- 1Laboratoire d’Océanographie de Villefranche-sur-Mer (LOV), UMR 7093, CNRS, Sorbonne Université, Villefranche-sur-Mer, France
- 2Laboratoire de Géologie de Lyon, Terre, Planètes, Environnement (LGL-TPE), UMR 5276, CNRS, Université Claude Bernard, Lyon1/Ecole Normale Supérieure de Lyon, Villeurbanne, France
- 3Littoral Environnement et Sociétés (LIENSs), UMR 7266 CNRS- La Rochelle Université, La Rochelle, France
- 4Centre d’Etudes Biologiques de Chizé (CEBC), UMR 7372 CNRS—La Rochelle Université, Villiers-en-Bois, France
- 5Institut Universitaire de France (IUF), Paris, France
Non-conventional stable isotopes have received increasing attention in the past decade to investigate multi-level ecological connections from individuals to ecosystems. More recently, isotopes from trace and non-nutrient elements, potentially toxic (i.e., Hg), have also been recognized of great significance to discriminate sources, transports, and bioaccumulation, as well as trophic transfers. In contrast, lithium (Li) concentrations and its isotope compositions (δ7Li) remain poorly documented in aquatic ecosystems, despite its possible accumulation in marine organisms, its increasing industrial production, and its demonstrated hazardous effects on biota. Here, we present the first Li isotope investigation of various soft tissues, organs or whole organisms, from marine plankton, bivalves, cephalopods, crustaceans, and fish of different biogeographical regions [North Mediterranean Sea, North Atlantic Ocean (Bay of Biscay), South East Pacific Ocean (New Caledonia), and Southern Indian Ocean (Kerguelen Islands)]. Independently of the considered organisms, δ7Li values range widely, from 4.6‰ (digestive gland of bivalves) to 32.0‰ (zooplankton). Compared to homogeneous seawater (δ7Li ∼ 31.2‰ ± .3‰), marine organisms mostly fractionate Li isotopes in favor of the light isotope (6Li). Within the same taxonomic group, significant differences are observed among organs, indicating a key role of physiology on Li concentrations and on the distribution of Li isotopes. Statistically, the trophic position is only slightly related to the average Li isotope composition of soft tissues of marine organisms, but this aspect deserves further investigation at the organ level. Other potential influences are the Li uptake by ingestion or gill ventilation. Overall, this work constitutes the first δ7Li extensive baseline in soft tissues of coastal organisms from different large geographic areas mostly preserved from significant anthropogenic Li contamination.
1 Introduction
Traditional stable isotopes of light elements (C, N, O, and S) have been intensively used in the past decades in marine organisms to study the dynamics of trophic webs and to characterize the diet of marine predators (Wada et al., 1991; Hobson et al., 1995; Bustamante and Branch, 1996; Jennings et al., 1997; Connolly et al., 2004). Recently, these questions have started to be investigated using non-traditional isotopes, e.g., Cu, Hg, Zn (e.g., Jaouen et al., 2016; Briant et al., 2019; Köbberich and Vance, 2019; Nitzsche et al., 2020). Stable isotopes of trace metals are emergently used as biomonitoring tools for tracing their sources (Kwon et al., 2015; Xu and Wang, 2017), pollution effects (Araújo et al., 2022; Ma et al., 2019; Bonsignore et al., 2020), physiological investigations (Balter and Vigier, 2014; Bolea-Fernandez et al., 2019; Manceau et al., 2021; Renedo et al., 2021; Queipo-Abad et al., 2022), species ecology (Martin et al., 2015; McCormack et al., 2022), and studying their modern and past biogeochemical cycles (Blum et al., 2013).
Yet, despite the exponential use of lithium (Li) in high-tech, ceramics/glass, and medication industries and its low recycling rate (Swain, 2017), Li has received little attention in marine and coastal organisms. A recent study highlights Li pollution in an urban area, the megalopolis of Seoul, and shows that Li isotopes measured in river water can help to quantify Li contamination (Choi et al., 2019). It remains unknown how Li influences aquatic species and ecosystems while filtering organisms are able to bioaccumulate it (Figueroa et al., 2013; Thibon et al., 2021a). Its toxicity has been evidenced for small crustaceans (Kszos et al., 2003). However, biologically, Li is also known to bind with selective DNA (Kuznetsov et al., 1971) and may involve in DNA synthesis and repair. In a free-living nematode model (C. elelgans), Li excess decreased growth, maturation and reproductive capacity (Shahzad et al., 2017). Although Li metabolism is still not well characterized, some authors recently highlighted its benefit to organisms (Schrauzer and Shrestha, 1990; Ohgami et al., 2009; Giotakos et al., 2013; Liaugaudaite et al., 2017), and some of them even recommended for humans a daily Li intake of 14.3 µg kg−1 (Schrauzer, 2002). A recent review precisely describes Li proteome, transcriptome, and metabolome highlighting the role of Li for some gene expression, mitochondrial function, and its influence on other cellular processes such as neural communication and cell proliferation (Roux and Dosseto, 2017). Li is mainly present as a free ion within cells, and transported through specific ions channels such as Na-H exchangers (Counillon et al., 2016). Exploring Li isotopes in marine soft tissues will help better understand Li behavior in the food webs and the underlying mechanisms behind Li accumulation, transport, and toxicity. As for a terrestrial mammal (Balter and Vigier, 2014), they also may lead to a better understanding of Li physiology. Only a few works report Li concentration in marine food webs (Figueroa et al., 2013; Thibon and Weppe et al., 2021c), while Li isotopic variations in soft tissues of marine organisms are observed in one experimental study (Thibon et al., 2021a). These first studies highlight the biodilution of Li concentrations through the trophic webs, and suggest the potential of Li isotopes to be used in coastal pollution surveys.
In contrast, in geosciences, Li isotopes have been widely measured in many different carbonated minerals formed biogenically or inorganically, as they are used for reconstructing the isotope evolution of past oceans. δ7Li values of seawater is vertically and horizontally homogeneous (δ7Li ∼ 31.2‰ ± .3‰), but has varied through geological timescales (Misra and Froelich, 2012). The δ7Li record in past oceanic sediments has been used as a paleo-oceanographic proxy to investigate the relationships between past climates and continental weathering (Hall et al., 2005; Hathorne and James, 2006; Misra and Froelich, 2012; Pogge von Strandmann et al., 2020; Washington et al., 2020; Kalderon-Asael et al., 2021). While most palaeoceanographic reconstructions assume negligible, or constant, vital effects (no influence of biology on the carbonate Li isotope signature during its formation), experimental studies do show an influence of environmental parameters during foraminifera growth (ocean pH, dissolved inorganic carbon) (Vigier et al., 2015; Roberts et al., 2018). However, published Li isotope compositions of modern bivalve shells, foraminifera calcite, or corals aragonite are scarce, and their interpretation remains debated (Vigier et al., 2007; Vigier et al., 2015; Rollion-Bard et al., 2009; Misra and Froelich, 2012; Thébault and Chauvaud, 2012; Dellinger et al., 2018; Roberts et al., 2018). It is, therefore, of interest to document the role of biology and ecology on Li isotope compositions of marine organisms, and we propose here to evaluate these effects based on the study of soft tissues.
The aims of the present study are 1) to quantify the isotopic variations among marine organisms from different trophic groups, and 2) to identify the ecological or environmental factors driving Li biological isotope fractionation in the marine environment. We performed 78 measurements of Li isotopic ratios of soft tissues across different marine groups (zooplankton, bivalves, cephalopods, crustaceans, and fish) from biogeographically contrasted zones represented by different oceans (tropical: New Caledonia; temperate: Bay of Biscay and North Mediterranean; subantarctic: Kerguelen Islands). When possible (i.e., depending on Li concentration and the amount of available material), we analyzed various organs (brain, digestive gland (invertebrates), gonads, kidneys, liver (fish), heart, and muscles) from 15 species.
2 Materials and methods
2.1 Sampling and sample preparation
The analyzed organisms included zooplankton, mollusks [bivalves—Crassostrea gigas (n = 9 individuals), Pecten maximus (n = 4)—and cephalopods—Loligo vulgaris (n = 3), Onykia ingens (n = 3), Sepioteuthis lessoniana (n = 3)], crustaceans [Malacostraca—Maja brachydactyla (n = 3), Litopenaeus stylirostris (n = 3)], and fish [Chondrichthyes and Actinopterygii—Lophius piscatorius (n = 2), Merluccius merluccius (n = 2), Sardina pilchardus (n = 2), Scyliorhinus canicula (n = 2), Solea solea (n = 2), Spondyliosoma cantharus (n = 2), Zeus faber (n = 2), Plectropomus leopardus (n = 2)]. These were divided into trophic groups to assess the influence of food and feeding ecology characteristics on Li bioaccumulation and Li isotopy. We defined the trophic groups according to feeding mode (filter-feeders, grazers/scavengers, predators) and the preferred predator diet (i.e., invertebrates, small fish, or both) (Metian et al., 2013; Cipro et al., 2018). For precision on the ecology of the studied species, see Supplementary Table S1 and previous work (Bustamante et al., 2003; Bustamante and Miramand, 2005; Chouvelon et al., 2009; Chouvelon et al., 2012; Metian et al., 2013; Cipro et al., 2018; Noger-Huet et al., 2022).
Fifty-eight of the 78 samples were previously characterized in Li concentration (Thibon and Weppe et al., 2021c) and selected from distinct biogeographic areas: the Bay of Villefranche-sur-Mer (North Mediterranean sea—2020), the Bay of Biscay (northeast Atlantic Ocean—2018), New Caledonia (western tropical Pacific Ocean—2007), and the Kerguelen Islands (southern Indian Ocean—1998). The remaining 30 samples consist of six zooplankton samples and 14 fish organs sampled from 2 John Dory. These two fish were collected in the Bay of Biscay in 2020. Zooplankton was collected on 12 March 2020 (9:30–10:30a.m.) from the outer part of the Villefranche-sur-Mer Bay using the CNRS Sagitta-III boat. To sample all the zooplanktonic community, we used a WP2 net of 200 µm size along a bottom-surface (75–0 m) transect. We then sub-sampled the planktonic community >500 µm with appropriate sieves. In these coastal Mediterranean waters and at this period of the year, zooplankton from 200 to 500 µm are mainly constituted by filter-feeder copepods (Clausocalanids), whereas zooplankton >500 µm are mainly carnivorous copepods (Candacia spp.) and jellyfishes (gelatinous zooplankton) (Seguin, 1981; Romagnan et al., 2015; Nival et al., 2021). Concerning bivalves, cephalopods, and fish, some individuals were dissected in a clean laboratory to sample specific organs (dorsal muscle, liver, kidneys, gills, and brain for fish; adductor muscle, gonads, digestive gland, and gills for bivalves; mantle muscle and digestive gland for cephalopods). Tissue samples were stored in clean individual plastic bags, fresh weighed (wet weight—ww), and put at −20°C until being freeze-dried for 48 h. After drying, samples were again weighed (dry weight—dw), ground to a fine powder with a porcelain mortar and pestle, and stored in clean individual plastic vials. For more details on bivalves, crustaceans, cephalopods and fish sampling and sample preparation, see Thibon and Weppe et al. (2021c).
2.2 Chemical preparation
All analytical preparations were performed in a pressurized clean laboratory under a fume-hood to minimize procedural blanks using ultra pure reagents (HCl, HNO3, and Milli-Q water) and pre-cleaned vessels. The samples were first dissolved with a mixture of concentrated HNO3 (64%—3 mL) and H2O2 (32%—.4 mL). After 48 h, concentrated HCl (37%—1.5 mL) was added to pursue the digestion in inverse aqua regia, and heated for 24 h before being evaporated. A second step of inverse aqua regia ensured total digestion.
Prior to Li isotopic analyses, the Li in the samples must be isolated. Lithium extraction and purification were performed following the procedures of Vigier et al. (2008, 2009). Sample aliquots of ∼30 mg were dried out and taken up in .5 mL of 1 N HCl and loaded on AG 50-X12 (200–400 mesh) cationic resin in 8.5 cm-high Teflon columns. Lithium was eluted and purified with 1 N HCl. This fraction was evaporated to dryness before the analysis. Five total procedural blanks, five replicates of seawater standard solution (SW-1G, Thibon et al., 2021b) were included, as well as several biological materials, allowing us to evaluate the precision and accuracy of the overall procedure (Thibon et al., 2021b).
2.3 Analytical measurements
Lithium has two naturally occurring isotopes, one heavy (7Li, 92.41% abundance) and one light (6Li, 7.59% abundance). The isotope compositions of geological and biological materials are reported as per-mil variations relative to an international isotopic standard (NIST RM 8545 reference material, known as LSVEC) as δ7Lisample [‰] = [((Rsample/RLSVEC)−1) × 1000], where R is the corresponding ratio (7Li/6Li) in the sample and in the international standard. Li isotopic analyses were performed using a multi-collector inductively coupled plasma mass spectrometer (Thermo-Fisher Neptune Plus at the CNRS-INSU Facilities, École Normale Supérieure de Lyon, France) following the procedure developed for low Li concentrations in biological materials (Balter and Vigier, 2014).
Samples and standards were analyzed under dry plasma conditions in low-resolution mode. The standard bracketing method (using the LSVEC reference material) was used to performed instrumental mass bias correction. The five total Li procedural blanks, ranged between 7 and 22 pg, with an average of 13 pg (n = 5). For 30 mg of material with a Li mass fraction of 1 μg/g, the maximum blank contribution is negligible (<.07%). On average, the internal reproducibility (within-run precision) was ±.04‰. Between-run-precision (external reproducibility) was estimated from two to five replicate measurements of five different aliquots, and are about ±.3‰, except for DORM-2 and TORT-2 references, which display Li concentrations lower than .2 mg/g (Thibon et al., 2021b). The accuracy of this method has been confirmed by a standard addition method applied to fish muscle reference material (Thibon et al., 2021b). Seawater standard solution (SW-1G: EDMM-1-G from Analytical Environnemental Laboratory), previously published at 31.3‰ ± .8‰ (n = 4, 2 s) (Thibon et al., 2021b), has been measured here at 30.8‰ ± .5‰ (n = 5, 2 s), which confirmed the absence of measurements bias.
Lithium concentrations were previously published (Thibon and Weppe et al., 2021c), and determined at the LIENSs laboratory using an Inductively Coupled Plasma Mass Spectrometer (Thermo Fisher X-Series II). For samples collected in the Bay of Biscay, concentrations of several trace metals (Ag, As, Cd, Co, Cr, Cu, Fe, Hg, Mn, Ni, Pb, Se, V, and Zn) were also performed at LIENSs using the same instrument or a Varian Pro Inductively Coupled Plasma Optical Emission Spectrometer and, only for the Hg, a Altec AMA254 Atomic Absorption spectroscopy (Noger-Huet et al., 2022).
2.4 Statistical treatments
Since normality and homoscedasticity of the Li compositional and isotopic data were not validated, we adopted non-parametric statistical tests to compare population of more than two samples. The Mann-Whitney-Wilcoxon and Kruskal-Wallis tests were used to compare median values between two or more observational series, respectively. Tests were performed using R software (R Core Team, 2017) using the functions wilcox.test or kruskal.test. The level of significance for statistical analyses was set at p-value < .05.
A multifactor ANOVA has been performed using R software (R Core Team, 2017) on the whole dataset to test whether the δ7Li (bcPower-transformed to approach normality and homoscedasticity) is influenced by any of the environmental and ecological factors investigated or by an interaction of the factors.
In addition, the relationship between quantitative variables was investigated using principal component analysis (PCA, see Section 4.2), a statistical tool that reduces the number of original quantitative variables to fewer dimensions that may explain the majority of the observed variability. Here, we performed a PCA on two groups (fish and bivalves from the Bay of Biscay), considering the following initial variables: As, Co, Hg, Li, and Zn concentrations, which include nutrients, non-nutrients, moderately to highly toxic elements, and δ7Li.
3 Results
3.1 Results for tissues separated by taxonomic group and geographical origin
Marine organisms display a wide range of δ7Li values, from 4.6‰ (digestive gland of bivalves from the Bay of Biscay) to 32.0‰ (zooplankton from the Bay of Villefranche-sur-Mer; Table 1, Supplementary Table S1 and Figure 1). There is no visible simple correlation with a single environmental or ecological parameter.
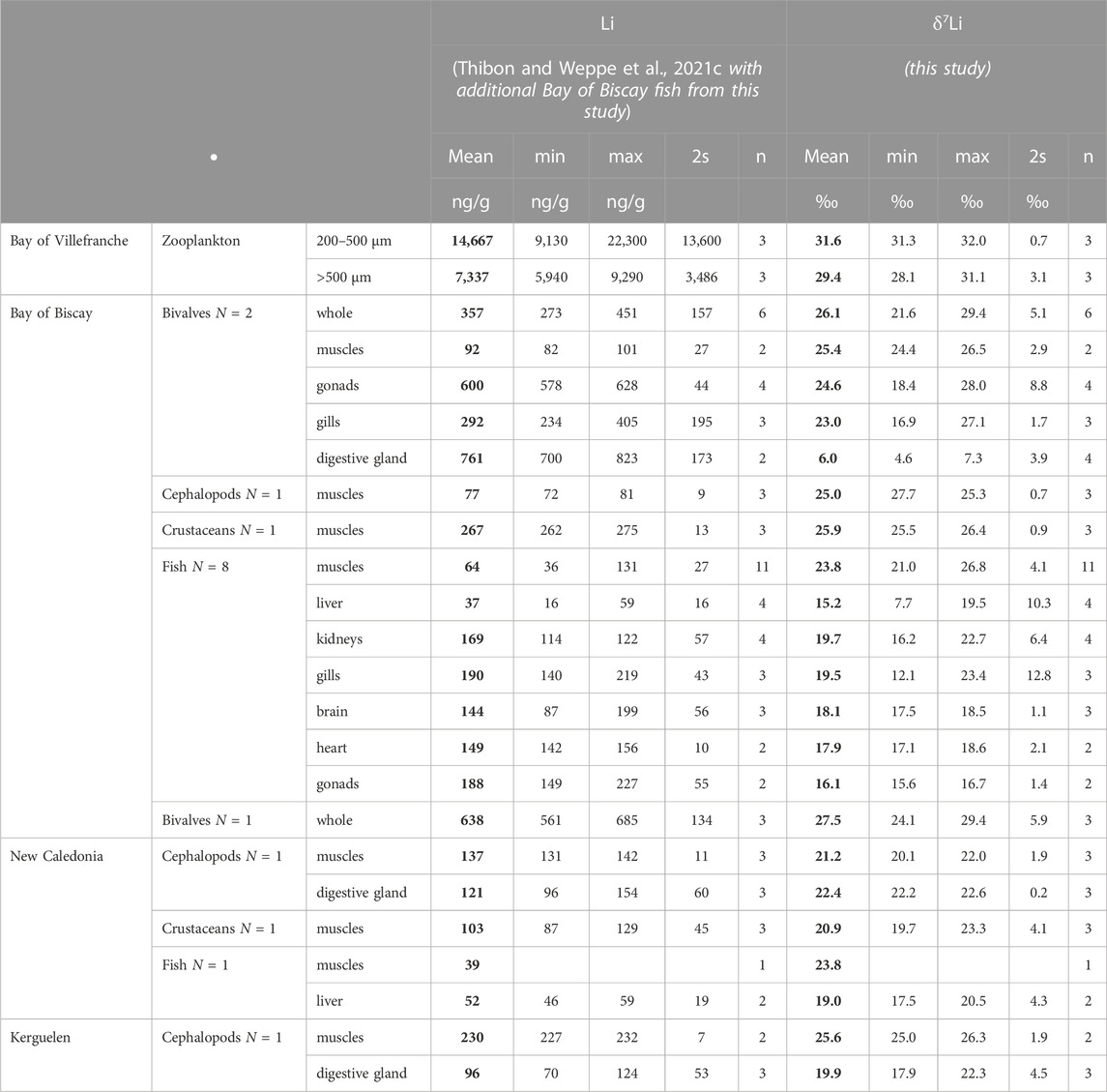
TABLE 1. Lithium mass fractions and its isotope compositions of the collected samples. The mean (in bold), minimum, maximum, and twice the standard deviation (2s) are reported. “N” indicates the number of species, “n” indicates the number of individuals for bivalves, cephalopods, crustaceans, and fish, and the number of samples for plankton.
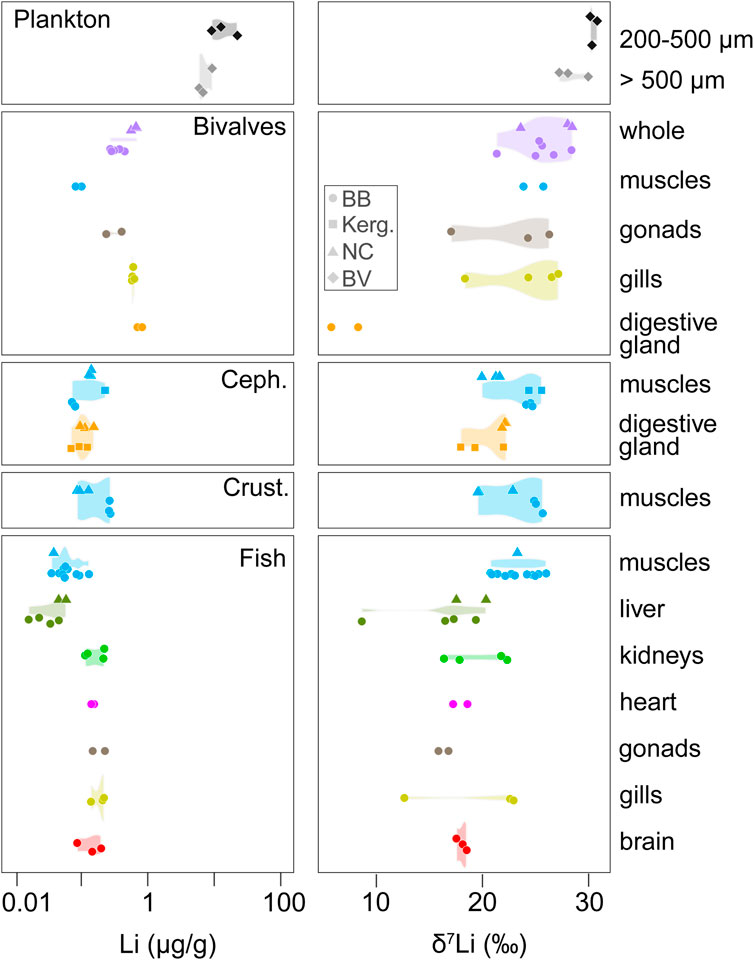
FIGURE 1. Li concentrations and Li isotope compositions measured in the whole body (“whole”) and different tissues of marine organisms. Abbreviations: Crus., crustaceans; Ceph., cephalopods. Li concentrations are from (Thibon and Weppe et al., 2021c), except for zooplankton (this study).
For zooplankton and bivalves, whole organisms were analyzed. Zooplankton from 200 to 500 µm in size display δ7Li values close to the seawater one (31.2‰), ranging from 31.3‰ to 32.0‰. Zooplankton larger than 500 µm in size display slightly lower values compared to seawater, from 28.1‰ to 31.1‰, although not significantly different than the smaller size one on average (Wilcoxon test: p-val. > .05). Bivalves (without shells) display similar δ7Li average values in the Bay of Biscay and in New Caledonia (26.1‰ ± 5.1‰ and 27.5‰ ± 5.9‰ respectively). In the bivalves from Bay of Biscay, muscles, gonads, and gills display similar average δ7Li values (muscles: 25.4‰ ± 2.9‰, n = 2; gonads: 24.6‰ ± 8.8‰, n = 3; gills: 23.0‰ ± 1.7‰, n = 3, Table 1). In contrast, digestive glands display the most fractionated δ7Li value (6.0‰ ± 3.9‰, n = 2). For cephalopods, muscles and digestive gland were analyzed separately. Muscle δ7Li values are 25.0‰ ± .7‰ (n = 3), and 25.6‰ ± 1.9‰ (n = 3) in the Bay of Biscay and in the Kerguelen Islands, respectively (Wilcoxon test: p-val. > .05). In New Caledonia, cephalopod muscles are slightly lower than those from the other studied regions (21.2‰ ± 1.9‰, n = 3), although not significantly (Wilcoxon test: p-val. > .05). The digestive gland of cephalopods from New Caledonia (22.4‰ ± .2‰, n = 3) is not isotopically fractionated compared to the muscles (Wilcoxon test: p-val. > .05), in contrast to bivalves. In the Kerguelen Island, the digestive gland average δ7Li value (19.9‰ ± 4.5‰, n = 3) is lower than that in muscles (Table 1), the same feature as observed in bivalves from the Bay of Biscay. In crustaceans, δ7Li values were measured in muscles only, from both the Bay of Biscay and New Caledonia individuals, and they show non-significant differences (25.9‰ ± .9‰, n = 3 vs. 20.9‰ ± 4.1‰, n = 3, respectively, Wilcoxon test: p-val. > .05).
For fish, we analyzed Li isotope composition of various organs from samples collected in the Bay of Biscay (muscles, kidneys, gills, brain, heart, and gonads). Liver and muscles from fish collected in New Caledonia were also analyzed for comparison. In the Bay of Biscay, muscles depict an average δ7Li value of 23.8‰ ± 4.1‰ (n = 11), similar to the value determined in a New Caledonia fish, although only one data has been collected (n = 1). Bay of Biscay and New Caledonia fish livers have lower δ7Li values than corresponding muscles (15.2‰ ± 10.3‰, n = 4, and 19.0‰ ± 4.3‰, n = 2, respectively, Table 1). Kidneys, gills, brain, heart, and gonads also display lower δ7Li values when compared to muscles, i.e., 19.7‰ ± 6.4‰ (n = 4), 19.5‰ ± 12.8‰ (n = 3), 18.1‰ ± 1.1‰ (n = 3), 17.9‰ ± 2.1‰ (n = 2), and 16.1‰ ± 1.4‰ (n = 2), respectively, the lowest value being measured in gills (12.1‰).
3.2 Comparing muscles and gills at the Bay of Biscay and in New Caledonia
All taxonomic groups combined (bivalves, crustaceans, cephalopods and fish), muscles from the Bay of Biscay and Kerguelen species present higher δ7Li than muscles from New Caledonia species (24.5‰ ± 3.6‰, n = 19, and 25.6‰ ± 1.9‰, n = 2, vs. 21.5‰ ± 3.3‰, n = 7, respectively) (Table 1). In contrast, whole bivalves (without shells) do not depict any difference between individuals from the Bay of Biscay and New Caledonia (26.1‰ ± 5.1‰, n = 6, vs. 27.5‰ ± 5.9‰, n = 3, respectively, Wilcoxon test: p-val. > .05, Table 1; Figure 1).
Within a single location, muscles from the different taxonomic groups display similar average δ7Li values (Table 1): 25.4‰ ± 2.9‰ (n = 2), 25.9‰ ± .9‰ (n = 3), 25.0‰ ± .7‰ (n = 3), 23.8‰ ± 4.1‰ (n = 11) for bivalves, crustaceans, cephalopods and fish from the Bay of Biscay, and 20.9‰ ± 4.1‰ (n = 3), 21.2‰ ± 1.9‰ (n = 3), 23.8‰ (n = 1), for crustaceans, cephalopods and fish from New Caledonia, (see also Table 1; Figure 1). This feature is not observed for gills, which display distinct isotope compositions in bivalves and fish from the Bay of Biscay (23.0‰ ± 1.7‰, n = 3, vs. 19.5‰ ± 12.8‰, n = 3), but with a high variability associated to a low number of samples.
3.3 Principal component analysis
To help understand Li behaviour in marine biological systems, the organisms Li concentrations and Li isotopic distribution can be compared to those of other metals measured in the same tissues—using a principal component analysis—PCA applied to the Bay of Biscay. The PCA is performed on two groups (fish and bivalves, all tissues), considering the following initial variables: As, Co, Hg, Li, and Zn concentration and δ7Li (Thibon and Weppe et al., 2021c; Noger-Huet et al., 2022). Variables and individuals are plotted on the space constituted by the first two components—PC1 and PC2—in Figure 2. The two first components together represent 87% and 67% of the dataset variability for bivalves and fish, respectively. This implies that PC1 and PC2 are sufficient to explain the majority of the dataset variability. According to their position on PC1-PC2 space, variables can be split into two groups for bivalves (As, Hg, and Zn vs. Co, Li, and δ7Li), whereas the separation is not straightforward for fish. The first two components are adequate to fully discriminate the different tissues sampled. In bivalves, Co correlates with Li (r = .75) and anti-correlates with δ7Li (r = −.66), whereas it is not the case for fish (Supplementary Figure S1).
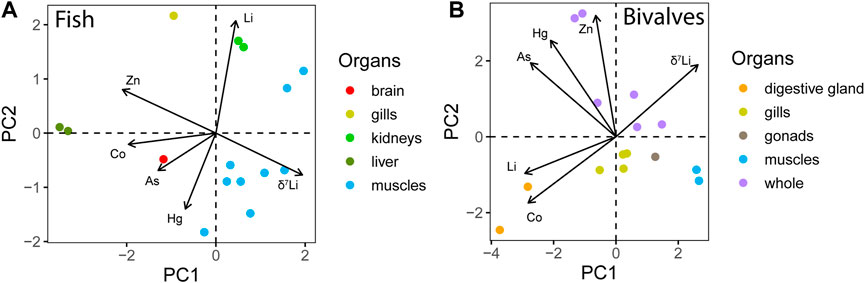
FIGURE 2. Principal component analysis (PCA) of all fish (A) and bivalves (B) from the Bay of Biscay. PC1 and PC2 are principal components 1 and 2. Arrow length is proportional to the percentage contribution of these variables to the first two principal components. PC1 and PC2 together represent (A) 67% and (B) 87% of the dataset variability for fish (A) and bivalves (B). Variables can be split into two groups for Bivalves (As, Hg, and Zn vs. Co, Li, and δ7Li), whereas the separation is not straightforward for fish. Note that the correlation between Co and Li is exhibited in the Supplementary Figure S1).
3.4 Multifactor analysis
To evaluate the percentage of δ7Li variability that environmental and ecological factors can explain, we performed a linear model on δ7Li, bcPower-transformed to approach normality and homoscedasticity. This model reports an adjusted R-squared around 60%, indicating that the studied factors cannot explain 40% of δ7Li variability. Further studies are therefore needed to consider additional extrinsic parameters (environmental ones such as pH, salinity, climate conditions, presence of contaminants, season), and intrinsic parameters (e.g., organisms’ sex, age, reproductive status).
Nevertheless, since 60% of δ7Li variability can be explained, a multifactor ANOVA has been performed on the whole dataset, using R software (R Core Team, 2017), to test whether the δ7Li is influenced by either any of the factors investigated or by an interaction of these latter. The ANOVA results show that the variability of δ7Li is statistically impacted by both the type of sampled tissue and the taxonomic group (p-val. < .001) and at a smaller level by the habitat (pelagic—demersal—benthic) (p-val. < .01) and the trophic group (p-val. < .05). On top of that, there is a significant interaction between the tissue and the location (p-val. < .001). Therefore, their combined effect is stronger than the effect of these latter factors considered separately. We further discuss the impact of tissue and habitat on Li isotopes fractionation in the following sections.
4 Discussion
4.1 Relationship between the trophic group, Li concentration and δ7Li
As described in the introduction, the studied samples evidence that Li concentrations in marine organisms follow a biodiminution effect along the food webs, from filter-feeders to predatory fish, i.e., Li concentrations decrease from species of low trophic level to the ones of high trophic level (Thibon and Weppe et al., 2021c). Our study adds to this observation the first Li concentrations of zooplankton samples and these data confirm the biodiminution effect previously highlighted. Indeed, zooplankton displays Li concentrations from 5.9 to 22.3 µg/g, i.e., at least one order of magnitude higher compared to those of bivalves and all other groups analyzed so far (Li = .01–1.20 µg/g, Thibon and Weppe et al., 2021c). The fact that filter-feeders display higher Li concentrations than predators has been interpreted as a major role of filtration on Li uptake and bioaccumulation pathway in this group.
Concerning Li isotopes, we find that δ7Li values measured in zooplankton (28.1‰–32.0‰) are higher and statistically different from the other taxonomic groups (Wilcoxon test: p-val. < .01). They are also not different from the seawater value that is homogenous at the world ocean scale (31.0‰ ± .3‰, Millot et al., 2004; Carignan et al., 2007). For the other groups, their average δ7Li values are lower than seawater, meaning that they are enriched in the light 6Li isotope compared to their environment (Table 1). Although not always significant, their δ7Li values seem to decrease from the trophic groups representing low trophic levels—i.e., plankton—to trophic groups representing high trophic levels—i.e., PSM (Table 1; Figure 3). It can also be noticed that the maximum δ7Li value of each trophic group decreases from zooplankton to bivalves, and to crustaceans, cephalopods, and fish, suggesting a potential role of the trophic level on Li isotopes that would deserve to be further investigated. The Li isotopic variability among each trophic groups is however high and might be explained by either additional physiological effects or environmental factors.
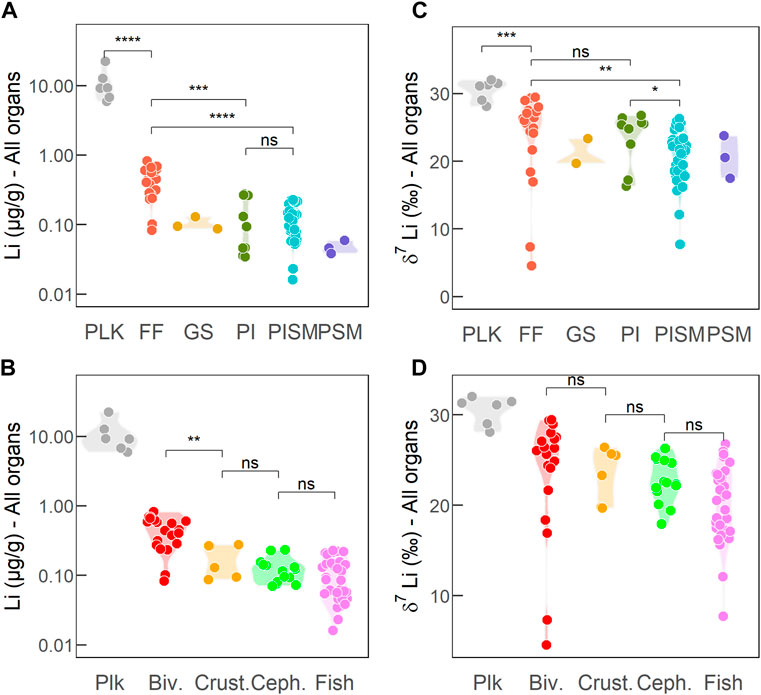
FIGURE 3. Li concentrations (A,B) and Li isotope compositions (C,D) in the whole body (ȁwhole”) and different tissues of marine organisms vs. (B,D) taxonomic groups and (A,C) trophic groups. Abbreviations: Plk, plankton, Biv., bivalves; Crust., crustaceans; Ceph., cephalopods. Trophic groups are: FF, filter-feeders; GS, grazers/scavengers; PI, predators of invertebrates; PISM, predators of invertebrates and small fish; PSM, predators of small fish. Li concentrations are from Thibon and Weppe et al., 2021c, except for zooplankton (this study).
4.2 Physiology of Li isotopes
Our dataset shows that Li isotopes are not homogeneously distributed among organs and tissues. Indeed, the various organs and tissues display a large range of δ7Li values (Figure 1), implying a crucial role of physiology in Li isotope fractionation. However, the Li physiology itself in the marine environment is little known (Thibon and Weppe et al., 2021c). Lithium intake, accumulation, and elimination may fractionate Li isotopes, as it has been indicated by cultured bivalves (Thibon et al., 2021a). These processes may vary differently according to the species, tissue physiological function, and environmental parameters. For instance, clams and mussels are able to bioaccumulate Li (Figueroa et al., 2013; Thibon et al., 2021a) and this is accompanied by an enrichment in 7Li. Li has long been considered as a non-essential element for the organisms, in contrast to micronutrients such as Zn and Cu. Nevertheless, Li essentiality and functions are currently debated even for human beings for whom the number of studies is consequent, in relation with its role in the brain and the toxic risks for treated bipolar patients (e.g., Schrauzer, 2002; Roux and Dosseto, 2017; Shahzad et al., 2017). It is known that active transporters present in all living organisms play a key role in the Li transfer to cells and organs (Counillon et al., 2016). Kinetic biological Li isotope fractionation have recently been highlighted (Thibon et al., 2021a; Poet et al., 2022) during active Li transfer, along with the intracellular pH and Na regulation, which influences the Li levels in cells and in specific organs (e.g., kidneys, brain).
When considering only bivalves and fish from the Bay of Biscay, the combination of Li concentrations and Li isotopes allows to fully discriminate tissues (Figure 4), which strongly suggests that Li is involved in distinct paths and functions among these internal components. The high variability observed within fish tissues reflects a species effect. Indeed, the four kidneys’ samples depict two groups corresponding to two different species (monk fish L. piscatorius and John Dory Z. faber), although these species have distinct trophic ecology (in the Bay of Biscay, Figure 4).
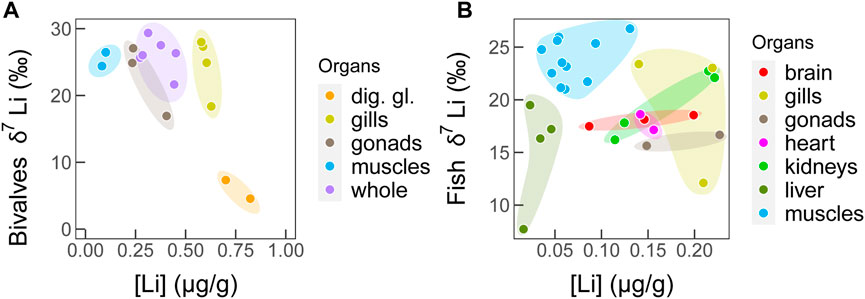
FIGURE 4. Li isotope compositions vs. tissue Li concentration for (A) bivalves, and (B) fish tissues from the Bay of Biscay. Colors code for each organ.
We selected two individuals from the same fish species (John Dory—Z. faber), from which all organs were analysed (Figure 5; Supplementary Table S1). At first, results confirm significant δ7Li heterogeneity between tissues since the values range from 7.7‰ to 23.4‰, similar as it has been reported for one terrestrial mammal, sheep, although over a smaller range (Balter and Vigier, 2014). With the exception of the liver, the two studied individuals highlight a good similarity in their Li concentrations and δ7Li values (Figure 5A). One way to comprehend these tissue specific Li signatures is to understand how Li behaves within and between these tissues (e.g., exchange, storage, transfer, remobilisation, …). However, we do not know yet if any specific tissue governs the Li distribution in marine organisms [such as the liver for copper in humans; Kim et al. (2008)]. Liver is particularly depleted in Li, and there is not a unique tissue that strongly bioaccumulates Li within the John Dory organism. At the cellular level, Li homeostasis and regulation are controlled by fluxes through ionic cellular transporters such as Na/H exchangers (NHE, Counillon et al., 2016). Thus, brain, kidneys, heart, and gills—highly implicated with Na exchanges—can actively exchange or bioaccumulate Li in fish. Gills show the highest δ7Li values, with a Li concentration close to that of brain, gonads, and heart. Gills are in direct contact with seawater, which may explain its isotopic difference with other tissues and its high δ7Li value. On average, brain, heart, and kidneys depict similar δ7Li values, suggesting a negligible isotope fractionation of the Li internal transport from the intestine to the excretion system (Figures 5A, B), in contrast to what was observed in sheep (Balter and Vigier, 2014). Another difference is that in sheep, liver and muscles display the heaviest isotope compositions while in the John Dory, gills display higher δ7Li values than muscles. This difference of Li behavior between terrestrial mammals and fish may partly come from the primary Li intake pathways (filtration and ingestion for fish and ingestion for sheep), which may fractionate Li isotopes differently.
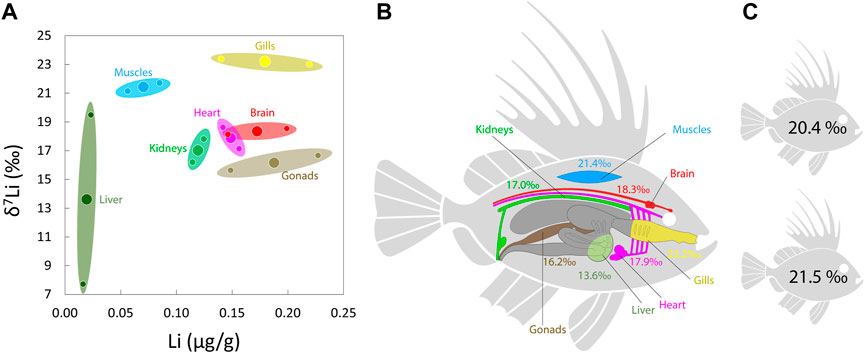
FIGURE 5. (A) Li isotope compositions vs. tissue Li concentration of two John Dory (Z. faber) fish. Large circles represent the mean of the values from the two individuals. (B) Schematic representation of organs Li isotope compositions. (C) Whole body δ7Li of the two individuals calculated from mass balance equations. Colors code for each organ.
A noticeable feature is that none of the tissues display a δ7Li value heavier than that of seawater or gills, while a Li mass balance implies that if some tissues display lower δ7Li than the Li intake, other tissues must be enriched in 7Li. This low δ7Li values may suggest that the Li intake is not dominated by a seawater-like value and therefore is not due primarily to the gills filtering system. Likely, a non-negligible part of the incorporated Li may come from prey with lower δ7Li values than seawater.
It is possible to calculate and compare the whole organisms δ7Li for the two John Dory using the following equation:
with i the various organs, and M the mass. These calculations result in a similar whole body δ7Li value: 20.4‰ and 21.5‰, respectively for the two individuals (Figure 5C). These two John Dory having different total body weight (.9 vs. 1.3 kg), this homogeneity of Li isotope composition of a single species supports a species-specific Li regulation, and a weak effect of metabolism, even if more individuals would be needed to confirm this observation. Both John Dory δ7Li values are fractionated by −10‰ compared to seawater, in favor of the light 6Li. This fractionation is stronger than most Li isotopic data displayed by crustaceans and mollusks muscles (Figure 3), the classical fish prey. Perhaps other prey with lower δ7Li values are an important source of Li for John Dory. Another possibility is that gills transfer Li to the fish with a strong Li isotope fractionation in favor of the 6Li, but with no effect on the δ7Li value of the gill itself. This last hypothesis would a priori be consistent with previous conclusions based on Li concentrations, but would need to be tested in laboratory-controlled conditions.
For bivalves, the different organs and tissues were analyzed in the king scallop P. maximus (Figure 1). Contrary to fish, scallop’s gills δ7Li values were similar to those of the muscles and gonads (Figure 1). This may be explained by the different volume of seawater filtered by their gills or by the different roles that gills can play between these groups. Both bivalves and fish use gills for respiration purposes, but bivalves use also gills to feed, and they filter a much larger volume of seawater. This may result in a distinct activity of the NHE transporters, explaining the observed weaker fractionation in bivalves, where gills are used for both respiration and feeding purposes. Another possible explanation is that for invertebrates, blood composition is influenced by fewer processes in contrast to vertebrate fish with bones (Li being able to incorporate bone mineralized tissues; Thibon et al., 2022, in press).
Among bivalve tissues, digestive gland displays the highest Li concentrations, with a distinct δ7Li value compared to muscle, gonad, and gills. This likely results from a higher bioaccumulation in this tissues, such as already observed for other trace metals (Bustamante and Miramand, 2004; Metian et al., 2008a; Metian et al., 2008b; Saavedra et al., 2008). However, we cannot exclude some contamination due to the potential presence of small Li-rich/low δ7Li aluminosilicate particles within the cells of the digestive gland (Chassard-Bouchaud et al., 1984; Thibon and Weppe et al., 2021c).
Finally, the results of our PCA, which includes Li and other trace metals (see Section 3.3) measured in bivalves and fish from the Bay of Biscay, highlight an intimate link between Co and Li, and a major role of digestive gland on their homeostasis (Figure 2; Section 3.3). As shown in Supplementary Figure S1, digestive gland of scallops is distinct from the other bivalve tissues, with a higher Li concentration and a particularly lower δ7Li value, suggesting a major role of this organ in both Co and Li metabolism. This also suggests a role of digestive glands in Li and Co detoxification, by storage or excretion (Bustamante et al., 2000). In contrast, in fish, Li and kidneys are close in the PCA, suggesting a key role of this organ in the Li regulation. However, in Figures 1, 4B, Li concentrations are low in all fish tissues, and kidneys do not display a distinct Li concentration nor Li isotope composition compared to other fish tissues. Overall, these results support distinct Li regulation pathways between those two taxonomic groups, and the Li isotope variability among tissues is higher in the invertebrate while their Li concentrations are higher.
4.3 Role of ecological and environmental factors
It is possible—as a first approximation—to consider muscles only, from all taxonomic groups combined, in order to examine the effect of environmental factors on δ7Li (Supplementary Figure S2). Looking at the role of habitat, δ7Li of muscles from the Bay of Biscay organisms does not appear to be different between demersal, pelagic, and benthic species (Supplementary Figure S2b). This suggests a negligible role of the habitat on Li isotopes, which is consistent to what has been observed for Li concentrations (Thibon and Weppe et al., 2021c). It also implies that Li isotopes behave similarly in muscles, independently of the habitat of their host. Nevertheless, the number of individuals is limited for each habitat (n = 2–10) and more data would be needed to verify more precisely the role of this parameter.
In contrast, differences appear at a broad geographic scale. Thus, muscles of the organisms from New Caledonia display a δ7Li statistically lower than those from the two other locations (Table 1; Supplementary Figure S2). Such a geographical effect on Li isotopes is mainly triggered by cephalopods and crustaceans (Figure 1). New Caledonia is known to account for a large proportion of Co and Ni worldwide production (3% and 10% in 2016, respectively; Plaza-Toledo, 2016). However, Co and Ni mining releases other trace metals in the environment, such as Cr and Fe (Pasquet et al., 2016). Moreover, New Caledonia Co bearing minerals can also be Li-rich, such as lithiophorite (.3 wt% LiO2 and 7.9 wt% Co2O3) or asbolan-lithiophorite intermediate (.01 wt% LiO2 and 13.9 wt% Co2O3) (Llorca and Monchoux, 1991). Although Li release from Co or Ni mining has not been quantified precisely yet, mining processes may release some Li compounds, similarly to other trace metals. Therefore, the δ7Li shift of New Caledonia cephalopods and crustaceans might be related to Li-rich prey carrying local terrestrial Li isotopic signatures, generally enriched in 6Li (with low δ7Li). Minerals and rocks from the continental crust, and more particularly from mafic rocks as in New Caledonia, have a low δ7Li value, generally lower than 4‰. It is interesting to observe that this 6Li-rich signature is not recorded in fish (see Figure 1). Focusing on δ7Li in low trophic level organisms such as coastal filter-feeders or grazer/scavenger may therefore help to identify anthropogenic or geogenic contamination processes. Indeed, a recent laboratory study highlighted the capacity of Li isotopes in mussel soft tissue to track Li levels in the environment (Thibon et al., 2021a). Moreover, Li isotopes measured in an Asian river revealed their potential to identify the source of Li contamination (Choi et al., 2019).
5 Implications and conclusion
Overall, our results constitute the first baseline of Li isotope composition of organisms living in contrasted environments. This dataset put forward several observations. First, the 200–500 µm zooplankton community from the Mediterranean Sea presents δ7Li values indistinguishable from that of seawater (31.0‰ ± .5‰) and high Li bioaccumulation capacity. However, the zooplankton community is mainly represented by copepods from one single geographical location and more data appear necessary to confirm this trend as well as the potential of small zooplankton community to trace coastal Li contamination. Secondly, significant differences are observed between organs and taxonomic group, whereas the location play only a minor role on δ7Li variability.
Another observation is that the internal biological regulation of Li by marine organisms is consistent with a physiological control of Li isotopes—as suggested by Dellinger et al. (2018) from the isotope composition of shells formed by various species of mollusks, brachiopods, and echinoderms. It is therefore likely that the δ7Li variability observed in the soft tissues of marine invertebrates may influence the Li isotope compositions of their calcified parts (shells). As shown in Figure 6, the range in δ7Li values observed in biogenic carbonates (from 14.9‰ to 40.7‰, Dellinger et al., 2018) is close to that observed in marine biogenic soft tissues (from 4.6‰—digestive gland of bivalves‒ to 32.0‰—small zooplankton). This good consistency between both phases strongly suggests an intimate control of biological processes for the carbonate Li isotope composition. Several paleoenvironmental studies support that similar δ7Li values between inorganic and biogenic carbonates indicates the lack of vital effects on Li isotopes. Our dataset suggests that similarity cannot be an argument in favor of the absence of Li biogenic fractionation, since biologically active soft tissues also display δ7Li values close to those of carbonates. New models of lithium incorporation during the biomineralization process must be developed which can reconcile both datasets, in soft and biomineralized tissues.
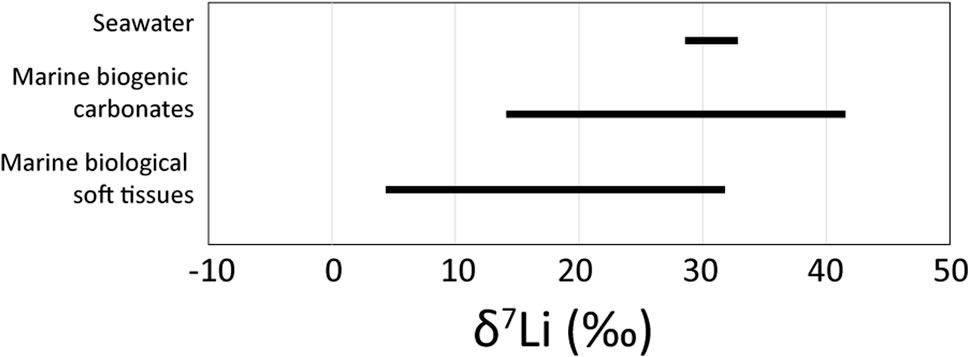
FIGURE 6. Compilation of Li isotope composition of modern marine biogenic carbonates (Dellinger et al., 2018) and soft tissues (this study). δ7Li value of modern seawater is homogeneous [31.3‰ ± .8‰–(Thibon et al., 2021b)—31.0‰ ± .5‰, Marriott et al. (2004)] and shown for comparison.
Data availability statement
The original contributions presented in the study are included in the article/Supplementary Material, further inquiries can be directed to the corresponding author.
Ethics statement
Ethical review and approval was not required for the animal study because Vertebrates and cephalopods tissues from this study are from fisheries industries.
Author contributions
FT: Conceptualization, methodology, validation, investigation, formal analysis, writing—original draft, visualization, writing—review and editing. LW: Methodology, validation, investigation, formal analysis, writing—review and editing. CC: Methodology, validation, investigation, writing—review and editing. TL-L: Resources, writing—review and editing. SG: Methodology—review and editing. YC: Resources, writing—review and editing. PB: Resources, writing—review and editing. NV: Conceptualization, supervision, project administration, writing—review and editing.
Funding
We acknowledge financial support from the ANR ISO2MET (ANR-18-CE34-0002, www.iso2met-project.fr/).
Acknowledgments
We are grateful for access to facilities provided by the laboratory LIttoral ENvironnement et Sociétés (LIENSs) and the Ecole Normale Supérieure de Lyon (ENSL, Philippe Télouk). This work benefited from the support of the PNEC Programme (Chantier Nouvelle-Calédonie), the IRD (Institut de Recherche pour le Développement), the IPEV (Institut Polaire Français Paul Emile Victor, program 109, C. Barbraud), and the TAAF (Terres Australes et Antarctiques Françaises). The authors are grateful to O. Pringault (IRD-Nouméa) for his help in organizing the fieldwork in New Caledonia, and to Captain M. Clarque (IRD-Nouméa) and the IRD Diver Team for providing access to their diving equipment. We thank G. Duhamel and the crew of the RV “La Curieuse” for their help with collecting organisms in the Kerguelen Islands. We thank Michel Warnau, Marc Metian and Fernando Pedraza for their support during fieldwork in New Caledonia, and Maud Brault-Favrou and Elise Noger-Huet for their help during sample preparation. The IUF (Institut Universitaire de France) is acknowledged for its support to PB as a Senior Member.
Conflict of interest
The authors declare that the research was conducted in the absence of any commercial or financial relationships that could be construed as a potential conflict of interest.
Publisher’s note
All claims expressed in this article are solely those of the authors and do not necessarily represent those of their affiliated organizations, or those of the publisher, the editors and the reviewers. Any product that may be evaluated in this article, or claim that may be made by its manufacturer, is not guaranteed or endorsed by the publisher.
Supplementary material
The Supplementary Material for this article can be found online at: https://www.frontiersin.org/articles/10.3389/fenvc.2022.1060651/full#supplementary-material
References
Araújo, D., Knoery, J., Briant, N., Vigier, N., and Ponzevera, E. (2022). “Non-traditional” stable isotopes applied to the study of trace metal contaminants in anthropized marine environments. Mar. Pollut. Bull. 175, 113398. doi:10.1016/j.marpolbul.2022.113398
Balter, V., and Vigier, N. (2014). Natural variations of lithium isotopes in a mammalian model. Metallomics 6, 582–586. doi:10.1039/c3mt00295k
Blum, J. D., Popp, B. N., Drazen, J. C., Anela Choy, C., and Johnson, M. W. (2013). Methylmercury production below the mixed layer in the north Pacific Ocean. Nat. Geosci. 6, 879–884. doi:10.1038/ngeo1918
Bolea-Fernandez, E., Rua-Ibarz, A., Krupp, E. M., Feldmann, J., and Vanhaecke, F. (2019). High-precision isotopic analysis sheds new light on mercury metabolism in long-finned pilot whales (Globicephala melas). Sci. Rep. 9, 7262. doi:10.1038/s41598-019-43825-z
Bonsignore, M., Manta, D. S., Barsanti, M., Conte, F., Delbono, I., Horvat, M., et al. (2020). Mercury isotope signatures in sediments and marine organisms as tracers of historical industrial pollution. Chemosphere 258, 127435. doi:10.1016/j.chemosphere.2020.127435
Briant, N., Knoery, J., Chouvelon, T., Thomas, B., Ponzevera, E., Brach-Papa, C., et al. (2019). Use of Hg, C and N stable isotopes as tracers of mercury sources and transfer through temperate coastal marine food webs. in, B51I-2361. Available at: https://ui.adsabs.harvard.edu/abs/2019AGUFM.B51I2361B.
Bustamante, P., Bocher, P., Chérel, Y., Miramand, P., and Caurant, F. (2003). Distribution of trace elements in the tissues of benthic and pelagic fish from the Kerguelen Islands. Sci. Total Environ. 313, 25–39. doi:10.1016/S0048-9697(03)00265-1
Bustamante, P., Grigioni, S., Boucher-Rodoni, R., Caurant, F., and Miramand, P. (2000). Bioaccumulation of 12 trace elements in the tissues of the nautilus nautilus macromphalus from New Caledonia. Mar. Pollut. Bull. 40, 688–696. doi:10.1016/S0025-326X(00)00005-9
Bustamante, P., and Miramand, P. (2004). Interspecific and geographical variations of trace element concentrations in Pectinidae from European waters. Chemosphere 57, 1355–1362. doi:10.1016/j.chemosphere.2004.08.077
Bustamante, P., and Miramand, P. (2005). Subcellular and body distributions of 17 trace elements in the variegated scallop Chlamys varia from the French coast of the Bay of Biscay. Sci. Total Environ. 337, 59–73. doi:10.1016/j.scitotenv.2004.07.004
Bustamante, R. H., and Branch, G. M. (1996). The dependence of intertidal consumers on kelp-derived organic matter on the west coast of South Africa. J. Exp. Mar. Biol. Ecol. 196, 1–28. doi:10.1016/0022-0981(95)00093-3
Carignan, J., Vigier, N., and Millot, R. (2007). Three secondary reference materials for lithium isotope measurements: Li7-N, Li6-N and LiCl-N solutions. Geostand. Geoanalytical Res. 31, 7–12. doi:10.1111/j.1751-908X.2007.00833.x
Chassard-Bouchaud, C., Galle, P., Escaig, F., and Miyawaki, M. (1984). [Bioaccumulation of lithium by marine organisms in European, American, and asian coastal zones: Microanalytic study using secondary ion emission]. C. R. Acad. Sci. III 299, 719–724.
Choi, H.-B., Ryu, J.-S., Shin, W.-J., and Vigier, N. (2019). The impact of anthropogenic inputs on lithium content in river and tap water. Nat. Commun. 10, 5371. doi:10.1038/s41467-019-13376-y
Chouvelon, T., Spitz, J., Caurant, F., Mèndez-Fernandez, P., Autier, J., Lassus-Débat, A., et al. (2012). Enhanced bioaccumulation of mercury in deep-sea fauna from the Bay of Biscay (north-east Atlantic) in relation to trophic positions identified by analysis of carbon and nitrogen stable isotopes. Deep Sea Res. Part Oceanogr. Res. Pap. 65, 113–124. doi:10.1016/j.dsr.2012.02.010
Chouvelon, T., Warnau, M., Churlaud, C., and Bustamante, P. (2009). Hg concentrations and related risk assessment in coral reef crustaceans, molluscs and fish from New Caledonia. Environ. Pollut. 157, 331–340. doi:10.1016/j.envpol.2008.06.027
Cipro, C. V. Z., Cherel, Y., Bocher, P., Caurant, F., Miramand, P., and Bustamante, P. (2018). Trace elements in invertebrates and fish from Kerguelen waters, southern Indian Ocean. Polar Biol. 41, 175–191. doi:10.1007/s00300-017-2180-6
Connolly, R. M., Guest, M. A., Melville, A. J., and Oakes, J. M. (2004). Sulfur stable isotopes separate producers in marine food-web analysis. Oecologia 138, 161–167. doi:10.1007/s00442-003-1415-0
Counillon, L., Bouret, Y., Marchiq, I., and Pouyssegur, J. (2016). Na+/H+ antiporter (NHE1) and lactate/H+ symporters (MCTs) in pH homeostasis and cancer metabolism. Biochim. Biophys. Acta BBA-Mol. Cell Res. 1863, 2465–2480. doi:10.1016/j.bbamcr.2016.02.018
Dellinger, M., West, A. J., Paris, G., Adkins, J. F., Pogge von Strandmann, P. A. E., Ullmann, C. V., et al. (2018). The Li isotope composition of marine biogenic carbonates: Patterns and mechanisms. Geochim. Cosmochim. Acta 236, 315–335. doi:10.1016/j.gca.2018.03.014
Figueroa, L. T., Razmillic, B., Zumeata, O., Aranda, G. N., Barton, S. A., Schull, W. J., et al. (2013). Environmental lithium exposure in the north of Chile--II. Natural food sources. Biol. Trace Elem. Res. 151, 122–131. doi:10.1007/s12011-012-9543-1
Giotakos, O., Nisianakis, P., Tsouvelas, G., and Giakalou, V. (2014). (2013) - Lithium in the public water supply and suicide mortality in Greece, Biol. Trace. Elem. Res., 156, 376, 379. doi:10.1007/s12011-013-9815-4
Hall, J. M., Chan, L.-H., McDonough, W. F., and Turekian, K. K. (2005). Determination of the lithium isotopic composition of planktic foraminifera and its application as a paleo-seawater proxy. Mar. Geol. 217, 255–265. doi:10.1016/j.margeo.2004.11.015
Hathorne, E. C., and James, R. H. (2006). Temporal record of lithium in seawater: A tracer for silicate weathering? Earth Planet. Sci. Lett. 246, 393–406. doi:10.1016/j.epsl.2006.04.020
Hobson, K., Ambrose Wg, J., and Renaud, P. (1995). Sources of primary production, benthic-pelagic coupling, and trophic relationships within the Northeast Water Polynya:insights from delta13C and delta15N analysis. Mar. Ecol. Prog. Ser. 128, 1–10. doi:10.3354/meps128001
Jaouen, K., Szpak, P., and Richards, M. P. (2016). Zinc isotope ratios as indicators of diet and trophic level in arctic marine mammals. PLOS ONE 11, e0152299. doi:10.1371/journal.pone.0152299
Jennings, S., Reñones, O., Morales-Nin, B., Polunin, N. V. C., Moranta, J., and Coll, J. (1997). Spatial variation in the 15N and 13C stable isotope composition of plants, invertebrates and fishes on Mediterranean reefs: Implications for the study of trophic pathways. doi:10.3354/meps146109
Kalderon-Asael, B., Katchinoff, J. A. R., Planavsky, N. J., Hood, A. v. S., Dellinger, M., Bellefroid, E. J., et al. (2021). A lithium-isotope perspective on the evolution of carbon and silicon cycles. Nature 595, 394–398. doi:10.1038/s41586-021-03612-1
Kim, B.-E., Nevitt, T., and Thiele, D. J. (2008). Mechanisms for copper acquisition, distribution and regulation. Nat. Chem. Biol. 4, 176–185. doi:10.1038/nchembio.72
Köbberich, M., and Vance, D. (2019). Zn isotope fractionation during uptake into marine phytoplankton: Implications for oceanic zinc isotopes. Chem. Geol. 523, 154–161. doi:10.1016/j.chemgeo.2019.04.004
Kszos, L. A., Beauchamp, J. J., and Stewart, A. J. (2003). Toxicity of lithium to three freshwater organisms and the antagonistic effect of sodium. Ecotoxicology 12, 427–437. doi:10.1023/a:1026160323594
Kuznetsov, I. A., Lukanin, A. S., and Tsurkanov, L. F. (1971). Effect of alkaline metal ions on DNA secondary structure. IV. Thermal denaturation of alkaline metal deoxyribonucleates in solutions of low ionic strength. Biofizika 16, 144–146.
Kwon, S. Y., Blum, J. D., Nadelhoffer, K. J., Timothy Dvonch, J., and Tsui, M. T.-K. (2015). Isotopic study of mercury sources and transfer between a freshwater lake and adjacent forest food web. Sci. Total Environ. 532, 220–229. doi:10.1016/j.scitotenv.2015.06.012
Liaugaudaite, V., Mickuviene, N., Raskauskiene, N., Naginiene, R., and Sher, L. (2017). Lithium levels in the public drinking water supply and risk of suicide: A pilot study. J. Trace Elem. Med. Biol. Organ Soc. Min. Trace Elem. GMS 43, 197–201. doi:10.1016/j.jtemb.2017.03.009
Llorca, S., and Monchoux, P. (1991). Supergene cobalt minerals from New Caledonia. Can. Mineral. 29, 149–161.
Ma, L., Li, Y., Wang, W., Weng, N., Evans, R. D., and Wang, W.-X. (2019). Zn isotope fractionation in the oyster Crassostrea hongkongensis and implications for contaminant source tracking. Environ. Sci. Technol. 53, 6402–6409. doi:10.1021/acs.est.8b06855
Manceau, A., Brossier, R., Janssen, S. E., Rosera, T. J., Krabbenhoft, D. P., Cherel, Y., et al. (2021). Mercury isotope fractionation by internal demethylation and biomineralization reactions in seabirds: Implications for environmental mercury science. Environ. Sci. Technol. 55, 13942–13952. doi:10.1021/acs.est.1c04388
Marriott, C. S., Henderson, G. M., Belshaw, N. S., and Tudhope, A. W. (2004). Temperature dependence of δ7Li, δ44Ca and Li/Ca during growth of calcium carbonate. Earth Planet. Sci. Lett. 222. Available at: 615, 624. doi:10.1016/j.epsl.2004.02.031https://ora.ox.ac.uk/objects/uuid:da911db7-6061-4c8d-9959-241667f59bbf [Accessed October 2, 2020].
Martin, J. E., Vance, D., and Balter, V. (2015). Magnesium stable isotope ecology using mammal tooth enamel. Proc. Natl. Acad. Sci. 112, 430–435. doi:10.1073/pnas.1417792112
McCormack, J., Griffiths, M. L., Kim, S. L., Shimada, K., Karnes, M., Maisch, H., et al. (2022). Trophic position of Otodus megalodon and great white sharks through time revealed by zinc isotopes. Nat. Commun. 13, 2980. doi:10.1038/s41467-022-30528-9
Metian, M., Bustamante, P., Cosson, R. P., Hédouin, L., and Warnau, M. (2008a). Investigation of Ag in the king scallop Pecten maximus using field and laboratory approaches. J. Exp. Mar. Biol. Ecol. 367, 53–60. doi:10.1016/j.jembe.2008.08.019
Metian, M., Bustamante, P., Hédouin, L., and Warnau, M. (2008b). Accumulation of nine metals and one metalloid in the tropical scallop Comptopallium radula from coral reefs in New Caledonia. Environ. Pollut. 152, 543–552. doi:10.1016/j.envpol.2007.07.009
Metian, M., Warnau, M., Chouvelon, T., Pedraza, F., Rodriguez y Baena, A. M., and Bustamante, P. (2013). Trace element bioaccumulation in reef fish from New Caledonia: Influence of trophic groups and risk assessment for consumers. Mar. Environ. Res. 87–88, 26–36. doi:10.1016/j.marenvres.2013.03.001
Millot, R., Guerrot, C., and Vigier, N. (2004). Accurate and high-precision measurement of lithium isotopes in two reference materials by MC-ICP-MS. Geostand. Geoanalytical Res. 28, 153–159. doi:10.1111/j.1751-908X.2004.tb01052.x
Misra, S., and Froelich, P. N. (2012). Lithium isotope history of cenozoic seawater: Changes in silicate weathering and reverse weathering. Science 335, 818–823. doi:10.1126/science.1214697
Nitzsche, K. N., Shin, K.-C., Kato, Y., Kamauchi, H., Takano, S., and Tayasu, I. (2020). Magnesium and zinc stable isotopes as a new tool to understand Mg and Zn sources in stream food webs. Ecosphere 11, e03197. doi:10.1002/ecs2.3197
Nival, P., Lombard, F., Stemmann, L., Goy, J., and Cuzin, J. (2021). “Zooplancton: Macroplancton et séries à long terme,” in La mer Méditerranée face au changement global 2. Dynamique biologique et évolution de la mer Ligure, eds. C. Migon, A. Sciandra, and P. Nival (ISTE Editions Ltd), London, 115–154. Available at: https://hal.archives-ouvertes.fr/hal-03200031 [Accessed December 9, 2022].
Noger-Huet, É., Vagner, M., Le Grand, F., Graziano, N., Bideau, A., Brault-Favrou, M., et al. (2022). Risk and benefit assessment of seafood consumption harvested from the Pertuis Charentais region of France. Environ. Pollut. 292, 118388, doi:118388doi:10.1016/j.envpol.2021.118388
Ohgami, H., Terao, T., Shiotsuki, I., Ishii, N., and Iwata, N. (2009). Lithium levels in drinking water and risk of suicide. Br. J. Psychiatry J. Ment. Sci. 194, 464–465. doi:10.1192/bjp.bp.108.055798
Pasquet, C., Le Monier, P., Monna, F., Durlet, C., Brigaud, B., Losno, R., et al. (2016). Impact of nickel mining in New Caledonia assessed by compositional data analysis of lichens. SpringerPlus 5, 2022. doi:10.1186/s40064-016-3681-4
Plaza-Toledo, M. (2016). “The mineral industry of New Caledonia,” in USGS Minerals Yearbook. Reston, VA: Minerals Information Center.
Poet, M., Vigier, N., Bouret, Y., Jarretou, G., Bendahhou, S., Montanes, M., et al. (2022). Biological fractionation of lithium isotopes by cellular Na+/H+ exchangers unravels fundamental transport mechanisms. bioRxiv. doi:10.1101/2022.10.06.510772
Pogge von Strandmann, P. A. E., Kasemann, S. A., and Wimpenny, J. B. (2020). Lithium and lithium isotopes in earth’s surface cycles. Elements 16, 253–258. doi:10.2138/gselements.16.4.253
Queipo-Abad, S., Pedrero, Z., Marchán-Moreno, C., El Hanafi, K., Bérail, S., Corns, W. T., et al. (2022). New insights into the biomineralization of mercury selenide nanoparticles through stable isotope analysis in giant petrel tissues. J. Hazard. Mat. 425, 127922. doi:10.1016/j.jhazmat.2021.127922
R Core Team (2017). R: A language and environment for statistical computing. Available at: https://www.R-project.org/.
Renedo, M., Pedrero, Z., Amouroux, D., Cherel, Y., and Bustamante, P. (2021). Mercury isotopes of key tissues document mercury metabolic processes in seabirds. Chemosphere 263, 127777. doi:10.1016/j.chemosphere.2020.127777
Roberts, J., Kaczmarek, K., Langer, G., Skinner, L., Bijma, J., Bradbury, H., et al. (2018). Lithium isotopic composition of benthic foraminifera: A new proxy for paleo-pH reconstruction. Geochim. Cosmochim. Acta 236, 336–350. doi:10.1016/j.gca.2018.02.038
Rollion-Bard, C., Vigier, N., Meibom, A., Blamart, D., Reynaud, S., Rodolfo-Metalpa, R., et al. (2009). Effect of environmental conditions and skeletal ultrastructure on the Li isotopic composition of scleractinian corals. Earth Planet. Sci. Lett. 286, 63–70. doi:10.1016/j.epsl.2009.06.015
Romagnan, J.-B., Legendre, L., Guidi, L., Jamet, J.-L., Jamet, D., Mousseau, L., et al. (2015). Comprehensive model of annual plankton succession based on the whole-plankton time series approach. PLOS ONE 10, e0119219. doi:10.1371/journal.pone.0119219
Roux, M., and Dosseto, A. (2017). From direct to indirect lithium targets: A comprehensive review of omics data. Metallomics 9, 1326–1351. doi:10.1039/C7MT00203C
Saavedra, Y., González, A., and Blanco, J. (2008). Anatomical distribution of heavy metals in the scallop Pecten maximus. Food Addit. Contam. Part A 25, 1339–1344. doi:10.1080/02652030802163398
Schrauzer, G. N. (2002). Lithium: Occurrence, dietary intakes, nutritional essentiality. J. Am. Coll. Nutr. 21, 14–21. doi:10.1080/07315724.2002.10719188
Schrauzer, G. N., and Shrestha, K. P. (1990). Lithium in drinking water and the incidences of crimes, suicides, and arrests related to drug addictions. Biol. Trace Elem. Res. 25, 105–113. doi:10.1007/BF02990271
Seguin, G. (1981). Dynamique des Copépodes pélagiques en rade de Villefranche-sur-Mer à partir de prélèvements quotidiens (année 1972). Bilan quantitatif et écologique. Oceanol. Acta 4, 405–414.
Shahzad, B., Mughal, M. N., Tanveer, M., Gupta, D., and Abbas, G. (2017). Is lithium biologically an important or toxic element to living organisms? An overview. Environ. Sci. Pollut. Res. 24, 103–115. doi:10.1007/s11356-016-7898-0
Swain, B. (2017). Recovery and recycling of lithium: A review. Sep. Purif. Technol. 172, 388–403. doi:10.1016/j.seppur.2016.08.031
Thébault, J., and Chauvaud, L. (2012). Li/Ca enrichments in great scallop shells (Pecten maximus) and their relationship with phytoplankton blooms. Palaeogeogr. Palaeoclimatol. Palaeoecol. 373, 108–122. doi:10.1016/j.palaeo.2011.12.014
Thibon, F., Goedert, J., Séon, N., Weppe, L., Martin, J. E., Amiot, R., et al. (2022). The ecology of modern and fossil vertebrates revisited by lithium isotopes, Earth Planet. Sci. Lett., 599, 117840, doi:10.1016/j.epsl.2022.117840
Thibon, F., Metian, M., Oberhänsli, F., Montanes, M., Vassileva, E., Orani, A. M., et al. (2021a). Bioaccumulation of lithium isotopes in mussel soft tissues and implications for coastal environments. ACS Earth Space Chem. 5, 1407, 1417. doi:10.1021/acsearthspacechem.1c00045
Thibon, F., Weppe, L., Montanes, M., Telouk, P., and Vigier, N. (2021b). Lithium isotopic composition of reference materials of biological origin TORT-2, DORM-2, TORT-3, DORM-4, SRM-1400 and ERM-CE278k. J. Anal. At. Spectrom. 36, 1381, 1388. doi:10.1039/D1JA00045D
Thibon, F., Weppe, L., Vigier, N., Churlaud, C., Lacoue-Labarthe, T., Metian, M., et al. (2021c). Large-scale survey of lithium concentrations in marine organisms. Sci. Total Environ. 751, 141453. doi:10.1016/j.scitotenv.2020.141453
Vigier, N., Decarreau, A., Millot, R., Carignan, J., Petit, S., and France-Lanord, C. (2008). Quantifying Li isotope fractionation during smectite formation and implications for the Li cycle. Geochim. Cosmochim. Acta 72, 780–792. doi:10.1016/j.gca.2007.11.011
Vigier, N., Gislason, S. R., Burton, K. W., Millot, R., and Mokadem, F. (2009). The relationship between riverine lithium isotope composition and silicate weathering rates in Iceland. Earth Planet. Sci. Lett. 287, 434–441. doi:10.1016/j.epsl.2009.08.026
Vigier, N., Rollion-Bard, C., Levenson, Y., and Erez, J. (2015). Lithium isotopes in foraminifera shells as a novel proxy for the ocean dissolved inorganic carbon (DIC). Comptes Rendus Geosci. 347, 43–51. doi:10.1016/j.crte.2014.12.001
Vigier, N., Rollion-Bard, C., Spezzaferri, S., and Brunet, F. (2007). In situ measurements of Li isotopes in foraminifera. Geochem. Geophys. Geosystems 8. doi:10.1029/2006GC001432
Wada, E., Mizutani, H., and Minagawa, M. (1991). The use of stable isotopes for food web analysis. Crit. Rev. Food Sci. Nutr. 30, 361–371. doi:10.1080/10408399109527547
Washington, K. E., West, A. J., Kalderon-Asael, B., Katchinoff, J. A. R., Stevenson, E. I., and Planavsky, N. J. (2020). Lithium isotope composition of modern and fossilized Cenozoic brachiopods. Geology 48, 1058–1061. doi:10.1130/G47558.1
Keywords: marine organisms, trophic webs, ecotoxicology, lithium, isotopes
Citation: Thibon F, Weppe L, Churlaud C, Lacoue-Labarthe T, Gasparini S, Cherel Y, Bustamante P and Vigier N (2023) Lithium isotopes in marine food webs: Effect of ecological and environmental parameters. Front. Environ. Chem. 3:1060651. doi: 10.3389/fenvc.2022.1060651
Received: 03 October 2022; Accepted: 19 December 2022;
Published: 06 January 2023.
Edited by:
Robert Peter Mason, University of Connecticut, United StatesReviewed by:
Jong-Sik Ryu, Pukyong National University, South KoreaKlervia Jaouen, UMR5563 Géosciences Environnement Toulouse (GET), France
Copyright © 2023 Thibon, Weppe, Churlaud, Lacoue-Labarthe, Gasparini, Cherel, Bustamante and Vigier. This is an open-access article distributed under the terms of the Creative Commons Attribution License (CC BY). The use, distribution or reproduction in other forums is permitted, provided the original author(s) and the copyright owner(s) are credited and that the original publication in this journal is cited, in accordance with accepted academic practice. No use, distribution or reproduction is permitted which does not comply with these terms.
*Correspondence: Fanny Thibon, dGhpYm9uLmZhbm55QG9yYW5nZS5mcg==