- 1Institute of Gene Science and Industrialization for Bamboo and Rattan Resources, International Center for Bamboo and Rattan, Beijing, China
- 2Key Laboratory of National Forestry and Grassland Administration, Beijing for Bamboo and Rattan Science and Technology, Beijing, China
Invertases (INVs) can irreversibly hydrolyze sucrose into fructose and glucose, which play principal roles in carbon metabolism and responses to various stresses in plants. However, little is known about the INV family in bamboos, especially their potential function in drought stress. In this study, 29 PeINVs were identified in moso bamboo (Phyllostachys edulis). They were clustered into alkaline/neutral invertase (NINV) and acid invertase (AINV) groups based on the gene structures, conserved motifs, and phylogenetic analysis results. The collinearity analysis showed nine segmental duplication pairs within PeINVs, and 25 pairs were detected between PeINVs and OsINVs. PeINVs may have undergone strong purification selection during evolution, and a variety of stress and phytohormone-related regulatory elements were found in the promoters of PeINVs. The tissue-specific expression analysis showed that PeINVs were differentially expressed in various moso bamboo tissues, which suggested that they showed functional diversity. Both the RNA-seq and quantitative real-time PCR results indicated that four PeINVs were significantly upregulated under drought stress. Co-expression network and Pearson’s correlation coefficient analyses showed that these PeINVs co-expressed positively with sugar and water transport genes (SWTGs), and the changes were consistent with sugar content. Overall, we speculate that the identified PeINVs are spatiotemporally expressed, which enables them to participate in moso bamboo growth and development. Furthermore, PeINVs, together with SWTGs, also seem to play vital roles in the response to drought stress. These results provide a comprehensive information resource for PeINVs, which will facilitate further study of the molecular mechanism underlying PeINVs involvement in the response to drought stress in moso bamboo.
Introduction
Carbon autotrophy is a prominent characteristic of higher plants (Roitsch and González, 2004). Sucrose is the main carbohydrate produced by photosynthetically active tissues and is translocated through the phloem from the source leaves to sink organs as a transport molecule (Tiessen and Padilla-Chacon, 2012; Bihmidine et al., 2013). The absorption of sucrose from sinks can occur either directly or through the cleavage of sucrose (Cho et al., 2005). Sucrose can be reversibly hydrolyzed to UDP-glucose and fructose by sucrose synthase (SuSy; EC 2.4.1.13) (Koch, 2004) and irreversibly divided into simple hexoses (glucose and fructose) by invertases (INV; EC 3.2.1.26) (Tang et al., 1999). The present results show that SuSy mainly participates in the biosynthesis of sugar polymers (Roitsch and González, 2004; Hummel et al., 2009). In addition, INV is known to be widely involved in regulating plant growth and development, such as reproductive development and stress tolerance responses (Koch, 2004; Barratt et al., 2009; Wang and Ruan, 2016). In particular, sucrose and its hydrolysis products are indispensable in gene expression, signal transduction, and stress responses to plant growth (Ruan, 2014).
The INVs of higher plants are classified into two subfamilies based on their optimal pH: acid invertases (AINVs) (pH between 4.5 and 5.0) and alkaline/neutral invertases (NINVs) (pH 6.5 to 8.0) (Salerno and Curatti, 2003), respectively. NINVs are believed to be closely related to cyanobacterial invertases, which belong to glycoside hydrolase family 100 (GH 100) (Vargas et al., 2003). In contrast, AINVs arise from respiratory eukaryotes and aerobic bacteria, which belong to glycoside hydrolase family 32 (GH 32) (Sturm and Chrispeels, 1990) and are classified as cell-wall invertases (CWINVs) and vacuole invertases (VINVs) according to their location in cell wall and vacuole, respectively. Although CWINVs and VINVs are found in different locations, they share some common biochemical properties (Ruan et al., 2010). For example, they both catalyze sucrose and other β-fructoses, including oligosaccharides, therefore, they are also referred to as β-fructofuranosidases (Stitt et al., 1991). In contrast, NINVs, which lack the N-terminal signal peptide and are not β-fructofuranosidases, specifically catalyze sucrose (Sturm, 1999). There are two conserved motifs, which are the β-fructosidase motif (NDPD/NG) and the cysteine catalytic domain motif (WECV/PD). They are associated with AINVs active site (Verhaest et al., 2006; Canam et al., 2008). Little is known about NINV functions and their regulatory mechanisms because it is difficult to purify them and determine their expressions (Koch, 2004).
In the past decades, with the development of genome sequencing technology, INV family genes have been identified in many plants, such as Arabidopsis thaliana, Oryza sativa (Ji et al., 2005), Triticum aestivum (Francki et al., 2006), Zea mays (Koch et al., 1996), Populus trichocarpa (Chen et al., 2015), and Capsicum annuum (Shen et al., 2018a, b). The functional characterization of INVs and their regulatory mechanisms have also been widely documented. Many studies on various plants have confirmed that CWINVs play essential roles in the regulation of sucrose partitioning (Koch et al., 1996), seed and pollen development (Chourey et al., 2006), and environmental responses (Proels and Hückelhoven, 2014). Furthermore, VINVs have been found to function in fruits and storage organs (Yu et al., 2008), and during drought and hypoxia stress (Roitsch and González, 2004). Incw2 mRNA levels increased in the ovary after pollination and tended to be lower in response to drought stress (Andersen et al., 2002). IVR2 plays different regulatory roles in the sink and source organs of maize under drought stress (Kim et al., 2000), and OsVIN2 is upregulated rather than downregulated in rice under drought stress (Ji et al., 2005). The proposed functions of NINVs have also been gradually identified, including sugar signal transduction (Lou et al., 2007), cellulose biosynthesis (Rende et al., 2017), shoot and root growth (Martín et al., 2013), flower and fruit development (Roitsch and González, 2004), and response to biological and abiotic stresses (Liu et al., 2015). A. thaliana plants that overexpress PtrA/NINV have a better capacity to adjust their osmotic potential in response to drought stress (Dahro et al., 2016). The function of INVs is diverse in plants, and a comprehensive analysis of INVs may improve our understanding of their function during in the sucrose metabolism in plants.
Moso bamboo (Phyllostachys edulis), which belongs to the Bambusoideae of the Poaceae, and is one of the most important bamboo species in China, occupies a forestland area of 4.68 million hm2, accounting for 72.96% of the bamboo forest in China (Li and Feng, 2019). Moso bamboo can grow more than 1 m in height per day during its rapid growth period and its height growth within one and a half months (Peng et al., 2013). It has been reported that PeAINVs may be involved in internode elongation in moso bamboo shoots (Guo et al., 2020). However, drought is one of the leading detrimental environmental factors affecting the rapid growth of moso bamboo. It has been reported that the transcription factors (bHLH and WRKY) and structural genes (LEA, UGE, ZEP, and AQP) participate in the response to drought stress (Huang et al., 2016b; Sun et al., 2016; Li et al., 2017; Lou et al., 2017; Sun et al., 2017; Cheng et al., 2018). Furthermore, sugar transport pathway genes also play essential roles in the response to stress (Roitsch and González, 2004; Ruan et al., 2010). However, the possible role of INVs in bamboo remains unclear. Understanding the molecular characteristics and evolution of INV family members is the first step in studying their function. In this study, a comprehensive analysis of INV gene members was conducted based on the updated genome for moso bamboo (Zhao et al., 2018). It mainly focused on the gene structure, conserved motifs, sequence phylogeny, gene syntenic, expression patterns in various tissues and in leaves of moso bamboo under drought stress. We also built a co-expression network for PeINVs, sugar and water transport genes (SWTGs), whose expression patterns were further validated by qRT-PCR. This study aimed to: (1) identify the INVs members in moso bamboo; (2) analyze the genetic diversity and functional differentiation of PeINVs; and (3) validate the expression patterns and relationships of PeINVs and SWTGs under drought stress.
Materials and Methods
Identification of Invertase Genes in Moso Bamboo
We used two different approaches to annotate and identify the INV genes in moso bamboo. First, the known sequences of INVs from the O. sativa and A. thaliana databases (Supplementary Table 1) were used as queries to search for potential INVs in the moso bamboo genome database1 using the Basic Local Alignment Tool for Protein (BLASTP 2.9.0) program (e-value < 1 × 10–5). Second, HMMRE 3.0 software was used to identify INVs using hidden Markov model (HMM) profile of the invertase domain (NINV: PF12899, CWINV: PF08244 and PF00251, and VINV: PF11837, PF08244, and PF00251) from the Pfam database as queries (e-value < 1 × 10–5). Subsequently, each putative INV was further examined for the presence of conserved domains by submitting them to Pfam2 (Finn et al., 2014) and the simple modular architecture research tool (SMART) database3 (Letunic et al., 2012), and the sequences with complete conserved domains were preserved. In addition, the INVs in Bonia amplexicaulis and Olyra latifolia were also investigated and used for comparative analysis.
Sequence Analysis, Protein Properties, and Phylogenetic Tree Construction of Invertase Family Members
A gene structure analysis of INVs was performed using the Gene Structure Display Server4 (Hu et al., 2015). The ExPASy website (ProtParam5) was used to calculate the molecular weight, theoretical isoelectric point (pI), and protein instability index of predicted INV proteins in three bamboo species (Gasteiger et al., 2003). MEME server6 and TBtools 1.08 were employed to analyze the motif composition of INV proteins in three bamboo species (Brown et al., 2013; Chen et al., 2020).
The amino acid sequences of NINVs and AINVs from six plant species (P. edulis, B. amplexicaulis, O. latifolia, O. sativa, A. thaliana, and P. trichocarpa) (Supplementary Table 1) were used to construct two unrooted phylogenetic trees using MEGA 7.0, respectively (Kumar et al., 2016). Neighbor-joining topologies were generated as the consensus of 1,000 bootstrap alignment replicates by running MEGA 7.0 with ClustalW alignment.
Physical Localization and Gene Duplication Analysis of PeINVs
The chromosome localization data for each INV identified in moso bamboo were retrieved from the generic file format (GFF) file using TBtools 1.08. MCScanX software with the default settings was used to identify duplicated and syntenic INVs within the moso bamboo genome and those that were orthologous between moso bamboo and the O. sativa, B. amplexicaulis, or O. latifolia genomes (Wang et al., 2012). The chromosome distributions and syntenic relationships of the INVs were visualized using Circos 0.69-9 software (Krzywinski et al., 2009). The syntenic analysis maps were constructed using TBtools 1.08 software to display the syntenic relationships among the orthologous INVs in moso bamboo and other selected species (Chen et al., 2020), and the non-synonymous substitution (Ka), synonymous substitution (Ks), and Ka/Ks ratios of syntenic INV gene pairs were calculated using KaKs_Calculator 2.0 software (Wang et al., 2010).
Promoter Sequence Analysis of PeINVs
The 2.0 kb genomic DNA sequences upstream of the translation start of PeINVs were retrieved from the moso bamboo database (Zhao et al., 2018). The PlantCARE7 database was used to search for cis-acting elements associated with abiotic stress and phytohormone-dependent responses within promoter regions (Lescot et al., 2002).
RNA-Seq Data Analysis
The RNA-seq data for 26 tissues from moso bamboo (Zhao et al., 2018), which contained information about rhizomes, and the different growth stages of roots and shoots, leaves, and shoot buds (Sequence Read Archive accession number: SRS1847048–SRS1847073), were used for specific expression analysis of PeINVs. The RNA-seq data for moso bamboo leaves under drought stress (Huang et al., 2016a) were used to determine the expression patterns for PeINVs and SWTGs. The differentially expressed genes (DEGs) were identified using the Limma 3.13 software package in R 3.6.1 (Ritchie et al., 2015). The fragments per kilobase of transcript per million fragments mapped (FPKM) of these genes were extracted. The heatmap was drawn using the Pheatmap 1.0 package in R 3.6.1, with Euclidean distances and the complete linkage method for hierarchical clustering (Yang et al., 2017).
Plant Materials and Sucrose, Glucose, and Fructose Contents Determination
Moso bamboo seedlings were grown in a climatic chamber under a 12 h light/12 h dark photoperiod at 25°C. The treatment consisted of subjecting 2-month-old seedlings to drought stress [simulated by 20% (w/v) polyethylene glycol-6000 (PEG-6000)]. At stipulated times, the leaves were immediately collected at 0, 1, 2, 4, or 8 h, frozen in liquid nitrogen and stored at −80°C. All samples were collected from at least three biological replicates.
The sucrose, glucose, and fructose contents in leaves were determined using a sucrose-glucose-fructose content (hexokinase method) determination kit (Suzhou Grace Biotechnology Co., Ltd., Jiangsu, China). Sucrose and fructose are converted to glucose by specific enzymes, and glucose is reduced to nicotinamide adenine dinucleotide phosphate (NADPH) to NADP+ by enzyme complexes. The sucrose, glucose, and fructose contents were calculated by measuring the increase in NADPH.
RNA Isolation, qRT-PCR Analysis
Total RNA was extracted from the collected samples using an RNAprep Pure Plant kit (Tianmo, Beijing, China) according to the manufacturer’s instructions. First-strand cDNA was synthesized using a PrimeScript RT Reagent kit (Takara, Dalian, China). And qRT-PCR was performed using specific primers (Supplementary Table 2) designed by Primer Premier 5.0 software to check the DEGs identified by the RNA-seq data. PeTIP41 was used as an internal control (Fan et al., 2013). The relative expression level of each gene was calculated using the 2–ΔΔCT method with three technical repetitions (Livak and Schmittgen, 2001). The results were visualized using the Origin Pro 2017 software.
Gene Cloning, Yeast Transformation, and Drought Tolerance Analysis
Specific primers were designed for the open reading frame (ORF) based on the nucleotide sequences for PeCWINV8 in the bamboo genome database8 (Zhao et al., 2018; Supplementary Table 2). The cDNA was used as a template in the PCR process, which was performed using the primer pair PrimeSTAR Max mix (Takara, Japan). The PCR amplification products were inserted into the pGEM-T easy vector (Promega, Madison, WI, United States) and subsequently verified by sequencing (Ruibiotech, China). The PCR fragments were subcloned into the corresponding sites of the pYES2 vector (Invitrogen, Carlsbad, CA, United States), and then the vectors were introduced into INVSc1 yeast cells (Invitrogen, United States) using the transformation kit (Weidibio, China). Yeast cells expressing PeCWINV8 along with control cells were grown on Yeast Extract Peptone Dextrose Medium (YPD) solid medium (1% yeast extract, 2% peptone, and 2% glucose). For the stress analysis, transformed yeast cells were propagated in SC-U medium containing 2% galactose for 12 h and the cell density was adjusted to 1.0 of OD600 followed by serial dilutions. Yeast cell were spotted on YPD medium supplemented with PEG-6000 (0, 1, and 2%). The plates were maintained at 30°C and growth was monitored after 2 days (Alavilli et al., 2016; Sun et al., 2021).
Correlations and Co-expression Network Analyses
The correlations between the gene expression levels of 16 DEGs and the sugar contents of bamboo leaves under drought stress were constructed by calculating pairwise Pearson’s correlation coefficients (PCC) (Schober et al., 2018). Based on these correlations, a correlation heatmap of gene expression quantity and sugar content was generated using the Pheatmap 1.0 package in R 3.6.1. In addition, a co-expression network was constructed using Cytoscape 3.7.1 (Shannon et al., 2003) based on the results of the PCCs between PeINVs and SWTGs. The threshold for new edges was set with a PCC magnitude >0.8 and a p-value < 0.1, which is believed to indicate strongly co-expressed genes.
Statistical Analysis
The statistical analyses were preformed using IBM SPSS Statistics 22.0 (Armonk, NY, United States), and the mean and standard deviation of three biological replicates were presented. Significant differences are indicated at ∗p < 0.05, ∗∗p < 0.01.
Results
Invertase Family Members in Moso Bamboo
To identify the INVs in moso bamboo, the rice and Arabidopsis INVs were used as queries subjected to a Basic Local Alignment Search Tool (BLASTN) search in moso bamboo database. A total of 29 PeINVs containing fully conserved INV domains were identified in moso bamboo. The INV family in plants can be classified into three major classes: NINVs, CWINVs, and VINVs, based on their sequence homology. Among the 29 PeINVs, 15 were neutral/alkaline INVs (PeNINVs), and 14 were acid INVs (PeAINVs), which consisted of 10 cell-wall INVs (PeCWINVs) and four vacuolar INVs (PeVINVs) (Table 1).
We found that the protein molecular weight, theoretical isoelectric point, and protein instability index of the INV proteins varied dramatically among the three classes. However, they were relatively stable within the same class (Figure 1 and Supplementary Table 3). The protein molecular weight of the NINV class ranged from 46.7 to 69.4 kDa, those of the CWINV class ranged from 61.7 to 66.0 kDa, and those of the VINV class ranged from 71.7 to 73.7 kDa (Figure 1A and Supplementary Table 3). The theoretical isoelectric point (pI) of the NINV class ranged from 5.11 (PeNINV1) to 9.39 (PeNINV10), with an average of 6.32; those of the VINV class were all less than 6, but the CWINV class ranged from 5.11 (PeCWINV1) to 9.10 (PeCWINV5) with an average of 6.93 (Figure 1B and Supplementary Table 3). All the proteins in the NINV class were predicted to be unstable proteins with an instability index higher than 40. In contrast, 85% (12/14) of the CWINV and VINV classes were predicted to be stable proteins (Figure 1C and Supplementary Table 3).
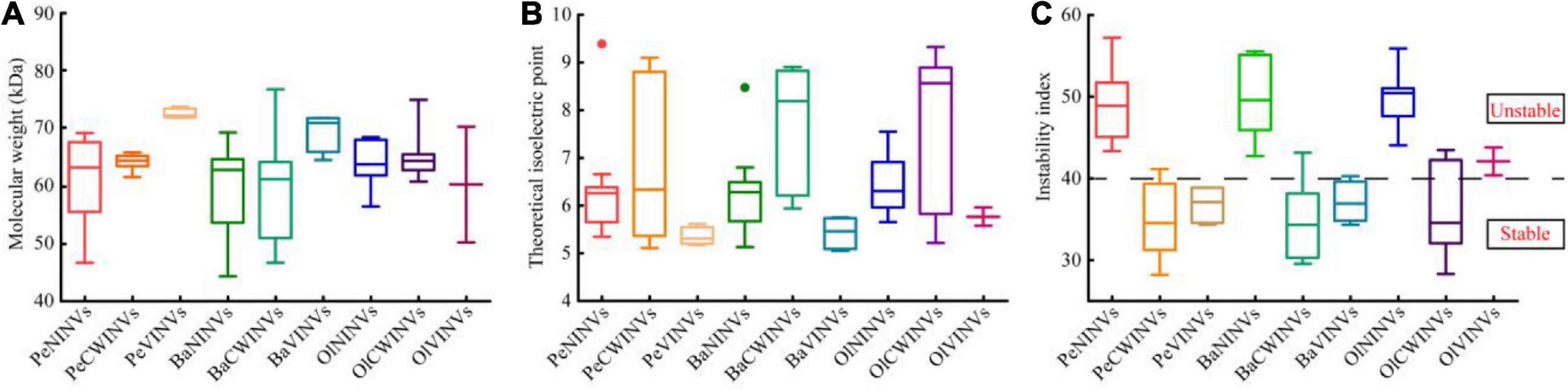
Figure 1. Amino acid characteristics of INVs in three bamboo species (Pe, Ba, and Ol indicate Phyllostachys edulis, Bonia amplexicaulis, and Olyra latifolia, respectively). (A) Molecular weight of proteins. (B) Theoretical isoelectric point (pI) of proteins. (C) Instability index of proteins.
Meanwhile, as the representative for different ploidy bamboo species, a hexaploid woody bamboo (B. amplexicaulis) and a diploid herbaceous bamboo (O. latifolia) were selected for comparative analysis. The result showed that 28 BaINVs and 17 OlINVs were discovered in B. amplexicaulis and O. latifolia, respectively, using the same procedure. They had similar classifications and characteristics as their orthologs in moso bamboo (Table 1 and Figure 1). The number of INV members in woody bamboo (P. edulis and B. amplexicaulis) was higher than that of the herbaceous plants (O. latifolia, O. sativa, and A. thaliana).
Gene Structure Analysis of PeINVs
Information about the distribution of exons and introns is vital when attempting to understand gene structure. Thus, we analyzed exon-intron features of 29 PeINVs, including the number and length of exons and introns (Figure 2 and Supplementary Table 4). The phylogenetic analysis revealed that the AINV subfamily members in moso bamboo could be divided into two clades, which were cell-wall-targeted (CWINV), and vacuole-targeted (VINV). The exon numbers for PeCWINVs ranged from 6 to 7, except for PeCWINV9, which had three exons. Four PeVINVs encoded irregular exons. Two contained seven exons, and the other two genes contained three or four exons. Interestingly, the first exon in PeCWINVs was shorter than that in PeVINVs, and 11 members of the PeAINVs encoded a mini-exon, which is one of the smallest exons in plants (Bournay et al., 1996). Many genes in the NINV subfamily are typically encoded by four or six exons in different classes (Nonis et al., 2008; Shen et al., 2018b; Bezerra-Neto et al., 2019). In line with previous reports, PeNINVs usually possess four or six exons, except PeNINV1 and PeNINV12 with three exons, and PeNINV3 and PeNINV10 with seven exons. In addition, seven members of the PeNINVs contained one shorter exon, as if it had been erroneously predicted by an intron or had originated within other exons. Most PeINVs clustered in the same group had similar numbers of exons and introns. Further analyses of the structural features of INVs in B. amplexicaulis and O. latifolia also supported this observation (Supplementary Figure 1).
Distribution of Conserved Motifs in PeINVs
We employed the MEME web server combined with TBtools software to determine the motif type and location in different PeINVs. The results showed that 15 conserved motifs were identified in PeAINVs and PeNINVs (Figure 3). Among these AINV motifs, most motifs classified as CWINVs or VINVs were similar. However, compared to the CWINVs, the non-conserved sequences at the N-terminus of VINVs were longer, and motif 11 was specifically distributed in the CWINVs. The conserved motifs-NDPNG, WECP/YDF, and RDP were localized in motif 1, motif 10, and motif 12 (Figure 3A and Supplementary Figure 3B), respectively (Lammens et al., 2009).
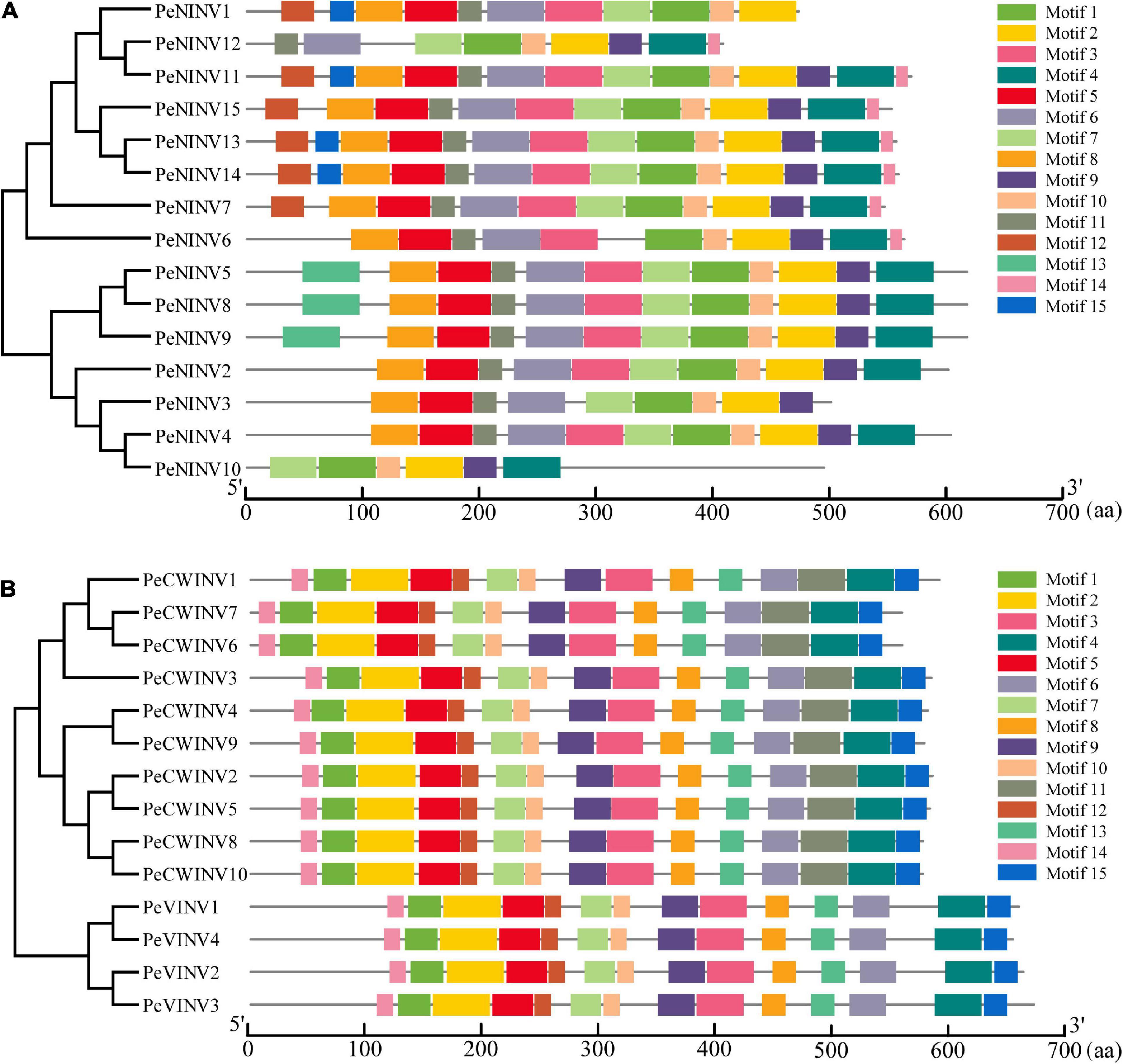
Figure 3. Distribution of motifs in PeINVs. (A) Motifs in PeNINVs. (B) Motifs in PeAINVs (PeCWINVs and PeVINVs). These motifs were investigated using the MEME web server and TBtools software. The different motifs were represented by different colors.
The NINVs were conserved for the putative functional motifs of their orthologs in moso bamboo. In general, after excluding some NINVs lacking complete sequences (PeNINV1, PeNINV10, and PeNINV12), the paralogs of PeNINVs were generally conserved with regards to motif distribution, except for motif 12, motif 13, and motif 15 in the N-terminus as well as motif 14 in the C-terminus. Eleven motifs were observed to be consistent in their distributions and sizes, which included two motifs (motif 3 and motif 6) containing catalytic residues (Figure 3B and Supplementary Figure 3A; Itoh et al., 2004; Ji et al., 2005). Motif 14 was unique to eight PeINVs, motif 12 was specifically distributed in the N-terminus of six genes, motif 15 was specifically distributed in the N-terminus of four genes, and motif 13 was distributed in the N-terminus of three PeINVs. Further analyses of the motif features of INVs in B. amplexicaulis and O. latifolia produced results that were similar to the moso bamboo results (Supplementary Figure 2).
Chromosome Localization, Gene Duplication, and Syntenic Analyses of PeINVs
The coordinates of 29 PeINVs were extracted from the moso bamboo GFF file to analyze their localization on the moso bamboo genome. All the PeINVs were distributed on 13 moso bamboo chromosomes at different densities (Figure 4A). Chromosome 24 contained the largest number of PeINVs (six), followed by chromosome 20 and chromosome 23 with five PeINVs, and one or two on the other chromosomes.
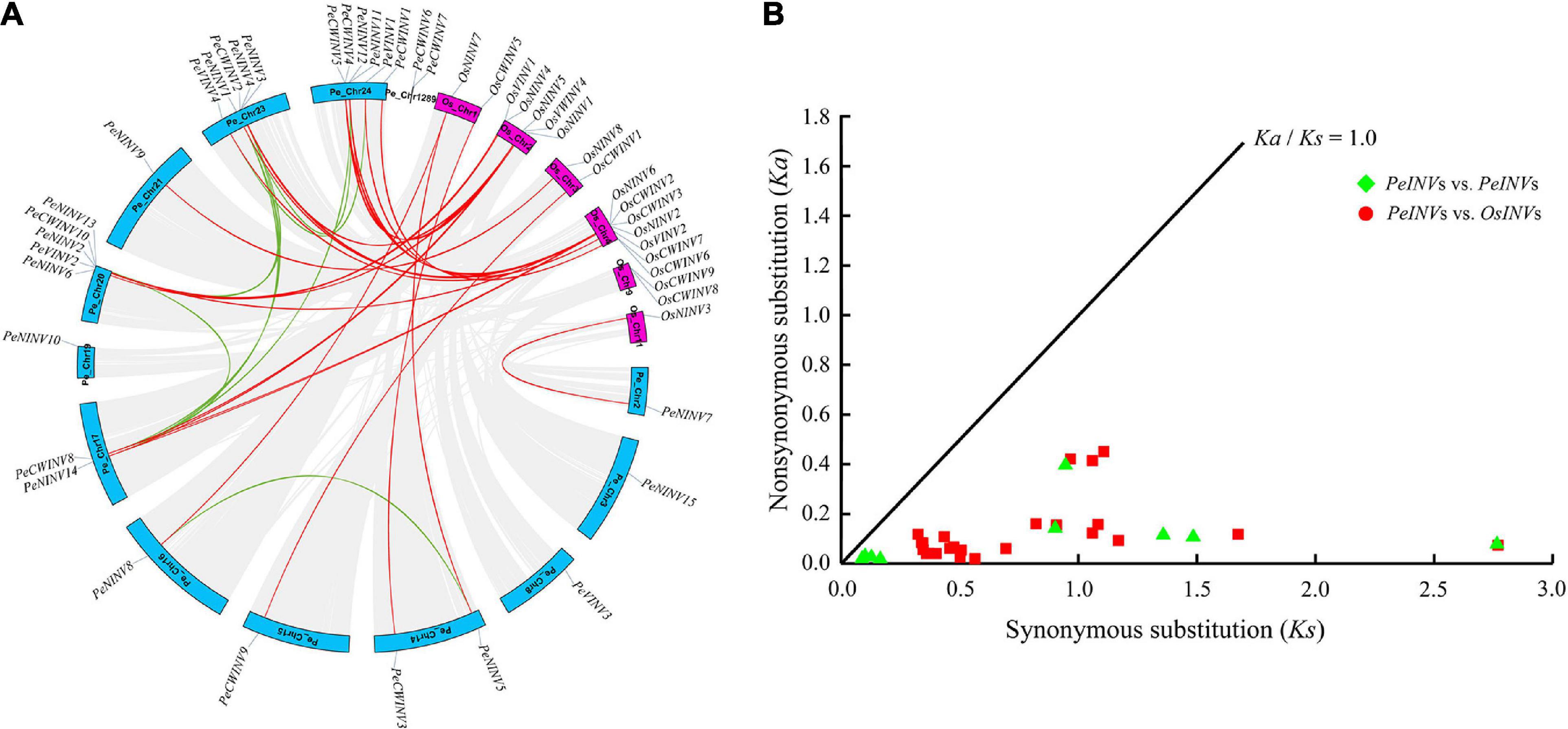
Figure 4. Synteny regions and Ka/Ks ratios analyses of INV genes. (A) Chromosome location and synteny regions of all PeINVs and OsINVs. The duplicated genes of PeINVs and PeINVs/OsINVs on different chromosomes were indicated with green and red lines, respectively. (B) The Ka/Ks ratios of INV homologous gene pairs.
All moso bamboo and rice INVs were analyzed using the BLAST and MCScanX, and visualized using the basic functions in the TBtools software to investigate their syntenic status. In this study, nine segmental duplication pairs were found within PeINVs, and 25 segmental duplication pairs were detected in PeINVs/OsINVs, but no tandem duplication events were detected (Figure 4A). These results indicate that the driving force for the expansion of INVs in moso bamboo and rice was segmental duplication events.
To further investigate the evolutionary selection pressure on INVs in moso bamboo and rice, the Ka, synonymous substitution (Ks), and the Ka/Ks ratios of all segmental duplication pairs were calculated. The Ka/Ks values of 34 pairs of duplicated genes were all below 1.0 (Figure 4B and Supplementary Table 5), suggesting that the evolutionary selection pressure acting on INVs in moso bamboo tended to be purification. A further synteny analysis comparison of PeINVs/OlINVs, and PeINVs/BaINVs in moso bamboo with B. amplexicaulis and O. latifolia showed consistent results (Supplementary Figure 4 and Supplementary Table 5).
Phylogenetic Analysis of INVs
To understand the evolutionary relationships among the INVs across different species, two unrooted phylogenetic trees were constructed for AINVs and NINVs using protein sequences from moso bamboo, B. amplexicaulis, O. latifolia, O. sativa, A. thaliana, and P. trichocarpa (Figure 5). Six phylogenetic trees were also constructed using INVs from these plant species, respectively (Supplementary Figure 5). A comparison of these phylogenetic trees demonstrated that the relationships among the INVs in six plant species were consistent and conserved. All INVs can be classified into AINV and NINV classes. AINVs can be divided into VINV and CWINV subgroups, and NINVs can be further subdivided into α and β subgroups (Figure 5).
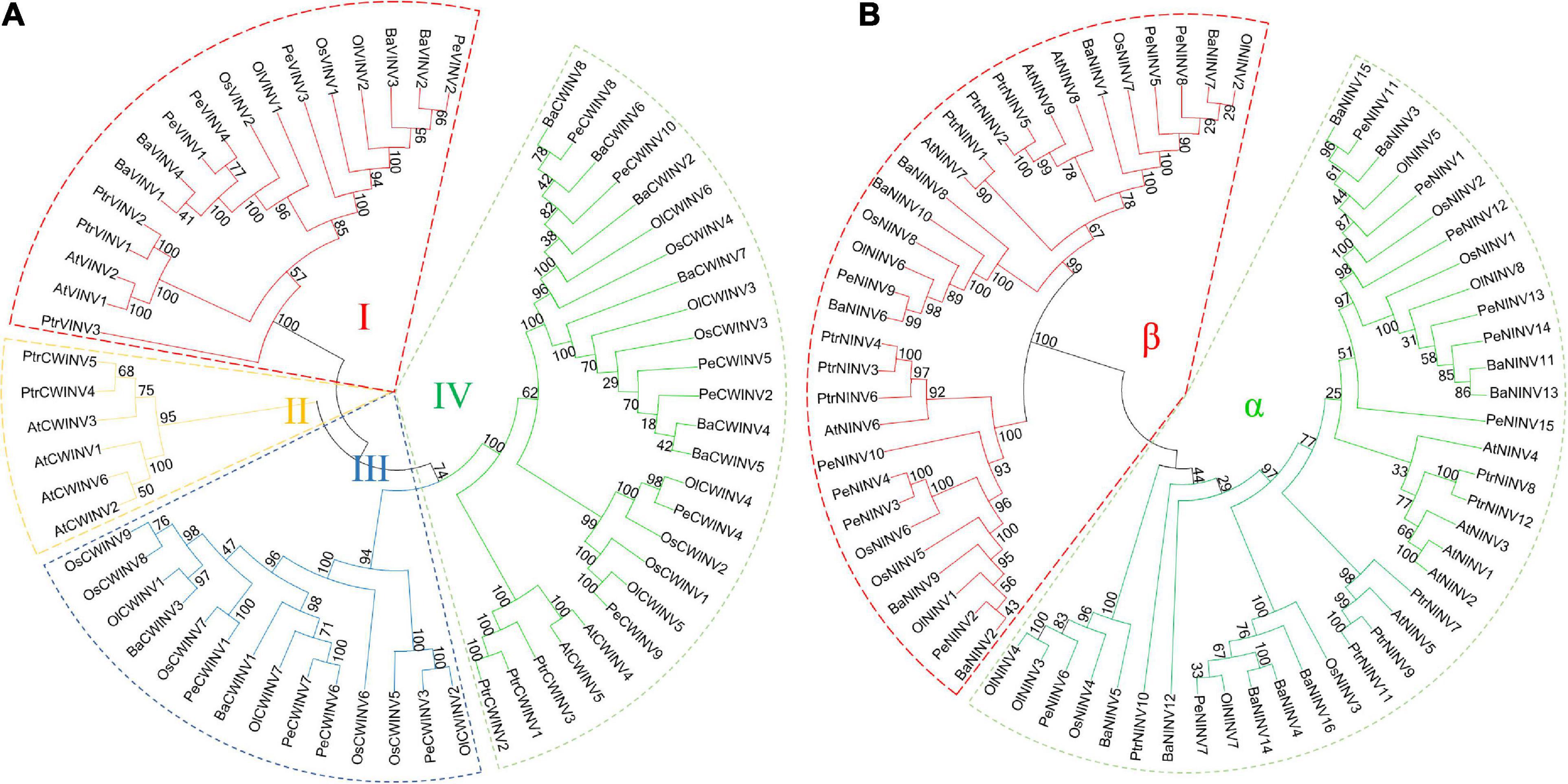
Figure 5. Phylogenetic tree of INVs in bamboo and other plants. (A) Acid invertase (CWINV and VINV). (B) Neutral/alkaline invertase (NINV). The phylogenetic tree was constructed via the neighbor-joining method (1,000 bootstrap replicates) using MEGA 7.0 software. The Pe, Ba, Ol, Os, At, and Ptr indicate invertase genes of Phyllostachys edulis, Bonia amplexicaulis, Olyra latifolia, Oryza sativa, Arabidopsis thaliana, and Populus trichocarpa, respectively.
The phylogenetic tree for the AINVs from six plant species formed four branches designated as branches I to IV (Figure 5A), and the members of different species in each branch were diverse. For example, branch I included four PeVINVs, branch III contained four PeCWINVs, and six PeCWINVs were clustered in branch IV. More than 70% (10/14) of PeAINVs from moso bamboo were clustered in branch I and branch IV, whereas only two and four members of rice were clustered in these two branches, respectively. All the members in branch I were VINVs and were found in six plant species, while those in branch II and branch III were only from dicots and monocots, respectively. The number of monocot members in branch IV was higher than the number of dicots.
The phylogenetic tree for the NINVs from six plant species could be separated into two distinct branches, referred to as the α and β branches (Figure 5B; Wang et al., 2017). There were eight and seven PeNINVs in the α and β branches, respectively. Moso bamboo also has more members (14) in the NINV subfamily than rice (eight), which may be related to the genomic replication events experienced during the evolution of moso bamboo. In the α branch, most NINVs from dicots were clustered together, suggesting that the gene duplication was more ancient in monocots than that in dicots (Wang et al., 2017).
Expression Patterns of PeINVs in Different Tissues
We used a published RNA-seq dataset of moso bamboo to investigate the expression patterns of PeINVs in diverse tissues. Twenty-nine PeINVs were divided into six groups based on the hierarchical clustering of expression patterns (Figure 6). There were 14 PeINVs in group I, most of which were scarcely expressed in 80% of the 26 tissues; group II contained three PeINVs expressed in every tissue, especially in the shoots and roots at different growth stages; and group III contained three PeINVs, which were preferentially expressed in shoots at different stages and in the 3.0 m shoot buds. These results also indicate that the PeINVs in group II and group III might be directly or indirectly involved in the rapid growth of bamboo shoots. Only PeVINV2 in group IV, was mainly expressed in shoots and leaves. Group V included four PeINVs that were primarily detected in tender tissues (0.1 cm roots, 0.2 m shoots, leaves, and shoot buds). Four PeINVs in group VI were scarcely expressed in bamboo shoots and shoot buds, but were detected in the rhizomes and roots. These results suggest that PeINVs may play different roles in the growth of moso bamboo.
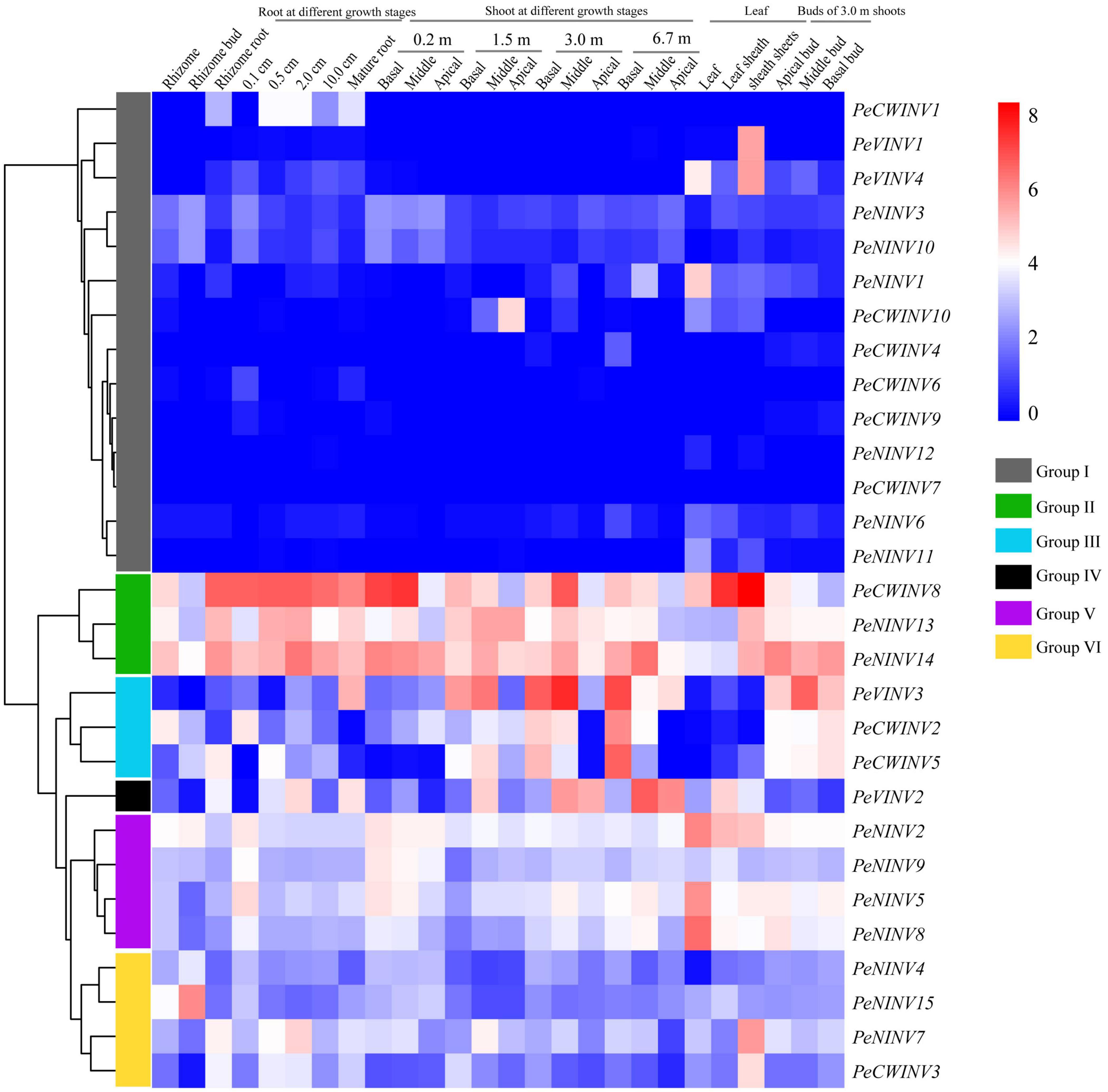
Figure 6. Expression patterns of 29 PeINVs in 26 different tissues based on RNA-seq data of moso bamboo. FPKM-normalized values from RNA sequence data of different tissues in the moso bamboo were used to construct the heatmap. The PeINVs were divided into six groups.
Sugar Content and Expression of PeINVs and SWTGs in Bamboo Leaves Under Drought Stress
The glucose, fructose, and sucrose contents in bamboo leaves were investigated to understand the roles of PeINVs during drought stress (Supplementary Figure 6). The results showed that the glucose and fructose contents gradually increased with the prolonged treatment. The sucrose content also gradually increased, reached a maximum at 4 h, and then decreased at 8 h to a level that was similar to that at 2 h. Further analysis found that the fructose and glucose/sucrose ratio was about 70.0% at 0–4 h, but significantly increased to 93.7% at 8 h. These results indicated that the sugar content might be influenced by drought stress.
The promoter region analysis of PeINVs showed that a large number of cis-acting elements were associated with drought stress (Supplementary Figure 7), indicating that PeINVs might be involved in response to drought stress. INVs have previously been reported to act as significant regulators of water deficit (Ruan et al., 2010), and some studies have shown that SWTGs are induced under drought conditions (Zanor et al., 2009; Kelly et al., 2017). Therefore, the expression patterns of 182 genes in five gene families (29 PeINVs, 36 PeSWEETs, 19 PePLTs, 41 PeSTPs, and 57 PeAQPs) were analyzed using RNA-seq data generated from the leaves of moso bamboo under drought stress. As shown in Supplementary Figure 8, 16 upregulated DEGs (4 PeINVs and 12 SWTGs), were identified using FPKM (|log2 (fold change)| ≥ 1 and p-value < 0.05). The expression patterns of these genes in moso bamboo leaves under drought treatment (20% PEG-6000) were further validated by qRT-PCR.
Overall, all 4 PeINVs and 12 SWTGs were upregulated with different patterns under drought stress (Figure 7). Four genes (PeNINV8, PeSWEET_PH02Gene29130, PePLT_PH02Gene179 09, and PeAQP_PH02Gene33633) continuously upregulated, which were significantly higher at the late stage of stress treatment (4 or 8 h) than those in the control (0 h). The expression of eight genes (PeNINV14, PeCWINV8, PeVINV2, PeSWEET_PH02Gene29130, PePLT_PH02Gene17908, PeSTP_P H02Gene36491, PeSTP_PH02Gene37329, and PeAQP_PH02G ene34465) increased at first, reached their highest expression level at 2 or 4 h and then decreased. In contrast, two water transport genes (PeAQP_PH02Gene16291 and PeAQP_PH02Gene26641) decreased at first and then increased, reaching their highest expression levels at 8 h. In addition, PeSTP_PH02Gene07007 and PeSTP_PH02Gene11741 expressions under drought stress were significantly higher than those in the control group (0 h), but their expression patterns fluctuated. These results suggest that the spatio-temporal expressions of PeINVs and SWTGs might play different roles in moso bamboo leaves under drought stress.
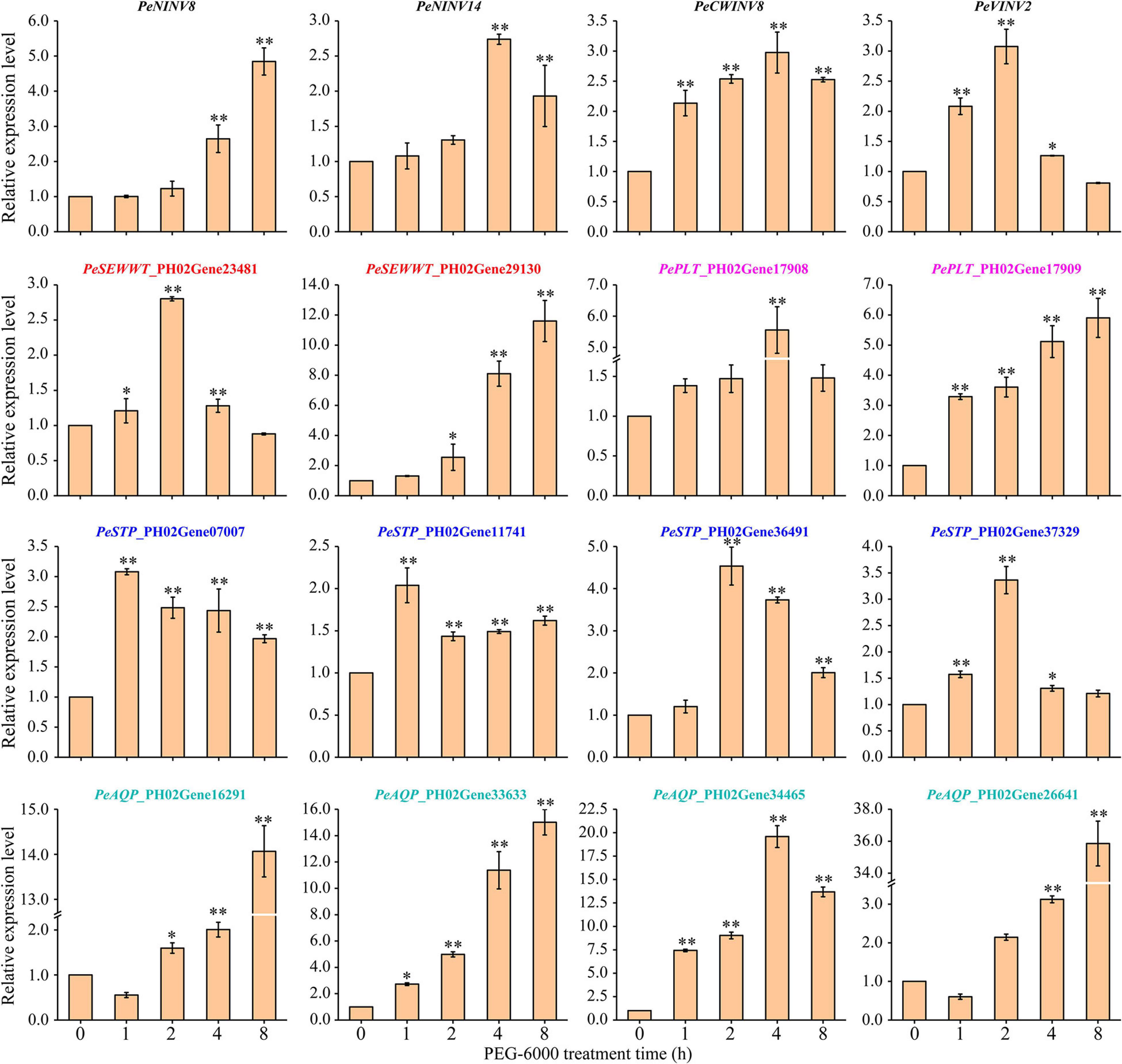
Figure 7. Expression patterns of 4 PeINVs and 12 SWTGs of moso bamboo leaves under drought stress. Fold-change values were used PeTIP41 as internal controls with three technical replicates for each of three biological replicates in the qRT-PCR. The results are expressed as ±SD. The single and double asterisks indicate significant differences at 0.05 and 0.01 levels of the gene expression after different treated times according to student’s t test.
Correlation Analyses of Sugar Contents With the Expression Levels of PeINVs and SWTGs
Based on the results of sugar content measurement and qRT-PCRs analysis, a PCC analysis was conducted. The results showed that the PeNINV8 expression level had a significantly positive correlation with glucose and fructose contents, with PCC values of 0.911 and 0.982, respectively, and PeINV14 and PeCWINV8 were positively correlated with sucrose content, with values of 0.906 and 0.917, respectively. Conversely, PeVINV2 expression level was negatively correlated with glucose and fructose, with values of −0.208 and −0.441, respectively (Figure 8A and Supplementary Table 6). Furthermore, the expression levels of four SWTGs (PeAQP_PH02Gene33633, PeAQP_PH02Gene34465, PePLT_PH02Gene17909, and PeSWEET_PH02Gene29130) also showed positive correlations with sucrose, glucose or fructose contents. In addition, PeINVs were co-expressed with SWTGs, which suggested that they may be key genes related to sugar hydrolysis and transport in moso bamboo (Figure 8B). These results suggest that all of the above genes might respond to drought stress by influencing sugar content through gene expression.
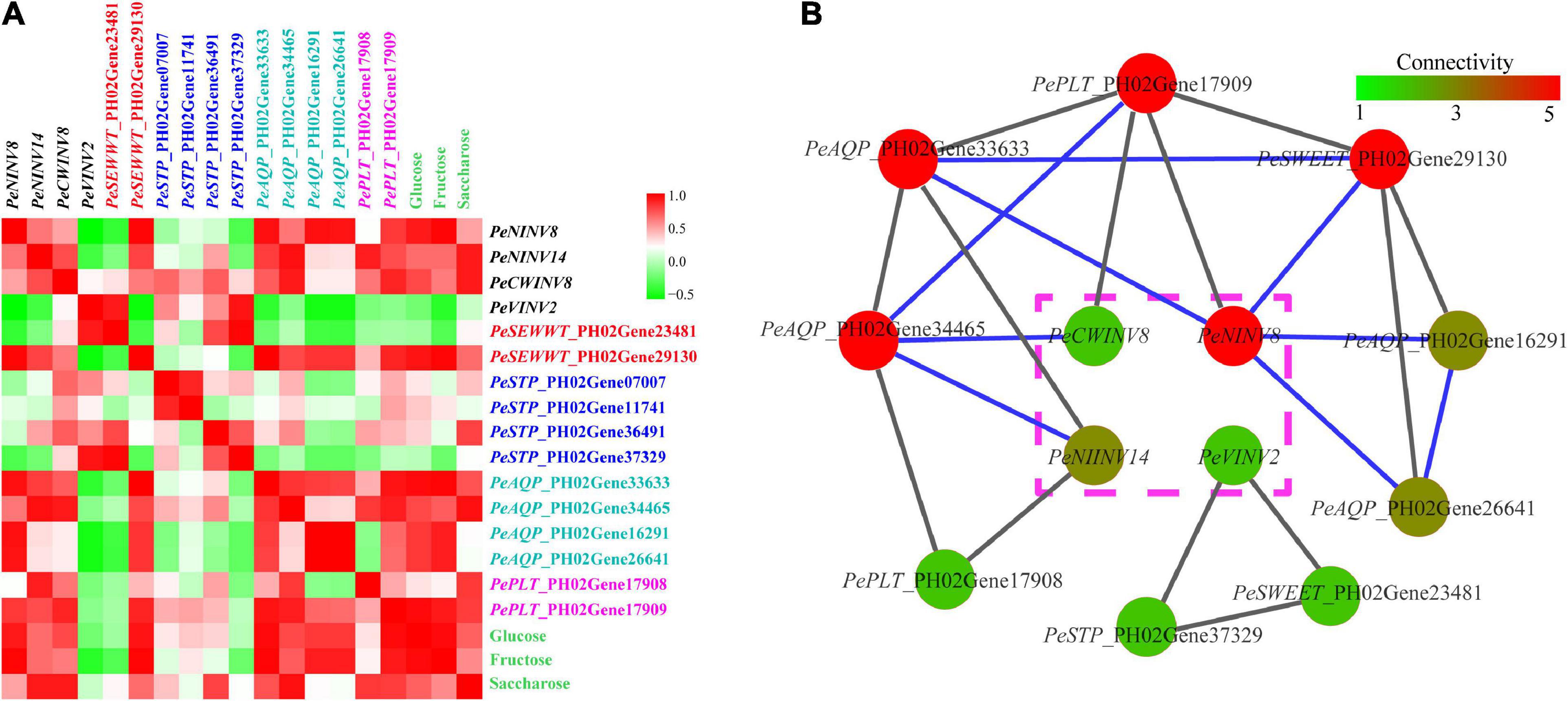
Figure 8. Correlation and co-expression analyses. (A) The correlation analysis of sugar contents with the expression levels of 4 PeINVs and 12 SWTGs. Each correlation is shown by the shades of green to red square shape. The gradual change color of green to red indicate negative to positive correlation. (B) Co-expression network of four PeINVs with nine SWTGs. Gray lines represent PCC magnitude from 0.8 to 0.9, and blue lines represent PCC magnitude from 0.9 to 1.0. The gradual change in the color of nodes represents the difference in the enriched number of co-expressed genes.
Validation of PeINVs Involved in Drought Stress
To validate the function of PeINVs involved in drought stress, PeCWINV8 was selected and overexpressed in yeast cells, followed by PEG-6000 treatment. The result showed that both transgenic yeast cells harboring pYES2:PeCWINV8 and pYES2 vector could grow well on SC-U medium after 10–5 dilution, and two vectors grew slower on SC-U supplemented with 2% PEG-6000 than those on SC-U medium. The cells containing pYES2:PeCWINV8 were able to grow until 10–5 dilution, whereas those containing pYES2 vector only to 10–4 dilution on the SC-U medium supplemented with 1 or 2% PEG-6000 (Figure 9). These results indicated that overexpressed PeCWINV8 can increase the drought tolerance of yeast cells, suggesting that PeCWINV8 may be a key gene related to drought stress in moso bamboo.
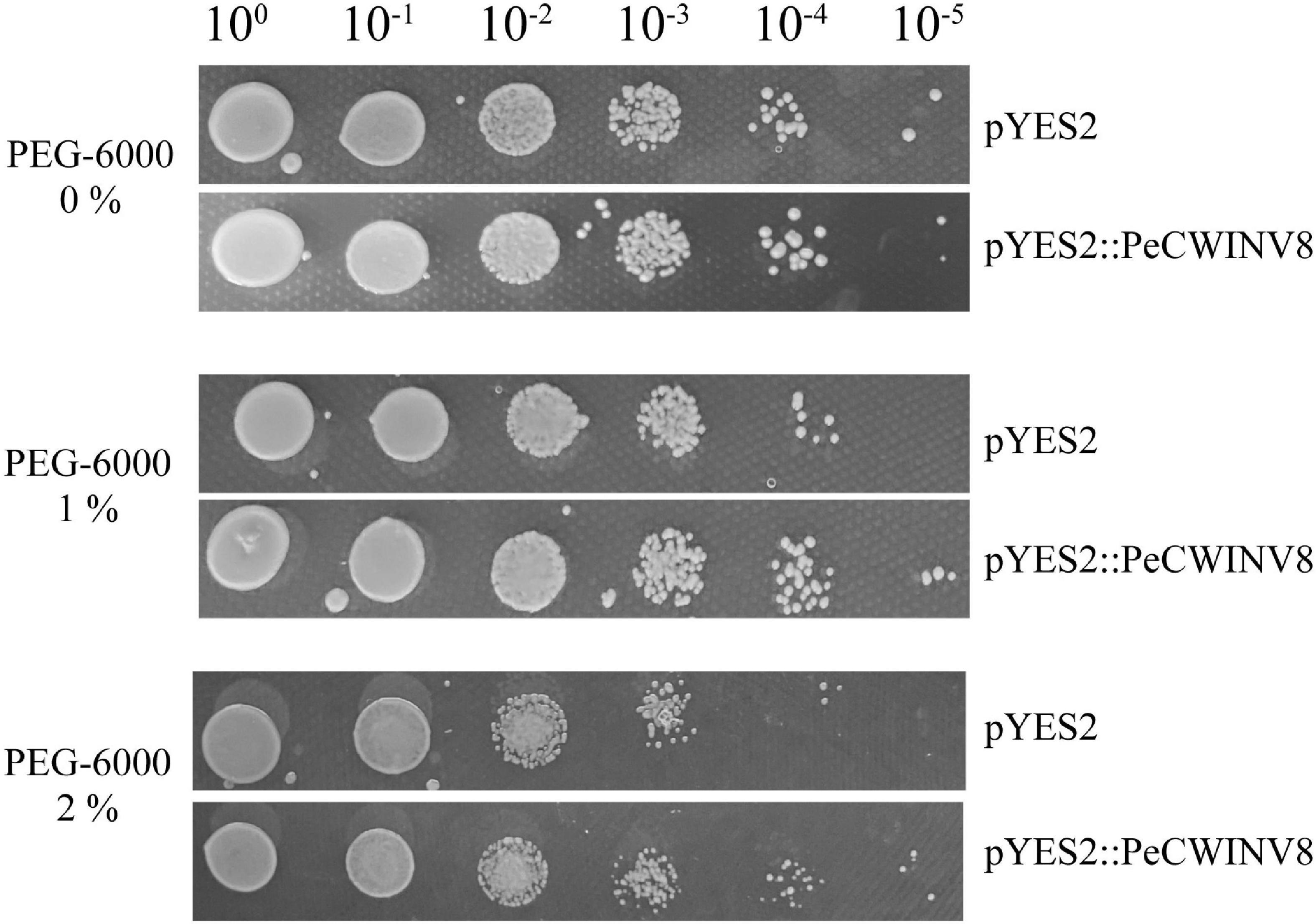
Figure 9. Effect of PeCWNV8 expression on yeast under drought stress. Yeast cells with pYES2 vector only (pYES2) and yeast cells harboring PeCWINV8 expressing construct (pYES2:PeCWINV8) were subjected to 1 or 2% PEG-6000. Cell density adjusted to OD600 at 1.0 and serial dilutions were made at each step. Five microliters of each dilution were inoculated on SC-U medium, SC-U medium supplemented with PEG-6000. Photographs were taken after 2 days of incubation at 30°C.
Discussion
Invertases are common in plants and play essential roles in vegetative development, seed germination, and fruit maturation (Sherson et al., 2003). Sugars and INVs play important roles in reproductive development under drought stress (Ruan et al., 2010). Therefore, a full understanding of the specific characteristics of INVs in different plant species will help us explore this particular function. A previous study reported that PeAINVs are involved in the internode elongation of moso bamboo shoots (Guo et al., 2020). However, no further information is available regarding the INV gene family in moso bamboo based on the updated genome (Zhao et al., 2018). To explore the INV gene family in moso bamboo, a comprehensive analysis of its gene structure, conserved motifs, evolutionary conservation, functional prediction, and expression patterns was conducted in this study. The results provide a basis for further function study of bamboo INVs.
Conservation and Diversity of INVs
Many INVs have been identified in various plants over the past decades, and both monocotyledonous and dicotyledonous diploid plants contain no less than 13 members (Supplementary Table 1). In the present study, 29 members were identified in moso bamboo, which was more than the numbers identified in the diploid plants investigated in this study (19 in A. thaliana, 17 in O. sativa, and 17 in O. latifolia). Gene duplication and divergence play essential roles in the expansion of gene families and novel gene function during evolution (Jaillon et al., 2007). The moso bamboo genome had undergone at least one round of whole-genome duplication, followed by multiple segment duplication. These events are thought to be the reason for the expansion of INV family members during evolution (Peng et al., 2013). Gene duplication and syntenic analyses of INVs supported this assumption. A total of nine segmental duplication pairs were found within the PeINVs, and 25 segmental duplication pairs were detected between PeINVs and OsINVs (Figure 4A). Nevertheless, all 34 duplicated gene pairs had Ka/Ks < 1 (Figure 4B and Supplementary Table 5), indicating that PeINVs had undergone negative selection pressures with limited functional divergence after duplication (Hurst, 2002).
In the AINV sub-family, the PeAINVs contained NDPNG, WECP/VDF, and RDP motifs (Figure 3A and Supplementary Figure 3B). The NDPNG motif is partly encoded by a mini-exons, one of the smallest exons known to date in plants (Bournay et al., 1996), and it contributes the tripeptide DPN to the first conserved motif of AINVs in moso bamboo (Figure 3B). The phylogenetic analysis revealed that all AINVs could be separated into VINV and CWINV subgroups (Figure 5A and Supplementary Figure 5). The WECP/VDF motif contains two subgroups with different amino acids (a proline in the VINVs and a valine in the CWINVs), which might be responsible for the different enzyme activities (Alberto et al., 2004). The NINVs in bamboo could be classified into α and β branches based on 68 NINVs from six plant species (Figure 5B), which was consistent with the evolutionary characteristics of INVs (Roitsch and González, 2004). The PeNINVs in the α branch had similar protein lengths and had the same number of exons (Figure 2). However, the PeNINVs in the β branch were distinct from those in the α branch and formed two subgroups (Figure 5B; Pan et al., 2019). The diversity of PeINVs suggests that they might have undergone different evolutionary selections in moso bamboo.
Multiple Functions of INVs Involved in Plants Growth and Development
The INVs are essential for plant growth and development (Ruan, 2014). Recent studies have shown that CWINVs play a critical role in flowering (Zanor et al., 2009), and seed and fruit development (Li et al., 2012; Wang and Ruan, 2012). Furthermore, VINVs are essential for hexose accumulation (Yamada et al., 2007) and cell expansion (Wang et al., 2014). High INV activity could result in the generation of a sugar concentration gradient in the phloem to facilitate sucrose unloading from source organs to sink organs (Qian et al., 2016). In maize (Z. mays), CWINVs have been implicated in early floral development, especially ovary expansion and seed filling (Cheng et al., 1996; Chourey et al., 2012). The detailed expression patterns for INVs have been studied in many other plant species (Ji et al., 2005; Bezerra-Neto et al., 2019), but the possible function of PeINVs remains unclear. Gene expression patterns are an important manifestation of gene functions. Multiple expression patterns for PeINVs were observed in different tissues of moso bamboo based on the RNA-seq data (Figure 6), indicating that they had different functions.
PeNINV13 and PeNINV14 were expressed in all tissues and were highly expressed in shoots and roots during the growth stage. This result was consistent with the homologous NINV gene in rice, which has been reported to play a role in carbon and energy supply during early root development (Yao et al., 2009). The expression levels of PeCWINV2, PeCWINV5, and PeVINV2 were higher in immature shoots and buds than in other tissues, indicating that they have a potential role in tissue growth and development (Dahro et al., 2016). PeCWINV1 and PeVINV1 were significantly expressed in the roots or sheath sheets, and might be involved in cell wall modification (Ru et al., 2017). In contrast, the expression of some PeINVs was not detected at any stage, suggesting that they might be pseudogenes, are functionally redundant, or might be expressed in other specific tissues or under particular conditions (Wang and Ruan, 2012; Tauzin et al., 2014). PeCWINV4, PeCWINV6, PeCWINV7, PeCWINV9, and PeNINV12 expressions were almost undetectable in 26 tissues. These results indicate that the 29 PeINVs might function differently in moso bamboo. In addition, the PeNINV genes were more abundant than the PeAINV genes, suggesting that PeNINVs might play more significant roles in moso bamboo growth.
INVs Combined With Multiple Genes Involved in Response to Drought Stress
It has been reported that a double subcellular location gene (PtrA/NINV) can be upregulated by dehydration, cold, and salt stress (Dahro et al., 2016). A/N-Invs might help maintain the balance of reactive oxygen species (ROS) in mitochondria. Alternatively, they may or reduce oxidative damage and maintain the balance between adenosine diphosphate (ADP) and adenosine triphosphate (ATP) production under oxidative stress, most likely by delivering glucose as a substrate to hexokinase (Xiang et al., 2011). A previous study showed that sugar transporter pathway genes might play important roles in the response to stress (Wang et al., 2013). Sucrose is cleaved into two hexose molecules by VINVs, which facilitates water flow by doubling the osmotic action (Ruan et al., 2010). Hexokinase can influence sugar levels by regulating water conductance controlled by the expression of AQPs (Kelly et al., 2017; Zhang et al., 2019). Furthermore, INVs are also affected by other genes (such as BFN1, PLD1, and CCP1) in response to drought stress (McLaughlin and Boyer, 2004; Ruan et al., 2010). The RNA-seq and qRT-PCR results showed that the expression levels of the four PeINVs were significantly affected by drought stress (Supplementary Figure 8 and Figure 7). The upregulated expression of PeINVs in response to drought stress was probably due to more INVs being required to cleave sucrose into hexose sugars, which subsequently provided cells with more energy to sustain increased respiration activity (Sturm, 1999). The sugar content changes in moso bamboo leaves and overexpressed PeCWINV8 in yeast cells under drought stress all supported this assumption (Supplementary Figure 6 and Figure 9).
Twelve SWTGs were detected as positive factors in response to drought stress (Figure 7). Nevertheless, gene expression is a dynamic process, and it is possible that most genes are transcriptionally activated only at a particular time point until the enzymes exert their functions (Dubrovina et al., 2013). Thus, the different expression patterns of the 12 SWTGs indicated that they might perform their function spatiotemporally under drought stress. Together, both the gene expression patterns and the sugar content changes could further improve our understanding about the co-expression network between PeINVs and sugar signaling pathways under drought stress (Wang et al., 2017). The co-expression network showed that four PeINVs co-expressed with nine SWTGs (Figure 8 and Supplementary Table 6), in which PeNINV8 and PeNINV14 may contribute the most to the NINV family when responding to drought stress. These results indicate that PeINVs, PeAQPs, and sugar transporters might work together in response to drought stress by modulating cellular osmotic potential (Ruan et al., 2010). However, the precise regulatory mechanisms of the PeINV and SWTG cascade in moso bamboo under drought stress require further investigation.
Conclusion
This study has identified 29 PeINVs in moso bamboo. A sequence comparison of the paralogous gene analyses revealed that PeINVs had been subjected to stronger functional constraints during evolution. The PeINVs were both relatively conservative and diverse, with different expression patterns in different tissues and at different moso bamboo growth stages. Based on the sugar content correlation analyses and the expression levels of PeINVs and SWTGs in moso bamboo leaves, we propose that PeINVs might positively work together with SWTGs in response to drought stress. Drought regulation in plants is a complex network, and more work is required to define the function of PeINVs and elucidate their molecular mechanisms in moso bamboo under drought stress.
Data Availability Statement
The datasets presented in this study can be found in online repositories. The names of the repository/repositories and accession number(s) can be found in the article/Supplementary Material.
Author Contributions
ZG conceived and designed the experiments. CZ performed the experiments. KY analyzed the data. GL and YL contributed reagents, materials, and analysis tools. CZ, KY, and ZG wrote the manuscript. All authors contributed to the article and approved the submitted version.
Funding
This work was funded by the special fund for fundamental scientific research on professional work from International Center for Bamboo and Rattan (No. 1632020004) and the National Natural Science Foundation of China (Grant No. 31971736).
Conflict of Interest
The authors declare that the research was conducted in the absence of any commercial or financial relationships that could be construed as a potential conflict of interest.
Publisher’s Note
All claims expressed in this article are solely those of the authors and do not necessarily represent those of their affiliated organizations, or those of the publisher, the editors and the reviewers. Any product that may be evaluated in this article, or claim that may be made by its manufacturer, is not guaranteed or endorsed by the publisher.
Supplementary Material
The Supplementary Material for this article can be found online at: https://www.frontiersin.org/articles/10.3389/fgene.2021.696300/full#supplementary-material
Abbreviations
ADP, adenosine diphosphate; AINV, acid invertase; AQP, aquaporin; ATP, adenosine triphosphate; AtINVs, Arabidopsis thaliana invertase genes; BaINVs, Bonia amplexicaulis invertase genes; BFN1, bifunctional nuclease 1; bHLH, basic Helix–Loop–Helix transcription factor; BLASTP, basic local alignment tool for protein; CCP1, cysteine protease 1; cDNA, complementary deoxyribonucleic acid; CWINV, cell-wall invertase; DEG, differentially expressed gene; FPKM, fragments per kilobase of transcript per million fragments mapped; FW, fresh weight; GFF, generic file format; HMM, hidden Markov model; INV, invertase; Ka, non-synonymous substitution; kDa, kilodalton; Ks, synonymous substitution; LEA, late embryogenesis abundant; NADPH, nicotinamide adenine dinucleotide phosphate; NINV, alkaline/neutral invertase; OsINVs, Oryza sativa invertase genes; OlINVs, Olyra latifolia invertase genes; PCC, Pearson’s correlation coefficient; PeAINVs, Phyllostachys edulis acid invertase genes; PeCWINVs, Phyllostachys edulis cell-wall invertase genes; PEG-6000, polyethylene glycol-6000; PeNINVs, Phyllostachys edulis alkaline/neutral invertase genes; PeINVs, Phyllostachys edulis invertase genes; PeVINVs, Phyllostachys edulis vacuole invertase genes; pI, theoretical isoelectric point; PLD1, phospholipase D; PLT, polyol/monosaccharide transporter; PtrINVs, Populus trichocarpa invertase genes; qRT-PCR, quantitative real-time PCR; ROS, reactive oxygen species; SC-U, SC dropout medium without uracil; SMART, simple modular architecture research tool; STP, sugar transporter protein; SuSy, sucrose synthase; SWEET, sugars will eventually be exported transporter; SWTGs, sugar and water transport genes; UGE, UDP-galactose-4-epimerase; VINV, vacuole invertase; YPD, Yeast Extract Peptone Dextrose Medium; ZEP, zeaxanthin epoxidase.
Footnotes
- ^ http://bamboo.bamboogdb.org/#/
- ^ http://pfam.xfam.org/
- ^ http://smart.embl-heidelberg.de/
- ^ http://gsds.cbi.pku.edu.cn/
- ^ https://web.expasy.org/protparam/
- ^ http://meme-suite.org/tools/meme
- ^ http://bioinformatics.psb.ugent.be/webtools/plantcare/html/
- ^ http://www.bamboogdb.org
References
Alavilli, H., Awasthi, J. P., Rout, G. R., Sahoo, L., Lee, B. H., and Panda, S. K. (2016). Overexpression of a Barley aquaporin gene, HvPIP2;5 confers salt and osmotic stress tolerance in yeast and plants. Front. Plant Sci. 7:1566. doi: 10.3389/fpls.2016.01566
Alberto, F., Bignon, C., Sulzenbacher, G., Henrissat, B., and Czjzek, M. (2004). The three-dimensional structure of invertase (beta-fructosidase) from Thermotoga maritima reveals a bimodular arrangement and an evolutionary relationship between retaining and inverting glycosidases. J. Biol. Chem. 279, 18903–18910. doi: 10.1074/jbc.M313911200
Andersen, M. N., Asch, F., Wu, Y., Jensen, C. R., Naested, H., Mogensen, V. O., et al. (2002). Soluble invertase expression is an early target of drought stress during the critical, abortion-sensitive phase of young ovary development in maize. Plant Physiol. 130, 591–604. doi: 10.1104/pp.005637
Barratt, D. H. P., Derbyshire, P., Findlay, K., Pike, M., Wellner, N., Lunn, J., et al. (2009). Normal growth of arabidopsis requires cytosolic invertase but not sucrose synthase. Proc. Natl. Acad. Sci. U. S. A. 106, 13124–13129. doi: 10.1073/pnas.0900689106
Bezerra-Neto, J. P., de Araujo, F. C., Ferreira-Neto, J. R. C., da Silva, M. D., Pandolfi, V., Aburjaile, F. F., et al. (2019). Plant aquaporins: diversity, evolution and biotechnological applications. Curr. Protein Pept. Sci. 20, 368–395. doi: 10.2174/1389203720666181102095910
Bihmidine, S., Hunter, C. T., Johns, C. E., Koch, K. E., and Braun, D. M. (2013). Regulation of assimilate import into sink organs: update on molecular drivers of sink strength. Front. Plant Sci. 4:177. doi: 10.3389/fpls.2013.00177
Bournay, A. S., Hedley, P. E., Maddison, A., Waugh, R., and Machray, G. C. (1996). Exon skipping induced by cold stress in a potato invertase gene transcript. Nucleic Acids Res. 24, 2347–2351. doi: 10.1093/nar/24.12.2347
Brown, P., Baxter, L., Hickman, R., Beynon, J., Moore, J. D., and Ott, S. (2013). MEME-LaB: motif analysis in clusters. Bioinformatics 29, 1696–1697. doi: 10.1093/bioinformatics/btt248
Canam, T., Unda, F., and Mansfield, S. D. (2008). Heterologous expression and functional characterization of two hybrid poplar cell-wall invertases. Planta 228, 1011–1019. doi: 10.1007/s00425-008-0801-6
Chen, C., Chen, H., Zhang, Y., Thomas, H. R., Frank, M. H., He, Y., et al. (2020). TBtools: an integrative toolkit developed for interactive analyses of big biological data. Mol. Plant 13, 1194–1202. doi: 10.1016/j.molp.2020.06.009
Chen, Z., Gao, K., Su, X., Rao, P., and An, X. (2015). Genome-wide identification of the invertase gene family in Populus. PLoS One 10:e0138540. doi: 10.1371/journal.pone.0138540
Cheng, W. H., Taliercio, E. W., and Chourey, P. S. (1996). The miniature1 seed locus of maize encodes a cell wall invertase required for normal development of endosperm and maternal cells in the pedicel. Plant Cell 8, 971–983. doi: 10.1105/tpc.8.6.971
Cheng, X., Xiong, R., Liu, H., Wu, M., Chen, F., Hanwei, Y., et al. (2018). Basic helix-loop-helix gene family: genome wide identification, phylogeny, and expression in moso bamboo. Plant Physiol. Biochem. 132, 104–119. doi: 10.1016/j.plaphy.2018.08.036
Cho, J. I., Lee, S. K., Ko, S., Kim, H. K., Jun, S. H., Lee, Y. H., et al. (2005). Molecular cloning and expression analysis of the cell-wall invertase gene family in rice (Oryza sativa L.). Plant Cell Rep. 24, 225–236. doi: 10.1007/s00299-004-0910-z
Chourey, P. S., Jain, M., Li, Q. B., and Carlson, S. J. (2006). Genetic control of cell wall invertases in developing endosperm of maize. Planta 223, 159–167. doi: 10.1007/s00425-005-0039-5
Chourey, P. S., Li, Q. B., and Cevallos-Cevallos, J. (2012). Pleiotropy and its dissection through a metabolic gene Miniature1 (Mn1) that encodes a cell wall invertase in developing seeds of maize. Plant Sci. 184, 45–53. doi: 10.1016/j.plantsci.2011.12.011
Dahro, B., Wang, F., Peng, T., and Liu, J. H. (2016). PtrA/NINV, an alkaline/neutral invertase gene of Poncirus trifoliata, confers enhanced tolerance to multiple abiotic stresses by modulating ROS levels and maintaining photosynthetic efficiency. BMC Plant Biol. 16:76. doi: 10.1186/s12870-016-0761-0
Dubrovina, A. S., Kiselev, K. V., and Khristenko, V. S. (2013). Expression of calcium-dependent protein kinase (CDPK) genes under abiotic stress conditions in wild-growing grapevine Vitis amurensis. J. Plant Physiol. 170, 1491–1500. doi: 10.1016/j.jplph.2013.06.014
Fan, C., Ma, J., Guo, Q., Li, X., Wang, H., and Lu, M. (2013). Selection of reference genes for quantitative real-time PCR in bamboo (Phyllostachys edulis). PLoS One 8:e56573. doi: 10.1371/journal.pone.0056573
Finn, R. D., Bateman, A., Clements, J., Coggill, P., Eberhardt, R. Y., Eddy, S. R., et al. (2014). Pfam: the protein families database. Nucleic Acids Res. 2014, D222–D230. doi: 10.1093/nar/gkt1223
Francki, M. G., Walker, E., Forster, J. W., Spangenberg, G., and Appels, R. (2006). Fructosyltransferase and invertase genes evolved by gene duplication and rearrangements: rice, perennial ryegrass, and wheat gene families. Genome 49, 1081–1091. doi: 10.1139/g06-066
Gasteiger, E., Gattiker, A., Hoogland, C., Ivanyi, I., Appel, R. D., and Bairoch, A. (2003). ExPASy: the proteomics server for in-depth protein knowledge and analysis. Nucleic Acids Res. 31, 3784–3788. doi: 10.1093/nar/gkg563
Guo, X., Chen, H., Liu, Y., Chen, W., Ying, Y., Han, J., et al. (2020). The acid invertase gene family are involved in internode elongation in Phyllostachys heterocycla cv. pubescens. Tree Physiol. 40, 1217–1231. doi: 10.1093/treephys/tpaa053
Hu, B., Jin, J., Guo, A. Y., Zhang, H., Luo, J., and Gao, G. (2015). GSDS 2.0: an upgraded gene feature visualization server. Bioinformatics 31, 1296–1297. doi: 10.1093/bioinformatics/btu817
Huang, Z., Jin, S. H., Guo, H. D., Zhong, X. J., He, J., Li, X., et al. (2016a). Genome-wide identification and characterization of TIFY family genes in Moso Bamboo (Phyllostachys edulis) and expression profiling analysis under dehydration and cold stresses. PeerJ. 4:e2620. doi: 10.7717/peerj.2620
Huang, Z., Zhong, X. J., He, J., Jin, S. H., Guo, H. D., Yu, X. F., et al. (2016b). Genome-wide identification, characterization, and stress-responsive expression profiling of genes encoding LEA (Late Embryogenesis Abundant) proteins in moso bamboo (Phyllostachys edulis). PLoS One 11:e0165953. doi: 10.1371/journal.pone.0165953
Hummel, M., Rahmani, F., Smeekens, S., and Hanson, J. (2009). Sucrose-mediated translational control. Ann. Bot. 104, 1–7. doi: 10.1093/aob/mcp086
Hurst, L. D. (2002). The Ka/Ks ratio: diagnosing the form of sequence evolution. Trends Genet. 18:486. doi: 10.1016/s0168-9525(02)02722-1
Itoh, T., Akao, S., Hashimoto, W., Mikami, B., and Murata, K. (2004). Crystal structure of unsaturated glucuronyl hydrolase, responsible for the degradation of glycosaminoglycan, from Bacillus sp. GL1 at 1.8 Å resolution. J. Biol. Chem. 279, 31804–31812. doi: 10.1074/jbc.M403288200
Jaillon, O., Aury, J. M., Noel, B., Policriti, A., Clepet, C., Casagrande, A., et al. (2007). The grapevine genome sequence suggests ancestral hexaploidization in major angiosperm phyla. Nature 449, 463–467. doi: 10.1038/nature06148
Ji, X., Van den Ende, W., Van Laere, A., Cheng, S., and Bennett, J. (2005). Structure, evolution, and expression of the two invertase gene families of rice. J. Mol. Evol. 60, 615–634. doi: 10.1007/s00239-004-0242-1
Kelly, G., Sade, N., Doron-Faigenboim, A., Lerner, S., Shatil-Cohen, A., Yeselson, Y., et al. (2017). Sugar and hexokinase suppress expression of PIP aquaporins and reduce leaf hydraulics that preserves leaf water potential. Plant J. 91, 325–339. doi: 10.1111/tpj.13568
Kim, J. Y., Mahé, A., Brangeon, J., and Prioul, J. L. (2000). A maize vacuolar invertase, IVR2, is induced by water stress. Organ/tissue specificity and diurnal modulation of expression. Plant Physiol. 124, 71–84. doi: 10.1104/pp.124.1.71
Koch, K. (2004). Sucrose metabolism: regulatory mechanisms and pivotal roles in sugar sensing and plant development. Curr. Opin. Plant Biol. 7, 235–246. doi: 10.1016/j.pbi.2004.03.014
Koch, K. E., Wu, Y., and Xu, J. (1996). Sugar and metabolic regulation of genes for sucrose metabolism: potential influence of maize sucrose synthase and soluble invertase responses on carbon partitioning and sugar sensing. J. Exp. Bot. 47, 1179–1185. doi: 10.1093/jxb/47.Special_Issue.1179
Krzywinski, M., Schein, J., Birol, I., Connors, J., Gascoyne, R., Horsman, D., et al. (2009). Circos: an information aesthetic for comparative genomics. Genome Res. 19, 1639–1645. doi: 10.1101/gr.092759.109
Kumar, S., Stecher, G., and Tamura, K. (2016). MEGA7: molecular evolutionary genetics analysis version 7.0 for bigger datasets. Mol. Biol. Evol. 33, 1870–1874. doi: 10.1093/molbev/msw054
Lammens, W., Le Roy, K., Schroeven, L., Van Laere, A., Rabijns, A., and Van den Ende, W. (2009). Structural insights into glycoside hydrolase family 32 and 68 enzymes: functional implications. J. Exp. Bot. 60, 727–740. doi: 10.1093/jxb/ern333
Lescot, M., Déhais, P., Thijs, G., Marchal, K., Moreau, Y., Van de Peer, Y., et al. (2002). PlantCARE, a database of plant cis-acting regulatory elements and a portal to tools for in silico analysis of promoter sequences. Nucleic Acids Res. 30, 325–327. doi: 10.1093/nar/30.1.325
Letunic, I., Doerks, T., and Bork, P. (2012). SMART 7: recent updates to the protein domain annotation resource. Nucleic Acids Res. 40, D302–D305. doi: 10.1093/nar/gkr931
Li, L., Mu, S., Cheng, Z., Cheng, Y., Zhang, Y., Miao, Y., et al. (2017). Characterization and expression analysis of the WRKY gene family in moso bamboo. Sci. Rep. 7:6675. doi: 10.1038/s41598-017-06701-2
Li, Y., and Feng, P. (2019). ∗Bamboo resources in china based on the ninth national forest inventory data. World Bamboo and Rattan 17, 45–48. doi: 10.12168/sjzttx.2019.06.010
Li, Z., Palmer, W. M., Martin, A. P., Wang, R., Rainsford, F., Jin, Y., et al. (2012). High invertase activity in tomato reproductive organs correlates with enhanced sucrose import into, and heat tolerance of, young fruit. J. Exp. Bot. 63, 1155–1166. doi: 10.1093/jxb/err329
Liu, J., Han, L., Huai, B., Zheng, P., Chang, Q., Guan, T., et al. (2015). Down-regulation of a wheat alkaline/neutral invertase correlates with reduced host susceptibility to wheat stripe rust caused by Puccinia striiformis. J. Exp. Bot. 66, 7325–7338. doi: 10.1093/jxb/erv428
Livak, K. J., and Schmittgen, T. D. (2001). Analysis of relative gene expression data using real-time quantitative PCR and the 2 (-Delta Delta C (T)) Method. Methods 25, 402–408. doi: 10.1006/meth.2001.1262
Lou, Y., Gou, J. Y., and Xue, H. W. (2007). PIP5K9, an arabidopsis phosphatidylinositol monophosphate kinase, interacts with a cytosolic invertase to negatively regulate sugar-mediated root growth. Plant Cell 19, 163–181. doi: 10.1105/tpc.106.045658
Lou, Y., Sun, H., Li, L., Zhao, H., and Gao, Z. (2017). Characterization and primary functional analysis of a bamboo ZEP gene from Phyllostachys edulis. DNA Cell Biol. 36, 747–758. doi: 10.1089/dna.2017.3705
Martín, M. L., Lechner, L., Zabaleta, E. J., and Salerno, G. L. (2013). A mitochondrial alkaline/neutral invertase isoform (A/N-InvC) functions in developmental energy-demanding processes in arabidopsis. Planta 237, 813–822. doi: 10.1007/s00425-012-1794-8
McLaughlin, J. E., and Boyer, J. S. (2004). Sugar-responsive gene expression, invertase activity, and senescence in aborting maize ovaries at low water potentials. Ann. Bot. 94, 675–689. doi: 10.1093/aob/mch193
Nonis, A., Ruperti, B., Pierasco, A., Canaguier, A., Adam-Blondon, A. F., Di Gaspero, G., et al. (2008). Neutral invertases in grapevine and comparative analysis with arabidopsis, poplar and rice. Planta 229, 129–142. doi: 10.1007/s00425-008-0815-0
Pan, L., Guo, Q., Chai, S., Cheng, Y., Ruan, M., Ye, Q., et al. (2019). Evolutionary conservation and expression patterns of neutral/alkaline invertases in solanum. Biomolecules 9:763. doi: 10.3390/biom9120763
Peng, Z., Lu, Y., Li, L., Zhao, Q., Feng, Q., Gao, Z., et al. (2013). The draft genome of the fast-growing non-timber forest species moso bamboo (Phyllostachys heterocycla). Nat. Genet. 45, e1–e2. doi: 10.1038/ng.2569
Proels, R. K., and Hückelhoven, R. (2014). Cell-wall invertases, key enzymes in the modulation of plant metabolism during defence responses. Mol. Plant Pathol. 15, 858–864. doi: 10.1111/mpp.12139
Qian, W., Yue, C., Wang, Y., Cao, H., Li, N., Wang, L., et al. (2016). Identification of the invertase gene family (INVs) in tea plant and their expression analysis under abiotic stress. Plant Cell Rep. 35, 2269–2283. doi: 10.1007/s00299-016-2033-8
Rende, U., Wang, W., Gandla, M. L., Jönsson, L. J., and Niittylä, T. (2017). Cytosolic invertase contributes to the supply of substrate for cellulose biosynthesis in developing wood. New Phytol. 214, 796–807. doi: 10.1111/nph.14392
Ritchie, M. E., Phipson, B., Wu, D., Hu, Y., Law, C. W., Shi, W., et al. (2015). limma powers differential expression analyses for RNA-sequencing and microarray studies. Nucleic Acids Res. 43:e47. doi: 10.1093/nar/gkv007
Roitsch, T., and González, M. C. (2004). Function and regulation of plant invertases: sweet sensations. Trends Plant Sci. 9, 606–613. doi: 10.1016/j.tplants.2004.10.009
Ru, L., Osorio, S., Wang, L., Fernie, A. R., Patrick, J. W., and Ruan, Y. L. (2017). Transcriptomic and metabolomics responses to elevated cell wall invertase activity during tomato fruit set. J. Exp. Bot. 68, 4263–4279. doi: 10.1093/jxb/erx219
Ruan, Y. L. (2014). Sucrose metabolism: gateway to diverse carbon use and sugar signaling. Annu. Rev. Plant Biol. 65, 33–67. doi: 10.1146/annurev-arplant-050213-040251
Ruan, Y. L., Jin, Y., Yang, Y. J., Li, G. J., and Boyer, J. S. (2010). Sugar input, metabolism, and signaling mediated by invertase: roles in development, yield potential, and response to drought and heat. Mol. Plant 3, 942–955. doi: 10.1093/mp/ssq044
Salerno, G. L., and Curatti, L. (2003). Origin of sucrose metabolism in higher plants: when, how and why? Trends Plant Sci. 8, 63–69. doi: 10.1016/S1360-1385(02)00029-8
Schober, P., Boer, C., and Schwarte, L. A. (2018). Correlation coefficients: appropriate use and interpretation. Anesth. Analg. 126, 1763–1768. doi: 10.1213/ANE.0000000000002864
Shannon, P., Markiel, A., Ozier, O., Baliga, N. S., Wang, J. T., Ramage, D., et al. (2003). Cytoscape: a software environment for integrated models of biomolecular interaction networks. Genome Res. 13, 2498–2504. doi: 10.1101/gr.1239303
Shen, L. B., Qin, Y. L., Qi, Z. Q., Niu, Y., Liu, Z. J., Liu, W. X., et al. (2018a). Genome-wide analysis, expression profile, and characterization of the acid invertase gene family in pepper. Int. J. Mol. Sci. 20:15. doi: 10.3390/ijms20010015
Shen, L. B., Yao, Y., He, H., Qin, Y. L., Liu, Z. J., Liu, W. X., et al. (2018b). Genome-wide identification, expression, and functional analysis of the alkaline/neutral invertase gene family in pepper. Int. J. Mol. Sci. 19:224. doi: 10.3390/ijms19010224
Sherson, S. M., Alford, H. L., Forbes, S. M., Wallace, G., and Smith, S. M. (2003). Roles of cell-wall invertases and monosaccharide transporters in the growth and development of arabidopsis. J. Exp. Bot. 54, 525–531. doi: 10.1093/jxb/erg055
Stitt, M., von Schaewen, A., and Willmitzer, L. (1991). “Sink” regulation of photosynthetic metabolism in transgenic tobacco plants expressing yeast invertase in their cell wall involves a decrease of the Calvin-cycle enzymes and an increase of glycolytic enzymes. Planta 183, 40–50. doi: 10.1007/BF00197565
Sturm, A. (1999). Invertases. Primary structures, functions, and roles in plant development and sucrose partitioning. Plant Physiol. 121, 1–8. doi: 10.1104/pp.121.1.1
Sturm, A., and Chrispeels, M. J. (1990). cDNA cloning of carrot extracellular beta-fructosidase and its expression in response to wounding and bacterial infection. Plant Cell 2, 1107–1119. doi: 10.1105/tpc.2.11.1107
Sun, H., Li, L., Lou, Y., Zhao, H., Yang, Y., and Gao, Z. (2016). Cloning and preliminary functional analysis of PeUGE gene from moso bamboo (Phyllostachys edulis). DNA Cell Biol. 35, 706–714. doi: 10.1089/dna.2016.3389
Sun, H., Li, L., Lou, Y., Zhao, H., Yang, Y., Wang, S., et al. (2017). The bamboo aquaporin gene PeTIP4,1-1 confers drought and salinity tolerance in transgenic arabidopsis. Plant Cell Rep. 36, 597–609. doi: 10.1007/s00299-017-2106-3
Sun, H., Wang, S., Lou, Y., Zhu, C., Zhao, H., Li, Y., et al. (2021). A bamboo leaf-specific aquaporin gene PePIP2;7 is involved in abiotic stress response. Plant Cell Rep. 40, 1101–1114. doi: 10.1007/s00299-021-02673-w
Tang, G. Q., Lüscher, M., and Sturm, A. (1999). Antisense repression of vacuolar and cell wall invertase in transgenic carrot alters early plant development and sucrose partitioning. Plant Cell 11, 177–189. doi: 10.1105/tpc.11.2.177
Tauzin, A. S., Sulzenbacher, G., Lafond, M., Desseaux, V., Reca, I. B., Perrier, J., et al. (2014). Functional characterization of a vacuolar invertase from Solanum lycopersicum: post-translational regulation by N-glycosylation and a proteinaceous inhibitor. Biochimie 101, 39–49. doi: 10.1016/j.biochi.2013.12.013
Tiessen, A., and Padilla-Chacon, D. (2012). Subcellular compartmentation of sugar signaling: links among carbon cellular status, route of sucrolysis, sink-source allocation, and metabolic partitioning. Front. Plant Sci. 3:306. doi: 10.3389/fpls.2012.00306
Vargas, W., Cumino, A., and Salerno, G. L. (2003). Cyanobacterial alkaline/neutral invertases. Origin of sucrose hydrolysis in the plant cytosol? Planta 216, 951–960. doi: 10.1007/s00425-002-0943-x
Verhaest, M., Lammens, W., Le Roy, K., De Coninck, B., De Ranter, C. J., Van Laere, A., et al. (2006). X-ray diffraction structure of a cell-wall invertase from Arabidopsis thaliana. Acta Crystallogr. D Biol. Crystallogr. 62, 1555–1563. doi: 10.1107/S0907444906044489
Wang, D., Zhang, Y., Zhang, Z., Zhu, J., and Yu, J. (2010). KaKs_Calculator 2.0: a toolkit incorporating gamma-series methods and sliding window strategies. Genomics Proteomics Bioinformatics 8, 77–80. doi: 10.1016/S1672-0229(10)60008-3
Wang, L., and Ruan, Y. L. (2012). New insights into roles of cell wall invertase in early seed development revealed by comprehensive spatial and temporal expression patterns of GhCWIN1 in cotton. Plant Physiol. 160, 777–787. doi: 10.1104/pp.112.203893
Wang, L., and Ruan, Y. L. (2016). Critical roles of vacuolar invertase in floral organ development and male and female fertilities are revealed through characterization of GhVIN1-RNAi cotton plants. Plant Physiol. 171, 405–423. doi: 10.1104/pp.16.00197
Wang, L., Cook, A., Patrick, J. W., Chen, X. Y., and Ruan, Y. L. (2014). Silencing the vacuolar invertase gene GhVIN1 blocks cotton fiber initiation from the ovule epidermis, probably by suppressing a cohort of regulatory genes via sugar signaling. Plant J. 78, 686–696. doi: 10.1111/tpj.12512
Wang, L., Zheng, Y., Ding, S., Zhang, Q., Chen, Y., and Zhang, J. (2017). Molecular cloning, structure, phylogeny and expression analysis of the invertase gene family in sugarcane. BMC Plant Biol. 17:109. doi: 10.1186/s12870-017-1052-0
Wang, X. C., Zhao, Q. Y., Ma, C. L., Zhang, Z. H., Cao, H. L., Kong, Y. M., et al. (2013). Global transcriptome profiles of Camellia sinensis during cold acclimation. BMC Genomics 14:415. doi: 10.1186/1471-2164-14-415
Wang, Y., Tang, H., Debarry, J. D., Tan, X., Li, J., Wang, X., et al. (2012). MCScanX: a toolkit for detection and evolutionary analysis of gene synteny and collinearity. Nucleic Acids Res. 40:e49. doi: 10.1093/nar/gkr1293
Xiang, L., Le Roy, K., Bolouri-Moghaddam, M. R., Vanhaecke, M., Lammens, W., Rolland, F., et al. (2011). Exploring the neutral invertase-oxidative stress defence connection in Arabidopsis thaliana. J. Exp. Bot. 62, 3849–3862. doi: 10.1093/jxb/err069
Yamada, K., Kojima, T., Bantog, N., Shimoda, T., Mori, H., Shiratake, K., et al. (2007). Cloning of two isoforms of soluble acid invertase of Japanese pear and their expression during fruit development. J. Plant Physiol. 164, 746–755. doi: 10.1016/j.jplph.2006.05.007
Yang, Q., Guo, B., Sun, H., Zhang, J., Liu, S., Hexige, S., et al. (2017). Identification of the key genes implicated in the transformation of OLP to OSCC using RNA-sequencing. Oncol Rep. 37, 2355–2365. doi: 10.3892/or.2017.5487
Yao, S. G., Kodama, R., Wang, H., Ichii, M., Taketa, S., and Yoshida, H. (2009). Analysis of the rice SHORT-ROOT5 gene revealed functional diversification of plant neutral/alkaline invertase family. Plant Sci. 176, 627–634. doi: 10.1016/j.plantsci.2009.02.002
Yu, X., Wang, X., Zhang, W., Qian, T., Tang, G., Guo, Y., et al. (2008). Antisense suppression of an acid invertase gene (MAI1) in muskmelon alters plant growth and fruit development. J. Exp. Bot. 59, 2969–2977. doi: 10.1093/jxb/ern158
Zanor, M. I., Osorio, S., Nunes-Nesi, A., Carrari, F., Lohse, M., Usadel, B., et al. (2009). RNA interference of LIN5 in tomato confirms its role in controlling Brix content, uncovers the influence of sugars on the levels of fruit hormones, and demonstrates the importance of sucrose cleavage for normal fruit development and fertility. Plant Physiol. 150, 1204–1218. doi: 10.1104/pp.109.136598
Zhang, S., Feng, M., Chen, W., Zhou, X., Lu, J., Wang, Y., et al. (2019). In rose, transcription factor PTM balances growth and drought survival via PIP2,1 aquaporin. Nat. Plants 5, 290–299. doi: 10.1038/s41477-019-0376-1
Keywords: Phyllostachys edulis, invertase, gene identification, expression pattern, drought stress
Citation: Zhu C, Yang K, Li G, Li Y and Gao Z (2021) Identification and Expression Analyses of Invertase Genes in Moso Bamboo Reveal Their Potential Drought Stress Functions. Front. Genet. 12:696300. doi: 10.3389/fgene.2021.696300
Received: 16 April 2021; Accepted: 06 August 2021;
Published: 30 August 2021.
Edited by:
Meng Li, Nanjing Agricultural University, ChinaReviewed by:
Hui Song, Qingdao Agricultural University, ChinaLiangliang Gao, Kansas State University, United States
Xiaojun Nie, Northwest A&F University, China
Nadeem Khan, Agriculture and Agri-Food Canada (AAFC), Canada
Copyright © 2021 Zhu, Yang, Li, Li and Gao. This is an open-access article distributed under the terms of the Creative Commons Attribution License (CC BY). The use, distribution or reproduction in other forums is permitted, provided the original author(s) and the copyright owner(s) are credited and that the original publication in this journal is cited, in accordance with accepted academic practice. No use, distribution or reproduction is permitted which does not comply with these terms.
*Correspondence: Zhimin Gao, Z2FvemhpbWluQGljYnIuYWMuY24=
†These authors have contributed equally to this work