- 1Department of Animal Genetics, Breeding and Reproduction, College of Animal Science, South China Agricultural University, Guangzhou, China
- 2Guangdong Provincial Key Lab of Agro-Animal Genomics and Molecular Breeding and Key Lab of Chicken Genetics, Breeding and Reproduction, Ministry of Agriculture, Guangzhou, China
Non-coding RNAs play a regulatory role in the growth and development of skeletal muscle. Our previous study suggested that gga-mir-133a-3p was a potential candidate for regulating myoblast proliferation and differentiation in skeletal muscle. The purpose of our study was to reveal the regulatory mechanism of gga-mir-133a-3p in the proliferation and differentiation of chicken myoblasts. Through the detection of cell proliferation activity, cell cycle progression and EdU, we found that gga-mir-133a-3p can significantly inhibit the proliferation of myoblasts. In the process of myogenic differentiation, gga-mir-133a-3p is up-regulated, while gga-mir-133a-3p can significantly promote the up-regulation of differentiation-related muscle-derived factors, indicating that gga-mir-133a-3p can promote the differentiation of myoblasts. Validation at the transcriptional level and protein level proved that gga-mir-133a-3p can inhibit the expression of PRRX1, and the dual-luciferase assay also showed their direct targeting relationship. Correspondingly, PRRX1 can significantly promote myoblast proliferation and inhibit myoblast differentiation. In our study, we confirmed that gga-mir-133a-3p participates in the regulation of proliferation and differentiation of myoblasts by targeting PRRX1.
Introduction
Skeletal muscle is the most important part of the body of the meat-producing animals, and its growth and development are closely related to the meat production. Skeletal muscle growth and development is a very complex biological process, subject to many signal pathways and factors of regulation (Bassel-Duby and Olson, 2006; Schiaffino et al., 2007). Myogenesis is a process in which somatic cells undergo a series of processes of proliferation, migration, differentiation, and ultimately formation of muscle tissue during embryonic development (Perry and Rudnick, 2000; Rehfeldt et al., 2000). The coordinated regulation of myogenic renewal is a major part of skeletal muscle growth and development, including myoblast proliferation, differentiation, apoptosis, and fusion (Sartorelli and Fulco, 2004). The process of myoblast proliferation and differentiation is also regulated by many signaling pathways and regulatory factors, including DNA, non-coding RNAs (ncRNAs), and peptides.
Non-coding RNAs are mainly composed of microRNAs (miRNAs), long-chain non-coding RNAs (lncRNAs), circular RNAs (circRNAs). The function and role of non-coding RNAs in the myogenic proliferation and differentiation had been characterized (Wei et al., 2013; Jia et al., 2017; Liang et al., 2018). The presence of circRNAs and lncRNAs have been confirmed in chickens, and some studies showed that circRNAs and lncRNAs can regulate the proliferation and differentiation of myoblast (Kallen et al., 2013; Ballarino et al., 2015; Wang et al., 2015; Zhuang et al., 2015; Ouyang et al., 2017; Panda et al., 2017; Wei et al., 2017). MicroRNAs are endogenous non-coding RNA molecules with a sequence of 20∼22 nt, which were identified to be the most important non-coding RNAs (Lin et al., 2012). MicroRNAs can regulate expression of target protein-coding genes at the transcription level by binding the 3′ untranslated region (3′UTR) of the target mRNAs (Lin et al., 2009). In addition, miRNAs can also inhibit translation and regulate the splicing process by binding to the 5′untranslated region (5′UTR) and the coding region of the target gene (Grey et al., 2010; Hausser et al., 2013; Chung et al., 2017; Agarwal et al., 2018; Zhang et al., 2018). The role of miRNAs in the proliferation and differentiation of myoblasts has also been characterized (Bartel, 2004; Luo et al., 2014; Shenoy and Blelloch, 2014). The study has shown that non-coding RNAs are critical for controlling myogenesis, including myoblast proliferation, differentiation, through the interaction of myogenic-related genes (Lewis et al., 2005). A recent study indicated that mir-200a can inhibit the proliferation and differentiation of myoblasts in the skeletal muscle of chicken (Jiang et al., 2017). In a similar vein, another study showed that mir-16-5p regulates p53 signaling pathways to affect myoblast proliferation apoptosis and differentiation by target to SESN1 (Cai et al., 2018). These results further identify the importance of microRNAs in the growth and development of chicken skeletal muscles.
Gga-mir-133a expressed differently in breast muscle of fast-growing and slow-growing broilers, and it was the most abundant miRNA in the breast muscle sequencing libraries (Ouyang et al., 2015). Similarly, based on our previous study (accession number GSE100321) (Jebessa et al., 2018), gga-mir-133a-3p was differentially expressed in the leg muscles between the E11 and E16 XingHua chickens. The mir-133 family includes two members, mir-133a and mir-133b, as encoded by four loci in chick. Gga-mir-133a-3p is the mature miRNA which derived from two precursor miRNAs (gga-mir-133a-1 and gga-mir-133a-2), with a mature sequence of 22nt. In the previous studies, mir-133 had the characteristic of muscle-specific expression, it regulated the process of proliferation and differentiation of skeletal muscle cells through coordinated transcriptional regulation of muscle growth, regulation of alternative splicing regulators, and regulation of growth hormone pathway regulatory factors (Chen et al., 2006; Rao et al., 2006). It has been shown that mir-133 can enhance the proliferation of mouse myoblasts by targeting SRF (Chen et al., 2006). Another study has shown that mir-133 is upregulated during myoblast differentiation (Huang et al., 2011), which suggested that gga-mir-133a-3p may also be involved in myogenic differentiation of skeletal muscle as a member of mir-133 family. Gga-mir-133 can stabilize the myogenic differentiation program in the chick embryo (Goljanek-Whysall et al., 2014). Myoblasts first proliferate and differentiate into myocytes, and then myocytes fusion into myotubes and eventually mature into muscle fibers, which is the most important process of skeletal muscle growth. In mammals or poultry, the numbers of muscle fibers have been fixed before birth or within 1 week of birth (Smith, 1963; Li et al., 2015), therefore, the growth of skeletal muscle before birth mainly depends on the proliferation and differentiation of myoblasts. In our previous study (accession number GSE100321), gga-mir-133a-3p was differentially expressed in the leg muscles between the E11 and E16 XingHua chickens, which indicated that it may participate in the proliferation and differentiation of myoblasts. However, the study of gga-mir-133a-3p in poultry is rare, especially concerning of skeletal muscle growth and development, it is still very unclear.
PRRX1 (paired related homeobox 1), a member of the Homeobox (HoX) genes, is an important gene with a higher genetic level and involves in formation of cartilage and bone, cell differentiation and morphological development (Martin et al., 1995; Ten et al., 1998; Higuchi et al., 2014). The PRRX1 gene was highly expressed during the embryonic stage and had an important influence on the development and differentiation of embryos and the development of limb structures and organs in mice (Higuchi et al., 2013). The PRRX1 gene, was mainly involved in the development of bone and cardiovascular system in the embryo, such as limbs, craniofacial, cartilage and arterial tubes (White et al., 2003; Yoshida et al., 2004). In addition, the PRRX1 gene was also differentially expressed in the leg muscles between the E11 and E16 XingHua chickens in our previous study (accession number: GSE91060) (Jebessa et al., 2018). However, the PRRX1 gene is rarely studied in the myogenesis of chicken skeletal muscle, and whether its role in the growth and development of skeletal muscle is regulated by microRNAs, it is still unknown.
In this study, we analyzed the expression pattern of gga-mir-133a-3p in skeletal muscle differentiation of fetal stage chickens and characterized its regulation on the growth and development of chicken skeletal muscles. In order to investigate the regulatory mechanism of gga-mir-133a-3p on the proliferation and differentiation of myoblasts, we conducted a targeted study of the PRRX1 gene and demonstrated their direct target relationship. We also explored the effect of the PRRX1 gene in the proliferation and differentiation of myoblasts. Our result demonstrated that gga-mir-133a-3p inhibits myoblast proliferation and promotes myoblast differentiation by targeting PRRX1.
Materials and Methods
Ethics Statement
The animal experiments in this study were approved by the Animal Care Committee of South China Agricultural University (Approval number: SCAU#0014). The experiments were conducted according to the rules and policies formulated by the committee and in accordance with the Animal Protection Law of the People’s Republic of China.
Cell Culture
Chicken primary myoblasts (CPMs) were isolated from the leg muscles of 11- embryo-age chicken. The leg muscle was separated from the bone and crushed into pieces smaller than 1 mm3, and shaken in a shaker with 0.25% trypsin (Invitrogen, United States) at 37°C for 20 min. An equal amount of the RPMI 1640 Medium (Gibco, United States) with 20% fetal bovine serum (Gibco, United States) and 0.2% penicillin/streptomycin (Invitrogen, United States) was used to stop digestion. After centrifugation at 2,000 × g at room temperature, the supernatant was discarded and the cells were resuspended in the RPMI 1640 Medium with 20% fetal bovine serum and 0.2% penicillin/streptomycin and inoculated into a cell culture flask. Incubate in the incubator for 40 min and transfer the supernatant to a new flask. This step is repeated once. The culture of CPMs was performed using the RPMI 1640 Medium with 20% fetal bovine serum and 0.2% penicillin/streptomycin in a 37°C, 5% of CO2 incubator. Different media were used to induce differentiation of myoblasts, consisting of 2% horse serum (Hyclone, United States) and 0.2% penicillin/streptomycin (Invitrogen, United States) in 1640 medium. Quail muscle clone 7 (QM-7) cells were cultured in Medium 199 basic (Gibco, United States) with 10% fetal bovine serum (Gibco, United States), 10% tryptose phosphate broth solution (Sigma, United States) and 0.2% penicillin/streptomycin (Invitrogen) in a 37°C, 5% CO2 incubator. Dermal fibroblast cells (DF-1) were cultured with DMEM (Gibco, United States) with 10% fetal bovine serum (Gibco, United States) and 0.2% penicillin/streptomycin (Invitrogen, United States).
Reverse Transcription and RT-PCR Analysis
Total RNA from tissues or cells was extracted following to the standard protocol of chloroform method. The tissue was ground and 1 mL of TRizol cell lysate (Invitrogen, Carlsbad, CA, United States) was added and incubated for 5 min at room temperature. Then add 0.2 mL of chloroform (Damao, Tianjin, China), shake for 15 s, incubate for 5 min at room temperature, centrifuge at 12,000 × g for 15 min at 4°C. After centrifugation, the supernatant was transferred to a new tube, and 0.5 mL of isopropanol (Damao, Tianjin, China) was added and shaken for 15 s. After incubation for 30 min at -80°C, centrifugation was performed at 4°C for 12,000 × g for 10 min. The supernatant was discarded and washed with 75% anhydrous ethanol (Damao, Tianjin, China). After centrifugation at 7,500 × g at 4°C for 5 min, the supernatant was discarded and dried for 4 min and diluted with 25 μL of RNAfree water (SangonBio, Shanghai, China). The reverse transcription kit (Toyobo, Japan) was used to perform RNA reverse transcription and synthesize double-stranded cDNA. The expression of mRNA and miRNA were detected by RT-PCR with Bio-rad CFX96 instrument (Bio-Rad, United States) using iTAPTM universal SYBR GREEN superMIX (Bio-Rad, United States). The internal controls used in the experiments for quantifying microRNA and mRNA were U6 and GAPDH, respectively. The primers used during the study are listed in Table 1. Relative expression of miRNA and mRNAs were calculated using the 2-ΔΔCT method (ΔCT = CTtarget gene-CTreference gene, ΔΔCT = ΔCTtarget gene-ΔCTcontrol gene). Three replicates were performed for RT-PCR analysis.
RNA Oligonucleotides and Plasmids Construction
Gga-mir-133a-3p mimic, gga-mir-133a-3p inhibitor, mimic NC, inhibitor NC, small interfering RNA (siRNA) and siRNA negative control used in this study were designed and synthesized (RiboBio, Guangzhou, China). The information of oligonucleotides sequences in the study is listed in Table 2.
The complete CDS sequence of PRRX1 was amplificated by PCR and was cloned into expression vector, pcDNA3.1 (Promega, United States). The restriction sites we chose were HindIII and XhoI. The segment sequence of the PRRX1 3′UTR that contained the putative gga-mir-133a-3p binding sequence was amplified and was subcloned into HindIII and XhoI sites in the pmirGLO dual-luciferase reporter vector (Promega, United States). The PRRX1-3′UTR mutant plasmid was obtained by converting the binding site of gga-mir-133a-3p from GGGACCA to CCCTAAT. PCR amplification of the mutants and DPNI digestion removed the parental DNA. The primers used to construct the plasmids were listed in Table 3.
MiRNA Targets Prediction and RNAhybrid Detection
MiRDB was used to predicted target genes of gga-mir-133a-3p (miRDB1). RNAhybrid detection was used to calculate the combined minimum free energy (MFE) of gga-mir-133a-3p and the 3′UTR of PRRX1 to determine the binding stability of the duplex (RNAhybrid2).
Cell Transfection
When the cells grow to 70% confluence, Lipofectamine 3000 Reagent (Invitrogen, United States) was used to perform transient transfections following its protocol. The concentrations used for plasmid transfections were as follow: 2.5 μg/well for 6-well plate; 1 μg/well for 12-well plate; 0.5 μg/well for 24-well plate; 0.25 μg/well for 48-well plate; 0.1 μg/well for 96-well plate. For mimics, inhibitors and siRNA, a concentration of 20, 100, and 100 nM were used, respectively.
Dual-Luciferase Reporter Assay
After co-transfection of pmirGLO-PRRX1-3′UTR-WT or pmirGLO-PRRX1-3′UTR-MT with gga-mir-133a-3p mimic or mimic NC for 48 h, respectively, firefly and Renilla luciferase activities were detected using a Dual-GLO Luciferase Assay System Kit (Promega, United States) according its instruction. Multi-function microplate reader (Biotek, United States) was used to detect the firefly luciferase and Renilla luminescence activities.
CCK-8 (Cell Counting Kit 8) Assay
Cell Counting Kit 8 assay was used to detect cell proliferation. CPMs or QM-7 cells were seeded in a 96-well plate and cultured in mediums. After transfection, cell proliferation was monitored at 12, 24, 36, and 48 h using a TransDetect CCK Kit (TransGen Biotech, Beijing, China), following the manufacturer’s protocol. Add 10 uL CCK solutions to each well and incubate for 1 h at 37°C in a 5% CO2 cell incubator. Then absorbance was measured using a Model 680 Microplate Reader (Bio-Rad, United States) by optical density at a wavelength of 450 nm.
EdU (5-Ethynyl-2′-Deoxyuridine) Cell Proliferation Assay
For EdU cell proliferation assay, cells were seeded in 96-well plates. After transfection for 48 h, the cells were incubated at 37°C for 2 h in the presence of 50 μM EdU (RiboBio, Guangzhou, China). The cells were then fixed 4% paraformaldehyde for 30 min and neutralized using 2 mg/mL glycine solution for 5 min. Then the cells were permeabilized by 0.5% Triton X-100 for 10 min. The solution with EdU (RiboBio, Guangzhou, China) was added and the cells were incubated at room temperature for 30 min. Subsequently, the cells were permeabilized 3 times with 0.5% Triton X-100 for 10 min each time and then added with methanol for 5 min to reduce the dye background. The nuclear stain Hoechest 33342 was then added and incubation for another 30 min. Three randomly selected interfaces were captured using a fluorescence microscope (DMi8; Leica, Germany) to visualize the number of EdU-stained cells.
Flow Cytometric Analysis
CPMs and QM-7 cells were seeded in 12-well plates. Transfection was performed when the cell density reached 50%. After 48 h of culture, cells were digested by trypsin, and then ceased the digestion using DMEM with 10% FBS, transfer the lysate to 1.5 mL micro-centrifuge tubes and centrifuge at 2,000 × g for 5 min at 4°C. After the centrifugation, discarded the supernatant and then washed them with 1 mL PBS and centrifuged at 2,000 × g for 5 min at 4°C, the step was repeated twice, and then fixed the cells in 70% ethanol overnight at -20°C. The fixed cells were stained with propidium iodide (Beyotime, Shanghai, China). And then the cells were incubated at 37°C for 30 min in the dark. CytoFLEX FCM (Beckman, United States) was used to flow cytometric analysis and the data was analyzed using FlowJo7.6 software.
Western Blot
Ice-cold radio immunoprecipitation (RIPA) lysis buffer (Beyotime, Shanghai, China) with 1 mM phenylmethyl sulfonyl fluoride (Beyotime, Shanghai, China) was used in cell protein extraction. Proteins were separated by 10% SDS-PAGE, transferred to a nitrocellulose membrane (Maidstone, United Kingdom, Whatman), and then detected using antibodies according to standard procedures. The antibodies used for Western blots and their dilutions were as follows: Anti-PRRX1 antibody (Abcam, United Kingdom) 1: 1,000 and GAPDH (Boster, Wuhan, China) 1: 2,000. Finally, a secondary antibody (Boster, Wuhan, China) containing horseradish peroxidase chain reaction (HRP) anti-rabbit IgG antibody (Boster, Wuhan, China) was incubated in a 1: 5,000 dilution.
Immunofluorescence
For immunofluorescence, cells were seeded in 24-well plates. After transfection for 48 h, the cells were washed twice with PBS for 5 min and then fixed with 4% paraformaldehyde for 30 min. The cells were permeabilized by the addition of 0.1% TritonX-100 for 15 min and blocked with goat serum for 30 min. The cells were spiked with MYHC (B103; DHSB, United States; 0.5 μg/mL) and incubated overnight at 4°C. Subsequently, Goat Anti-Mouse IgG/FITC antibody (BS0296G; Bioss, China) was added and incubated for 1 h at room temperature. The DAPI was used for staining of the nucleus for 5 min. Fluorescence microscopy (DMi8; Leica, Germany) was used to capture images. The ImageJ software (National Institutes of Health) was used to measure the area of cells that were resistant to MYHC, and the calculated value is the percentage of the total area of the myotubes that occupy the image area.
Statistical Analysis
All data were mean ± standard deviation (S.E.M.), and there were at least three independent experiments for each treatment. The p-values were calculated using an unpaired two-sample t-tests (Student’s t-tests), with a significant difference (p < 0.05).
Results
gga-mir-133a-3p Inhibits Myoblast Proliferation
To verify the regulation of gga-mir-133a-3p in the proliferation and differentiation of chicken myoblasts, we synthesized gga-mir-133a-3p mimic and inhibitor for the over-expression and suppression of gga-mir-133a-3p at the cellular level. First, we transfected gga-mir-133a-3p mimic and inhibitor in QM-7 cells and CPMs to observe the over-expression effect of mimic and the inhibitory effect of inhibitor for gga-mir-133a-3p. The results showed that the mimic could significantly overexpress gga-mir-133a-3p, and the inhibitor also has significant inhibitory effect for gga-mir-133a-3p (Figures 1A,B), proved that they can be used for the next verification experiments.
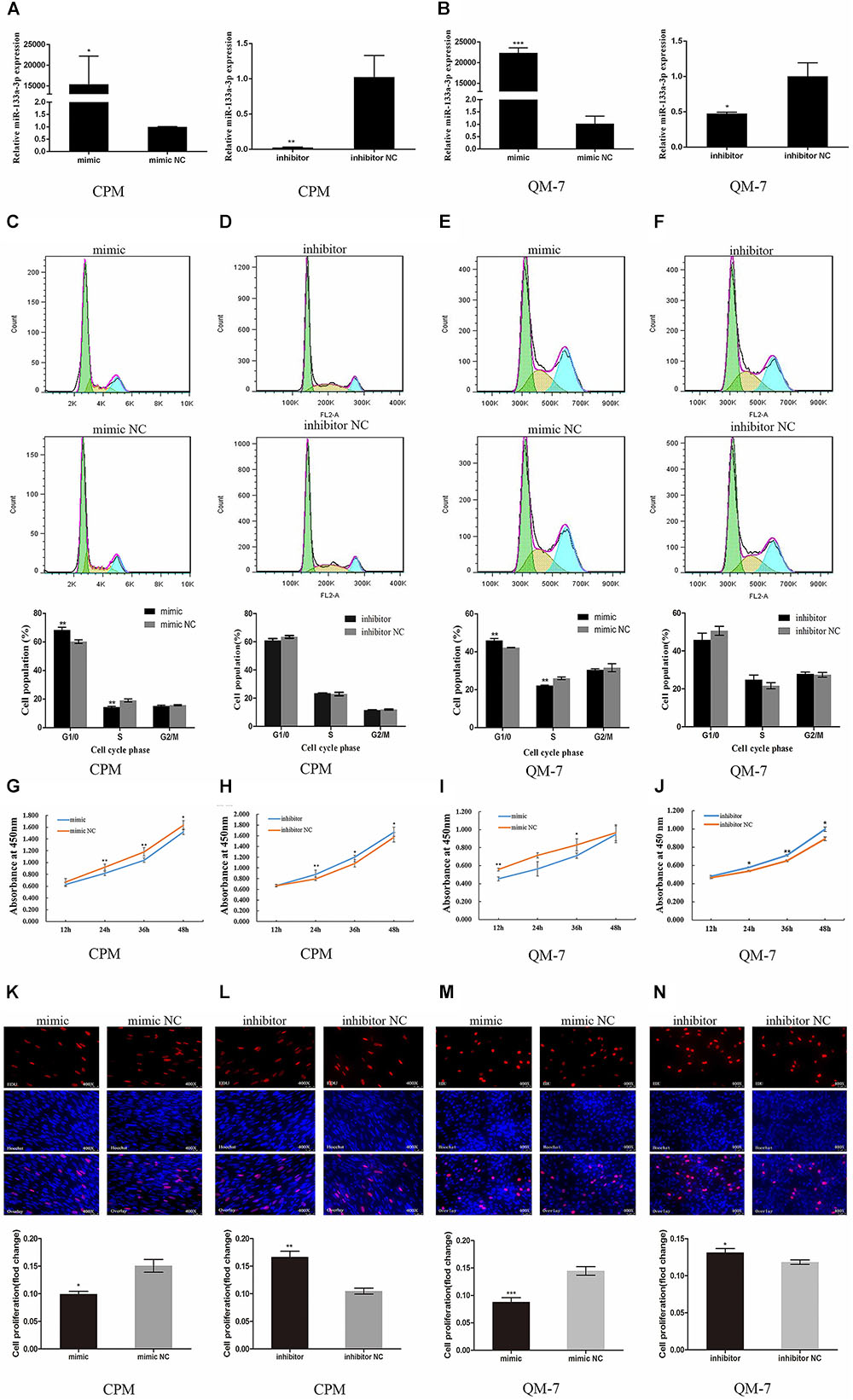
FIGURE 1. gga-mir-133a-3p inhibits myoblast proliferation. (A,B) Overexpression and inhibitory effects of gga-mir-133a-3p mimic and inhibitor in CPMs and QM-7 cells. (C,E) Cell cycle after overexpression of gga-mir-133a-3p in CPMs and QM-7 cells. (D,F) Cell cycle after inhibition of gga-mir-133a-3p in CPMs and QM-7 cells. (G–J) Cell growth curves were measured following the transfection of gga-mir-133a-3p mimic and inhibitor in CPMs and QM-7 cells. (K,M) Evaluation of the proliferation of gga-mir-133a-3p mimic-transfected CPMs and QM-7 cells by EdU incorporation, EdU-stained cell proportions were counted. (L,N) Evaluation of the proliferation of gga-mir-133a-3p inhibitor-transfected CPMs and QM-7 cells by EdU incorporation, EdU-stained cell proportions were counted. The results of all groups are shown as mean ± S.E.M., and the data represent three independent assessment methods. Statistical significance of the mean difference was assessed using an unpaired two-sample t-tests (∗p < 0.05; ∗∗p < 0.01; ∗∗∗p < 0.001) vs. NC (negative control).
We performed flow cytometric experiment to examine the effect of gga-mir-133a-3p in the cell cycle of QM-7 cells and CPMs. We found that the proportion of G1 phase cells in CPMs was significantly higher than the control group (p < 0.01), while the proportion of cells in S phase was significantly lower than that of the control group (p < 0.01) after the overexpression of gga-mir-133a-3p (Figure 1C). The number of cells in the G1 phase was significantly increased (p < 0.01), when the overexpressed of gga-mir-133a-3p in QM-7 cells, and the number of cells in the S phase was significantly decreased (p < 0.01) (Figure 1E). In contrast, after the transfection of gga-mir-133a-3p inhibitor, the results showed the opposite trend effect (Figures 1D,F). The results of cell cycle assay showed that gga-mir-133a-3p plays an inhibitory effect on the cell cycle progression of CPMs and QM-7 cells.
CCK-8 assay was used for the detection of proliferation vitality in CPMs and QM-7 cells, and the results showed that the proliferation vitality of the cells which had been transfected with gga-mir-133a-3p mimic were significantly lower than that of the control (Figures 1G,I). After the inhibition of gga-mir-133a-3p, the proliferation vitality of cells was significantly increased (Figures 1H,J). It indicates that gga-mir-133a-3p can effectively inhibit the proliferation vitality of myoblasts.
We also conducted EdU experiment to determine the proportion of proliferating cells of myoblasts. The EdU experiment showed that the overexpression of gga-mir-133a-3p in CPMs can significantly decrease the number of the cells in proliferation period (p < 0.05), in other hand, the number of cells in proliferation phase was extremely increased with the treatment of gga-mir-133a-3p inhibitor (p < 0.01) (Figures 1K,L). There is also a similar effect on the QM-7 cells (Figures 1M,N). These results fully demonstrate that gga-mir-133a-3p can inhibit the proliferating of myoblasts.
gga-mir-133a-3p Promotes Myoblast Differentiation
To investigate the potential mechanism of gga-mir-133a-3p in myogenesis of chicken skeletal muscle during the embryonic period, hence we isolated leg muscles of XingHua chicken embryo. During the period of skeletal muscle development in embryo of XingHua chicken, the expression of gga-mir-133a-3p in E16 and E18 were significantly up-regulated relative to E10 and E12 (Figure 2A), which suggests that gga-mir-133a-3p may be related to the differentiation of skeletal myoblasts. To further verify the potential role of gga-mir-133a-3p, CPMs were induced to differentiate in vitro (Figure 2B). With the process of differentiation in CPMs, the expression of gga-mir-133a-3p was significantly up-regulated, which suggest that gga-mir-133a-3p may be involved in the regulation of differentiation of myoblasts (Figure 2C). We also performed the same experiment in QM-7 cells, and similar results have been confirmed (Figures 2D,E).
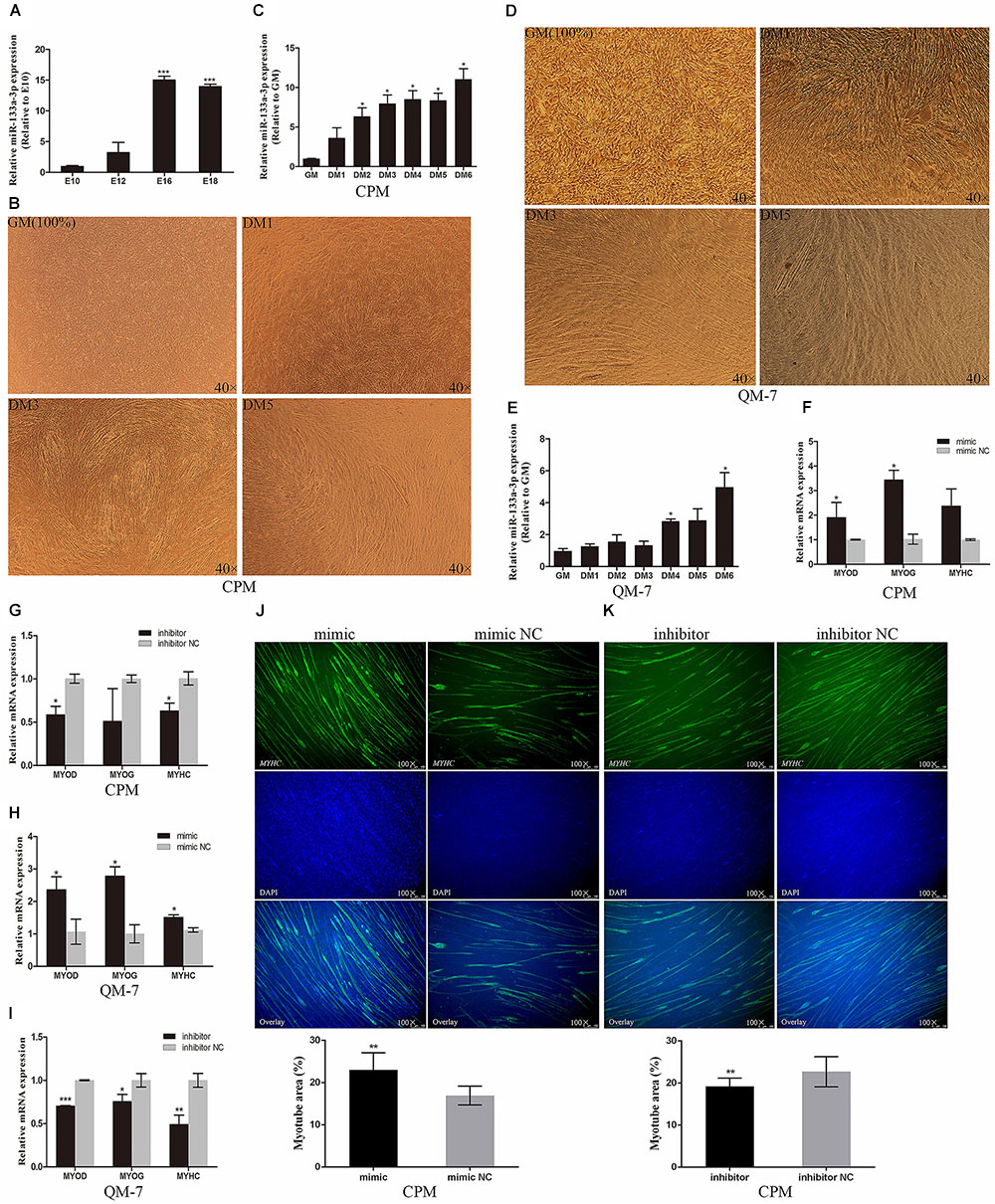
FIGURE 2. gga-mir-133a-3p promotes myoblast differentiation. (A) The relative expression of gga-mir-133a-3p in chicken embryonic leg muscle. (B) Morphology of CPMs induced differentiation. (C) The expression of gga-mir-133a-3p in the process of CPMs induced differentiation. (D) Morphology of QM-7 cells induced differentiation. (E) The expression of gga-mir-133a-3p in the process of QM-7 cells induced differentiation. (F,H) The expression of MYOD, MYOG and MYHC in CPMs and QM-7 cells after overexpression of gga-mir-133a-3p. (G,I) The expression of MYOD, MYOG and MYHC in CPMs and QM-7 cells after inhibition of gga-mir-133a-3p. (J,K) Immunofluorescence of MyHC and comparison of the area of myotubes. The results of all groups are shown as mean ± S.E.M., and the data represent three independent assessment methods. Statistical significance of the mean difference was assessed using an unpaired two-sample t-tests (∗p < 0.05; ∗∗p < 0.01; ∗∗∗p < 0.001) vs. NC (negative control).
Differentiation of myoblasts is accompanied by an increase in the expression of myogenic differentiation marker genes, including MYOD, MYOG, and MYHC. Here further determine of the relationship between gga-mir-133a-3p and differentiation of myoblasts, we performed the overexpression and the inhibition of gga-mir-133a-3p in CPMs and QM-7 cells, and then, induced cells differentiation. The results showed that gga-mir-133a-3p can significantly increase the expression of myogenic differentiation marker genes in CPMs (Figures 2F,G). And the same effect has shown in QM-7 cells (Figures 2H,I).
Using immunofluorescence, we traced the effects of gga-mir-133a-3p on myoblast differentiation. After transfecting gga-mir-133a-3p mimics, the area of myotubes formed by cell fusion was larger relative to the control group (Figure 2J), while the area of the myotube formed by cell fusion was smaller than that of the control group after the transfection of gga-mir-133a-3p inhibitor (Figure 2K). The results show that gga-mir-133a-3p play a promotional effect on myoblast differentiation.
gga-mir-133a-3p Targets Directly PRRX1 Gene
In order to explore the regulatory mechanism of gga-mir-133a-3p in growth and development of chicken embryo skeletal muscle and how gga-mir-133a-3p regulates the proliferation and differentiation of skeletal muscle myoblasts, we tried to determine the target genes of gga-mir-133a-3p related to the proliferation and differentiation of skeletal muscle myoblasts. The literature has confirmed that the functions of miRNAs are regulating the expression of its target genes.
The miRDB was used to predict and search the possible target genes of gga-mir-133a-3p. Data collection and analysis showed that PRRX1 gene was a potential target gene for gga-mir-133a-3p (Target Score: 99). In our previous study, the expression level of PRRX1 was down-regulated in the leg muscles between the E11 and E16 XingHua chickens, which were opposite to the expression level of gga-mir-133a-3p. The predicted binding site is at position 149–170 of the 3′UTR and the seed binding sequence is located at position 163–169 of the 3′UTR (Figure 3A). Besides, the RNAhybrid was used to analyze the duplex and the MFE between gga-mir-133a-3p and PRRX1 3′UTR. The RNA duplex has MFE approximately -22.9 kcal/mol, which indicating that more stable state (Figure 3B). The predicted target binding site at the 3′UTR of PRRX1 is conserved among species, including human, chimp, mouse, rat, rabbit and pig (Figure 3C).
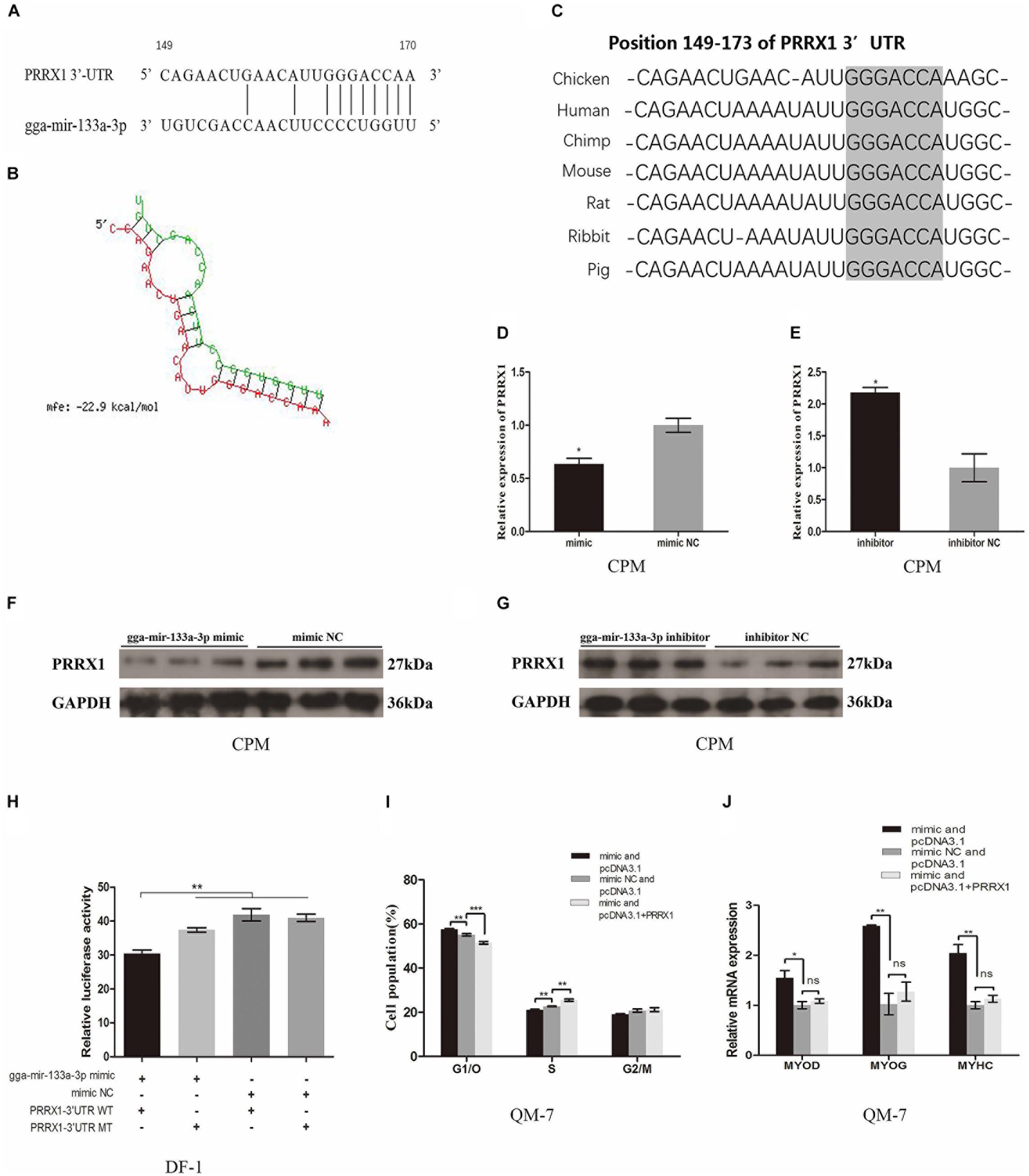
FIGURE 3. gga-mir-133a-3p targets directly PRRX1 gene. (A) The potential binding sites of gga-mir-133a-3p in PRRX1 3′UTR. (B) Duplex and MFE between gga-mir-133a-3p and PRRX1 3′UTR were analyzed by RNAhybrid. (C) Interspecies conservation in seed binding regions. (D,E) The expression of PRRX1 in CPMs after overexpression and inhibition of gga-mir-133a-3p. (F,G) The protein expression of PRRX1 in CPMs after overexpression and inhibition of gga-mir-133a-3p. (H) Luciferase assay was conducted by co-transfecting wild type or mutant PRRX1 3′UTR with gga-mir-133a-3p mimic or mimic NC in DF-1 cells. (I) Cell cycle analysis of QM-7 cells after co-transfection with the listed nucleic acids. (J) The mRNA expression levels of myoblast differentiation marker genes from QM-7 cells after co-transfection. The results of all groups are shown as mean ± S.E.M., and the data represent three independent assessment methods. Statistical significance of the mean difference was assessed using an unpaired two-sample t-tests (∗p < 0.05; ∗∗p < 0.01; ∗∗∗p < 0.001) vs. NC (negative control).
To verify the possible target relationship between gga-mir-133a-3p and PRRX1 in myoblasts, we overexpressed and inhibited gga-mir-133a-3p in CPMs, and then, quantified the expression of PRRX1 by RT-PCR and western blot. The results showed that gga-mir-133a-3p can curb the expression of PRRX1 both in the level of mRNA and protein (Figures 3D–G).
To further verify the target relationship between gga-mir-133a-3p and PRRX1, we performed a dual-luciferase reporter assay. The 3′UTR target sequence was cloned into the luciferase reporter vector (pmirGLO-PRRX1-3′UTR WT) and a luciferase reporter vector was constructed that mutated the target sequence binding site (pmirGLO-PRRX1-3′UTR MT). We transfected pmirGLO-PRRX1-3′UTR WT or pmirGLO-PRRX1-3′UTR MT with the gga-mir-133a-3p mimic or mimic NC into the DF-1 cells, respectively, and detected the luciferase activity after 48 h. Expression of luciferase can be inhibited by binding of gga-mir-133a-3p to 3′UTR of PRRX1. The luciferase activity was significantly decreased after co-transfection of gga-mir-133a-3p and PRRX1-3′UTR WT (Figure 3H).
To evaluate the roles of PRRX1 in the biological action of gga-mir-133a-3p, we conducted a recovery validation experiment of gga-mir-133a-3p function by co-transfecting with: gga-mir-133a-3p mimic and pcDNA3.1, mimic NC and pcDNA3.1, gga-mir-133a-3p mimic and pcDNA3.1+PRRX1 to test their effects on myoblast proliferation and differentiation. The results have shown that gga-mir-133a-3p can inhibit the proliferation of myoblasts, upregulate the expression of differentiation marker genes. However, these effects on myoblast proliferation and differentiation were restored or even reversed by co-expression of gga-mir-133a-3p and PRRX1 (Figures 3I,J). These results sufficiently indicated a direct target relationship between gga-mir-133a-3p and PRRX1.
PRRX1 Promotes Myoblast Proliferation
We constructed the overexpression vector of PRRX1 gene and synthesized its siRNA, to determine whether PRRX1 regulates the proliferation and differentiation of myoblasts. And their over-expression efficiency and interference efficiency were determined in CPMs and QM-7 cells, respectively (Figures 4A–D).
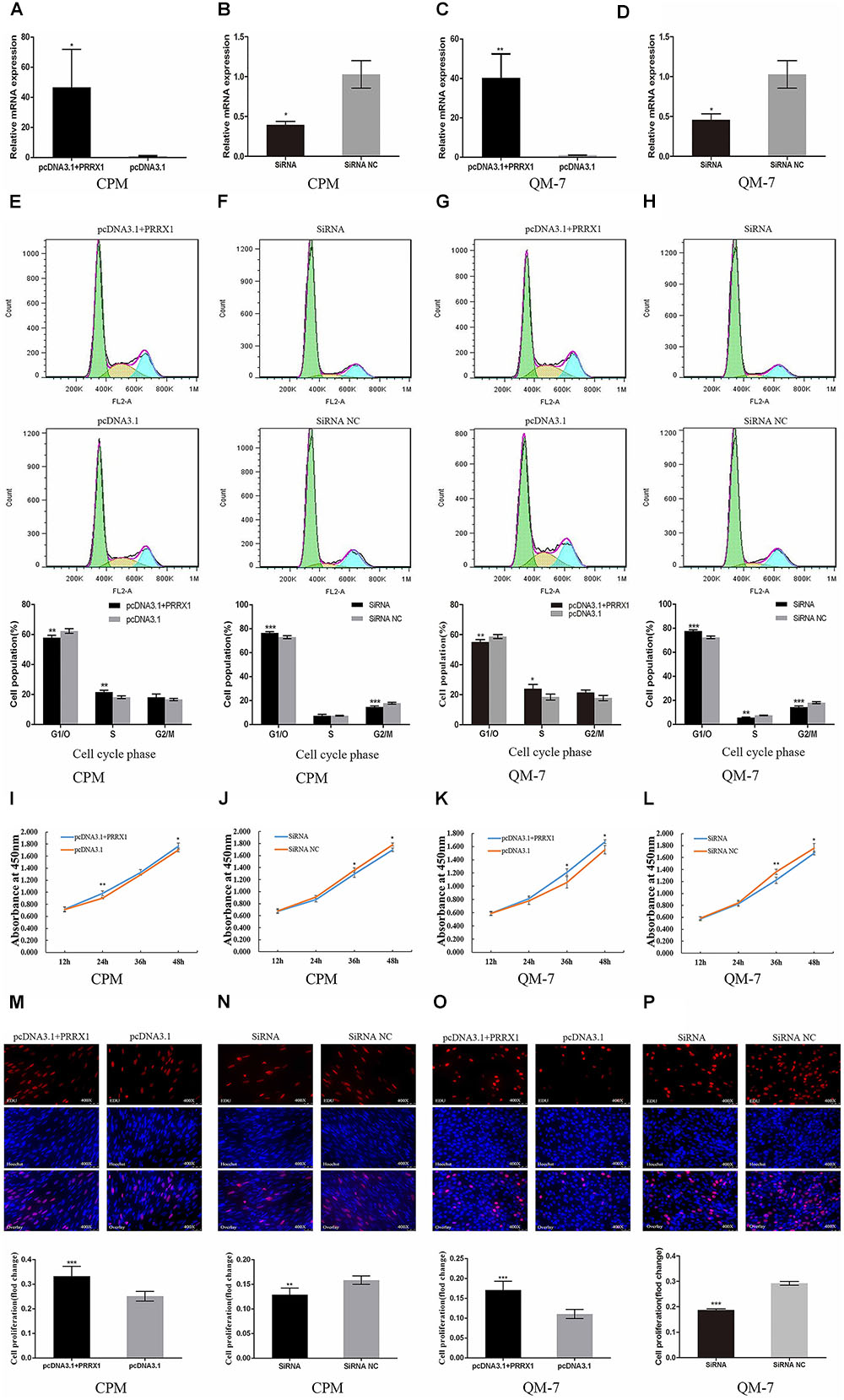
FIGURE 4. PRRX1 promotes myoblast proliferation. (A,C) The overexpression efficiency of the pcDNA3.1 with PRRX1 in CPMs and QM-7 cells. (B,D) The interference efficiency of PRRX1 SiRNA in CPMs and QM-7 cells. (E,G) Cell cycle after overexpression of PRRX1 in CPMs and QM-7 cells. (F,H) Cell cycle after interference of PRRX1 in CPMs and QM-7 cells. (I–L) Cell growth curves were measured following the transfection of PRRX1 overexpression vector and SiRNA in CPMs and QM-7 cells. (M,O) Evaluation of the proliferation of PRRX1-transfected CPMs and QM-7 cells by EdU incorporation, EdU-stained cell proportions were counted. (N,P) Evaluation of the proliferation of PRRX1 SiRNA-transfected CPMs and QM-7 cells by EdU incorporation, EdU-stained cell proportions were counted. The results of all groups are shown as mean ± S.E.M., and the data represent three independent assessment methods. Statistical significance of the mean difference was assessed using an unpaired two-sample t-tests (∗p < 0.05; ∗∗p < 0.01; ∗∗∗p < 0.001) vs. NC (negative control).
Flow cytometry results showed that overexpression of PRRX1 in CPMs can significantly reduce the proportion of cells in G1/0 phase and increase the proportion of cells in S phase, contrary to the results after siRNA treatment (Figures 4E,F). We also got similar results after conducting the same test in QM-7 cells (Figures 4G,H). The results indicate that PRRX1 can effectively promote the cycle progression of myoblasts.
Similarly, we examined the proliferation viability of myoblasts through CCK-8. After the treatment of overexpression of PRRX1 in CPMs, the proliferation viability of cells has been significantly increased (Figure 4I). Interfering with PRRX1 has reduced the viability of cells (Figure 4J). The results were consistent with that of the experiments in QM-7 cells (Figures 4K,L). The experimental results demonstrate that PRRX1 can enhance the proliferation activity of myoblasts.
Higher and fewer proliferating cells were detected after overexpression and interference with PRRX1 in CPMs, respectively (Figures 4M–P), which indicating that PRRX1 can promote CPMs and QM-7 cells proliferation.
PRRX1 Suppresses Myoblast Differentiation
During the period of skeletal muscle development in embryo of XingHua chicken, the expression of PRRX1 in E16 and E18 were significantly down-regulated relative to E10 (Figure 5A), which shows that PRRX1 may be related to the differentiation of skeletal myoblasts.
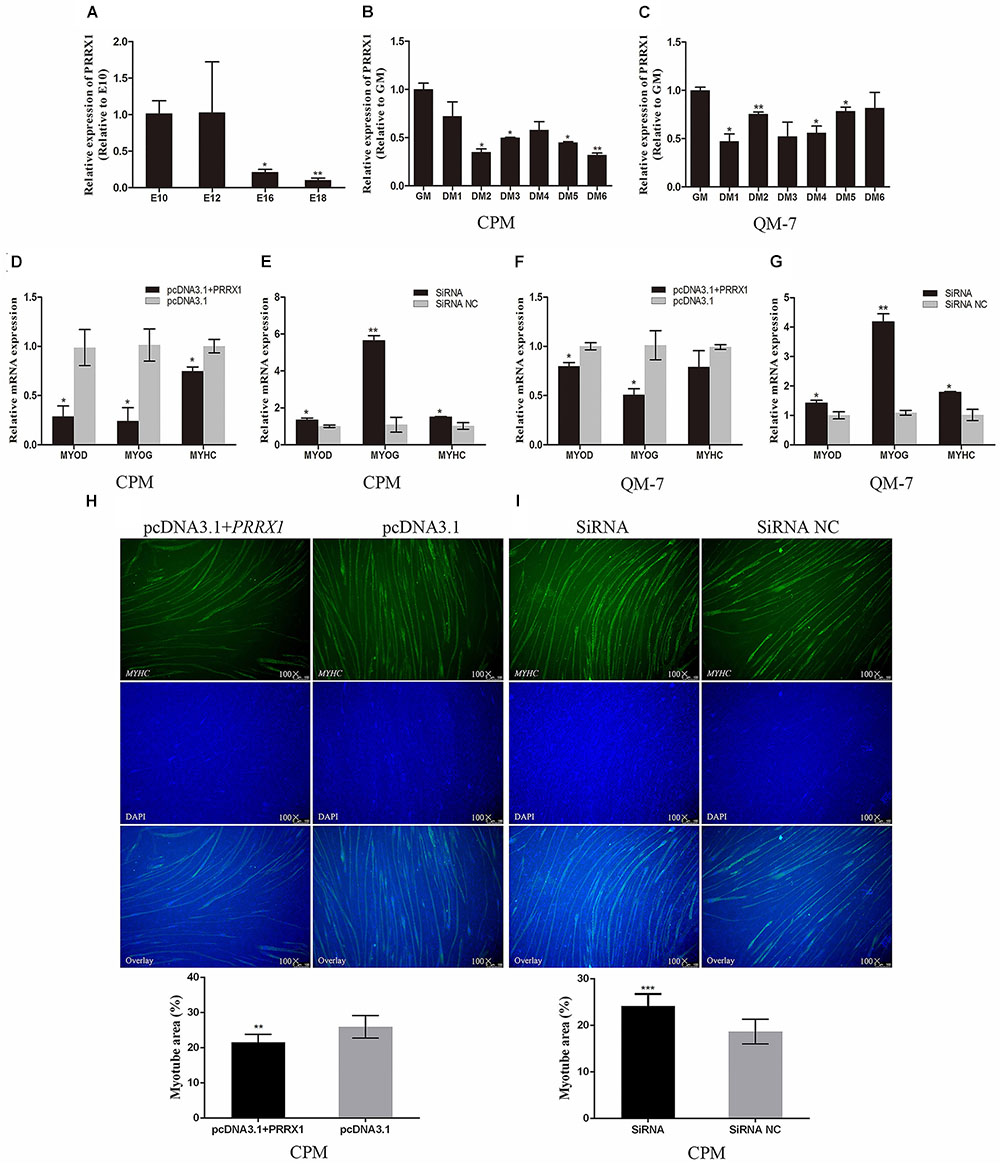
FIGURE 5. PRRX1 suppresses myoblast differentiation. (A) The relative expression of PRRX1 in chicken embryonic leg muscle. (B) The expression of PRRX1 in the process of CPMs induced differentiation. (C) The expression of PRRX1 in the process of QM-7 cells induced differentiation. (D,F) The expression of MYOD, MYOG, and MYHC in CPMs and QM-7 cells after overexpression of PRRX1. (E,G) The expression of MYOD, MYOG, and MYHC in CPMs and QM-7 cells after interference of PRRX1. (H,I) Immunofluorescence of MyHC and comparison of the area of myotubes. The results of all groups are shown as mean ± S.E.M., and the data represent three independent assessment methods. Statistical significance of the mean difference was assessed using an unpaired two-sample t-tests (∗p < 0.05; ∗∗p < 0.01; ∗∗∗p < 0.001) vs. NC (negative control).
After CPMs and QM-7 cells were induced to differentiate, we performed RT-PCR to quantify the expression level of PRRX1. The expression of PRRX1 in the differentiation phase is lower than in the proliferative phase in CPMs and QM-7 cells (Figures 5B,C). PRRX1 can significantly decrease the expression level of myogenic marker genes related to the differentiation of myoblasts, including MYOD, MYOG, MYHC (Figures 5D,F). After interference treatment to PRRX1, the expression level of the myogenic marker genes was significantly up-regulated (Figures 5E,G).
In immunofluorescence experiments, the area of cell-fused myotubes was smaller than that of the control group after over-expressing PRRX1, while the area of cell-fused myotubes was larger relative to the control group after silencing PRRX1 (Figures 5H,I). The results indicated that PRRX1 has an inhibitory effect on myoblast differentiation.
Discussion
In this study, we revealed a role of gga-mir-133a-3p in the proliferation and differentiation of chicken myoblasts, and we also found it is based on the function of regulating the proliferation and differentiation of myoblasts by inhibiting PRRX1 (Supplementary Figure S1). The proliferation and differentiation of skeletal muscle cells were relatively independent and antagonistic process, and miRNAs can regulate this process and control the proliferation and differentiation of myoblasts. Studies have also shown that mir-206 and mir-486 can accelerate muscle cell proliferation from the proliferative phase to the differentiation phase by inhibiting the expression of PAX7 (Dey et al., 2011). Mir-133a-3p is associated with proliferation and differentiation of embryonic muscle cells, as well as oral squamous cell carcinoma (Lewis et al., 2005). In our current study, gga-mir-133a-3p is differentially expressed between the leg muscles of XingHua chickens at embryonic E11 and E16. In our study, we found the transient expression pattern of gga-mir-133a-3p during skeletal muscle development and it was upregulated, which suggests that, a potential role in skeletal muscle myogenesis. To verify the potential role of gga-mir-133a-3p for skeletal muscle development, we performed experiments on the proliferation of CPMs and QM-7 cells. We confirmed that gga-mir-133a-3p can inhibit the proliferation of myoblasts. In other hand, we performed a series of experiments to confirm that gga-mir-133a-3p can promote myoblast differentiation. Mir-133 participated in myoblasts proliferation and differentiation by regulating the negatively modulates IGF-1R/PI3K/Akt signaling through repression of IGF-1R (Huang et al., 2011). The study completed by Chen et al. indicated that mir-133 can enhance myoblast proliferation (Chen et al., 2006), which is inconsistent with our results. However, they did research in mouse myoblasts and we performed that the effect of gga-mir-133a-3p on the growth and development of chicken skeletal muscle. In addition, in vitro study of Chen et al. overexpressed mir-133 using an overexpression vector inserted with a precursor sequence of mir-133 to verify pho-H3 expression in C2C12 cells, which they concluded that mir-133 enhances myoblast proliferation without further validation experiments. Our study indicated that the chicken mir-133 inhibits myoblast proliferation with more evidence supported by flow cytometric analysis, CCK-8 assay as well as EdU assay. These may be the reason why our results are contrasting with Chen et al. (2006) studied. It has been demonstrated that mir-133 inhibits cyclin D1 expression and induces myoblast G1 arrest, thereby inhibiting myoblast proliferation (Zhang et al., 2012). Another study has also demonstrated that mir-133 inhibits myoblast proliferation and promotes myoblast differentiation by repressing the activity of the ERK1/2 pathway through targeting FGFR1 and PP2AC (Feng et al., 2013).
MiRNAs mainly exert their biological effects by regulating the expression of target genes by binding to the 3′UTR of their target genes (Lewis et al., 2005). We performed target gene prediction analysis on the miRDB website. 131 genes were predicted and the top 10 scored genes were listed (Supplementary Table S1). These genes are mainly involved a variety of biological functions, including post-transcriptional splicing, DNA repair, mRNA maturation and so on, which also play a role in multiple biological processes, such as limb growth, muscle development, cellular immunity. We found that the mature sequence of the gga-mir-133a-3p can match the 3′UTR of PRRX1, which was downregulated between the leg muscles of E11 and E16 in XingHua chickens. Through data analysis, we found that the 3′UTR of PRRX1 and mir-133a-3p can be stably combined, and its seed binding area is conserved between multiple species. Comparing the conservation of the seed sequence of mir-133a-3p among these species, we found that the seed sequence of pig and chicken is exactly the same, and the other several species have only 85.71% homology with chicken (Supplementary Figure S2). It was shown that gga-mir-133a-3p and ssc-miR-133a-3p are specific for the targeted binding of PRRX1 relative to the other species. The result of dual luciferase assay demonstrated the direct targeting relationship between gga-mir-133a-3p and PRRX1. After overexpression and inhibition of gga-mir-133a-3p, the expression of PRRX1 was also decreased and increased in the levels of transcription and translation, respectively.
PRRX1 is known as a transcription factor, and its temporal and spatial expression are involved in the differentiation of cells and the development of tissues and organs (Higuchi et al., 2013). Previous studies have reported that the PRRX1 gene is involved in cell proliferation and reorganization (White et al., 2003). PRRX1 is localized to differentiate endothelial cells in the fetal lung mesenchyme and is critical for pulmonary angiogenesis (Ihida-Stansbury et al., 2004). At present, the research on PRRX1 mainly focused on embryonic limb development, vascular smooth muscle differentiation and osteogenic differentiation (Martin et al., 1995; Ten et al., 1998; Cretekos et al., 2008; Shang et al., 2008). In addition, PRRX1, was shown decreasing expression during chicken skeletal muscle development (Huang et al., 2011; Higuchi et al., 2013; He et al., 2018), which indicated that potential role in skeletal muscle growth and development. CCK-8 assay, EdU assay and flow cytometry were used to detect the effect of PRRX1 in myoblast proliferation. A series of in vitro experiments have demonstrated that PRRX1 can significantly promote the proliferation of myoblasts. At the same time, PRRX1 is also down-expressed during myoblast differentiation, which revealed that PRRX1 may be related to differentiation of myoblasts. The overexpression of PRRX1 can significantly reduce the expression of myogenic factors which were related to myoblast differentiation, including MYOD, MYOG, and MYHC. And overexpression of PRRX1 can inhibit myotube formation. It indicated that PRRX1 can suppress myoblast differentiation.
In summary, our study revealed a novel mechanism of gga-mir-133a-3p in regulating skeletal muscle growth and development. Gga-mir-133a-3p can regulate myogenesis by inhibiting myoblast proliferation and promoting myoblast differentiation, and it exerts biological effects through targeted inhibition of PRRX1 expression.
Author Contributions
LG conducted most of experiments, data analysis, and drafted the manuscripts. WH and BC performed parts of verification experiments and manuscript writing. EJB was responsible for the English editing of the article and part of experiments. BC and XC provided experimental ideas. QN participated in the design of the whole study. All authors read and approved the final manuscript.
Funding
This work was supported by grants from Science and Technology Planning Project of Guangzhou City (201604020007; 201504010017), the grand from Guangdong Province (2014KZDXM016), the Graduate Student Overseas Study Program from South China Agricultural University (2017LHPY025), and the Special Project for Development and Construction of Modern Agricultural Industry in Guangdong Province.
Conflict of Interest Statement
The authors declare that the research was conducted in the absence of any commercial or financial relationships that could be construed as a potential conflict of interest.
Acknowledgments
QN expresses his sincere thanks to Zhenhui Li and Hongjia Ouyang for their help in analyzing the data.
Supplementary Material
The Supplementary Material for this article can be found online at: https://www.frontiersin.org/articles/10.3389/fgene.2018.00577/full#supplementary-material
FIGURE S1 | Model of gga-mir-133a-3p-mediated regulatory mechanism for myoblast proliferation and differentiation. In simple terms, gga-mir-133a-3p inhibits the expression level of both PRRX1 mRNA and protein by targeting the 3 ’UTR of PRRX1 gene, resulting in the inhibition of myoblasts proliferation but promoting myoblasts differentiation.
FIGURE S2 | Comparison of the seed sequence of mir-133a-3p among different species, including chicken, human, mouse, rat, rabbit and pig.
TABLE S1 | Top 10 target gene scores for gga-mir-133a-3p as predicted using the miRNA target prediction and functional annotations miRDB tool (URL: http://www.mirdb.org/).
Footnotes
References
Agarwal, V., Subtelny, A. O., Thiru, P., Ulitsky, I., and Bartel, D. P. (2018). Predicting microRNA targeting efficacy in Drosophila. Genome Biol. 19:152. doi: 10.1186/s13059-018-1504-3
Ballarino, M., Cazzella, V., D’Andrea, D., Grassi, L., Bisceglie, L., Cipriano, A., et al. (2015). Novel long noncoding RNAs (lncRNAs) in myogenesis: a miR-31 overlapping lncRNA transcript controls myoblast differentiation. Mol. Cell Biol. 35, 728–736. doi: 10.1128/MCB.01394-14
Bartel, D. P. (2004). MicroRNAs: genomics, biogenesis, mechanism, and function. Cell 116, 281–297. doi: 10.1016/S0092-8674(04)00045-5
Bassel-Duby, R., and Olson, E. N. (2006). Signaling pathways in skeletal muscle remodeling. Annu. Rev. Biochem. 75, 19–37. doi: 10.1146/annurev.biochem.75.103004.142622
Cai, B., Ma, M., Chen, B., Li, Z., Abdalla, B. A., Nie, Q., et al. (2018). MiR-16-5p targets SESN1 to regulate the p53 signaling pathway, affecting myoblast proliferation and apoptosis, and is involved in myoblast differentiation. Cell Death Dis. 9:367. doi: 10.1038/s41419-018-0403-6
Chen, J. F., Mandel, E. M., Thomson, J. M., Wu, Q., Callis, T. E., Hammond, S. M., et al. (2006). The role of microRNA-1 and microRNA-133 in skeletal muscle proliferation and differentiation. Nat. Genet. 38, 228–233. doi: 10.1038/ng1725
Chung, B. Y., Deery, M. J., Groen, A. J., Howard, J., and Baulcombe, D. C. (2017). Endogenous miRNA in the green alga Chlamydomonas regulates gene expression through CDS-targeting. Nat. Plants 3, 787–794. doi: 10.1038/s41477-017-0024-6
Cretekos, C. J., Wang, Y., Green, E. D., Martin, J. F., Rasweiler, J. J., and Behringer, R. R. (2008). Regulatory divergence modifies limb length between mammals. Genes Dev. 22, 141–151. doi: 10.1101/gad.1620408
Dey, B. K., Gagan, J., and Dutta, A. (2011). miR-206 and -486 induce myoblast differentiation by downregulating Pax7. Mol. Cell Biol. 31, 203–214. doi: 10.1128/MCB.01009-10
Feng, Y., Niu, L., Wei, W., Zhang, W., Li, X., Cao, J., et al. (2013). A feedback circuit between miR-133 and the ERK1/2 pathway involving an exquisite mechanism for regulating myoblast proliferation and differentiation. Cell Death Dis. 4:e934. doi: 10.1038/cddis.2013.462
Goljanek-Whysall, K., Mok, G. F., Fahad Alrefaei, A., Kennerley, N., Wheeler, G. N., and Munsterberg, A. (2014). myomiR-dependent switching of BAF60 variant incorporation into Brg1 chromatin remodeling complexes during embryo myogenesis. Development 141, 3378–3387. doi: 10.1242/dev.108787
Grey, F., Tirabassi, R., Meyers, H., Wu, G., McWeeney, S., Hook, L., et al. (2010). A viral microRNA down-regulates multiple cell cycle genes through mRNA 5’UTRs. PLoS Pathog. 6:e1000967. doi: 10.1371/journal.ppat.1000967
Hausser, J., Syed, A. P., Bilen, B., and Zavolan, M. (2013). Analysis of CDS-located miRNA target sites suggests that they can effectively inhibit translation. Genome Res. 23, 604–615. doi: 10.1101/gr.139758.112
He, B., Lin, X., Tian, F., Yu, W., and Qiao, B. (2018). MiR-133a-3p inhibits oral squamous cell carcinoma (OSCC) proliferation and invasion by suppressing COL1A1. J. Cell Biochem. 119, 338–346. doi: 10.1002/jcb.26182
Higuchi, M., Kato, T., Chen, M., Yako, H., Yoshida, S., Kanno, N., et al. (2013). Temporospatial gene expression of Prx1 and Prx2 is involved in morphogenesis of cranial placode-derived tissues through epithelio-mesenchymal interaction during rat embryogenesis. Cell Tissue Res. 353, 27–40. doi: 10.1007/s00441-013-1632-8
Higuchi, M., Yoshida, S., Ueharu, H., Chen, M., Kato, T., and Kato, Y. (2014). PRRX1 and PRRX2 distinctively participate in pituitary organogenesis and a cell-supply system. Cell Tissue Res. 357, 323–335. doi: 10.1007/s00441-014-1861-5
Huang, M. B., Xu, H., Xie, S. J., Zhou, H., and Qu, L. H. (2011). Insulin-like growth factor-1 receptor is regulated by microRNA-133 during skeletal myogenesis. PLoS One 6:e29173. doi: 10.1371/journal.pone.0029173
Ihida-Stansbury, K., McKean, D. M., Gebb, S. A., Martin, J. F., Stevens, T., Nemenoff, R., et al. (2004). Paired-related homeobox gene Prx1 is required for pulmonary vascular development. Circ. Res. 94, 1507–1514. doi: 10.1161/01.RES.0000130656.72424.20
Jebessa, E., Ouyang, H., Abdalla, B. A., Li, Z., Abdullahi, A. Y., Liu, Q., et al. (2018). Characterization of miRNA and their target gene during chicken embryo skeletal muscle development. Oncotarget 9, 17309–17324. doi: 10.18632/oncotarget.22457
Jia, X., Ouyang, H., Abdalla, B. A., Xu, H., Nie, Q., and Zhang, X. (2017). miR-16 controls myoblast proliferation and apoptosis through directly suppressing Bcl2 and FOXO1 activities. Biochim. Biophys. Acta 1860, 674–684. doi: 10.1016/j.bbagrm.2017.02.010
Jiang, K., Zhang, M., Li, F., Li, D., Sun, G., Liu, X., et al. (2017). Study on the role of gga-miRNA-200a in regulating cell differentiation and proliferation of chicken breast muscle by targeting Grb2. Anim. Cells Syst. 21, 365–373. doi: 10.1080/19768354.2017.1400465
Kallen, A. N., Zhou, X. B., Xu, J., Qiao, C., Ma, J., Yan, L., et al. (2013). The imprinted H19 lncRNA antagonizes let-7 microRNAs. Mol. Cell 52, 101–112. doi: 10.1016/j.molcel.2013.08.027
Lewis, B. P., Burge, C. B., and Bartel, D. P. (2005). Conserved seed pairing, often flanked by adenosines, indicates that thousands of human genes are microRNA targets. Cell 120, 15–20. doi: 10.1016/j.cell.2004.12.035
Li, M., Zhou, X., Chen, Y., Nie, Y., Huang, H., Chen, H., et al. (2015). Not all the number of skeletal muscle fibers is determined prenatally. BMC Dev. Biol. 15:42. doi: 10.1186/s12861-015-0091-8
Liang, T., Zhou, B., Shi, L., Wang, H., Chu, Q., Xu, F., et al. (2018). lncRNA AK017368 promotes proliferation and suppresses differentiation of myoblasts in skeletal muscle development by attenuating the function of miR-30c. FASEB J. 32, 377–389. doi: 10.1096/fj.201700560RR
Lin, C. H., Jackson, A. L., Guo, J., Linsley, P. S., and Eisenman, R. N. (2009). Myc-regulated microRNAs attenuate embryonic stem cell differentiation. EMBO J. 28, 3157–3170. doi: 10.1038/emboj.2009.254
Lin, S., Li, H., Mu, H., Luo, W., Li, Y., Jia, X., et al. (2012). Let-7b regulates the expression of the growth hormone receptor gene in deletion-type dwarf chickens. BMC Genomics 13:306. doi: 10.1186/1471-2164-13-306
Luo, W., Wu, H., Ye, Y., Li, Z., Hao, S., Kong, L., et al. (2014). The transient expression of miR-203 and its inhibiting effects on skeletal muscle cell proliferation and differentiation. Cell Death Dis. 5:e1347. doi: 10.1038/cddis.2014.289
Martin, J. F., Bradley, A., and Olson, E. N. (1995). The paired-like homeo box gene MHox is required for early events of skeletogenesis in multiple lineages. Genes Dev. 9, 1237–1249. doi: 10.1101/gad.9.10.1237
Ouyang, H., Chen, X., Wang, Z., Yu, J., Jia, X., Li, Z., et al. (2017). Circular RNAs are abundant and dynamically expressed during embryonic muscle development in chickens. DNA Res. doi: 10.1093/dnares/dsx039 [Epub ahead of print].
Ouyang, H., He, X., Li, G., Xu, H., Jia, X., Nie, Q., et al. (2015). Deep sequencing analysis of miRNA expression in breast muscle of fast-growing and slow-growing broilers. Int. J. Mol. Sci. 16, 16242–16262. doi: 10.3390/ijms160716242
Panda, A. C., De, S., Grammatikakis, I., Munk, R., Yang, X., Piao, Y., et al. (2017). High-purity circular RNA isolation method (RPAD) reveals vast collection of intronic circRNAs. Nucleic Acids Res. 45:e116. doi: 10.1093/nar/gkx297
Perry, R. L., and Rudnick, M. A. (2000). Molecular mechanisms regulating myogenic determination and differentiation. Front. Biosci. 5, D750–D767. doi: 10.2741/A548
Rao, P. K., Kumar, R. M., Farkhondeh, M., Baskerville, S., and Lodish, H. F. (2006). Myogenic factors that regulate expression of muscle-specific microRNAs. Proc. Natl. Acad. Sci. U.S.A. 103, 8721–8726. doi: 10.1073/pnas.0602831103
Rehfeldt, C., Fiedler, I., Dietl, G., and Ender, K. (2000). Myogenesis and postnatal skeletal muscle cell growth as influenced by selection. Livest. Prod. Sci. 66, 177–188. doi: 10.3382/ps.2010-00641
Sartorelli, V., and Fulco, M. (2004). Molecular and cellular determinants of skeletal muscle atrophy and hypertrophy. Sci. STKE 2004:re11.
Schiaffino, S., Sandri, M., and Murgia, M. (2007). Activity-dependent signaling pathways controlling muscle diversity and plasticity. Physiology 22, 269–278. doi: 10.1152/physiol.00009.2007
Shang, Y., Yoshida, T., Amendt, B. A., Martin, J. F., and Owens, G. K. (2008). Pitx2 is functionally important in the early stages of vascular smooth muscle cell differentiation. J. Cell Biol. 181, 461–473. doi: 10.1083/jcb.200711145
Shenoy, A., and Blelloch, R. H. (2014). Regulation of microRNA function in somatic stem cell proliferation and differentiation. Nat. Rev. Mol. Cell Biol. 15, 565–576. doi: 10.1038/nrm3854
Smith, J. H. (1963). Relation of body size to muscle cell size and number in the chicken. Poult. Sci. 42, 283–290. doi: 10.3382/ps.0420283
Ten, B. D., Brouwer, A., Korving, J., Martin, J. F., and Meijlink, F. (1998). Prx1 and Prx2 in skeletogenesis: roles in the craniofacial region, inner ear and limbs. Development 125, 3831–3842.
Wang, L., Zhao, Y., Bao, X., Zhu, X., Kwok, Y. K., Sun, K., et al. (2015). LncRNA Dum interacts with Dnmts to regulate Dppa2 expression during myogenic differentiation and muscle regeneration. Cell Res. 25, 335–350. doi: 10.1038/cr.2015.21
Wei, W., He, H. B., Zhang, W. Y., Zhang, H. X., Bai, J. B., Liu, H. Z., et al. (2013). miR-29 targets Akt3 to reduce proliferation and facilitate differentiation of myoblasts in skeletal muscle development. Cell Death Dis. 4:e668. doi: 10.1038/cddis.2013.184
Wei, X., Li, H., Yang, J., Hao, D., Dong, D., Huang, Y., et al. (2017). Circular RNA profiling reveals an abundant circLMO7 that regulates myoblasts differentiation and survival by sponging miR-378a-3p. Cell Death Dis. 8:e3153. doi: 10.1038/cddis.2017.541
White, P., Thomas, D. W., Fong, S., Stelnicki, E., Meijlink, F., Largman, C., et al. (2003). Deletion of the homeobox gene PRX-2 affects fetal but not adult fibroblast wound healing responses. J. Invest. Dermatol. 120, 135–144. doi: 10.1046/j.1523-1747.2003.12015.x
Yoshida, T., Hoofnagle, M. H., and Owens, G. K. (2004). Myocardin and Prx1 contribute to angiotensin II-induced expression of smooth muscle alpha-actin. Circ. Res. 94, 1075–1082. doi: 10.1161/01.RES.0000125622.46280.95
Zhang, D., Li, X., Chen, C., Li, Y., Zhao, L., Jing, Y., et al. (2012). Attenuation of p38-mediated miR-1/133 expression facilitates myoblast proliferation during the early stage of muscle regeneration. PLoS One 7:e41478. doi: 10.1371/journal.pone.0041478
Zhang, J., Zhou, W., Liu, Y., Liu, T., Li, C., and Wang, L. (2018). Oncogenic role of microRNA-532-5p in human colorectal cancer via targeting of the 5’UTR of RUNX3. Oncol. Lett. 15, 7215–7220. doi: 10.3892/ol.2018.8217
Keywords: gga-mir-133a-3p, PRRX1, myoblast, proliferation, differentiation
Citation: Guo L, Huang W, Chen B, Jebessa Bekele E, Chen X, Cai B and Nie Q (2018) gga-mir-133a-3p Regulates Myoblasts Proliferation and Differentiation by Targeting PRRX1. Front. Genet. 9:577. doi: 10.3389/fgene.2018.00577
Received: 05 September 2018; Accepted: 08 November 2018;
Published: 04 December 2018.
Edited by:
Enrique Medina-Acosta, Universidade Estadual do Norte Fluminense Darcy Ribeiro, BrazilReviewed by:
Giuseppe Intini, University of Pittsburgh, United StatesGuirong Sun, Henan Agricultural University, China
Cíntia Barros Santos-Rebouças, Rio de Janeiro State University, Brazil
Copyright © 2018 Guo, Huang, Chen, Jebessa Bekele, Chen, Cai and Nie. This is an open-access article distributed under the terms of the Creative Commons Attribution License (CC BY). The use, distribution or reproduction in other forums is permitted, provided the original author(s) and the copyright owner(s) are credited and that the original publication in this journal is cited, in accordance with accepted academic practice. No use, distribution or reproduction is permitted which does not comply with these terms.
*Correspondence: Qinghua Nie, bnFpbmdodWFAc2NhdS5lZHUuY24=