB, and tonB; Diancourt et al., 2005).
Plasmid Extraction, Sequencing, and Analysis
Five plasmids (pHS062105-3, pHS082416, pHS092753, pHS092839, and pHS10505) were extracted from carbapenem-resistant K. pneumoniae isolated at our hospital. pHS062105-3, the first plasmid extracted, was completely sequenced; the others were sequenced partially according to the literature (Shen et al., 2016). A map of pHS062105-3 was generated using the web server (Grant and Stothard, 2008).
Analysis of Complete Plasmid Gene Sequences Containing blaKPC−2 Deposited in GenBank
Complete plasmid sequences were searched in GenBank using the restrictive words “blaKPC−2 and plasmid complete sequence;” repeated records were deleted. All plasmid sequences harboring the blaKPC−2 and KlcAHS genes were aligned with the reference sequence, pHS10842, using Blast Ring Image Generator software (Alikhan et al., 2011).
Detection of blaKPC−2 and KlcAHS Genes
The blaKPC−2 and KlcAHS genes were detected among the carbapenem-resistant K. pneumoniae isolates by PCR and primers (P1/P2 and P3/P4, Table 1) designed using Primer Premier 6.22 software (Premier Biosoft, Palo Alto, CA).
Strains, Plasmids, and Antibiotics
E. coli strains, selected to assess RM and anti-RM activities in vivo (Table 2), were the gift of Dr. D.T. Dryden (University of Edinburgh, UK). Ampicillin (AMP) and kanamycin (Kan) were purchased from Sigma-Aldrich Corp. (St. Louis, MO, USA), and added to the culture media where indicated.
Preparation of Competent Cells
A set of E. coli strains that carry different Type I RM systems was made competent by a modification of the protocol described by Chan et al. (2013). Briefly, bacteria in LB broth were grown to OD600 ≈ 0.5, collected by centrifugation, washed and resuspended in ice-cold 100 mM CaCl2 in 10% (v/v) glycerol. The OD600 of each strain was adjusted to the same value, dispersed into aliquots and stored at −70°C until use.
Correlate the RM System and Transformation Efficiency
A series of E. coli strains with different RM systems listed in Table 2 were transformed with equivalent moles of pHS10842 plasmid. The transformants of each strain were counted.
Correlate EcoKI Enzyme Recognition Sites and Transformation Efficiency
The two EcoKI recognition sites in pHS10842 were knocked out using a modification of the protocol described by Zhang et al. (2014). The P5/P6 and P7/P8 primer pairs were designed based upon the adjacent sequences outside the two extremes of each EcoKI recognition site using Primer Premier 6.22 software (Table 1). The primers were used to amplify the entire pHS10842 sequence except for each EcoKI recognition site. The target DNA fragment was amplified using a high-fidelity PCR Kit (Takara, Dalian, China). The PCR-product was electrophoresed, the target band was recovered and the DNA was purified using a Gel Extraction kit (E.Z.N.A.®Gel Extraction kit, Omega Biotek, Norcross, GA, USA). The linear DNA was cyclized into mutant pHS10842 with the HieffClone One Step Cloning Kit according to the manufacturer's instruction (Yeasen, Shanghai, China).
Competent, RM-deficient cells were transformed with the cloning products generated in the preceding paragraph. EcoKI recognition site-deleted pHS10842 clones were selected by colony-PCR with the P9/P10 and P11/P12 primer pairs (Table 1), inoculated into LB broth containing 100 μg/ml AMP, and incubated overnight. Each site deleted pHS10842 was obtained, confirmed using DNA sequencing technology with P13/P14 or P15/P16 primer pairs, and named Δsite1 and Δsite2 pHS10842, respectively. Δsite1 plus Δsite2 pHS10842 was obtained using Δsite2 pHS10842 as the DNA template and the same methodology. The same number of E. coli competent cells (NM1049, Type IA RM system [EcoKI]-positive) was transformed with equal moles of Δsite1, Δsite2, and Δsite1 plus Δsite2 pHS10842 under identical experiment conditions, and the transformants were quantified.
Construct Recombinant pET24a-KlcAHS
The KlcAHS gene was PCR-amplified with primers P17/P18 (Table 1) using pHS092839 extracted from a clinical, carbapenem-resistant K. pneumoniae isolate as the DNA template. The primers P17/P18 were predesigned with NdeI and EcoRI recognition sites. The PCR-products were combined with pET24a vector, digested with NdeI and EcoRI (New England Biolabs, Inc., Beijing, China), purified and ligated with T4 DNA ligase (New England Biolabs); competent E. coli DH5α cells were subsequently transformed. Recombinant pET24a-KlcAHS-expressing clones were identified using colony PCR and confirmed by DNA sequencing both strands with primers P19/P20 (Table 1) to assure no mistakes were introduced during amplification.
Compare the Transformation Efficiencies of pET24a and Recombinant pET24a-KlcAHS
To evaluate the anti-restriction activity of KlcAHS, competent, RM system-defective cells were transformed with unmethylated wild-type pET24a or recombinant pET24a-KlcAHS plasmids, and the transformation efficiencies were compared. The two unmethylated plasmids were extracted with Plasmid Mini Kit I (E.Z.N.A.® Plasmid Mini Kit I), each was quantified using NANODROP (NanoDrop2000, Thermo Fisher Scientific, USA), and the molecular weights were calculated with Snap gene viewer software (GSL Biotech, available at snapgene.com). Equal moles of pET24a and recombinant pET24a-KlcAHS were added to competent cells, mixed and placed on ice before heat shocking at 42°C for 90 s. Afterwards, the cells were placed on ice, then added to LB broth and allowed to recover at 37°C with shaking. Aliquots were transferred to LB agar plates containing 25 μg/ml Kan and incubated overnight. The transformant colonies were counted the next day. Transformation efficiency ratios were calculated by dividing the pET24a-KlcAHS transformants by the pET24a transformants.
The anti-modification function of KlcAHS was estimated by comparing the transformation efficiencies of wild pET24a and recombinant pET24a-KlcAHS extracted from the transformants obtained in the step above. Each transformant was transferred to fresh LB broth and cultured overnight; both plasmids were extracted from strains carrying different Type I RM systems using E.Z.N.A.® Plasmid Mini Kit I. Competent cells were transformed with the newly extracted plasmids, pET24a-KlcAHS and pET24a. Subsequently, the transformants of both pET24a-KlcAHS and pET24a were counted. Transformation efficiencies ratios were calculated by dividing the pET24a-KlcAHS transformants with the pET24a transformants. Both the anti-restriction and anti-modification activities of KlcAHS were considered significant in vivo at ratios >2 (McMahon et al., 2009).
Statistical Analysis
The results were analyzed using the SPSS 13.0 software program compatible with Windows. Data derived from more than two groups were compared by one-way analysis of variance (ANOVA) followed by a Dunnett's or Tukey's test to identify the groups that differed significantly (P < 0.05).
Results
Carbapenem-Resistance Rates of K. pneumoniae Isolated between 2006 and 2016
The first carbapenem-resistant K. pneumoniae isolate was identified at Huashan Hospital in 2006. The years 2007 and 2008 represented a temporary transition period. The outbreak of carbapenem-resistant K. pneumoniae occurred in 2009; the rate has increased yearly thereafter suggesting the trend will continue (Figure 1).
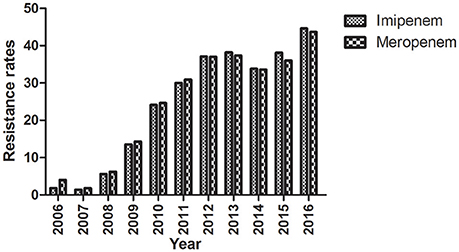
Figure 1. Imipenem- and meropenem-resistance rates from 2006 to 2016. Clinical K. pneumoniae isolates were obtained during the period 2006–2016 and the resistance to imipenem and meropenem was determined.
MLSTs of Carbapenem-Resistant K. pneumoniae Isolates
The MLSTs of 102 clinical, carbapenems-resistant K. pneumoniae isolates obtained between 2009 and 2010 were determined in an effort to define their relationship (Diancourt et al., 2005). All isolates belonged to a predominant epidemic clone, ST11, which exhibits a distinctive allelic profile based upon seven housekeeping genes that differentiates these isolates from those that belong to other epidemiologic clusters.
Analyses of the Plasmid Sequences Derived from the Carbapenem-Resistant K. pneumoniae Isolates
Experiments were undertaken to explore the connection between KlcAHS and blaKPC−2. The complete plasmid sequence of pHS062105-3 and the partial sequences of pHS082416, pHS092753, pHS092839, and pHS10505 were obtained by sequencing technology. These sequences were deposited in the GenBank under accession number NC_023331.1, KF724507.1, KF826293.1, KF724506.1, and KF826292.1, respectively. The results showed that KlcAHS and blaKPC−2 genes coexisted in the plasmids analyzed (Figure 2).
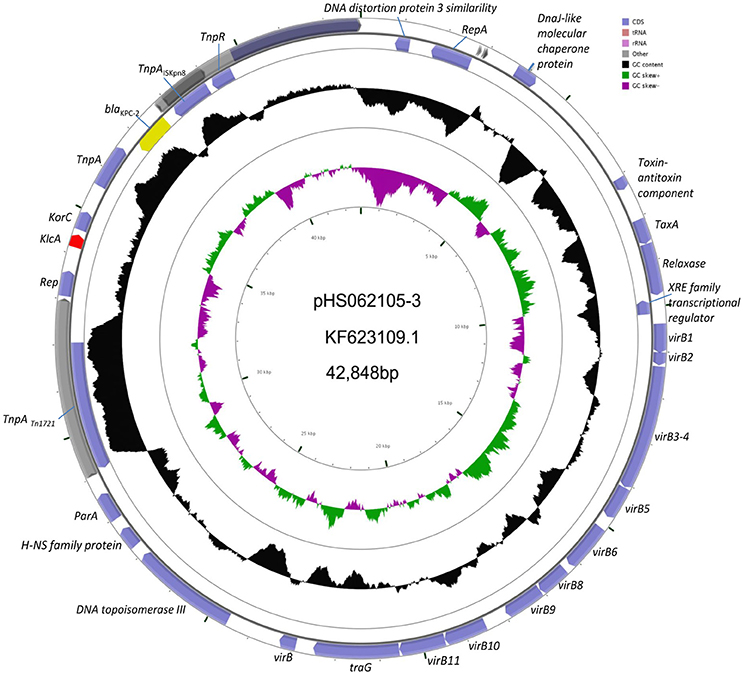
Figure 2. Schematic map of pHS062105-3. The KlcAHS and blaKPC−2 genes are demarcated by red and yellow, respectively, in the schematic map of pHS062105-3 shown.
Analysis of the Genetic Structure of blaKPC−2-Harboring Plasmids
Thirty-one complete plasmid sequences were analyzed and summarized (Table 3), showing that the common genetic environment near the blaKPC−2 gene was categorized arbitrarily into three groups. The first group had a Tn1721-like genetic background, which is often encountered in China. Within this group, 15 plasmid sequences harbored a backbone region in which the KlcAHS and blaKPC−2 genes coexisted (Figure 3). The second group had a Tn4401-like genetic structure. The third group was comprised of plasmids that had no obvious common feature.
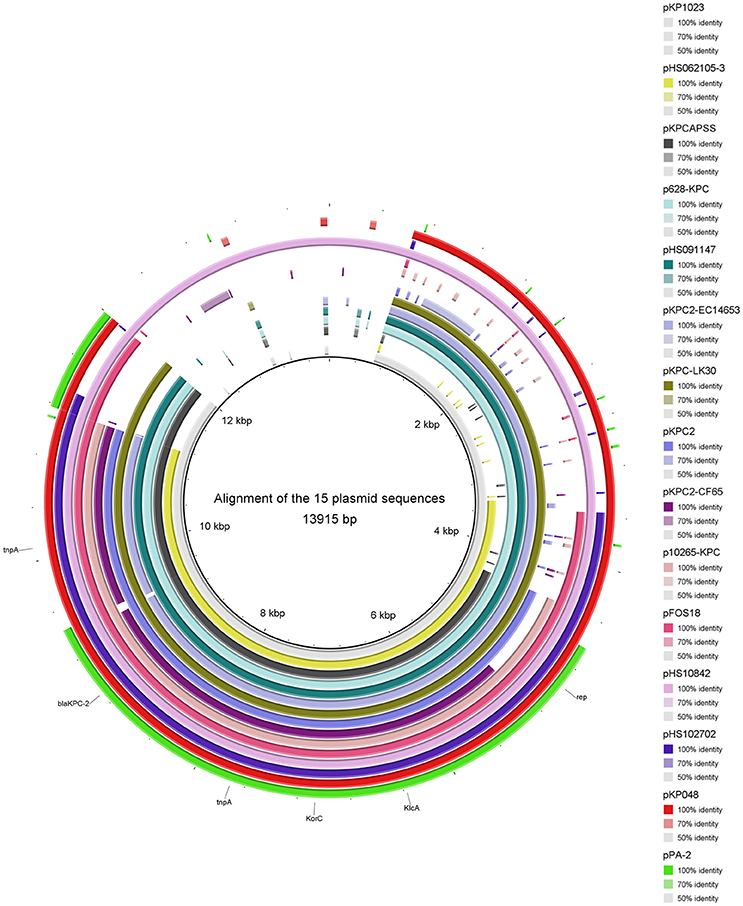
Figure 3. Alignment of 15 plasmid sequences that harbor KlcAHS. The backbone region of plasmids that harbor blaKPC−2 was confirmed. The KlcAHS gene combined with other genes constitutes the backbone region of many plasmids submitted mainly from China.
Detection of the blaKPC−2 and KlcAHS Genes in Carbapenem-Resistant K. pneumoniae
The results of PCR-based screening demonstrated the presence of the blaKPC−2 gene in all 102 clinical, carbapenem-resistant K. pneumoniae isolates. The KlcAHS gene was found in 98% (100/102) of those isolates. These findings document the common coexistence of blaKPC−2 and KlcAHS gene in carbapenem-resistant K. pneumoniae isolates, at least those recovered at Huashan hospital.
RM Systems of the Host Restrict Transformation
Bacterial hosts possess RM systems, which cleave internalized plasmids that possess restriction enzyme sites recognized by RM system-associated REases. As a consequence, the rate of transformation is reduced. Bacterial strains that carry different RM systems exhibited varied rates of transformation by an equivalent number of pHS10842. E. coli strains that possess EcoKI (Type IA, 5′-AACNNNNNNGTGC-3′) and EcoR124I (Type IC, 5′-GAANNNNNNRTCG-3′) REases can recognize and degrade internalized pHS10842. On the other hand, internalized pHS10842 lacks sequences recognized by EcoAI (Type IB, 5′-GAGNNNNNNNGTCA -3′) and StySBLI (Type ID, 5′-GGTANNNNNNTCG-3′) REases and, therefore, remains intact. The transformation of E. coli strains that possessed Type IA or IC system enzymes (i.e., EcoKI and EcoR124I, respectively) were reduced significantly relative to transformation of strains that possessed IB, ID, and null RM systems (Figure 4).
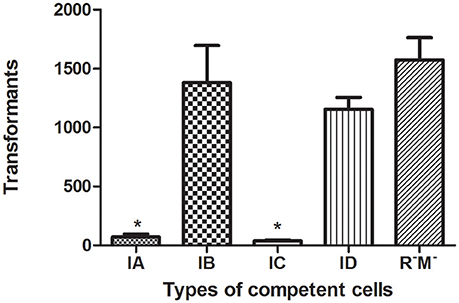
Figure 4. Transformation of E. coli strains that possess different RM systems. The X axis indicates the absence (R−M−) and presence of a Type I RM system, represented by restriction enzymes EcoKI (IA), EcoIA (IB), EcoR124I (IC), and StySBLI (ID), expressed by an E. coli strain. The Y axis denotes the number of unmethylated pHS10842 transformants. Data are the means ± SD derived from three similar experiments. *Significantly less than R−M−; P < 0.05.
Restriction Enzyme Recognition Sites Exert a Critical Effect on Transformation
The presence of restriction enzyme recognition sites in plasmids exert a negative impact on transformation. Compared to wild-type pHS10842, the number of transformants was increased when either of the two EcoKI recognition sites was deleted. When both EcoKI recognition sites were disrupted, the rate of transformation increased dramatically (Figure 5).
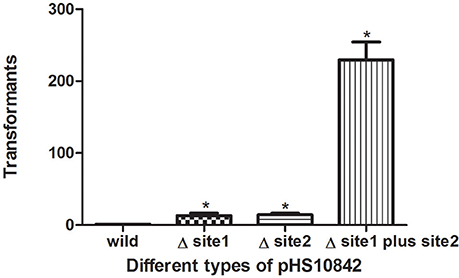
Figure 5. Restriction enzyme recognition site and transformation efficacy. E. coli strain (NM1049, EcoKI+) transformed with pHS10842 that lacks one or both restriction enzyme recognition sites. The number of transformants is indicated. Data are the means ± SD derived from three similar experiments. *Significantly greater than wild-type; P < 0.05.
KlcAHS Expression Promotes Transformation Efficiency
Experiments were initiated to determine the ability of KlcAHS to counteract the negative effect(s) of the host RM system on transformation. KlcAHS promoted the transformation efficiency of pET24a by 3- to 6-fold in E. coli strains that carry IA-D RM systems. The anti-restriction activity varied when subjected to different Type I RM system enzymes (Figure 6). In contrast, the anti-modification activity expressed by KlcAHS was not affected significantly; the efficiency of transformation varied ≤2-fold (data not shown).
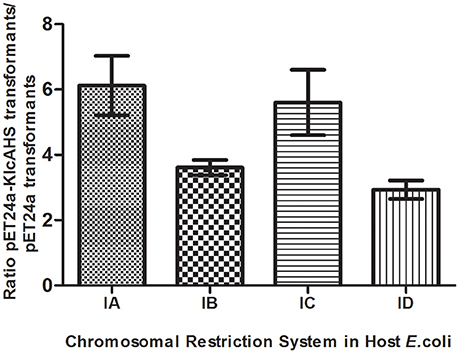
Figure 6. Anti-restriction activity of KlcAHS. Four Type I RM systems, represented by restriction enzymes EcoKI (IA), EcoIA (IB), EcoR124I (IC), and StySBLI (ID), are indicated on the X-axis. All plasmids used were extracted from an R−M− strain. The assay was repeated in triplicate. Data are the means ± SD.
Discussion
The rate of carbapenem resistance among K. pneumoniae isolated at Huashan Hospital rose steadily during the past decade. Currently, the rate of imipenem resistance is 44.6% compared to 1.8% in 2006. The initial outbreak of carbapenem–resistant K. pneumoniae occurred between 2009 and 2010. Carbapenem resistance is increasing worldwide (Nordmann et al., 2011; Hu et al., 2016; Tian et al., 2016). The increase in carbapenem-resistant K. pneumoniae resulted in the availability of fewer antibiotics, and a huge economic and social burdens. Little is known about the exact mechanism of carbapenemase dissemination. In this study, we found that Type I RM systems and the anti-restriction protein, KlcAHS exerted major effects on HGT. Disparate rates of transformation occurred when E. coli strains with different RM systems were transformed with equivalent concentrations of pHS10842. No specific sequences in pHS10842 are recognized by EcoAI (Type IB) and StySBLI (Type ID) REases, but E. coli strains that possess EcoKI (Type IA) and EcoR124I (Type IC) REases can recognize and degrade pHS10842. Consequently, the transformation rate of E. coli strains that possess restriction enzymes EcoKI and EcoR124I was 30- to 40-fold less than strains that possess EcoAI, StySBLI, or no RM system, indicating the significant ability of RM systems to restrict HGT. The rate of transformation increased ~100-fold when both enzyme recognition sites in pHS10842 were deleted and E. coli strains that possessed a Type IA RM system (i.e., EcoKI) were transformed with the resultant plasmid. When either of the two recognition sites was deleted, the transformation rate increased ~10-fold; no significant difference existed between the two sites. Clearly, the plasmid sequence and the RM system carried by the host were crucial factors affecting HGT. The distribution of RM systems among K. pneumoniae isolates is sporadic and not well-studied; most systems were predicted using bioinformatic tools (Roberts et al., 2015). As such, model E. coli strains were selected to examine KlcAHS. activity.
Anti-restriction systems are important counterstrategies employed by mobile genetic elements to evade the RM systems in recipient host bacteria. RM systems have been studied extensively as primitive defense mechanisms (Tock and Dryden, 2005; Vasu et al., 2012; Roberts et al., 2015; Roer et al., 2015; Oliveira et al., 2016). RM systems comprise a major, imperfect barrier to HGT; anti-restriction proteins, such as ArdA, ArdB, ArdC, ArdD, and KlcA136, possess anti-restriction activity in transduction (Belogurov et al., 2000; McMahon et al., 2009; Serfiotis-Mitsa et al., 2010; Balabanov et al., 2012). Those anti-restriction proteins augmented the plating efficiency of lambda phage more than 10-fold (Serfiotis-Mitsa et al., 2010). Our understanding of anti-RM systems is inadequate, however, especially regarding their role in disseminating drug-resistance genes among clinical bacterial isolates. Here, anti-RM gene KlcAHS and blaKPC−2 coexisted in the backbone structure in many complete plasmid sequences submitted mainly by Chinese institutes. Furthermore, all the carbapenem-resistant K. pneumoniae isolates collected at our hospital at the beginning of the outbreak harbored the blaKPC−2 gene; 98% of them contained the KlcAHS gene. The biological significance of this finding remains obscure, however; whether it contributes to HGT is presently unclear. Transformation was selected as a means of HGT to explore the role KlcAHS.
Transformation involves the uptake and functional establishment of DNA by recipient bacteria. Many pathogenic bacteria, e.g., Staphylococcus and Streptococcus, are naturally transformable (Lorenz and Wackernagel, 1994). Indeed, ~1% of bacterial species are naturally transformable, facilitating their ability to obtain beneficial characteristics from distantly-related species (Jonas et al., 2001). The low-level expression of KlcAHS by unmethylated wild pET24a, which normally occurs in the absence of induction (Nie et al., 2013), increased the rates of transformation in the present study by 6.1-, 3.6-, 5.6-, and 2.9-fold when the recombinant plasmid was transformed into a series of E. coli strains that harbored IA, IB, IC, and ID RM systems, respectively. A single target sequence in pET24a is recognized by the Type I RM enzyme, EcoKI (IA), or EcoR124I (IC); however, no target sequence is recognized by restriction enzyme EcoAI (IB) or StySBLI (ID). Consequently, the enhanced rate of transformation as a function of KlcAHS expression was greater for the Type IA and IC strains than for the Type IB and ID strains. Intriguingly, KlcAHS expression also increased the rate of transformation in E. coli strains that possessed Type IB and ID RM systems. Presumably, in the latter cases, KlcAHS increased the mobility of plasmids by unknown strategies in addition to disrupting the RM system. Furthermore, when both wild pET24a and recombinant pET24a-KlcAHS were extracted from the transformants of each E. coli strain, then transformed back into the same competent strain, the transformation rates of the two plasmids never varied more than 2-fold. It is conceivable that the extracted plasmids were modified by RM enzymes, rendering them immune to restriction. It seems reasonable to conjecture that KlcAHS possesses anti-restriction, but no anti-modification, function which is consistent with the findings of other investigators (Serfiotis-Mitsa et al., 2010). It is probable, therefore, that KlcAHS anti-restriction protein facilitates HGT in the natural microbial community by transformation, especially among certain bacterial species.
Transformation is a means of HGT that facilitate the movement of foreign DNA into new bacterial strains. The balance between the RM systems in the recipient and the anti-restriction systems in the mobile genetic elements constitute one of most important factors affecting the rate of HGT. The mechanism(s) by which KlcAHS affects the RM systems is elusive. The effect of KlcAHS on other HGT mechanisms, i.e., conjugation and transduction, is unclear and the subject of considerable, ongoing effort in our laboratory.
In conclusion, KlcAHS was found effective in negating the Type I RM systems, probably facilitating HGT by functioning as an anti-restriction protein. Conceivably, the frequent occurrence of the KlcAHS gene in carbapenem-resistant K. pneumoniae isolates contributes to the yearly increase in these organisms isolated in our hospital.
Author Contributions
XJ and YL conceived and designed the study. WL performed the experiments and wrote the paper. YX, WX, YT, and GL provided suggestions and helped perform the experiments. All authors have read and approved this manuscript.
Funding
This study was supported by grants from the National Natural Science Foundation of China (NSFC 81571365, 81372141).
Conflict of Interest Statement
The authors declare that the research was conducted in the absence of any commercial or financial relationships that could be construed as a potential conflict of interest.
Acknowledgments
All model bacteria were presented as a gift by Prof. D. T. Dryden (University of Edinburgh, UK). Dr. Stephen H. Gregory (Providence, Rhode Island, USA) helped write and edit this manuscript.
References
Agarwal, A., Kapila, K., and Kumar, S. (2009). WHONET software for the surveillance of antimicrobial susceptibility. Med. J. Armed Forces India 65, 264–266. doi: 10.1016/S0377-1237(09)80020-8
Alikhan, N. F., Petty, N. K., Ben Zakour, N. L., and Beatson, S. A. (2011). BLAST Ring Image Generator (BRIG): simple prokaryote genome comparisons. BMC Genomics 12:402. doi: 10.1186/1471-2164-12-402
Balabanov, V. P., Kotova, V. Y., Kholodii, G. Y., Mindlin, S. Z., and Zavilgelsky, G. B. (2012). A novel gene, ardD, determines antirestriction activity of the non-conjugative transposon Tn5053 and is located antisense within the tniA gene. FEMS Microbiol. Lett. 337, 55–60. doi: 10.1111/1574-6968.12005
Belogurov, A. A., Delver, E. P., Agafonova, O. V., Belogurova, N. G., Lee, L. Y., and Kado, C. I. (2000). Antirestriction protein Ard (Type C) encoded by IncW plasmid pSa has a high similarity to the protein transport domain of TraC1 primase of promiscuous plasmid RP4. J. Mol. Biol. 296, 969–977. doi: 10.1006/jmbi.1999.3493
Brown-Jaque, M., Calero-Caceres, W., and Muniesa, M. (2015). Transfer of antibiotic-resistance genes via phage-related mobile elements. Plasmid 79, 1–7. doi: 10.1016/j.plasmid.2015.01.001
Carattoli, A. (2013). Plasmids and the spread of resistance. Int. J. Med. Microbiol. 303, 298–304. doi: 10.1016/j.ijmm.2013.02.001
Chan, W. T., Verma, C. S., Lane, D. P., and Gan, S. K. (2013). A comparison and optimization of methods and factors affecting the transformation of Escherichia coli. Biosci. Rep. 12:33. doi: 10.1042/BSR20130098
Chen, K., Reuter, M., Sanghvi, B., Roberts, G. A., Cooper, L. P., Tilling, M., et al. (2014). ArdA proteins from different mobile genetic elements can bind to the EcoKI Type I DNA methyltransferase of E. coli K12. Biochim. Biophys. Acta 1844, 505–511. doi: 10.1016/j.bbapap.2013.12.008
CLSI (2012). Methods for Dilution Antimicrobial Susceptibility Tests for Bacteria that Grow Aerobically; Approved Standard, 9th Edn. CLSI Document M07-A9. Wayne, PA: Clinical and Laboratory Standards Institute.
Diancourt, L., Passet, V., Verhoef, J., Grimont, P. A., and Brisse, S. (2005). Multilocus sequence typing of Klebsiella pneumoniae nosocomial isolates. J. Clin. Microbiol. 43, 4178–4182. doi: 10.1128/JCM.43.8.4178-4182.2005
Grant, J. R., and Stothard, P. (2008). The CGView Server: a comparative genomics tool for circular genomes. Nucleic Acids Res. 36, W181–W184. doi: 10.1093/nar/gkn179
Hu, F. P., Guo, Y., Zhu, D. M., Wang, F., Jiang, X. F., Xu, Y. C., et al. (2016). Resistance trends among clinical isolates in China reported from CHINET surveillance of bacterial resistance, 2005-2014. Clin. Microbiol. Infect. 22(Suppl. 1), S9–S14. doi: 10.1016/j.cmi.2016.01.001
Jonas, D. A., Elmadfa, I., Engel, K. H., Heller, K. J., Kozianowski, G., Konig, A., et al. (2001). Safety considerations of DNA in food. Ann. Nutr. Metab. 45, 235–254. doi: 10.1159/000046734
Kamachi, K., Sota, M., Tamai, Y., Nagata, N., Konda, T., Inoue, T., et al. (2006). Plasmid pBP136 from Bordetella pertussis represents an ancestral form of IncP-1beta plasmids without accessory mobile elements. Microbiology 152, 3477–3484. doi: 10.1099/mic.0.29056-0
Koraimann, G., and Wagner, M. A. (2014). Social behavior and decision making in bacterial conjugation. Front. Cell. Infect. Microbiol. 4:54. doi: 10.3389/fcimb.2014.00054
Lorenz, M. G., and Wackernagel, W. (1994). Bacterial gene transfer by natural genetic transformation in the environment. Microbiol. Rev. 58, 563–602.
McMahon, S. A., Roberts, G. A., Johnson, K. A., Cooper, L. P., Liu, H., White, J. H., et al. (2009). Extensive DNA mimicry by the ArdA anti-restriction protein and its role in the spread of antibiotic resistance. Nucleic Acids Res. 37, 4887–4897. doi: 10.1093/nar/gkp478
Naas, T., Cuzon, G., Villegas, M. V., Lartigue, M. F., Quinn, J. P., and Nordmann, P. (2008). Genetic structures at the origin of acquisition of the beta-lactamase bla KPC gene. Antimicrob. Agents Chemother. 52, 1257–1263. doi: 10.1128/AAC.01451-07
Nie, Y., Yan, W., Xu, Y., Chen, W. B., Mu, X. Q., Wang, X., et al. (2013). High-level expression of Bacillus naganoensis pullulanase from recombinant Escherichia coli with auto-induction: effect of lac operator. PLoS ONE 8:e78416. doi: 10.1371/journal.pone.0078416
Nordmann, P., Naas, T., and Poirel, L. (2011). Global spread of Carbapenemase-producing Enterobacteriaceae. Emerg. Infect. Dis. 17, 1791–1798. doi: 10.3201/eid1710.110655
Oliveira, P. H., Touchon, M., and Rocha, E. P. (2016). Regulation of genetic flux between bacteria by restriction-modification systems. Proc. Natl. Acad. Sci. U.S.A. 113, 5658–5663. doi: 10.1073/pnas.1603257113
Pitout, J. D., Nordmann, P., and Poirel, L. (2015). Carbapenemase-producing Klebsiella pneumoniae, a key pathogen set for global nosocomial dominance. Antimicrob. Agents Chemother. 59, 5873–5884. doi: 10.1128/AAC.01019-15
Roberts, R. J., Vincze, T., Posfai, J., and MacElis, D. (2015). REBASE–a database for DNA restriction and modification: enzymes, genes and genomes. Nucleic Acids Res. 43, D298–D299. doi: 10.1093/nar/gku1046
Roer, L., Aarestrup, F. M., and Hasman, H. (2015). The EcoKI type I restriction-modification system in Escherichia coli affects but is not an absolute barrier for conjugation. J. Bacteriol. 197, 337–342. doi: 10.1128/JB.02418-14
San Millan, A., Toll-Riera, M., Qi, Q., and MacLean, R. C. (2015). Interactions between horizontally acquired genes create a fitness cost in Pseudomonas aeruginosa. Nat. Commun. 6:7845. doi: 10.1038/ncomms7845
Serfiotis-Mitsa, D., Herbert, A. P., Roberts, G. A., Soares, D. C., White, J. H., Blakely, G. W., et al. (2010). The structure of the KlcA and ArdB proteins reveals a novel fold and antirestriction activity against Type I DNA restriction systems in vivo but not in vitro. Nucleic Acids Res. 38, 1723–1737. doi: 10.1093/nar/gkp1144
Shen, P., Zhang, Y., Li, G., and Jiang, X. (2016). Characterization of the genetic environment of the blaKPC-2 gene among Klebsiella pneumoniae isolates from a Chinese Hospital. Braz. J. Infect. Dis. 20, 384–388. doi: 10.1016/j.bjid.2016.04.003
Thomas, C. M., and Nielsen, K. M. (2005). Mechanisms of, and barriers to, horizontal gene transfer between bacteria. Nat. Rev. Microbiol. 3, 711–721. doi: 10.1038/nrmicro1234
Tian, L., Tan, R., Chen, Y., Sun, J., Liu, J., Qu, H., et al. (2016). Epidemiology of Klebsiella pneumoniae bloodstream infections in a teaching hospital: factors related to the carbapenem resistance and patient mortality. Antimicrob. Resist. Infect. Control 5:48. doi: 10.1186/s13756-016-0145-0
Tock, M. R., and Dryden, D. T. (2005). The biology of restriction and anti-restriction. Curr. Opin. Microbiol. 8, 466–472. doi: 10.1016/j.mib.2005.06.003
Vasu, K., Nagamalleswari, E., and Nagaraja, V. (2012). Promiscuous restriction is a cellular defense strategy that confers fitness advantage to bacteria. Proc. Natl. Acad. Sci. U.S.A. 109, E1287–E1293. doi: 10.1073/pnas.1119226109
Veiga, H., and Pinho, M. G. (2009). Inactivation of the SauI type I restriction-modification system is not sufficient to generate Staphylococcus aureus strains capable of efficiently accepting foreign DNA. Appl. Environ. Microbiol. 75, 3034–3038. doi: 10.1128/AEM.01862-08
Webb, J. L., King, G., Ternent, D., Titheradge, A. J., and Murray, N. E. (1996). Restriction by EcoKI is enhanced by co-operative interactions between target sequences and is dependent on DEAD box motifs. EMBO J. 15, 2003–2009.
Wiedenbeck, J., and Cohan, F. M. (2011). Origins of bacterial diversity through horizontal genetic transfer and adaptation to new ecological niches. FEMS Microbiol. Rev. 35, 957–976. doi: 10.1111/j.1574-6976.2011.00292.x
Wilkins, B. M. (2002). Plasmid promiscuity: meeting the challenge of DNA immigration control. Environ. Microbiol. 4, 495–500. doi: 10.1046/j.1462-2920.2002.00332.x
Yigit, H., Queenan, A. M., Anderson, G. J., Domenech-Sanchez, A., Biddle, J. W., Steward, C. D., et al. (2001). Novel carbapenem-hydrolyzing beta-lactamase, KPC-1, from a carbapenem-resistant strain of Klebsiella pneumoniae. Antimicrob. Agents Chemother. 45, 1151–1161. doi: 10.1128/AAC.45.4.1151-1161.2001
Keywords: K. pneumoniae, KlcAHS, Type I RM systems, horizontal gene transfer, transformation
Citation: Liang W, Xie Y, Xiong W, Tang Y, Li G, Jiang X and Lu Y (2017) Anti-Restriction Protein, KlcAHS, Promotes Dissemination of Carbapenem Resistance. Front. Cell. Infect. Microbiol. 7:150. doi: 10.3389/fcimb.2017.00150
Received: 08 December 2016; Accepted: 11 April 2017;
Published: 02 May 2017.
Edited by:
Jing-Ren Zhang, Tsinghua University, ChinaReviewed by:
Jens Kreth, Oregon Health & Science University, USAShaolin Wang, China Agricultural University, China
Copyright © 2017 Liang, Xie, Xiong, Tang, Li, Jiang and Lu. This is an open-access article distributed under the terms of the Creative Commons Attribution License (CC BY). The use, distribution or reproduction in other forums is permitted, provided the original author(s) or licensor are credited and that the original publication in this journal is cited, in accordance with accepted academic practice. No use, distribution or reproduction is permitted which does not comply with these terms.
*Correspondence: Xiaofei Jiang, jiangxi2154@aliyun.com
Yuan Lu, luyuan@sccl.org.cn