- Université Paris Cité, CNRS, INSERM, Unité de Biologie Fonctionnelle et Adaptative, Paris, France
Because of its scale and suddenness, the SARS-CoV-2 pandemic has created an unprecedented challenge in terms of drug development. Apart from being natural candidates for vaccine design, peptides are a class of compounds well suited to target protein-protein interactions, and peptide drug development benefits from the progress of in silico protocols that have emerged within the last decade. Here, we review the different strategies that have been considered for the development of peptide drugs against SARS-CoV-2. Thanks to progress in experimental structure determination, structural information has rapidly become available for most of the proteins encoded by the virus, easing in silico analyses to develop drugs or vaccines. The repurposing of antiviral/antibacterial peptide drugs has not been successful so far. The most promising results, but not the only ones, have been obtained targeting the interaction between SARS-CoV-2 spike protein and the Angiotensin-Converting Enzyme 2, which triggers cellular infection by the virus and its replication. Within months, structure-based peptide design has identified competing for picomolar candidates for the interaction, proving that the development of peptide drugs targeting protein-protein interactions is maturing. Although no drug specifically designed against SARS-CoV-2 has yet reached the market, lessons from peptide drug development against SARS-CoV-2 suggest that peptide development is now a plausible alternative to small compounds.
1 Introduction
The severe acute respiratory syndrome coronavirus 2 (SARS-CoV-2) is responsible for COVID-19 (coronavirus disease 2019) and spread rapidly following its emergence in Wuhan in December 2019. While the majority of COVID-19 infections are relatively mild, with most patients recovering in 2–3 weeks, a significant number of patients can develop a severe respiratory illness. Due to the instability of its genome, numerous mutations have appeared in the SARS-CoV-2. Some of them have induced traits considered beneficial for viral adaptation (Pachetti et al., 2020), such as an increase of its transmissibility, an enhancement of its ability to evade natural immunity or a decreasing susceptibility to neutralizing antibodies. Currently, the World Health Organization (WHO) has declared five variants of concern: Alpha, Beta, Delta, Gamma, and Omicron variants (Karim and Karim, 2021; Starr et al., 2021; Tegally et al., 2021; Xie et al., 2021), but the list keeps growing.
Since the end of 2020, several COVID-19 vaccines are available, enabling the reduction of the spread, severity, and death caused worldwide. A monoclonal antibody (sotrovimab) and two combinations of two monoclonal antibodies (casirivimab/imdevimab and bamlanivimab/etesevimab) have been approved as drugs. However, their manufacturing costs are high and they are not convenient for patients since they are administered by intravenous injection. Currently, four non-biologic drugs have been approved. In October 2020, Remdesivir was the first small molecule approved by Food and Drug Administration (FDA). It is a broad-spectrum antiviral medication, which is administrated by intravenous injection. Remdesivir can be also administrated in combination with baricitinib, a drug approved for the treatment of rheumatoid arthritis. Since July 2021, the FDA authorized the use of baricitinib without remdesivir for patients requiring supplemental oxygen. Interestingly, in March 2022, the RECOVERY (Randomised Evaluation of COVID-19 Therapy) trial showed that baricitinib alone is able to reduce mortality by about 20 percent. In December 2021, Molnupiravir has been approved by the FDA for certain patients for whom other treatments are not possible. Molnupiravir is an anti-viral drug, which acts by preventing RNA virus replication. Since the end of December 2021, a fourth drug, Paxlovid, has been available for people who are at high risk of having severe COVID-19. Paxlovid contains the antiviral medications nirmatrelvir and ritonavir. Baricitinib, Molnupiravir, and Paxlovis are administrated orally to the patient.
Although vaccines and the four available drugs are great therapeutic advances to cure COVID-19, it is still necessary to develop new treatments, which are more convenient or less limiting for the patients (Fenton and Keam, 2022). Even if the small molecules are usually more easily manufactured, more stable, and less costly than biologics (Makurvet, 2021), it is important not to just focus on small molecules to design new drugs. In particular, peptide-based drugs have significant advantages such as low toxicity and better specificity.
Peptides have in recent years gained increased interest as candidate therapeutics, with presently over 80 peptide drugs on the market, more than 150 peptides in clinical development, and over 400 undergoing preclinical studies (Muttenthaler et al., 2021). Peptides are easy to develop both in terms of time and technology and are cost-effective, which makes them good candidates for the development of hits or probes up to the proof of concept. Compared to small compounds, despite they have the advantage of low intrinsic toxicity and better specificity, the main limitations of peptides have long been the mode of delivery, mostly parenteral, and the renal clearance that reduces quickly the bioavailability of the peptide drugs (Muttenthaler et al., 2021). However, recent developments have led to progress on those limitations: new modes of formulation and protection, to cite some, have resulted in improved bioavailability, biostability, and biodelivery. Some peptide drugs on the market such as the Semaglutide or plecanatide, can be administered orally (Zhang & Chen, 2021; Lewis et al., 2022). In addition, progress in cell-penetrating peptides now makes it likely to design peptides tissue, cell, or organelle-specific (Xu et al., 2019), enlarging the landscape of possible applications of peptides targeting PPIs to almost any kind of interaction. Apart from synthetic hormones (Vlieghe et al., 2010) and peptides targeting GPCRs (Davenport et al., 2020), peptides are especially well suited to target protein-protein interactions (Nevola and Giralt, 2015; Wójcik and Berlicki, 2016; Bruzzoni-Giovanelli et al., 2018). Compared to small compounds, peptides have higher molecular weights and can bind to a larger surface, which usually results in binding targets with higher specificity and affinity.
The development of peptide drugs also benefits from the progress of structural biology and structural bioinformatics. SARS-CoV-2 illustrates very well the reactivity of structural biology. Thanks to X-ray diffraction and electron microscopy, many SARS-CoV-2 protein structures have been available as soon as 2020, and most structures have been available not later than mid-2021, with some exceptions, though, as illustrated from the compendium available at RCSB or EBI (https://www.rcsb.org/news/feature/5e74d55d2d410731e9944f52; https://www.ebi.ac.uk/pdbe/covid-19). Furthermore, the structure of some protein complexes contributing to viral infection has also been solved, particularly that of the SARS-CoV-2 Spike protein receptor-binding domain (RBD) in interaction with the Angiotensin-Converting Enzyme 2 (ACE2) [PDB: 6M0J (Wang et al., 2020)]. Although not all molecular mechanisms related to SARS-CoV-2 viral infection are fully understood, this structural information has revealed precious to propose peptide candidates combating the viral attack. Concomitantly, the progress of in silico approaches for the prediction of peptide structure, protein-peptide, and protein-protein interactions, as well as progress in conformational sampling, including molecular dynamics (MD) simulations and docking can in principle make in silico protocols starting from the structure of the targets effective to identify peptide candidates.
Here, we review the various results obtained so far that exploit in silico protocols for peptide development in the context of the SARS-CoV-2 pandemic. We describe shortly efforts to identify immunogenic peptides from the available structures as well as efforts to identify candidate peptide drugs targeting viral infection, and we discuss their expected impact on the road to SARS-CoV-2 drug development.
2 The Design of Vaccine Based on Synthetic Peptides
T cells have a crucial role in the immune response to viral infections like COVID-19. The presentation of short viral peptides by the human leukocyte antigen (HLA) complex is the first step in the development of T-cell immunity. Thus, the viral peptides presented by HLA class I molecules and HLA class II molecules can activate CD8 T cells and CD4 T cells, respectively. Once activated, CD8 T cells can recognize and kill virus-infected cells. Activated CD4 T cells act by the release of cytokines, which stimulate other immune cells to trigger the appropriate immune response.
At the beginning of 2020, understanding how the immune system reacts to the SARS-CoV-2 infection is critical to the development of vaccines. In this aim, the T cell memory in 42 patients who recovered from SARS-CoV-2 infection and 16 unexposed donors has been studied by experimental assays using peptides spanning SARS-CoV-2 (Peng et al., 2020). 41 peptides containing CD4+ and/or CD8+ epitopes have been identified. Among these peptides, three peptides deriving from spike protein, two from membrane protein, and one from nucleocapsid protein were frequently targeted by T cells, suggesting that they can trigger an immune response.
A systematic vaccine-informatics approach has been applied to the spike protein to identify antigenic peptides, which could be used to design a novel vaccine candidate (Alam et al., 2021). Based on the spike protein sequence, they applied several bioinformatics tools to highlight potential immunogenic peptides and assess their autoimmune, allergic, and toxic response. Thus, 12 antigenic peptides have been identified. They have 80%–90% identity with experimentally identified epitopes of SARS-CoV. They are predicted as nontoxic, nonallergenic, and highly antigenic. Moreover, the authors performed docking computations of eight peptides on the surface of the HLA to understand how the peptides interact with HLA. Although the authors are confident in the ability of these peptides to trigger an effective immune response against the SARS-CoV-2, no experimental confirmation supports their conclusions.
Recently, a peptide vaccine candidate, named CoVac-1, completed a phase I clinical trial (Heitmann et al., 2022). CoVac-1 is a peptide composed of multiple SARS-CoV-2 epitopes derived from various viral proteins (Figure 1), such as spike, nucleocapsid, membrane, envelope, and open reading frame 8. It contains a synthetic toll-like receptor 1/2 ligand, which acts as an adjuvant to help the vaccine produce a better immune response. From 28 November 2020 to 1 April 2021, 36 healthy adults were enrolled and received one dose of CoVac-1. The participants have been followed up until 56 days after the CoVac-1 injection. 56 adverse effects have been observed, but they were predominately mild (headache, fatigue, nausea …) indicating that CoVac-1 has a favorable safety profile. Moreover, T cell responses were persistent 3 months following vaccination and were not affected by mutation of Alpha and Beta variants. CoVac-1 is currently in phase II clinical trial.
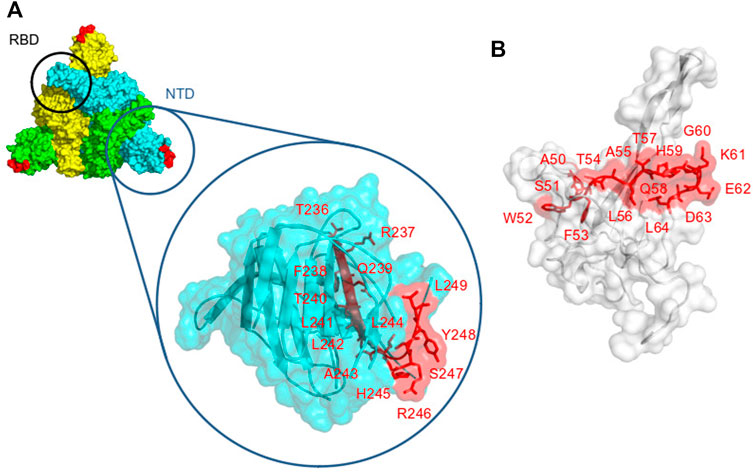
FIGURE 1. Localization of the epitopes that are contained in CoVac-1 on the viral protein’s surfaces. (A) spike protein surface [PDB id: 6XLU (Zhou et al., 2020)], spike monomers are colored in green, yellow, and cyan, respectively. The Receptor Binding Domain (RBD) and the N Terminal Domain (NTD) are indicated. The epitope (T236RFQTLLALHRSYL249) is in red. (B) nucleocapsid protein surface in white (PDB id: 7CR5 (Kang et al., 2021)), the epitope (A50SWFTALTQHGKEDL64) is displayed in red.
3 The Search for Drugs Combating Severe Acute Respiratory Syndrome Coronavirus 2 Attack
3.1 Structure Based Peptide Design
Figure 2 summarizes the different strategies that have been explored to block SARS-CoV-2 proliferation. The main strategies are to prevent virus entry into the cell and to block its replication. We discuss these in the next section. A summary of the identified peptides, the methods to identify them, and their experimental properties if measured, is provided Table 1.
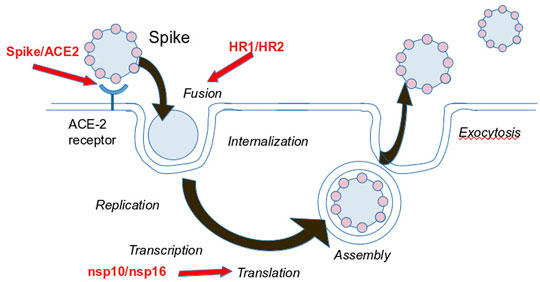
FIGURE 2. Foreseen strategies to block SARS-CoV-2 infection. Red Arrows indicate the targets contributing to the reproduction cycle considered for peptide development.
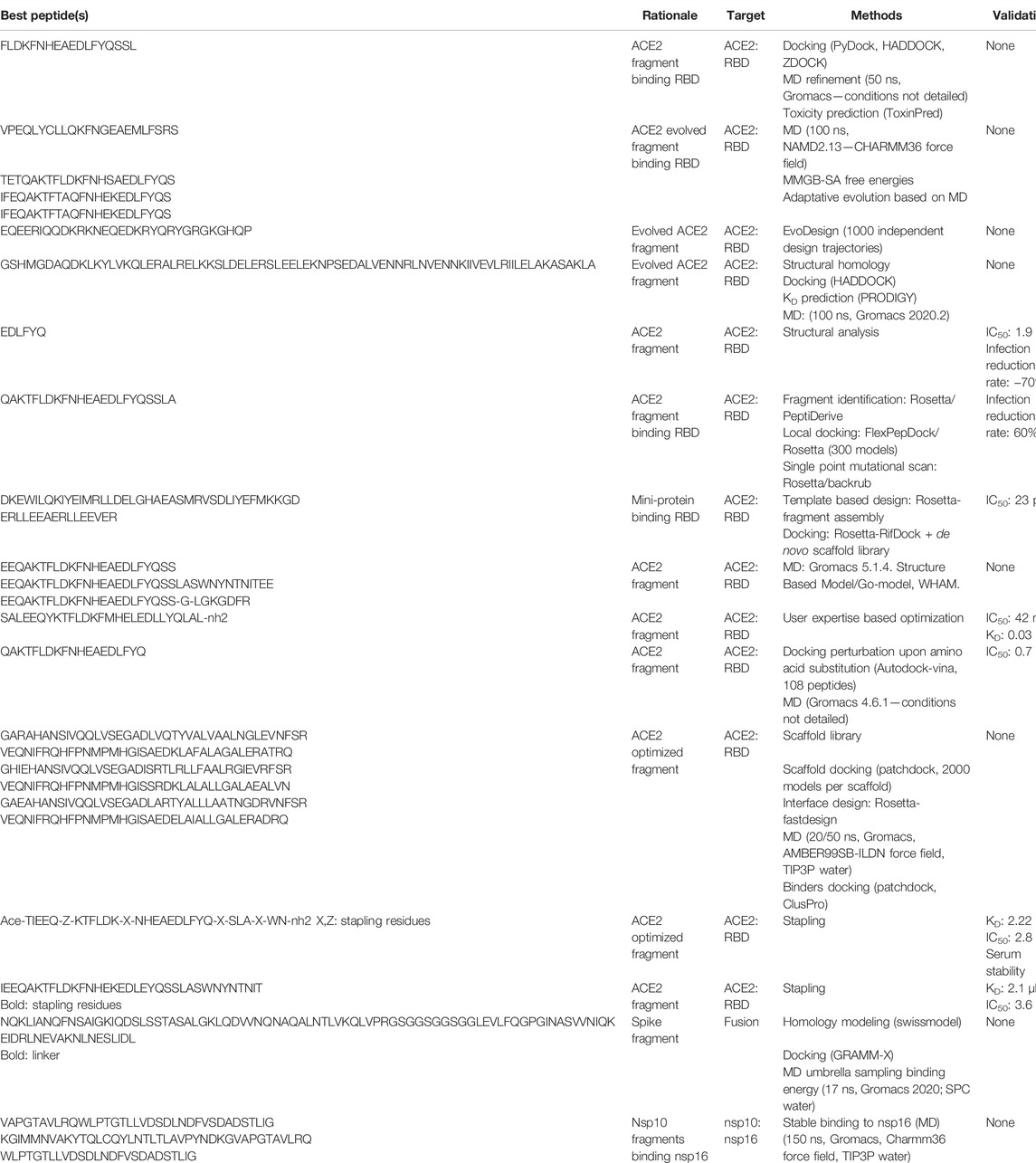
TABLE 1. Candidate peptides. For each, we report its sequence, the rationale underlying its development, the target, a brief summary of the methods employed, the status about its experimental validation (- means none) and the reference related to the peptide identification.
3.1.1 Preventing Virus Internalization by Inhibiting the Spike-Angiotensin-Converting Enzyme 2 Interaction
SARS-CoV-22 enters cells through the interaction of the spike glycoprotein with the ACE2 human receptor (Gheblawi et al., 2020; Papageorgiou and Mohsin, 2020), making the spike/ACE2 interaction a preferential target to prevent cell infection. A structure obtained by electron microscopy at 2.9 Å resolution has been reported at the beginning of 2020 (Yan et al., 2020). Figure 3A shows that on the ACE2 side, the interaction involves mostly the N terminal helix—α1-helix (residues 24–53, sequence: QAKTFLDKFNHEAEDLFYQSSLASWNYNTN), and to a lesser extent residues 79–83 (sequence: LAQMY) and 353–357 (sequence: KGDFR). On the spike protein side, contacts with residues from the RBD, involve mostly the stretch encompassing residues 480–501, and to a lesser extent the stretch encompassing residues 448–454 (Figure 3B). More precisely Barh et al. (2020), have suggested that effective peptides must bind to key positions of the RBD (G485, F486, N487, Q493, and Q498, T500, N501) and that F486, Q493, and N501 are critical residues.
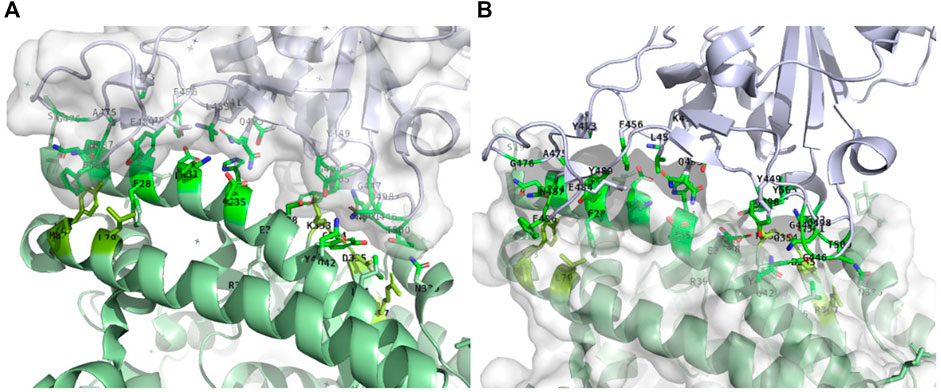
FIGURE 3. Receptor binding domain of spike (within surface) in interaction with the ACE2 receptor (green). Only the side chains of the residues at the interface are displayed. (A): ACE2-receptor (ACE2) residues interacting with the Receptor Binding Domain (RBD) of spike. The bright green residues correspond to the α1-helix (residues 24–53), pale green residues below the α1-helix to residues 79–83 and pale green residues in the back to residues 353–357. (B): Residues of the RBD interacting with ACE2. PDB structure: 6m0j, image generated using PyMOL. Identification of the residues at the ACE2/RBD interface was performed using a distance cut-off of 5 Å.
The dominant strategy has been to design peptides directly inspired by the ACE2 peptidase, binding the RBD and thus competing with the interaction of the RBD with ACE2, preventing downstream cell penetration.
Predominantly, special attention has been brought to the 30 amino acid long α1-helix critical for the RBD-ACE2 binding. From the analysis of the structures of SARS-CoV-2 and SARS-CoV in interaction with ACE2, Larue et al. (2020) have identified two ACE2-derived peptides able to bind Spike RBD in affinity precipitation assays (Larue et al., 2020). Those peptides have shown the ability to inhibit Spike-mediated infection with IC50 values in the low millimolar range. Starting from the fragment between the residues 21 and 45 of the α1-helix, Sitthiyotha and Chunsrivirot (2020) have used computational protein design and molecular dynamics (MD) simulations to design peptides with enhanced theoretical affinity for SARS-CoV-2 RBD (Sitthiyotha and Chunsrivirot, 2020). During this iterative process, the design focus was on positions not reported to form favorable interactions with SARS-CoV-2-RBD, thus avoiding perturbing those corresponding to existing favorable interactions. Finally, Karoyan et al. (2021) have started with a peptide mimicking the α1-helix of hACE2, to end with the best peptide-mimics able to block SARS-CoV-2 human pulmonary cell infection with an inhibitory concentration (IC50) in the nanomolar range upon binding to the virus spike protein (Karoyan et al., 2021).
Such studies were based on the implicit hypothesis that the ACE2 fragments would adopt a conformation similar to that observed in the structure of ACE2 and that the binding of the peptide alone would result in a pose similar to that observed in the complex structure. Several early docking experiments have supported this hypothesis. Jaiswal and Kumar (2020) have for instance reported that the fragments of the α1-helix are found to bind at the α1-helix/RBD interface (Jaiswal and Kumar, 2020). In addition, Baig et al. (2020) have reported that some peptides they designed starting from 23 amino acids of the N-terminal helix, using alanine scanning to identify critical binding positions, can maintain their secondary structure during MD simulations and provide a highly specific and stable binding to SARS-CoV-2 (Baig et al., 2020). However, things might not be so straightforward in terms of structural behavior. Freitas et al. (2021) have also focused on the α1-helix and explored the binding and folding dynamics of the natural and designed ACE2-based peptides by MD simulations using coarse-grained representations (Freitas et al., 2021). Their results show a difference in the folding mechanisms of the modified peptides (a two-state folding mechanism) binding the RBD, as opposed to the naturally occurring α1 helix peptides, suggesting that amino acid substitution on the alpha-helical sequences can result in subtle changes in dynamic properties compared to the wild-type sequence. Moreover, Kuznetsov et al. (2022) have observed experimentally that a peptide comprising positions 24 to 42 of the ACE2 α1 helix can inhibit the formation of the S1-ACE2 complex in a manner dependent on the peptide concentration (Kuznetsov et al. 2022). They also observed the formation of a ternary complex suggesting that the peptide could bind to other sites besides that observed in the structure of the complex. The consequences of this observation on design are so far unknown. In summary, although effective, the peptides derived from the α1-helix, validated in vitro and in cellulo by the different groups could exert a functional effect through mechanisms that are not necessarily those expected.
Attempts to combine different fragments of ACE2 distant in the sequence have also been considered. Barh et al. (2020) have for instance considered the residues of ACE2 interacting with RBD, searched databases of known activity for peptides blocking nCoV-RBD, and proposed chimeric peptides combining several candidates. Huang et al. (2020a) have designed peptides by grafting fragments from ACE2. The initial design of a peptide combining two segments of ACE2 (a.a. 22–44 and 351–357) was followed by an iterative redesign to enhance the binding to the RBD, using an in-house effective force-field, evoEF2, to drive the optimization (Huang et al., 2020a). The effectiveness of the designed peptides has however not yet been confirmed experimentally. Chatterjee et al. (2020) have, for their part, extended the strategy of ACE2 derived peptide design to target both the RBD and recruit E3 ubiquitin ligases for subsequent intracellular degradation of SARS-CoV-2 in the proteasome (Chatterjee et al., 2020). The design was performed using a protocol relying on the Rosetta program (Rohl et al., 2004), and in cellulo tests showed that one peptide is able to reduce the infection rate by −60%. Jaiswal and Kumar (2020) have extended the peptide up to a two helix bundle, using a protocol combining docking and MD simulations to identify stabilizing substitutions (Jaiswal and Kumar, 2020). The peptides showed predicted kD values on the order of 1 to a few nM, but experimental confirmation is missing. More recently, however, Zhou et al. (2021) have performed a thorough investigation of a strategy considering the two alpha-helices of hACE (Zhou et al., 2021). They concluded that the two helices cannot bind Spike when split from the ACE protein, the two peptides showing a propensity for disorder when out of ACE. They further concluded that stapling could be a relevant way to reduce the entropic cost upon binding of peptides containing one or two alpha-helices of ACE. Curreli et al. (2020); Maas et al. (2021) have reported, for lactam-stapled hACE2 peptides, experimental inhibition of the spike protein RBD-hACE2 complex formation, for concentrations on the order of 1–10 μM (Curreli et al., 2020; Maas et al., 2021).
Finally, the de novo design of peptides binding the RBD has also been explored. Chaturvedi et al. (2020) performed the de novo design of peptides targeting the RBD (Chaturvedi et al., 2020). Starting from selected ACE2 segments, natural RBD binder, the templates have been gradually modified by random mutations, while retaining those mutations that maximize their RBD-binding free energies. In this adaptive evolution, atomistic molecular dynamics simulations of the template-RBD complexes were iteratively perturbed by the peptide mutations, which were retained under favorable Monte Carlo decisions. The best candidate peptides remain however to be tested experimentally. Cao et al. (2020), have successfully designed alpha-helix bundle miniproteins encompassing the α1-helix, with median inhibitory concentration (IC50) values between 24 p.m. and 35 nM (Cao et al., 2020). The most potent exhibits a median inhibitory concentration (IC50) of close to 0.16 ng ml−1. The experimentally determined structure of the interaction between the peptides and the RBD is in excellent agreement with the computational models.
Of note, the interaction between RBD and ACE2 might be more complex than anticipated and involve more partners. Recently, another direction has been proposed by Beddingfield et al. (2021). It does not target directly the RBD ACE2 interface, but instead the spike protein and ACE2 interactions with the α5β1 integrin. Using docking, they identified three candidate binding sites for a candidate peptide active in vitro with an IC50 value of 3.16 μM. These results however require more explorations.
3.1.2 Preventing Virus Internalization by Preventing the Formation of the Fusion Core
The SARS-CoV-2 spike proteins consist of two subunits named S1, which contains the RBD, and S2 (Figure 4A). S2 is highly conserved among SARS-Like Coronaviruses and the mechanism responsible for the virus internalization is expected to be common to those viruses. After interacting with ACE2, the spike protein is cleaved into S1 and S2, and S2 undergoes a conformational rearrangement mediating viral fusion and cell entry. S2 is composed of a fusion peptide (FP), two heptad repeats (HR1 and HR2), a transmembrane domain (TM), and a cytoplasmic domain fusion (CP). After cleavage, the FP is inserted into the target cell membrane, which results in HR1 and HR2 forming a 6-helix bundle (Figure 4B). The formation of this bundle brings the cellular and viral lipid bilayers into proximity, which initiates the membrane fusion process (Belouzard et al., 2012). Preventing the formation of the helix bundle has been described as a possible strategy to block membrane fusion and prevent the entry of the virus into cells. Ling et al. (2020) have explored the design of peptides mimicking HR2 and binding HR1, to block the fusion process (Ling et al., 2020). After modeling the structure of the 6 helix bundle using homology with other viruses of the family, they investigated the binding energy of HR1 to HR2 and conversely concluded that HR2-derived peptides are probably more efficient than HR1-derived peptides to prevent the formation of the 6-helix bundle and viral infection. Efaz et al. (2021) analyzed 17 SARS-CoV-1 HR2-derived fusion inhibitor peptides known to show effective antiviral activity against the HR1 of SARS-CoV-1 and SARS-CoV-2 (Efaz et al., 2021). Using MD simulations and monitoring the free energy landscape of their binding with HR1, they identified the two best candidates. Experimental validation is however not provided at this time.
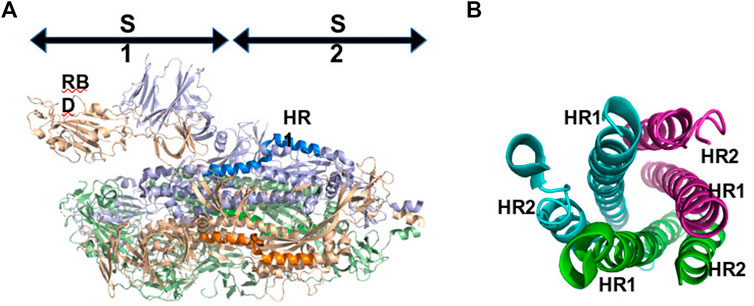
FIGURE 4. (A): Localization of subdomains S1 and S2 of SPIKE in the pre-fusion conformation. HR1 residues are in blue. (A): Conformation of the 6 helix bundle (fusion conformation).
3.1.3 Towards Targeting Intra-cellular Interactions
Targeting cell penetration of the virus is not the only strategy that could reveal effectiveness. Once the virus has entered the cell, other protein-protein interactions have been considered of potential interest.
One of these is the interaction between nsp10 and nsp16 (Figure 5). The virus replicates in the cytoplasm, and cannot access the capping machinery of the host located in the nucleus. To compensate the virus encodes its own capping enzymes, and several nsp such as nsp14 and nsp16, which are involved in viral RNA capping. Nsp16 has a binding pocket for S-adenosyl-L-methionine (SAM) which acts as a methyl group donor for the 2′-O-methylation reaction. This pocket is stabilized by the interaction with another nsp, nsp10 and consequently, the inhibition of the nsp16/nsp10 interaction is a possible strategy to prevent virus replication. Dutta and Iype (2021) have analyzed the binding interface of the nsp10/nsp16 complex [PDB id: 6W4H (Rosas-Lemus et al., 2020)] to identify peptides of nsp16 blocking the interaction between nsp10 and nsp16 (Dutta and Iype, 2021). Combined docking with MD simulations, they prospectively analyzed the binding of several candidates and concluded that two of them two and five were stable and able to bind to the nsp16 interacting region of nsp10, thus potentially preventing the interaction between the two proteins. Again, experimental confirmation is still required.
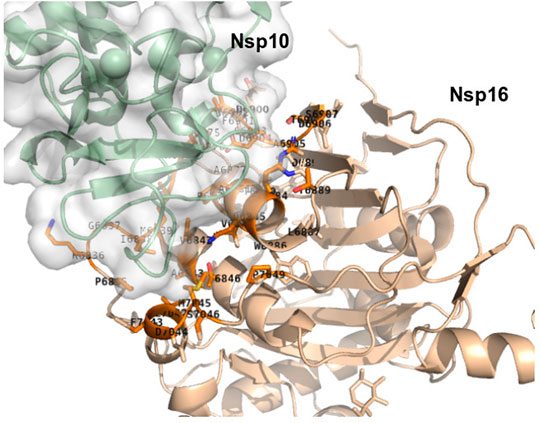
FIGURE 5. Interaction between nsp10 in green and nsp16 in wheat (PDB id: 6w4h) generated using PyMOL. Only the side chains of the residues at the interface are displayed. Identification of the residues at the nsp10/nsp16 interface was performed using a distance cut-off of 5 Å.
Another interaction is that of the PDZ binding domain of the envelope protein (E-protein) of SARS-CoV-2 with PALS1. The presence of PDZ binding motifs (PBM) that bind specific cellular PDZ domain proteins is frequent in viruses, leading to pathogenic dysfunctions of these proteins. The E-protein of SARS-CoV-2 has such a PBM known to interact with PALS1. Despite the PBM/PDZ interactions being usually weak, Toto et al. (2020) have proposed to design peptides mimicking SARS-CoV-2 E-protein targeting the PDZ domain of PALS1 (Toto et al., 2020). PALS1 participates in the maintenance of epithelial polarity, and it has been suggested that E-protein/PALS1 interaction is involved in the degradation of the integrity of the lung epithelia, resulting in dramatically increased viral dissemination. Analyzing the structure of the E-protein, they identified peptide mimics of the E-protein, assessed their relative affinity for PALS1 compared to the equivalent peptides of SARS-CoV-2, and concluded an increased affinity of the E-protein of SARS-CoV-2 for PALS1. However, no successful inhibitors have been obtained to date.
3.2 Searching for Natural Peptides Active Against Severe Acute Respiratory Syndrome Coronavirus 2 Infection
Not considering the structural information available, a direction that has repeatedly proven effective is the search among natural peptides known to have biological activities. The urgency to respond to the SARS-CoV-2 pandemic has non surprisingly stimulated the search for such candidates, although in some cases no clear rationale underlying the search existed, leading to mostly conceptual studies. It is for instance the case for the search for active peptides in the colostrum and milk. Çakır et al. (2021) have considered peptides from the goat milk whey fraction obtained by enzymatic digestion and assessed their potential combining in silico data-based prediction and docking against ACE2 and DPP-4 enzymes (Çakır et al., 2021). Pradeep et al. (2021) have reported a study in the same orientation, starting from peptides previously identified from Buffalo colostrum and milk, targeting entry points such as ACE2, Spike, TMPRSS, Cathepsin-L, or Furin, the endosomal maturation components such as AAK1, GAK, PIKfyve or TPC2, the replication transcription complex (PLpro, clpro, nsp12, nsp13) and Virion Assembly (N Protein) combining docking with MD simulations, assessing the stability of the binding of the peptide with the target (Pradeep et al., 2021). Although both studies identified some candidates of interest, these remain to be further assessed in vitro and in vivo. Yu et al. (2021) have prospectively analyzed the potential of peptides resulting from the in silico digestion of Tuna myosin to block ACE2 (Yu et al., 2021).
Several studies have considered targeting side effects of the virus infection, instead of directly addressing it. For instance, the antioxidant and anti-inflammatory effects of grehlin, have been considered to reduce the complications of the SARS-CoV-2 (Jafari et al., 2021). As well, since cardiovascular diseases are strong negative prognostic factors since they exacerbate the effects of the viral infection and lead to worse outcomes, it has been suggested that natriuretic peptides could exert a key protective role toward the virus infection whereas an impairment of NPs release contributes to the virus deleterious effects (Rubattu et al., 2021).
Anti-Microbial Peptides (AMPs) are another class of peptides with potential interest. Indeed, among the close to 3,200 AMPs discovered, close to 200 have also been reported to have antiviral activities (Wang, 2020; Mousavi Maleki et al., 2021). However, these peptides seem to have varied mechanisms of action in varied contexts. The interaction of Nisin, a food-grade antimicrobial peptide produced by lactic acid bacteria, with ACE2, has been assessed using modeling and docking. The results suggest that Nisin could act as a competitor of the RBD to bind ACE2 (Bhattacharya et al., 2021).
Finally suppressing the activity of the PLpro enzyme by using potential plant-derived protease inhibitor peptides has also been considered. (Moradi et al., 2022) have tested 11 plant-derived peptides selected from the literature that could potentially inhibit protease activity. Docking experiments suggest that VcTI from Veronica hederifolia provides effective molecular interactions at both the liable Zn site and the classic active site of PLpro. These results remain to be confirmed experimentally.
Overall, however, the exploration of natural peptides has so far led to few promises, if any.
4 Discussion
In the context of the SARS-CoV-2 pandemic, the urgent need to identify means to fight against the SARS-CoV-2 attack has raised an unprecedented effort, based on a wide panel of strategies. These encompass drug and vaccine development, or drug repurposing. Among these, peptide-based development was a possible direction to consider. Here, we have reviewed how in silico protocols have contributed to such structure-based development. It is obvious that peptide drug development does not require, per se, in silico approaches. It could proceed for instance by developing and screening experimentally peptide libraries. For instance, Rathod et al. (2020) have searched peptides from the AntiViral Peptide Database (AVPdb), with a repurposing perspective (Rathod et al., 2020). As well, cyclic peptides targeting the RBD have been experimentally designed using mRNA display (Norman et al., 2021), and both linear and cyclic peptides targeting the Mproprotease have been identified using in vitro screening (Pisarchik, 2021). As summarized in Table 1, it is however striking that the vast majority of studies have consisted of structure-based in silico design.
Indeed, the knowledge of the structure of the RBD in interaction with ACE2 has revealed extremely valuable to the design of candidate peptide drugs. In silico protocols and particularly MD simulations have made it possible to analyze in detail the interaction between the RBD and ACE2 to identify the key residues of the interaction. Their use has in turn led to the development of peptides validated in vitro as binders of the RBD, just using the expertise of some researchers focusing on substitutions at not essential sites for the interaction (Karoyan et al., 2021), or using stapling to optimize the binding (Curelli et al., 2020; Maas et al., 2021). More sophisticated studies have combined the use of docking and MD simulations to explore the stability of the binding of evolved peptides to the RBD. For the docking, peptides traditionally pose specific problems compared to small compounds due to their larger flexibility. To address this issue, it is striking to note that various, mostly flexible, docking approaches have been considered, often complemented by MD/refinement protocols to sample the conformational flexibility of the poses (Table 1). Probably here, the helical conformation of the ACE2 fragment helps making docking easier. Varied MD protocols have also been employed, using explicit or implicit solvent models, and sometimes sophisticated protocols such as umbrella sampling (Ling et al., 2020) or WHAM (Freitas et al., 2021). Finally, it is noticeable that the use of the Rosetta software (Leaver-Fay et al., 2011) has led to the effective design of several peptides binding the RBD with very low IC50 values, on the order of a few tens of pM (Cao et al., 2020), which is remarkable. The size of the peptides matters however, longer peptides tend to have lower IC50 values. Larue et al. (2020) reported that a 6 amino acid ACE2 fragment with a mM order IC50 is able to reduce cell infection by approximately 70% (Larue et al., 2020), while Chatterjee et al. (2020) reported 23 amino-acid peptides able to reduce the infection rate by 60% (Chatterjee et al., 2020), Kuznetsov et al. (2022) reported that a 19 amino acid peptide has an IC close to μM (Kuznetsov et al., 2022), and Karoyan et al. (2021) described a 27 amino acid with IC50 on the order of nM (Karoyan et al., 2021). The miniproteins designed by Cao et al. (2020) have much longer sizes, with over 55 amino acids for the best ones (Cao et al., 2020). Finally, strategies to stabilize the peptides using stapling end with peptides having IC50 values on the order of a few μM.
The identification of peptides able to compete with the RBD/ACE2 interaction, and even to slow down viral infection reduction in cellular assays does not mean however that peptide drugs are close to getting on the market. The more divergent the sequences are from the natural sequence, and the longer they are, the more likely they could become associated with adverse effects, particularly in terms of the immune response. Finally, the longer they are, the more costly they become, which could become an obstacle to their development. To a lesser extent, studies are now escaping the RBD-ACE2 interaction to tackle other interactions that occur internally in the cells. These studies also benefit from the 3D information available, although not all the structures of the proteins in interaction are known. These developments have started later on and will be confronted with harder challenges for cell internalization.
As for synthetic vaccine development, many studies have proposed candidate immunogenic peptides, that for their vast majority, lack experimental validation. It is nevertheless seizing that in silico approaches now address a wide range of considerations, including MHC I, MHC II as well as CD4 or CD8 immune response. Nevertheless, the use of peptides as a vaccine for SARS-CoV-2 treatment seems to be promising. Thus, CoVac-1, which is a combination of viral proteins is in phase II clinical trials. To note, EpiVacCorona, a peptide-based vaccine is already accepted as medicine in Russia since December 2020. Because questions arose about how the peptides were selected and what is its real immunogenicity, EpiVacCoron is used almost exclusively in Russia.
Another point to consider is the emergence of variants. Drugs and vaccines that have been developed for the wild-type SARS-CoV-2 may become less effective. For example, all the variants of concern have mutations in the spike protein that enable to partially escape the immune response and/or to increase the interaction with ACE2. The efficacy of drugs that target spike protein could be therefore drastically affected by the mutations. In this case, the use of therapeutic peptides is particularly relevant to face these issues, since the peptides of interest can be adapted for a given variant with appropriate changes in the peptide sequence.
To summarize, the SARS-CoV-2 pandemic has highlighted the reactivity of the actors of drug development. Vaccine development has proven very effective and fast, whereas synthetic peptide vaccines are still under development. For chemical drug development, drug repurposing has been so far the more effective strategy. Peptide drug development assisted by in silico analyzes has proven very reactive, able to identify promising candidates within a few months. It keeps progressing on new targets (Chan et al., 2021). The same responsiveness has been observed to search for small compounds, although through much higher investment. So far, none has been able to propose convincing enough drugs able to reach the market. It will be interesting to reconsider, hopefully in a few months, lessons from drug development against SARS-CoV-2 in terms of drug development strategy.
Author Contributions
GM and PT contributed to conception of this review. GM and PT wrote sections of the manuscript. All authors contributed to manuscript revision, read, and approved the submitted version. GM and PT contributed equally to this work.
Funding
This work was supported, in part, by IdEX Université Paris Cité ANR-18-IDEX-0001 (HS-PS project, No. IdEx-2021-I-053).
Conflict of Interest
The authors declare that the research was conducted in the absence of any commercial or financial relationships that could be construed as a potential conflict of interest.
Publisher’s Note
All claims expressed in this article are solely those of the authors and do not necessarily represent those of their affiliated organizations, or those of the publisher, the editors and the reviewers. Any product that may be evaluated in this article, or claim that may be made by its manufacturer, is not guaranteed or endorsed by the publisher.
Acknowledgments
The authors gratefully acknowledge the financial support of the Université Paris Cité, the CNRS institute, and the INSERM institute.
References
Alam, A., Khan, A., Imam, N., Siddiqui, M. F., Waseem, M., Malik, M. Z., et al. (2021). Design of an Epitope-Based Peptide Vaccine against the SARS-CoV-2: a Vaccine-Informatics Approach. Briefings Bioinforma. 22 (2), 1309–1323. doi:10.1093/bib/bbaa340
Baig, M. S., Rajpoot, S., Saqib, U., and Saqib, U. (2020). Identification of a Potential Peptide Inhibitor of SARS-CoV-2 Targeting its Entry into the Host Cells. Drugs R. D. 20 (3), 161–169. doi:10.1007/s40268-020-00312-5
Barh, D., Tiwari, S., Silva Andrade, B., Giovanetti, M., Almeida Costa, E., Kumavath, R., et al. (2020). Potential Chimeric Peptides to Block the SARS-CoV-2 Spike Receptor-Binding Domain. F1000Res 9, 576. doi:10.12688/f1000research.24074.1
Beddingfield, B. J., Iwanaga, N., Chapagain, P. P., Zheng, W., Roy, C. J., Hu, T. Y., et al. (2021). The Integrin Binding Peptide, ATN-161, as a Novel Therapy for SARS-CoV-2 Infection. JACC Basic Transl. Sci. 6 (1), 1–8. doi:10.1016/j.jacbts.2020.10.003
Belouzard, S., Millet, J. K., Licitra, B. N., and Whittaker, G. R. (2012). Mechanisms of Coronavirus Cell Entry Mediated by the Viral Spike Protein. Viruses 4 (6), 1011–1033. doi:10.3390/v4061011
Bhattacharya, R., Gupta, A. M., Mitra, S., Mandal, S., and Biswas, S. R. (2021). A Natural Food Preservative Peptide Nisin Can Interact with the SARS-CoV-2 Spike Protein Receptor Human ACE2. Virology 552, 107–111. doi:10.1016/j.virol.2020.10.002
Bruzzoni-Giovanelli, H., Alezra, V., Wolff, N., Dong, C.-Z., Tuffery, P., and Rebollo, A. (2018). Interfering Peptides Targeting Protein-Protein Interactions: the Next Generation of Drugs? Drug Discov. Today 23 (2), 272–285. doi:10.1016/j.drudis.2017.10.016
Çakır, B., Okuyan, B., Şener, G., and Tunali-Akbay, T. (2021). Investigation of Beta-Lactoglobulin Derived Bioactive Peptides against SARS-CoV-2 (COVID-19): In Silico Analysis. Eur. J. Pharmacol. 891, 173781. doi:10.1016/j.ejphar.2020.173781
Cao, L., Goreshnik, I., Coventry, B., Case, J. B., Miller, L., Kozodoy, L., et al. (2020). De Novo design of Picomolar SARS-CoV-2 Miniprotein Inhibitors. Science 370 (6515), 426–431. doi:10.1126/science.abd9909
Chan, H. H., Moesser, M. A., Walters, R. K., Malla, T. R., Twidale, R. M., John, T., et al. (2021). Discovery of SARS-CoV-2 M Pro Peptide Inhibitors From Modelling Substrate and Ligand Binding. Chemical Sci. 12 (41), 13686–13703. doi:10.1039/d1sc03628a
Chatterjee, P., Ponnapati, M., Kramme, C., Plesa, A. M., Church, G. M., and Jacobson, J. M. (2020). Targeted Intracellular Degradation of SARS-CoV-2 via Computationally Optimized Peptide Fusions. Commun. Biol. 3 (1), 715–718. doi:10.1038/s42003-020-01470-7
Chaturvedi, P., Han, Y., Král, P., and Vuković, L. (2020). Adaptive Evolution of Peptide Inhibitors for Mutating SARS‐CoV‐2. Adv. Theory Simul. 3 (12), 2000156. doi:10.1002/adts.202000156
Curreli, F., Victor, S. M. B., Ahmed, S., Drelich, A., Tong, X., Tseng, C. K., et al. (2020). Stapled Peptides Based on Human Angiotensin-Converting Enzyme 2 (ACE2) Potently Inhibit SARS-CoV-2 Infection In Vitro. Mbio 11 (6), e02451–20. doi:10.1128/mBio.02451-20
Davenport, A. P., Scully, C. C. G., de Graaf, C., Brown, A. J. H., and Maguire, J. J. (2020). Advances in Therapeutic Peptides Targeting G Protein-Coupled Receptors. Nat. Rev. Drug Discov. 19 (6), 389–413. doi:10.1038/s41573-020-0062-z
Dutta, M., and Iype, E. (2021). Peptide Inhibitors against SARS-CoV-2 2′-O-Methyltransferase Involved in RNA Capping: A Computational Approach. Biochem. Biophysics Rep. 27, 101069. doi:10.1016/j.bbrep.2021.101069
Efaz, F. M., Islam, S., Talukder, S. A., Akter, S., Tashrif, M. Z., Ali, M. A., et al. (2021). Repurposing Fusion Inhibitor Peptide against SARS‐CoV ‐2. J. Comput. Chem. 42 (32), 2283–2293. doi:10.1002/jcc.26758
Fenton, C., and Keam, S. J. (2022). Emerging Small Molecule Antivirals May Fit Neatly into COVID-19 Treatment. Drugs & Ther. Perspect. 2022, 1–15. doi:10.1007/s40267-022-00897-8
Freitas, F. C., Ferreira, P. H. B., Favaro, D. C., and Oliveira, R. J. D. (2021). Shedding Light on the Inhibitory Mechanisms of SARS-CoV-1/CoV-2 Spike Proteins by ACE2-Designed Peptides. J. Chem. Inf. Model. 61 (3), 1226–1243. doi:10.1021/acs.jcim.0c01320
Gheblawi, M., Wang, K., Viveiros, A., Nguyen, Q., Zhong, J.-C., Turner, A. J., et al. (2020). Angiotensin-Converting Enzyme 2: SARS-CoV-2 Receptor and Regulator of the Renin-Angiotensin System. Circ. Res. 126 (10), 1456–1474. doi:10.1161/circresaha.120.317015
Heitmann, J. S., Bilich, T., Tandler, C., Nelde, A., Maringer, Y., Marconato, M., et al. (2022). A COVID-19 Peptide Vaccine for the Induction of SARS-CoV-2 T Cell Immunity. Nature 601 (7894), 617–622. doi:10.1038/s41586-021-04232-5
Huang, X., Pearce, R., and Zhang, Y. (2020a). De Novo design of Protein Peptides to Block Association of the SARS-CoV-2 Spike Protein with Human ACE2. Aging 12 (12), 11263–11276. doi:10.18632/aging.103416
Huang, X., Pearce, R., and Zhang, Y. (2020b). EvoEF2: Accurate and Fast Energy Function for Computational Protein Design. Bioinformatics 36 (4), 1135–1142. doi:10.1093/bioinformatics/btz740
Jafari, A., Sadeghpour, S., Ghasemnejad-Berenji, H., Pashapour, S., and Ghasemnejad-Berenji, M. (2021). Potential Antioxidative, Anti‐inflammatory and Immunomodulatory Effects of Ghrelin, an Endogenous Peptide from the Stomach in SARS-CoV2 Infection. Int. J. Pept. Res. Ther. 27 (3), 1875–1883. doi:10.1007/s10989-021-10217-9
Jaiswal, G., and Kumar, V. (2020). In-silico Design of a Potential Inhibitor of SARS-CoV-2 S Protein. PLoS One 15 (10), e0240004. doi:10.1371/journal.pone.0240004
Kang, S., Yang, M., He, S., Wang, Y., Chen, X., Chen, Y. Q., et al. (2021). A SARS-CoV-2 Antibody Curbs Viral Nucleocapsid Protein-Induced Complement Hyperactivation. Nat. Commun. 12 (1), 1–11. doi:10.1038/s41467-021-23036-9
Karim, S. S. A., and Karim, Q. A. (2021). Omicron SARS-CoV-2 Variant: a New Chapter in the COVID-19 Pandemic. Lancet 398 (10317), 2126–2128. doi:10.1016/s0140-6736(21)02758-6
Karoyan, P., Vieillard, V., Gómez-Morales, L., Odile, E., Guihot, A., Luyt, C. E., et al. (2021). Human ACE2 Peptide-Mimics Block SARS-CoV-2 Pulmonary Cells Infection. Commun. Biol. 4 (1), 197–199. doi:10.1038/s42003-021-01736-8
Kuznetsov, A., Arukuusk, P., Härk, H., Juronen, E., Ustav, M., Langel, Ü., et al. (2022). ACE2 Peptide Fragment Interaction with Different S1 Protein Sites. Int. J. Pept. Res. Ther. 28 (1), 7. doi:10.1007/s10989-021-10324-7
Larue, R. C., Xing, E., Kenney, A. D., Zhang, Y., Tuazon, J. A., Li, J., et al. (2020). Rationally Designed ACE2-Derived Peptides Inhibit SARS-CoV-2. Bioconjugate Chem. 32 (1), 215–223. doi:10.1021/acs.bioconjchem.0c00664
Leaver-Fay, A., Tyka, M., Lewis, S. M., Lange, O. F., Thompson, J., Jacak, R., et al. (2011). Rosetta3. Methods Enzym. 487, 545–574. doi:10.1016/b978-0-12-381270-4.00019-6
Lewis, A. L., McEntee, N., Holland, J., and Patel, A. (2022). Development and Approval of Rybelsus (Oral Semaglutide): Ushering in a New Era in Peptide Delivery. Drug Deliv. Transl. Res. 12 (1), 1–6. doi:10.1007/s13346-021-01000-w
Ling, R., Dai, Y., Huang, B., Huang, W., Yu, J., Lu, X., et al. (2020). In Silico design of Antiviral Peptides Targeting the Spike Protein of SARS-CoV-2. Peptides 130, 170328. doi:10.1016/j.peptides.2020.170328
Maas, M. N., Hintzen, J. C. J., Löffler, P. M. G., and Mecinović, J. (2021). Targeting SARS-CoV-2 Spike Protein by Stapled hACE2 Peptides. Chem. Commun. 57 (26), 3283–3286. doi:10.1039/d0cc08387a
Makurvet, F. D. (2021). Biologics vs. Small Molecules: Drug Costs and Patient Access. Med. Drug Discov. 9, 100075. doi:10.1016/j.medidd.2020.100075
Moradi, M., Golmohammadi, R., Najafi, A., Moosazadeh Moghaddam, M., Fasihi-Ramandi, M., and Mirnejad, R. (2022). In Silico Analysis of Inhibiting Papain-like Protease from SARS-CoV-2 by Using Plant-Derived Peptides. Int. J. Pept. Res. Ther. 28 (1), 24–11. doi:10.1007/s10989-021-10331-8
Mousavi Maleki, M. S., Rostamian, M., and Madanchi, H. (2021). Antimicrobial Peptides and Other Peptide-like Therapeutics as Promising Candidates to Combat SARS-CoV-2. Expert Rev. anti-infective Ther. 19 (10), 1205–1217. doi:10.1080/14787210.2021.1912593
Muttenthaler, M., King, G. F., Adams, D. J., and Alewood, P. F. (2021). Trends in Peptide Drug Discovery. Nat. Rev. Drug Discov. 20 (4), 309–325. doi:10.1038/s41573-020-00135-8
Nevola, L., and Giralt, E. (2015). Modulating Protein-Protein Interactions: the Potential of Peptides. Chem. Commun. 51 (16), 3302–3315. doi:10.1039/c4cc08565e
Norman, A., Franck, C., Christie, M., Hawkins, P. M. E., Patel, K., Ashhurst, A. S., et al. (2021). Discovery of Cyclic Peptide Ligands to the SARS-CoV-2 Spike Protein Using mRNA Display. ACS Cent. Sci. 7 (6), 1001–1008. doi:10.1021/acscentsci.0c01708
Pachetti, M., Marini, B., Benedetti, F., Giudici, F., Mauro, E., Storici, P., et al. (2020). Emerging SARS-CoV-2 Mutation Hot Spots Include a Novel RNA-Dependent-RNA Polymerase Variant. J. Transl. Med. 18 (1), 179–9. doi:10.1186/s12967-020-02344-6
Papageorgiou, A. C., and Mohsin, I. (2020). The SARS-CoV-2 Spike Glycoprotein as a Drug and Vaccine Target: Structural Insights into its Complexes with ACE2 and Antibodies. Cells 9 (11), 2343. doi:10.3390/cells9112343
Peng, Y., Mentzer, A. J., Liu, G., Yao, X., Yin, Z., Dong, D., et al. (2020). Broad and Strong Memory CD4+ and CD8+ T Cells Induced by SARS-CoV-2 in UK Convalescent Individuals Following COVID-19. Nat. Immunol. 21 (11), 1336–1345. doi:10.1038/s41590-020-0782-6
Pisarchik, A. (2021). A Novel Approach for Selecting Potent Peptide Inhibitors of the SARS-CoV2-Mpro Protease. Available at Research Square [doi: doi:10.21203/rs.3.rs-396336/v1
Pradeep, H., Najma, U., and Aparna, H. S. (2021). Milk Peptides as Novel Multi‐Targeted Therapeutic Candidates for SARS-CoV2. Protein J. 40 (3), 310–327. doi:10.1007/s10930-021-09983-8
Rathod, S. B., Prajapati, P. B., Punjabi, L. B., Prajapati, K. N., Chauhan, N., and Mansuri, M. F. (2020). Peptide Modelling and Screening against Human ACE2 and Spike Glycoprotein RBD of SARS-CoV-2. Silico Pharmacol 8 (1), 3–9. doi:10.1007/s40203-020-00055-w
Rohl, C. A., Strauss, C. E. M., Misura, K. M. S., and Baker, D. (2004). Protein Structure Prediction Using Rosetta. Methods Enzym. 383, 66–93. Academic Press. doi:10.1016/s0076-6879(04)83004-0
Rosas-Lemus, M., Minasov, G., Shuvalova, L., Inniss, N. L., Kiryukhina, O., Brunzelle, J., et al. (2020). High-resolution Structures of the SARS-CoV-2 2'-O-Methyltransferase Reveal Strategies for Structure-Based Inhibitor Design. Sci. Signal 13 (651), eabe1202. doi:10.1126/scisignal.abe1202
Rubattu, S., Gallo, G., and Volpe, M. (2021). A Contemporary View of Natriuretic Peptides in the SARS-CoV-2 Era. Front. Physiol. 12, 643721. doi:10.3389/fphys.2021.643721
Sitthiyotha, T., and Chunsrivirot, S. (2020). Computational Design of 25-mer Peptide Binders of SARS-CoV-2. J. Phys. Chem. B 124 (48), 10930–10942. doi:10.1021/acs.jpcb.0c07890
Starr, T. N., Greaney, A. J., Dingens, A. S., and Bloom, J. D. (2021). Complete Map of SARS-CoV-2 RBD Mutations that Escape the Monoclonal Antibody LY-CoV555 and its Cocktail with LY-CoV016. Cell Rep. Med. 2 (4), 100255. doi:10.1016/j.xcrm.2021.100255
Tegally, H., Wilkinson, E., Giovanetti, M., Iranzadeh, A., Fonseca, V., Giandhari, J., et al. (2021). Detection of a SARS-CoV-2 Variant of Concern in South Africa. Nature 592 (7854), 438–443. doi:10.1038/s41586-021-03402-9
Toto, A., Ma, S., Malagrinò, F., Visconti, L., Pagano, L., Stromgaard, K., et al. (2020). Comparing the Binding Properties of Peptides Mimicking the Envelope Protein of SARS‐CoV and SARS‐CoV ‐2 to the PDZ Domain of the Tight Junction‐associated PALS1 Protein. Protein Sci. 29 (10), 2038–2042. doi:10.1002/pro.3936
Vlieghe, P., Lisowski, V., Martinez, J., and Khrestchatisky, M. (2010). Synthetic Therapeutic Peptides: Science and Market. Drug Discov. Today 15 (1-2), 40–56. doi:10.1016/j.drudis.2009.10.009
Wang, G. (2020). The Antimicrobial Peptide Database Provides a Platform for Decoding the Design Principles of Naturally Occurring Antimicrobial Peptides. Protein Sci. 29 (1), 8–18. doi:10.1002/pro.3702
Wang, X., Lan, J., Ge, J., Yu, J., and Shan, S. (2020). Crystal Structure of SARS-CoV-2 Spike Receptor-Binding Domain Bound with ACE2 Receptor. Nature 581 (7807), 215–220.
Wójcik, P., and Berlicki, Ł. (2016). Peptide-based Inhibitors of Protein–Protein Interactions. Bioorg. Med. Chem. Lett. 26 (3), 707–713.
Xie, X., Liu, Y., Liu, J., Zhang, X., Zou, J., Fontes-Garfias, C. R., et al. (2021). Neutralization of SARS-CoV-2 Spike 69/70 Deletion, E484K and N501Y Variants by BNT162b2 Vaccine-Elicited Sera. Nat. Med. 27 (4), 620–621. doi:10.1038/s41591-021-01270-4
Xu, J., Khan, A. R., Fu, M., Wang, R., Ji, J., and Zhai, G. (2019). Cell-penetrating Peptide: a Means of Breaking through the Physiological Barriers of Different Tissues and Organs. J. Control. Release 309, 106–124. doi:10.1016/j.jconrel.2019.07.020
Yan, R., Zhang, Y., Li, Y., Xia, L., Guo, Y., and Zhou, Q. (2020). Structural Basis for the Recognition of SARS-CoV-2 by Full-Length Human ACE2. Science 367 (6485), 1444–1448. doi:10.1126/science.abb2762
Yu, Z., Kan, R., Ji, H., Wu, S., Zhao, W., Shuian, D., et al. (2021). Identification of Tuna Protein-Derived Peptides as Potent SARS-CoV-2 Inhibitors via Molecular Docking and Molecular Dynamic Simulation. Food Chem. 342, 128366. doi:10.1016/j.foodchem.2020.128366
Zhang, H., and Chen, S. (2022). Cyclic Peptide Drugs Approved in the Last Two Decades (2001-2021). RSC Chem. Biol. 3 (1), 18–31. doi:10.1039/d1cb00154j
Zhou, P., Wang, H., Chen, Z., and Liu, Q. (2021). Context Contribution to the Intermolecular Recognition of Human ACE2-Derived Peptides by SARS-CoV-2 Spike Protein: Implications for Improving the Peptide Affinity but Not Altering the Peptide Specificity by Optimizing Indirect Readout. Mol. Omics 17 (1), 86–94. doi:10.1039/d0mo00103a
Zhou, T., Tsybovsky, Y., Gorman, J., Rapp, M., Cerutti, G., Chuang, G.-Y., et al. (2020). Cryo-EM Structures of SARS-CoV-2 Spike without and with ACE2 Reveal a pH-dependent Switch to Mediate Endosomal Positioning of Receptor-Binding Domains. Cell host microbe 28 (6), 867–879. doi:10.1016/j.chom.2020.11.004
Keywords: SARS-CoV-2, peptide, in silico, protein-protein interaction, synthetic vaccine
Citation: Moroy G and Tuffery P (2022) Peptide-Based Strategies Against SARS-CoV-2 Attack: An Updated In Silico Perspective. Front. Drug. Discov. 2:899477. doi: 10.3389/fddsv.2022.899477
Received: 18 March 2022; Accepted: 21 April 2022;
Published: 10 May 2022.
Edited by:
Bruno Villoutreix, Institut National de la Santé et de la Recherche Médicale (INSERM), FranceReviewed by:
Ashvinder Raina, Post Graduate Institute of Medical Education and Research (PGIMER), IndiaClaudio N. Cavasotto, Universidad Austral, Argentina
Copyright © 2022 Moroy and Tuffery. This is an open-access article distributed under the terms of the Creative Commons Attribution License (CC BY). The use, distribution or reproduction in other forums is permitted, provided the original author(s) and the copyright owner(s) are credited and that the original publication in this journal is cited, in accordance with accepted academic practice. No use, distribution or reproduction is permitted which does not comply with these terms.
*Correspondence: P. Tuffery, cGllcnJlLnR1ZmZlcnlAdW5pdi1wYXJpcy1kaWRlcm90LmZy, cGllcnJlLnR1ZmZlcnlAaW5zZXJtLmZy