- 1College of Animal Science and Veterinary Medicine, Henan Agricultural University, Zhengzhou, China
- 2Henan Innovative Engineering Research Center of Poultry Germplasm Resource, Zhengzhou, China
- 3The First Bethune Hospital, Jilin University, Changchun, China
Poultry meat quality is affected by many factors, among which intramuscular fat (IMF) is predominant. IMF content affects the tenderness, juiciness, and flavor of chicken. An increasing number of studies are focusing on the functions of lncRNAs in adipocyte differentiation. However, little is known about lncRNAs associated with intramuscular adipocyte differentiation. In the present study, we focused on an up-regulated lncRNA during intramuscular adipogenetic differentiation, which we named intramuscular fat-associated long non-coding RNA (IMFNCR). IMFNCR promotes intramuscular adipocyte differentiation. In-depth analyses showed that IMFNCR acts as a molecular sponge for miR-128-3p and miR-27b-3p and that PPARG is a direct target of miR-128-3p and miR-27b-3p in chicken. High-fat and high-protein diet inhibited chicken IMFNCR level in vivo. Moreover, IMFNCR level was positively correlated with PPARG mRNA level in chicken breast muscle tissues, a vital corollary to ceRNA function. Altogether, our research showed that IMFNCR acts as a ceRNA to sequester miR-128-3p and miR-27b-3p, leading to heightened PPARG expression, and thus promotes intramuscular adipocyte differentiation. Taken together, our findings may contribute to a more thorough understanding of chicken IMF deposition and the improvement of poultry meat quality.
Introduction
Intramuscular fat (IMF) content is an important factor associated with meat quality, while abdominal fat is regarded as one of the main factors affecting poultry slaughter efficiency. It is generally believed that increasing IMF content is usually accompanied by an increase in abdominal fat content. Therefore, achieving carcass with high IMF content and minimal abdominal fat content, balancing IMF, and abdominal fat content has been a major aim of poultry breeding. Previous studies suggested that there exist different fat deposition rates in different parts of the body, and different fat tissues have distinct lipid accumulation and metabolism ability. However, the molecular mechanisms underlying depot-specific fat deposition remains largely unknown.
Peroxisome proliferator-activated receptor gamma (PPARG) is a critical transcriptional factor for regulating preadipocyte differentiation and lipid metabolism. PPARG is induced during the entire terminal differentiation process and is required for the activation of a number of adipogenic genes (Rosen et al., 2002). MicroRNAs are an important class of small non-coding RNAs that emerging as a post-transcriptional regulator involving different physiological activities such as growth, development and metabolism. Previous studies found that miR-27 increasing the beige/brown fat mass as a central upstream regulator of the transcriptional network involved in beige and brown adipogenesis after cold exposure (Karbiener et al., 2009; Lin et al., 2009; Lei and Mirko, 2014). Qiu et al. (2013) found that miR-128a inhibit the differentiation of pre-adipocyte in rat via regulating the expression of PPARG. Chen et al. (2018) demonstrated that miR-128-3p impeded preadipocyte differentiation and lipolysis by targeting PPARG and Sertad2 in 3T3-L1.
lncRNAs are a class of non-protein coding transcripts that are greater than 200 nt in length and found to be involved in various biological processes, such as cell cycle, cell differentiation, cell proliferation, and genomic imprinting (Koerner et al., 2009; Cesana et al., 2011; Batista and Chang, 2013; Tripathi et al., 2013). Recently, a growing number of reports have indicated that lncRNAs can communicate with and regulate protein-coding genes by direct competition for miRNA binding, thus acting as competing endogenous RNAs (ceRNAs) (Salmena et al., 2011; Legnini et al., 2014). Furthermore, it is now becoming evident that lncRNAs are emerging as critical regulators of skeletal muscle formation and fat deposition (Sun et al., 2013; Mueller et al., 2015). Linc-MD1, a muscle-specific long non-coding RNA was found to be regulating myogenesis by acting a sponge for miR-133 and miR-135 (Legnini et al., 2014). Sun et al. (2016) reported that a myoblast differentiation-associated long non-coding RNA, lncMD acts as a molecular sponge for miR-125b and that IGF2 is a direct target of miR-125b in cattle. Li et al. (2016) found adipocyte differentiation-associated long non-coding RNA (ADNCR) was downregulated during the differentiation of adipocyte and inhibited adipocyte differentiation by functioning as a competing endogenous RNA for miR-204. These individual studies demonstrate the growing importance of lncRNAs in myogenesis and adipogenesis. However, our knowledge of lncRNAs related to intramuscular adipocyte differentiation still remains limited.
Our previous transcriptome data of breast muscle at different physiological periods identified a lot of differentially expressed lncRNAs in breast muscle between juvenile and later laying-period hens (Zhang et al., 2017). A lncRNA, ALDB00000918, which we named IMF associated long non-coding RNA (IMFNCR), was found to be significantly upregulated in later laying-period hens. To investigate the potential function of lncRNAs associated with chicken IMF deposition, we further characterized the function and regulation mechanism of IMFNCR. Mechanistically, IMFNCR promotes intramuscular adipocyte differentiation by sponging miR-128-3p and miR-27b-3p, thereby augmenting the expression of the miR-128-3p and miR-27b-3p target gene, PPARG, which cooperates with C/EBPA and a number of adipogenic genes to execute the adipogenic differentiation program. Our findings provide new insights into understanding the mechanisms of IMF deposition and improvement of the meat quality of poultry.
Materials and Methods
Ethics Statement
All animal experiments were carried out in accordance with the Guidelines for Experimental Animals established by the Ministry of Agricultural of China (Beijing, China). This study was approved by the Animal Welfare Committee of College of Animal Science and Veterinary Medicine, Henan Agricultural University (Zhengzhou, Henan, China) (Permit Number:11-0085; Date: 06-2011).
Animals and Tissue Collection
Eighteen Gushi chickens were divided into three groups: Low-fat diet group (Linoleic acid, 5%), middle-fat diet group (Linoleic acid, 10%), and High-fat diet group (Linoleic acid, 20%); another eighteen Gushi chickens were divided into three groups: Low-protein diet group (Crude protein, 10%), Middle-protein diet group (Crude protein, 20%) and High-protein diet group (Crude protein, 30%). All birds were raised in the same environmental conditions with ad libitum water and food for 3 weeks. Chickens were weighed and then killed by stunning and exsanguination 12 h after feed was withheld. Tissues were immediately collected, snap-frozen in liquid nitrogen and stored at −80°C until RNA extraction.
Isolation, Culture of Primary Preadipocyte and Adipogenic Differentiation
preadipocyte were isolated from the breast muscle and abdominal adipose tissue of female chickens at 2-week-old following methods described previously described (Ramsay and Rosebrough, 2003; Zhang et al., 2018). Cells were maintained in DMEM/F12 (1:1) supplemented with 10% FBS (Gibco, Beijing, China) and 1% penicillin/streptomycin solution in a humidified atmosphere with 5% (v/v) CO2 at 37°C. After cells reached confluence, differentiation was induced with differentiation medium [0.5 mM 3-isobutyl-1-methylxanthine, 1 μM dexamethasone, 50 nM insulin and 300 μM oleate (dissolved in DMSO) (all from Sigma, Beijing, China)] for 48 h. Then, the differentiation medium was replaced with maintenance medium [50 nM insulin and 300 μM oleate (Sigma)] and incubated for 48 h. The detailed procedure for the induction of intramuscular preadipocyte is described in Figure 1. Cells were harvested at 0, 2, 4, 6, 8, and 10 days after induction. Each stage included three biological replicates (n = 3).
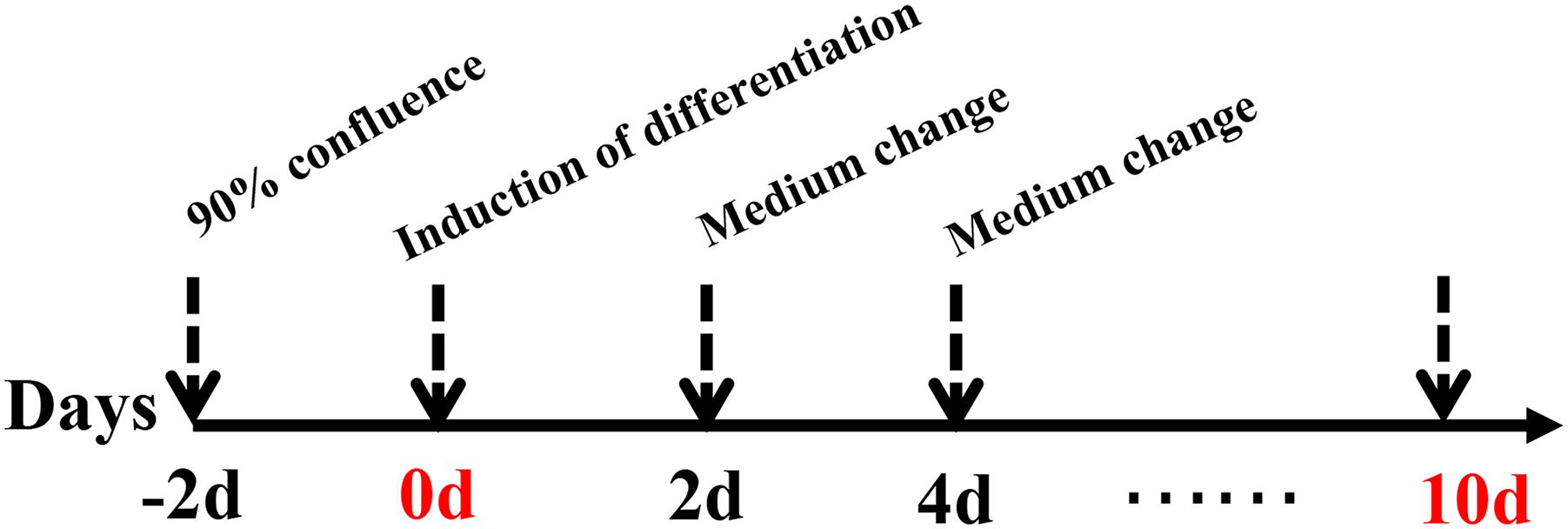
Figure 1. Induction of differentiation in intramuscular and abdominal preadipocyte. The basic medium consisted of DMEM/F-12 and 10% FBS. The induction differentiation medium consisted of basic medium, insulin, dexamethasone, 3-isobutyl-1-methylxanthine, and oleate. The maintenance medium consisted of basic medium, insulin, and oleate. The induction differentiation medium was replaced with the maintenance medium at 48 h, whereas the maintenance medium was replaced with basic medium at 96 h.
Plasmid Construction and Cell Transfection
The wild type and mutated sequences of IMFNCR and 3′UTR of PPARG (perfected the seed region of the miR-128-3p binding sites) were cloned into the XhoI–NotI site of the psiCHECK-2 (Promega, Maddison, WI, United States). The mutated sequences of IMFNCR and 3′UTR of PPARG were generated by mutating the seed region of the miR-128-3p binding sites by overlapping PCR. The siRNAs of IMFNCR were:IMFNCR-si1, 5′ GCUCUGGUCAAACACGCUUTT 3′, IMFNCR-si1, 5′ AAGCGUGUUUGACCAGAGCTT 3′; IMFNCR-si2, 5′ GCUAUAGAACGUCAGAAAUTT 3′ and IMFNCR-si2, 5′ AUUUCUGACGUUCUAUAGCTT 3′. miR-128-3p and miR-27b-3p mimics, inhibitor and negative control were purchase from GenePharma (Shanghai, China). Plasmid DNA was sequenced by Sangon Biotech (Shanghai, China) and extracted using an EndoFree Maxi Plasmid Kit (TIANGEN, Beijing, China). DF1 cells were cultured in DMEM with 10% fetal bovine serum (FBS) and 1% penicillin/streptomycin solution at 37°C with 5% CO2 in a humidified incubator.
Luciferase Assays
DF1 cells were seeded in 6-well plates at a density of 5 × 105 cells/well and cultured under routine conditions with 10% FBS. When the cells reached 70 or 80% confluence, the IMFNCR wild-type or mutant construct was cotransfected with 50 nM negative control or miR-128-3p mimic (GenePharma, Shanghai, China) using Kretschmer-Kazemi and Sczakiel (2003) (Invitrogen, Carlsbad, CA, United States) according to the manufacturer’s instructions, and the medium was replaced 6 h later. The relative luciferase activity was measured 48 h after transfection by the Dual-Luciferase Reporter Assay System (Promega) on a Fluoroskan Ascent FL instrument (Thermo Fisher Scientific, Shanghai, China). Renilla luciferase activity was normalized to firefly luciferase activity.
RNA Isolation and Real-Time Quantitative PCR (qPCR)
Total RNA from tissues and preadipocyte were isolated using extracted with Trizol reagent according to the manufacturer’s protocol (Takara, Dalian, China). RNA samples were stored at −80 C until used. cDNA synthesis and qPCR were carried out as described (Zhang et al., 2017, 2018). qPCR primers are reported in Supplementary Table S1. The expression of miRNA was detected by stem-loop real-time qPCR. The stem-loop primers used for the qPCR, miRNA mimics, miRNA inhibitor and negative control were purchased from GenePharma Co., Ltd. (Shanghai, China). We used the 2-ΔΔCt method to analyze relative expression levels of mRNA, lncRNA and miRNA.
Western Blot Analysis
Total protein was extracted from cells using a RIPA buffer (Solarbio) supplemented with PMSF (Servicebio) (100:1). Protein was separated on 10% SDS-PAGE gels. The proteins were transferred to PVDF membranesm, and then blocked with 5% non-fat milk for 2 h. The membranes were washed with PBST three times (5 min/time) and incubated with the primary antibodies (Abcam) at 4°C for overnight. Then the membranes were washed three times using PBST and incubated with secondary antibody conjugated with HRP (Abcam) for 1 h at room temperature. Signals were detected by ECL Plus (Solarbio). β-Actin was used as an internal control.
RNA Fluorescence in situ Hybridization (RNA FISH)
FITC-labeled IMFNCR probes were obtained from servicebio (Wuhan, Hubei, China). RNA FISH was performed using fluorescent in situ hybridization kit (RiboBio) following the manufacturer’s instructions. The cytopalsmic and nuclear RNA were isolated by PARISTM Kit (Life) according to the manufacturer’s protocol.
Oil Red O Staining
Cells were washed with PBS three times and fixed with 10% formaldehyde for 30 min. The fixed cells were washed with PBS three times and incubated with 1% filtered Oil Red O solution (0.3% Oil Red O, 60% isopropanol, and 40% PBS) for 30 min, and then phase-contrast microscope (Olympus, Japan) was used to check for positive cells appearing red. Subsequently, Oil Red O was eluted from the stained cells with 100% isopropanol (v/v) and quantified by microplate reader (Thermo Fisher Scientific) at 510 nm.
Statistical Analysis
Data were analyzed using Student’s tests in SPSS 20.0 software. Data are presented as the mean ± SEM (standard error of the mean) based on at least three replicates. Correlation analysis was conducted using Pearson of bivariate correlation. A p-value of <0.05 was considered significant.
Results
Identification of IMFNCR as a Candidate lncRNA
According to our previous mRNA and lncRNA sequencing data (NCBI Project No: PRJNA380024), a candidate intramuscular fat-associated long non-coding RNA (IMFNCR) was remarkably up-regulated in chicken breast muscle at later-laying period compared with that at pre-laying period. The genomic location and gene structure of IMFNCR are shown in Supplementary Figure S1. qRT-PCR results showed that IMFNCR was highly expressed in pancreas, abdominal fat and breast muscle tissues (Figures 2A,B) and the expression trend of IMFNCR was consisted with PPARG in breast muscle at different periods (Figure 2C). It is interesting to note that IMFNCR were highly expressed in intramuscular adipocyte compared with abdominal adipocyte (Figure 2D), implying their potential biological functions in chicken IMF deposition.
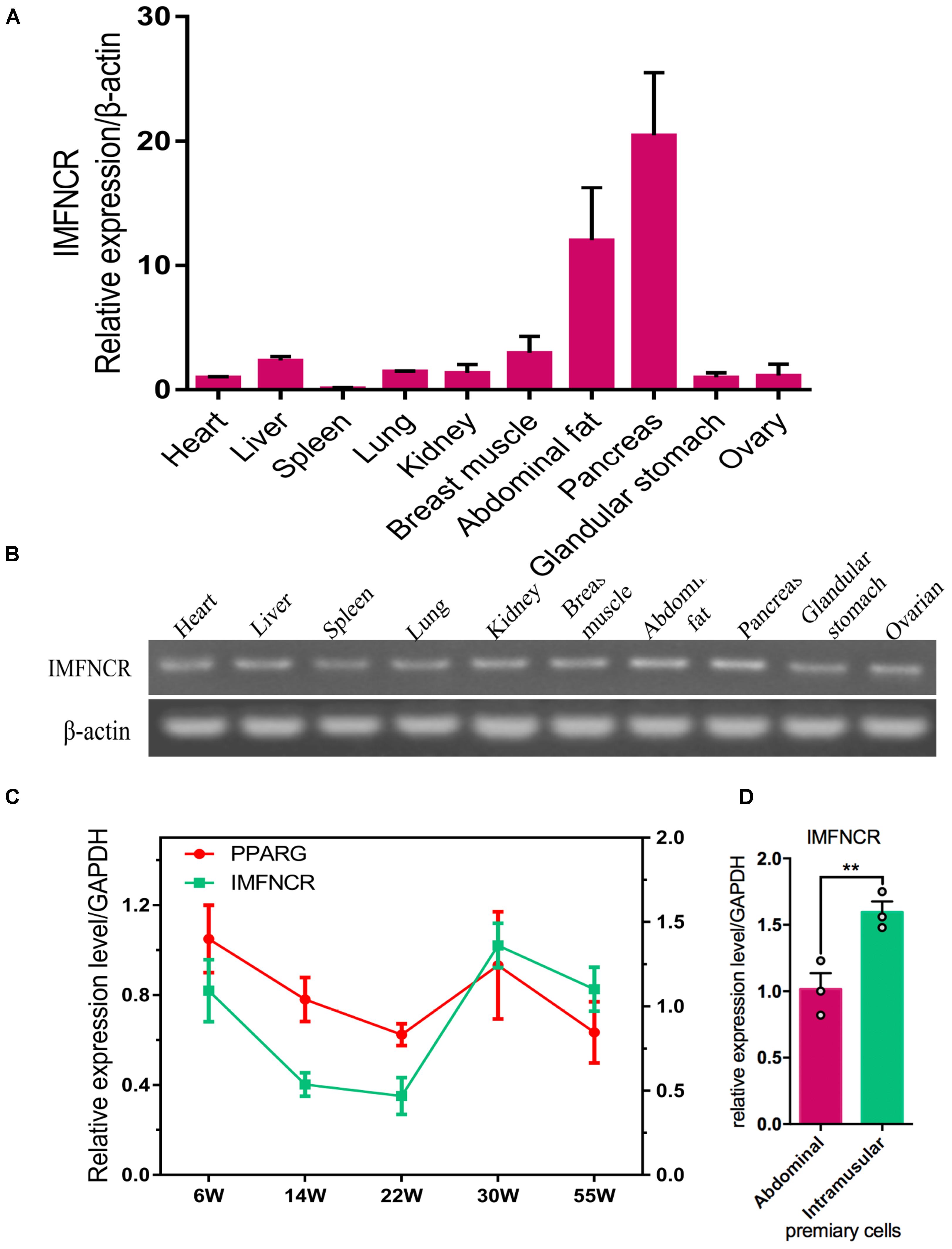
Figure 2. Characteristics of chicken IMFNCR in different tissues and cells. (A) Tissue expression profile of IMFNCR by qRT-PCR. (B) Tissue expression profile of IMFNCR by semi-quantitative PCR. (C) The expression dynamics of IMFNCR in breast muscle tissues at different physiological periods. (D) The expression level of IMFNCR in abdominal and intramuscular preadipocyte. Data represent means ± SEM (n = 3). ∗p ≤ 0.05; ∗∗p ≤ 0.01.
RNA FISH and semi-quantitative PCR of nuclear and cytoplasmic fractions analyses indicated that IMFNCR is predominantly localized in the cytoplasm of preadipocyte (Figures 3A,C). To assess whether IMFNCR has protein coding ability, online software was used in the present study. CPC (Coding Potential Calculator) software showed that IMFNCR has a very low coding potential, similar to GHR-AS, a well-known lncRNA (Zhang et al., 2016; Figure 3B).
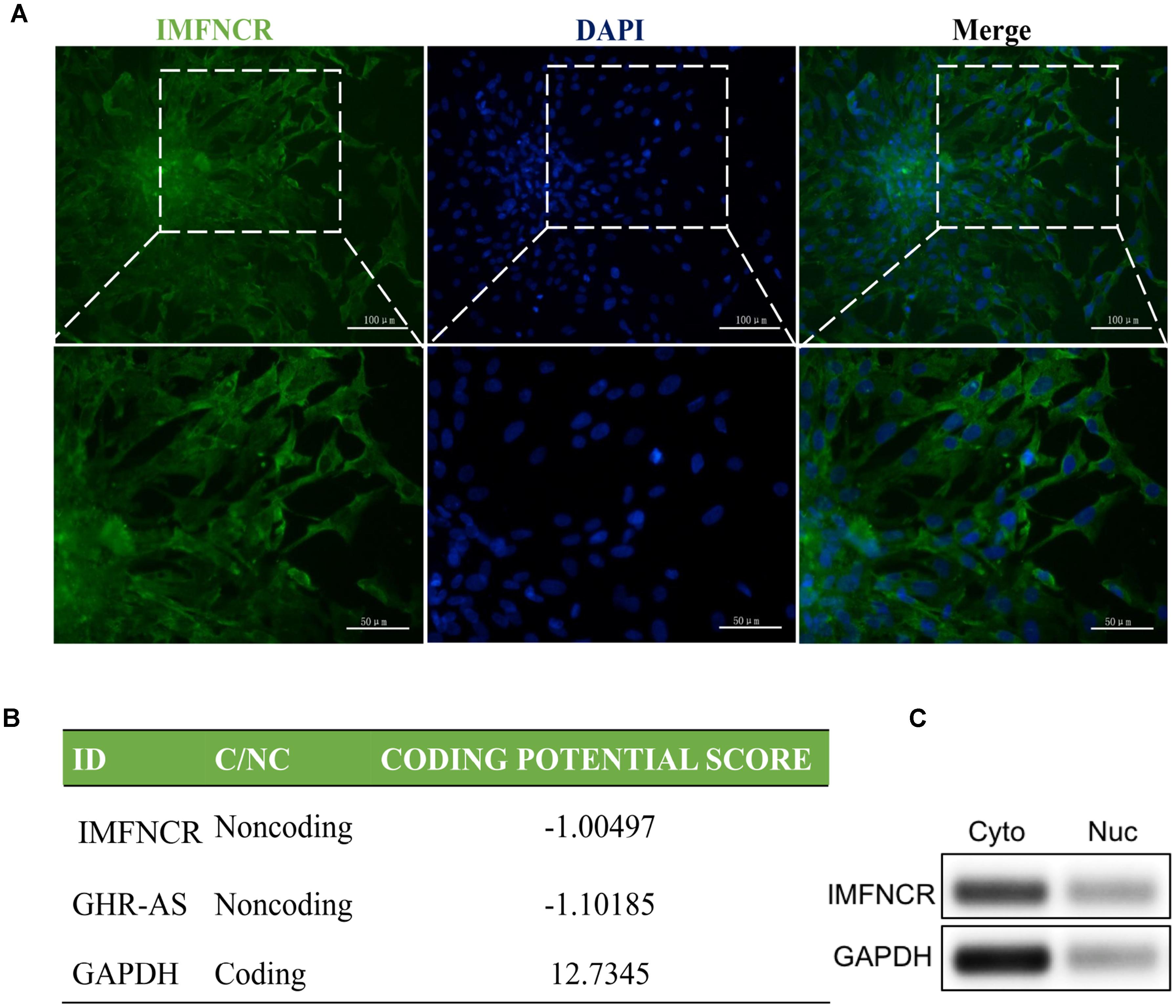
Figure 3. Subcellular localization and encoding ability prediction of IMFNCR. (A) Intramuscular preadipocyte were stained with FITC-labeled IMFNCR probes and visualized by fluorescence. (B) The RNA sequences of IMFNCR, GHR-AS, and GAPDH were put into the Coding Potential Calculator (CPC) program, and both IMFNCR and GHR-AS were predicted to be non-coding RNAs, while GAPDH was identified to code for protein. (C) IMFNCR is mainly localized in the cytoplasm of intramuscular preadipocyte. RNA isolated from cytoplasm (Cyto) and nuclear (Nuc) fractions of preadipocyte and adipocyte was used to analyze the expression level of IMFNCR by semi-quantitative PCR. GAPDH mRNA was used as control.
IMFNCR Promotes Intramuscular Adipocyte Differentiation
To explore the function of IMFNCR in IMF deposition, we constructed intramuscular adipocyte differentiation model according to our previous research (Zhang et al., 2018). After inducing with adipogenic agents for 10 days, chicken intramuscular adipocyte was fully differentiated and filled with large lipid droplets (Figure 4A). QPCR results suggested that IMFNCR and adipocyte markers PPARG and CEBPA were significantly upregulated with adipocyte differentiation (Figure 4B). miR-27b-3p has been identified to participate in adipocyte differentiation (Lin et al., 2009). Interestingly, we noticed that miR-128-3p share almost the same seed region with miR-27b-3p, which indicates that they may target the same genes. There are 322 intersection genes between miR-128-3p and miR-27b-3p. Bioinformatics analysis showed that there exist a putative miR-128-3p and miR-27b-3p binding site in IMFNCR sequence (Figure 5A). qRT-PCR result showed that miR-128-3p and miR-27b-3p were significantly downregulated, while IMFNCR and PPARG were significantly upregulated with intramuscular adipocyte differentiation (Figure 5B). Furthermore, we noticed that those target genes were mainly enriched in Insulin signaling pathway, MAPK signaling pathway, Focal adhesion, and PPARG signaling pathway (Figure 5C).
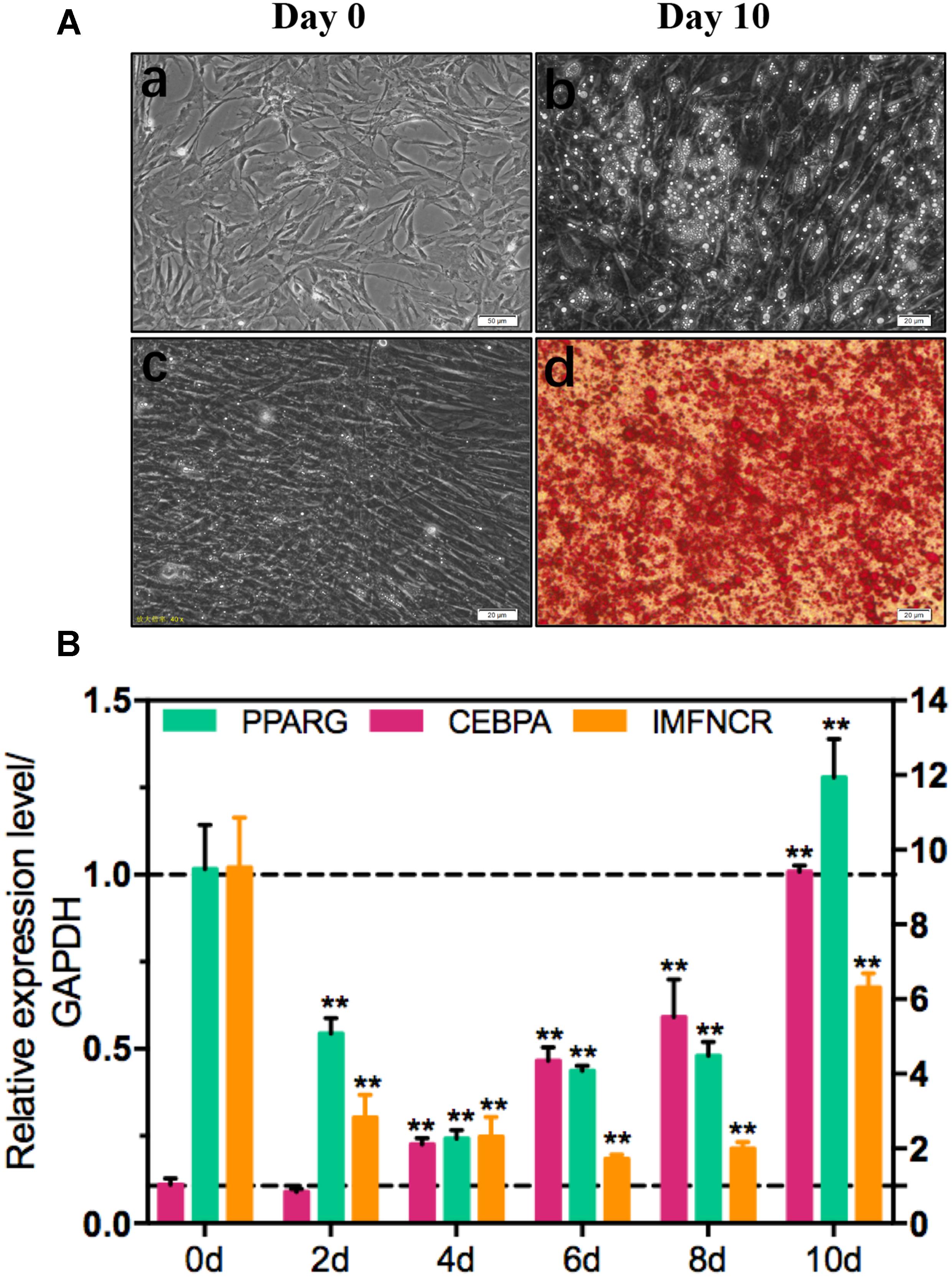
Figure 4. Induction of chicken intramuscular preadipocyte differentiation. Oil Red O staining (A) and qRT-PCR (B) analysis of IMFNCR and adipogenic markers, PPARG, and CEBPA confirm the identity of chicken intramuscular preadipocyte. Gene expression is plotted as fold-change relative to day 0 (mean ± SEM, n = 3, ∗p ≤ 0.05, ∗∗p ≤ 0.01).
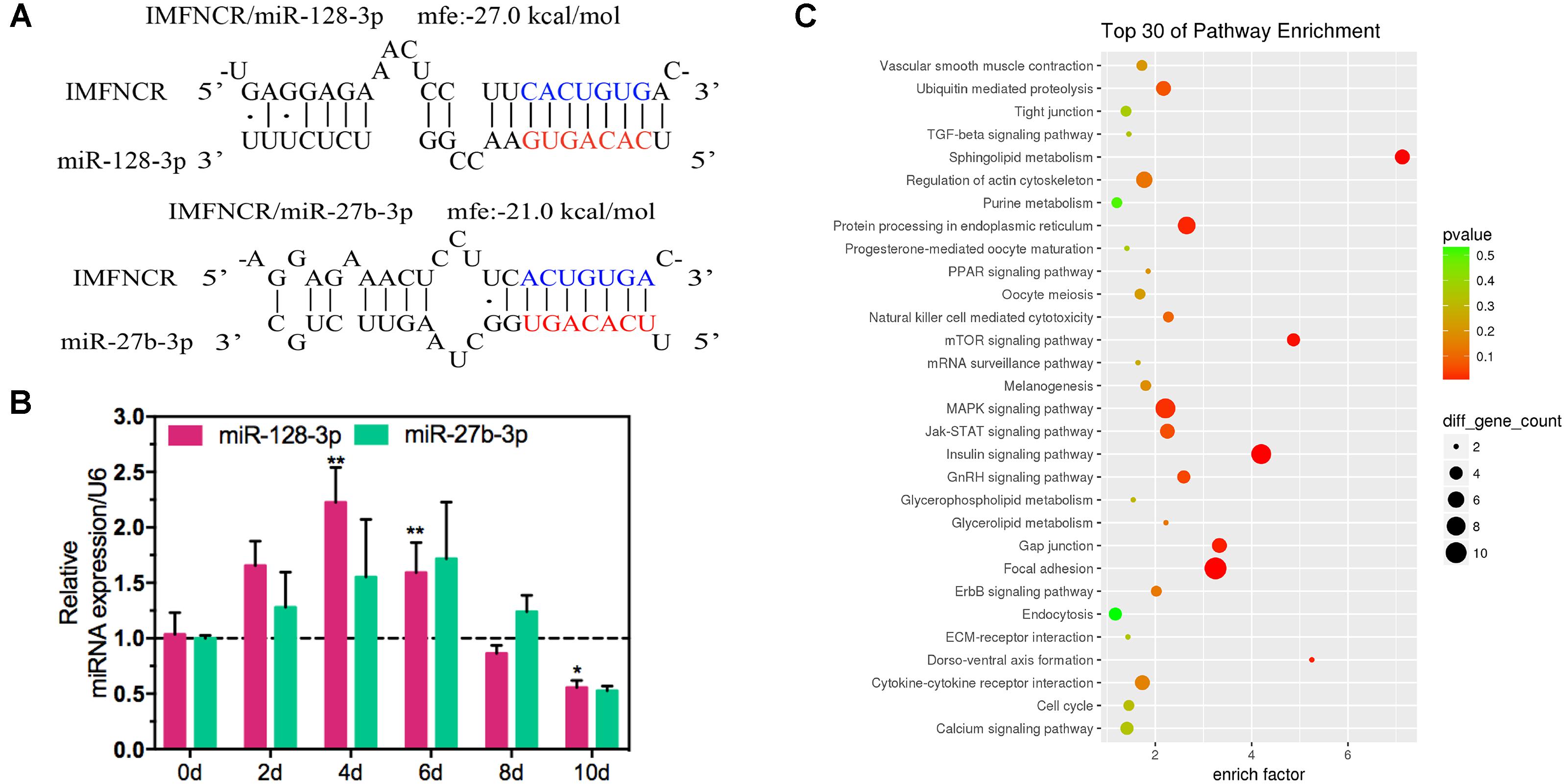
Figure 5. lncRNA IMFNCR was a potential target gene of miR-128-3p and miR-27b-3p. (A) RNA hybrid predicted miR-128-3p and miR-27b-3p binding sites in IMFNCR. Mfe, minimal free energy (kcal/mol). Nucleotides of the miR-128-3p and miR-27b-3p seed region (positions 2–8) are marked in red. Mutated nucleotides are in blue. (B) Expression pattern of miR-128-3p and miR-27b-3p during chicken intramuscular preadipocyte differentiation. (C) KEGG pathway analysis of intersection target genes of miR-128-3p and miR-27b-3p. (mean ± SEM, n = 6, ∗p ≤ 0.05, ∗∗p ≤ 0.01).
To better understand the role of IMFNCR function, we performed knockdown IMFNCR in intramuscular adipocyte (Figure 6A). Our result showed that siRNAs specific to IMFNCR reduced its RNA level by 40 and 44%, and the expression of adipogenic markers PPARG and FABP4 decreased significantly (Figures 6A,B). Moreover, Oil red O staining suggested that knockdown of IMFNCR inhibited adipogenesis (Figure 6C). Furthermore, miR-128-3p and miR-27b-3p was significantly upregulated in IMFNCR knockdown group compared with control group (Figure 6D). When we overexpressed miR-128-3p or miR-27b-3p, the expression level of IMFNCR decreased significantly (Figure 6E). We observed that miR-128-3p or miR-27b-3p and both miR-128-3p and miR-27b-3p significantly reduced the Rluc activity of the wild-type IMFNCR reporter vector (psiCH2-IMFNCR-WT) in DF1 cells, respectively (Figure 6F).
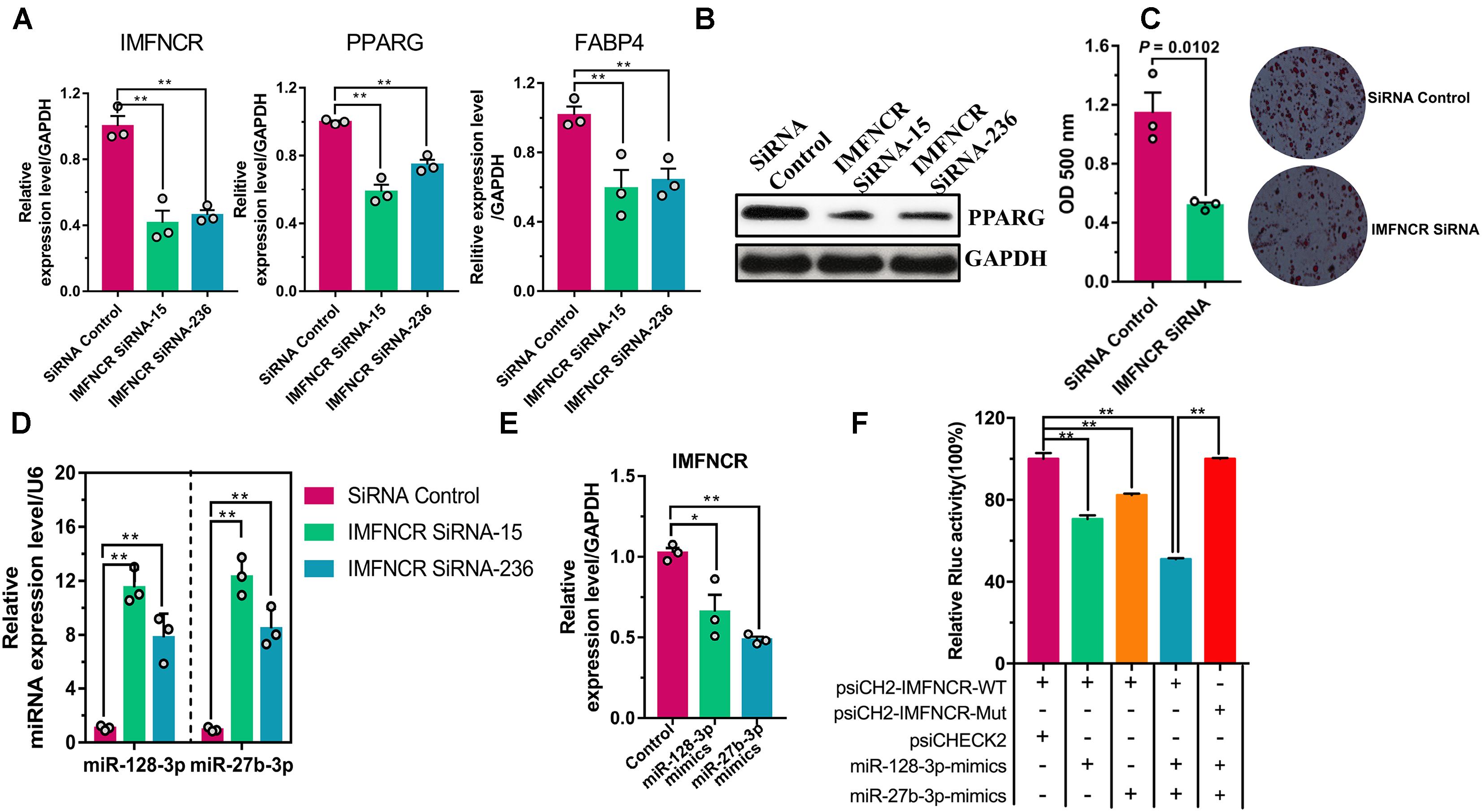
Figure 6. lncRNA IMFNCR functions as a miRNA sponge. (A) IMFNCR knockdown decreased PPARG and FABP4 expression. (B) IMFNCR knockdown inhibited intramuscular adipogenesis, as indicated by Oil Red O staining and quantified by microplate reader at 510 nm. (C) IMFNCR knockdown inhibited the protein expression of PPARG. (D) IMFNCR knockdown increased the expression of miR-128-3p and miR-27b-3p. (E) miR-128-3p and miR-27b-3p inhibited the expression of IMFNCR. (F) miR-128-3p and miRNA mixture overexpression inhibited Rluc expression of the Rluc activity of psiCH2-IMFNCR-WT, while psiCH2-IMFNCR-WT-Mut no longer responded to miRNA mixture (miR-128-3p and miR-27b-3p). MiR-128-3p and miR-27b-3p mimics were transfected into DF1 cells, together with psiCH2-IMFNCR-WT, or psiCH2-IMFNCR-WT-Mut. The data represent means ± SEM (n = 3). ∗p ≤ 0.05; ∗∗p ≤ 0.01.
miR-128-3p and miR-27b-3p Inhibits Adipogenesis by Targeting PPARG
In search of potential downstream effectors of IMFNCR-miR-128-3p/miR-27b-3p mediated regulation of intramuscular adipocyte differentiation, we focused on PPARG, a major regulator of adipogenic genes. Bioinformatics analysis for miRNA recognition sequences on chicken PPARG also revealed the presence of a putative miR-128-3p and miR-27b-3p binding site (Figure 7A). To verify the correlation between PPARG and miR-128-3p and miR-27b-3p in chicken, we fused the 3′UTR of chicken PPARG to a luciferase reporter gene. A remarkable repression of Rluc activity of psiCHECK2-PPARG-WT reporter was observed in DF1 cells transfected with miR-128-3p and miR-27b-3p, whereas, the Rluc activity of psiCHECK2-PPARG-WT transfected with both miR-128-3p and miR-27b-3p mimics group was significantly reduced comparing with psiCHECK2-PPARG-Mut group (Figure 7B). In addition, overexpressed and knockdown miR-128-3p altered PPARG and IRS1 (a well-known target gene of miR-128-3p) expression levels in chicken intramuscular adipocyte (Figures 7C,D). These results suggested that PPARG is indeed a direct target of miR-128-3p and miR-27b-3p in chicken.
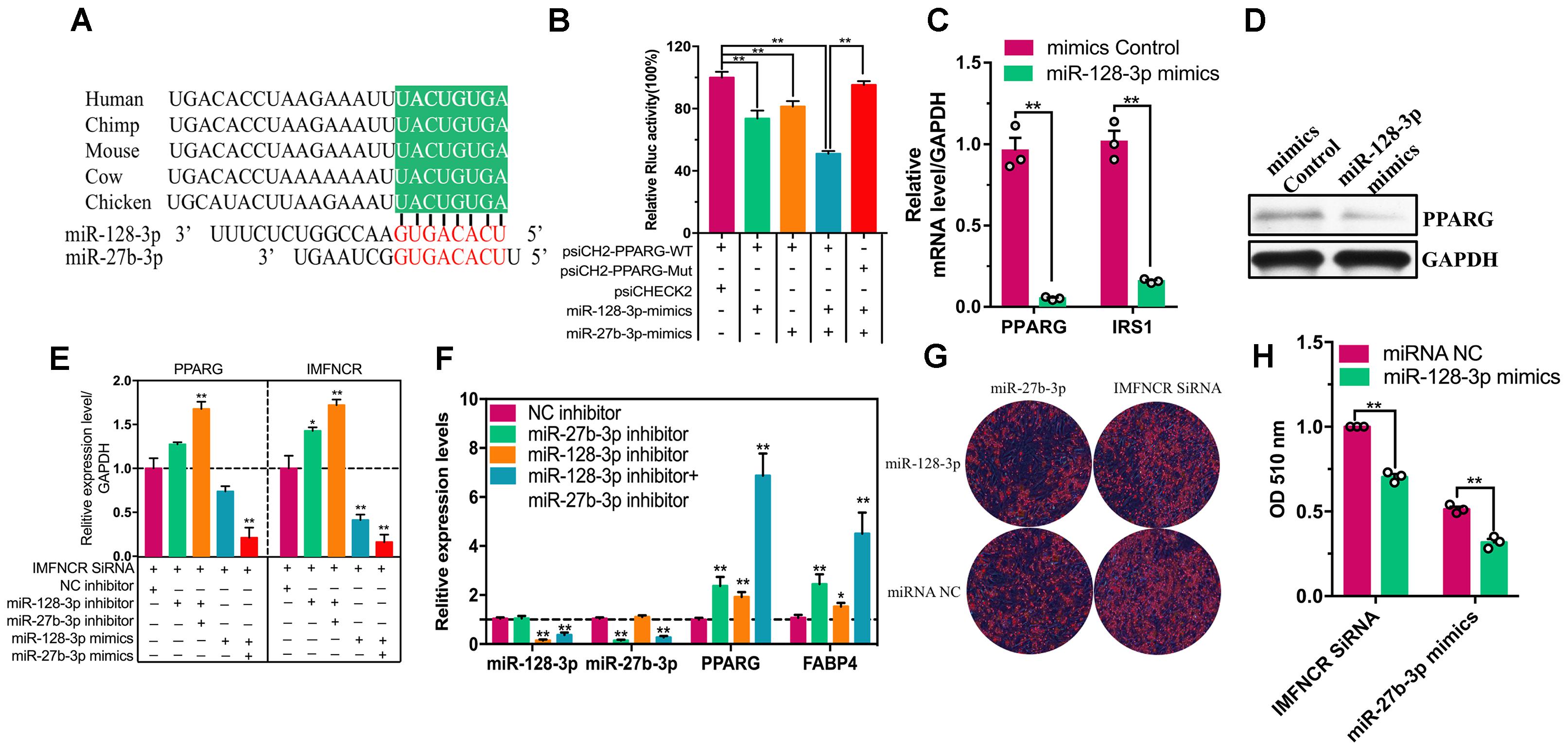
Figure 7. IMFNCR promotes intramuscular adipocyte differentiation by sponging miR-128-3p and miR-27b-3p. (A) The putative miR-128-3p and miR-27b-3p-binding sites at PPARG 3′UTR (green) are evolutionarily conserved across species. (B) miR-128-3p and miR-27b-3p suppresses PPARG translation. miR-128-3p and miR-27b-3p were transfected into DF1 cells, along with PPARG-UTR-WT but not PPARG-UTR-Mut. The luciferase activity was analyzed 48 h later. (C) miR-128-3p suppresses the expression of PPARG and IRS1. Intramuscular preadipocyte was infected with miR-128-3p mimics or mimics Control at 37°C, followed by the addition of fresh growth medium. RNA and protein were extracted 48 h later, and levels were accessed by qRT-PCR and (D) western blot. (E) IMFNCR siRNA and inhibitor (miR-128-3p inhibitor, mixture inhibitor) or mimics (miR-128-3p mimics, mixture mimics) were transfected into chicken intramuscular preadipocyte. qRT-PCR assays were performed to determine the expression levels of PPARG and IMFNCR. (F) Negative control inhibitor (NC inhibitor) or miR-128-3p or mixture (miR-128-3p and miR-27b-3p) inhibitor was transfected into chicken intramuscular preadipocyte. Forty-eight hours later, qRT-PCR assays were performed to determine the expression levels of miR-128-3p, miR-27b-3p, PPARG, and FABP4. (G) IMFNCR siRNA and miR-27b-3p were transfected into miRNA mimics NC or miR-128-3p mimics. At 48 h intracellular triglyceride content was measured by Oil Red O staining and (H) quantified by microplate reader at 510 nm. The data represent means ± SEM (n = 3). ∗p ≤ 0.05; ∗∗p ≤ 0.01.
IMFNCR Increases the Expression of PPARG in a miR-128-3p and miR-27b-3p Dependent Manner
To confirm that the observed effects of IMFNCR were due to miR-128-3p and miR-27b-3p-mediated regulation of PPARG, we transfected miR-128-3p and miR-27b-3p mimics or inhibitor into chicken intramuscular preadipocyte. As expected, miR-128-3p overexpression strongly decreased the mRNA levels of IMFNCR and PPARG and inhibited adipogenesis (Figures 6E, 7G,H). In a reciprocal experiment, increased mRNA levels of both IMFNCR and PPARG were observed in miR-128-3p inhibitor and mix (mixed miR-128-3p and miR-27b-3p inhibitor-treated cells) group (Figure 7E). As shown in Figure 7F, miR-27b-3p and miR-128-3p inhibitor increased the mRNA levels of adipogenic markers (PPARG and FABP4). These results agreed with the ceRNA hypothesis that IMFNCR inhibits miR-128-3p and miR-27b-3p function, leading to derepression of their target gene PPARG.
Effects of Dietary Nutrient Level on IMFNCR Expression
In order to understand the effect of different nutrient levels on miRNA expression, chicken was fed with high fat (HFD) and high protein diet (HPD) for 2 weeks. As indicated in Figures 8A,B, the expression level of IMFNCR and PPARG were significantly decreased in both HFD and FPD groups (P < 0.01) (Figures 8A,B). Moreover, a significantly positive correlation of expression between IMFNCR and PPARG was observed in chicken breast muscle tissues (P = 0.0075 and P = 0.0007, respectively) (Figure 8).
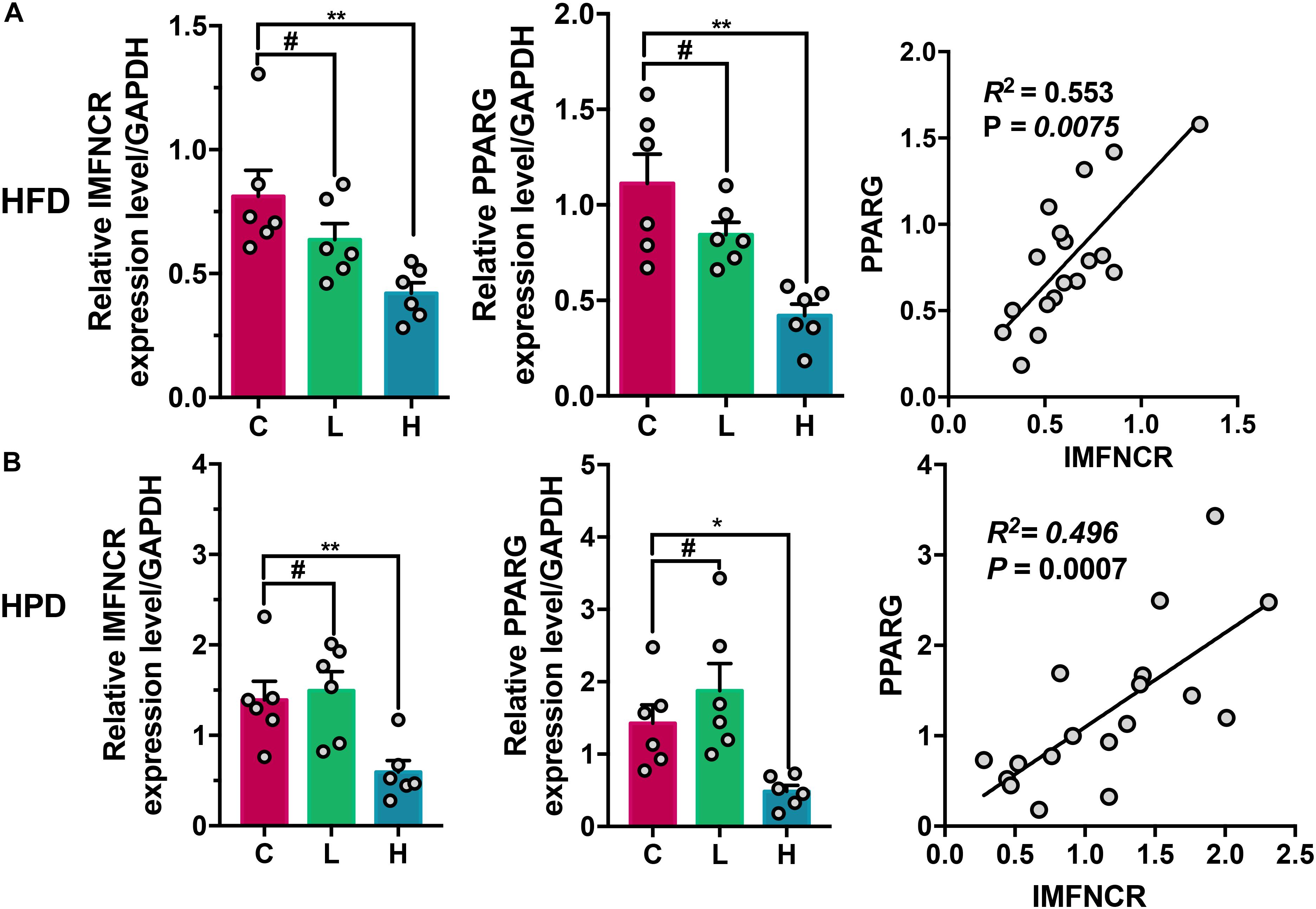
Figure 8. Effects of dietary nutrient level on IMFNCR expression. (A) HFD inhibits IMFNCR expression in breast muscle tissues. The breast muscle tissues of chicken were collected after 3-weeks feeding (C, control diet group; L, low-fat diet group; H, high-fat diet group). (B) HPD inhibits IMFNCR expression in breast muscle tissues. The breast muscle tissues of chicken were collected after 3-weeks feeding (C, control diet group; L, low-protein diet group; H, high-protein diet group). Data are presented as means ± SEM, n = 6, ∗p ≤ 0.05, ∗∗p ≤ 0.01. #No significant difference.
Taken together, these results strongly suggest that IMFNCR, which was regulated by nutrient factor and by binding both miR-128-3p and miR-27b-3p, acts as a decoy to abolish miRNA repressing activity on PPARG and thereby promotes chicken intramuscular differentiation (Figure 9).
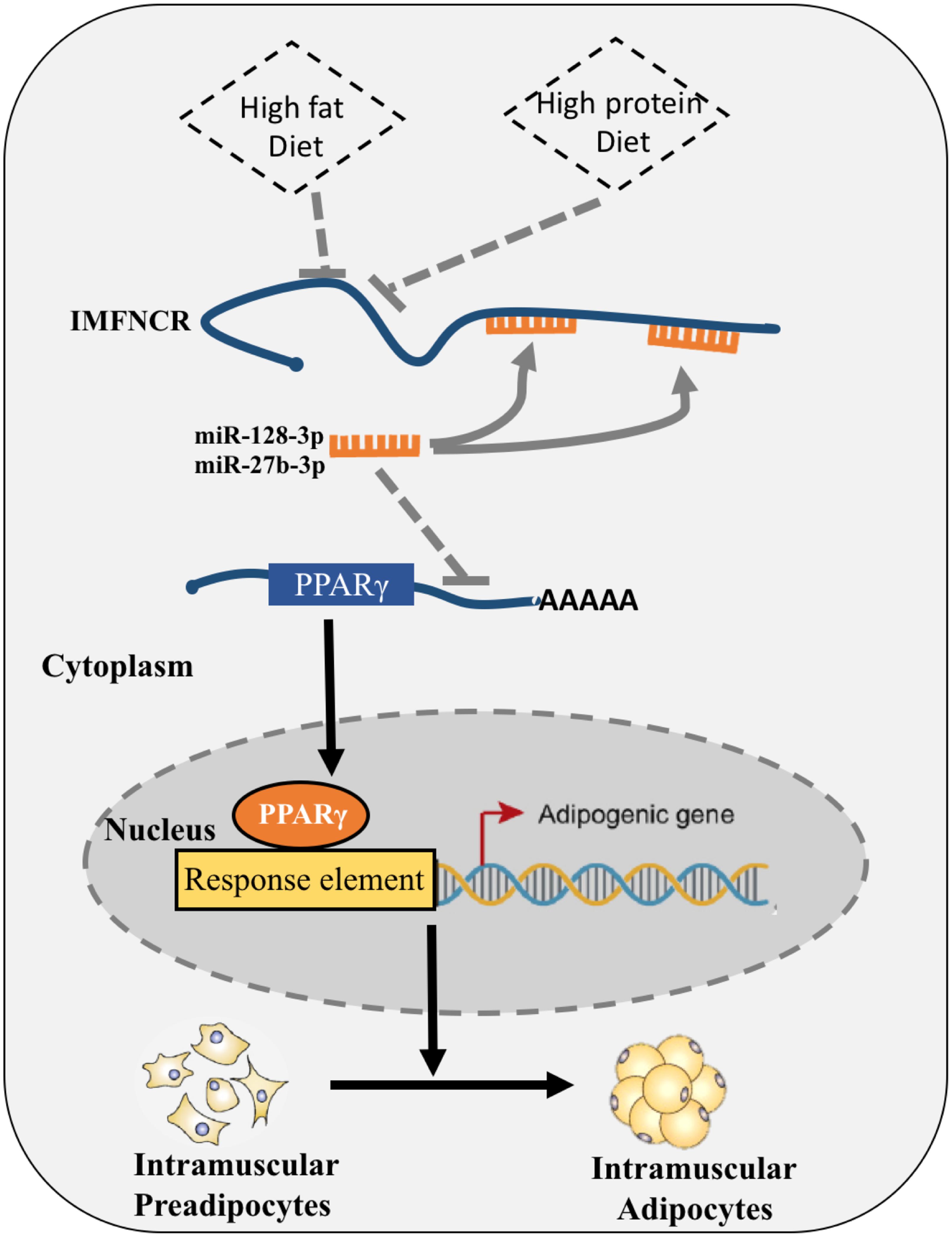
Figure 9. Proposed model of IMFNCR regulation on intramuscular adipocyte differentiation. High-protein and High-fat diet inhibit the expression of IMFNCR; IMFNCR promotes intramuscular adipogenesis differentiation by functioning as a ceRNA, which sequesters miR-128-3p and miR-27b-3p, thereby protecting PPARG transcripts from miRNA-mediated suppression in chicken.
Discussion
Meat quality is affected by many factors, among which IMF content contributes to the tenderness, juiciness and flavor of the meat (Aaslyng et al., 2003). IMF is mainly deposited in the muscle mass with fat, distributed in the myometrium, myofibrils, and endometrium, as well as in the form of lipid droplets in the muscle cytoplasm, which includes intracellular phospholipid, triglyceride, and cholesterol (Qiu et al., 2017; Zhang et al., 2018). IMF content is an important quantitative trait, which is affected by both nutritional and genetic factors, such as mRNA, DNA methylation and miRNAs (Gerbens et al., 2001; Cui et al., 2012; Chen et al., 2017; Du et al., 2018). Our previous studies have found that the late-laying-period hens exhibited a higher IMF content in breast muscle tissues, moreover, some miRNAs regulated mRNAs to affect IMF content (Zhang et al., 2017, 2018). Several lncRNAs have been identified and suggested to regulate adipogenesis, such as steroid receptor RNA Activator (SRA) (Xu et al., 2010), adipogenic differentiation induced non-coding RNA (ADINR) (Xiao et al., 2015) and adipocyte differentiation-associated long non-coding RNA (ADNCR) (Li et al., 2016). These individual researches demonstrate the growing importance of lncRNAs in adipogenesis, but our knowledge of lncRNAs related to intramuscular adipocyte differentiation still remains limited.
In the present study, we reanalyzed the transcriptome data of chicken breast muscle tissue at different physiological periods. Further analysis revealed a large number of differentially expressed lncRNAs among different development stages, including IMFNCR, a novel fat-specific lncRNA that can promote adipogenesis in primary chicken intramuscular adipocyte. Furthermore, we found IMFNCR promotes chicken intramuscular adipocyte differentiation via binding miR-128-3p and miR-27b-3p.
LncRNAs have been suggested to function as miRNA sponges, however, only a few of such lncRNAs have been reported to have functions in adipogenesis. For instance, lncRNA ADNCR acts as a ceRNA by sponging miR-204 thereby protecting SIRT1 transcripts in bovine Adipocyte-derived stem cells (ADSCs) (Li et al., 2016). Here we provide direct evidence that IMFNCR can function as a ceRNA by sponging miR-128-3p and miR-27b-3p, thereby protecting PPARG transcripts from miR-128-3p- and miR-27-3p-mediated suppression (Figure 9). IMFNCR RNAi and miRNA (miR-128-3p, miR-27b-3p or mix miR-128-3p and miR-27b-3p) overexpression in chicken intramuscular preadipocyte produced a similar decrease in the expression of adipogenic markers (PPARG and FABP4), whereas IMFNCR RNAi and miRNA (miR-128-3p, miR-27b-3p or mix miR-128-3p and miR-27b-3p) knockdown led to increased adipogenic differentiation.
In search of potential downstream effector of IMFNCR/miR-128-3p and miR-27b-3p mediated regulation of intramuscular adipocyte differentiation, we focused on PPARG, a major regulator of adipogenic differentiation (Nedergaard et al., 2005; Bai et al., 2007). Wang et al. (2011) found that miR-27a suppressed porcine adipocyte differentiation. Kim et al. (2010) found that miR-27a would suppress adipocyte differentiation through targeting PPARG. Qiu et al. (2013) also found that miR-128a inhibit the differentiation of pre-adipocyte in rat via regulating the expression of PPARG. In line with the results observed from rat pre-adipocyte, our experiments suggested that miR-128-3p and miR-27b-3p were negative regulator of chicken intramuscular adipocyte differentiation. Mutation of the seed region of the predicted miR-128-3p and miR-27b-3p target site abolished the regulation of the PPARG 3′UTR reporter, demonstrating that PPARG is a direct target of miR-128-3p and miR-27b-3p in chicken intramuscular adipocyte differentiation. Furthermore, we show that the observed effects of IMFNCR on adipogenic differentiation were due to miR-128-3p and miR-27b-3p mediated regulation of PPARG. IMFNCR modulates PPARG expression by competing for miR-128-3p and miR-27b-3p as a ceRNA to regulate intramuscular adipocyte differentiation.
Nutrients can regulate gene expression in the body’s activities at various levels and pathways, that is, nutrients affect the growth, development, and reproduction of animals (Hsu and Ding, 2003; Zhou et al., 2010). One of the ways is to influence gene expression. Tian et al. (2017) found that feeding low-protein diets can promote fatty acid transport and synthesis-related genes in the longissimus dorsi (LDM), but the deposition of IMF in pig LDM is significantly reduced. Several studies suggest that the addition of PUFAs diets inhibit fat accumulation and adipocyte differentiation (Madsen et al., 2005; Zhou et al., 2010; Wójcik et al., 2014). Hsu et al. demonstrated that polyunsaturated FA inhibit the ADD1 expression and involved in lipid metabolism in porcine adipocyte (Hsu and Ding, 2003). Wójcik et al. (2014) found that omega-3 polyunsaturated fatty acids (0309-3 PUFAs) decrease expression of SREBP1 while inducing expression of adipophilin and GLUT4. Our results suggested that high-fat diet decreased the expression levels of PPARG and IMFNCR in breast muscle tissues. Increasing the energy level in the feed increases the percentage of abdominal fat and the adiponectin gene expression abundance, while lowering the dietary protein level increases the percentage of abdominal fat and adiponectin gene expression in abdominal fat (Tahmoorespur et al., 2010). In the present study, we found that high-protein diet significantly decreased both PPARG and IMFNCR mRNA levels. Moreover, the IMFNCR RNA levels was significantly positively correlated with PPARG mRNA level between different nutrient level (P < 0.001). Our results indicated that lncRNA IMFNCR may contribute to molecular genetic selection to balance IMF and abdominal fat content in chicken breeding. In addition, IMFNCR may serve as epigenetic markers for evaluating meat quality in chicken and will be likely to contribute to the improvement of poultry meat quality.
In summary, our study provides the first evidence of lncRNAs which could have functional roles in chicken intramuscular adipocyte differentiation. Importantly, we combine nutrient factor with detailed mechanistic studies to describe an adipocyte-differentiation related lncRNA, IMFNCR, that functions as a ceRNA to promote intramuscular adipogenic differentiation. Our study provides a better understanding of researches underlying intramuscular adipogenic differentiation in poultry and may also contribute to improving poultry meat quality.
Author Contributions
MZ, G-rS, and X-tK conceived of and designed the experiments. MZ, FL, and J-wS performed the experiments. MZ, D-hL, and G-xL analyzed the data. W-tL, Z-jL, X-jL, Y-bW, Y-dT, R-lH, and R-rJ contributed to reagents, materials, and analysis tools. MZ wrote the manuscript.
Funding
This study was supported by the China Agriculture Research System (CARS-40-K04), Program for Innovation Research Team of Ministry of Education (IRT16R23), Key Science and Technology Research Project of Henan Province (151100110800), National Natural Science Foundation of China (31572356), National Natural Science Foundation of China (31501948), Research on the Foundation and Frontier Technology of Henan Science and Technology Department (162300410162).
Conflict of Interest Statement
The authors declare that the research was conducted in the absence of any commercial or financial relationships that could be construed as a potential conflict of interest.
Supplementary Material
The Supplementary Material for this article can be found online at: https://www.frontiersin.org/articles/10.3389/fgene.2019.00042/full#supplementary-material
FIGURE S1 | Characterization of the IMFNCR Sequence.
TABLE S1 | Specific primers used for qRT-PCR.
References
Aaslyng, M. D., Bejerholm, C., Ertbjerg, P., Bertram, H. C., and Andersen, H. J. (2003). Cooking loss and juiciness of pork in relation to raw meat quality and cooking procedure. Food Q. Prefer. 14, 277–288. doi: 10.1016/S0950-3293(02)00086-1
Bai, P., Houten, S. M., Huber, A., Schreiber, V., Watanabe, M., Kiss, B., et al. (2007). PARP-2 controls adipocyte differentiation and adipose tissue function through the regulation of the activity of the RXR/PPARgamma heterodimer. Exp. Eye Res. 17, 765–771.
Batista, P. J., and Chang, H. Y. (2013). Long noncoding RNAs: cellular address codes in development and disease. Cell 152, 1298–1307. doi: 10.1016/j.cell.2013.02.012
Cesana, M., Cacchiarelli, D., Legnini, I., Santini, T., Sthandier, O., Chinappi, M., et al. (2011). A long noncoding RNA controls muscle differentiation by functioning as a competing endogenous RNA. Cell 147, 358–369. doi: 10.1016/j.cell.2011.09.028
Chen, C., Deng, Y., Hu, X., Ren, H., Zhu, J., Fu, S., et al. (2018). miR-128-3p regulates 3T3-L1 adipogenesis and lipolysis by targeting Pparg and Sertad2. J. Physiol. Biochem. 74, 381–393. doi: 10.1007/s13105-018-0625-1
Chen, F. F., Xiong, Y., Peng, Y., Gao, Y., Qin, J., Chu, G. Y., et al. (2017). miR-425-5p inhibits differentiation and proliferation in porcine intramuscular preadipocytes. Int. J. Mol. Sci. 18:E2101. doi: 10.3390/ijms18102101
Cui, H. X., Liu, R. R., Zhao, G. P., Zheng, M. Q., Chen, J. L., and Jie, W. (2012). Identification of differentially expressed genes and pathways for intramuscular fat deposition inpectoralis majortissues of fast-and slow-growing chickens. BMC Genomics 13:213. doi: 10.1186/1471-2164-13-213
Du, J., Xu, Y., Zhang, P., Zhao, X., Gan, M., Li, Q., et al. (2018). MicroRNA-125a-5p affects adipocytes proliferation, differentiation and fatty acid composition of porcine intramuscular fat. Int. J. Mol. Sci. 19:E501. doi: 10.3390/ijms19020501
Gerbens, F., Verburg, F. J., Van Moerkerk, H. T., Engel, B., Buist, W., Veerkamp, J. H., et al. (2001). Associations of heart and adipocyte fatty acid-binding protein gene expression with intramuscular fat content in pigs. J. Anim. Sci. 79, 347–354. doi: 10.2527/2001.792347x
Hsu, J. M., and Ding, S. T. (2003). Effect of polyunsaturated fatty acids on the expression of transcription factor adipocyte determination and differentiation-dependent factor 1 and of lipogenic and fatty acid oxidation enzymes in porcine differentiating adipocytes. Br. J. Nutr. 90, 507–513. doi: 10.1079/BJN2003918
Karbiener, M., Fischer, C., Nowitsch, S., Opriessnig, P., Papak, C., Ailhaud, G., et al. (2009). RNA miR-27b impairs human adipocyte differentiation and targets PPARgamma. Biochem. Biophys. Res. Commun. 390, 247–251. doi: 10.1016/j.bbrc.2009.09.098
Kim, S. Y., Kim, A. Y., Lee, H. W., Son, Y. H., Lee, G. Y., Lee, J. W., et al. (2010). miR-27a is a negative regulator of adipocyte differentiation via suppressing PPARgamma expression. Biochem. Biophys. Res. Commun. 392, 323–328. doi: 10.1016/j.bbrc.2010.01.012
Koerner, M. V., Pauler, F. M., Huang, R., and Barlow, D. P. (2009). The function of non-coding RNAs in genomic imprinting. Development 136, 1771–1783. doi: 10.1242/dev.030403
Kretschmer-Kazemi Far, R., and Sczakiel, G. (2003). The activity of siRNA in mammalian cells is related to structural target accessibility: a comparison with antisense oligonucleotides. Nucleic Acids Res. 31, 4417–4424. doi: 10.1093/nar/gkg649
Legnini, I., Morlando, M., Mangiavacchi, A., Fatica, A., and Bozzoni, I. (2014). A feedforward regulatory loop between HuR and the long noncoding RNA linc-MD1 controls early phases of myogenesis. Mol. Cell 53, 506–514. doi: 10.1016/j.molcel.2013.12.012
Lei, S., and Mirko, T. (2014). MiR-27 orchestrates the transcriptional regulation of brown adipogenesis. Metab. Clin. Exp. 63, 272–282. doi: 10.1016/j.metabol.2013.10.004
Li, M., Sun, X., Cai, H., Sun, Y., Plath, M., Li, C., et al. (2016). Long non-coding RNA ADNCR suppresses adipogenic differentiation by targeting miR-204. Biochim. Biophys. Acta 1859, 871–882. doi: 10.1016/j.bbagrm.2016.05.003
Lin, Q., Gao, Z., Alarcon, R. M., Ye, J., and Yun, Z. (2009). A role of miR-27 in the regulation of adipogenesis. FEBS J. 276, 2348–2358. doi: 10.1111/j.1742-4658.2009.06967.x
Madsen, L., Petersen, R. K., and Kristiansen, K. (2005). Regulation of adipocyte differentiation and function by polyunsaturated fatty acids. Biochim. Biophys. Acta 1740, 266–286. doi: 10.1016/j.bbadis.2005.03.001
Mueller, A. C., Cichewicz, M. A., Dey, B. K., Layer, R., Reon, B. J., Gagan, J. R., et al. (2015). MUNC, a long noncoding RNA that facilitates the function of MyoD in skeletal myogenesis. Mol. Cell. Biol. 35, 498–513. doi: 10.1128/MCB.01079-14
Nedergaard, J., Petrovic, N., Lindgren, E. M., Jacobsson, A., and Cannon, B. (2005). PPARgamma in the control of brown adipocyte differentiation. Biochim. Biophys. Acta 1740, 293–304. doi: 10.1016/j.bbadis.2005.02.003
Qiu, F., Xie, L., Ma, J. E., Luo, W., Zhang, L., Chao, Z., et al. (2017). Lower expression of SLC27A1 enhances intramuscular fat deposition in chicken via down-regulated fatty acid oxidation mediated by CPT1A. Front. Physiol. 8:449. doi: 10.3389/fphys.2017.00449
Qiu, Y., Liu, S., Ning, X. M., Dong, P. Y., Cheng, J., He, J. J., et al. (2013). Overexpression of miR-128a inhibits rat precursor adipocyte differentiation. J. Agric. Biotechnol. 21, 388–395. doi: 10.3969/j.issn.1674-7968.2013.04.002
Ramsay, T. G., and Rosebrough, R. W. (2003). Hormonal regulation of postnatal chicken preadipocyte differentiation in vitro. Comp. Biochem. Physiol. B 136, 245–253. doi: 10.1016/S1096-4959(02)00261-0
Rosen, E. D., Hsu, C. H., Wang, X., Sakai, S., Freeman, M. W., Gonzalez, F. J., et al. (2002). C/EBPα induces adipogenesis through PPARγ: a unified pathway. Genes Dev. 16, 22–26. doi: 10.1101/gad.948702
Salmena, L., Poliseno, L., Tay, Y., Kats, L., and Pandolfi, P. P. (2011). ceRNA hypothesis: the rosetta stone of a hidden RNA language? Cell 146, 353–358. doi: 10.1016/j.cell.2011.07.014
Sun, L., Goff, L. A., Trapnell, C., Alexander, R., Lo, K. A., Hacisuleyman, E., et al. (2013). Long noncoding RNAs regulate adipogenesis. Proc. Natl. Acad. Sci. U. S. A. 110, 3387–3392. doi: 10.1073/pnas.1222643110
Sun, X., Li, M., Sun, Y., Cai, H., Lan, X., Huang, Y., et al. (2016). The developmental transcriptome sequencing of bovine skeletal muscle reveals a long noncoding RNA, lncMD, promotes muscle differentiation by sponging miR-125b. Biochim. Biophys. Acta 1863, 2835–2845. doi: 10.1016/j.bbamcr.2016.08.014
Tahmoorespur, M., Ghazanfari, S., and Nobari, K. (2010). Evaluation of adiponectin gene expression in the abdominal adipose tissue of broiler chickens: feed restriction, dietary energy, and protein influences adiponectin messenger ribonucleic acid expression. Poult. Sci. 89, 2092–2100. doi: 10.3382/ps.2010-00772
Tian, Z., Xian-yong, M. A., Wang, L., Xiong, Y., Qiu, Y., Yang, X., et al. (2017). Effects of long-term feeding diets with different protein levels on gene expressions related to lipid metabolism of pigs. Chin. J. Anim. Nutr. 29, 3761–3772.
Tripathi, V., Shen, Z., Chakraborty, A., Giri, S., Freier, S. M., Wu, X., et al. (2013). Long noncoding RNA MALAT1 controls cell cycle progression by regulating the expression of oncogenic transcription factor B-MYB. PLoS Genetics 9:e1003368. doi: 10.1371/journal.pgen.1003368
Wang, T., Li, M., Guan, J., Li, P., Wang, H., Guo, Y., et al. (2011). MicroRNAs miR-27a and miR-143 regulate porcine adipocyte lipid metabolism. Int. J. Mol. Sci. 12, 7950–7959. doi: 10.3390/ijms12117950
Wójcik, C., Lohe, K., Kuang, C., Xiao, Y., Jouni, Z., and Poels, E. (2014). Modulation of adipocyte differentiation by omega-3 polyunsaturated fatty acids involves the ubiquitin-proteasome system. J. Cell. Mol. Med. 18, 590–599. doi: 10.1111/jcmm.12194
Xiao, T., Liu, L., Li, H., Yu, S., Luo, H., Li, T., et al. (2015). Long noncoding RNA ADINR regulates adipogenesis by transcriptionally activating C/EBPα. Stem Cell Rep. 5, 856–865. doi: 10.1016/j.stemcr.2015.09.007
Xu, B., Gerin, I., Miao, H., Vuphan, D., Johnson, C. N., Xu, R., et al. (2010). Multiple roles for the non-coding RNA SRA in regulation of adipogenesis and insulin sensitivity. PLoS One 5:e14199. doi: 10.1371/journal.pone.0014199
Zhang, L., Lin, S., An, L., Ma, J., Qiu, F., Jia, R., et al. (2016). Chicken GHR natural antisense transcript regulates GHR mRNA in LMH cells. Oncotarget 7, 73607–73617. doi: 10.18632/oncotarget.12437
Zhang, M., Li, D. H., Li, F., Sun, J. W., Jiang, R. R., Li, Z. J., et al. (2018). Integrated analysis of MiRNA and genes associated with meat quality reveals that Gga-MiR-140-5p affects intramuscular fat deposition in chickens. Cell. Physiol. Biochem. 46, 2421–2433. doi: 10.1159/000489649
Zhang, M., Yan, F. B., Li, F., Jiang, K. R., Li, D. H., Han, R. L., et al. (2017). Genome-wide DNA methylation profiles reveal novel candidate genes associated with meat quality at different age stages in hens. Sci. Rep. 7:45564. doi: 10.1038/srep45564
Keywords: long non-coding RNA, ceRNA, miRNA, intramuscular adipocyte differentiation, chicken
Citation: Zhang M, Li F, Sun J-w, Li D-h, Li W-t, Jiang R-r, Li Z-j, Liu X-j, Han R-l, Li G-x, Wang Y-b, Tian Y-d, Kang X-t and Sun G-r (2019) LncRNA IMFNCR Promotes Intramuscular Adipocyte Differentiation by Sponging miR-128-3p and miR-27b-3p. Front. Genet. 10:42. doi: 10.3389/fgene.2019.00042
Received: 14 August 2018; Accepted: 21 January 2019;
Published: 11 February 2019.
Edited by:
Douglas Mark Ruden, Wayne State University, United StatesReviewed by:
Gokul Gopinath, Texas A&M University Baylor College of Dentistry, United StatesShicheng Guo, Marshfield Clinic Research Institute, United States
Copyright © 2019 Zhang, Li, Sun, Li, Li, Jiang, Li, Liu, Han, Li, Wang, Tian, Kang and Sun. This is an open-access article distributed under the terms of the Creative Commons Attribution License (CC BY). The use, distribution or reproduction in other forums is permitted, provided the original author(s) and the copyright owner(s) are credited and that the original publication in this journal is cited, in accordance with accepted academic practice. No use, distribution or reproduction is permitted which does not comply with these terms.
*Correspondence: Gui-rong Sun, Z3JzdW4yMDAwQDEyNi5jb20=