- 1Splicing and Genetic Susceptibility to Cancer, Instituto de Biología y Genética Molecular (CSIC-UVa), Valladolid, Spain
- 2Instituto de Biología y Genética Molecular (CSIC-UVa), Valladolid, Spain
- 3VISAVET-Universidad Complutense de Madrid, Madrid, Spain
A relevant fraction of BRCA2 variants is associated with splicing alterations and with an increased risk of hereditary breast and ovarian cancer (HBOC). In this work, we have carried out a thorough study of variants from BRCA2 exons 14 and 15 reported at mutation databases. A total of 294 variants from exons 14 and 15 and flanking intronic sequences were analyzed with the online splicing tools NNSplice and Human Splicing Finder. Fifty-three out of these 294 variants were selected as candidate splicing variants. All variants but one, were introduced into the minigene MGBR2_ex14-20 (with exons 14–20) by site-directed mutagenesis and assayed in MCF-7 cells. Twelve of the remaining 52 variants (23.1%) impaired splicing at different degrees, yielding from 5 to 100% of aberrant transcripts. Nine variants affected the natural acceptor or donor sites of both exons and three affected putative enhancers or silencers. Fluorescent capillary electrophoresis revealed at least 10 different anomalous transcripts: (E14q5), Δ (E14p10), Δ(E14p246), Δ(E14q256), Δ(E14), Δ(E15p12), Δ(E15p13), Δ(E15p83), Δ(E15) and a 942-nt fragment of unknown structure. All transcripts, except for Δ(E14q256) and Δ(E15p12), are expected to truncate the BRCA2 protein. Nine variants induced severe splicing aberrations with more than 90% of abnormal transcripts. Thus, according to the guidelines of the American College of Medical Genetics and Genomics, eight variants should be classified as pathogenic (c.7008-2A > T, c.7008-1G > A, c.7435+1G > C, c.7436-2A > T, c.7436-2A > G, c.7617+1G > A, c.7617+1G > T, and c.7617+2T > G), one as likely pathogenic (c.7008-3C > G) and three remain as variants of uncertain clinical significance or VUS (c.7177A > G, c.7447A > G and c.7501C > T). In conclusion, functional assays by minigenes constitute a valuable strategy to primarily check the splicing impact of DNA variants and their clinical interpretation. While bioinformatics predictions of splice site variants were accurate, those of enhancer or silencer variants were poor (only 3/23 spliceogenic variants) which showed weak impacts on splicing (∼5–16% of aberrant isoforms). So, the Exonic Splicing Enhancer and Silencer (ESE and ESS, respectively) prediction algorithms require further improvement.
Introduction
Since the discovery of the breast cancer genes BRCA1 (OMIM #113705) and BRCA2 (OMIM #600185) (Miki et al., 1994; Wooster et al., 1995), nearly 17,000 different variants of both genes have been recorded at the ClinVar database1 (date last accessed; November 2018). Germline inactivating variants in BRCA1 and BRCA2 confer high lifetime risks of breast and ovarian cancers (Mavaddat et al., 2013). Also, other cancer types, such as prostate, pancreatic and melanoma, are associated with pathogenic variants in these genes (Petrucelli et al., 2013). Despite the high penetrance of BRCA pathogenic variants, they are responsible for only ∼15–20% of hereditary breast and ovarian cancer (HBOC) (Stratton and Rahman, 2008). In fact, HBOC is a highly genetically heterogeneous disease with about 25 known or proposed susceptibility genes (Nielsen et al., 2016). Apart from the BRCA genes, PALB2 (OMIM #610355), ATM (OMIM #607585), and CHEK2 (OMIM #604373) have a prominent contribution since, in a recent study, more than 30% of pathogenic variants were found in these genes (Buys et al., 2017).
Commonly, the variants are classified attending to their predicted effect on the protein so that truncating variants (frameshift and nonsense) are directly classified as pathogenic, while intronic, missense and synonymous variants are usually considered to be variants of uncertain clinical significance (VUS). In fact, VUS are identified by a relevant proportion of BRCA genetic tests (∼20%), which hamper genetic counseling and subsequent preventive or therapeutic actions, since risk assessment is solely based on family history (Radice et al., 2011; Eccles et al., 2015; Ricks et al., 2015).
Furthermore, other upstream gene-expression processes, such as transcription or splicing, can be impaired if regulatory motifs are targeted by nucleotide variations (Wang and Cooper, 2007). Splicing is the process by which introns are removed from a pre-mRNA and exons are consecutively joined. This mechanism is performed in the nucleus by the spliceosome, a macrocomplex constituted by 5 small nuclear ribonucleoproteins (snRNPs) and many other associated proteins (De Conti et al., 2012). The spliceosome recognizes in the pre-mRNA specific sequences which define the exons/introns boundaries and other elements needed to carry out the process. These sequences are: the acceptor or 3′ splice site (3′ss), the donor or 5′ splice site (5′ss), the branch point, the polypyrimidine tract and the auxiliary cis sequences known as splicing regulatory elements (SREs) where enhancer or silencer trans factors can bind. Therefore, any change in the sequence may disrupt splicing (Cartegni et al., 2002). Splicing variants usually break the 3′ss or 5′ss leading to abnormal splicing events such as exon skipping, alternative site usage or intron retention. However, they may also create new splicing sites or strengthen cryptic ones that would then be recognized. Other mechanism that may alter splicing is the disruption of exonic/intronic splicing enhancers (ESEs/ISEs) or the creation of exonic/intronic splicing silencers (ESSs/ISSs) (Abramowicz and Gos, 2018). Nevertheless, it is extremely difficult to identify active SREs and predict the impact of the DNA variants on splicing given the low accuracy of SRE-detection softwares. Therefore, splicing variants can induce abnormal transcripts that either introduce premature termination codons (PTC), in-frame loss of essential protein domains or even inclusion of new translated sequences. Consequently, variants with impact on splicing (or spliceogenic variants) may be associated with an increased risk of a given disease. This ethiopathogenic mechanism has been so far underestimated, even though some authors have suggested that spliceogenic variants may represent more than 60% of disease-causing mutations (López-Bigas et al., 2005).
Previous studies have shown that a significant number of splicing variants have been detected in BRCA2 (Spurdle et al., 2008; Rebbeck et al., 2018). In fact, previous results from our group showed that more than 50% of tested variants of BRCA2 exons 16–27 impaired splicing (Acedo et al., 2012, 2015; Fraile-Bethencourt et al., 2017, 2018). Likewise, at least 24 different BRCA2 alternative transcripts have been identified. They are helpful to interpret the splicing outcomes of genetic variations (Fackenthal et al., 2016) and suggest a fine regulation of BRCA2 exon processing. This feature is supported by the fact that several ESE-rich regions have been functionally mapped by exonic deletions throughout most BRCA2 exons. Thus, these motifs would be involved in precise exon recognition and alternative splicing events (Acedo et al., 2015; Fraile-Bethencourt et al., 2017). Moreover, we showed that functional mapping is an optimal approach that improves ESE-software predictions and facilitates the identification of spliceogenic mutations of this sort of cis-elements.
In this work, we have extended our analysis to BRCA2 exons 14 and 15 by carrying out an in-depth study of candidate spliceogenic variants. We have explored the presence of splicing enhancers in exons 14 and 15 and have undertaken RNA assays of 52 selected variants from both exons.
Materials and Methods
Ethical approval for this study was obtained from the Ethics Review Committee of the Hospital Universitario Río Hortega de Valladolid (6/11/2014).
Bioinformatics: Databases and in silico Analysis
We collected BRCA2 variants from the main databases: ClinVar2, the BRCA Share Database (UMD3) (Beroud et al., 2016) and the Breast Cancer Information Core (BIC4) (Supplementary Table S1). Variants and transcripts were annotated according to the Human Genome Variation Society (HGVS) guidelines on basis of the BRCA2 GenBank sequence NM000059.1. In order to simplify, we identified transcripts with a shortened code that combines the following symbols (Lopez-Perolio et al., 2019): Δ (skipping of reference exonic sequences), (inclusion of reference intronic sequences), E (exon), p (acceptor shift), q (donor shift). When necessary, the exact number of skipped or retained nucleotides is indicated. For example, transcript Δ(E14p10) indicates the use of an alternative acceptor site 10-nt downstream that causes a 10-nt deletion.
In silico analysis was made with the online softwares: NNSplice5 (Reese et al., 1997) and Human Splicing Finder version 3.1 (HSF6) that contain several prediction algorithms of different splicing motifs (Desmet et al., 2009). The following matrices were used: MaxEntScan (MES) (Yeo and Burge, 2004), the HSF branch point detection tool, ESE-finder (Cartegni et al., 2003), the HSF matrices for 9G8 and Tra2β and the HSF matrix for hnRNPA1. All the analyses were carried out with the default threshold values of NNSplice and HSF (NNSplice, 0.4; MES, 3.0; Branch point – no cut-off-; SRE (0–100 scale): SF2/ASF, 72.98; SF2/ASF (IgM – BRCA1), 70.51; SC35, 75.05; SRp40, 78.08; SRp55 73.86; 9G8, 59.245; Tra2β, 75.964 and hnRNPA1, 65.476.
Minigene Construction and Mutations
The minigene MGBR2_14-20 was built as previously reported (Fraile-Bethencourt et al., 2017). A total of 52 variants and 8 microdeletions were introduced into the wild type (wt) minigene by site-directed mutagenesis with the QuikChange Lightning Kit (Agilent, Santa Clara, CA, United States), following the manufacturer’s instructions (Supplementary Table S2). All mutant clones were confirmed by sequencing (Macrogen, Madrid, Spain).
MCF-7 Transfections
Approximately 2 × 105 MCF-7 cells (human breast adenocarcinoma cell line) were plated in four-well plates (Nunc, Roskilde, Denmark). They were grown to 90% confluency in 0.5 mL of medium (MEME, 10% fetal bovine serum, 2 mM glutamine, 1% non-essential amino acids and 1% penicillin/streptomycin). Then, 1 μg of minigene was transfected into MCF-7 cells using low toxicity Lipofectamine (Life Technologies, Carlsbad, CA, United States) in GibcoTM Opti-MemTM medium (Thermo Fisher Scientific, Waltham, MA, United States). Cells were incubated during 48h and then treated with cycloheximide 300 μg/ml (Sigma-Aldrich, St. Louis, MO, United States) for 4 h to inhibit the nonsense-mediated mRNA decay (NMD). The RNA was purified with the Genematrix Universal RNA Purification Kit (EURx, Gdańsk, Poland) with on-column DNAse I digestion.
siRNA Assays
SR proteins were silenced in MCF7 cells by small interfering RNAs (siRNA) against the main SR proteins: SRSF1 (SF2), SRSF2 (SC35), SRSF3 (SRp20), SRSF5 (SRp40), SRSF7 (9G8), SRSF9 (SRp30c), and Tra2β (Supplementary Table S3), using anti-Luciferase siRNA as negative control. Approximately 1.5 × 105 cells were subjected to a two-hit transfection in Optimem medium (Gibco – Life Technologies, Carlsbad, CA, United States) with 3 μl of Oligofectamine (Thermo Fisher Scientific) and the specific siRNA at a final concentration of 0.08 μM on day 2. Then, 2 μg of the wt minigene were transfected with low toxicity Lipofectamine (Thermo Fisher Scientific) on day 4, and RNA was extracted on day 5. Silencing was confirmed by qPCR using 10 ng of cDNA in 25 μl reaction (Supplementary Table S3). Amplification was made with SG qPCR Master Mix (Eurx, Gdańsk, Poland). Each siRNA/minigene transfection as well as all the qPCR experiments were carried out in duplicate.
RT-PCR and Transcripts Amplification
Retrotranscription was carried out with 400 ng of RNA and the RevertAid First Strand cDNA Synthesis Kit (Life Technologies), using the specific minigene primer RTPSPL3-RV (5′-TGAGGAGTGAATTGGTCGAA-3′). Samples were incubated at 42°C for 1 h, followed by 5 min at 70°C. Transcripts were amplified with Platinum Taq DNA polymerase (Life Technologies) using 40 ng of cDNA and the primers pMAD_607FW (Patent P201231427, CSIC) and RTBR2_ex17RV2 (5′-GGCTTAGGCATCTATTAGCA-3′). PCR consisted of: denaturation step at 94°C for 2 min, followed by 35 cycles 94°C-30 s, 60°C-30 s and 72°C-1 min/kb, and a final extension step at 72°C for 5 min. Transcripts were sequenced at the Macrogen Spain facility.
In order to relatively quantify all transcripts, semi-quantitative fluorescent RT-PCRs were undertaken in triplicate with the primers pMAD_607FW (FAM-labeled) and RTBR2_ex17RV2 and Platinum Taq DNA polymerase (Life Technologies) under standard conditions except that 26 cycles were herein applied (Acedo et al., 2015). FAM-labeled products were run with LIZ-1200 Size Standard at the Macrogen facility and analyzed with the Peak Scanner software V1.0 (Life Technologies). Only peak heights ≥ 50 RFU (Relative Fluorescence Units) were considered.
Results
Minigene Construction and ESE Mapping
The minigene MGBR2_14-20 had previously been used to study variants of exons 16, 17, and 18, proving that it is a reliable and robust tool to functionally assay splicing variants (Fraile-Bethencourt et al., 2017, 2018; Montalban et al., 2018). The MGBR2_14-20 is a 10.7 Kb construct which, after transfection in MCF-7 cells, produces a transcript with the following structure: V1-BRCA2_exons from 14 to 20-V2 (1,806 nt) (Figure 1). To study exons 14 and 15, cDNA was amplified with a forward primer located in V1 (pMAD_607FW) and a reverse primer located in exon 17 (RTBR2_Ex17RV2), with an expected transcript size of 1028 nt (Figures 1B,C).
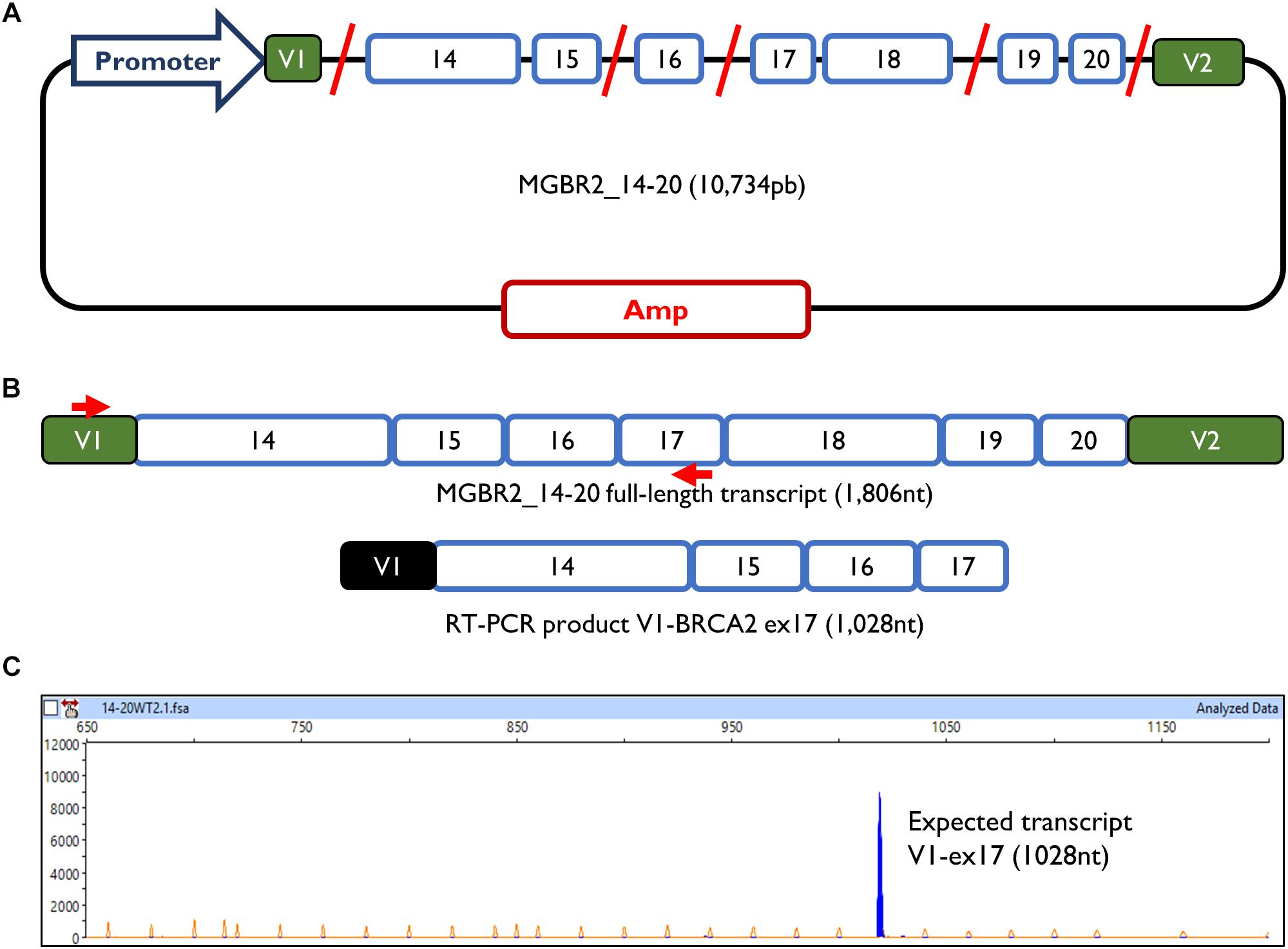
Figure 1. Minigene MGBR2_14-20. (A) Schematic representation of MGBR2_14-20. BRCA2 exons 14–20 are represented in blue boxes. Red lines represented the shortened introns. (B) Expected MGBR2_14-20 transcript (1,806 nt) and the amplified RT-PCR product. Specific amplification primers are shown as red arrows. (C) Capillary electrophoresis result of the functional assay of the wild type MGBR2_14-20 in MCF-7 cells. The full-length transcript is shown as a blue peak. The Genescan Liz-1200 size standard is shown as orange/faint peaks. Fragment sizes (nt) and relative fluorescent units are indicated on the x- and y-axes, respectively.
To map ESEs, 30-nt overlapping microdeletions were performed along the first and the last 55-nt of exons 14 and 15 (Acedo et al., 2015), always preserving the splice site conserved positions (the first 2 nt and the last 3 nt of the exon). None of the deletions but one altered splicing, suggesting the absence of cis-regulatory motifs within these segments. Only exon 15 deletion c.7463_7492 impaired splicing generating a major aberrant transcript (62%) with a deletion of 83 nt [Δ(E15p83)]. This transcript was caused by use of a cryptic 3′ss 83-nt downstream, which is stronger than the canonical one, according to Max Ent Scan (MES; 6.18 vs. 5.16) (Figure 2). To determine which ESEs were implied in exon 15 recognition, siRNA experiments against the main splicing factors (SFSR1, SFSR2, SFSR3, SFSR5, SRSF7, SFSR9, and Tra2β) were accomplished (Supplementary Figure S1). However, none of them affected the recognition of exons 14 and 15.
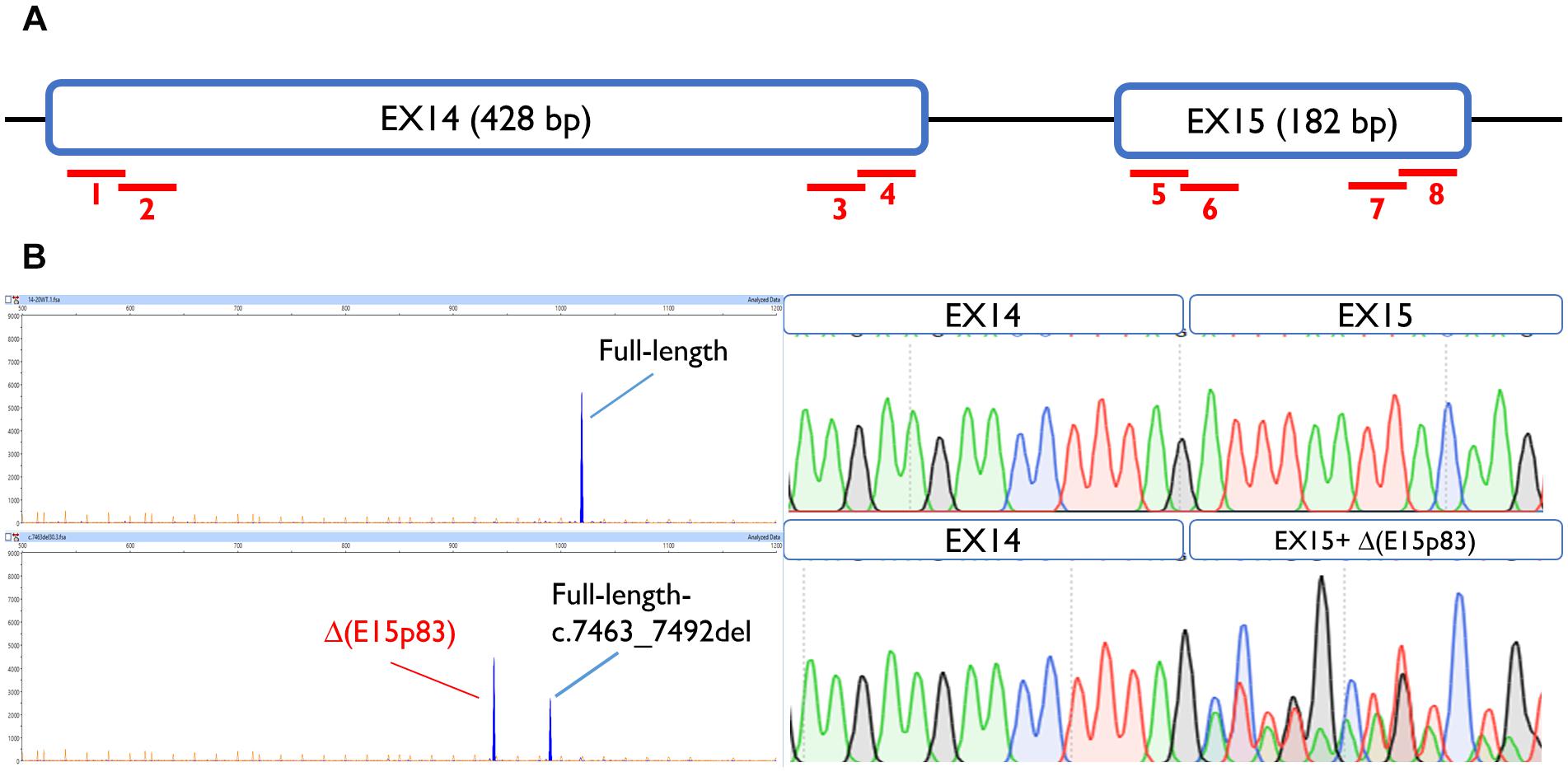
Figure 2. Exon 15 ESE mapping: functional assay of c.7463_7492del positive microdeletion. (A) Schematic representation of BRCA2 exons 14 and 15 and the eight microdeletions: 1, c.7010_7039del; 2, c.7035_7064del; 3, c.7378_7407del; 4, c.7402_7432del; 5, c.7438_7467del; 6, c.7463_7492del; 7, c.7561_7590del; 8, c.7586_7615del. (B) Capillary electrophoresis and sequence results of functional assays of microdeletion c.7463_7492del in MCF-7 cells.
Variant Collection and Bioinformatics Analysis
A total of 294 different variants, spread throughout exons 14 and 15 and flanking introns, were collected from the main databases (ClinVar, UMD and BIC) (Supplementary Table S1). They were analyzed in silico with MES and NNSplice for splice site prediction, and with ESE/ESS estimation algorithms integrated in Human Splicing Finder (HSF). Potential splicing variants were selected following these criteria: creation or disruption of splice sites (according to MES or NNSplice); disruption of the branch point; disruption of the polypyrimidine tract; elimination of enhancers or creation of silencers. Some of the selected variants had a combined effect, for example, they were predicted to simultaneously create an ESS and removed an ESE. A total of 53 candidate variants (∼18%) that included 19 intronic, 18 missense, 5 nonsense, 8 synonymous, and 3 frameshift variants were selected (Table 1). According to their previous clinical classification, the selection contained: 8 benign or likely benign variants, 30 VUS and 15 pathogenic or likely pathogenic variants.
Bioinformatics indicated that 13 variants disrupted the natural splice sites, three decreased their scores (one disrupted the polypyrimidine tract), 11 created new splice sites, one decreased the branch point score (HSF: 79.34→55.5), three altered intronic splicing elements (ISEs and ISSs) and 22 altered exonic splicing elements (ESEs and ESSs) (Table 1). Exceptionally, variants c.7008-5T > C (ivs13-5T > C) and c.7435+5T > C (ivs14+5T > C) were also selected because, even though the bioinformatics did not show significant score changes (MES: 10.37→8.48 and 5.64→5.56, respectively), these positions are relevant for exon recognition. Thus, c.7008-5T > C is the closest position of the polypyrimidine tract to the canonical acceptor site, and c.7435+5T > C is part of the consensus 5′ss sequence and +5 changes were previously associated with disease (Sharma et al., 2014; Montalban et al., 2018).
Functional Splicing Assays Using the Minigene MGBR2_14-20
A total of 52 variants were genetically engineered in the wt MGBR2_14-20 by using specific primers (Supplementary Table S2). Despite 53 variants were initially selected, mutagenesis experiments did not work for c.7598C > G variant. The 52 mutant minigenes were checked by Sanger sequencing and assayed in MCF-7 cells. Results showed that 12 of them (23%) altered splicing (Table 2 and Figure 3), seven of which had previously been classified as pathogenic and five as VUS (Table 3). Among these 12 variants, there were 9 intronic, 2 missense and 1 nonsense changes. Functionally, the 9 intronic variants (c.7008-3C > G, c.7008-2A > T, c.7008-1G > A, c.7435+1G > C, c.7436-2A > T, c.7436-1G > A, c.7617+1G > A, c.7617+1G > T, and c.7617+2T > G) disrupted the natural splice sites, the two missense changes (c.7177A > G and c.7447A > G) triggered the use of de novo splice sites and originated other transcripts, and the nonsense one (c.7501C > T) probably altered SREs despite it was primarily selected because of the creation of a new 5′ss (Table 1). According to Vallée et al. (2016), nine variants induced severe splicing disruptions as they produced more than 60% of aberrant transcripts, ranging from 92.8 to 100% (Table 3).
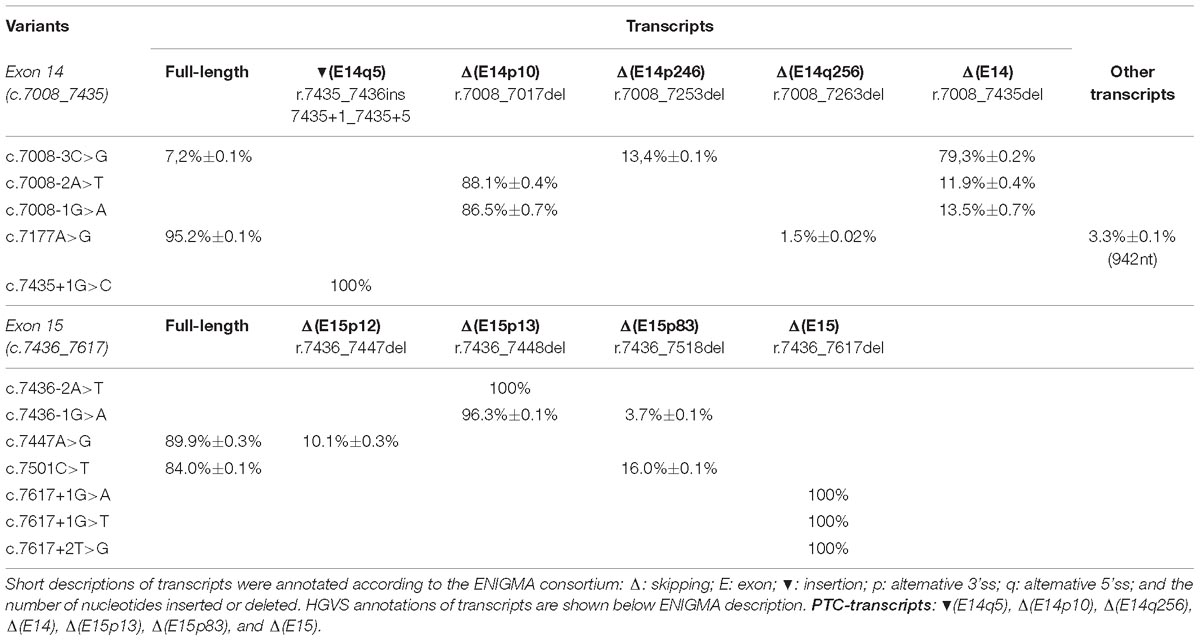
Table 2. Quantification of the transcripts found by capillary electrophoresis after functional assays of BRCA2 exons 14 and 15 variants.
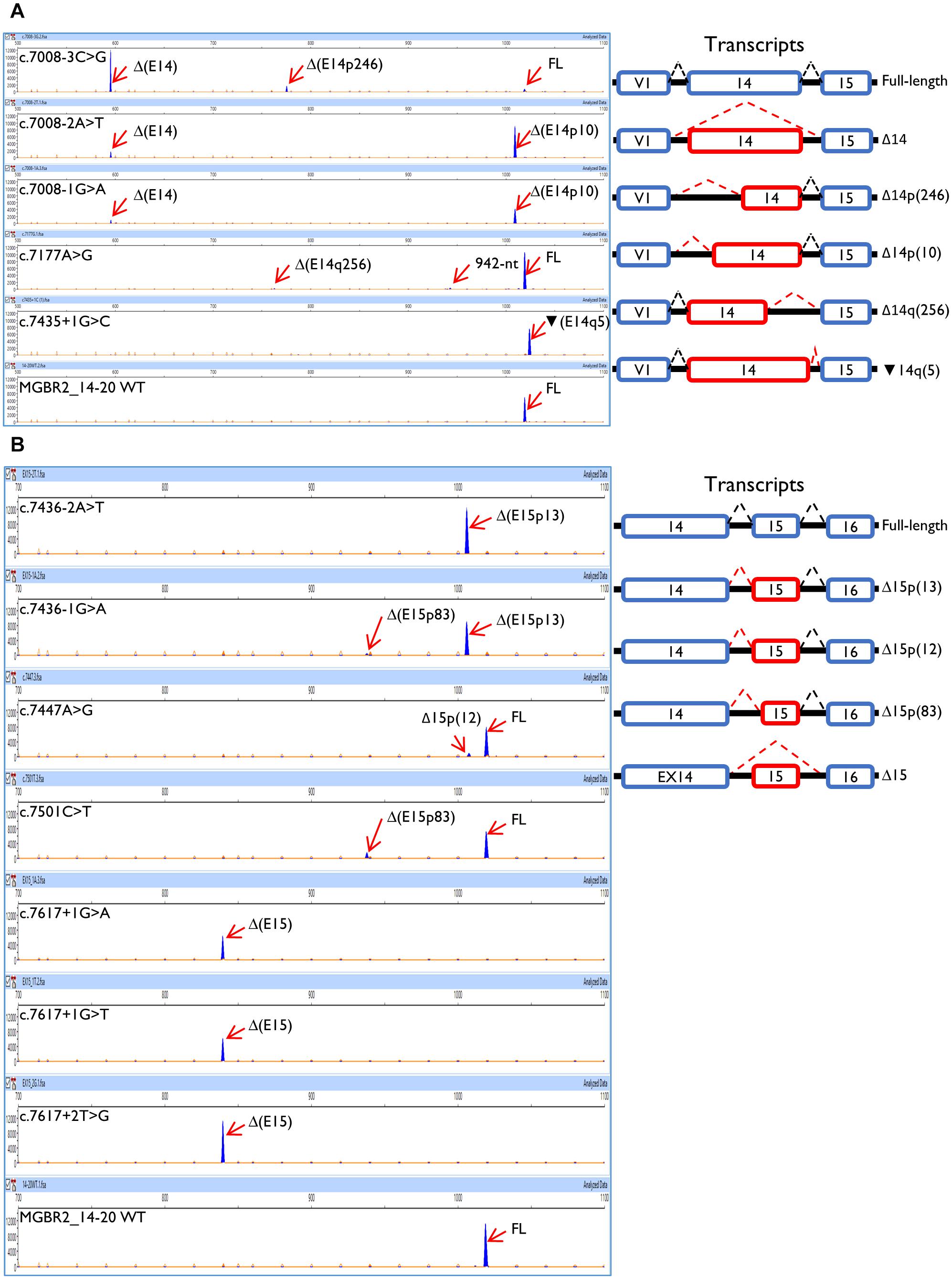
Figure 3. Functional assays of the BRCA2 exons 14 and 15 variants using the MGBR2_14-minigene. (A) Exon 14 variants. (B) Exon 15 variants. On the left, capillary electropherograms are shown. Transcripts were amplified using pMAD_607FW-FAM and RTBR2_Ex17RV2. Labeled transcripts are shown as blue peaks, LIZ1200 was used as size standard (orange peaks). The expected size of the full length transcript is 1028 nt (1018–1019 nt according to Peak Scanner). On the right, splicing patterns are represented. While blue boxes are natural exons, red boxes represent aberrant exons; dashed black and red lines show canonical and aberrant splicing events, respectively.
Acceptor Site Variants
Exon 14 3′ss was affected by c.7008-3C > G, c.7008-2A > T, and c.7008-1G > A, whereas c.7008-5T > C only produced the canonical transcript (Table 2). Curiously, while the main outcome of c.7008-3C > G was exon skipping (ΔE14), the variants c.7008-2A > T and c.7008-1G > A produced mostly the aberrant transcript Δ(E14p10), in which a cryptic 3′ss was recognized by the spliceosome 10-nt downstream. This cryptic 3′ss was not detected by NNSplice or MES tools. The loss of 10-nt at the beginning of exon 14 would generate a PTC 27 codons downstream (p.Thr2337Asnfs∗27). Our results also revealed the use of another cryptic 3′ss (MES = 4.44) within exon 14, located 246-nt downstream the natural one, originating the transcript Δ(E14p246) (13%) in the c.7008-3C > G assay (Table 2). The transcript Δ(E14p246) led to an in-frame deletion of 82 amino acids from position p.2337 to p.2418 (p.Thr2337_Arg2418del).
The branch point (c.7436-22C > T), polypyrimidine tract (c.7436-14T > G) and −4 (c.7436-4A > G, c.7436-4A > T) variants of exon 15 did not impair splicing. Other exon 15 acceptor variants, such as c.7436-2A > T and c.7436-1G > A, mainly caused isoform Δ(E15p13) through use of a cryptic acceptor 13-nt downstream (Table 2). The use of this cryptic acceptor would provoke a frameshift deletion, leading to a PTC in the protein (p.Asp2479Valfs∗41). Like exon 14 cryptic acceptor, this exon 15 cryptic 3′ss was not detected by NNSplice or MES. Variant c.7436-1G > A also produced the minor transcript ΔE15p83 (3.7%) a 83-nt deletion that introduced a frameshift (p.Asp2479Alafs∗32) and a PTC 32 codons downstream. This transcript was generated by a cryptic acceptor site 83-nt downstream (MES = 6.28). In summary, we found 5 variants (c.7008-3C > G, c.7008-2A > T, c.7008-1G > A, c.7436-2A > T, and c.7436-1G > A) that altered 3′ss recognition of exons 14 and 15, leading to aberrant splicing (Table 2). Remarkably, all of them showed the total absence of canonical transcript, except for c.7008-3C > G that produced 7% of the full-length transcript. Moreover, our results unveiled exon 14 and 15 cryptic splice sites that are only recognized when natural acceptors are disrupted.
Donor Site Variants
Seven variants were predicted to disrupt donor sites: c.7435+1G > C, c.7435+3A > G, c.7435+5T > C, and c.7435+6G > A (exon 14) and c.7617+1G > A, c.7617+1G > T, and c.7617+2T > G (exon 15; Table 1). Among the exon 14 variants, only c.7435+1G > C impaired splicing (Table 2) producing a single transcript with a 5-nt insertion [(E14q5)], due to the recognition of a cryptic 5′ss in ivs14. The
(E14q5) is an aberrant splicing isoform which leads to PTC (p.Asp2479Glyfs∗4). Surprisingly, this cryptic donor was not detected by NNSplice software as the canonical one was. Regarding exon 15 donor variants, our results showed that all of them (c.7617+1G > A, c.7617+1G > T, and c.7617+2T > G) produced Δ(E15) as unique transcript (Table 2), which generates a PTC eight codons downstream (p.Asp2479Alafs∗8).
Splicing Regulatory Element-Variants
A total of 26 SRE variants were selected according to the criteria above described and assayed in MCF-7 cells (Table 1). Only c.7177A > G altered weakly splicing resulting in about 5% of aberrant transcripts (Table 2). This matches with the creation of a new donor site that was not detected by the splicing prediction software. Conversely, none of the exon 15 SRE-variants impaired splicing even though microdeletion tests had revealed a presumed ESE interval (c.7463_7492).
New Splice Site Variants
We have assayed 10 variants of this type, six in exon 14 (c.7030A > G, c.7180A > T, c.7182A > G, c.7203A > G, c.7266T > A, and c.7418G > A) and four in exon 15 (c.7447A > G, c.7466A > G, c.7501C > T, and c.7611_7615delTAAAC) (Table 1). Results showed that two exon 15 variants (c.7447A > G and c.7501C > T) slightly disrupted splicing (Table 2). The variant c.7447A > G generated a new acceptor but most of its outcome was a full-length transcript (Table 2). The variant c.7501C > T was predicted to create a new strong donor (NNSplice: < 0.4→0.96 and MES: 2.44→10.19) (Table 1). However, only 16% of the transcripts made use of this cryptic donor 83-nt upstream of the natural one (Table 2).
Analysis of Transcripts
The so called full-length or canonical transcript (expected size: 1028-nt) was amplified with primers placed on vector exon V1 and BRCA2 exon 17. Apart from the canonical transcript, we have detected other ten different ones (Figure 3). Aberrant exon 14 splicing produced six different isoforms, but only five of them were characterized: Δ(E14), Δ(E14p10), Δ(E14p246), Δ(E14q256), and (E14q5). A 942-nt transcript of unknown structure could also be detected by capillary electrophoresis. All exon 14 isoforms, except for Δ(E14p246), introduced PTCs into the mRNA. The isoform Δ(E14p246) was seen in up to 13% of the c.7008-3C > G transcripts (Table 2). Exon 15 variants produced 4 different isoforms besides the canonical one: Δ(E15p12), Δ(E15p13), Δ(E15q83), and Δ(E15). The Δ(E15p13), Δ(E15q83) and Δ(E15) isoforms created PTCs while Δ(E15p12) (new acceptor 12-nt downstream) kept the reading frame, although this isoform only accounted for 10% of the transcripts (Table 2).
Discussion
Due to the implementation of Next Generation Sequencing in the clinical setting (Slavin et al., 2015), a large number of variants have been detected in disease-responsible genes. HBOC and the breast cancer susceptibility genes are not exceptions, where thousands of different variants have been reported although many of them are considered as VUS (Spurdle et al., 2012; Slavin et al., 2017). In this context, the functional and clinical classifications pose a challenge for Medical Genetics. We have herein functionally assayed 52 BRCA2 variants using the minigene MGBR2_14-20, whose reliability had been previously proven (Fraile-Bethencourt et al., 2017, 2018; Montalban et al., 2018). We found 12 variants that altered splicing, nine of which would severely alter the protein. This study forms part of a comprehensive study of our group concerning potential splicing BRCA2 variants, where 22 exons and up to 335 variants have been assayed using three minigenes: MGBR2_2-9 (Fraile-Bethencourt et al., 2019), MGBR2_14-20 (Fraile-Bethencourt et al., 2017, 2018) and MGBR2_19-27 (Acedo et al., 2012, 2015). The following advantages of the minigene technology should be underlined: (i) analysis of a single allele outcome without the interference of the wt counterpart of a patient sample; (ii) simple and fast quantification of generated transcripts by fluorescent capillary electrophoresis with minimum hands-on time versus other proposed methods (Farber-Katz et al., 2018); (iii) versatility, one single multi-exon minigene allows to assay variants from different exons; (iv) capability of analysis in many cell types to check effects derived from tissue-specific alternative splicing; (v) high reproducibility of splicing physiological/pathological patterns. In fact, we have previously provided many examples of the minigene reproducibility. In the case of BRCA2 exons 14 and 15, variants c.7008-2A > T, c.7617+1G > A, and c.7617+2T > G displayed similar patterns in patient RNA and minigene assays (Vreeswijk et al., 2009; Colombo et al., 2013; de Garibay et al., 2014). Moreover, another 31 variants of this and other constructs replicated previously reported patient splicing outcomes (Acedo et al., 2015; Fraile-Bethencourt et al., 2017, 2018, 2019), confirming that splicing reporters are robust and valuable tools to test the impact of variants on splicing, especially when patient RNA is not available.
Here, we have shown that nine variants drastically disrupted the splicing pattern. We found five 3′ss disrupting variants, one of which (c.7008-3C > G) provoked exon 14 skipping, whereas the rest of them induced the use of cryptic sites (c.7008-2A > T, c.7008-1G > A, c.7436-2A > T, and c.7436-1G > A). Moreover, variant c.7435+1G > C, which disrupt the exon 14 donor site, provoked the use of a cryptic donor. Curiously, neither c.7435+3A > G, c.7435+5T > C nor c.7435+6G > A, which are also part of the consensus 5′ss, affected splicing. This may be due to the low frequency of +5T and +6G at these positions, so that any nucleotide change only equals or improves splice site strength. Conversely, +3A is the main nucleotide at this position (71%) but +3G is also relatively frequent (24%) (Zhang, 1998). Thus, a substitution A to G might have a reduced or no splicing impact, as it is the case of the c.7435+3A > G variant. As a matter of fact, variant c.9501+3A > T produced 87% of the canonical transcript (Acedo et al., 2015). On the other hand, the three variants of exon 15 donor site c.7617+1G > A, c.7617+1G > T, and c.7617+2T > G resulted in exon skipping. In this regard, it was recently recommended the use of a combination of the computational tools HSF plus Splice Site Finder-like to select candidate splice site variants with high sensitivity and specificity (Moles-Fernández et al., 2018). According to HSF, variants c.7435+3A > G, c.7435+5T > C, and c.7435+6G > A showed only minimal changes of the splice site scores (±1%) so that they should have been excluded from subsequent functional tests.
Concerning other splicing motifs, minigenes also allow the identification of regulatory sequences (splicing enhancers and silencers) and splicing factors involved in the specific regulation (Baralle and Buratti, 2017). Indeed, the SRE mapping constitutes an interesting experimental approach since it identifies critical regions for exon recognition. In this context, our group previously found exonic variants that disrupted splicing through elimination of ESEs or creation of de novo silencers, such as BRCA2 variants c.7985C > G (predicted missense p.Thr2662Arg) or c.8009C > A (predicted nonsense p.Ser2670∗) (Fraile-Bethencourt et al., 2017). The splicing assays showed how both variants elicited complete splicing aberrations (mainly exon 18 skipping). However, they were a priori classified as missense and nonsense variants, respectively, due to their predicted protein effect. After microdeletion mapping, we have identified a putative ESE-region in exon 15 (c.7463_7492). These ESEs might be involved in exon 15 3′ss recognition since their loss produced the use of a cryptic acceptor 83-nt downstream (Figure 2). Curiously, we did not find any ESE-variants that affected pre-mRNA processing. Only, c.7501C > T, which lays near to this presumed ESE interval, provoked a similar outcome to that of r.7463_7492 deletion (Table 2). In summary, we have tested a total of 117 different variants in minigene MGBR2_14-20, from exons 14–18 (Table 4), 51 of which (43.6%) induced abnormal splicing patterns: 31 disrupted the natural splice sites (16 3′ss and 15 5′ss), 11 affected SREs, six created de novo splice sites and three altered the polypyrimidine tract.
Transcript Interpretation
The BRCA2 exons 14 (c.7008_7435) and 15 (c.7436_7617) encode for amino acids 2336 to p.2539, which are part of the DNA Binding Domain (DBD; p.2459_p.3190). The DBD is the largest conserved region of BRCA2 and is composed of a helical domain (HD), three oligonucleotide binding sites (OB) and a tower domain (TD) (Guidugli et al., 2014). Specifically, exons 14 and 15 are part of the HD (p.2481_2667) that binds to the protein DSS1 (deleted in split-hand/split-foot syndrome) in the region comprised by the residues 2472–2957 (Marston et al., 1999). Among these residues, a total of 125 are strictly conserved from human to sea urchin. DSS1 is important for BRCA2 stability, since its loss leads to a reduction of BRCA2 levels in human cells (Li et al., 2006). Moreover, exons 14 and 15 coding region is also recognized by FANCD2 (Fanconi anemia group D2) protein, which binds to the BRCA2 protein between codons 2350 and 2545 (Hussain et al., 2004). FANCD2, like BRCA2, is one of the 16 proteins that form the Fanconi Anemia complex, aimed to repair DNA interstrand crosslinks. However, it was shown that BRCA2-FANCD2 association has an independent function in the Fanconi Anemia pathway. The BRCA2-FANCD2 complex is involved in the restart of the replication fork, by protecting the nascent DNA strands from degradation (Raghunandan et al., 2015). Taken together, exons 14 and 15 contain crucial sequences of BRCA2, owing to its function in homologous recombination and other relevant biological processes. Moreover, the biological relevance of exons 14 and 15 is supported by the presence of numerous pathological nonsense and frameshift variants at the mutation databases. Hence, the exon 14–15 spliceogenic variants that induce PTC-transcripts may be associated with an increased risk of HBOC.
Clinical Interpretation of Spliceogenic Variants
Twelve variants altered splicing with different patterns. While some variants caused the total absence of canonical transcript, others originated just ∼5% of aberrant transcripts (Table 2). Variants c.7008-2A > T, c.7008-1G > A, c.7435+1G > C, c.7436-2A > T, c.7436-1G > A, c.7617+1G > A, c.7617+1G > T, and c.7617+2T > G did not produce the canonical transcript. Moreover, all the transcripts generated by these variants were frameshift transcripts. Thus, following the criteria of the American College of Medical Genetics and Genomics (ACMG), these eight variants should be classified as pathogenic variants (Table 3). Variant c.7008-3C > G produced Δ(E14) as the major transcript, but the full-length (∼7%) and the in-frame transcript Δ(E14p246) (∼13%) were also identified. The Δ(E14p246) isoform contains a deletion of 82 non-conserved amino acids (p.Thr2337_Arg2418del) that form part of the FANCD2 binding site (p.Thr2350_Val2545). At the UMD database, c.7008-3C > G is classified as a “causal” variant because of the skipping of exon 14, but ClinVar shows it as VUS. According to the ACMG criteria, this variant should be cataloged as likely pathogenic (Table 3). On the other hand, according to the guidelines of the ENIGMA consortium7 eight variants should be classified as Class 4 (Likely pathogenic), three as VUS and one as pathogenic, though this is due to its predicted nonsense effect (Table 3).
On the other hand, missense variants c.7177A > G (p.Met2393Val) and c.7447A > G (p.Ser2483Gly) produced the canonical transcript as the main outcome and only 5 and 10% of aberrant isoforms, respectively (Table 2). The canonical transcript generated by these two variants carried missense changes, but in silico predictions with the PolyPhen tool8 suggested that p.Met2393Val and p.Ser2483Gly were both benign changes. So, following the ACMG criteria, c.7177A > G and c.7447A > G remain as VUS (Table 3). Finally, variant c.7501C > T generated mainly the canonical transcript (84%) that includes a nonsense change (pathogenic according to ClinVar) so this change should be classified as pathogenic under a combined splicing-protein viewpoint. Altogether, we have re-classified three variants (c.7008-3C > G, c.7008-1G > A, and c.7436-1G > A) from VUS to pathogenic or likely pathogenic, and we have provided further support for the classification of six spliceogenic variants (c.7008-2A > T, c.7435+1G > C, c.7436-2A > T, c.7617+1G > A, c.7617+1G > T, and c.7617+2T > G). Interestingly, c.7617G > A is classified as “causal” in the UMD database9 and indicated that causes exon 15 skipping but no functional proofs were provided. However, the functional assay of MGBR2_14-20-c.7617G > A only showed the canonical transcript (Supplementary Figure S2). In fact, NNSplice, HSF and MES estimated just a small decrease (−19, −11.6, and −24%, respectively) of the donor site score. Therefore, c.7617G > A behaves as a neutral variant from the splicing perspective. Finally, the minigene MGBR2_14-20 results of 5 exons suggest that a total of 39 spliceogenic variants should be classified as pathogenic or likely pathogenic (Table 4), lending further support to this strategy for the clinical interpretation of variants.
In summary, splicing is a finely regulated mechanism which can be altered by any change in the sequence. The disruption of this process might cause serious effects on the protein, from the loss of important domains to the generation of a PTC. Thus, splicing alteration is a common mechanism of gene inactivation, which is often involved in human disease. Here, we have revealed 12 spliceogenic variants of BRCA2 exons 14 and 15. The minigene based assays offer a relevant information about effects of splicing variants, since they allow to functionally assay almost any DNA change, to quantify all generated transcripts, including very rare ones, and to initially study the splicing regulation. Moreover, we have detected an ESE region that seems to be regulating exon 15 splicing, and therefore constitutes a hypothetical hotspot for putative ESE-mutations. Indeed, pSAD-based minigenes have constituted an invaluable technology to functionally test variants of other disease genes such as GRN (Frontotemporal Dementia), SERPINA1 (Severe alpha-1 antitrypsin deficiency) and CHD7 (Charge Syndrome) genes (Lara et al., 2014; Villate et al., 2018). Altogether, these results highlight, once more, the importance of RNA assays to know the splicing effects of DNA variants to give support to their clinical interpretation and consequently to activate preventive and/or therapeutic interventions.
Author Contributions
EF-B contributed to the bioinformatics analysis, minigene construction, manuscript writing, and performed most of the splicing functional assays. BD-G and AV-P participated in minigene construction, mutagenesis experiments, and functional assays. SG-B and MC carried out the data collection of variants and their computer analysis, as well as manuscript editing. EV conceived the study and the experimental design, supervised all the experiments, and wrote the manuscript. All authors contributed to data interpretation, revisions of the manuscript, and approved the final version of the manuscript.
Funding
EV’s lab was supported by grants from the Spanish Ministry of Science, Innovation and Universities, Plan Nacional de I+D+I 2013-2016, Instituto de Salud Carlos III (PI13/01749 and PI17/00227) co-funded by FEDER from Regional Development European Funds (European Union), and grants CSI090U14 (ORDEN EDU/122/2014) and CSI242P18 (actuación cofinanciada P.O. FEDER 2014-2020 de Castilla y León) from the Consejería de Educación, Junta de Castilla y León. EF-B was supported by a predoctoral fellowship from the University of Valladolid and Banco de Santander (2015–2019). AV-P was supported by a predoctoral fellowship from Consejería de Educación, Junta de Castilla y León (2018–2022).
Conflict of Interest Statement
The authors declare that the research was conducted in the absence of any commercial or financial relationships that could be construed as a potential conflict of interest.
Acknowledgments
We acknowledge support of the publication fee by the CSIC Open Access Publication Support Initiative through its Unit of Information Resources for Research (URICI).
Supplementary Material
The Supplementary Material for this article can be found online at: https://www.frontiersin.org/articles/10.3389/fgene.2019.00503/full#supplementary-material
Footnotes
- ^ https://www.ncbi.nlm.nih.gov/clinvar
- ^ https://www.ncbi.nlm.nih.gov/clinvar/
- ^ http://www.umd.be/BRCA2/
- ^ https://research.nhgri.nih.gov/projects/bic/index.shtml
- ^ http://www.fruitfly.org/seq_tools/splice.html
- ^ http://www.umd.be/HSF3/
- ^ https://enigmaconsortium.org/library/general-documents/enigma-classification-criteria/
- ^ http://genetics.bwh.harvard.edu/pph2/
- ^ https://goo.gl/bG2V4n
References
Abramowicz, A., and Gos, M. (2018). Splicing mutations in human genetic disorders: examples, detection, and confirmation. J. Appl. Genet. 59, 253–268. doi: 10.1007/s13353-018-0444-447
Acedo, A., Hernández-Moro, C., Curiel-García, Á, Díez-Gómez, B., and Velasco, E. A. ( (2015). Functional classification of BRCA2 DNA variants by splicing assays in a large minigene with 9 exons. Hum. Mutat. 36, 210–221. doi: 10.1002/humu.22725
Acedo, A., Sanz, D. J., Durán, M., Infante, M., Pérez-Cabornero, L., Miner, C., et al. (2012). Comprehensive splicing functional analysis of DNA variants of the BRCA2 gene by hybrid minigenes. Breast Cancer Res. 14:R87. doi: 10.1186/bcr3202
Baralle, D., and Buratti, E. (2017). RNA splicing in human disease and in the clinic. Clin. Sci. 131, 356–368. doi: 10.1042/CS20160211
Beroud, C., Letovsky, S. I., Braastad, C. D., Caputo, S. M., Beaudoux, O., Bignon, Y. J., et al. (2016). BRCA share: a collection of clinical BRCA gene variants. Hum. Mutat. 37, 1318–1328. doi: 10.1002/humu.23113
Buys, S. S., Sandbach, J. F., Gammon, A., Patel, G., Kidd, J., Brown, K. L., et al. (2017). A study of over 35,000 women with breast cancer tested with a 25-gene panel of hereditary cancer genes. Cancer 123, 1721–1730. doi: 10.1002/cncr.30498
Cartegni, L., Chew, S. L., and Krainer, A. R. (2002). Listening to silence and understanding nonsense: exonic mutations that affect splicing. Nat. Rev. Genet. 3, 285–298. doi: 10.1038/nrg775
Cartegni, L., Wang, J., Zhu, Z., Zhang, M. Q., and Krainer, A. R. (2003). ESEfinder: a web resource to identify exonic splicing enhancers. Nucleic Acids Res. 31, 3568–3571.
Colombo, M., De Vecchi, G., Caleca, L., Foglia, C., Ripamonti, C. B., Ficarazzi, F., et al. (2013). Comparative in vitro and in silico analyses of variants in splicing regions of brca1 and brca2 genes and characterization of novel pathogenic mutations. PLoS One 8:e57173. doi: 10.1371/journal.pone.0057173
De Conti, L., Baralle, M., and Buratti, E. (2012). Exon and intron definition in pre-mRNA splicing. Wiley Interdiscip. Rev. RNA 4, 49–60. doi: 10.1002/wrna.1140
de Garibay, G. R., Acedo, A., García-Casado, Z., Gutiérrez-Enríquez, S., Tosar, A., Romero, A., et al. (2014). Capillary electrophoresis analysis of conventional splicing assays: IARC analytical and clinical classification of 31 BRCA2 genetic variants. Hum. Mutat. 35, 53–57. doi: 10.1002/humu.22456
Desmet, F. O., Hamroun, D., Lalande, M., Collod-Beroud, G., Claustres, M., and Beroud, C. (2009). Human Splicing Finder: an online bioinformatics tool to predict splicing signals. Nucleic Acids Res. 37:e67. doi: 10.1093/nar/gkp215
Eccles, D. M., Mitchell, G., Monteiro, A. N. A., Schmutzler, R., Couch, F. J., Spurdle, A. B., et al. (2015). BRCA1 and BRCA2 genetic testing-pitfalls and recommendations for managing variants of uncertain clinical significance. Ann. Oncol. 26, 2057–2065. doi: 10.1093/annonc/mdv278
Fackenthal, J. D., Yoshimatsu, T., Zhang, B., de Garibay, G. R., Colombo, M., De Vecchi, G., et al. (2016). Naturally occurring BRCA2 alternative mRNA splicing events in clinically relevant samples. J. Med. Genet. 53, 548–558. doi: 10.1136/jmedgenet-2015-103570
Farber-Katz, S., Hsuan, V., Wu, S., Landrith, T., Vuong, H., Xu, D., et al. (2018). Quantitative Analysis of BRCA1 and BRCA2 Germline Splicing Variants Using a Novel RNA-Massively Parallel Sequencing Assay. Front. Oncol. 8:286. doi: 10.3389/fonc.2018.00286
Fraile-Bethencourt, E., Díez-Gómez, B., Velásquez-Zapata, V., Acedo, A., Sanz, D. J., and Velasco, E. A. (2017). Functional classification of DNA variants by hybrid minigenes: identification of 30 spliceogenic variants of BRCA2 exons 17 and 18. PLoS Genet. 13:e1006691. doi: 10.1371/journal.pgen.1006691
Fraile-Bethencourt, E., Valenzuela-Palomo, A., Díez-Gómez, B., Acedo, A., and Velasco, E. A. (2018). Identification of Eight Spliceogenic Variants in BRCA2 Exon 16 by Minigene Assays. Front. Genet. 9:188. doi: 10.3389/fgene.2018.00188
Fraile-Bethencourt, E., Valenzuela-Palomo, A., Díez-Gómez, B., Goina, E., Acedo, A., Buratti, E., et al. (2019). Mis-splicing in breast cancer: identification of pathogenic BRCA2 variants by systematic minigene assays. J. Pathol. doi: 10.1002/path.5268 [Epub ahead of print].
Guidugli, L., Carreira, A., Caputo, S. M., Ehlen, A., Galli, A., Monteiro, A. N., et al. (2014). Functional assays for analysis of variants of uncertain significance in BRCA2. Hum. Mutat. 35, 151–164. doi: 10.1002/humu.22478
Hussain, S., Wilson, J. B., Medhurst, A. L., Hejna, J., Witt, E., Ananth, S., et al. (2004). Direct interaction of FANCD2 with BRCA2 in DNA damage response pathways. Hum. Mol. Genet. 13, 1241–1248. doi: 10.1093/hmg/ddh135
Lara, B., Martínez, M. T., Blanco, I., Hernández-Moro, C., Velasco, E. A., Ferrarotti, I., et al. (2014). Severe alpha-1 antitrypsin deficiency in composite heterozygotes inheriting a new splicing mutation QOMadrid. Respir. Res. 15, 125. doi: 10.1186/s12931-014-0125-y
Li, J., Zou, C., Bai, Y., Wazer, D. E., Band, V., and Gao, Q. (2006). DSS1 is required for the stability of BRCA2. Oncogene 25, 1186–1194. doi: 10.1038/sj.onc.1209153
López-Bigas, N., Audit, B., Ouzounis, C., Parra, G., and Guigó, R. (2005). Are splicing mutations the most frequent cause of hereditary disease? FEBS Lett. 579, 1900–1903. doi: 10.1016/j.febslet.2005.02.047
Lopez-Perolio, I., Leman, R., Behar, R., Lattimore, V., Pearson, J. F., Castéra, L., et al. (2019). Alternative splicing and ACMG-AMP-2015-based classification of PALB2 genetic variants: an ENIGMA report. J. Med. Genet. 2019, 1–9. doi: 10.1136/jmedgenet-2018-105834
Marston, N. J., Richards, W. J., Hughes, D., Bertwistle, D., Marshall, C. J., and Ashworth, A. (1999). Interaction between the Product of the Breast Cancer Susceptibility Gene BRCA2 and DSS1, a Protein Functionally Conserved from Yeast to Mammals. Mol. Cell. Biol. 19, 4633–4642. doi: 10.1128/MCB.19.7.4633
Mavaddat, N., Peock, S., Frost, D., Ellis, S., Platte, R., Fineberg, E., et al. (2013). Cancer risks for BRCA1 and BRCA2 mutation carriers: results from prospective analysis of EMBRACE. J. Natl. Cancer Inst. 105, 812–822. doi: 10.1093/jnci/djt095
Miki, Y., Swensen, J., Shattuck-Eidens, D., Futreal, P. A., Harshman, K., Tavtigian, S., et al. (1994). A strong candidate for the breast and ovarian cancer susceptibility gene BRCA1. Science 266, 66–71.
Moles-Fernández, A., Duran-Lozano, L., Montalban, G., Bonache, S., López-Perolio, I., Menéndez, M., et al. (2018). Computational tools for splicing defect prediction in breast/ovarian cancer genes: how efficient are they at predicting rna alterations? Front. Genet. 9:366. doi: 10.3389/fgene.2018.00366
Montalban, G., Fraile-Bethencourt, E., López-Perolio, I., Pérez-Segura, P., Infante, M., Durán, M., et al. (2018). Characterization of spliceogenic variants located in regions linked to high levels of alternative splicing: BRCA2 c.7976+5G > T as a case study. Hum. Mutat. 39, 1155–1160. doi: 10.1002/humu.23583
Nielsen, F. C., van Overeem Hansen, T., and Sørensen, C. S. (2016). Hereditary breast and ovarian cancer: new genes in confined pathways. Nat. Rev. Cancer 16, 599–612. doi: 10.1038/nrc.2016.72
Petrucelli, N., Daly, M. B., and Feldman, G. L. (2013). “BRCA1 and BRCA2 hereditary breast and ovarian cancer”. GeneReviews®[Internet] eds MP., Adam, H. H., Ardinger Seattle, WA: University of Washington, Seattle 1–11.
Radice, P., De, S. S., Caleca, L., and Tommasi, S. (2011). Unclassified variants in BRCA genes: guidelines for interpretation. Ann.Oncol. 22(Suppl. 1), i18–i23. doi: 10.1093/annonc/mdq661
Raghunandan, M., Chaudhury, I., Kelich, S. L., Hanenberg, H., and Sobeck, A. (2015). FANCD2. FANCJ and BRCA2 cooperate to promote replication fork recovery independently of the Fanconi Anemia core complex. Cell Cycle 14, 342–353. doi: 10.4161/15384101.2014.987614
Rebbeck, T. R., Friebel, T. M., Friedman, E., Hamann, U., Huo, D., Kwong, A., et al. (2018). Mutational spectrum in a worldwide study of 29,700 families with BRCA1 or BRCA2 mutations. Hum. Mutat. 39, 593–620. doi: 10.1002/humu.23406
Reese, M. G., Eeckman, F. H., Kulp, D., and Haussler, D. (1997). Improved splice site detection in Genie. J. Comput. Biol. 4, 311–323. doi: 10.1089/cmb.1997.4.311
Ricks, T. K., Chiu, H.-J., Ison, G., Kim, G., McKee, A. E., Kluetz, P., et al. (2015). Successes and challenges of PARP inhibitors in cancer therapy. Front. Oncol. 5:222. doi: 10.3389/fonc.2015.00222
Sharma, N., Sosnay, P. R., Ramalho, A. S., Douville, C., Franca, A., Gottschalk, L. B., et al. (2014). Experimental assessment of splicing variants using expression minigenes and comparison with in silico predictions. Hum. Mutat. 35,1249–1259. doi: 10.1002/humu.22624
Slavin, T. P., Maxwell, K. N., Lilyquist, J., Vijai, J., Neuhausen, S. L., Hart, S. N., et al. (2017). The contribution of pathogenic variants in breast cancer susceptibility genes to familial breast cancer risk. NPJ Breast Cancer 3, 22. doi: 10.1038/s41523-017-0024-8
Slavin, T. P., Niell-Swiller, M., Solomon, I., Nehoray, B., Rybak, C., Blazer, K. R., et al. (2015). Clinical application of multigene panels: challenges of next-generation counseling and cancer risk management. Front. Oncol. 5:208. doi: 10.3389/fonc.2015.00208
Spurdle, A. B., Couch, F. J., Hogervorst, F. B. L., Radice, P., and Sinilnikova, O. M. (2008). Prediction and assessment of splicing alterations: implications for clinical testing. Hum. Mutat. 29, 1304–1313. doi: 10.1002/humu.20901
Spurdle, A. B., Healey, S., Devereau, A., Hogervorst, F. B. L., Monteiro, A. N. A., Nathanson, K. L., et al. (2012). ENIGMA-evidence-based network for the interpretation of germline mutant alleles: an international initiative to evaluate risk and clinical significance associated with sequence variation in BRCA1 and BRCA2 genes. Hum. Mutat. 33, 2–7. doi: 10.1002/humu.21628
Stratton, M. R., and Rahman, N. (2008). The emerging landscape of breast cancer susceptibility. Nat. Genet. 40, 17–22.
Vallée, M. P., Di Sera, T. L., Nix, D. A., Paquette, A. M., Parsons, M. T., Bell, R., et al. (2016). Adding in silico assessment of potential splice aberration to the integrated evaluation of brca gene unclassified variants. Hum. Mutat. 37, 627–639. doi: 10.1002/humu.22973
Villate, O., Ibarluzea, N., Fraile-Bethencourt, E., Valenzuela, A., Velasco, E. A., Grozeva, D., et al. (2018). Functional analyses of a novel splice variant in the CHD7 gene. found by next generation sequencing, confirm its pathogenicity in a spanish patient and diagnose him with CHARGE syndrome. Front. Genet. 9, 26–31. doi: 10.3389/fgene.2018.00007
Vreeswijk, M. P. G., Kraan, J. N., van der Klift, H. M., Vink, G. R., Cornelisse, C. J., Wijnen, J. T., et al. (2009). Intronic variants in BRCA1 and BRCA2 that affect RNA splicing can be reliably selected by splice-site prediction programs. Hum. Mutat. 30, 107–114. doi: 10.1002/humu.20811
Wang, G.-S., and Cooper, T. A. (2007). Splicing in disease: disruption of the splicing code and the decoding machinery. Nat. Rev. Genet. 8, 749–761. doi: 10.1038/nrg2164
Wooster, R., Bignell, G., Lancaster, J., Swift, S., Seal, S., Mangion, J., et al. (1995). Identification of the breast cancer susceptibility gene BRCA2. Nature 378, 789–792. doi: 10.1038/378789a0
Yeo, G., and Burge, C. B. (2004). Maximum entropy modeling of short sequence motifs with applications to RNA splicing signals. J. Comput. Biol. 11, 377–394. doi: 10.1089/1066527041410418
Keywords: breast cancer, BRCA2, DNA variants, splicing, hybrid minigenes
Citation: Fraile-Bethencourt E, Valenzuela-Palomo A, Díez-Gómez B, Caloca MJ, Gómez-Barrero S and Velasco EA (2019) Minigene Splicing Assays Identify 12 Spliceogenic Variants of BRCA2 Exons 14 and 15. Front. Genet. 10:503. doi: 10.3389/fgene.2019.00503
Received: 05 February 2019; Accepted: 07 May 2019;
Published: 28 May 2019.
Edited by:
Naoyuki Kataoka, The University of Tokyo, JapanReviewed by:
Logan Walker, University of Otago, New ZealandKatarzyna Gaweda-Walerych, Mossakowski Medical Research Centre (PAN), Poland
Mads Thomassen, Odense University Hospital, Denmark
Copyright © 2019 Fraile-Bethencourt, Valenzuela-Palomo, Díez-Gómez, Caloca, Gómez-Barrero and Velasco. This is an open-access article distributed under the terms of the Creative Commons Attribution License (CC BY). The use, distribution or reproduction in other forums is permitted, provided the original author(s) and the copyright owner(s) are credited and that the original publication in this journal is cited, in accordance with accepted academic practice. No use, distribution or reproduction is permitted which does not comply with these terms.
*Correspondence: Eladio A. Velasco, eavelsam@ibgm.uva.es