- 1College of Life Sciences, Fujian Agriculture and Forestry University, Fuzhou, China
- 2Institute of Tobacco Science, Fujian Provincial Tobacco Company, Fuzhou, China
- 3Department of Biological and Forensic Sciences, Fayetteville State University, Fayetteville, NC, United States
- 4Fujian Key Laboratory of Crop Breeding by Design, Fujian Agriculture and Forestry University, Fuzhou, China
The Golden2-like (GLK) transcription factors play important roles in regulating chloroplast growth, development, and senescence in plants. In this study, a total of 89 NtGLK genes (NtGLK1–NtGLK89) were identified in the tobacco genome and were classified into 10 subfamilies with variable numbers of exons and similar structural organizations based on the gene structure and protein motif analyses. Twelve segmental duplication pairs of NtGLK genes were identified in the genome. These NtGLK genes contain two conserved helix regions related to the HLH structure, and the sequences of the first helix region are less conserved than that of the second helix motif. Cis-regulatory elements of the NtGLK promoters were widely involved in light responsiveness, hormone treatment, and physiological stress. Moreover, a total of 206 GLK genes from tomato, tobacco, maize, rice, and Arabidopsis were retrieved and clustered into eight subgroups. Our gene expression analysis indicated that NtGLK genes showed differential expression patterns in tobacco leaves at five senescence stages. The expression levels of six NtGLK genes in group C were reduced, coinciding precisely with the increment of the degree of senescence, which might be associated with the function of leaf senescence of tobacco. Our results have revealed valuable information for further functional characterization of the GLK gene family in tobacco.
Introduction
The GOLDEN2-LIKE (GLK) proteins are included in the GARP (Golden2, ARR-B and Psr1) domain superfamily of transcription factors (TFs) (Riechmann et al., 2000; Xiao et al., 2019). The GLK transcription factor was originally identified in the C4 plant of maize (Zea mays L.) (Hall et al., 1998). A typical GLK protein usually contains two conserved domains, namely, a Myb-DNA binding domain (DBD) and a C-terminal (GCT) box (Rossini et al., 2001).
Members of the GLK family play important roles in the formation and development of chloroplasts (Rossini et al., 2001; Fitter et al., 2002; Waters et al., 2008; Powell et al., 2012; Jarvis and López-Juez, 2013) and have been involved in the various defense processes of organisms, including biotic and abiotic stresses (Savitch et al., 2007; Schreiber et al., 2011; Murmu et al., 2014; Han et al., 2016; Nagatoshi et al., 2016). In Arabidopsis (Arabidopsis thaliana), the AtGLK1 and AtGLK2 genes act redundantly to regulate chloroplast development (Fitter et al., 2002; Yasumura et al., 2005). AtGLK1 overexpression enhances resistance to the pathogen Fusarium graminearum (Savitch et al., 2007; Schreiber et al., 2011) and increases susceptibility to the virulent oomycete pathogen Hyaloperonospora arabidopsidis (Hpa) (Murmu et al., 2014), whereas the glk1 glk2 double mutant increases the resistance to the Hpa gene compared to that of the wild type (Murmu et al., 2014). In addition, it has been reported that GLKs can interact with ANAC092 (ORE-1) to regulate leaf senescence (Rauf et al., 2013). In tomato (Solanum lycopersicum), the overexpression of GLK increases the expressions of genes related to chloroplast development and fruit photosynthesis, and these changes result in the enhancement of the carbohydrate and carotenoid levels in ripe fruits (Powell et al., 2012). In maize, ZmGlk1 has been considered to play important roles in the chloroplast development of mesophyll cell in C4 plant tissues (Rossini et al., 2001; Fitter et al., 2002).
The senescence of tobacco (Nicotiana tabacum) leaves is a positive and orderly process involving the transformation and mobilization of nutrients. As a model plant, the study of the plant senescence and internal material transport rules in tobacco has special significance in the research of plant physiology and development (Gregersen et al., 2013). During the phase of leaf senescence, leaf cells undergo orderly changes in structure, metabolism, and gene expression, along with a series of degradations, including the chlorophyll content depletion and diminishing photosynthetic capacity (Lira et al., 2017; Schippers et al., 2015; Gan and Amasino, 1997; Lim et al., 2003). Normally, leaf color change is the most intuitive phenomenon, which is due to the degradation of chlorophyll in chloroplasts (Hörtensteiner, 2006; Kräutler, 2016); therefore, the degree of etiolation has usually been used as an important criterion to assess the senescence of leaves. Because of the importance of the members of the GLK gene family for the development of chloroplasts (Fitter et al., 2002; Waters et al., 2008; Powell et al., 2012) and its association with leaf senescence (Rauf et al., 2013), it is important to investigate this gene family and assess the relationship between gene expression and senescence in leaf.
The GLK gene family has been identified and characterized in several plant species, including maize (Zea mays L.) (Liu et al., 2016) and tomato (Liu, 2018). However, the GLK gene family has not been thoroughly examined in tobacco, to the best of our knowledge. A comprehensive investigation of all the GLK genes with current tobacco genome sequence data, including the family members and the detailed organization of the gene sequences in tobacco, should be conducted. The objectives of this study were to analyze the GLK gene family including the GLK gene structure, chromosomal localization, and phylogenetic relationship in the tobacco genome and to reveal the expression regulation of the GLK gene family members at different senescence stages of tobacco leaves. The information derived from this study will be useful for further functional exploration on the GLK gene family in tobacco.
Materials and Methods
Identification of GLK Genes in Tobacco
The published GLK protein sequences of maize (Liu et al., 2016), rice (Oryza sativa) (Rossini et al., 2001), and A. thaliana (Fitter et al., 2002) were used as query sequences to identify the GLK proteins in tobacco using the BLASTP tool and the tobacco genome sequences (Edwards et al., 2017) in the Sol Genomics Network database1. More than 30% similarity and an E value less than E––10 were set as the parameters to define the tobacco candidate GLK proteins. The domains of all the candidate GLK proteins of tobacco were checked using the Conserved Domain Database (CDD) tool2 (Lu et al., 2020). Finally, the sequences with complete GLK domains were retained and were renamed (NtGLK). Detailed information of the NtGLK genes, including the gene IDs, physical position, sequences of the genes and proteins, and the coding sequences (CDS), were retrieved from the Sol Genomics Network database3. The features of the NtGLK proteins were calculated using online ExPASy programs4 (Bjellqvist et al., 1993; Bjellqvist et al., 1994; Wilkins et al., 1999).
Multiple Sequence Alignment and Phylogenetic Analysis
The amino acid sequences of all the tobacco GLK proteins were aligned using the ClustalX1.83 tool (Thompson et al., 1997). The alignment of the NtGLK protein conserved domain sequences was exhibited by DNAMAN5 (Altschul et al., 1990). A phylogenetic tree with 1,000 bootstrap replicates was generated using the neighbor-joining method of the MEGAX software (Kumar et al., 2018). The classifications of tobacco GLK proteins were determined according to the topology and bootstrap values of the phylogenetic tree.
Chromosomal Location and Gene Duplication
Information on the physical position image of the NtGLK genes was obtained based on the MapInspect tool6. To investigate gene duplication, the criteria for the proportion of overlap and the similarity between the two sequences were set to be >70% (Gu et al., 2002; Yang et al., 2008). Segmental duplication and tandem duplication were defined based on the method reported by Wang et al. (2010).
Gene Structure and Conserved Motif Identification
The gene structures of the NtGLK genes were identified by the GSDS7 platform using the complete sequence of the genomic sequence and CDS for NtGLK downloaded from the tobacco genome (Hu et al., 2015). The conserved motifs of the NtGLK proteins were analyzed by the MEME program8 (Ma et al., 2014), and the optimum motif width and the maximum number of motifs were set to 5–100 and 20 residues, respectively, with the remaining parameters in default. Motif annotation was identified using the CDD tools. About 1,500 bp of DNA sequence upstream of the starting codon of the NtGLK genes were extracted from the tobacco genome to decipher the cis-elements with the online tool PlantCARE9 (Lescot et al., 2002) for cis-element prediction and the TBtools software (Chen et al., 2020) for the visualization.
NtGLK Expression Analysis in Different Senescence Stages of Tobacco Leaves
Nicotiana tabacum cv. Cuibi 1 (CB-1) was used in this study. According to the morphological characteristics of leaf color, vein, and villus (Xu et al., 2017), five senescence stages (M1, M2, M3, M4, and M5) of the middle leaves (eighth to 10th, counted from the bottom to the top) were collected in the same field as samples. Three biological replicates with each replicate containing three leaves from different plant were collected. Total RNA was extracted using a total RNA isolation kit (PR2401, Bioteke Corporation, China). A total of 15 RNA samples were sequenced on Illumina HiSeq 2000 performed by BioMarker Technologies10 (BioMarker, Beijing, China). The gene expression level was assessed according to the FPKM (fragments per kilobase of transcript sequence per million base pairs sequenced) value (Trapnell et al., 2010). The complementary DNA (cDNA) samples were synthesized by a SMART cDNA synthesis kit, and quantitative reverse transcriptase (qRT-PCR) reactions were conducted with the SYBR Premix Ex Taq based on the manufacturer’s instruction (Takara). The primers used for qRT-PCR analysis are listed in Supplementary Table 1. The specific exon regions of the target genes were used for primer design. The primer pair of each target gene was also prescreened to ensure the uniqueness of the amplification product. Three biological replicates and three technical replicates were performed. The expression level of each selected NtGLK gene in the M1 stage was used as the control, while the relative expression level of the NtGLK genes in the different senescence stages was calculated using the 2−ΔΔCt method (Livak and Schmittgen, 2001). A t test was conducted to assess the expression differences from the M1 stage to the M2, M3, M4, and M5 stages. Significant difference was set as p < 0.05 or p < 0.01.
Results
Characterization of GLK Genes in Tobacco
A BLASTP search was performed using 117 known GLK protein sequences as the query sequences against the tobacco genome database to analyze the GLK genes in tobacco, including 59 from maize (Liu et al., 2016), 54 from tomato (Liu et al., 2016), two from rice (Rossini et al., 2001), and two from A. thaliana (Fitter et al., 2002). A total of 89 GLK genes were identified in tobacco, and these genes were named NtGLK1 through NtGLK89. Detailed information of these genes and their corresponding proteins are listed in Table 1, including the gene ID, gene location, number of exons, protein length (amino acids, aa), molecular mass (MS), and pI. The NtGLK genes showed a wide range of amino acid sequence lengths and molecular weights. The amino acid residues of NtGLK proteins were oscillating from 114 aa (NtGLK31) to 779 aa (NtGLK41), with an average of 408 amino acids, whereas the molecular mass was from 13,358.46 Da (NtGLK31) to 85,665.25 Da (NtGLK41) and the theoretical isoelectric points (pI) range from 4.81 (NtGLK49) to 9.91 (NtGLK31).
Structure and Phylogenetic Tree of Tobacco GLK Gene Members
To explore the evolutionary relationships among the tobacco GLK genes, an unrooted phylogenetic tree was generated using the 89 tobacco GLK protein sequences (Figure 1A); moreover, the gene structures for each GLK gene were analyzed (Figure 1B). According to the results of the gene structure and the bootstrap values (>50%) of the phylogenetic tree, the tobacco GLK gene family was grouped into 10 subfamilies (I to X). However, there were two genes (NtGLK82 and NtGLK88) that could not be clustered into any of the 10 subfamilies because of their low bootstrap values (<50%). Among these subfamilies, subfamily I (containing 30 members) was the largest group and represented more than 30% of the total NtGLK members. In contrast, subfamilies III and VIII only contained two members each.
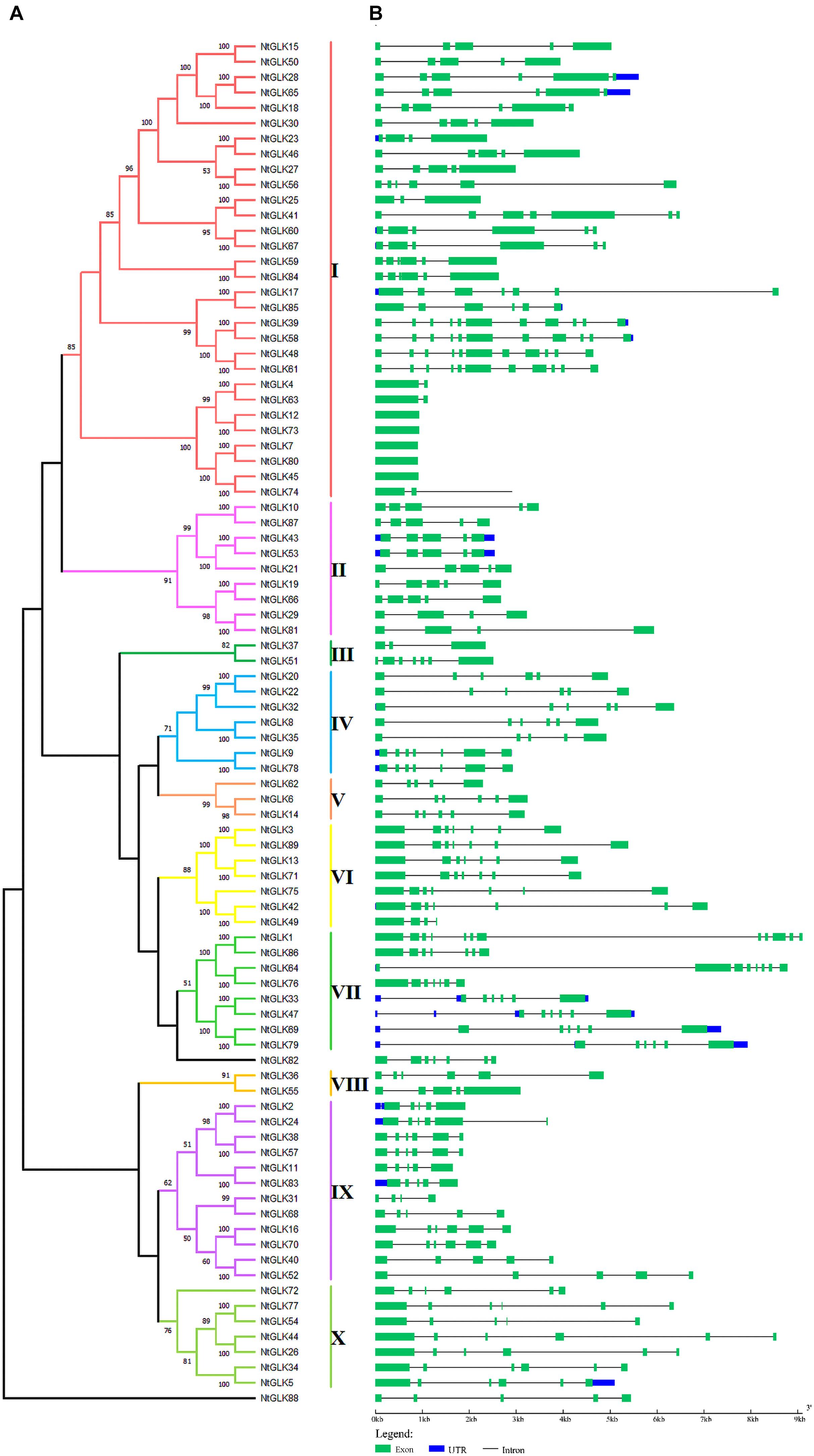
Figure 1. Phylogenetic tree and gene structures of the NtGLK gene family. (A) A neighbor-joining (NJ) phylogenetic tree was generated by MEGAX based on the NtGLK protein sequences. The different subfamilies are distinguished by different colors. (B) The exon–intron structures for the NtGLK genes were obtained using the online software GSDS. The horizontal black lines and the green boxes represent introns and exon, respectively, and the lengths of the exons and introns can be estimated using the scale.
Structure analysis of the NtGLK genes (Figure 1B) showed that the number of introns in the subfamilies ranged from 0 to 11. Among them, five genes (NtGLK12, NtGLK73, NtGLK7, NtGLK80, and NtGLK45) clustered into subfamily I, which did not contain any intron, and two genes (NtGLK4 and NtGLK63) contained only one intron. Most of the NtGLK genes that were clustered into the same phylogenetic groups showed similar exon/intron structures, including the intron numbers and exon length. Variations in the intron number might be one of the key factors that resulted in the diversity of the gene structure and function in the course of evolution.
Motif Analysis of NtGLK Proteins
The conserved motifs of the 89 NtGLK proteins within each subfamily were identified and analyzed using the online MEME tool (Figure 2). A total of 20 motifs were identified; the detailed conserved sequences of each motif are shown in Supplementary Table 2. With the CDD tool, seven putative motifs were functionally annotated, which were defined as Myb-SHAQKYF for motif 1, components of the conserved GLK domain for motifs 2 and 11, Myb-CC-LHEQLE for motif 3, and REC superfamily for motifs 4, 5, and 8. However, no functional annotation was assigned for the remaining 13 putative motifs. The motif of Myb-SHAQKYF is highly conserved in a number of myb-related genes. It was reported that the conserved motif of SHAQKYF could bind to the I-box with a DNA-binding domain located in the carboxy terminal domain and acted as a transcriptional activator in yeast and plants (Rose et al., 2000). The motif of Myb-CC-LHEQLE was found toward the C-terminus of Myb-CC-type transcription factors, the member of the protein family reported to be involved in phosphate starvation signaling both in vascular plants and in unicellular algae (Rubio et al., 2001). The functions of the REC domains are annotated as phosphorylation-mediated switches within response regulators (RRs), and some also transfer phosphoryl groups in multistep phosphorelays.
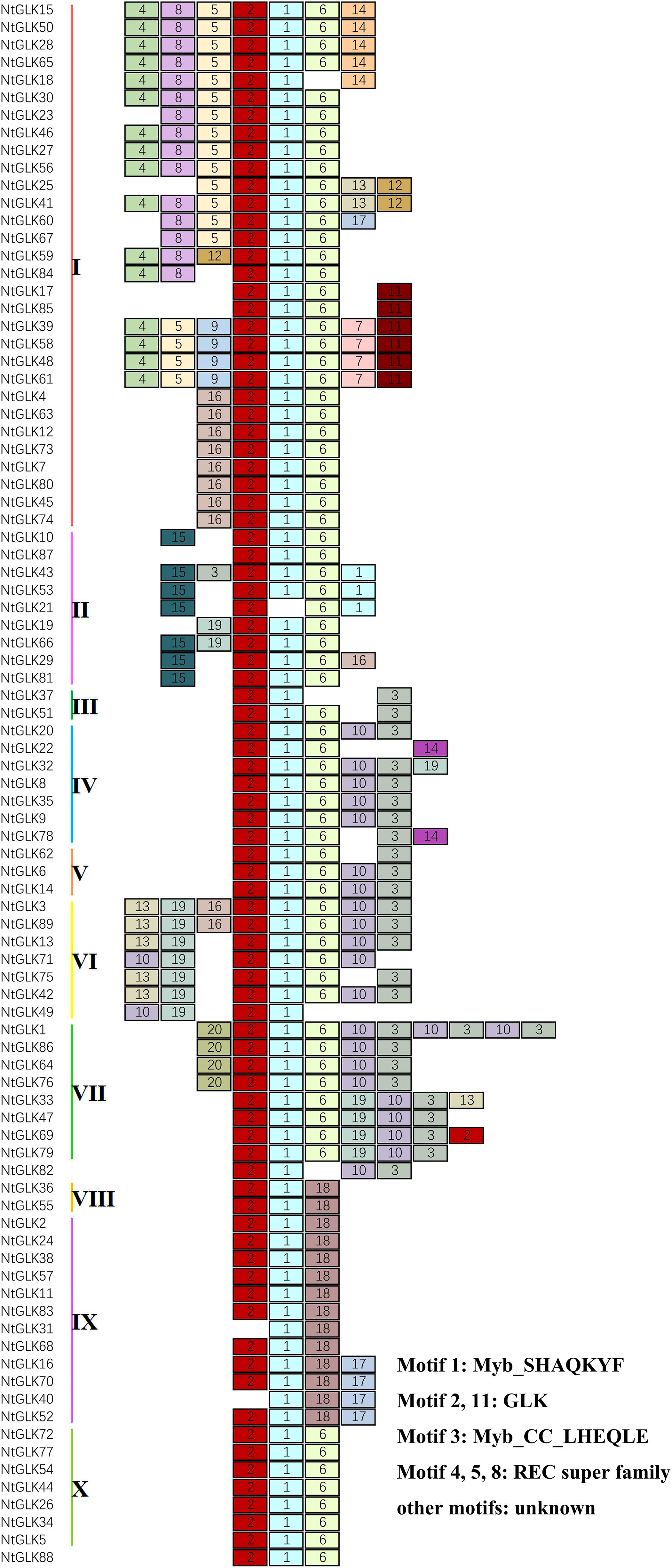
Figure 2. Schematic diagram of the conserved motifs for NtGLK proteins (1–20). The motifs were ordered manually based on the results of the MEME analysis. The annotation information for each motif is shown on the right.
The majority of the NtGLK proteins contained motifs 1 and 2 (Figure 2). In addition, the NtGLK protein members grouped into the same subfamily contained similar motif components and spatial distributions. Moreover, most of the members of groups III, IV, V, VI, and VII possessed a Myb-CC-LHEQLE domain (motif 3: a type of Myb-like domain), which appeared to respond to various abiotic stresses and played diverse roles in plant development (Murmu et al., 2014). This result suggested that the NtGLK proteins in the same subfamily might have similar functions. In addition, the specificity within a subfamily was also identified. For instance, motif 15 is only possessed by subfamily II and motif 16 only appeared in subfamily I (Figure 2). To further decipher the similarity among the tobacco GLK domains, 89 tobacco GLK domain sequences were aligned using the DNAMAN 811 platform (Figure 3). Our results showed that the GLKs contained two regions of a putative DNA-binding domain with an HLH structure, which was also identified in maize (Liu et al., 2016). In this putative domain, the first helix had initial sequences of PELHRR and the second helix contained the conserved NI/VASHLQ. In addition, although the sequences had particularly conserved L and H, some variants were found for the GLK gene members of tobacco in this motif. The second helix region contained a highly conserved sequence of VK/VASHLQ (Liu et al., 2016), which was also similar to the GLK members in tomato (Liu, 2018).
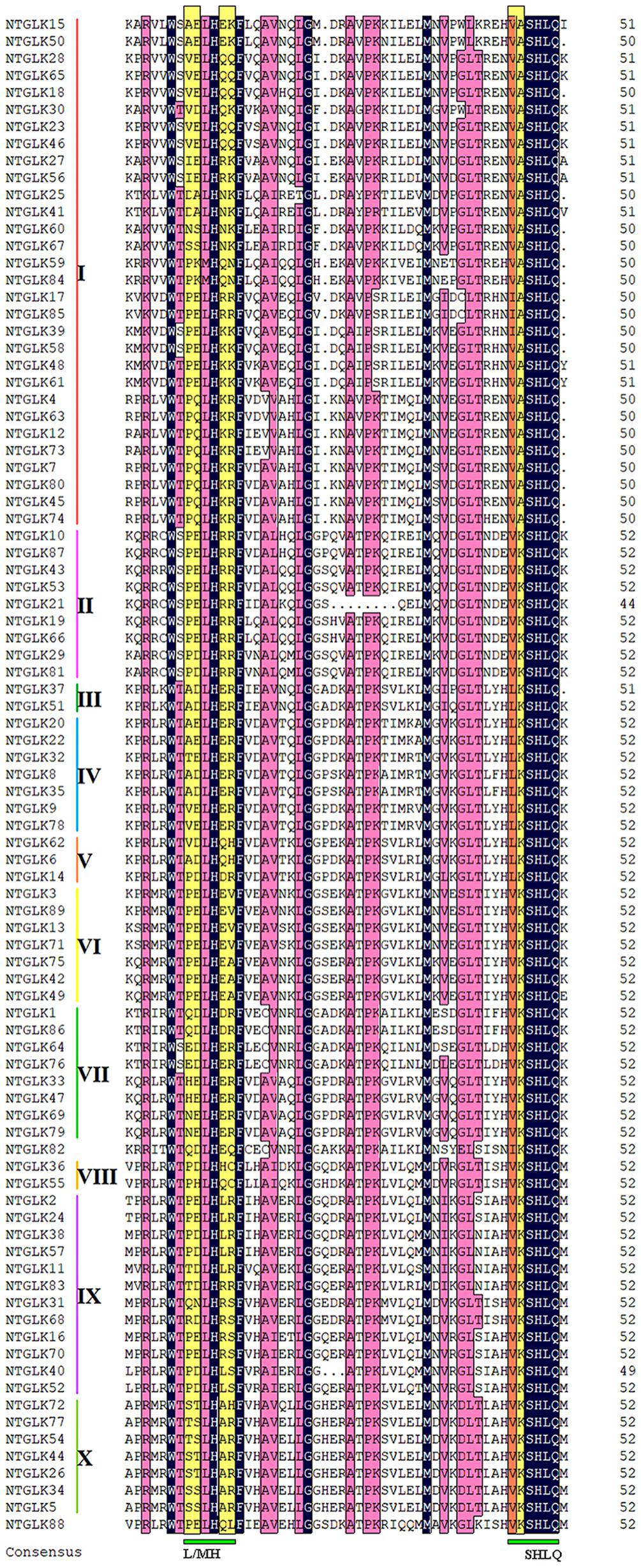
Figure 3. Multiple sequence alignment of the tobacco GLK conserved domain. Identical residues are shaded in black and similar residues in pink.
Analysis of Cis-Regulatory Elements in the Promoter Regions of NtGLK Genes
Cis-acting elements in the upstream region of the NtGLK genes are very important in regulating gene expression in response to various stresses as well as during different developmental stages. To explore the possible expression patterns of the NtGLK genes under various stress conditions and senescence processes, cis-acting elements including components related to stress, light response, and hormone response were investigated in the promoter regions. Various potential cis-acting elements were identified in NtGLK promoter regions (Figure 4 and Supplementary Table 3). Among them, the most abundant cis-acting elements were light-responsive elements, including GT1-motif, ACE, G-box, ATCT-motif, Box 4, TCT-motif, chs-CMA1a, GATA-motif, I-box, chs-CMA2a, GA-motif, AE-box, MBS, MRE, and TCCC-motif; Box 4 and G-box appear to be the most abundant light-responsive elements, being distributed in the promoter regions of 65 and 56 NtGLK genes, respectively. In terms of the hormone response-related cis-acting elements, a total of nine types of elements were identified, namely, TGA-element, AuxRR-core, TCA-element, ABRE, CGTCA-motif, TGACG-motif, P-box, GARE-motif, and TATC-box; among them, ABRE was the most abundant cis-acting hormone-responsive element in the promoter regions of 89 NtGLK genes, which was involved in abscisic acid (ABA) responsiveness. This abundance of hormone-responsive elements indicated that NtGLK genes appeared to play important roles in tobacco hormone signal transduction and senescence. In addition, a total of two kinds of cis-acting elements involved in various stresses were found, including long terminal repeat (LTR) in low-temperature responsiveness and TC-rich repeats in defense and stress responsiveness (Supplementary Table 3). Notably, many cis-elements have two or more copies in the 1.5-kb upstream region within the same NtGLK gene, which appear to enhance their binding effects to their corresponding trans-acting factors. Furthermore, the NtGLK genes in the same phylogenetic clade only showed moderate consistency in their distributions of the cis-elements, reflecting the complex evolutionary relationship of the diverged NtGLK genes, especially in promoter regions.
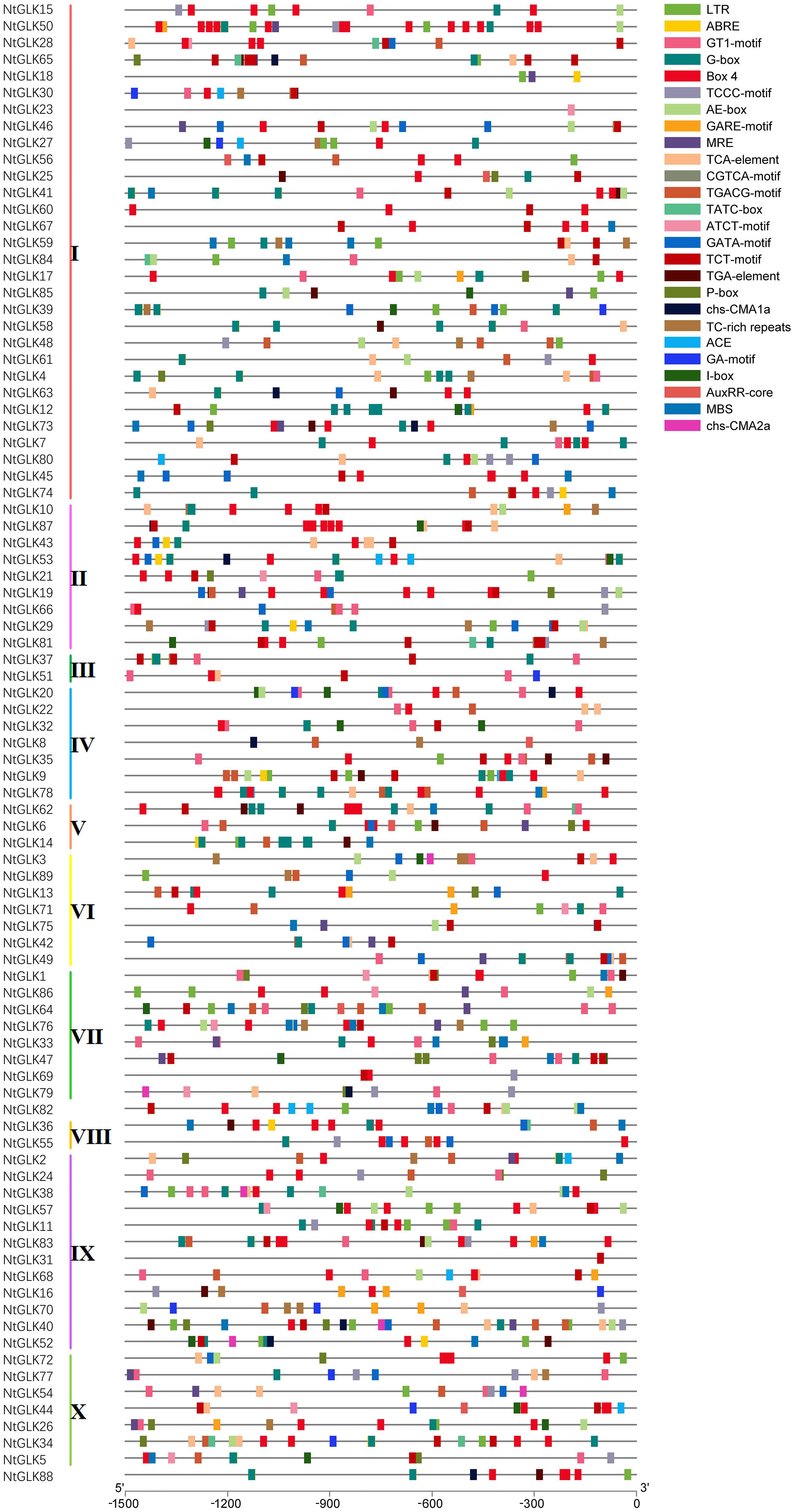
Figure 4. Promoter cis-element analysis of NtGLK genes. The different types of cis-elements are represented by different shapes and colors.
Chromosomal Locations and Duplications of NtGLK Genes
Except for chromosomes 1 and 10, 40 out of the 89 NtGLK genes were obtained and were assigned to the 22 tobacco chromosomes (Figure 5). Chromosome 4 contained the largest number of NtGLK genes (five), and chromosomes 3, 6, 9, 11, 12, 14, 15, 16, 18, 19, and 21 possessed only one NtGLK gene each. A similar uneven distribution pattern of the GLK gene family was also found in maize (Liu et al., 2016). In addition, the potential duplication events were investigated to explore the potential mechanism for the NtGLK gene family. A total of 12 duplicated pairs of NtGLK genes were identified as segmental duplication gene pairs (Figure 5 and Supplementary Table 4). However, no pairs of tandem duplicated genes were observed in this study. A biased distribution pattern was also found among the 12 segmental duplication pairs, and no pairs were distributed on chromosomes 1, 3, 6, 10, 12, 14, 16, 17, 19, 21, and 22. These results infer that segmental duplication events may play important roles in the amplification of the tobacco GLK gene family.
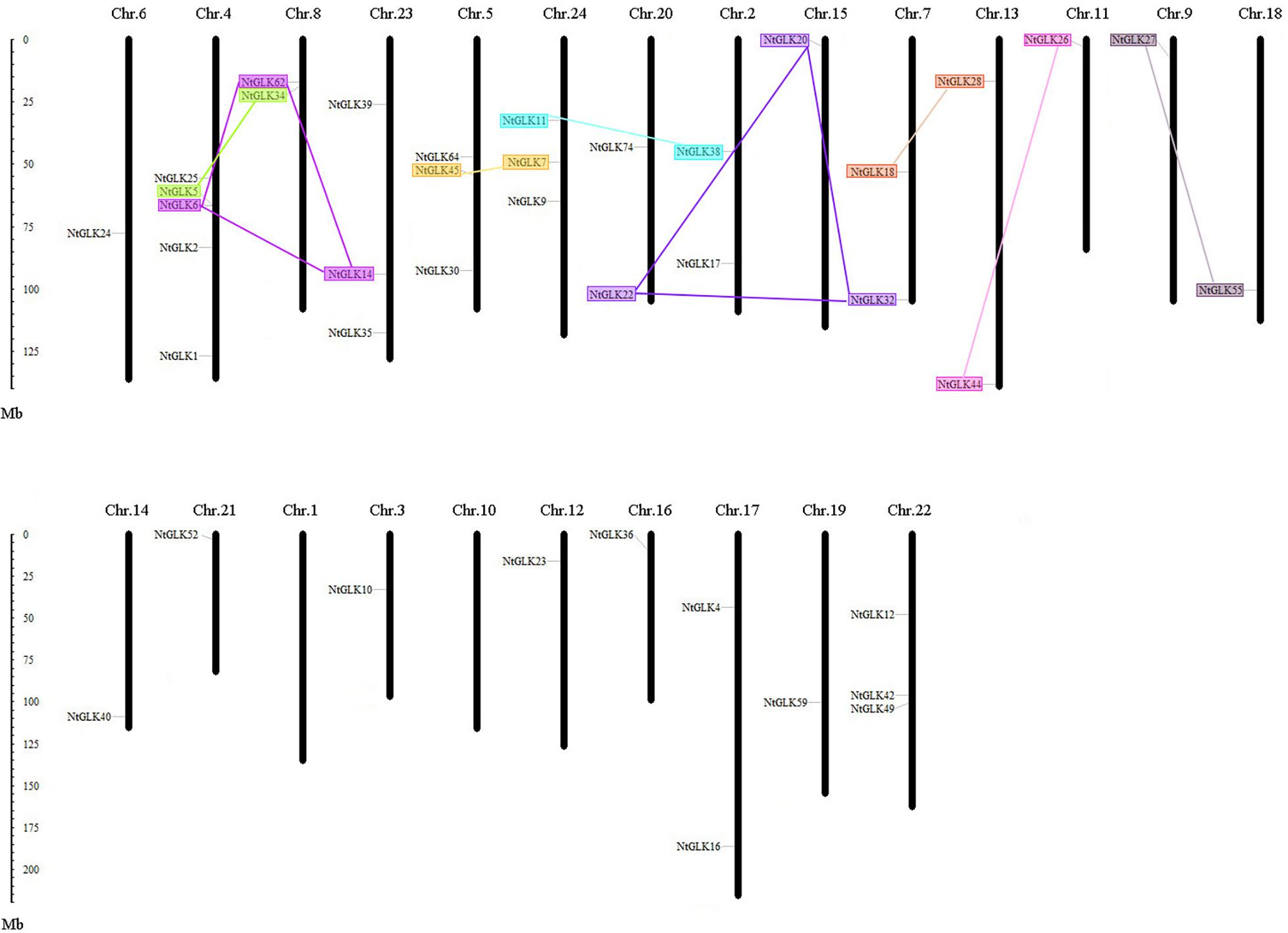
Figure 5. Chromosomal locations of tobacco GLK genes. Segmented duplicated gene pairs are displayed as color boxes connected by lines.
Phylogenetic Analysis of the NtGLK Gene Family
To analyze the evolutionary process of the tobacco GLK gene family, the 89 NtGLK protein sequences were aligned with 59, 54, two, and two GLK proteins from maize (Liu et al., 2016), tomato (Liu et al., 2016), rice (Rossini et al., 2001), and Arabidopsis (Fitter et al., 2002), respectively. In total, 206 GLK proteins were clustered into eight groups (A to H; Figure 6). Group E was the largest subfamily, which contained 61 proteins, including 22 from tobacco, 18 from tomato, and 21 from maize. Groups B (40) and H (41) also had large numbers of GLK members, and these three groups represented 68.9% of the total NtGLK proteins. In contrast, group F was the smallest clade, which had only two GLK gene members. Notably, group C contained 14 GLK members, and among them, two genes (At5G44190.1 and At2G20570.2) were confirmed to be involved in leaf senescence in Arabidopsis (Rauf et al., 2013). It appears that the members clustered into group C, including six members from tobacco (NtGLK85, NtGLK17, NtGLK61, NtGLK48, NtGLK58, and NtGLK39) may share similar functions. In addition, the GLK members from tobacco, tomato, and maize were distributed into the major subfamilies, suggesting that the GLK gene family existed before the separation of monocotyledons and dicotyledons.
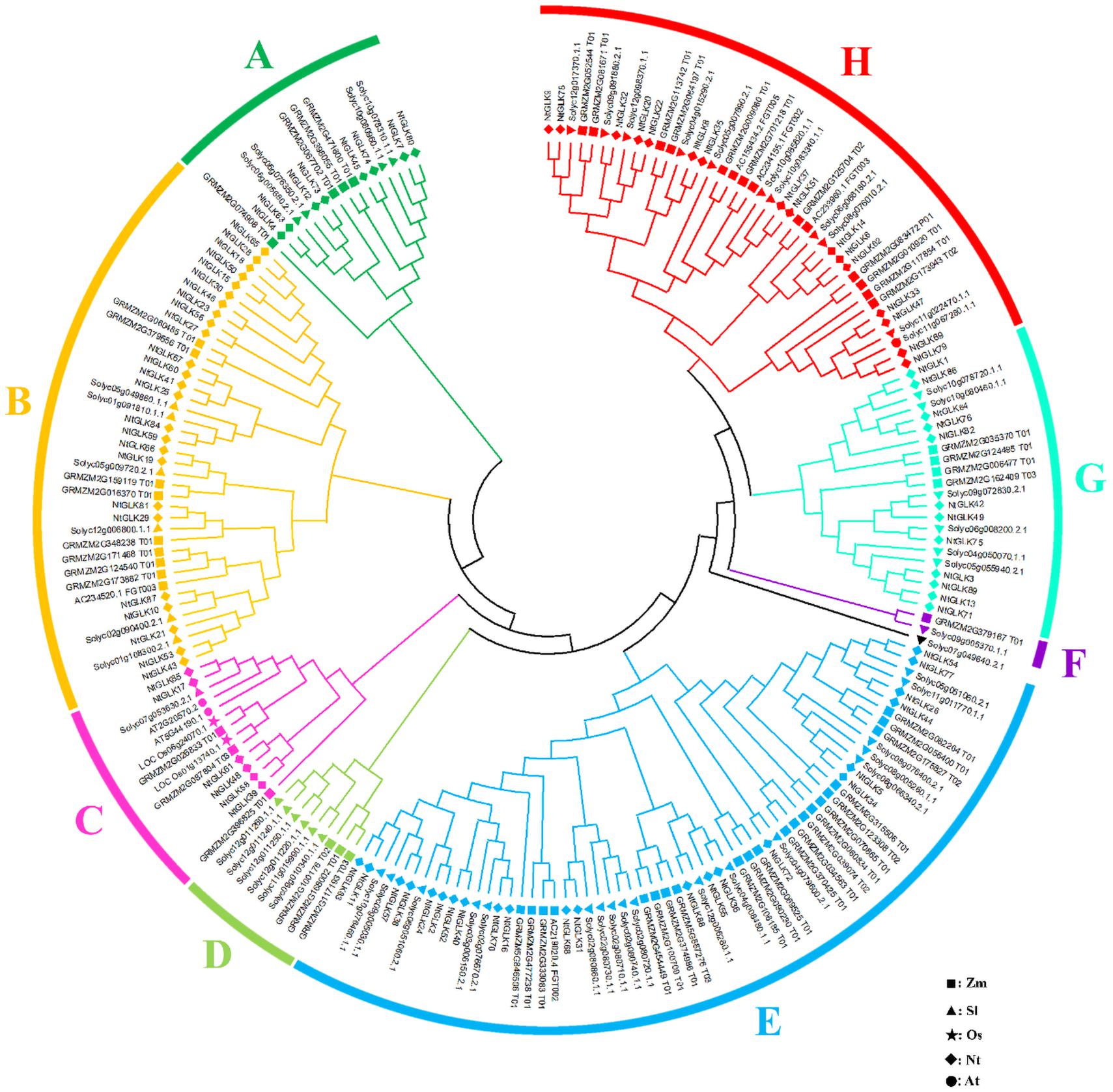
Figure 6. Phylogenetic analysis of tobacco, maize, rice, Arabidopsis, and tomato GLK proteins. Different GLK subfamilies are shown as different colors. The square, triangle, star, diamond, and circle represent maize, tomato, rice, tobacco, and Arabidopsis GLK proteins, respectively.
Expression Patterns of NtGLK Genes in Different Senescence Stages of Tobacco Leaves
Tobacco leaves at the M1, M2, M3, M4, and M5 senescence stages were collected, which had yellowing rates of 50, 65, 75, 85, and 100%, respectively (Figure 7). To explore the potential functions of the NtGLK genes, an RNA sequencing (RNA-Seq) experiment was performed for tobacco leaves in the five senescence stages (M1–M5), and the FPKM values of the NtGLK genes derived from the RNA-Seq data were used to evaluate the expression levels of these NtGLK genes in the different senescence stages. Among them, 25 NtGLK genes were excluded from further heat map analysis because of low expression (FPKM < 0.5) or lack of expression in all the senescence stages. The NtGLK genes showed differential expressions in tobacco leaves at the different senescence stages (Figure 8). A total of 64 NtGLK genes were clustered into three groups (Figure 8). Among them, 20 genes (NtGLK49, NtGLK33, NtGLK78, NtGLK69, NtGLK42, NtGLK9, NtGLK35, NtGLK8, NtGLK43, NtGLK81, NtGLK13, NtGLK65, NtGLK47, NtGLK75, NtGLK29, NtGLK46, NtGLK79, NtGLK48, NtGLK89,and NtGLK3) were included in group III (Figure 8), which exhibited high expression levels in all the analyzed stages, hinting that these genes were essential in the five senescence stages of tobacco leaves. However, 23 NtGLK genes (NtGLK54, NtGLK20, NtGLK6, NtGLK32, NtGLK66, NtGLK19, NtGLK77, NtGLK44, NtGLK27, NtGLK36, NtGLK10, NtGLK73, NtGLK88, NtGLK87, NtGLK12, NtGLK67, NtGLK2, NtGLK24, NtGLK30, NtGLK26, NtGLK60, NtGLK15, and NtGLK72) were clustered into group II and showed relatively lower expression levels in all stages. In general, the expression levels of most NtGLK genes exhibited a decreased trend with the increase of senescence level, such as NtGLK89, NtGLK3, NtGLK85, NtGLK17, NtGLK58, etc. However, inverse expression patterns were also found in some NtGLK genes, such as NtGLK9, NtGLK35, NtGLK8, and NtGLK43. It is worth noting that NtGLK85, NtGLK17, and NtGLK58 were all grouped into subfamily I, and NtGLK9, NtGLK35, and NtGLK8 were all clustered into subfamily IV (Figure 1). The results showed that the expression patterns of the NtGLK genes in the different senescence stages were different. The expression patterns of the NtGLK genes provided preliminary information for their further functional exploration.
To validate the RNA-Seq data, 10 NtGLK genes were selected for qRT-PCR analysis (Supplementary Table 1). Among them, variations of the transcript abundances of six genes (NtGLK3, NtGLK19, NtGLK36, NtGLK69, NtGLK72, and NtGLK78) corresponded to the increase of the senescence degrees based on the RNA-Seq data. Another four GLK genes (NtGLK85, NtGLK17, NtGLK58, and NtGLK39) and two Arabidopsis genes (At5G44190.1 and At2G20570.2) were grouped together (group C; Figure 6), and these two Arabidopsis genes were previously confirmed to be involved in leaf senescence (Rauf et al., 2013). The results of qRT-PCR showed that the expression levels of NtGLK3, NtGLK17, NtGLK19, NtGLK39, NtGLK58, NtGLK72, and NtGLK85 were decreased, precisely consistent with the increase of the senescence degrees, while the expressions of NtGLK36, NtGLK69, and NtGLK78 showed a trend of rising first and then decreasing (Figure 9). The expression patterns of these 10 genes detected by qRT-PCR showed similar trends of gene expression pattern to those detected by the RNA-Seq approach (Figure 8), indicating that the RNA-Seq data were reliable.
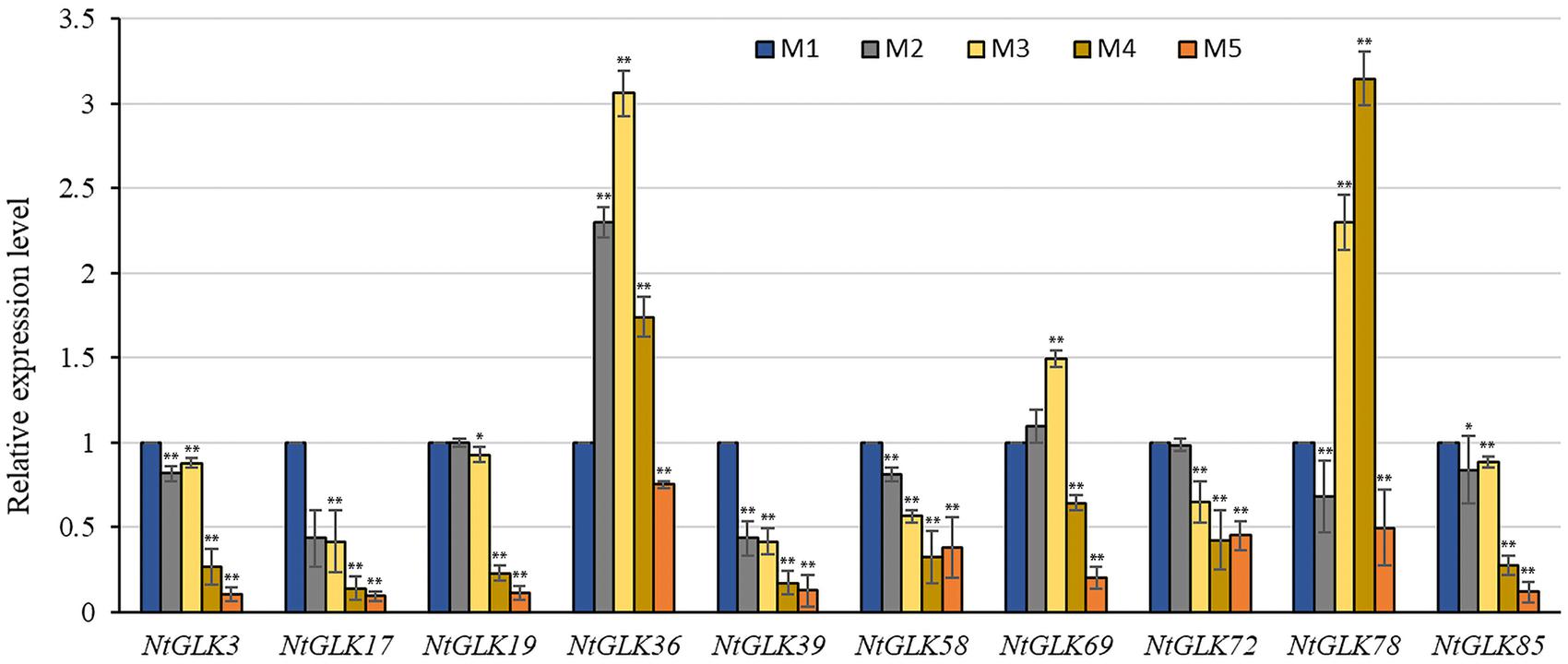
Figure 9. Relative expression levels of 10 NtGLK genes in tobacco leaves at five senescence stages. Error bars indicate standard deviation. Asterisks indicate the significant degree of expression level compared to the value of the control (∗P < 0.05, ∗∗P < 0.01).
Discussion
The function of GLK genes was first recognized from the analysis of a maize mutant with pale green phenotype (Langdale and Kidner, 1994) and acted as a transcription regulatory element (Hall et al., 1998). The GLK gene sequences have only been identified in photosynthetic eukaryotes such as green algae and higher plants, but absent in the genome of the cyanobacterium Synechocystes, suggesting that the function of GLK genes is associated with the development of chloroplasts (Rossini et al., 2001). Members of the GLK gene family are considered as one of the most characterizable genes among the nuclear-encoded genes, which regulate chloroplast biogenesis (Fitter et al., 2002). Chloroplast converts light energy into chemical energy, and it plays important roles in the growth and development of plants (Kirchhoff, 2019). The color of leaves gradually changes during leaf senescence with the chlorophyll broken down and the photosynthetic capacity declined (Lira et al., 2017; Schippers et al., 2015; Gan and Amasino, 1997; Lim et al., 2003); therefore, the leaf senescence of tobacco can be assessed by the degree of leaf etiolation. Two Arabidopsis genes (At5G44190.1 and At2G20570.2) were confirmed to be involved in leaf senescence (Rauf et al., 2013). Generally, genes that belong to the same subfamily share similar functions compared to those from different subfamilies. In our analysis, a total of six tobacco GLK genes (NtGLK85, NtGLK17, NtGLK39, NtGLK48, NtGLK58, and NtGLK61) were clustered with the Arabidopsis GLK genes (At5G44190.1 and At2G20570.2) in group C (Figure 6), suggesting that these genes across different species might share structural and functional similarity. Thus, it has been postulated that these six putative NtGLK genes play roles in the leaf senescence of tobacco. According to the FPKM values generated from the RNA-Seq data, we found that the transcript abundance of the six NtGLK genes in subfamily C decreased, closely corresponding to the increase of leaf senescence level, indicating that these six NtGLK genes might play important roles in the process of tobacco leaf senescence. The harvest maturity of tobacco leaf is closely related to leaf senescence, and it is considered as a fundamental index to measure tobacco quality. To improve the quality of tobacco, it is very important to develop proper parameters for harvest maturity. Hence, the variations of the transcript abundance of these six GLK genes can be further developed as markers to evaluate the maturity of tobacco leaves.
The NtGLK genes are not evenly distributed in the tobacco genome. A similar uneven distribution pattern of the GLK gene family was also identified in maize (Liu et al., 2016). Unlike prokaryotes, it is common that functionally related genes in eukaryotes are distributed throughout genomes. The physical arrangement of genes on chromosomes is largely derived from insertions, deletions, duplications, and inversions. As a regulatory mechanism of gene expression in cells, microRNAs (miRNAs) target specific messenger RNAs (mRNAs) to regulate gene expression through the mechanism of RNA interference (RNAi). It was observed that the miRNA counts were extremely high in certain chromosomes for the 20 different species, suggesting that the higher number of miRNA genes in these chromosomes might be associated with a regulatory role in cellular functions (Atanu and Utpal, 2014). The co-localized and “operon-like” biosynthetic gene clusters have also been identified in eukaryotes with unknown mechanisms related to highly interactive domains for the regulation of co-expression within clusters of genes (Nützmann et al., 2020). Therefore, the cluster of NtGLK may be related to the regulation of genes with leaf senescence.
As the transcription factor, the G2 gene is activated by the N-terminal region of a heterologous system, and the GLK gene family members contain the highly conserved HLH region (DNA-binding domain) and the GCT box (which functions in dimerization) (Rossini et al., 2001). The sequence of the DNA-binding domain belongs to the GARP transcription factor family (Hosoda et al., 2002), and the two regions of the HLH DNA-binding domain are also conserved (Liu et al., 2016). These two conserved regions of HLH structure were also identified in the tobacco genome in this study; however, the conserved sequences were not totally identical in tobacco. Multiple sequence alignment demonstrated that the second helix region of the NtGLK genes was highly conserved (VK/VASHLQ), while a number of variants were found for the NtGLK genes in the first helix, except for the fairly conserved L and H, suggesting that the first helix appeared to be more important in the functional differentiation of the GLK gene family in tobacco. This diversity in function derived from sequence variants was also observed in maize research, where the ZmGLK genes were overall much conserved within the course of evolution (Liu et al., 2016). Actually, the genetic variants derived from the single nucleotide polymorphisms (SNPs) were responses for the gene function of cultivar adatation in tropical and temperate lines, which are linked to resistance to cold and drought stresses (Liu et al., 2016). In our study, the number of variants in each subfamily seemed different among the 10 subfamilies of the GLK gene family in tobacco (Supplementary Table 5), and subfamilies I, IV, VII, and IX showed more sequence variants. The results suggest that sequence diversity was the important factor leading to the more diverse functions in subfamilies I, IV, VII, and IX than in the other groups.
Multigenic families are usually derived from gene duplications, and the expansion mechanism includes unequal crossing-over, various transposition events, duplication of large chromosome segments, or polyploidization events (Zhang, 2003; Passardi et al., 2004). Theoretically, duplication events can often produce two gene copies, and one or both copies can acquire novel functions under a smaller selective pressure of evolution (Van de Peer et al., 2009). It appears that the loss and the insertion of new introns are frequent events, and they play important roles in gene evolution. It was reported that the number of introns was largely reduced and less frequently gained in eukaryotes in the course of evolution (Rogozin et al., 2012). In addition, analysis of segmental duplication events in rice showed that more introns were lost than gained (Lin et al., 2006). In this study, the intron distribution within NtGLK genes is quite variable, and the range varied from 0 to 11 (Figure 1), inferring that the shuffling of introns has been a main configuration for the evolution of NtGLK genes since their origin. Moreover, a total of four duplication gene pairs, including NtGLK6/62, NtGLK14/62, NtGLK11/38, and NtGLK27/55 (Supplementary Table 4), appeared to have experienced intron loss events based on our analysis. Notably, one of the transposition events, the retrotransposition of cDNA, is characterized by the loss of all introns and regulatory sequences and by a random insertion within the genome (Casacuberta and Santiago, 2003). In this study, there were five NtGLK genes with no introns (NtGLK12, NtGLK73, NtGLK7, NtGLK80, and NtGLK45), suggesting that these genes might be derived from the retrotransposition events. One of the gene pairs with no introns (NtGLK7/NtGLK45) met the parameters of segmental duplication; thus, they might be due to retrotransposition, but not originated from segmental duplication.
The GLK genes have been derived from independent gene duplication as a group of pairs in plants including monocots, eudicots, and bryophytes, but the regulation of GLK gene expression appears to be different (Yasumura et al., 2005). The GLK genes in maize act differentially in mesophyll cells and bundle sheath for chloroplast development, while they direct the development of chloroplasts in Arabidopsis to be monomorphic (Rossini et al., 2001; Fitter et al., 2002). To explore the potential functions of the NtGLK genes, RNA-Seq (Figure 8) and qRT-PCR for tobacco leaves in five senescence stages (Figure 9) were conducted for gene expression analyses. The expression levels of some NtGLK genes were decreased or increased, precisely corresponding consistently to the increase of the senescence degrees. Whether or not the differential expressions of GLK genes lead to assessing thylakoid deformation and impaired chlorophyll biosynthesis in chloroplasts still needs to be investigated. Studies with mutant and cross-species complementation experiments have demonstrated that, although GLK gene functions appear to be conserved in different species, the cross-species regulatory elements cannot drive gene expression in other species, suggesting that the GLK functional pathway has been diverged and species-specific during land colonization (Bravo-Garcia et al., 2009). Therefore, analysis of the specific expression patterns for these NtGLK genes provide preliminary information for their further functional exploration.
Conclusion
In this study, a total of 89 NtGLK genes were identified in the tobacco genome. They were classified into 10 subfamilies with diverse structures. Twelve pairs of NtGLK genes were found to be originated from segmental duplication. Phylogenetic analysis of the NtGLK genes showed that the GLK gene family existed prior to the separation of monocotyledons and dicotyledons. The NtGLK genes showed differential expression patterns in tobacco leaves at five senescence stages; among them, the expression levels of six genes (NtGLK85, NtGLK17, NtGLK39, NtGLK48, NtGLK58, and NtGLK61) were reduced, coinciding precisely with the increment of the degree of senescence, suggesting that these genes can be further developed as marker genes for maturity evaluation. Our results provide valuable information for further functional study of the NtGLK genes.
Data Availability Statement
The raw data supporting the conclusions of this article will be made available by the authors, without undue reservation.
Author Contributions
XX designed this research and wrote the manuscript. MQ and BZ performed the experiments and analyzed the data. GG and XY collected the plant materials and performed the experiments. JZY helped draft the manuscript. JHY participated in handling the figures and tables. All authors contributed to the article and approved the submitted version.
Funding
This project has been supported by grants from Fujian Tobacco Company (2019350000240137), Fujian Agriculture and Forestry University Innovation Foundation (no. CXZX2019052G), and the National Natural Science Foundation of China (no. 31501085).
Conflict of Interest
The authors declare that the research was conducted in the absence of any commercial or financial relationships that could be construed as a potential conflict of interest.
Supplementary Material
The Supplementary Material for this article can be found online at: https://www.frontiersin.org/articles/10.3389/fgene.2021.626352/full#supplementary-material
Footnotes
- ^ https://solgenomics.net/organism/Nicotiana_attenuata/genome
- ^ https://www.ncbi.nlm.nih.gov/Structure/cdd/wrpsb.cgi
- ^ https://solgenomics.net
- ^ http://web.expasy.org/protparam/
- ^ http://dnaman.software.informer.com/
- ^ http://www.plantbreeding.wur.nl/uk/software_mapinspect.html
- ^ http://gsds.cbi.pku.edu.cn/
- ^ http://alternate.meme-suite.org/tools/meme
- ^ http://bioinformatics.psb.ugent.be/webtools/plantcare/html/
- ^ http://www.biomarker.com.cn/
- ^ http://dnaman.software.informer.com/
References
Altschul, S. F., Gish, W., Miller, W., Myers, E. W., and Lipman, D. J. (1990). Basic local alignment search tool. J. Mol. Biol. 215, 403–410. doi: 10.1016/S0022-2836(05)80360-2
Atanu, G., and Utpal, G. (2014). MiRNA gene counts in chromosomes vary widely in a species and biogenesis of miRNA largely depends on transcription or post-transcriptional processing of coding genes. Front. Genet. 5:100. doi: 10.3389/fgene.2014.00100
Bjellqvist, B., Basse, B., Olsen, E., and Celis, J. E. (1994). Reference points for comparisons of two-dimensional maps of proteins from different human cell types defined in a pH scale where isoelectric points correlate with polypeptide compositions. Electrophoresis 15, 529–539. doi: 10.1002/elps.1150150171
Bjellqvist, B., Hughes, G. J., Pasquali, C., Paquet, N., Ravier, F., Sanchez, J. C., et al. (1993). The focusing positions of polypeptides in immobilized pH gradients can be predicted from their amino acid sequences. Electrophoresis 14, 1023–1031. doi: 10.1002/elps.11501401163
Bravo-Garcia, A., Yasumura, Y., and Langdale, J. A. (2009). Specialization of the Golden2-like regulatory pathway during land plant evolution. New Phytol. 183, 133–141. doi: 10.1111/j.1469-8137.2009.02829.x
Casacuberta, J. M., and Santiago, N. (2003). Plant LTR-retrotransposons and MITEs: control of transposition and impact on the evolution of plant genes and genomes. Gene 311, 1–11. doi: 10.1016/s0378-1119(03)00557-2
Chen, C. T., Chen, H., Zhang, Y., Thomas, H. R., Frank, M. H., He, Y. H., et al. (2020). TBtools: an integrative toolkit developed for interactive analyses of big biological data. Mol. Plant 13, 1194–1202. doi: 10.1016/j.molp.2020.06.009
Edwards, K. D., Fernandez-Pozo, N., Drake-Stowe, K., Humphry, M., Evans, A. D., Bombarely, A., et al. (2017). A reference genome for Nicotiana tabacum enables map-based cloning of homeologous loci implicated in nitrogen utilization efficiency. BMC Genomics 18:448. doi: 10.1186/s12864-017-3791-6
Fitter, D. W., Martin, D. J., Copley, M. J., Scotland, R. W., and Langdale, J. A. (2002). GLK gene pairs regulate chloroplast development in diverse plant species. Plant J. 31, 713–727. doi: 10.1046/j.1365-313x.2002.01390.x
Gan, S., and Amasino, R. M. (1997). Making sense of senescence (molecular genetic regulation and manipulation of leaf senescence). Plant Physiol. 113, 313–319. doi: 10.1104/pp.113.2.313
Gregersen, P. L., Culetic, A., Boschian, L., and Krupinska, K. (2013). Plant senescence and crop productivity. Plant Mol. Biol. 82, 603–622. doi: 10.1007/s11103-013-0013-8
Gu, Z. L., Cavalcanti, A., Chen, F. C., Bouman, P., and Li, W. H. (2002). Extent of gene duplication in the genomes of Dro-sophila, Nematode, and Yeast. Mol. Biol. Evol. 19, 256–262.
Hall, L. N., Rossini, L., Cribb, L., and Langdale, J. A. (1998). GOLDEN 2: a novel transcriptional regulator of cellular differentiation in the maize leaf. Plant Cell 10, 925–936. doi: 10.1105/tpc.10.6.925
Han, X. Y., Li, P. X., Zou, L. J., Tan, W. R., Zheng, T., Zhang, D. W., et al. (2016). GOLDEN2-LIKE transcription factors coordinate the tolerance to Cucumber mosaic virus in Arabidopsis. Biochem. Biophys. Res. Commun. 477, 626–632. doi: 10.1016/j.bbrc.2016.06.110
Hörtensteiner, S. (2006). Chlorophyll degradation during senescence. Annu. Rev. Plant Biol. 57, 55–77. doi: 10.1146/annurev.arplant.57.032905.105212
Hosoda, K., Imamura, A., Katoh, E., Hatta, T., Tachiki, M., Yamada, H., et al. (2002). Molecular structure of the GARP family of plant Myb-related DNA binding motifs of the Arabidopsis response regulators. Plant Cell 14, 2015–2029. doi: 10.1105/tpc.002733
Hu, B., Jin, J., Guo, A. Y., Zhang, H., Luo, J., and Gao, G. (2015). GSDS 2.0: an upgraded gene feature visualization server. Bioinformatics 31, 1296–1297. doi: 10.1093/bioinformatics/btu817
Jarvis, P., and López-Juez, E. (2013). Biogenesis and homeostasis of chloroplasts and other plastids. Nat. Rev. Mol. Cell Biol. 14, 787–802. doi: 10.1038/nrm3702
Kirchhoff, H. (2019). Chloroplast ultrastructure in plants. New Phytol. 223, 565–574. doi: 10.1111/nph.15730
Kräutler, B. (2016). Breakdown of chlorophyll in higher plants–phyllobilins as abundant, yet hardly visible signs of ripening, senescence, and cell death. Angew. Chem. Int. Ed. Engl. 55, 4882–4907. doi: 10.1002/anie.201508928
Kumar, S., Stecher, G., Li, M., Knyaz, C., and Tamura, K. (2018). MEGA X: molecular evolutionary genetics analysis across computing platforms. Mol. Biol. Evol. 35, 1547–1549. doi: 10.1093/molbev/msy096
Langdale, J. A., and Kidner, C. A. (1994). bundle sheath defective, a mutation that disrupts cellular differentiation in maize leaves. Development 120, 673–681.
Lescot, M., Déhais, P., Thijs, G., Marchal, K., Moreau, Y., Van de Peer, Y., et al. (2002). PlantCARE, a database of plant cis-acting regulatory elements and a portal to tools for in silico analysis of promoter sequences. Nucleic Acids Res. 30, 325–327. doi: 10.1093/nar/30.1.325
Lim, P. O., Woo, H. R., and Nam, H. G. (2003). Molecular genetics of leaf senescence in Arabidopsis. Trends Plant Sci. 8, 272–278. doi: 10.1016/S1360-1385(03)00103-1
Lin, H. N., Zhu, W., Silva, J. C., Gu, X., and Buell, C. R. (2006). Intron gain and loss in segmentally duplicated genes in rice. Genome Biol. 7:R41. doi: 10.1186/gb-2006-7-5-r41
Lira, B. S., Gramegna, G., Trench, B. A., Alves, F., Silva, E. M., Silva, G., et al. (2017). Manipulation of a senescence-associated gene improves fleshy fruit yield. Plant Physiol. 175, 77–91. doi: 10.1104/pp.17.00452
Liu, F., Xu, Y. J., Han, G. M., Zhou, L. Y., Ali, A., Zhu, S. W., et al. (2016). Molecular evolution and genetic variation of G2-Like transcription factor genes in maize. PLoS One 11:e0161763. doi: 10.1371/journal.pone.0161763
Liu, J. F. (2018). Bioinformatics Analysis of tomato G2-like Transcription Factor Family and Identification of Resistance-Related Genes. Dissertation’s thesis. Harbin: Northeast Agricultural University.
Livak, K. J., and Schmittgen, T. D. (2001). Analysis of relative gene expression data using real-time quantitative PCR and the 2(-Delta Delta C(T)) method. Methods 25, 402–408. doi: 10.1006/meth.2001.1262
Lu, S. N., Wang, J. Y., Chitsaz, F., Derbyshire, M. K., Geer, R. C., Gonzales, N. R., et al. (2020). CDD/SPARCLE: the conserved domain database in 2020. Nucleic Acids Res. 48, D265–D268. doi: 10.1093/nar/gkz991
Ma, W. X., Noble, W. S., and Bailey, T. L. (2014). Motif-based analysis of large nucleotide data sets using MEME-ChIP. Nat. Protoc. 9, 1428–1450. doi: 10.1038/nprot.2014.083
Murmu, J., Wilton, M., Allard, G., Pandeya, R., Desveaux, D., Singh, J., et al. (2014). Arabidopsis GOLDEN2-LIKE (GLK) transcription factors activate jasmonic acid (JA)-dependent disease susceptibility to the biotrophic pathogen Hyaloperonospora arabidopsidis, as well as JA-independent plant immunity against the necrotrophic pathogen Botrytis cinerea. Mol. Plant Pathol. 15, 174–184. doi: 10.1111/mpp.12077
Nagatoshi, Y., Mitsuda, N., Hayashi, M., Inoue, S., Okuma, E., Kubo, A., et al. (2016). GOLDEN 2-LIKE transcription factors for chloroplast development affect ozone tolerance through the regulation of stomatal movement. Proc. Natl. Acad. Sci. U.S.A. 113, 4218–4223. doi: 10.1073/pnas.1513093113
Nützmann, H. W., Daniel, D., América, R. C., Jesús, E. S., Eva, W., Marco, D. S., et al. (2020). Active and repressed biosynthetic gene clusters have spatially distinct chromosome states. Proc. Natl. Acad. Sci. U.S.A. 117, 13800–13809. doi: 10.1073/pnas.1920474117
Passardi, F., Longet, D., Penel, C., and Dunand, C. (2004). The class III peroxidase multigenic family in rice and its evolution in land plants. Phytochemistry 65, 1879–1893. doi: 10.1016/j.phytochem.2004.06.023
Powell, A. L., Nguyen, C. V., Hill, T., Cheng, K. L., Figueroa-Balderas, R., Aktas, H., et al. (2012). Uniform ripening encodes a Golden 2-like transcription factor regulating tomato fruit chloroplast development. Science 336, 1711–1715. doi: 10.1126/science.1222218
Rauf, M., Arif, M., Dortay, H., Matallana-Ramírez, L. P., Waters, M. T., Gil Nam, H., et al. (2013). ORE1 balances leaf senescence against maintenance by antagonizing G2-like-mediated transcription. EMBO Rep. 14, 382–388. doi: 10.1038/embor.2013.24
Riechmann, J. L., Heard, J., Martin, G., Reuber, L., Jiang, C., Keddie, J., et al. (2000). Arabidopsis transcription factors: genome-wide comparative analysis among eukaryotes. Science 290, 2105–2110. doi: 10.1126/science.290.5499.2105
Rogozin, I. B., Carmel, L., Csuros, M., and Koonin, E. V. (2012). Origin and evolution of spliceosomal introns. Biol. Direct 7:11. doi: 10.1186/1745-6150-7-11
Rose, A., Meier, I., and Wienand, U. (2000). The tomato I-box binding factor LeMBYI is a member of a novel class of myb-like proteins. Plant J. 20, 641–652.
Rossini, L., Cribb, L., Martin, D. J., and Langdale, J. A. (2001). The maize golden2 gene defines a novel class of transcriptional regulators in plants. Plant Cell 13, 1231–1244. doi: 10.1105/tpc.13.5.1231
Rubio, V., Linhares, F., Solano, R., Martin, A. C., Iglesias, J., Leyva, A., et al. (2001). A conserved MYB transcription factor involved in phosphate starvation signaling both in vascular plants and in unicellular algae. Genes Dev. 15, 2122–2133.
Savitch, L. V., Subramaniam, R., Allard, G. C., and Singh, J. (2007). The GLK1 ‘regulon’ encodes disease defense related proteins and confers resistance to Fusarium graminearum in Arabidopsis. Biochem. Biophys. Res. Commun. 359, 234–238. doi: 10.1016/j.bbrc.2007.05.084
Schippers, J. H., Schmidt, R., Wagstaff, C., and Jing, H. C. (2015). Living to die and dying to live: the survival strategy behind leaf senescence. Plant Physiol. 169, 914–930. doi: 10.1104/pp.15.00498
Schreiber, K. J., Nasmith, C. G., Allard, G., Singh, J., Subramaniam, R., and Desveaux, D. (2011). Found in translation: high-throughput chemical screening in Arabidopsis thaliana identifies small molecules that reduce Fusarium head blight disease in wheat. Mol. Plant Microbe Interact. 24, 640–648. doi: 10.1094/MPMI-09-10-0210
Thompson, J. D., Gibson, T. J., Plewniak, F., Jeanmougin, F., and Higgins, D. G. (1997). The ClustalX windows interface: flexible strategies for multiple sequence alignment aided by quality analysis tools. Nucleic Acids Res. 25, 4876–4882.
Trapnell, C., Williams, B. A., Pertea, G., Mortazavi, A., Kwan, G., van Baren, M. J., et al. (2010). Transcript assembly and quantification by RNA-Seq reveals unannotated transcripts and isoform switching during cell differentiation. Nat. Biotechnol. 28, 511–515. doi: 10.1038/nbt.1621
Van de Peer, Y., Maere, S., and Meyer, A. (2009). The evolutionary significance of ancient genome duplications. Nat. Rev. Genet. 10, 725–732. doi: 10.1038/nrg2600
Wang, L. Q., Guo, K., Li, Y., Tu, Y. Y., Hu, H. Z., Wang, B. R., et al. (2010). Expression profiling and integrative analysis of the CESA/CSL superfamily in rice. BMC Plant Biol. 10:282. doi: 10.1186/1471-2229-10-282
Waters, M. T., Moylan, E. C., and Langdale, J. A. (2008). GLK transcription factors regulate chloroplast development in a cell-autonomous manner. Plant J. 56, 432–444. doi: 10.1111/j.1365-313X.2008.03616.x
Wilkins, M. R., Gasteiger, E., Bairoch, A., Sanchez, J. C., Williams, K. L., Appel, R. D., et al. (1999). Protein identification and analysis tools in the ExPASy server. Methods Mol. Biol. 112, 531–552. doi: 10.1385/1-59259-584-7:531
Xiao, Y., You, S., Kong, W., Tang, Q., Bai, W., Cai, Y., et al. (2019). A GARP transcription factor anther dehiscence defected 1 (OsADD1) regulates rice anther dehiscence. Plant Mol. Biol. 101, 403–414.
Xu, X. Y., Liao, K. F., Dai, J. R., Hu, W. Z., Cheng, S. Y., Duan, Y. M., et al. (2017). A study on different harvest maturity levels in leaf structures and physiological and biochemical properties of fresh tobacco leaves. J. Yunnan Univ. 39, 313–323.
Yang, S. H., Zhang, X. H., Yue, J. X., Tian, D. C., and Chen, J. Q. (2008). Recent duplications dominate NBS-encoding gene expansion in two woody species. Mol. Genet. Genomics 280, 187–198.
Yasumura, Y., Moylan, E. C., and Langdale, J. A. (2005). A conserved transcription factor mediates nuclear control of organelle biogenesis in anciently diverged land plants. Plant Cell 17, 1894–1907. doi: 10.1105/tpc.105.033191
Keywords: gene expression, senescence, phylogenetic analysis, G2-like transcription factors, Nicotiana tobacum
Citation: Qin M, Zhang B, Gu G, Yuan J, Yang X, Yang J and Xie X (2021) Genome-Wide Analysis of the G2-Like Transcription Factor Genes and Their Expression in Different Senescence Stages of Tobacco (Nicotiana tabacum L.). Front. Genet. 12:626352. doi: 10.3389/fgene.2021.626352
Received: 05 November 2020; Accepted: 22 April 2021;
Published: 31 May 2021.
Edited by:
Diane Maria Beckles, University of California, Davis, United StatesReviewed by:
Jingwei Yu, Southern University of Science and Technology, ChinaErtugrul Filiz, Duzce University, Turkey
Jun Zheng, Chinese Academy of Agricultural Sciences (CAAS), China
Copyright © 2021 Qin, Zhang, Gu, Yuan, Yang, Yang and Xie. This is an open-access article distributed under the terms of the Creative Commons Attribution License (CC BY). The use, distribution or reproduction in other forums is permitted, provided the original author(s) and the copyright owner(s) are credited and that the original publication in this journal is cited, in accordance with accepted academic practice. No use, distribution or reproduction is permitted which does not comply with these terms.
*Correspondence: Xiaofang Xie, eHhmMzE3QGZhZnUuZWR1LmNu
†These authors have contributed equally to this work