- 1Department of Genome Medicine and Science, College of Medicine, Gachon University, Incheon, South Korea
- 2Gachon Institute of Genome Medicine and Science, Gachon University Gil Medical Center, Incheon, South Korea
- 3Gachon Advanced Institute of Health Sciences and Technology, Gachon University, Incheon, South Korea
- 4Department of Life Sciences, Gachon University, Seongnam, South Korea
- 5Division of Gastroenterology, Department of Internal Medicine, Gachon University Gil Medical Center, School of Medicine, Gachon University, Incheon, South Korea
- 6Gachon Medical Research Institute, Gachon University Gil Medical Center, Incheon, South Korea
- 7Department of Internal Medicine, Gachon University Gil Medical Center, Incheon, South Korea
- 8Department of Internal Medicine, Gachon University College of Medicine, Incheon, South Korea
The well-known signal mediator and small GTPase family member, RHOA, has now been associated with the progression of specific malignancies. In this review, we appraise the biomedical literature regarding the role of this enzyme in gastric cancer (GC) signaling, suggesting potential clinical significance. To that end, we examined RHOA activity, with regard to second-generation hallmarks of cancer, finding particular association with the hallmark “activation of invasion and metastasis.” Moreover, an abundance of studies show RHOA association with Lauren classification diffuse subtype, in addition to poorly differentiated GC. With regard to therapeutic value, we found RHOA signaling to influence the activity of specific widely used chemotherapeutics, and its possible antagonism by various dietary constituents. We also review currently available targeted therapies for GC. The latter, however, showed a paucity of such agents, underscoring the urgent need for further investigation into treatments for this highly lethal malignancy.
Introduction
The RHO GTPase enzyme family, including RHOA, Rac, and cdc42, is essential for diverse biological processes, including cell morphology phenotypes, cell polarity, and cell migration, in diverse cancer types (Etienne-Manneville and Hall, 2002; Hanna and El-Sibai, 2013; Lin and Zheng, 2015; Haga and Ridley, 2016; Woldu et al., 2018). Recently, it was shown that RHOA can be targeted by small molecule inhibitors, in cancer, implicating it as a potential druggable target (Shang et al., 2012; Chang et al., 2016a). Due to a scarcity of RHOA reviews in the field of gastric cancer (GC), our focus herein is limited to RHOA, and its related GTPase family members (Etienne-Manneville and Hall, 2002; Heasman and Ridley, 2008; Hanna and El-Sibai, 2013; Haga and Ridley, 2016; Woldu et al., 2018) in GC.
Structural domains of RHO GTPase family members include a downstream effector protein-binding, and a GTP-/GDP-binding, domain (Hanna and El-Sibai, 2013). RHOA, like other RHO GTPases, is regulated by guanine nucleotide-exchange factors (GEFs), GTPase-activating proteins (GAPs), and guanine nucleotide-dissociation inhibitors (GDIs) (Hanna and El-Sibai, 2013). RHOA activation, by GEFs, facilitates its binding to GTP, as well as its release of GDP (Heasman and Ridley, 2008). Activated RHOA then recruits downstream effector proteins, including ROCK, LIMK, MLC, cofilin, PKN1, MYPT-1, and mammalian homolog of diaphanous (mDia) (Schwartz, 2004; Hanna and El-Sibai, 2013; Prudnikova et al., 2015), and it is involved in actin reorganization, cell motility, and cell migration (Hanna and El-Sibai, 2013; Prudnikova et al., 2015) (Figure 1). As opposed to GEFs, GAPs inactivate various RHO-GTPase forms (e.g., RHOA-GDP) (Hanna and El-Sibai, 2013). Finally, GDIs interact with RHOA-GDP complexes to sequester RHOA from membranes, thus suppressing their activation (Dovas and Couchman, 2005; Knezevic et al., 2007).
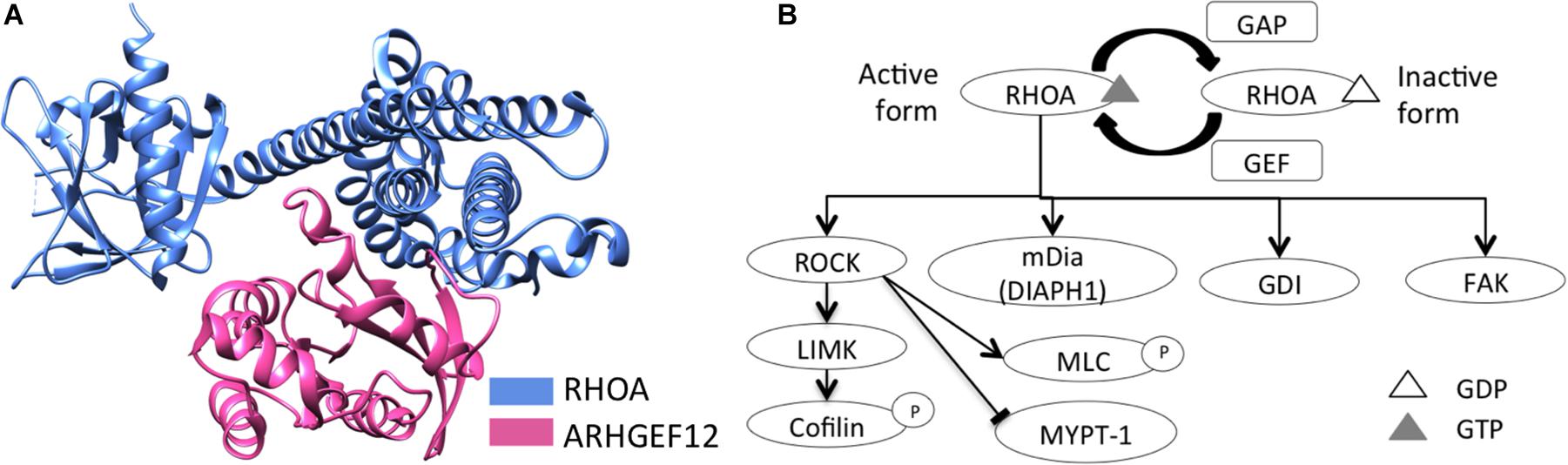
Figure 1. RHOA schematic structure and its downstream signaling. (A) Schematic structure of RHOA and a GEF protein, LARG (ARHGEF12), were represented (RCSB PDB ID: 1X86). (B) RHOA downstream signaling was represented, regarding actin reorganization, cell motility, and cell migration.
Recently, roles for RHOA in cell motility have been identified, in many diverse types of cancer (Prudnikova et al., 2015), but RHOA’s clinical significance (including therapeutic feasibility), to GC, one of the most lethal cancers in East Asia (Jemal et al., 2011), remains little known. Herein, the functional roles of RHOA, and its clinical relevance to GC, are systematically reviewed throughout the literature. Specifically, we find that RHOA is a strong potential druggable target, as well as a biomarker candidate, for GC, which currently lacks effective targeted therapies.
RHOA Functions and Its Biological Behavior in GC
RHOA Functions in Second-Generation Cancer Hallmarks
First, we explored literature regarding RHOA, in gastric cancer, in PubMed, using the search terms, “RHOA,” “expression,” and “cancer,” as of March 6, 2018, resulting in 1,536 articles. Subsequently, we manually inspected the presence of term “gastric” in the article titles to secure RHOA’s relevance to GC, resulting in 63 publications. Then, we co-searched the term “RHOA” with the terms in the ten 2nd-generation hallmark phrases (Hanahan and Weinberg, 2011) in abstracts and main texts of the 63 publications. We extracted 47 most relevant articles, regarding hallmarks of the cancer. These 47 were the basis for this section (Supplementary Figure S1). Along with the 47, manually selected publications were also reviewed in this section.
Recently, a second generation of cancer hallmarks was proposed (Hanahan and Weinberg, 2011), including: (1) activation of invasion and metastasis; (2) resistance to cell death; (3) sustainment of proliferative signaling; (4) evasion of growth suppressors; (5) dysregulation of cellular energetics; (6) replicative immortality; (7) angiogenesis; (8) genome instability and mutation; (9) tumor-promoting inflammation; and (10) avoidance of immune destruction. Using these, we assigned RHOA functions, from the 47 identified publications, according to relevance to the 10 cancer hallmarks. These 47 were then aligned to the three hallmark terms: activation of invasion and metastasis (term #1, above); resistance to cell death (term #2); and sustainment of proliferative signaling (term #3) (Supplementary Figure S2). The other cancer hallmark terms were not revealed in these 47 publications, but we strongly believe that RHOA signaling associates with all 10.
As shown in Supplementary Figure S2, most of the publications were assigned to term #1, “activation of invasion and metastasis.” In GC, this hallmark term associates with RHOA, in signal pathways (Chiou et al., 2001; Murray et al., 2008; Bennett et al., 2011; Zhou et al., 2011; Gomes et al., 2013). During GC cancer cell migration, a sialylated glycan antigen, Sialyl-Lewis X (SLex, synthesized by ST3GAL4), is often expressed on cell surfaces (Gomes et al., 2013). Through ST3GAL4 expression, leading to SLex biosynthesis, activation of RHOA, as well as c-Met, associates with SLex-induced GC invasion phenotypes (Gomes et al., 2013). In recent studies (Murray et al., 2008; Bennett et al., 2011), lysophosphatidic acid (LPA), through Neuroepithelial Cell Transforming 1 (NET1, a protein upregulated in GC tissues) (Leyden et al., 2006), activates RHOA, leading to cell invasion and migration. We especially noted that NET1 mRNA expression was upregulated in gastric epithelia infected by Helicobacter pylori (H. pylori), the primary risk factor for gastric carcinogenesis (Chiou et al., 2001).
One proposed mechanism of tumorigenesis, via H. pylori infection of gastric epithelial cells, with regard to cell migration, is that CagA (cytotoxin-associated gene A product), secreted during H. pylori activation of RHOA, through SHP-2 (encoded by PTPN11), actuates a Raf/Mek/ERK signaling cascade (Hagymasi and Tulassay, 2014). Moreover, we found RHOA to be involved in LPA-induced transcription of the metastasis-associated urokinase-type plasminogen activator receptor (uPAR, encoded by PLAUR), resulting in GC cell invasion, through an unknown mechanism (Kim et al., 2008). In another study, LPA-induced RHOA activity was suppressed by cross-linked hyaluronic acid gel, in a GC cell line (AGS), thereby inhibiting GC cell migration (Lan et al., 2016). While we could find no literature regarding germline RHOA mutations, a somatic mutation, RHOA-G17V, has been reported to positively associate with peripheral T-cell lymphoma chemoresponse (Manso et al., 2014).
Activating invasion by RHOA in GC is also mediated by CXCL12, a ligand for CXCR4, leading to activation of RHOA, Rac, and Cdc42 through mTOR signaling (Chen et al., 2012). In fact, rapamycin, an inhibitor of mTOR signaling, suppressed GC cell migration induced by CXCL12, indicating mTOR signaling as a possible therapeutic target in GC (Chen et al., 2012). Moreover, GC cell motility was induced by the C5a receptor (CD88), in association with activated RHOA (Kaida et al., 2016), while more recently, RHOA’s role, in activating invasion, was revealed to be epigenetically regulated by the non-coding RNA, miR-31, potentially targeting RHOA, in MKN-45 GC cells (Korourian et al., 2017b).
Growth factors can also induce GC cell invasion and migration. For example, TGFβ1 signaling activated RHOA, leading to scirrhous GC cell migration, via the epithelial-to-mesenchymal transition (EMT) (Shinto et al., 2010). More specifically, in scirrhous GC cell lines, RHOA activity was successfully repressed by an antagonist of the TGFβ receptor type I (encoded by TGFBR1), a mediator of TGFβ1 signaling (Shinto et al., 2010). Additionally, RHOA activity was reported as promoted by the maternal embryonic leucine zipper kinase (gene symbol, MELK), in GC cell migration (Du et al., 2014).
Dietary constituents can also regulate GC cell migration and invasion, through RHOA suppression (Ho et al., 2011). For example, benzyl isothiocyanate (BITC), an isothiocyanate found in mustards, repressed both RHOA and FAK mRNAs, inhibiting migration of AGS GC cells (Ho et al., 2011). RHOA also closely aligned with ROCK, which regulated invasion of OCUM-2MD3, a scirrhous GC cell line (Matsuoka et al., 2011).
Another dietary constituent, of watercress, phenethyl isothiocyanate (PEITC), downregulated AGS GC cell migration, through RHOA activity inhibition, leading to suppression of the metastasis-promoting urokinase-type plasminogen activator (UPA), cyclooxygenase-2 (COX-2), inducible nitric oxide synthase (iNOS), and NF-κB (Yang et al., 2010). A constituent of numerous plants, gallic acid, also suppressed RHOA activity, and that of the GTPases Cdc42, and Rac1, leading to inhibition of AGS GC cell migration (Ho et al., 2010). The flavonoid nobiletin, isolated from citrus fruit peels, was similarly reported to inhibit FAK/Ras enzymatic activity, downregulating RHOA/Cdc42/Rac1 protein expression, to subsequently inhibit AGS GC cell migration (Lee et al., 2011). We note that the study of dietary agents associated with reduced cancer risk, by identifying their potentially antineoplastic constituents in treatment of cultured cancer cells, is an essential first preclinical step (Yang et al., 2016). However, this must be then translated to animals, disease models, etc., prior to any remote possibility of use in humans (Cherng et al., 2007).
Epigenetically, GC cell invasion was suppressed by the non-coding RNA, miR-647, through a RHOA-mediated SRF/MYH9 axis (Ye et al., 2017), while miR-29, in association with chemotherapy, inhibited GC cell invasion and migration, in vitro and in vivo (Wang et al., 2015).
The cancer hallmark term, “resistance to cell death” (#2 above), also highly associated with RHOA. While a role for RHOA in apoptosis remains unresolved in GC (Cai et al., 2008), evidence does exist for apoptotic effects of RHOA/Rock signal pathway inhibition, in GC (Cai et al., 2008; Xu et al., 2012). One recent report showed that RHOA activation, in association with cell detachment-induced apoptosis (i.e., anoikis, cell death due to loss of cell-extracellular matrix contacts), resulted in enhanced assembly of actin filaments and focal adhesions (Cai et al., 2008). Also, resistance to chemotherapy-induced apoptosis (Kaufmann and Earnshaw, 2000), in GC cells, was reported to be mediated by RHOA activation (Kang et al., 2005). Activation of RHOA and NF-κB, by H. pylori infection, induced plasminogen activator inhibitor-2 (PAI-2; SERPINB2), leading to inhibition of apoptosis in gastric epithelial cells (Varro et al., 2004).
The cancer hallmark term, “sustainment of proliferative signaling” (hallmark #3 above), has yet to be clearly linked to GC, with specific regard to RHOA (Ghosh et al., 1999). However, a few studies have implicated RHOA as playing roles in GC cell proliferation. For example, one study showed that RHOA inhibition suppressed GC cell growth, albeit with lack of a proposed molecular mechanism (Liu et al., 2004). Also, when RHOA was inhibited in the GC cells, via siRNA, G1/S progression was slowed, through upregulation of the INK4 family cell cycle inhibitors, p15INK4b (CDKN2B), p16INK4a (CDKN2A), p18INK4c (CDKN2C), and p19INK4d (CDKN2B). These events were postulated as mediated by RHOA/Rock pathway inhibition (Zhang et al., 2009), and resulted in inhibition of CDK4 and CDK6 activity. Also, p21CIP1 (CDKN1A) and p27KIP1 (CDKN1B), cell cycle inhibitors of CDK2, were upregulated through a RHOA/mDia pathway, during RHOA suppression (Zhang et al., 2009). However, the detailed mechanisms of this phenomenon remain unknown.
The tumor microenvironment (e.g., stromal cells, cancer-associated fibroblasts, etc.) also plays an important role in multiple cancer hallmarks (Hanahan and Weinberg, 2011). In tumor microenvironment, tumor stroma interacting with cancer cells support tumor growth and progression, and include heterogeneous cell types (fibroblasts, myofibroblasts, endothelial cells, macrophages, diverse immune cells, and extracellular matrix (ECM)) (Tevis et al., 2017). Spheroids can mimic these multicellular nature and ECM, while monolayer system is too simplified to represent the interaction of a growing tumor and stroma (Tevis et al., 2017). Moreover, it is believed that distinct regions of the microenvironment comprise a cancer stem cell (CSC) “niche” (Plaks et al., 2015). Also, tumor-derived spheroids are used to purpose for the enrichment of CSCs or stem-like cells (Ishiguro et al., 2017). In one CSC assay, spheroid formation (Zhao et al., 2015), RHOA was hyperactivated in spheroid GC cells, compared to monolayer GC cell colonies of diffuse type GC cells (Yoon et al., 2016). Another stemness phenotype, drug expulsion by the membrane transporter P-glycoprotein, was also found to be attenuated by RHOA pharmacological inhibition (Pinzon-Daza et al., 2014). These findings may implicate RHOA signaling in the promotion of CSC phenotypes (Yoon et al., 2016).
Aberrant post-transcriptional events may also contribute to regulation of RHOA signaling. For example, recently, a contradictory role for RHOA, in two Lauren diffuse type GC cell lines (HSC-59, GSU) of 17 GC cell lines (12 for diffuse type and five for intestinal type), was suggested, in that low RHOA protein expression, due to aberrant alternative splicing of RHOA transcripts, was found in the two GC cell lines (HSC-59, GSU) (Miyamoto et al., 2018).
GC cell lines ranges in diverse histology, Lauren classification, RHOA mutation statuses, and RHOA protein expression (Supplementary Table S1). Thus, different GC cell line characteristics may impact on RHOA function, and GC cell line studies above need to be carefully interpreted.
The molecular mechanisms in this section are summarized in Figure 2. Overall, the majority of RHOA functions, in invasion, primarily encompass cancer hallmark #2 (resistance to cell death). Other hallmarks should be investigated in future.
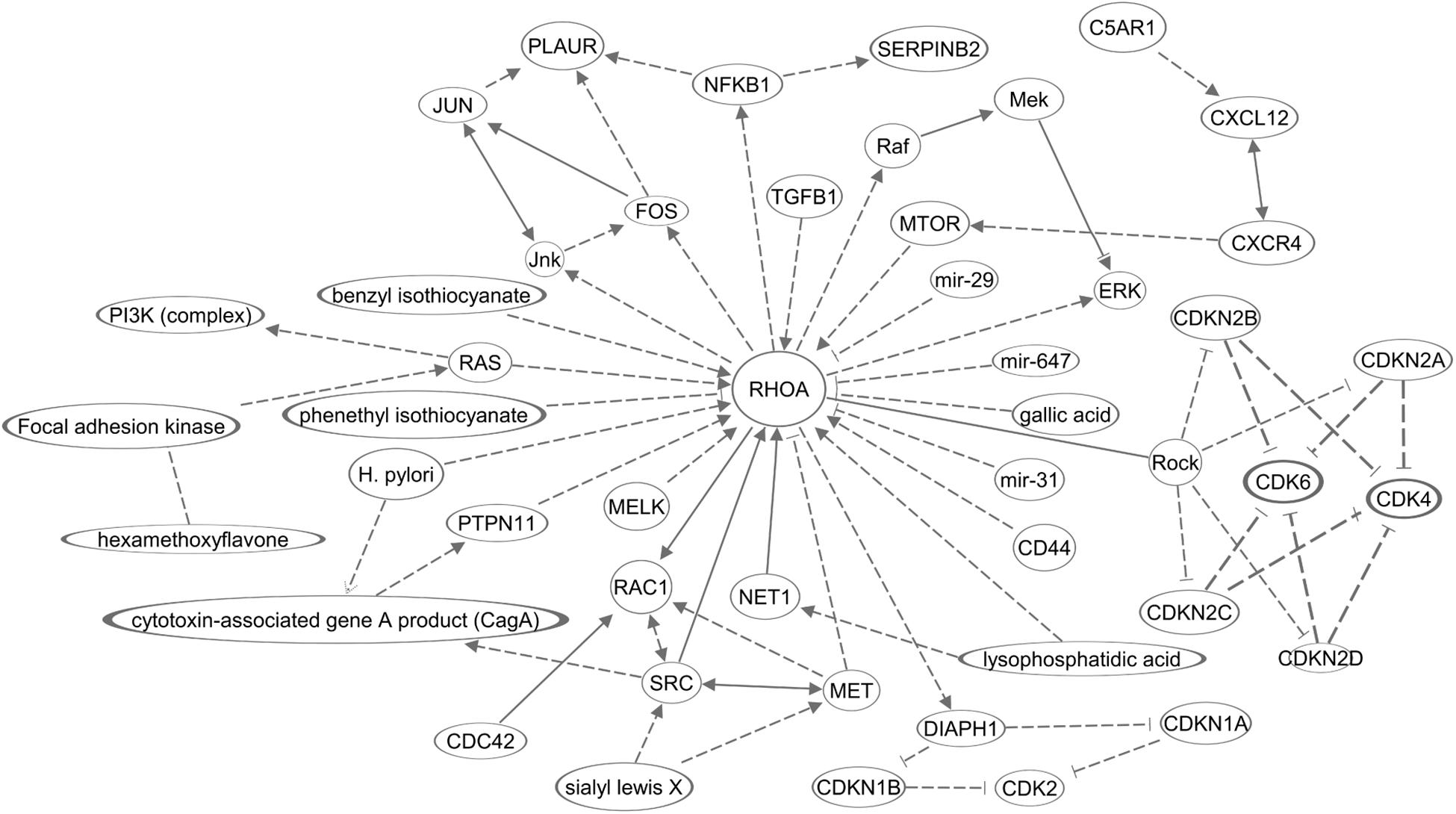
Figure 2. A diagram of molecular networks of RHOA in GC. In GC, regulation of RHOA described in this review was represented in the networks. Solid lines represents established regulations, and dashed lines the regulations described in this review for GC.
Biomarker and Clinical Relevance of RHOA, and Its Molecular Regulators
RHOA mRNA and its protein expression have demonstrated clinical relevance, including overall survival and GC tumor stage, and in this section, we searched for RHOA clinicopathological associations out of the 63 publications in the previous section. This search revealed 10 reports (Liu et al., 2004; Pan et al., 2004; Huang et al., 2015; Chen et al., 2016; Yoon et al., 2016, 2017; Ge et al., 2017; Korourian et al., 2017a,b; Song et al., 2017) of assessment of RHOA immunohistochemical (IHC) staining and its mRNA expression (Supplementary Figure S1). In addition, manually collected studies were reviewed in this section.
For example, Huang et al. (2015) reported that the Lauren classification, diffuse subtype, significantly associated with RHOA and specific clinicopathological characteristics, while the GC Lauren classification, intestinal subtype, did not. Moreover, IHC staining of diffuse subtype GC tumors, for RHOA, associated with advanced pathological N (nodal ingress) stages and poor prognosis (i.e., disease-free and overall survival), after surgery (Huang et al., 2015; Yoon et al., 2016). In another small sample size study (Yoon et al., 2017), advanced T and TMN stages showed higher RHOA mRNA and its protein expression, compared to early T and TMN stages. However, these results were inconsistent with those of Korourian et al. (2017b), who reported no statistically significant difference between RHOA IHC staining and TNM stage. The inconsistency may come from clinicopathological characteristics differences (including ethnicity) and different IHC grading schemes.
Liu et al. (2004) profiled RHOA IHC staining across the sequence of GC tumor development, i.e., normal mucosa, intestinal metaplasia, dysplasia, and invasion (Liu et al., 2004). Those results showed significantly higher RHOA expression in full-fledged tumors, compared to normal mucosa, intestinal metaplasia, and dysplasia, indicating progressively increasing RHOA expression during GC development. In another study, RHOA protein expression, in a small cohort (n = 53), showed positive association with poor GC differentiation (Pan et al., 2004). That study also confirmed statistically significant upregulation of RHOA mRNA expression, in malignant GC tissues, compared to adjacent normal (Pan et al., 2004). These studies correlating poor GC differentiation status with upregulated RHOA, as determined by IHC, are consistent with two other studies (Korourian et al., 2017a,b) reporting RHOA expression to be higher in Lauren classification subtype diffuse tumors, compared to the Lauren classification intestinal subtype, with the former also positively associating with vascular invasion.
RHOA is also a target of miR-31, which interestingly, showed significant clinical relevance to GC (Chen et al., 2016). In that regard, RHOA IHC staining was significantly higher in tumor tissues than in adjacent normal tissues, in negative correlation with miR-31 expression (Chen et al., 2016). Another recent study (Ge et al., 2017) showed possible clinical relevance of miR-31, in that its low expression associated with advanced pathologic N stages, and positive lymphatic vessel invasion status (Ge et al., 2017). That work also indicated that such a miR-31/RHOA axis could represent a clinical marker, and possible therapeutic target, in GC.
Our recent GC study (Chang et al., 2016a) also revealed that gene expression of RHOA was higher in stage I GC tissue samples than in adjacent normal tissues, revealing a clinical association with early stage GC. This finding was further confirmed in an independent RHOA IHC study showing that early GC (stages T1a and T1b) tissues exhibited higher RHOA protein expression, in comparison to adjacent tissues (Song et al., 2017).
RHOA also associated with signaling by the stemness-related pathway WNT (Chang et al., 2016a), as determined by a network generation algorithm, PATHOME (Nam et al., 2014), with both pathways sharing downstream genes/proteins (i.e., “crosstalking”). It has also been shown that the Wnt pathway β-catenin degenerative complex, when inhibited by Dishevelled, allows Rho/Rac to facilitate β-catenin translocation to the nucleus (Schlessinger et al., 2009). Analogously, Wnt5a (Liu et al., 2013; Kim et al., 2017) and Wnt3a (Kim et al., 2017) were positively correlated with RHOA activation, in association with GSK3-β phosphorylation. In the brain, RHOA inhibition enhanced GSK3-induced phosphorylation and degradation of β-catenin, while also inhibiting membrane efflux by P-glycoprotein, a “stemness” phenotype (Pinzon-Daza et al., 2014). Another recent finding showed GC clinical relevance of WNT signaling (WNT5A), in terms of lymph node metastasis, Lauren classification subtype diffuse, and advanced UICC stage (Nam et al., 2017). However, clinical application of RHOA and WNT signaling (Nam et al., 2014, 2017; Chang et al., 2016a; Kim et al., 2019) yet requires functional validation, prior to assessment of therapeutic feasibility.
Currently, next-generation sequencing (NGS) data for GC patients is available in the Cancer Genome Atlas (TCGA) (Cancer Genome Atlas Research Network, 2014). We inspected RHOA mutations, copy number variations, and gene expression the TCGA stomach cancer dataset (258 patients) (Cancer Genome Atlas Research Network, 2014) by using cBio Portal (Gao et al., 2013). In Supplementary Figure S3, we also displayed CpG island methylator phenotype (CIMP) categories, Epstein–Barr virus (EBV) presence, microsatellite instable (MSI) status and Lauren classification along with RHOA genetic alterations. Somatic mutation frequency was 5.4% (14/258), and copy number alteration (CNA) frequency was 2.3% (6/258). Two out of the 14 patients having RHOA mutations were EBV-present. The 6 patients having CNAs were deep deletion and EBV present. Also, five out of the 6 CNA patients belonged to Lauren classification intestinal type. The TCGA created the four molecular subtypes: EBV-positive tumors, MSI tumors, genomically stable (GS) tumors, and tumors with chromosomal instability (CIN) (Cancer Genome Atlas Research Network, 2014; Zhang, 2014). According to the TCGA, genetic events of RHOA and Rho-family GTPase-activating proteins (GAP) were the molecular subtype GS.
RHOA Therapeutic Implications in Gastric Cancer
GC Therapeutics and RHOA
To date, FDA-approved drugs directly targeting RHOA remain unapproved, according to the CIViC database (Griffith et al., 2017)1. However, GC RHOA signaling associated with clinical efficacy of various chemotherapeutics (Kang et al., 2005; Zhou et al., 2011; Wang et al., 2015; Yoon et al., 2016; Korourian et al., 2017b) (Table 1). Roles for RHOA in chemotherapeutic efficacy were also shown in Lauren diffuse GC cells (Yoon et al., 2016; Korourian et al., 2017b). Also, in Lauren intestinal GC cell lines (AGS, SNU-638), RHOA hyperactivity reduced chemosensitivity (Kang et al., 2005; Zhou et al., 2011).
RHOA signaling also associated with cisplatin and docetaxel therapeutic actions in GC, in addition to association with miR-29, a regulator of both catenin-δ (CTNND1) and RHOA, implicating non-coding RNA epigenetic effects of chemotherapeutic agents (Wang et al., 2015). Moreover, upregulation of miR-31 (a proposed regulator of RHOA (Mizoguchi et al., 2013), described above), enhanced chemosensitivity to 5-fluorouracil, in diffuse type GC MKN-45 cells (Korourian et al., 2017b).
Another study revealed that CD44+ CSCs associated with GC recurrence, following chemotherapy with 5-fluorouracil and cisplatin, in Lauren diffuse GC cells (Yoon et al., 2016). Further, combination of a potential RHOA signaling inhibitor, fasudil, with cisplatin, effectively suppressed numbers of CD44+ CSCs, in GC (Yoon et al., 2016). Although there is no postulated mechanism for CD44 association with RHOA activation, the Hippo-YAP signal pathway seems likely to link CD44 and RHOA, in other cell types (Zhang et al., 2014).
Regarding targeted therapies, the U.S. Food and Drug Administration (FDA) approved the HER2 antibody trastuzumab, combined with chemotherapy, for HER2+ GC patients (Bang et al., 2010). However, in diffuse type GC, HER2+ patients represent only 2–7% of the total, underscoring the crucial need for more subtype-specific therapeutics (Ushiku et al., 2016). Moreover, considering the clinical importance of RHOA in diffuse (and other) type GC (Nam et al., 2014; Chang et al., 2016a; Yoon et al., 2016; Korourian et al., 2017b; Nam et al., 2017), therapeutic development strategies remain largely undeveloped (Ushiku et al., 2016). Also recently, ramucirumab, a targeted therapy for metastatic GC, was approved by the FDA, although it is unclear whether it impacts RHOA signaling.
Small Molecule Inhibitors for Targeting RHOA Proteins in GC
One small molecule inhibitor, Rhosin (Shang et al., 2012), inhibits RHOA signaling by directly targeting the RHOA protein, in breast and hepatocellular cancer cells (Shang et al., 2012; Lin and Zheng, 2015; Olson, 2018), as well as GC cells (Yoon et al., 2016). In our recent study, we demonstrated a hydrazide derivative, JK-122, as a new small molecule inhibitor that binds the RHOA active site, as determined using surface plasmon resonance, and was also antimitogenic toward GC cell lines (Chang et al., 2016a).
Several chemical inhibitors of RHOA signaling do not directly target the RHOA protein, but affect RHOA signaling-related proteins (Lin and Zheng, 2015; Olson, 2018). For example, CCG-1423 (Evelyn et al., 2007) is a small molecule that suppresses RHOA signaling (Evelyn et al., 2007) by binding to the phosphatase and actin regulator RPEL, to inhibit RHOA/MKL/SRF signaling (Hayashi et al., 2014). While unsuitable for translational development, these compounds provide proof-of-concept for experimental investigation of RHOA (and its other family members’) signal inhibition (Olson, 2018), as the identification of therapeutic small molecule inhibitors, that selectively bind RHOA, remain urgently needed.
Conclusion
Here, we reviewed literature of how RHOA’s roles, in gastric cancer (GC), associate with the second-generation cancer hallmark term, “activation of invasion and metastasis” (Kim et al., 2008; Chen et al., 2012; Du et al., 2014). Two other cancer hallmarks (“resistance to cell death” and “sustainment of proliferative signaling”) also were linked to studies of GC. Cancer stem-like cells, well known inhabitants of the tumor microenvironment, have been also implicated in GC (Yoon et al., 2016). Thus, molecular and biochemical studies of RHOA, as related to other cancer hallmarks, need to be performed in the future.
RHOA expression in GC, as reviewed in the literature, shows clinical association with Lauren diffuse subtype GC, and may have potential prognostic value (Nam et al., 2014, 2017; Chang et al., 2016a; Yoon et al., 2016; Korourian et al., 2017b). However, in terms of GC therapeutic options, small chemical inhibitors that directly bind RHOA, have yet to translated to GC patients. However, our recent study (Chang et al., 2016a) showed that RHOA activity could be regulated by specific small chemicals that bind the protein, indicating RHOA to be a druggable target in GC. For facilitating therapeutic discovery in GC, GC in vitro models should be continuously developed (Chang et al., 2016b). In summary, although existing evidence demonstrates the feasibility of employment of RHOA as both a biomarker candidate and druggable target, further investigation of its application to GC (and other cancer) therapy, is urgently needed.
Author Contributions
SN supervised the study and drafted the manuscript. All authors procured and reviewed publications suitable for this review article, and read and approved the manuscript.
Funding
This research was supported by Basic Science Research Program, through the National Research Foundation of Korea (NRF), funded by the Ministry of Education (NRF-2016R1D1A1B03933145 to SN).
Conflict of Interest Statement
The authors declare that the research was conducted in the absence of any commercial or financial relationships that could be construed as a potential conflict of interest.
Supplementary Material
The Supplementary Material for this article can be found online at: https://www.frontiersin.org/articles/10.3389/fgene.2019.00438/full#supplementary-material
Footnote
References
Bang, Y. J., Van Cutsem, E., Feyereislova, A., Chung, H. C., Shen, L., Sawaki, A., et al. (2010). Trastuzumab in combination with chemotherapy versus chemotherapy alone for treatment of HER2-positive advanced gastric or gastro-oesophageal junction cancer (ToGA): a phase 3, open-label, randomised controlled trial. Lancet 376, 687–697. doi: 10.1016/S0140-6736(10)61121-X
Bennett, G., Sadlier, D., Doran, P. P., Macmathuna, P., and Murray, D. W. (2011). A functional and transcriptomic analysis of NET1 bioactivity in gastric cancer. BMC Cancer 11:50. doi: 10.1186/1471-2407-11-50
Cai, J., Niu, X., Chen, Y., Hu, Q., Shi, G., Wu, H., et al. (2008). Emodin-induced generation of reactive oxygen species inhibits RhoA activation to sensitize gastric carcinoma cells to anoikis. Neoplasia 10, 41–51.
Cancer Genome Atlas Research Network (2014). Comprehensive molecular characterization of gastric adenocarcinoma. Nature 513, 202–209. doi: 10.1038/nature13480
Chang, H. R., Nam, S., Lee, J., Kim, J. H., Jung, H. R., Park, H. S., et al. (2016a). Systematic approach identifies RHOA as a potential biomarker therapeutic target for Asian gastric cancer. Oncotarget 7, 81435–81451. doi: 10.18632/oncotarget.12963
Chang, H. R., Park, H. S., Ahn, Y. Z., Nam, S., Jung, H. R., Park, S., et al. (2016b). Improving gastric cancer preclinical studies using diverse in vitro and in vivo model systems. BMC Cancer 16:200. doi: 10.1186/s12885-016-2232-2
Chen, G., Chen, S. M., Wang, X., Ding, X. F., Ding, J., and Meng, L. H. (2012). Inhibition of chemokine (CXC motif) ligand 12/chemokine (CXC motif) receptor 4 axis (CXCL12/CXCR4)-mediated cell migration by targeting mammalian target of rapamycin (mTOR) pathway in human gastric carcinoma cells. J. Biol. Chem. 287, 12132–12141. doi: 10.1074/jbc.M111.302299
Chen, Z., Liu, S., Xia, Y., and Wu, K. (2016). MiR-31 regulates rho-associated kinase-myosin light chain (ROCK-MLC) pathway and inhibits gastric cancer invasion: roles of RhoA. Med. Sci. Monit. 22, 4679–4691. doi: 10.12659/msm.898399
Cherng, J. M., Shieh, D. E., Chiang, W., Chang, M. Y., and Chiang, L. C. (2007). Chemopreventive effects of minor dietary constituents in common foods on human cancer cells. Biosci. Biotechnol. Biochem. 71, 1500–1504. doi: 10.1271/bbb.70008
Chiou, C. C., Chan, C. C., Sheu, D. L., Chen, K. T., Li, Y. S., and Chan, E. C. (2001). Helicobacter pylori infection induced alteration of gene expression in human gastric cells. Gut 48, 598–604. doi: 10.1136/gut.48.5.598
Dovas, A., and Couchman, J. R. (2005). RhoGDI: multiple functions in the regulation of Rho family GTPase activities. Biochem. J. 390(Pt 1), 1–9. doi: 10.1042/BJ20050104
Du, T., Qu, Y., Li, J., Li, H., Su, L., Zhou, Q., et al. (2014). Maternal embryonic leucine zipper kinase enhances gastric cancer progression via the FAK/Paxillin pathway. Mol. Cancer 13:100. doi: 10.1186/1476-4598-13-100
Etienne-Manneville, S., and Hall, A. (2002). Rho GTPases in cell biology. Nature 420, 629–635. doi: 10.1038/nature01148
Evelyn, C. R., Wade, S. M., Wang, Q., Wu, M., Iniguez-Lluhi, J. A., Merajver, S. D., et al. (2007). CCG-1423: a small-molecule inhibitor of RhoA transcriptional signaling. Mol. Cancer Ther. 6, 2249–2260. doi: 10.1158/1535-7163.MCT-06-0782
Gao, J., Aksoy, B. A., Dogrusoz, U., Dresdner, G., Gross, B., Sumer, S. O., et al. (2013). Integrative analysis of complex cancer genomics and clinical profiles using the cBioPortal. Sci. Signal. 6:pl1. doi: 10.1126/scisignal.2004088
Ge, F., Wang, C., Wang, W., Liu, W., and Wu, B. (2017). MicroRNA-31 inhibits tumor invasion and metastasis by targeting RhoA in human gastric cancer. Oncol. Rep. 38, 1133–1139. doi: 10.3892/or.2017.5758
Ghosh, P. M., Ghosh-Choudhury, N., Moyer, M. L., Mott, G. E., Thomas, C. A., Foster, B. A., et al. (1999). Role of RhoA activation in the growth and morphology of a murine prostate tumor cell line. Oncogene 18, 4120–4130. doi: 10.1038/sj.onc.1202792
Gomes, C., Osorio, H., Pinto, M. T., Campos, D., Oliveira, M. J., and Reis, C. A. (2013). Expression of ST3GAL4 leads to SLe(x) expression and induces c-Met activation and an invasive phenotype in gastric carcinoma cells. PLoS One 8:e66737. doi: 10.1371/journal.pone.0066737
Griffith, M., Spies, N. C., Krysiak, K., McMichael, J. F., Coffman, A. C., Danos, A. M., et al. (2017). CIViC is a community knowledgebase for expert crowdsourcing the clinical interpretation of variants in cancer. Nat. Genet. 49, 170–174. doi: 10.1038/ng.3774
Haga, R. B., and Ridley, A. J. (2016). Rho GTPases: regulation and roles in cancer cell biology. Small GTPases 7, 207–221. doi: 10.1080/21541248.2016.1232583
Hagymasi, K., and Tulassay, Z. (2014). Helicobacter pylori infection: new pathogenetic and clinical aspects. World J. Gastroenterol. 20, 6386–6399. doi: 10.3748/wjg.v20.i21.6386
Hanahan, D., and Weinberg, R. A. (2011). Hallmarks of cancer: the next generation. Cell 144, 646–674. doi: 10.1016/j.cell.2011.02.013
Hanna, S., and El-Sibai, M. (2013). Signaling networks of Rho GTPases in cell motility. Cell Signal. 25, 1955–1961. doi: 10.1016/j.cellsig.2013.04.009
Hayashi, K., Watanabe, B., Nakagawa, Y., Minami, S., and Morita, T. (2014). RPEL proteins are the molecular targets for CCG-1423, an inhibitor of Rho signaling. PLoS One 9:e89016. doi: 10.1371/journal.pone.0089016
Heasman, S. J., and Ridley, A. J. (2008). Mammalian Rho GTPases: new insights into their functions from in vivo studies. Nat. Rev. Mol. Cell Biol. 9, 690–701. doi: 10.1038/nrm2476
Ho, C. C., Lai, K. C., Hsu, S. C., Kuo, C. L., Ma, C. Y., Lin, M. L., et al. (2011). Benzyl isothiocyanate (BITC) inhibits migration and invasion of human gastric cancer AGS cells via suppressing ERK signal pathways. Hum. Exp. Toxicol. 30, 296–306. doi: 10.1177/0960327110371991
Ho, H. H., Chang, C. S., Ho, W. C., Liao, S. Y., Wu, C. H., and Wang, C. J. (2010). Anti-metastasis effects of gallic acid on gastric cancer cells involves inhibition of NF-kappaB activity and downregulation of PI3K/AKT/small GTPase signals. Food Chem. Toxicol. 48, 2508–2516. doi: 10.1016/j.fct.2010.06.024
Huang, K. H., Lan, Y. T., Chen, M. H., Chao, Y., Lo, S. S., Li, A. F., et al. (2015). The correlation between RhoA expression and clinicopathological characteristics in gastric cancer patients after curative surgery. World J. Surg. 39, 2289–2299. doi: 10.1007/s00268-015-3095-4
Ishiguro, T., Ohata, H., Sato, A., Yamawaki, K., Enomoto, T., and Okamoto, K. (2017). Tumor-derived spheroids: relevance to cancer stem cells and clinical applications. Cancer Sci. 108, 283–289. doi: 10.1111/cas.13155
Jemal, A., Bray, F., Center, M. M., Ferlay, J., Ward, E., and Forman, D. (2011). Global cancer statistics. CA Cancer J. Clin. 61, 69–90. doi: 10.3322/caac.20107
Kaida, T., Nitta, H., Kitano, Y., Yamamura, K., Arima, K., Izumi, D., et al. (2016). C5a receptor (CD88) promotes motility and invasiveness of gastric cancer by activating RhoA. Oncotarget 7, 84798–84809. doi: 10.18632/oncotarget.12656
Kang, W. K., Lee, I., and Park, C. (2005). Characterization of RhoA-mediated chemoresistance in gastric cancer cells. Cancer Res. Treat. 37, 251–256. doi: 10.4143/crt.2005.37.4.251
Kaufmann, S. H., and Earnshaw, W. C. (2000). Induction of apoptosis by cancer chemotherapy. Exp. Cell. Res. 256, 42–49. doi: 10.1006/excr.2000.4838
Kim, J. G., Kim, M. J., Choi, W. J., Moon, M. Y., Kim, H. J., Lee, J. Y., et al. (2017). Wnt3A induces GSK-3beta phosphorylation and beta-catenin accumulation through RhoA/ROCK. J. Cell. Physiol. 232, 1104–1113. doi: 10.1002/jcp.25572
Kim, J. H., Eom, H. J., Lim, G., Park, S., Lee, J., Nam, S., et al. (2019). Differential effects, on oncogenic pathway signalling, by derivatives of the HNF4 alpha inhibitor BI6015. Br. J. Cancer 120, 488–498. doi: 10.1038/s41416-018-0374-5
Kim, M. H., Park, J. S., Chang, H. J., Baek, M. K., Kim, H. R., Shin, B. A., et al. (2008). Lysophosphatidic acid promotes cell invasion by up-regulating the urokinase-type plasminogen activator receptor in human gastric cancer cells. J. Cell. Biochem. 104, 1102–1112. doi: 10.1002/jcb.21696
Knezevic, N., Roy, A., Timblin, B., Konstantoulaki, M., Sharma, T., Malik, A. B., et al. (2007). GDI-1 phosphorylation switch at serine 96 induces RhoA activation and increased endothelial permeability. Mol. Cell. Biol. 27, 6323–6333. doi: 10.1128/MCB.00523-07
Korourian, A., Roudi, R., Shariftabrizi, A., Kalantari, E., Sotoodeh, K., and Madjd, Z. (2017a). Differential role of Wnt signaling and base excision repair pathways in gastric adenocarcinoma aggressiveness. Clin. Exp. Med. 17, 505–517. doi: 10.1007/s10238-016-0443-0
Korourian, A., Roudi, R., Shariftabrizi, A., and Madjd, Z. (2017b). MicroRNA-31 inhibits RhoA-mediated tumor invasion and chemotherapy resistance in MKN-45 gastric adenocarcinoma cells. Exp. Biol. Med. 242, 1842–1847. doi: 10.1177/1535370217728460
Lan, T., Pang, J., Wu, Y., Zhu, M., Yao, X., Wu, M., et al. (2016). Cross-linked hyaluronic acid gel inhibits metastasis and growth of gastric and hepatic cancer cells: in vitro and in vivo studies. Oncotarget 7, 65418–65428. doi: 10.18632/oncotarget.11739
Lee, Y. C., Cheng, T. H., Lee, J. S., Chen, J. H., Liao, Y. C., Fong, Y., et al. (2011). Nobiletin, a citrus flavonoid, suppresses invasion and migration involving FAK/PI3K/Akt and small GTPase signals in human gastric adenocarcinoma AGS cells. Mol. Cell. Biochem. 347, 103–115. doi: 10.1007/s11010-010-0618-z
Leyden, J., Murray, D., Moss, A., Arumuguma, M., Doyle, E., McEntee, G., et al. (2006). Net1 and myeov: computationally identified mediators of gastric cancer. Br. J. Cancer 94, 1204–1212. doi: 10.1038/sj.bjc.6603054
Lin, Y., and Zheng, Y. (2015). Approaches of targeting Rho GTPases in cancer drug discovery. Expert. Opin. Drug Discov. 10, 991–1010. doi: 10.1517/17460441.2015.1058775
Liu, J., Zhang, Y., Xu, R., Du, J., Hu, Z., Yang, L., et al. (2013). PI3K/Akt-dependent phosphorylation of GSK3beta and activation of RhoA regulate Wnt5a-induced gastric cancer cell migration. Cell Signal. 25, 447–456. doi: 10.1016/j.cellsig.2012.10.012
Liu, N., Bi, F., Pan, Y., Sun, L., Xue, Y., Shi, Y., et al. (2004). Reversal of the malignant phenotype of gastric cancer cells by inhibition of RhoA expression and activity. Clin. Cancer Res. 10(18 Pt 1), 6239–6247. doi: 10.1158/1078-0432.CCR-04-0242
Manso, R., Sanchez-Beato, M., Monsalvo, S., Gomez, S., Cereceda, L., Llamas, P., et al. (2014). The RHOA G17V gene mutation occurs frequently in peripheral T-cell lymphoma and is associated with a characteristic molecular signature. Blood 123, 2893–2894. doi: 10.1182/blood-2014-02-555946
Matsuoka, T., Yashiro, M., Kato, Y., Shinto, O., Kashiwagi, S., and Hirakawa, K. (2011). RhoA/ROCK signaling mediates plasticity of scirrhous gastric carcinoma motility. Clin. Exp. Metastasis 28, 627–636. doi: 10.1007/s10585-011-9396-6
Miyamoto, S., Nagamura, Y., Nakabo, A., Okabe, A., Yanagihara, K., Fukami, K., et al. (2018). Aberrant alternative splicing of RHOA is associated with loss of its expression and activity in diffuse-type gastric carcinoma cells. Biochem. Biophys. Res. Commun. 495, 1942–1947. doi: 10.1016/j.bbrc.2017.12.067
Mizoguchi, F., Murakami, Y., Saito, T., Miyasaka, N., and Kohsaka, H. (2013). miR-31 controls osteoclast formation and bone resorption by targeting RhoA. Arthritis Res. Ther. 15:R102. doi: 10.1186/ar4282
Murray, D., Horgan, G., Macmathuna, P., and Doran, P. (2008). NET1-mediated RhoA activation facilitates lysophosphatidic acid-induced cell migration and invasion in gastric cancer. Br. J. Cancer 99, 1322–1329. doi: 10.1038/sj.bjc.6604688
Nam, S., Chang, H. R., Kim, K. T., Kook, M. C., Hong, D., Kwon, C. H., et al. (2014). PATHOME: an algorithm for accurately detecting differentially expressed subpathways. Oncogene 33, 4941–4951. doi: 10.1038/onc.2014.80
Nam, S., Chung, J. W., and Yang, J. Y. (2017). WNT5A correlates with clinicopathological characteristics in gastric cancer: a meta-analysis. Cell Physiol. Biochem. 41, 33–40. doi: 10.1159/000455934
Olson, M. F. (2018). Rho GTPases, their post-translational modifications, disease-associated mutations and pharmacological inhibitors. Small GTPases 9, 203–215. doi: 10.1080/21541248.2016.1218407
Pan, Y., Bi, F., Liu, N., Xue, Y., Yao, X., Zheng, Y., et al. (2004). Expression of seven main Rho family members in gastric carcinoma. Biochem. Biophys. Res. Commun. 315, 686–691. doi: 10.1016/j.bbrc.2004.01.108
Pinzon-Daza, M. L., Salaroglio, I. C., Kopecka, J., Garzon, R., Couraud, P. O., Ghigo, D., et al. (2014). The cross-talk between canonical and non-canonical Wnt-dependent pathways regulates P-glycoprotein expression in human blood-brain barrier cells. J. Cereb. Blood Flow Metab. 34, 1258–1269. doi: 10.1038/jcbfm.2014.100
Plaks, V., Kong, N., and Werb, Z. (2015). The cancer stem cell niche: how essential is the niche in regulating stemness of tumor cells? Cell Stem Cell 16, 225–238. doi: 10.1016/j.stem.2015.02.015
Prudnikova, T. Y., Rawat, S. J., and Chernoff, J. (2015). Molecular pathways: targeting the kinase effectors of RHO-family GTPases. Clin. Cancer Res. 21, 24–29. doi: 10.1158/1078-0432.CCR-14-0827
Schlessinger, K., Hall, A., and Tolwinski, N. (2009). Wnt signaling pathways meet Rho GTPases. Genes Dev. 23, 265–277. doi: 10.1101/gad.1760809
Schwartz, M. (2004). Rho signalling at a glance. J. Cell. Sci. 117(Pt 23), 5457–5458. doi: 10.1242/jcs.01582
Shang, X., Marchioni, F., Sipes, N., Evelyn, C. R., Jerabek-Willemsen, M., Duhr, S., et al. (2012). Rational design of small molecule inhibitors targeting RhoA subfamily Rho GTPases. Chem. Biol. 19, 699–710. doi: 10.1016/j.chembiol.2012.05.009
Shinto, O., Yashiro, M., Kawajiri, H., Shimizu, K., Shimizu, T., Miwa, A., et al. (2010). Inhibitory effect of a TGFbeta receptor type-I inhibitor, Ki26894, on invasiveness of scirrhous gastric cancer cells. Br. J. Cancer 102, 844–851. doi: 10.1038/sj.bjc.6605561
Song, L., Guo, Y., and Xu, B. (2017). Expressions of ras homolog gene family, member A (RhoA) and cyclooxygenase-2 (COX-2) proteins in early gastric cancer and their role in the development of gastric cancer. Med. Sci. Monit. 23, 2979–2984. doi: 10.12659/msm.902367
Tevis, K. M., Cecchi, R. J., Colson, Y. L., and Grinstaff, M. W. (2017). Mimicking the tumor microenvironment to regulate macrophage phenotype and assessing chemotherapeutic efficacy in embedded cancer cell/macrophage spheroid models. Acta Biomater. 50, 271–279. doi: 10.1016/j.actbio.2016.12.037
Ushiku, T., Ishikawa, S., Kakiuchi, M., Tanaka, A., Katoh, H., Aburatani, H., et al. (2016). RHOA mutation in diffuse-type gastric cancer: a comparative clinicopathology analysis of 87 cases. Gastric Cancer 19, 403–411. doi: 10.1007/s10120-015-0493-0
Varro, A., Noble, P. J., Pritchard, D. M., Kennedy, S., Hart, C. A., Dimaline, R., et al. (2004). Helicobacter pylori induces plasminogen activator inhibitor 2 in gastric epithelial cells through nuclear factor-kappaB and RhoA: implications for invasion and apoptosis. Cancer Res. 64, 1695–1702. doi: 10.1158/0008-5472.can-03-2399
Wang, Y., Liu, C., Luo, M., Zhang, Z., Gong, J., Li, J., et al. (2015). Chemotherapy-Induced miRNA-29c/Catenin-delta signaling suppresses metastasis in gastric cancer. Cancer Res. 75, 1332–1344. doi: 10.1158/0008-5472.CAN-14-0787
Woldu, S. L., Hutchinson, R. C., Krabbe, L. M., Sanli, O., and Margulis, V. (2018). The Rho GTPase signalling pathway in urothelial carcinoma. Nat. Rev. Urol. 15, 83–91. doi: 10.1038/nrurol.2017.184
Xu, X. T., Song, Q. B., Yao, Y., Ruan, P., and Tao, Z. Z. (2012). Inhibition of RhoA/ROCK signaling pathway promotes the apoptosis of gastric cancer cells. Hepatogastroenterology 59, 2523–2526. doi: 10.5754/hge12147
Yang, C. S., Chen, J. X., Wang, H., and Lim, J. (2016). Lessons learned from cancer prevention studies with nutrients and non-nutritive dietary constituents. Mol. Nutr. Food Res. 60, 1239–1250. doi: 10.1002/mnfr.201500766
Yang, M. D., Lai, K. C., Lai, T. Y., Hsu, S. C., Kuo, C. L., Yu, C. S., et al. (2010). Phenethyl isothiocyanate inhibits migration and invasion of human gastric cancer AGS cells through suppressing MAPK and NF-kappaB signal pathways. Anticancer Res. 30, 2135–2143.
Ye, G., Huang, K., Yu, J., Zhao, L., Zhu, X., Yang, Q., et al. (2017). MicroRNA-647 targets SRF-MYH9 axis to suppress invasion and metastasis of gastric cancer. Theranostics 7, 3338–3353. doi: 10.7150/thno.20512
Yoon, C., Cho, S. J., Aksoy, B. A., Park, D. J., Schultz, N., Ryeom, S. W., et al. (2016). Chemotherapy resistance in diffuse-type gastric adenocarcinoma is mediated by RhoA activation in cancer stem-like cells. Clin. Cancer Res. 22, 971–983. doi: 10.1158/1078-0432.CCR-15-1356
Yoon, J. H., Choi, W. S., Kim, O., Choi, B. J., Nam, S. W., Lee, J. Y., et al. (2017). Gastrokine 1 inhibits gastric cancer cell migration and invasion by downregulating RhoA expression. Gastric Cancer 20, 274–285. doi: 10.1007/s10120-016-0617-1
Zhang, S., Tang, Q., Xu, F., Xue, Y., Zhen, Z., Deng, Y., et al. (2009). RhoA regulates G1-S progression of gastric cancer cells by modulation of multiple INK4 family tumor suppressors. Mol. Cancer Res. 7, 570–580. doi: 10.1158/1541-7786.MCR-08-0248
Zhang, W. (2014). TCGA divides gastric cancer into four molecular subtypes: implications for individualized therapeutics. Chin. J. Cancer 33, 469–470. doi: 10.5732/cjc.014.10117
Zhang, Y., Xia, H., Ge, X., Chen, Q., Yuan, D., Chen, Q., et al. (2014). CD44 acts through RhoA to regulate YAP signaling. Cell Signal. 26, 2504–2513. doi: 10.1016/j.cellsig.2014.07.031
Zhao, Y., Feng, F., and Zhou, Y. N. (2015). Stem cells in gastric cancer. World J. Gastroenterol. 21, 112–123. doi: 10.3748/wjg.v21.i1.112
Keywords: RHOA, gastric cancer, stomach cancer, therapeutics, functions
Citation: Nam S, Kim JH and Lee DH (2019) RHOA in Gastric Cancer: Functional Roles and Therapeutic Potential. Front. Genet. 10:438. doi: 10.3389/fgene.2019.00438
Received: 12 September 2018; Accepted: 29 April 2019;
Published: 15 May 2019.
Edited by:
Heather Cunliffe, University of Otago, New ZealandReviewed by:
Cristin Gregor Print, The University of Auckland, New ZealandRosa Marina Melillo, University of Naples Federico II, Italy
Copyright © 2019 Nam, Kim and Lee. This is an open-access article distributed under the terms of the Creative Commons Attribution License (CC BY). The use, distribution or reproduction in other forums is permitted, provided the original author(s) and the copyright owner(s) are credited and that the original publication in this journal is cited, in accordance with accepted academic practice. No use, distribution or reproduction is permitted which does not comply with these terms.
*Correspondence: Seungyoon Nam, bmFtc0BnYWhvbi5hYy5rcg==