- 1Key Laboratory for Zoonosis Research, Department of Infectious Diseases, First Hospital of Jilin University, Ministry of Education, College of Veterinary Medicine, College of Food Science and Engineering, Institute of Zoonosis, Jilin University, Changchun, China
- 2Department of Food Quality and Safety, College of Food Science and Engineering, Tonghua Normal University, Tonghua, China
- 3Key Lab for New Drug Research of TCM, Research Institute of Tsinghua University in Shenzhen, Shenzhen, China
- 4Jiangsu Co-innovation Center for Prevention and Control of Important Animal Infectious Diseases and Zoonoses, Yangzhou, China
A novel treatment regimen for bacterial infections is the pharmacological enhancement of the host's immune defenses. We demonstrated that biochanin A (BCA), an isoflavone constituent in some plants, could enhance both intra- and extracellular bactericidal activity of host cells. First, BCA could induce a complete autophagic response in nonphagocytic cells (HeLa) or macrophages (MΦ) via the AMPK/ULK1/mTOR pathway and Beclin-1-dependent manner, and BCA enhanced the killing of invading Salmonella by autophagy through reinforcing ubiquitinated adapter protein (LRSAM1, NDP52 and p62)-mediated recognition of intracellular bacteria and through the formation of autophagolysosomes. Second, we demonstrated that BCA could enhance the release of MΦ extracellular traps (METs) to remove extracellular Salmonella also via the AMPK/ULK1/mTOR pathway, not through reactive oxygen species (ROS) pathway. Furtherly, in a Salmonella-infected mouse model, BCA treatment increased intra- and extracellular bactericidal activity through the strengthening autophagy and MET production, respectively, in peritoneal MΦ, liver and spleen tissue. Additionally, our findings showed that BCA downregulated SPI-1 (Salmonella pathogenicity island 1) expression during Salmonella infection in vitro and in vivo to reverse the MΦ M2 polarization, which was different from the MΦ M1 phenotype caused by most of bacteria infection. Together, these findings suggest that BCA has an immunomodulatory effect on Salmonella-infected host cells and enhances their bactericidal activity in vitro and in vivo through autophagy, extracellular traps and regulation of MΦ polarization.
Introduction
The wide spread of drug-resistant bacteria and the slow development of new antimicrobial agents has created a desperate scarcity of new therapeutic approaches of bacterial infections (Viveiros et al., 2012). The enhancement of the host's immune defenses through pharmacological treatment is a novel area of study (Ankomah and Levin, 2014). There is an urgent need to discover agents that can enhance both extra- and intracellular bactericidal action in the host (Viveiros et al., 2012).
The innate immune system is the first line of defense against invading microorganisms through immune response elements such as reactive oxygen species (ROS), autophagy and extracellular traps (ETs) (Medzhitov, 2010). Autophagy is the natural, regulated mechanism of the cell, in which cytoplasmic components are delivered to lysosomes and autophagosomes for degradation (Yuan et al., 2012). The classical autophagy mechanism depends on two ubiquitin-like conjugation systems: Atg4-Atg7-Atg8 or Atg7-Atg12-Atg5. These two systems are important for the formation of an early complex containing class III phosphoinositide 3-kinase, which then forms the autophagosome (Yuan et al., 2012). Recent reports have demonstrated that AMP-activated protein kinase (AMPK) and mTOR coordinate autophagy initiation in mammalian cells (Shang and Wang, 2011). ETs were recognized recently (Brinkmann et al., 2004) and can be produced by many innate effector cells including macrophages (MΦ), eosinophils, mast cells and neutrophils. ETs are fiber-like extracellular structures that can defend against infections by trapping extracellular bacteria or fungi (Brinkmann et al., 2004). NADPH oxidase (NOX2)-dependent or NOX2-independent oxidative bursts have been reported to mediate ET formation (Remijsen et al., 2011).
According to the tissue microenvironment, peripheral monocytes could polarize to different MΦs subtypes, including M1 MΦs and M2 MΦs. M1 MΦs are the classical activated MΦs, which produce pro-inflammatory cytokines such as TNF-Φ and IL-12 and clear pathogens but also cause tissue damage. In contrast, M2 MΦs are alternatively activated, which generate anti-inflammatory mediators such as IL-10, suppress facilitate wound healing and inflammation (Erbel et al., 2013). It was proved that some medicine and compounds could induce MΦ polarization to M1-like MΦs or M2-like MΦs (Gharib et al., 2014).
Salmonella enterica Typhimurium (Salmonella) is an intracellular bacterial pathogen that is the leading cause of enteric disease in humans and in animal hosts. Salmonella can invade, survive and replicate in phagocytic and non-phagocytic cells (Davidson et al., 2015). The results proved that Salmonella could escape from Salmonella-containing vacuoles (SCVs) and enter the epithelial cells' cytosol. They are then selected for autophagy mediated by the accumulation of ubiquitin around target bacteria and for binding of the ubiquitin recognizing adaptor proteins NDP52, P62, and LRSAM1, which is essential because the E3 ubiquitin ligase is responsible for antibacterial ubiquitin association (Thurston et al., 2009; Cemma et al., 2011). Especially, it has been reported that Salmonella infection can polarize MΦs toward M2 phenotype that was dependent on the expression of Salmonella pathogenicity island 1 (SPI-1) (Kyrova et al., 2012), the M2 polarization caused by Salmonella infection was different from other bacteria infection that caused MΦ M1 phenotype polarization.
Until recently, few agents were found to enhance bacterial clearance by promoting anti-bacterial autophagy (Conway et al., 2013) or ETs (Chow et al., 2010). In this study, we found that Biochanin A (BCA), a major isoflavone constituent found in red clover, cabbage, alfalfa and some other herbal dietary supplements, could enhance bactericidal intra- and extracellular activity. BCA also has putative benefits in dietary cancer prophylaxis (Medjakovic and Jungbauer, 2008). BCA has potential antimicrobial activity against several types of bacteria, but these cases include only high minimum inhibitory concentration (MIC) values (Liu et al., 2011). BCA is a common product extracted from natural plant and is considered to be innocuous (Sklenickova et al., 2010). We found that BCA induced the autophagic response in epithelial cells and MΦs or induced ET formation of MΦs in vitro and in an in vivo mouse model. We also investigated the influence of BCA on SPI-1-dependent MΦ polarization during bacteria infection.
Materials and Methods
Antibodies, Chemicals, Plasmids, and Strains
BCA was purchased from Wako Pure Chemicals, Industries, Ltd. (Chuo-ku, Osaka, Japan). The following antibodies used for western blotting were purchased from Cell Signaling Technology (Massachusetts, USA): Beclin-1 antibody (3495S), p62/SQSTM1 antibody (5114S), p-ULK1ser317 antibody (6887S), p-ULK1ser757 antibody (6888S), p-p70S6K antibody (9234P), p-4E-BP1 antibody (9451P), p-mTOR antibody (5536P), p-AMPKβ antibody (4181P), β-actin antibody (4970S) and anti-rabbit horseradish peroxidase-labeled antibody (7054S). The LC3B antibody was purchased from Sigma Chemical Company (042M4774V). All other reagents were purchased from Sigma Chemical Co. (St. Louis, MO, U.S.A.) unless otherwise stated. Salmonella ATCC14028 was stored at −80°C.
Cells and Cell Cultures
All the cells (HeLa cells, Raw264.7 cells and THP-1 cells) were bought from ATCC (Maryland, USA) and maintained in RPMI 1640 medium with additional 10% fetal bovine serum (FBS). All the culture reagents were obtained from GIBCO Laboratories (NY, USA). The cells were incubated at 37°C with 5% CO2. Phorbol myristate acetate (PMA) (Sigma, Missouri, USA) with the concentration of 10 ng/ml for 24 h was used to induce the THP-1 cells to differentiate into MΦ-like cells.
Transmission Electron Microscopy
The specimen preparation was performed as other research report described (Yuan et al., 2012). A transmission electron microscope (Hitachi H-7650, Tokyo, Japan) was used to observe the autophagosome-like vesicles.
SDS/PAGE and Western Blotting
Western blotting and SDS/PAGE were operated as other research report described (Mizusaki et al., 2008; Yuan et al., 2012). A CanoScan LiDE 100 scanner (Canon) was used to obtain the images. The results were quantified with Image-J software.
Quantitative Real-Time RT-PCR (q-RT-PCR) Assays
RNA extraction was performed with RNAprep Pure Cell/Bacteria Kit (TIANGEN Biotech, Beijing, China) according to the manufacturer's instructions. cDNA was synthesized and all PCRs were performed as previous described (Mizusaki et al., 2008). The primers used in our study were as follows: for the 16S rRNA gene, forward primer TGTAGCGGTGAAATGCGTAG and reverse primer CAAGGGCACAACCTCCAAG (predicted length of the transcript, 161 bp); for hilA, forward primer CATGGCTGGTCAGTTGGAG and reverse primer CGTAATTCATCGCCTAAACG (predicted length of the transcript, 150 bp); for hilD, forward primer ACTCGAGATACCGACGCAAC and reverse primer CTTCTGGCAGGAAAGTCAGG (predicted length of the transcript, 129 bp). An ABI Prism 7500 Sequence Detection System (Applied Bio-systems) was used to perform the real-time PCR and set in the standard run mode. To collect the data of real-time PCR, we used the Rotor Gene 6000 series software 1.7. Reaction conditions were 5 m at 94°C, and 40 cycles at 94°C for 5 s and 60°C for 35 s. All real-time PCR reactions were carried out in triplicate and were repeated from three different bacterial cultures.
Confocal Microscopy and Immunofluorescence Staining
The cells were grown in glass-bottomed cell culture dishes. The samples were prepared as previously described (Cemma et al., 2011). An Olympus FV1000 confocal laser scanning microscope (Olympus, Tokyo, Japan) was used to catch the images with a 60 × objective lens. The images were analyzed using Fluoview ver. 1.7.3.0 (Olympus, Tokyo, Japan).
Detection of the Quantity of Extracellular DNA (eDNA)
Raw264.7 cells were seeded in 24-well plates. eDNA was labeled with 20 μM Sytox Green (Invitrogen, OR, USA) for 10 min at room temperature. The ODs were measured with a spectrofluorophotometer (Infinite F200 Pro, TECAN, Switzerland) at a 480-nm excitation and a 545-nm emission wavelength (Brinkmann et al., 2004).
MΦ Bactericidal Activity Assays
The intracellular bacterial killing assay was performed and evaluated by a gentamicin protection assay according to a previously published method (Wiedemann et al., 2012; Wang et al., 2016). The extracellular bacterial killing assay was performed and evaluated in accordance with a previously described method (Wang et al., 2016).
Detection of Cytosolic ROS
We used dihydrorhodamine (DHR) 123 for cytosolic ROS detection, (a fluorescent indicator of cytosolic ROS). The cells were stimulated with the indicated reagents or infected with bacteria and then incubated with 1 μM DHR 123 for 20 min without light. The results were subsequently obtained using a spectrofluorophotometer (Infinite F200 Pro, TECAN, Switzerland) at a 485-nm excitation and a 535-nm emission wavelength (Shen et al., 2016).
Flow Cytometry
Staining of cells and analysis on a flow cytometer (FACScan; BD Biosciences) were done as described (Wang et al., 2017). Briefly, M1 and M2 MΦs were identified using the following antibodies: FITC Anti-CCR7 (eBioscience, 11-1979-42), PE Anti-CD163 (eBioscience, 12-1639-41), FITC Anti-CD206 (Invitrogen, MA5-16870) and PE anti-CD86 (Bioss, bs-1035R). The data acquired were analyzed with FlowJo (Treestar software, Ashland, OR, USA).
Quantification of Cytokines
The cytokines TNF-α and IL-10 were quantified using ELISA with the Cytokine Duo-Set Kit (R&D Systems, Minneapolis, USA). The experiment was performed according the kit introduction.
In vivo Studies
Female BALB/c mice (8–10 weeks old) were bought from the Shanghai Experimental Animal Center (Shanghai, China). The mice were kept under pathogen-free condition before infection with Salmonella. The mice were infected by intragastric administration of an overnight culture of Salmonella (105 bacteria in 0.1 ml PBS) through a gavage tube. To make sure the number of bacteria inoculated, the same overnight culture was plated onto LB agar. At 24 h postinfection, mice were assigned to treatment groups and began receiving vehicle (0.5% methylcellulose-0.1% Tween 80 in sterile water) or BCA administered intragastrically by gavage once daily (Chiu et al., 2009).
The mice were treated with vehicle or BCA at 3.125–12.5 mg/kg intragastrically and/or 3-MA at 24 mg/kg/day intraperitoneally or 1000 U DNase I intraperitoneally once daily for 4 days (10 mice for each group). The mice were sacrificed by cervical dislocation at 5 days p.i. to collect the peritoneal fluid, liver and spleen for RNA extraction, bacterial burden quantification and protein extraction (Chiu et al., 2009; Croswell et al., 2009; Yipp et al., 2012).
Ethics Statement
All animal studies were conducted according to the experimental practices and standards that were approved by the Animal Welfare and Research Ethics Committee at Jilin University (No: IZ-2009-008). The mouse experiment was performed strictly according to the National Research Council Guide for Care and Use of Laboratory Animals of People's Republic of China.
Statistical Analysis
All results were expressed the as the mean ± SD. The group means were compared using one-way ANOVA, and Student's t-test was used to determine significance. P values of 0.05 or less were considered statistically significant.
Results
BCA Induced a Complete Autophagy in Nonphagocytic Cells and Phagocytic Cells
To detect whether BCA induces an autophagic response in vitro, we transfected the RFP-LC3 plasmid into nonphagocytic (HeLa) and phagocytic (THP-1) cells. Our confocal microscopy results showed that more cells had LC3 puncta after BCA treatment than untreated cells (P < 0.001) (Figures 1A,B), which suggested that BCA could induce the autophagic response in nonphagocytic and phagocytic cells. There were significantly more autophagosome vesicles in BCA-treated HeLa cells than in control cells (P < 0.05) (Figures 1C,D). This demonstrated that BCA could induce autophagy. In addition, our western blot results indicated that LC3-II/LC3-I ratio increased in BCA-treated HeLa cells, Raw264.7 cells and THP-1 cells compared to their respective control cells (P < 0.01; P < 0.05; P < 0.05), whereas the autophagic inducer rapamycin and the autophagic inhibitor 3-MA increased and decreased the ratio of LC3-II/I, respectively (Figure 1E). These data indicated that BCA can induce the autophagic response in epithelial cells and macrophages.
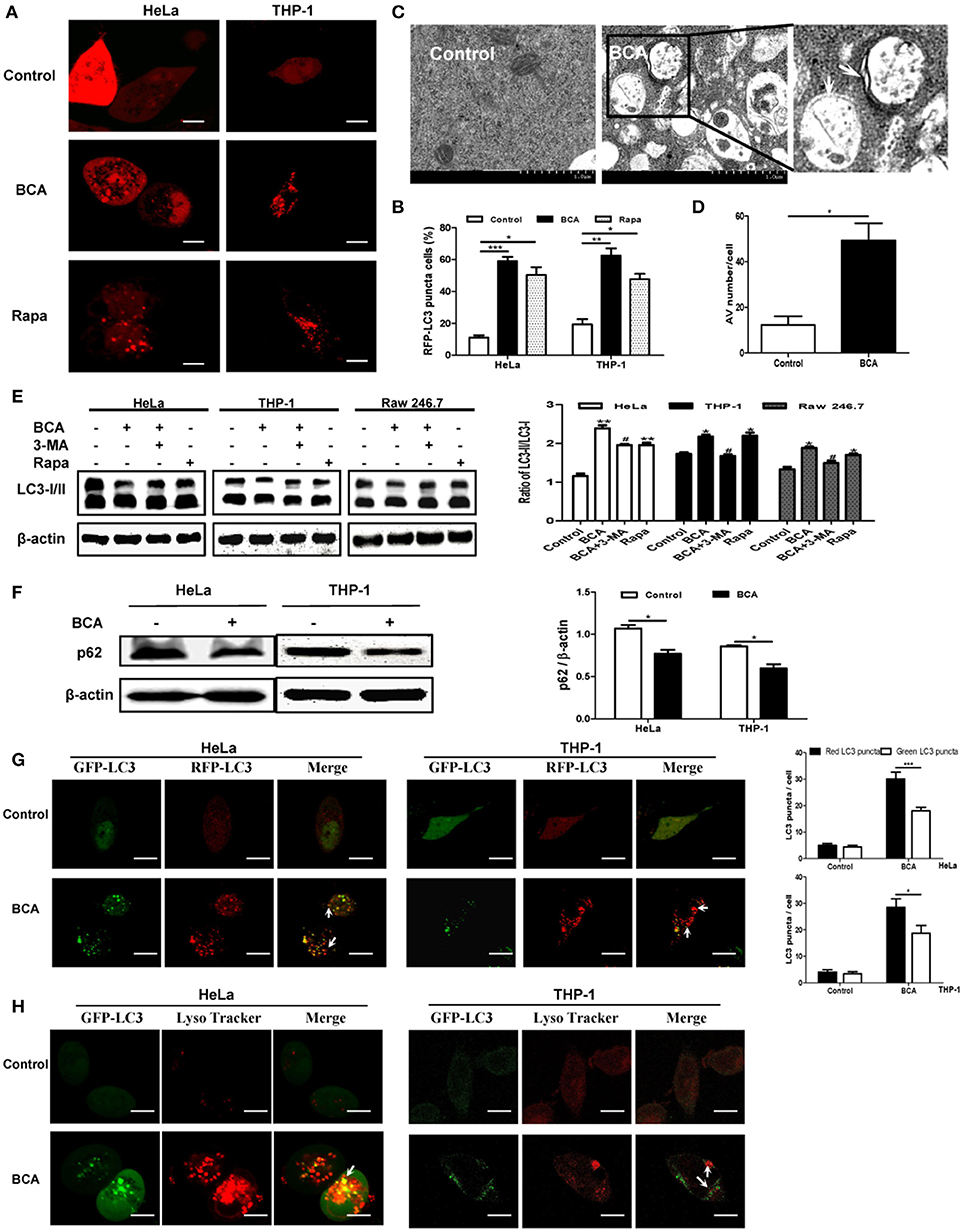
Figure 1. BCA induced a complete autophagy in mammalian cells. (A,B) HeLa and THP-1 cells were transfected with pmRFP-LC3 plasmid for 24 h and then treated with 4 μg/ml BCA for 2 h. The cells were pretreated with rapamycin (5 μM, 12 h) or 3-MA (3 μM, 3 h). Puncta in each cell were counted, and cells with more than 10 puncta were considered LC3-RFP puncta cells. The values were measured using 100 cells/sample. *P < 0.05; **P < 0.01; ***P < 0.001. (C,D) Treatment with BCA resulted in elevated autophagosome formation in HeLa cells. The cells were pretreated with rapamycin or 3-MA and then treated with 4 μg/ml BCA for 2 h. The cells were examined using TEM. Boxed areas are further enlarged images; arrows indicate autophagosomes. The number of autophagic vesicles (AVs) from 20 cells in each sample was determined. *P < 0.05. (E) HeLa, THP-1 and Raw264.7 cells were pretreated with rapamycin or 3-MA and then treated with 4 μg/ml BCA for 2 h. The cells were then prepared for western blotting for LC3 was performed. The ratio of LC3-II/LC3-I was calculated. *P < 0.05, **P < 0.01, compared with the control group. #P < 0.05, compared with BCA-treated cells. The data are representative of three experiments with similar results. (F) HeLa and THP-1 cells were treated with 4 μg/ml BCA for 2 h, and the western blotting for p62 was performed. The ratio of p62 to β-actin was calculated. *P < 0.05. (G) HeLa and THP-1 cells were transfected with the ptfLC3 plasmid for 24 h, treated with BCA for 2 h and then observed using confocal microscopy. The arrows indicate LC3 puncta. Red or green LC3 puncta per cell were counted. *P < 0.05, ***P < 0.001. (H) HeLa and THP-1 cells were transfected with pEGFP-LC3 for 24 h and treated with BCA for 2 h. The cells were incubated with 50 nM of LysoTracker for 1 h at 37°C and observed using confocal microscopy. The data are representative of three experiments with similar results.
Autophagy can be divided into two categories, complete and incomplete, according to whether autolysosome degradation occurs (Yuan et al., 2012). Previous studies proved that the decline of p62 expression is an important evidence for determining autophagic degradation (Klionsky et al., 2008). We found that the level of p62 expression decreased in HeLa and THP-1 cells treated with BCA (P < 0.05) (Figure 1F). In addition, an mRFP-GFP tandem fluorescent tagged LC3 plasmid (ptfLC3) was used in our study, which contains both red and green fluorescent proteins. When autophagosomes form, the ptfLC3 plasmid expresses both red and green fluorescence in the same puncta (Yuan et al., 2012). However, when autolysosomes form, which result from the fusion of autophagosomes and lysosomes, the GFP signal is disappeared, but RFP-LC3 is still expressed and represent LC3 puncta. In this study, after treatment with BCA, we observed the numbers of green and red LC3 puncta increased, and there were more red puncta than green puncta in BCA-treated HeLa and THP-1 cells (Figure 1G). This result confirmed the induction of autolysosome formation. In addition, we stained HeLa cells with LysoTracker DND 99, a weak lysosomotropic base that accumulates and fluoresces within acidic vesicles (Arroyo et al., 2013; Sun et al., 2014). The results showed that LC3 puncta colocalized with LysoTracker in BCA-treated HeLa and THP-1 cells (Figure 1H). This result suggested that BCA induced the acidification of autolysosomes. These data demonstrated that BCA treatment could induce a complete autophagic response and promote autophagic degradation in mammalian cells.
BCA Induced a Beclin-1-Dependent Autophagic Response Mediated by the AMPK/ULK1/mTOR Pathway
In our analysis of the possible pathways involved in the BCA induced autophagic response, we found that the phosphorylation levels of p70S6K, 4E-BP1 and mTOR were obviously declined in a time-dependent manner in HeLa and THP-1 cells after BCA treatment compared to the untreated cells, while the phosphorylations of AMPK and ULK1 (Ser 317 and Ser757) were increased. The LC3-II/LC3-I ratio also increased in a time-dependent manner after BCA treatment (Figure 2A). In addition, the cells were treated with BCA and compound C (an AMPK inhibitor) to better know this pathway (Park et al., 2013). The results showed that compound C lowered AMPK and ULK1 phosphorylation and decreased LC3-II expression induced by BCA (P < 0.05) (Figures 2B–E), while it increased the phosphorylation of mTOR (Figures 2B,D,E). Together, these results suggested that BCA treatment activated the AMPK/mTOR/Ulk1 autophagy pathway.
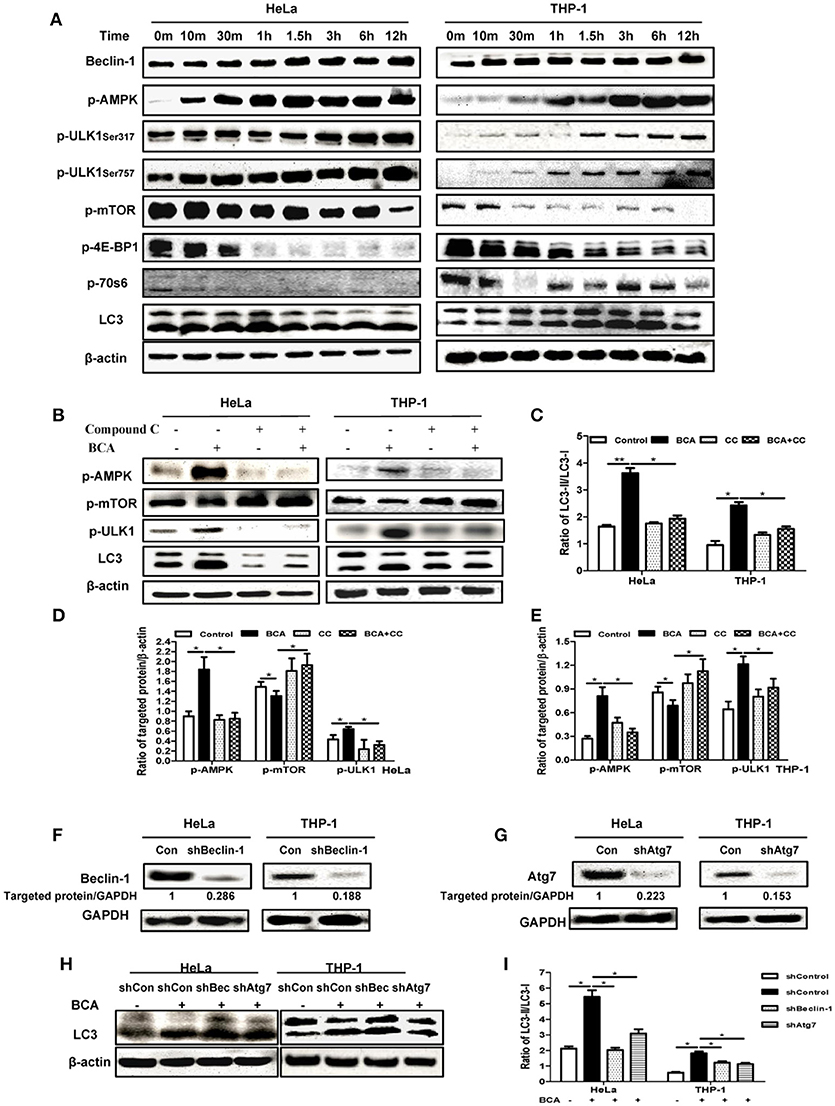
Figure 2. BCA induced a Beclin-1 dependent autophagic response mediated by the AMPK/ULK1/mTOR pathway. (A) HeLa and THP-1 cells were treated with 4 μg/ml BCA for 12 h. Then, western blotting for Beclin-1, p-AMPK, p-ULK1, p-mTOR, p-4E-BP1, p-70S6 and LC3 was performed. (B) HeLa and THP-1 cells were treated with 4 μg/ml BCA for 2 h with or without pretreatment with 5 μM compound C. Western blotting for p-AMPK, p-mTOR, p-ULK1 and LC3 was performed. (C) The ratio of LC3II/LC3-I was calculated. *P < 0.05; **P < 0.01. (D,E) The ratio of targeted protein/β-actin in HeLa or THP-1 cells was calculated. *P < 0.05. (F) HeLa and THP-1 cells were transfected with shRNA negative control, shBeclin-1 or shAtg7 plasmids for 48 h before treatment with BCA for 2 h. (G) Western blotting for Beclin-1 and Atg7. (H,I) Western blotting for LC3 was performed. *P < 0.05. The data are representative of three experiments with similar results.
In addition, we found that BCA treatment increased Beclin-1 expression in HeLa and THP-1 cells (Figure 2A), which implied that the autophagy induced by BCA might be via by Beclin-1. To further confirm the correlation between Beclin-1 and BCA-induced autophagy, we used specific shBeclin-1 plasmids to knock down Beclin-1 expression in these two cell lines (Figure 2F). The ratio of LC3-II/LC3-I in BCA-treated HeLa cells and THP-1 cells transfected with the shBeclin-1 plasmid markedly decreased compared with cells transfected with negative shRNA plasmid and control group (P < 0.05) (Figure 2H,I). To further investigate this pathway, we also used shAtg7 plasmids to knock down the expression of Atg7, a major autophagy related downstream protein, and the ratio of LC3-II/LC3-I in BCA-treated HeLa and THP-1 cells after Atg7-specific knockdown significantly decreased compared to the control group (P < 0.05) (Figures 2F–I). Taken together, these data indicated that Beclin 1 plays a crucial role in the mechanism of autophagy induced by BCA.
BCA Improved the Killing of Intracellular Salmonella Through Autophagy by Enhancing Adapter Protein Mediated Recognition of Invading Bacteria and the Formation of Autophagolysosomes
To examine the effect of autophagy induced by BCA on intracellular Salmonella, we compared LC3 expression in Salmonella infected HeLa cells with or without BCA treatment. Salmonella infection increased the LC3-II/I ratio, and pretreatment with BCA significantly enhanced the increase of the ratio (P < 0.05) (Figures 3A,B). Immunofluorescence staining showed that colocalization between NDP52, p62, LRSAM1, the LC3 puncta and intracellular bacteria was significantly increased in those BCA-pretreated cells (Figures 3C and Figure S1), and colocalization between LAMP1, LysoTracker, LC3 puncta and intracellular bacteria also increased significantly in those BCA-pretreated cells (Figure 3D and Figure S1). Moreover, western blot results showed that BCA enhanced the expression of ubiquitinated adapter proteins NDP52, p62, and ubiquitin ligase LRSAM1 (Figure 3E), which indicated that the enhanced expression of ubiquitinated adapter proteins NDP52, p62, and ubiquitin ligase LRSAM1 may be the direct result of restricting the proliferation of intracellular Salmonella through autophagy induced by BCA. A gentamicin protection assay indicated that the number of intracellular bacteria in the BCA-treated cells was significantly decreased (P < 0.05), whereas that of the 3-MA pre-treated cells showed the opposite result (P < 0.05) (Figure 3F). To investigate the anti-microbial activity on Salmonella alone, we also detected the minimum inhibitory concentration (MIC) of BCA and found the MIC was more than 512 μg/ml, which was much higher than the concentration we used to induce autophagy. Together, these data suggest that BCA improved the killing of intracellular Salmonella through autophagy by enhancing adapter proteins (LRSAM1, NDP52 and p62)-mediated recognition of invading bacteria and formation of autophagolysosome.
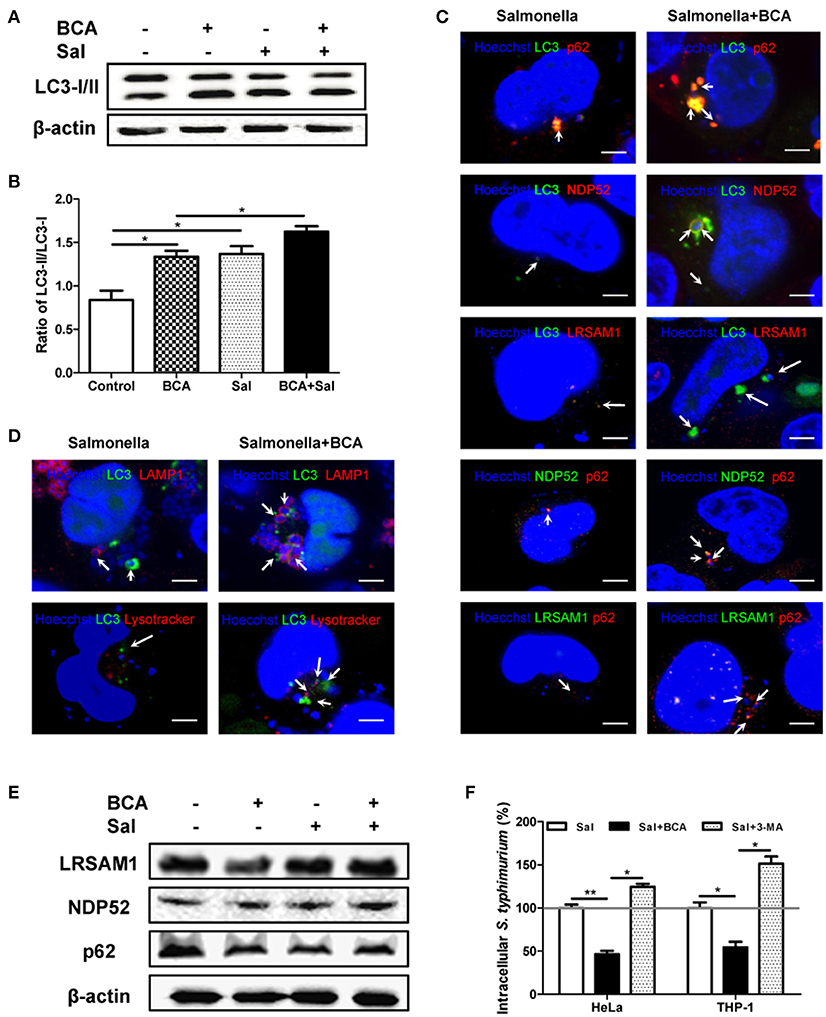
Figure 3. BCA enhanced the autophagic response induced by Salmonella. HeLa cells were pretreated with 4 μg/ml BCA for 2 h and then infected with Salmonella for 2 h (MOI = 10:1). (A) Western blotting for LC3 was conducted. (B) The ratio of LC3-II/LC3-I was calculated. *P < 0.05. (C,D) The cells were immunostained with antibodies against NDP52 and p62 and Hoechst 33342 then analyzed for colocalization of the adaptor proteins and intracellular bacteria. HeLa cells were transfected with pEGFP-LC3 for 24 h before treatment with BCA and infected with Salmonella. The cells were immunostained with different antibodies (NDP52, p62, and LAMP1), LysoTracker and Hoechst 33342 and then analyzed for colocalization of the adaptor proteins, LAMP1, LysoTracker, LC3 puncta and intracellular bacteria and were observed using confocal microscopy. (E) HeLa cells were pretreated with 4 μg/ml BCA for 2 h then infected with Salmonella for 2 h (MOI = 10:1). Then, western blotting of LRSAM1, NDP52, and p62 was performed. (F) HeLa cells and THP-1 cells were treated with BCA or 3-MA and infected with Salmonella for 2 h, then cultured in medium containing 100 μg of gentamicin ml−1 for 1 h to remove the extracellular bacteria. The intracellular bacteria were counted as CFUs. *P < 0.05; **P < 0.01.
BCA Induced MΦ Extracellular Trap (MET) Formation and Improved Extracellular Bacterial Killing in vitro
To investigate whether BCA could induce MET formation and its effect on the extracellular bacterial killing of Salmonella, BCA-treated cells were stained with Hoechst 33342 and Sytox Orange and observed with fluorescence microscopy. BCA treatment induced MET formation and significantly increased the MET production of Salmonella-infected Raw264.7 cells (Figure 4A); the results were confirmed by the quantification of eDNA (a major component of ETs) with a spectrofluorophotometer (Figure 4B). Furthermore, a phagocytosis inhibition assay showed that BCA significantly increased the amount of extracellular Salmonella killed by Raw264.7 cells (P < 0.05), while DNase I treatment abolished this effect of BCA (P < 0.05) (Figure 4C). Taken together, these results indicate that BCA could kill extracellular Salmonella through inducing MET formation.
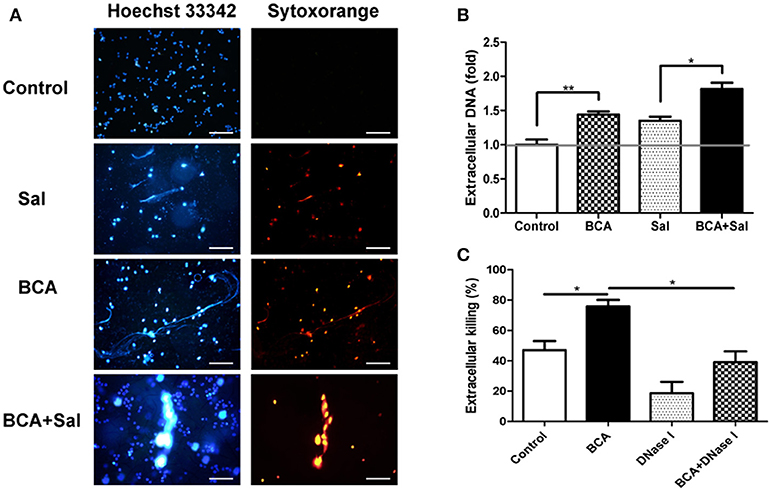
Figure 4. BCA induced MET formation and improved extracellular bacterial killing. (A,B) BCA induced Raw264.7 cells to release eDNA. Raw264.7 cells were incubated with serum-free and antibiotic-free medium and treated with 4 μg/ml BCA for 2 h. Then, the cells were infected with Salmonella at MOI = 10:1 for 2 h. (A) The cells were stained with Hoechst 33342 and Sytox orange and observed by fluorescence microscopy. (B) The cells were stained with 1 μM Sytox green for 10 min and detected with a plate reader. *P < 0.05; **P < 0.01. (C) Raw264.7 cells pretreated with 10 μg/ml cytochalasin D for 20 min were treated with 4 μg/ml BCA and/or 40 U/ml DNase I and infected with Salmonella at MOI = 10:1 for 2 h. The supernatants were collected for CFU quantification and the calculation of the extracellular bacterial killing rate. *P < 0.05. The data are representative of three experiments with similar results.
AMPK/ULK1/mTOR Mediated Met Formation Induced by BCA Without ROS Involvement
To investigate whether ROS takes part in ET formation induced by BCA in MΦ, we quantified the levels of cytosolic ROS and eDNA in BCA-treated Raw264.7 cells with or without DPI (a NADPH inhibitor) (Douda et al., 2015). Phorbol 12-myristate 13-acetate (PMA), a ROS inducer, was used as a positive control. The results showed that BCA inhibited cytosolic ROS production in a dose-dependent manner and 2 μg/ml was the minimal concentration of BCA, which inhibited ROS formation (Figures 5A,B). DPI treatment had no effect on the release of eDNA induced by BCA and the extracellular Salmonella killing, while both compound C (an AMPK inhibitor) and Torin 1 (a mTORC1/2 inhibitor) inhibited the release of eDNA induced by BCA and decreased the extracellular bacterial killing (Figures 5C–E). These results indicate that it is AMPK/ULK1/mTOR mediate the MET formation and MET-related extracellular bacterial killing of Salmonella induced by BCA, but not ROS.
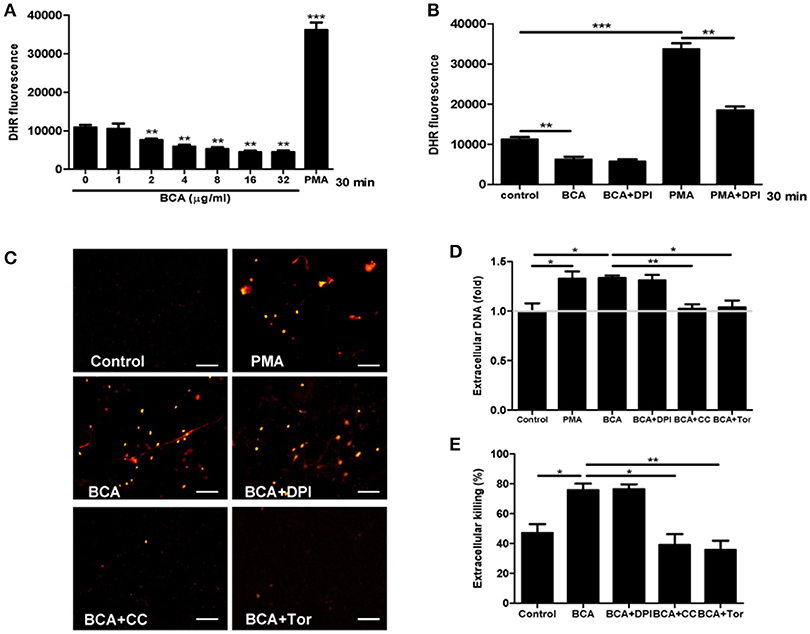
Figure 5. BCA induced AMPK-dependent MET formation without ROS involvement. (A,B) Raw264.7 cells were treated with BCA at different concentrations for 30 min or 4 μg/ml for 30 min with or without 50 μM DPI. Then, the cells were incubated with dihydrorhodamine (DHR) 123 for 20 min without light. The plates were examined with a spectrofluorophotometer. *P < 0.05, **P < 0.01, ***P < 0.001 compared with the control group. The gray horizontal line represents 1. (C,D) Raw264.7 cells that were pretreated with 50 μM DPI or 5 mM compound C or 2 μM Torin 1 for 1 h were treated with 4 μg/ml BCA for 2 h. (C) The cells were stained with Sytox orange and observed by fluorescence microscopy. (D) The cells were stained with Sytox green and then the results were obtained with a spectrofluorophotometer. *P < 0.05, **P < 0.01. The gray horizontal line represents 1. (E) Supernatants were collected for CFU quantification and the calculation of the extracellular bacterial killing rate. *P < 0.05. The data are representative of three experiments with similar results.
BCA Enhanced Intra- and Extra-Cellular Bacterial Killing in the Salmonella-Infected Mouse Model in vivo
To assay the effect of enhancement of intra- and extracellular bacterial killing against Salmonella by BCA, we conducted the experiment in a Salmonella-infected mouse model. BCA treatment markedly reduced the intracellular bacteria counts in a dose dependent manner in the peritoneal lavage fluid and reduced the bacterial burden in liver and spleen tissue (p < 0.01, Figure 6A). Treatment with 3-MA (an autophagy inhibitor) reversed the effect of BCA (Figure 6E). Moreover, BCA treatment increased Beclin-1 expression and the LC3-II/LC3-I and Beclin-1/β-actin ratio in peritoneal MΦ, liver, and spleen tissue in a dose dependent manner (Figures 6B–D). Treatment with 3-MA reversed this trend (Figures 6F–H). These data demonstrate that BCA promotes Salmonella clearance via autophagy in vivo. Next, to determine if BCA improves extracellular bacterial killing through enhancing MET formation in vivo, we treated Salmonella infected mice with 100 U DNase I (a reagent proven to degrade extracellular traps) by intraperitoneal injection daily (Von Bruhl et al., 2012). BCA treatment caused an increase of eDNA production in peritoneal lavage fluid ex vivo, and the treatment of DNase I abrogated the increase (Figure 6I) and increased the bacterial burden in peritoneal lavage fluid, liver and spleen tissue (Figure 6J). This demonstrated that BCA promoted extracellular Salmonella clearance via the induction of MET formation in vivo. BCA enhanced bactericidal extra- and intra-cellular activity in a Salmonella infected mouse model in vivo.
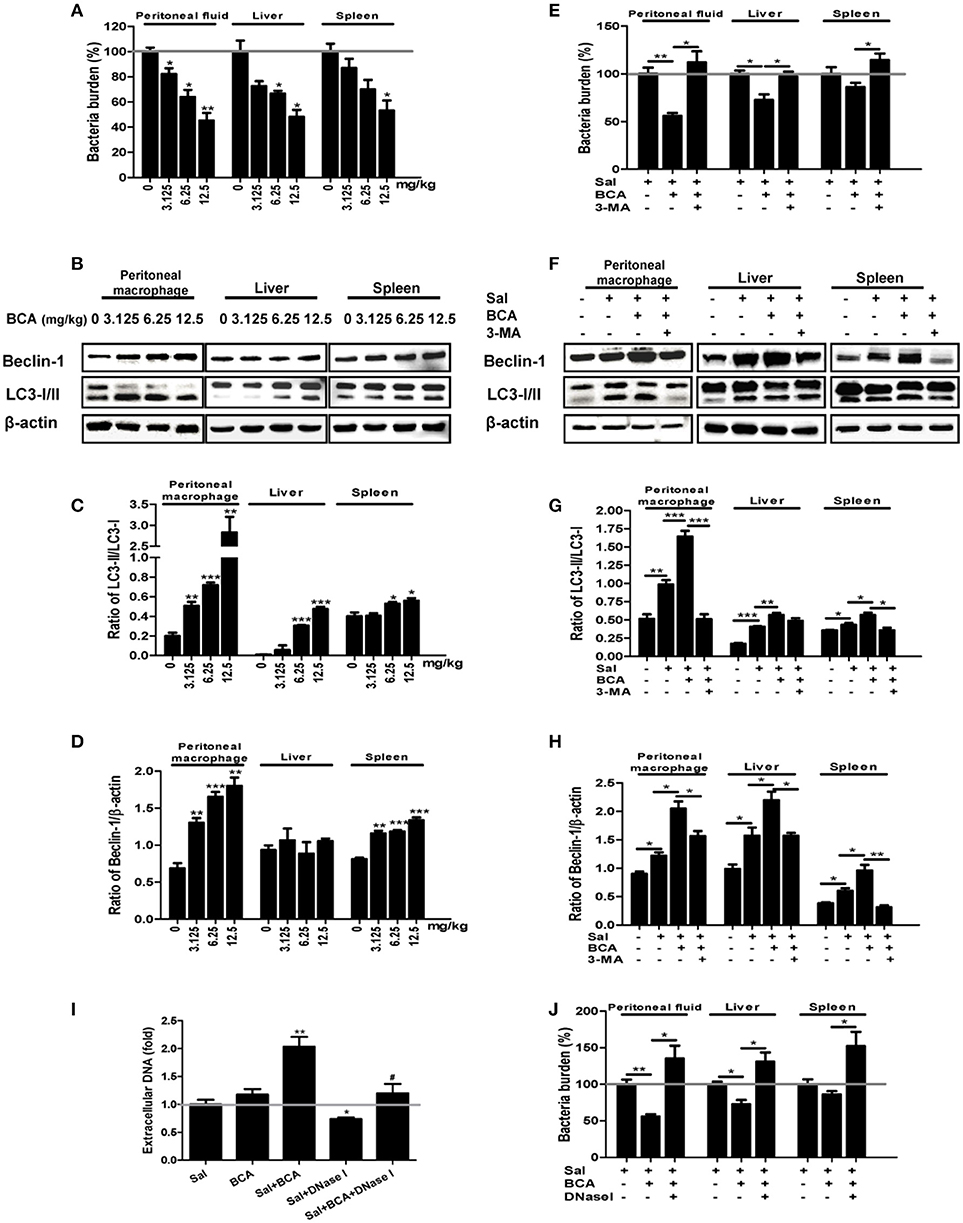
Figure 6. In vivo antibacterial activity of BCA. (A–D) Mice were infected by intragastric administration of an overnight culture of Salmonella (105 bacteria in 0.1 ml PBS) through a gavage tube and then treated with BCA at different doses (3.125–12.5 mg/kg) intragastrically by gavage daily (10 mice for each group). On the 5th day p.i., the mice were sacrificed and the samples were collected. (A) The bacteria burdens in the same amount of peritoneal fluid, liver tissue and spleen tissue were quantified. *P < 0.05, **P < 0.01. (B) Proteins from peritoneal MΦ, liver tissue and spleen tissue were extracted. (A) The expression of LC3, Beclin-1 was detected by western blotting. (C,D) The ratios of LC3-II/LC3-I and the ratio of Beclin-1/β-actin were calculated. *P < 0.05, **P < 0.01, ***P < 0.001, compared with the control group. (E–H) We treated the Salmonella-infected mice with 6.25 mg/kg BCA intragastrically by gavage or BCA intragastrically and 24 mg/kg 3-MA by intraperitoneal injection daily for 4 days. On the fifth day after infection, the mice were sacrificed and samples were collected. (E) The bacterial burdens in the same amount of peritoneal fluid, liver tissue and spleen tissue were quantified. *P < 0.05, **P < 0.01. (F) Proteins were extracted from peritoneal MΦ, liver and spleen tissue. (E) The expression of LC3 and Beclin-1 was detected by western blotting. (G,H) The ratio of LC3-II/LC3-I and the ratio of Beclin-1/β-actin were calculated. *P < 0.05, **P < 0.01, ***P < 0.001. (I,J) Mice were infected by the intragastric administration of an overnight culture of Salmonella (105 bacteria in 0.1 ml PBS) and then treated with 6.25 mg/kg BCA intragastrically by gavage with or without 100 U DNase I treatment by intraperitoneal injection for 4 days. Peritoneal fluid was stained with Sytox green, and the results were obtained subsequently with a spectrofluorophotometer. *P < 0.05, **P < 0.01, compared with the Salmonella-infected group; #P < 0.05, compared with the BCA-treated infected group. The gray horizontal line represents 1. (J) The bacteria burdens in the same amount peritoneal fluid, liver tissue and spleen tissue on the fifth day were quantified. *P < 0.05, **P < 0.01.
BCA Reversed Salmonella SPI-1-Dependent MΦ M2 Polarization in vitro and in vivo
To investigate the effect of BCA on MΦ polarization under the condition of Salmonella-infection, we detected the markers of M1/M2 phenotypes and cytokines of polarization in Raw246.7 cells in vitro. The results showed that the expression levels of M2 MΦ markers CD163 and CD206 increased significantly after infection in Raw246.7 cells, while BCA could reverse this trend partially (Figure 7A). Salmonella-infection increased significantly the secretion levels of M2 MΦ marker IL-10 in Raw246.7 cells. Moreover, BCA treatment could down-regulate significantly the levels of IL-10 in the infected macropahges notably (Figure 7B). However, Salmonella-infection had no effect on M1 MΦ markers CCR7 and CD86 expression and TNF-α secretion (Figures 7A,B). The previous studies has proved that Salmonella induce MΦ polarization to M2 phenotype dependent on SPI-1 gene expression (Kyrova et al., 2012). We tested the SPI-1 gene expression in vitro and in vivo. Our results showed BCA down-regulated the expression of hilA and hilD (SPI-1 major genes) significantly (Figures S2A,C–E) and inhibited secretion of SPI-1-encoded protein SipA, SipB and SipC significantly in a dose-dependent manner (Figure S2B). The results indicated that BCA could reverse the M2 phenotype polarization of the Salmonella-infected MΦ through down-regulation of SPI-1 expression.
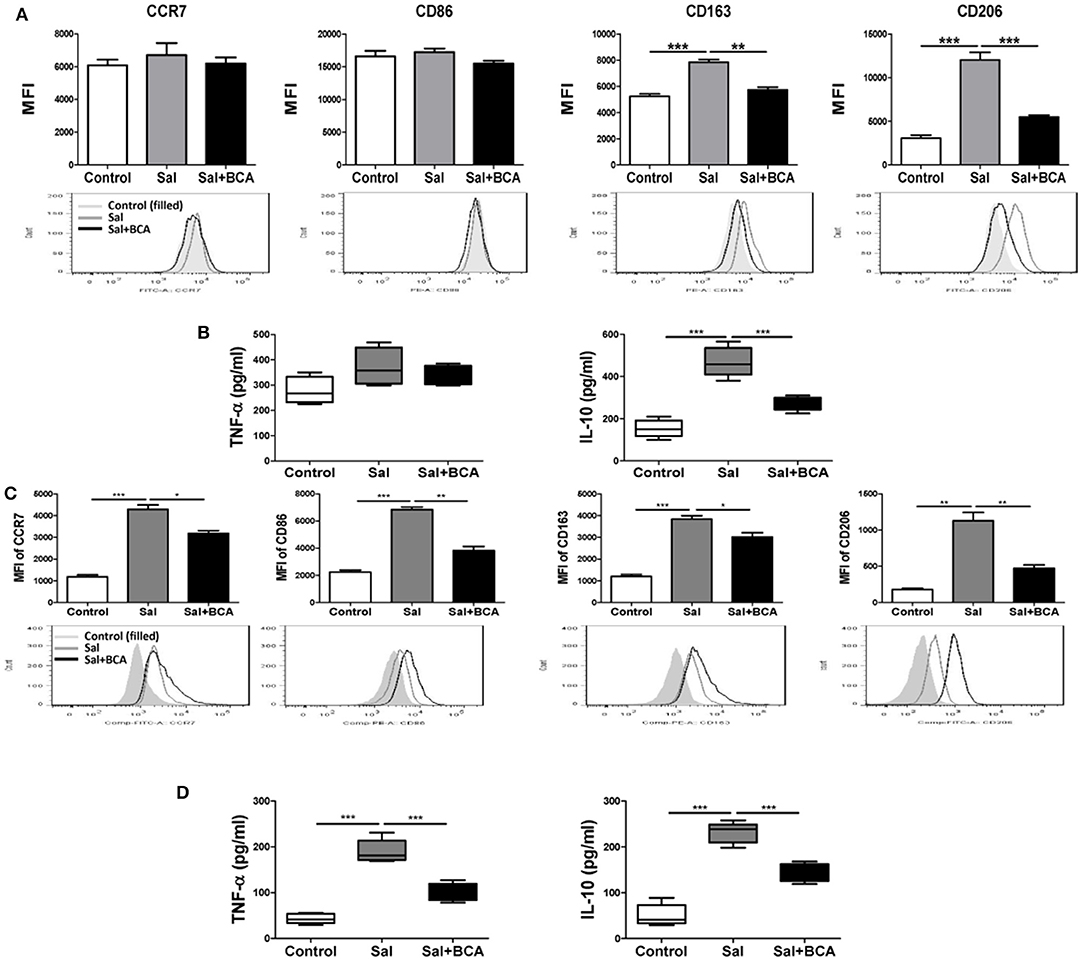
Figure 7. BCA regulate MΦ polarization in vitro and in vivo. (A,B) Raw246.7 cells were treated with 4 μg/ml BCA for 2 h. Then, the cells were infected with Salmonella at MOI = 10:1 for 2 h. (A) The expression of surface markers (CCR7, CD86, CD163, and CD206) was determined by flow cytometry. The results are presented as MFI. (B) TNF-α and IL-10 in the supernatants were detected by ELISA. **P < 0.01, ***P < 0.001. The data are representative of three experiments with similar results. (C,D) Mice were infected by intragastric administration of an overnight culture of Salmonella (105 bacteria in 0.1 ml PBS) through a gavage tube and then treated with 6.25 mg/kg BCA intragastrically by gavage daily (5 mice for each group). On the 5th day p.i., the mice were sacrificed and the peritoneal fluid was collected. (C) The expression of surface markers (CCR7, CD86, CD163, and CD206) in mouse peritoneal MΦs were determined by flow cytometry The results are presented as MFI. (D) TNF-α and IL-10 in ascitic fluid were detected by ELISA. *P < 0.05, **P < 0.01, ***P < 0.001.
To further test the influence of BCA on Salmonella-infected MΦ plarization in vivo, we detected the distinct M1/M2 markers in mouse peritoneal MΦs and the cytokines level in the mouse ascite in the Salmonella-infected mouse model. In mouse peritoneal MΦs, Salmonella infection up-regulated the expression of both of M1 MΦ markers (CCR7 and CD86), M2 MΦ marker (CD163 and CD206) and induced the secretion of M1 MΦ marker TNF-α and M2 MΦ marker IL-10 in ascitic fluid significantly (Figures 7C,D). We found BCA treatment could downregulate the expression of M1 MΦ markers (CCR7 and CD86) and M2 MΦ markers (CD163 and CD206) and decrease the secretion of M1 MΦ marker TNF-α and M2 MΦ marker IL-10 in the Salmonella-infected mouse ascitic fluid significantly (Figures 7C,D). These results indicate that BCA could regulate the bacteria-infected MΦ polarization and could alleviate both inflammatory response and anti-inflammatory response in the case of bacterial infection in vivo.
Discussion
Autophagy has been demonstrated to play an important role in pathogen infection in MΦ and in epithelial cells. Host cells can use autophagy to remove pathogens such as Mycobacterium tuberculosis, Salmonella, and Francisella tularensis (Chiu et al., 2009; Thurston et al., 2009). Some bacteria such as Staphylococcus aureus can escape from killing in host cells with the help of autophagic response (Schnaith et al., 2007). Several agents such as isoniazid, pyrazinamide (Kim et al., 2012), nitazoxanide (Lam et al., 2012), anticonvulsant carbamazepine (Schiebler et al., 2015), and small molecule AR-12 (Chiu et al., 2009) have been suggested to have the ability to stimulate autophagy and inhibit the intracellular proliferation of M. tuberculosis and F. tularensis (Chiu et al., 2009). In present study, we demonstrated that BCA can induce the complete autophagic response in epithelial cells (HeLa) or MΦ (THP-1 and Raw264.7).
Previous reports of autophagic inducers focused only on their effects on LC3 expression and other possible pathways. In this study, for the first time, we found that BCA could, in addition to the induction of autophagy, restrict the proliferation of invading Salmonella by upregulating the expression of the ubiquitinated adapter proteins LRSAM1, NDP52 and p62 and reinforcing the recognition of intracellular bacteria. In addition, BCA treatment increased the colocalization of LAMP1, LysoTracker, LC3 puncta and intracellular bacteria. LAMP1 is a marker of late endosomes/lysosomes that colocalizes with LC3 during autolysosome maturation. LysoTracker is a weak lysosomotropic base that accumulates and fluoresces within acidic vesicles. A gentamicin protection assay verified that BCA improved the killing of intracellular Salmonella through autophagy in HeLa and THP-1 cells. Interestingly, BCA did not show any antibacterial activity in vitro against the Salmonella strain used here when tested at concentrations up to 512 μg/mL. Therefore the concentrations of BCA tested were below MIC, demonstrating that the bactericidal intracellular activity of BCA due to an immunomodulating effect rather than the generalized growth inhibition of bacteria. Thus, BCA acts as an enhancer of the host's immune defense and increases the killing of invading Salmonella by autophagy.
We identified the pathways involved in BCA induced autophagy. BCA treatment increased the phosphorylation of AMPK, and Ulk1 Ser 317 and Ser 757, which are phosphorylation sites of AMPK and mTOR, respectively. BCA treatment decreased the phosphorylation of mTOR, 70S6 and 4E-BP1; these results demonstrated that BCA induces autophagy via the AMPK/ULK1/mTOR pathway. AMPK is believed to activate autophagy by inhibiting mTOR complex-1 (Zoncu et al., 2011).
Although Salmonellae are facultative intracellular bacteria, MacLennan CA et al. have identified that the high fatality rate among young African children is associated with extracellular bacterial growth (Maclennan et al., 2008). Furthermore, it was also indicated that the extracellular killing mechanism was critical to control Salmonella in a murine infection model (Siggins et al., 2011). Salmonella was reported to induce neutrophil ET formation (Brinkmann et al., 2004). Here, for the first time, we showed that Salmonella induced MET formation. To our knowledge, only statins have been demonstrated to be enhancers of bacterial killing by ETs; however, statins inhibit 3-hydroxy 3-methylglutaryl coenzyme A (HMG-CoA) reductase, the rate-limiting enzyme in cholesterol biosynthesis (Chow et al., 2010). This observation suggests that statins may be not suitable for use as enhancers of bacterial killing except in patients that have hypercholesterolemia. Our results showed that BCA enhanced the formation of METs and promotes extracellular Salmonella killing in vitro and in vivo. As a common plant product, BCA is generally considered to be innocuous (Zoncu et al., 2011); our results showed that there was no toxicity at concentrations up to 100 μM in HeLa, RAW 264.7, and THP-1 cell lines, which is consistent with a previous study (Kole et al., 2011).
As the important part of innate immune system and the role of MΦs in immune surveillance and immune regulation, the polarizaiton of MΦs are studied widely. Peripheral monocytes tend to polarize to different subtypes of MΦs according to the tissue microenvironment. The balance of M1/M2 MΦs determines the tissue inflammation and tissue damage (Dey et al., 2014). Different pathogen infections have different effects on the polarization of MΦs and most of them improve MΦs toward M1 phenotype (Khan et al., 2015; Dai et al., 2017; Farias et al., 2017; Hou et al., 2017). Salmonella has been proved to induce MΦ polarization toward M2 phenotype depending on SPI-1 (Kyrova et al., 2012), our results in vitro were similar to the previous reports. We also demonstrated that BCA could inhibit the polarization to M2 MΦ induced by Salmonella infection by down-regulation of SPI-1 genes expression. Noteworthily, we found both expressions of M1 MΦ markers (CCR7 and CD86) and M2 MΦ markers (CD163 and CD206) increased significantly in the peritoneal MΦs of Salmonella-infected mice and both levels of TNF-α and IL-10 increased significantly in the infected mice's ascitic fluid, we speculated that there was an imbalance of MΦ M1/M2 polarization associated with inflammatory conditions of Salmonella infection, this phenomenon was similar to the results of recent report that Salmonella infection could induce both IL-6 (pro-inflammatory) and IL-10 (anti-inflammatory) release in mice (Voinnet, 2011). Thus, our results demonstrated that BCA could deregulate both inflammatory response and anti-inflammatory response in the condition of bacteria infection through regulation of MΦ polarization in vivo.
ROS have been reported to mediate ET formation, such as PMA-induced neutrophil ETs (Davidson et al., 2015). In this study, our data showed that BCA inhibited cytosolic ROS release in a dose- and time-dependent manner, this suggested that BCA induced a ROS-independent MET formation, however, our result proved that it was mediated by AMPK/ULK1/mTOR pathway.
In summary, this study demonstrated BCA induced AMPK/ULK1/mTOR-mediated autophagy and METs, which enhanced the defense against Salmonella infection in vitro and in vivo. In the meantime, BCA inhibits both inflammatory response and anti-inflammatory response when the body was infected by bacteria. These findings provide basic data about the control of infections by the enhancement of the host's immune defenses. The use of BCA may represent a new strategy to overcome the desperate scarcity of new therapeutic approaches.
Author Contributions
XZ: data acquisition, data analysis, data interpretation; XT: revising of the manuscript; NG: data acquisition, data analysis; YA: data acquisition, data analysis, data interpretation, writing of the manuscript; XC: data acquisition; CS: data analysis, data interpretation; CW: data analysis, data interpretation; YL, data analysis, data interpretation; SL: revising of the manuscript; HX: revising of the manuscript; ML: reagents, materials, analysis tools provider; YW: reagents, materials, analysis tools provider, the experiments designer; LY: reagents, materials, analysis tools provider, the experiments designer.
Funding
This work was supported by National Key R&D Program of China (2016YFD0501302); National Natural Science Foundation (81801972); State's Key Project of Research and Development Plan (2017YFD0502200; 2016YFD0501302); the Project of the Education Department of Jilin Province (No. 2016444); Technology and Innovation Commission of Shenzhen Municipality (No. JCYJ2016031100720906, JSGG20160301100442775, GXZZ20140421112021913).
Conflict of Interest Statement
The authors declare that the research was conducted in the absence of any commercial or financial relationships that could be construed as a potential conflict of interest.
Supplementary Material
The Supplementary Material for this article can be found online at: https://www.frontiersin.org/articles/10.3389/fcimb.2018.00318/full#supplementary-material
References
Ankomah, P., and Levin, B. R. (2014). Exploring the collaboration between antibiotics and the immune response in the treatment of acute, self-limiting infections. Proc. Natl. Acad. Sci. U.S.A. 111, 8331–8338. doi: 10.1073/pnas.1400352111
Arroyo, D. S., Soria, J. A., Gaviglio, E. A., Garcia-Keller, C., Cancela, L. M., Rodriguez-Galan, M. C., et al. (2013). Toll-like receptor 2 ligands promote microglial cell death by inducing autophagy. FASEB J. 27, 299–312. doi: 10.1096/fj.12-214312
Brinkmann, V., Reichard, U., Goosmann, C., Fauler, B., Uhlemann, Y., Weiss, D. S., et al. (2004). Neutrophil extracellular traps kill bacteria. Science 303, 1532–1535. doi: 10.1126/science.1092385
Cemma, M., Kim, P. K., and Brumell, J. H. (2011). The ubiquitin-binding adaptor proteins p62/SQSTM1 and NDP52 are recruited independently to bacteria-associated microdomains to target Salmonella to the autophagy pathway. Autophagy 7, 341–345. doi: 10.4161/auto.7.3.14046
Chiu, H. C., Soni, S., Kulp, S. K., Curry, H., Wang, D., Gunn, J. S., et al. (2009). Eradication of intracellular Francisella tularensis in THP-1 human macrophages with a novel autophagy inducing agent. J. Biomed. Sci. 16:110. doi: 10.1186/1423-0127-16-110
Chow, O. A., Von Köckritz-Blickwede, M., Bright, A. T., Hensler, M. E., Zinkernagel, A. S., Cogen, A. L., et al. (2010). Statins enhance formation of phagocyte extracellular traps. Cell Host Microbe 8, 445–454. doi: 10.1016/j.chom.2010.10.005
Conway, K. L., Kuballa, P., Song, J. H., Patel, K. K., Castoreno, A. B., Yilmaz, O. H., et al. (2013). Atg16l1 is required for autophagy in intestinal epithelial cells and protection of mice from Salmonella infection. Gastroenterology 145, 1347–1357. doi: 10.1053/j.gastro.2013.08.035
Croswell, A., Amir, E., Teggatz, P., Barman, M., and Salzman, N. H. (2009). Prolonged impact of antibiotics on intestinal microbial ecology and susceptibility to enteric Salmonella infection. Infect. Immun. 77, 2741–2753. doi: 10.1128/IAI.00006-09
Dai, X., Mao, C., Lan, X., Chen, H., Li, M., Bai, J., et al. (2017). Acute Penicillium marneffei infection stimulates host M1/M2a macrophages polarization in BALB/C mice. BMC Microbiol. 17:177. doi: 10.1186/s12866-017-1086-3
Davidson, A. C., Humphreys, D., Brooks, A. B., Hume, P. J., and Koronakis, V. (2015). The Arf GTPase-activating protein family is exploited by Salmonella enterica serovar Typhimurium to invade nonphagocytic host cells. MBio 6:e02253–14. doi: 10.1128/mBio.02253-14
Dey, A., Allen, J., and Hankey-Giblin, P. A. (2014). Ontogeny and polarization of macrophages in inflammation: blood monocytes versus tissue macrophages. Front. Immunol. 5:683. doi: 10.3389/fimmu.2014.00683
Douda, D. N., Khan, M. A., Grasemann, H., and Palaniyar, N. (2015). SK3 channel and mitochondrial ROS mediate NADPH oxidase-independent NETosis induced by calcium influx. Proc. Natl. Acad. Sci. U.S.A. 112, 2817–2822. doi: 10.1073/pnas.1414055112
Erbel, C., Rupp, G., Helmes, C. M., Tyka, M., Linden, F., Doesch, A. O., et al. (2013). An in vitro model to study heterogeneity of human macrophage differentiation and polarization. J. Vis. Exp. 76:e50332. doi: 10.3791/50332
Farias, L. H. S., Rodrigues, A. P. D., Coêlho, E. C., Santos, M. F., Sampaio, S. C., and Silva, E. O. (2017). Crotoxin stimulates an M1 activation profile in murine macrophages during Leishmania amazonensis infection. Parasitology 144, 1458–1467. doi: 10.1017/S0031182017000944
Gharib, S. A., Johnston, L. K., Huizar, I., Birkland, T. P., Hanson, J., Wang, Y., et al. (2014). MMP28 promotes macrophage polarization toward M2 cells and augments pulmonary fibrosis. J. Leukoc. Biol. 95, 9–18. doi: 10.1189/jlb.1112587
Hou, Y., Yu, H., Liu, X., Li, G., Pan, J., Zheng, C., et al. (2017). Gingipain of Porphyromonas gingivalis manipulates M1 macrophage polarization through C5a pathway. In Vitro Cell. Dev. Biol. Anim. 53, 593–603. doi: 10.1007/s11626-017-0164-z
Khan, J., Sharma, P. K., and Mukhopadhaya, A. (2015). Vibrio cholerae porin OmpU mediates M1-polarization of macrophages/monocytes via TLR1/TLR2 activation. Immunobiology 220, 1199–1209. doi: 10.1016/j.imbio.2015.06.009
Kim, J. J., Lee, H. M., Shin, D. M., Kim, W., Yuk, J. M., Jin, H. S., et al. (2012). Host cell autophagy activated by antibiotics is required for their effective antimycobacterial drug action. Cell Host Microbe 11, 457–468. doi: 10.1016/j.chom.2012.03.008
Klionsky, D. J., Abeliovich, H., Agostinis, P., Agrawal, D. K., Aliev, G., Askew, D. S., et al. (2008). Guidelines for the use and interpretation of assays for monitoring autophagy in higher eukaryotes. Autophagy 4, 151–175. doi: 10.4161/auto.5338
Kole, L., Giri, B., Manna, S. K., Pal, B., and Ghosh, S. (2011). Biochanin-A, an isoflavon, showed anti-proliferative and anti-inflammatory activities through the inhibition of iNOS expression, p38-MAPK and ATF-2 phosphorylation and blocking NFkappaB nuclear translocation. Eur. J. Pharmacol. 653, 8–15. doi: 10.1016/j.ejphar.2010.11.026
Kyrova, K., Stepanova, H., Rychlik, I., Faldyna, M., and Volf, J. (2012). SPI-1 encoded genes of Salmonella Typhimurium influence differential polarization of porcine alveolar macrophages in vitro. BMC Vet. Res. 8:115. doi: 10.1186/1746-6148-8-115
Lam, K. K., Zheng, X., Forestieri, R., Balgi, A. D., Nodwell, M., Vollett, S., et al. (2012). Nitazoxanide stimulates autophagy and inhibits mTORC1 signaling and intracellular proliferation of Mycobacterium tuberculosis. PLoS Pathog. 8:e1002691. doi: 10.1371/journal.ppat.1002691
Liu, G., Liang, J. C., Wang, X. L., Li, Z. H., Wang, W., Guo, N., et al. (2011). In Vitro Synergy of Biochanin, A., and Ciprofloxacin against clinical isolates of Staphylococcus aureus. Molecules 16, 6656–6666. doi: 10.3390/molecules16086656
Maclennan, C. A., Gondwe, E. N., Msefula, C. L., Kingsley, R. A., Thomson, N. R., White, S. A., et al. (2008). The neglected role of antibody in protection against bacteremia caused by nontyphoidal strains of Salmonella in African children. J. Clin. Invest. 118, 1553–1562. doi: 10.1172/JCI33998
Medjakovic, S., and Jungbauer, A. (2008). Red clover isoflavones biochanin A and formononetin are potent ligands of the human aryl hydrocarbon receptor. J. Steroid Biochem. Mol. Biol. 108, 171–177. doi: 10.1016/j.jsbmb.2007.10.001
Medzhitov, R. (2010). Inflammation 2010: new adventures of an old flame. Cell 140, 771–776. doi: 10.1016/j.cell.2010.03.006
Mizusaki, H., Takaya, A., Yamamoto, T., and Aizawa, S. (2008). Signal pathway in salt-activated expression of the Salmonella pathogenicity island 1 type III secretion system in Salmonella enterica serovar Typhimurium. J. Bacteriol. 190, 4624–4631. doi: 10.1128/JB.01957-07
Park, C. W., Hong, S. M., Kim, E. S., Kwon, J. H., Kim, K. T., Nam, H. G., et al. (2013). BNIP3 is degraded by ULK1-dependent autophagy via MTORC1 and AMPK. Autophagy 9, 345–360. doi: 10.4161/auto.23072
Remijsen, Q., Vanden Berghe, T., Wirawan, E., Asselbergh, B., Parthoens, E., De Rycke, R., et al. (2011). Neutrophil extracellular trap cell death requires both autophagy and superoxide generation. Cell Res. 21, 290–304. doi: 10.1038/cr.2010.150
Schiebler, M., Brown, K., Hegyi, K., Newton, S. M., Renna, M., Hepburn, L., et al. (2015). Functional drug screening reveals anticonvulsants as enhancers of mTOR-independent autophagic killing of Mycobacterium tuberculosis through inositol depletion. EMBO Mol. Med. 7, 127–139. doi: 10.15252/emmm.201404137
Schnaith, A., Kashkar, H., Leggio, S. A., Addicks, K., Krönke, M., and Krut, O. (2007). Staphylococcus aureus subvert autophagy for induction of caspase-independent host cell death. J. Biol. Chem. 282, 2695–2706. doi: 10.1074/jbc.M609784200
Shang, L., and Wang, X. (2011). AMPK and mTOR coordinate the regulation of Ulk1 and mammalian autophagy initiation. Autophagy 7, 924–926. doi: 10.4161/auto.7.8.15860
Shen, F., Tang, X., Cheng, W., Wang, Y., Wang, C., Shi, X., et al. (2016). Fosfomycin enhances phagocyte-mediated killing of Staphylococcus aureus by extracellular traps and reactive oxygen species. Sci. Rep. 6:19262. doi: 10.1038/srep19262
Siggins, M. K., Cunningham, A. F., Marshall, J. L., Chamberlain, J. L., Henderson, I. R., and Maclennan, C. A. (2011). Absent bactericidal activity of mouse serum against invasive African nontyphoidal Salmonella results from impaired complement function but not a lack of antibody. J. Immunol. 186, 2365–2371. doi: 10.4049/jimmunol.1000284
Sklenickova, O., Flesar, J., Kokoska, L., Vlkova, E., Halamova, K., and Malik, J. (2010). Selective growth inhibitory effect of biochanin A against intestinal tract colonizing bacteria. Molecules 15, 1270–1279. doi: 10.3390/molecules15031270
Sun, Y., Yu, S., Ding, N., Meng, C., Meng, S., Zhang, S., et al. (2014). Autophagy benefits the replication of Newcastle disease virus in chicken cells and tissues. J. Virol. 88, 525–537. doi: 10.1128/JVI.01849-13
Thurston, T. L., Ryzhakov, G., Bloor, S., von Muhlinen, N., and Randow, F. (2009). The TBK1 adaptor and autophagy receptor NDP52 restricts the proliferation of ubiquitin-coated bacteria. Nat. Immunol. 10, 1215–1221. doi: 10.1038/ni.1800
Viveiros, M., Martins, M., Rodrigues, L., Machado, D., Couto, I., Ainsa, J., et al. (2012). Inhibitors of mycobacterial efflux pumps as potential boosters for anti-tubercular drugs. Expert Rev. Anti Infect. Ther. 10, 983–998. doi: 10.1586/eri.12.89
Voinnet, O. (2011). Micro-balancing innate immunity to Salmonella. EMBO J. 30, 1877–1879. doi: 10.1038/emboj.2011.134
von Brühl, M. L., Stark, K., Steinhart, A., Chandraratne, S., Konrad, I., Lorenz, M., et al. (2012). Monocytes, neutrophils, and platelets cooperate to initiate and propagate venous thrombosis in mice in vivo. J. Exp. Med. 209, 819–835. doi: 10.1084/jem.20112322
Wang, Y., Li, Y., Li, H., Song, H., Zhai, N., Lou, L., et al. (2017). Brucella dysregulates monocytes and inhibits macrophage polarization through LC3-dependent autophagy. Front. Immunol. 8:691. doi: 10.3389/fimmu.2017.00691
Wang, Y., Zhang, K., Shi, X., Wang, C., Wang, F., Fan, J., et al. (2016). Critical role of bacterial isochorismatase in the autophagic process induced by Acinetobacter baumannii in mammalian cells. FASEB J. 30, 3563–3577. doi: 10.1096/fj.201500019R
Wiedemann, A., Rosselin, M., Mijouin, L., Bottreau, E., and Velge, P. (2012). Involvement of c-Src tyrosine kinase upstream of class I phosphatidylinositol (PI) 3-kinases in Salmonella Enteritidis Rck protein-mediated invasion. J. Biol. Chem. 287, 31148–31154. doi: 10.1074/jbc.M112.392134
Yipp, B. G., Petri, B., Salina, D., Jenne, C. N., Scott, B. N., Zbytnuik, L. D., et al. (2012). Infection-induced NETosis is a dynamic process involving neutrophil multitasking in vivo. Nat. Med. 18, 1386–1393. doi: 10.1038/nm.2847
Yuan, K., Huang, C., Fox, J., Laturnus, D., Carlson, E., Zhang, B., et al. (2012). Autophagy plays an essential role in the clearance of Pseudomonas aeruginosa by alveolar macrophages. J. Cell Sci. 125, 507–515. doi: 10.1242/jcs.094573
Keywords: reactive oxygen species, autophagy, extracellular traps, polarization, Salmonella
Citation: Zhao X, Tang X, Guo N, An Y, Chen X, Shi C, Wang C, Li Y, Li S, Xu H, Liu M, Wang Y and Yu L (2018) Biochanin a Enhances the Defense Against Salmonella enterica Infection Through AMPK/ULK1/mTOR-Mediated Autophagy and Extracellular Traps and Reversing SPI-1-Dependent Macrophage (MΦ) M2 Polarization. Front. Cell. Infect. Microbiol. 8:318. doi: 10.3389/fcimb.2018.00318
Received: 31 January 2018; Accepted: 21 August 2018;
Published: 11 September 2018.
Edited by:
Igor Brodsky, University of Pennsylvania, United StatesReviewed by:
Vishvanath Tiwari, Central University of Rajasthan, IndiaTravis Bourret, Creighton University, United States
Copyright © 2018 Zhao, Tang, Guo, An, Chen, Shi, Wang, Li, Li, Xu, Liu, Wang and Yu. This is an open-access article distributed under the terms of the Creative Commons Attribution License (CC BY). The use, distribution or reproduction in other forums is permitted, provided the original author(s) and the copyright owner(s) are credited and that the original publication in this journal is cited, in accordance with accepted academic practice. No use, distribution or reproduction is permitted which does not comply with these terms.
*Correspondence: Yang Wang, wyang@jlu.edu.cn
Lu Yu, yu_lu@jlu.edu.cn
† These authors have contributed equally to this work