- 1Departamento de Química, Universidad de Córdoba, Córdoba, Colombia
- 2Environmental Chemistry Department, Institute of Environmental Assessment and Water Research, IDAEA-CSIC, Barcelona, Spain
Discharge of metals into the environment generates significant impact upon human health and biological cycles. Some microorganisms such as fungi are known for their high metal adsorption capacity. The aim of this work was to evaluate the capacity for Penicillium sp. molds in the removal of Pb, Cd, and Hg from aqueous solutions by isolating the fungal strain from an artisanal gold mine soil. The biosorption experiments showed that optimum conditions for metal removal were noted at 150 min, acidic pH (4–5), 60 °C, and 2 g of biomass. The accomplished removal was 92.4% for Pb, 80% for Cd, and 99.6% for Hg, at a concentration of 51.5 mg/L. Kinetic analyses and isotherms best fit the pseudo–second-order and Langmüir models, respectively. Infrared spectra show functional groups (–OH, –NH, C-N, C-H, N-H, and C=O) that play an essential role in the adsorption of Pb, Hg, and Cd on fungal biomass.
Introduction
Increasing industrial activity is responsible in great part for the principal problems of environmental contamination and damage to ecosystems, generating accumulation of toxic contaminants such as the metals Cr, Cu, Pb, Cd, Zn, Ni, and Hg (Pino et al., 2006; Dhir, 2014) and causing damage to human health. Among them, Pb is one of the most toxic metals, causing anemia, encephalopathy, hepatitis, and nephritic syndrome (Deng et al., 2006; Ji et al., 2012). Exposure to Cd generates severe risks to human health and may cause cancer, kidney damage, destruction of the mucous membrane, vomit, diarrhea, bone damage, and Itai-itai disease and affect the production of progesterone and testosterone (Godt et al., 2006; Stasenko et al., 2010), Finally, Hg has been known to generate deterioration of the nervous system, including poisoning of protoplasm (Hima et al., 2007) and Minamata disease (Harada, 1995).
Several competing technologies exist to remediate the load of metals in industrial and mining waste waters, where adsorption is highly viable on the industrial scale as far as the cost–benefit relationship is concerned (Lin et al., 2008). However, the high cost of adsorbents (usually activated carbon) is considered the main obstacle for industrial application. Therefore, the focus of study of adsorption of metals has been inclined toward natural materials available in vast quantities (Agarwal et al., 2006; Srivastav et al., 2021). Bioremediation by using microorganisms is an approach in which toxic contaminants can be removed from mediums such as soil and sediment of water (Rani et al., 2021); studies have been published regarding the possible use of Penicillium sp. mold as a potential bio-adsorbent of metals, as it shows extraordinary extracellular adsorption capacity (Fan et al., 2008).
Due to this, the aim of this work was to evaluate the capacity of simultaneous removal of Pb (II), Cd (II), and Hg (II) in aqueous solutions through Penicillium sp. molds by isolating the fungal strain from the soil collected from an artisanal gold mine containing a high load of contaminants. The influence of variables such as pH, time of contact, initial metal concentration, amount of biomass, and medium temperature was assessed. Moreover, the active functional groups of the fungal biomass that intervene in the adsorption phenomenon were verified through infrared analysis (IR), analyzing the adsorption models of the Langmüir and Freundlich isotherms, and pseudo–first- and pseudo–second-order kinetic studies.
Materials and Methods
Sampling and Characterization of Penicillium sp. Fungal Strains
The soil samples were collected from the Alacrán mine (Córdoba, Colombia) in January 2013; it is located at the following coordinates: 7°44′29.01″ N - 75°44′10.8″ W (Marrugo-Negrete et al., 2016). Ten random samples (N = 10) were taken at 330 m from the mine entrance by excavating using a sterile shovel for approximately 0.5 m and taking approximately 500 g of soil; the samples from each point were homogenized in a sterile plastic container. Close to 500 g in sealed sterile bags was delivered to the laboratory to perform the fungal strain isolation. Once in the laboratory, 1 g of the soil sample was weighed and dissolved in sterile bi-distilled water. Czapek-Dox Agar (CDA) plates were used (pH 5) with supplements of streptomycin sulfate (25 mg/100 ml) and cyclohexamide (6 mg/100 ml) to isolate metal-tolerant molds (Velmurugan et al., 2010). The diluted soil sample (0.1 ml) was inoculated in CDA plates. The inoculated plates were incubated at 25°C for 7 days, taking control plates with inoculations of sterilized bi-distilled water incubated under the same conditions. After the incubation period, the mold species grew up in the plates and were transferred to CDA plates, incubating until growth was completed. From the growth cultures in CDA medium with 7-day growth, the fungal samples cultured were divided, and macroscopic and microscopic characteristics were observed according to Valencia (2004). The pure fungal culture was kept in CDA plates and in broth. The massive growth of the mold takes place in Czapek-Dox liquid medium for 30 days. The Penicillium sp. mold biomass generated was extracted via screening, and this biomass is the base material for biosorption experiments (Velmurugan et al., 2010).
Experimental Design
A single-factor block experimental design was used where the response to be measured was the concentration of metallic ions in equilibrium. For each factor (initial metal concentration, time of contact, pH, amount of biomass, and temperature), the other variables were kept constant; a strongly recommended strategy supposing that factor variability is high. Results of analyses are presented by the mean
Analytical Methods and Quality Control
The determination of total Hg concentrations in water was performed by cold vapor atomic absorption spectrometry using a mercury analyzer RA 915 M combined with an RP-92 Cold Vapor accessory, using EPA Method 245.1 (US EPA, 1994). Pb and Cd concentrations were determined by microwave-assisted acid digestion, using an Ethos EZ microwave digestion system (Milestone Inc., Shelton, CT, US) (US EPA, 2007). Heavy metal concentrations were quantified using the technique of graphite furnace atomic absorption spectrophotometry (GFAAS) in a Thermo Electron Corporation atomic.
The readings were done in triplicate, with NIST-certified reference material; SRM 1643e for Pb (II) and Cd (II) and SRM 1641d for Hg (II) for natural waters; precision was evaluated as % Error (% E), obtaining 0.238% for Pb, 0.582% for Cd, and 0.230% for Hg. Precision was evaluated as relative standard deviation (%RSD), finding 0.752 for Pb, 0.613 for Cd, and 0.759 for Hg, well within the analytic quality criteria (Miller and Miller, 2018).
Biosorption Experiments
A mixed stock solution was prepared with Pb (II), Cd (II), and Hg (II) at 1,000 mg L−1, from the salts corresponding to the metals. All the biosorption experiments were carried out on the same type of stock solution (Velmurugan et al., 2010). The effect of the metal concentration was determined by using 100 ml of a multiple stock of Pb, Cd, and Hg at 8.0, 30.0, 51.5, and 103.0 mg L−1 (prepared from the stock solution) and 0.5 g of biosorbent at pH 4 and 30°C. These were incubated in flasks and agitated at 200 rpm min−1 for 24 h; after the incubation period, the supernatants were collected through centrifuge at 6,000 rpm min−1 for 30 min. Thereafter, the metal concentration was determined in the supernatant by atomic absorption with electrothermal atomization. The effect of the initial concentration yields the necessary data for analysis of Freundlich and Langmüir isotherms. The time-of-contact effect was studied by using a multiple stock at 51.5 mg L−1 of Pb (II), Cd (II), and Hg (II) and 0.5 g of biosorbent at pH 4 and 30°C. All the flasks were incubated in an agitator at 200 rpm min−1 during 30, 60, 90, 120, 150, 180, and 210 min; these results are used for kinetic studies. The effect of pH was assessed by preparing a mixed stock of Pb (II), Cd (II), and Hg (II) at 51.5 mg L−1 and 0.5 g of biosorbent. The pH of the solutions was 2, 3, 4, 5, 6, 7, 8, 9, and 10. The effect of the amount of biomass was studied by using multiple stock solutions of target metals at 51.5 mg L−1 each and 0.25, 0.50, 1.0, 2.0, and 3.0 g of biosorbent at pH 4 and 30°C. The effect of temperature was determined by using a multiple stock of metals at 51.5 mg L−1 and 0.5 g of biosorbent at pH 4; these were incubated in flasks and agitated at 200 rpm min−1 for 24 h at 20, 25, 30, 35, 40, 45, 50, 55, and 60°C, respectively. Each of the tests was run in duplicate. After the incubation period, the supernatant was collected and metal analyses were performed using a Thermo Scientific Spectrometer (model ICE 3000 Series) with wavelengths of 217, 228.8, and 253.7 nm for Pb (II), Cd (II), and Hg (II), respectively.
Experimental Analysis of Data
The amount of Pb (II), Cd (II), and Hg (II) adsorbed by the biomass was calculated by using Eq. 1.
where qe is the amount adsorbed by the biomass in (mg g−1), C0 is the initial concentration of the metals, Ce is the concentration of Pb (II) and Cd (II) ions in equilibrium, V is the volume of the solution (L), and W is the amount of adsorbent (g); the experiment was carried out at 30°C (Lee and Chang, 2011).
The adsorption data for adsorbate concentrations were described by the Langmüir and Freundlich isotherm models. The Langmüir model is described according to Eq. 2 and the Freundlich model by Eq. 3.
where qe is the amount adsorbed by the biomass in (mg g−1), Ce is the ion concentration in equilibrium, qmax represents the maximum amount adsorbed, b is the affinity between the biosorbent and the biosorbate, and Kf and 1/n are the Freundlich isotherm constant (Lee and Chang, 2011). Kinetic models of metal adsorption through Penicillium sp. were described via pseudo–first- and pseudo–second-order rate equations. Lagergren (1898) proposed the pseudo–first-order equation based on the solid capacity equation (Eq. 4) for the first time. The pseudo–second-order rate equation can be used to predict the behavior for the whole adsorption; it is expressed as follows (Eq. 5).
where the values of qe (mg g−1) and q (mg g−1) are the amount of metallic ions adsorbed per mass unit of the adsorbent in equilibrium and over time, t (min), respectively, and k1 (min−1) is the first-order adsorption kinetic constant. In the pseudo–second-order equation, the second-order constant, k2, in (g·mg−1·min) can be experimentally determined from the slope and the intercept of a graph, t/q, in function of t.
Evaluation of the Metal–Biomass Bond Sites
This was analyzed by preparing a mixed stock of metals at 51.5 mg L−1 and 0.5 g of biosorbent, after 24 h; then, the biomass was dried at room temperature. The IR spectra were conducted by using an FT-IR Nicolet Is5 Thermo Scientific Spectrophotometer in the 500–4,000 cm−1 region (Velmurugan et al., 2010).
Results and Discussion
Characterization of Penicillium sp. Fungal Strains
The mold showed white, velvety colonies; with advanced time of growth, it presented a dark green coloration and did not generate pigments in the growth media. It revealed concentric formations, conserving its white obverse. Its vegetative hyphae, formed through simple division, gave rise to metulae. Each metula gave rise to three phialides that ended in conidiogenous cells or conidiophores, which—in turn—gave rise to conidia. Its peculiar “brush head” shape was observed. Its conidia are oval-shaped and presented chain detachment, which confirms that it is the Penicillium sp. species (Valencia, 2004).
Effect of the Initial Metal Concentration
The effect of the initial metal concentration (Figure 1) shows the percentage of metal adsorption by concentration level. For Pb, the highest removal levels were found at concentrations of 51.5 and 103 mg L−1, with respective percentages of 62.9 and 60.4%; for Cd, the highest removals were found at 30 and 51.5 mg L−1 with percentages of 12.4 and 14.6%, respectively. The highest levels of Hg (II) adsorption were accomplished at 30 and 51.5 mg L−1 with percentages of 78.9 and 67.9%, respectively. As metal concentrations increased in the solution, the adsorption capacity remains constant; this is due to saturation of the sites available on the biosorbent surface (Volesky and Holan, 1995). In general, the level of 51.5 mg L−1 corresponds to the adequate concentration to find the highest percentages of removal of toxic contaminants. The different adsorption percentages for the contaminants show that the biosorbent adsorbs a greater amount of Hg (II) ions than Pb (II), and—in turn—Cd (II).
Time-of-Contact Effect
Figure 2 shows the time-of-contact effect in removal of contaminants. Removal of Pb was observed at 30 min of exposure between the metal and the fungal biomass and increased dramatically between 60 and 90 min; elimination equilibrium is noted as of 150 min. Elimination of Pb (II) began after 60 min of exposure to the biosorbent and increased simultaneously at 90 and 120 min. Biosorption between the metal ions and the adsorbent may be higher during the initial stages of exposure (Sathishkumar et al., 2007). For Cd, the maximum adsorption of the metal was noted at 150 min of treatment; elimination equilibrium was evidenced as of that moment. Elimination of Cd (II) began after 30 min of exposure to the biosorbent and increased simultaneously at 60, 90, 120, and 150 min. Finally, for Hg, the maximum elimination occurred at 180 min and elimination equilibrium was noted after 150 min. Adsorption of Hg (II) began after 30 min of exposure to the biosorbent and increased simultaneously during the whole remaining time. The results clearly indicate that elimination of Pb (II), Cd (II), and Hg (II) increases with time of contact. Similar results were also reported by Fan et al. (2008) during the single removal of Pb (II) by Penicillium sp.
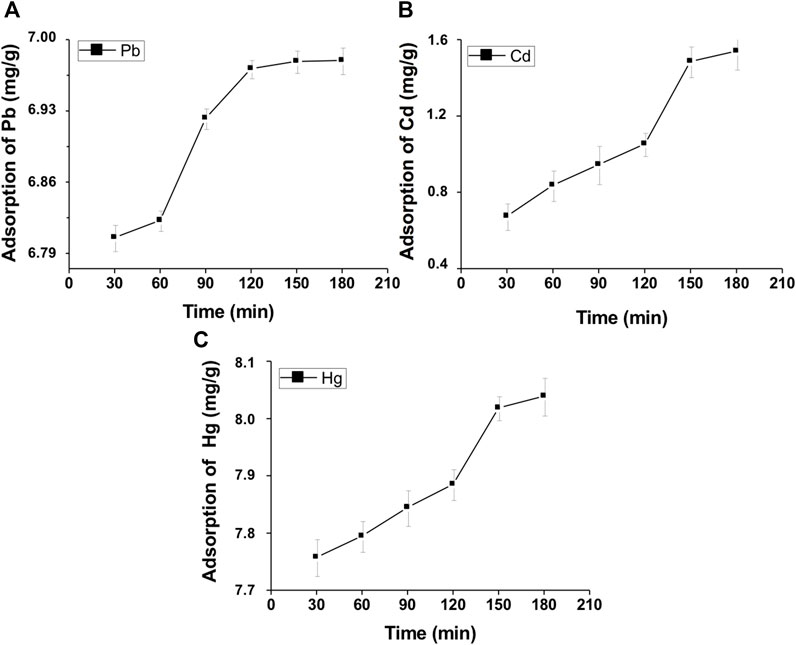
FIGURE 2. Effect of time of contact in metal adsorption; (A) Pb (II), (B) Cd (II), (C) Hg (II) at (51.5 mg L−1) with Penicillium sp. biomass.
Effect of pH
Metal ions binding by Penicillium sp. was clearly pH dependent. The three metals show (Figure 3) a similar tendency. The metal loading capacity increased with increasing pH under acidic conditions, with maximum removal values at pH 6 and stabilization between 7 and 10. Although the metals were at the same concentrations, a higher elimination capacity should be noted for Hg (II), followed by Pb (II) and Cd (II).
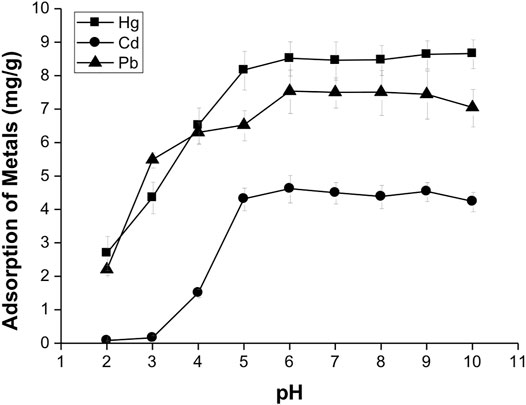
FIGURE 3. Effect of pH in Pb (II), Cd (II), and Hg (II) adsorption at 51.5 mg L−1 with Penicillium sp. biomass.
Thus, the highest adsorptions were accomplished in acidic pH, noting that adsorption is reduced in alkaline pH. At low pH, on the mold surface, positive charges prevent contact of the metallic ions and the negatively charged surface (Sathishkumar et al., 2007). However, increased pH between 4 and 5 increases elimination of the metal ions available in the solution, given that neutralization of the positive charges on the mold surface improves contact between the ions and the surface. Above pH 7, ions precipitate in the solution, and contact is inhibited between the negative charge of the mold surface and the ions (Gupta and Rastogi, 2008). Hence, elimination of ions is stabilized above pH 7. Similar results were published by Fan et al. (2008) for Pb (II) removal with Penicillium simplicissimum, whereas Yan and Viraragavan (2003) reported that Pb (II) elimination through Penicillium digitatum and Rhizopus nigricans decreased when pH decreased and increased as pH rose. Other researchers have reported similar results regarding Pb (II) and Hg (II) elimination through the Trametes versicolor mold (Bayramoğlu et al., 2003).
Effect of the Amount of Biomass
Removal of toxic metals shows a considerable increase as a function of the amount of biomass (Figure 4) where Hg (II) is the metal most removed in all the tests. In turn, Pb (II) removal was higher than Cd (II) removal in all the tests. Hg (II) was quickly removed in values from 0 to 0.5 g and remained constant up to 1–3 g of biosorbent, reaching the maximum removal of 51.5 mg L−1, corresponding to the initial concentration. Pb (II) rises rapidly between 0 and 0.5 g but reaches its highest level at 2 g, maintaining the same removal at 3 g of biosorbent. For Cd (II), removal increases with the amount of biomass; the maximum removal value was found at 2 g of biomass. Overall, at 2 g of biomass, percentages of removal are 80% for Cd, 92.4% for Pb, and 99.6% of Hg, at an initial concentration of 51.5 mg L−1.
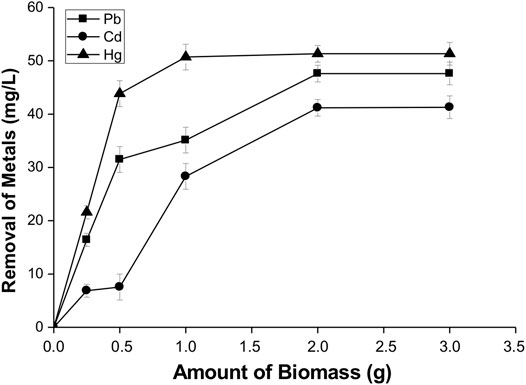
FIGURE 4. Effect of the amount of biomass (g) in Pb (II), Cd (II), and Hg (II) adsorption at 51.5 mg L−1 with Penicillium sp. biomass.
Effect of Temperature
The results are summarized in Figure 5, where the ratio of metal adsorption per unit of biomass increased with temperature for all metals and accomplishing maximum values at 60°C. Similar results were reported by Fan et al. (2008), who showed biosorption of Pb, Cd, and Zn with the Penicillium simplicissimum mold by conducting tests from 20 to 40°C. They concluded that Pb (II) adsorption was endothermic. The energy dependence of metal elimination by microorganisms is greatly influenced by temperature, given that the adsorption process is physicochemical. Meanwhile, Mishra and Chaudhury (1996) reported that Zn (II) adsorption by Penicillium sp. diminishes with increased temperature because temperature can affect the molds’ cell wall stability, which leads to cell wall hydrolysis. According to that which was reported by Fan et al. (2008), Pb (II) adsorption by Penicillium sp. was endothermic.
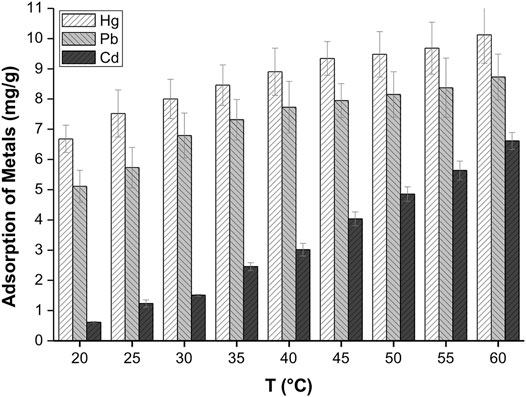
FIGURE 5. Effect of temperature (°C) in Pb (II), Cd (II), and Hg (II) adsorption at 51.5 mg L−1 with Penicillium sp. biomass.
Sorption Isotherms
The constants obtained from the Langmüir and Freundlich isotherms had high correlation coefficients (R2) (Table 1). Comparisons among correlation coefficients show that Langmüir and Freundlich isotherms with Penicillium sp. mold biomass best fit the Langmüir model rather than the Freundlich model, given that the R2 values for Langmüir are higher than the R2 values for Freundlich; additionally, the values of the b adsorption energy constant are very close to zero, indicating that the isotherm model for this study is more strongly supported by Langmüir isotherms than by Freundlich isotherms. Results suggest that all the active adsorption centers in the fungal biomass are equivalent and that the capacity of the metals to bind to the surface is independent of whether occupied proximal positions are available. Additionally, adsorption is restricted to a monolayer, and no lateral interactions exist among the ions (Prakash Tripathy et al., 2020).
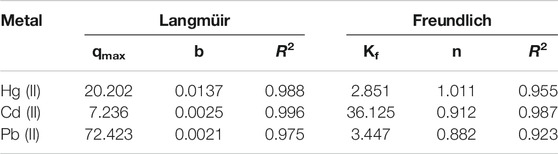
TABLE 1. Constant adsorption isotherms in Langmüir and Freundlich models of Hg (II), Cd (II), and Pb (II) for Penicillium sp.
The b values indicate the affinity of a biosorbent for an adsorbate; to the extent that these values are smaller, the biosorbent has more affinity to the metal, and hence, the biosorbent with greater affinity toward Pb (II) has a b value of 0.0021 L mg−1, followed by Cd (II) with 0.0025 L mg−1 and Hg (II) with 0.0137 L mg−1. The qmax representing the biosorbent’s maximum biosorption capacity for each metal is quite different, highlighting Pb (II) with the highest qmax value (72.423 mg g−1), followed by Hg (II) with 20.202 mg g−1 and Cd (II) with qmax of 7.236 mg g−1, which suggests that the maximum adsorption capacity for the three metals rests on Pb (II).
To analyze the results via Freundlich, the highest K values were found for Cd (II) and Pb (II). High K indicates a high adsorption volume and a high value 1/n (n > 1)/ indicates high adsorption force. The highest values of n were for Hg (II) and Cd (II) with 1.011 and 0.912, respectively. Similar results were reported by Fan et al. (2008) for single removal of Pb (II) by Penicillium simplicissium.
Sorption Kinetics
The kinetic parameters of the pseudo–first-order and pseudo–second-order models are shown in Table 2. The pseudo–first-order kinetic equation tends to be adequate for the first 30–60 min of adsorption. Hence, Lagergren’s first-order kinetic equation does not fit during the whole adsorption period (Prakash Tripathy et al., 2020).
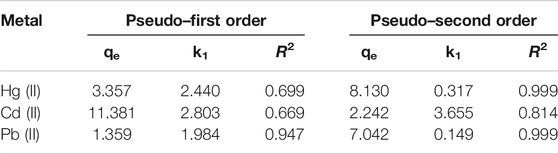
TABLE 2. Constant kinetics of pseudo–first- and pseudo–second-order models for Hg (II), Cd (II), and Pb (II) adsorption in Penicillium sp. mold biomass.
Significant variations were found of qe values between the first-order and pseudo–second-order kinetics. The pseudo–second-order correlation model had a higher correlation coefficient for all the metals: Hg (0.999), Pb (0.999), and Cd (0.814). The pseudo–second-order model fits best in the experimental data than the pseudo–first-order model. Sathishkumar et al. (2007) reported similar results for the adsorption kinetics of Procion blue HB in a Panus fulvus inactive mycelial biomass. This tendency suggests that the rate of limitation in metal biosorption is through chemisorption, which implies valence forces through shared use or electron exchange between the sorbent and the sorbate, complexation, coordination, and/or chelation, in lieu of physisorption (Gupta and Rastogi, 2008).
Evaluation of Metal–Biomass Bond Sites
Figure 6 shows the FT-IR spectrum of the Penicillium sp. mold biomass before (control) and after removal treatment of target metals. The molds’ surface structure is inferred with the functional groups found in the spectra, showing the Penicillium sp. active groups upon removal. Sample control groups are observed at (3,396), (2,925), (1,654), (1,548), and (1,150) cm−1.
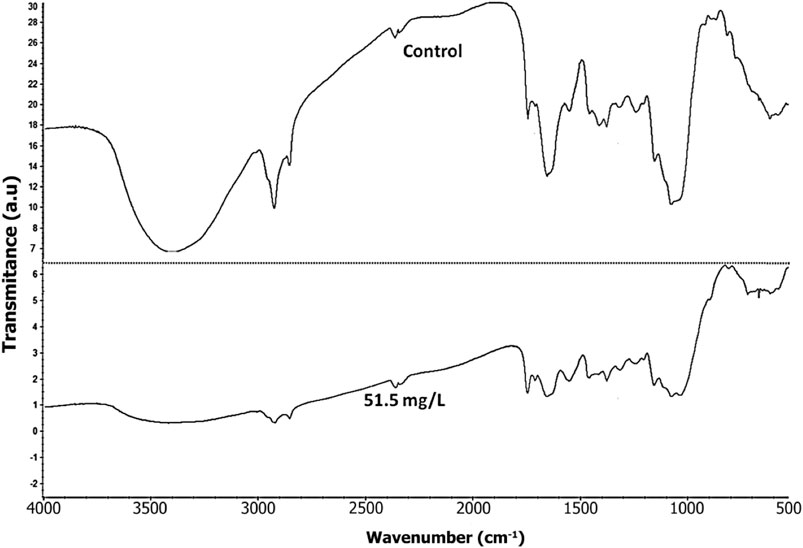
FIGURE 6. FT-IR of Penicillium sp. biomass before treatment (control) and after treatment with 51.5 mg L−1 of Pb (II), Cd (II), and Hg (II).
A wide peak observed between 3,200 and 3,600 cm−1 was attributed to tension modes of –OH and –NH groups. A strong peak appeared at (1,150) cm−1, indicating C-N stretching vibrations (Shriner et al., 2003). C-H bonds’ typical stretching and N-H vibrations were assigned to peaks at (2,925) and (1,548) cm−1. The strong peak at (1,654) indicates a group, stretching of the C=O group or amide I and amide II (Deng and Ting, 2005; Deng et al., 2006). The presence of a common chemical structure for polysaccharides was found between 1,200 and 800 cm−1, and the peak at (1,150) cm−1 is attributed to C-N stretching in the control sample, displaced at (1,152) cm−1 in the sample treated after metal absorption. Changes present in the region of 1,660 and 1,500 cm−1 for N-H were also due to absorption conduction (Deng and Ting, 2005).
Conclusion
A potential metal biosorbent of the fungal type of the Penicillium sp. genus was isolated from mine soils highly contaminated by metals. The experimental results showed the high capacity of Penicillium sp. to eliminate Pb (II), Cd (II), and Hg (II) from aqueous solutions. Optimal pH (5–6) and temperature (60°C) conditions to eliminate these metals were determined in the study. The experimental data were analyzed by using isotherm models to evaluate the performance of the biosorbent mold. Pseudo–first- and pseudo–second-order kinetic models were also studied. The IR analysis shows bands and peaks quite characteristic of bonds of functional groups –OH, –NH, C-N, C-H, N-H, C=O, amide I and amide II, and polysaccharides; the metal adsorption capacity can be attributed to the fact that the majority have partially positive charge that can generate attraction between the metals and the fungal biomass. Last, the results indicate that the molds isolated from the mine soils could be used as a profitable and easily culturable biosorbent to eliminate metal ions from environments contaminated with metals.
Data Availability Statement
The raw data supporting the conclusions of this article will be made available by the authors, without undue reservation.
Author Contributions
JS-C: writing—original draft, investigation, and methodology. IU-C: supervision, writing—original draft. GE-M: investigation. SB-N: supervision. JM-N: conceptualization, project administration, and funding acquisition. SD: writing—review and editing.
Funding
This work was supported by Colciencias and the University of Cordoba through the contract 223-2010.
Conflict of Interest
The authors declare that the research was conducted in the absence of any commercial or financial relationships that could be construed as a potential conflict of interest.
Publisher’s Note
All claims expressed in this article are solely those of the authors and do not necessarily represent those of their affiliated organizations, or those of the publisher, the editors, and the reviewers. Any product that may be evaluated in this article, or claim that may be made by its manufacturer, is not guaranteed or endorsed by the publisher.
Acknowledgments
The authors thank Colciencias and the University of Cordoba for financial support through the contract 223-2010. They also express their gratitude to the miners in the region of Cordoba, Colombia for their special support.
References
Agarwal, G. S., Bhuptawat, H. K., and Chaudhari, S. (2006). Biosorption of Aqueous Chromium(VI) by Tamarindus indica Seeds. Bioresour. Tech. 97, 949–956. doi:10.1016/j.biortech.2005.04.030
Bayramoglu, G., Bektaş, S., and Arica, M. Y. (2003). Biosorption of Heavy Metal Ions on Immobilized white-rot Fungus Trametes versicolor. J. Hazard. Mater. 101, 285–300. doi:10.1016/s0304-3894(03)00178-x
Deng, L., Su, Y., Su, H., Wang, X., and Zhu, X. (2006). Biosorption of Copper (II) and lead (II) from Aqueous Solutions by Nonliving green Algae Cladophora Fascicularis: Equilibrium, Kinetics and Environmental Effects. Adsorption 12, 267–277. doi:10.1007/s10450-006-0503-y
Deng, S., and Ting, Y.-P. (2005). Characterization of PEI-Modified Biomass and Biosorption of Cu(II), Pb(II) and Ni(II). Water Res. 39, 2167–2177. doi:10.1016/j.watres.2005.03.033
Dhir, B. (2014). Potential of Biological Materials for Removing Heavy Metals from Wastewater. Environ. Sci. Pollut. Res. 21, 1614–1627. doi:10.1007/s11356-013-2230-8
Fan, T., Liu, Y., Feng, B., Zeng, G., Yang, C., Zhou, M., et al. (2008). Biosorption of Cadmium(II), Zinc(II) and Lead(II) by Penicillium simplicissimum: Isotherms, Kinetics and Thermodynamics. J. Hazard. Mater. 160, 655–661. doi:10.1016/j.jhazmat.2008.03.038
Godt, J., Scheidig, F., Grosse-Siestrup, C., Esche, V., Brandenburg, P., Reich, A., et al. (2006). The Toxicity of Cadmium and Resulting Hazards for Human Health. J. Occup. Med. Toxicol. 1, 22. doi:10.1186/1745-6673-1-22
Gupta, V. K., and Rastogi, A. (2008). Biosorption of lead from Aqueous Solutions by green Algae Spirogyra Species: Kinetics and Equilibrium Studies. J. Hazard. Mater. 152, 407–414. doi:10.1016/j.jhazmat.2007.07.028
Harada, M. (1995). Minamata Disease: Methylmercury Poisoning in Japan Caused by Environmental Pollution. Crit. Rev. Toxicol. 25 (1), 1–24. doi:10.3109/10408449509089885
Hima, K. A., Srinivasa, R. R., Vijaya, S. S., Jayakumar, S. B., Suryanarayana, V., and Venkateshwar, P. (2007). Biosorption: An Eco-Friendly Alternative for Heavy Metal Removal. Afr. J. Biotechnol. 6, 2924–2931. doi:10.5897/AJB2007.000-2461
Ji, L., Xie, S., Feng, J., Li, Y., and Chen, L. (2012). Heavy Metal Uptake Capacities by the Common Freshwater green Alga Cladophora Fracta. J. Appl. Phycol 24, 979–983. doi:10.1007/s10811-011-9721-0
Lagergren, S. K. (1898). About the Theory of So-Called Adsorption of Soluble Substances. Sven. Vetenskapsakad. Handingarl 24, 1–39.
Lee, Y.-C., and Chang, S.-P. (2011). The Biosorption of Heavy Metals from Aqueous Solution by Spirogyra and Cladophora Filamentous Macroalgae. Bioresour. Tech. 102, 5297–5304. doi:10.1016/j.biortech.2010.12.103
Lin, L.-C., Li, J.-K., and Juang, R.-S. (2008). Removal of Cu(II) and Ni(II) from Aqueous Solutions Using Batch and Fixed-Bed Ion Exchange Processes. Desalination 225, 249–259. doi:10.1016/j.desal.2007.03.017
Marrugo-Negrete, J., Marrugo-Madrid, S., Pinedo-Hernández, J., Durango-Hernández, J., and Díez, S. (2016). Screening of Native Plant Species for Phytoremediation Potential at a Hg-Contaminated Mining Site. Sci. Total Environ. 542, 809–816. doi:10.1016/j.scitotenv.2015.10.117
Miller, J., and Miller, J. C. (2018). Statistics and Chemometrics for Analytical Chemistry. London, UK: Pearson education.
Mishra, S. P., and Chaudhury, G. R. (1996). Kinetics of Zn2+ Adsorption by Penicillium Sp. Hydrometallurgy 40, 11–23. doi:10.1016/0304-386X(94)00088-K
Pino, G. H., de Mesquita, L. M. S., Torem, M. L., and Pinto, G. A. S. (2006). Biosorption of Heavy Metals by Powder of Green Coconut Shell. Sep. Sci. Tech. 41, 3141–3153. doi:10.1080/01496390600851640
Prakash Tripathy, S., Acharya, R., Das, M., Acharya, R., and Parida, K. (2020). Adsorptive Remediation of Cr (VI) from Aqueous Solution Using Cobalt Ferrite: Kinetics and Isotherm Studies. Mater. Today Proc. 30, 289–293. doi:10.1016/j.matpr.2020.01.534
Rani, L., Srivastav, A. L., and Kaushal, J. (2021). Bioremediation: An Effective Approach of Mercury Removal from the Aqueous Solutions. Chemosphere 280, 130654. doi:10.1016/j.chemosphere.2021.130654
Sathishkumar, M., Binupriya, A. R., Vijayaraghavan, K., and Yun, S.-I. (2007). Two and Three-Parameter Isothermal Modeling for Liquid-phase Sorption of Procion Blue H-B by Inactive Mycelial Biomass ofPanus Fulvus. J. Chem. Technol. Biotechnol. 82, 389–398. doi:10.1002/jctb.1682
Shriner, R. L., Hermann, C. K., Morrill, T. C., Curtin, D. Y., and Fuson, R. C. (2003). The Systematic Identification of Organic Compounds. Hoboken, US: John Wiley & Sons.
Srivastav, A. L., Pham, T. D., Izah, S. C., Singh, N., and Singh, P. K. (in press Forthcoming 2021). Biochar Adsorbents for Arsenic Removal from Water Environment: A Review. Bull. Environ. Contam. Toxicol. doi:10.1007/s00128-021-03374-6
Stasenko, S., Bradford, E. M., Piasek, M., Henson, M. C., Varnai, V. M., Jurasović, J., et al. (2010). Metals in Human Placenta: Focus on the Effects of Cadmium on Steroid Hormones and Leptin. J. Appl. Toxicol. 30, 242–253. doi:10.1002/jat.1490
US EPA (1994). EPA Method 245.1: Determination of Mercury in Water by Cold Vapor Atomic Absorption Spectrometry. Available at: https://www.epa.gov/esam/epa-method-2451-determination-mercury-water-cold-vapor-atomic-absorption-spectrometry (Accessed December 2, 2021).
US EPA (2007). EPA Method 7010: Graphite Furnace Atomic Absorption spectrophotometry.Test Methods for Evaluating Solid Waste, Physical/Chemical Methods SW86. Available at: https://www.epa.gov/hw-sw846/sw-846-test-method-7010-graphite-furnace-atomic-absorption-spectrophotometry (Accessed December 2, 2021).
Valencia, H. (2004). Cultivo, microcultivo e identificación de hongos. Manual de prácticas de microbiología básica. Bogotá: Facultad de Ciencias de la Universidad Nacional de Colombia, p99–116.
Velmurugan, N., Hwang, G., Sathishkumar, M., Choi, T. K., Lee, K.-J., Oh, B.-T., et al. (2010). Isolation, Identification, Pb(II) Biosorption Isotherms and Kinetics of a lead Adsorbing Penicillium Sp. MRF-1 from South Korean Mine Soil. J. Environ. Sci. 22, 1049–1056. doi:10.1016/S1001-0742(09)60216-3
Volesky, B., and Holan, Z. R. (1995). Biosorption of Heavy Metals. Biotechnol. Prog. 11, 235–250. doi:10.1021/bp00033a001
Keywords: Biosorption, Metals, Penicillium sp., Langmuir model, Mercury
Citation: Sánchez-Castellón J, Urango-Cárdenas I, Enamorado-Montes G, Burgos-Nuñez S, Marrugo-Negrete J and Díez S (2022) Removal of Mercury, Cadmium, and Lead Ions by Penicillium sp.. Front. Environ. Chem. 2:795632. doi: 10.3389/fenvc.2021.795632
Received: 15 October 2021; Accepted: 08 December 2021;
Published: 24 January 2022.
Edited by:
Anne Helene Fostier, State University of Campinas, BrazilReviewed by:
Dong-Xing Guan, Zhejiang University, ChinaArun Lal Srivastav, Chitkara University, India
Copyright © 2022 Sánchez-Castellón, Urango-Cárdenas, Enamorado-Montes, Burgos-Nuñez, Marrugo-Negrete and Díez. This is an open-access article distributed under the terms of the Creative Commons Attribution License (CC BY). The use, distribution or reproduction in other forums is permitted, provided the original author(s) and the copyright owner(s) are credited and that the original publication in this journal is cited, in accordance with accepted academic practice. No use, distribution or reproduction is permitted which does not comply with these terms.
*Correspondence: Sergi Díez, c2VyZ2kuZGllemxAaWRhZWEuY3NpYy5lcw==; José Marrugo-Negrete, am1hcnJ1Z29AY29ycmVvLnVuaWNvcmRvYmEuZWR1LmNv