Corrigendum: Low-Dose Radiation Can Cause Epigenetic Alterations Associated With Impairments in Both Male and Female Reproductive Cell
- 1Hong Kong Branch of the Southern Marine Science and Engineering Guangdong Laboratory, Hong Kong, China
- 2Department of Chemistry, City University of Hong Kong, Kowloon Tong, Hong Kong
- 3School of Biological Sciences, The University of Hong Kong, Pok Fu Lam, Hong Kong
- 4State Key Laboratory of Marine Pollution, City University of Hong Kong, Kowloon Tong, Hong Kong
- 5Department of Physics, City University of Hong Kong, Kowloon Tong, Hong Kong
- 6School of Life Sciences, Hong Kong Bioinformatics Centre, The Chinese University of Hong Kong, Shatin, Hong Kong
- 7Department of Biology, Hong Kong Baptist University, Kowloon Tsai, Hong Kong
- 8Laboratory of Environmental Pollution and Integrative Omics, Guilin Medical University, Guilin, China
- 9Department of Science and Environmental Studies, The Education University of Hong Kong, Tai Po, Hong Kong
Humans are regularly and continuously exposed to ionizing radiation from both natural and artificial sources. Cumulating evidence shows adverse effects of ionizing radiation on both male and female reproductive systems, including reduction of testis weight and sperm count and reduction of female germ cells and premature ovarian failure. While most of the observed effects were caused by DNA damage and disturbance of DNA repairment, ionizing radiation may also alter DNA methylation, histone, and chromatin modification, leading to epigenetic changes and transgenerational effects. However, the molecular mechanisms underlying the epigenetic changes and transgenerational reproductive impairment induced by low-dose radiation remain largely unknown. In this study, two different types of human ovarian cells and two different types of testicular cells were exposed to low dose of ionizing radiation, followed by bioinformatics analysis (including gene ontology functional analysis and Ingenuity Pathway Analysis), to unravel and compare epigenetic effects and pathway changes in male and female reproductive cells induced by ionizing radiation. Our findings showed that the radiation could alter the expression of gene cluster related to DNA damage responses through the control of MYC. Furthermore, ionizing radiation could lead to gender-specific reproductive impairment through deregulation of different gene networks. More importantly, the observed epigenetic modifications induced by ionizing radiation are mediated through the alteration of chromatin remodeling and telomere function. This study, for the first time, demonstrated that ionizing radiation may alter the epigenome of germ cells, leading to transgenerational reproductive impairments, and correspondingly call for research in this new emerging area which remains almost unknown.
Introduction
Humans are regularly and continuously exposed to ionizing radiation from both natural and artificial sources. Natural background radiation (NBR) may come from radon and its daughter products, crust, cosmic rays, soil, water, food etc. According to the United States report in 2008, the average world population exposure to natural background radiation was about 2.4 millisievert (mSv) per annum, whereas additional radiation contributed from medical diagnosis is estimated at about 0.6 mSv annually (UNSCEAR, 2010). Radiation injuries, such as epithelial and stromal lesion, vascular lesions, fibrosis, and neoplasia may occur upon irradiation (Fajardo, 2005), and level of injuries mainly depends on the radiation dose, duration, and cell cycle stage. In general, male and female gametes are more susceptible to radiation which may affect the composition and biological integrity of their proteins, lipids, and nucleic acids, and hence compromising their capacity to produce normal embryos (García-Rodríguez et al., 2018). Indeed, the adverse effects of ionizing radiation on the reproductive system of mammals (including human being) have been clearly demonstrated by numerous studies. Radiation, for example, has been shown to reduce testis weight and sperm count in male rodent (Haines et al., 2002). In human, a radiation dose as low as 1 Gy can disrupt gonadotropin levels, reduce the number of spermatocytes and spermatids, while a higher radiation dose of 10 Gy can kill all spermatogonial stem cells, leading to permanent azoospermia (Rowley et al., 1974; Clifton and Bremner, 1983). In vivo studies in female rats demonstrated that radiation can reduce a number of germ cells and increase synaptonemal complex (SC) fragmentation (Pujol et al., 1996, 1997). Female cancer patients after irradiation treatments often experience premature ovarian failure, infertility, uterine damage, premature deliveries, and miscarriage (Critchley et al., 1992; Wallace et al., 2003).
At cellular level, ionizing radiation can induce DNA damage: (i) directly, via energy deposition to DNA molecules; and (ii) indirectly, via the attack from reactive oxygen species generated by other ionized molecules, such as free radicals (Ward, 1988). DNA breaks, if failed to repair, can increase the risk of mutagenesis and carcinogenesis (Goodhead, 1994; Ward, 1995; Little, 2000). Haploid germ cells are more susceptible to DNA mutation induced by radiation due to the absence of sister chromatid. DNA repair is thus performed by non-homologous end joining, which is generally accepted as an error-prone pathway (Ahmed et al., 2015; Wdowiak et al., 2019).
Apart from DNA damage, emerging evidence showed that ionizing radiation may further alter DNA methylation pattern (Miousse et al., 2017), modify histone and chromatin structure (Rogakou et al., 1998; Pogribny et al., 2005; Kumar R. et al., 2013), and miRNA expression levels (Halimi et al., 2012; Metheetrairut and Slack, 2013). These induced changes in paternal epigenome can be passed on and affect fertilization and embryogenesis in subsequent generations (Jenkins and Carrell, 2012), thereby causing transgenerational effects. Non-coding RNA is suggested to play a role in epigenetic inheritance by the trafficking of microRNA-containing vesicles to sperm via blood stream (Paris et al., 2015; Grandjean et al., 2016; Sharma et al., 2018; Szatmári et al., 2019). In zebrafish, it has been shown that differential methylation of specific genes in F0 caused by parental radiation exposure can be transmitted to their F3 offspring, which had never been exposed to radiation before throughout their life cycle (Kamstra et al., 2018). Nevertheless, existing knowledge on radiation-induced epigenetics alterations on reproductive system, especially female, are limited. Irradiated (2.5 Gy) mice showed an upregulation of miR-29 family in male germline, resulting in reduced expression of de novo DNA methyltransferase 3a and hypomethylation of interspersed nuclear elements associated to chromatin modification (Filkowski et al., 2010). Increased phosphorylation of histone H2AX (γ-H2AX) was observed in immature spermatozoa of cranially exposed (20 Gy) rats via bystander effect. Significant reduction in global DNA methylation was also found in testes and mature sperm cells (Tamminga et al., 2008). On the contrary, significant increase in global methylation was found in spermatozoa of a human exposed to occupational radiation (Kumar D. et al., 2013). Nevertheless, the epigenetic and transgenerational effects induced by radiation on the reproductive system are still not fully understood, and the underlying mechanisms remained obscure and need further studies (Skrzypek et al., 2019; Dubrova and Sarapultseva, 2020).
Using two different types of human ovarian cells and two different types of testicular cells as models, we carried out a series of in vitro experiments followed by bioinformatics analysis (including gene ontology functional analysis and Ingenuity Pathway Analysis), to unravel and compare epigenetic effects and pathway changes in male and female reproductive cells caused by environmentally relevant dose of ionizing radiation. Specifically, we hypothesize that: (a) ionizing radiation could alter the expression of gene cluster related to DNA damage response, and (b) reproductive impairment caused by ionizing radiation is gender specific and controlled by different gene networks.
Materials and Methods
Ovarian and Testicular Cell Culture and Ionizing Radiation Exposure
Two human ovarian cancer cells (SKOV3 and COV434) and two mouse testicular germ cells (GC-1 and TM4) were cultured under the conditions described in Supplementary Table 1. Only human and mouse cell lines (not primary cells) were used, and these were purchased from an international company. In accordance with the national legislation and the institutional requirements, the Human Research Ethics Committee of The University of Hong Kong waived the requirement for ethical approval and written informed consent for participants in this study. The cells were cultured at 37°C under 95% air and 5% carbon dioxide. For the ionizing radiation exposure, the cells were seeded onto six-well plate 1 day before exposure to 10 cGy of X-ray (320 kV, 2 mA) for 1 min (X-RAD 320 X-ray system).
Cell Viability Test
Cells were seeded in a 96-well plate (24 replicate wells for each treatment). After ionizing radiation exposure, cell viability was measured by the MTT assay (Sigma). Colorimetric reaction was measured at 570 nm.
RNA Isolation
After radiation exposure, total RNA of the cells was extracted using TRIzol Reagent (Invitrogen) according to the manufacturer’s instructions. Briefly, the cells were lysed in 1 ml of TRIzol. Then, 200 μl of chloroform was added, and the sample was centrifuged at 12,000 × g for 15 min. Next, 500 μl of the aqueous phase was mixed with 500 μl of isopropanol and stored in −20°C. After overnight precipitation, the mixture was centrifuged at 12,000 × g for 20 min. The RNA pellet was then washed twice using ice-cold 70% ethanol, followed by resuspension in RNAse-free distilled water. RNA quality was assessed using an Agilent 2100 Bioanalyzer system, and samples with an RNA integrity number (RIN) greater than eight was used for RNA library construction.
RNA Sequencing and Bioinformatics Analysis
RNA (cDNA) libraries (three biological replicates from each treatment) of cells were constructed as previously described (Li et al., 2021) and sequenced by the Beijing Genomics Institute (Wuhan, China). Single-end 50-bp read-length reads were sequenced on a BGISEQ-500RS sequencer. Sequence reads were dynamically trimmed according to the q algorithm of BWA (Li et al., 2021). At least 50 million quality-trimmed reads were obtained in each sample. Quality-trimmed sequence reads were mapped to human genome reference (GRCh38/hg38) for SKOV3 and COV434 cell lines, and mouse genome reference (GRCm39/mm39) for GC-1 and TM4 cell lines. Read-count data was then subjected to differential expression analysis using the edgeR package (Robinson et al., 2010). Genes with FDR < 0.05 were considered differentially expressed genes (DEGs). Furthermore, Gene Ontology (GO) enrichment analysis, Kyoto Encyclopedia of Genes and Genomes (KEGG) pathway analysis, and Ingenuity Pathway Analysis (IPA®, QIAGEN1) were used to decipher the biological effects and possible epigenetic effect of ionizing radiation on the female and male reproductive systems.
Data Availability
Sequencing data of transcriptome sequencing that support the findings of this study have been deposited in the NCBI BioProject database2 with the BioProject accession codes PRJNA730377.
Statistical Analysis
For bioinformatics analysis, all pathways, diseases, or biofunctions with p < 0.05 was considered statistically significant. In MTT assay, results were compared using paired Student’s t-test. All statistical analyses were performed using GraphPad Prism 3.02 (GraphPad Software Inc.), and results with p < 0.05 was considered statistically significant.
Results
Environmental Relevant Dose of Ionizing Radiation Has no Effect on the Viability of the Female and Male Reproductive Cells
MTT assay was employed to determine the cytotoxicity of the tested ionizing radiation (10 cGy). No significant change in cell viability was found after ionizing radiation exposure (Figure 1), suggesting that the level of radiation used in this study had no cytotoxic effect on both female and male reproductive cells.
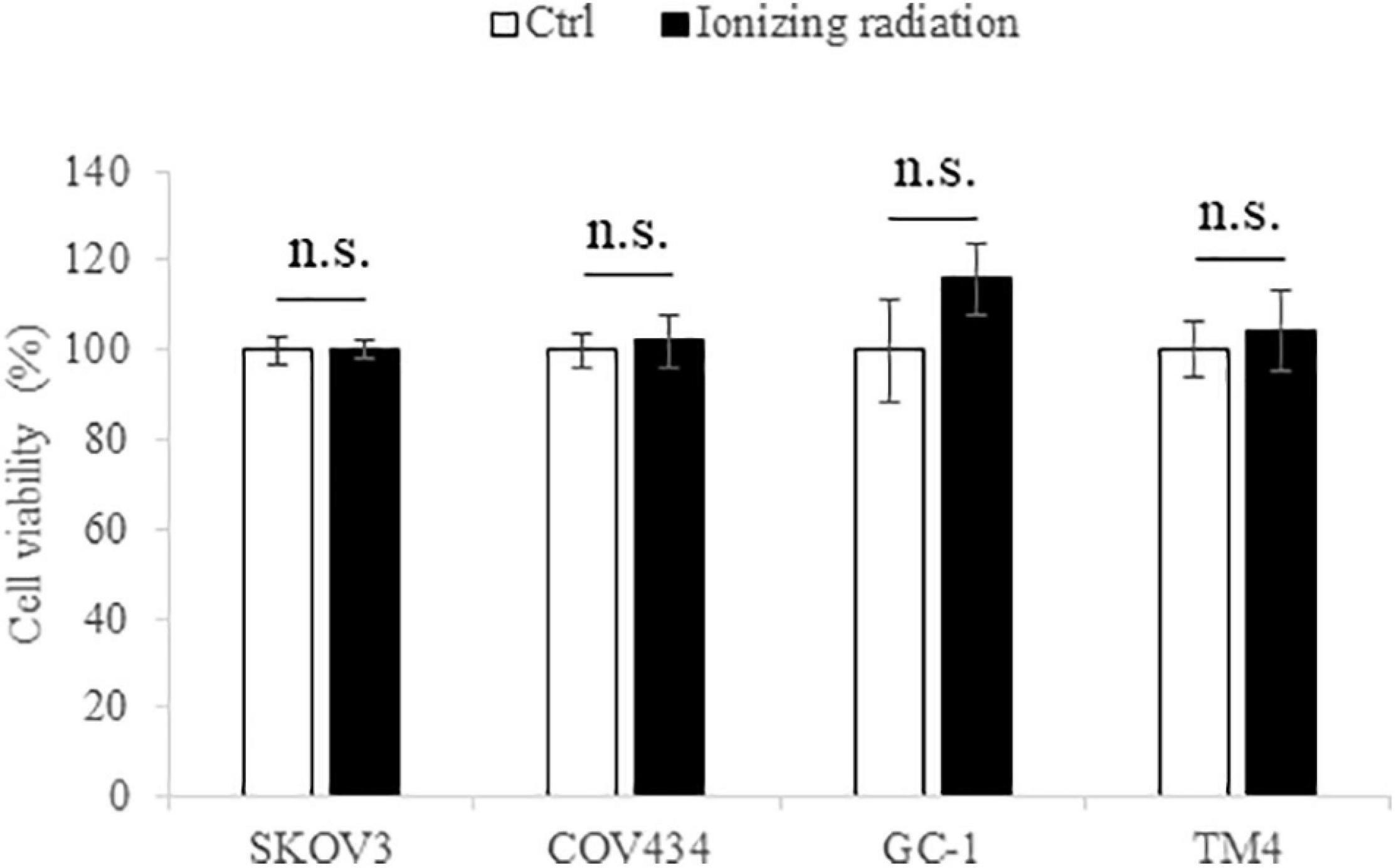
Figure 1. The ionizing radiation exposure (10 cGy) has no effect on the cell viability of SKOV3, COV434, GC-1, and TM4 cells. The white bar represented the control group; the black bar represented the ionizing radiation group. N.s., not statistically significant.
Ionizing Radiation Induced Differential Gene Expression in Both Female and Male Reproductive Cells
In an attempt to understand the biological functions altered by the ionizing radiation, a comparative transcriptomic analysis was conducted. Deep sequencing of RNA libraries derived from control and ionizing radiation treatment groups of each cell lines generated at least 42 million quality-trimmed clean reads (Supplementary Table 2). A total of 51.2 Gb quality-trimmed bases were obtained from the transcriptome sequencing (Supplementary Table 2). Over 95% of sequencing reads could be mapped to the reference genome (Supplementary Table 2). Comparative transcriptomic analysis of ovarian cancer SKOV3 cells found a total 1,144 differentially expressed genes (DEGs), including 574 upregulated and 570 downregulated genes in the ionizing radiation group as compared with the control group (Figure 2A and Supplementary Table 3). Four thousand three hundred ninety-nine DEGs, including 1,575 upregulated and 2,824 downregulated genes were found in ovarian cancer COV434 cells after ionizing radiation exposure (Figure 2B and Supplementary Table 4). Upregulation of 24 genes and downregulation of 35 genes were common in SKOV3 and COV434 after ionizing radiation (Figure 2C and Table 1). Comparative transcriptomic analysis further revealed a smaller number of DEGs in male reproductive cell lines compared with female ovarian cancer cell lines after ionizing radiation. In testicular TM4 germ cell, a total of 783 DEGs, including 278 upregulated and 505 downregulated genes, were found in ionizing radiation group as compared with control group (Figure 3A and Supplementary Table 5). In another testicular GC-1 germ cell, we found 248 DEGs including 150 upregulated and 98 downregulated genes under ionizing radiation (Figure 3B and Supplementary Table 6). Notably, TM4 and GC-1 cells shared 26 upregulated and 32 downregulated genes (Figure 3C and Table 2).
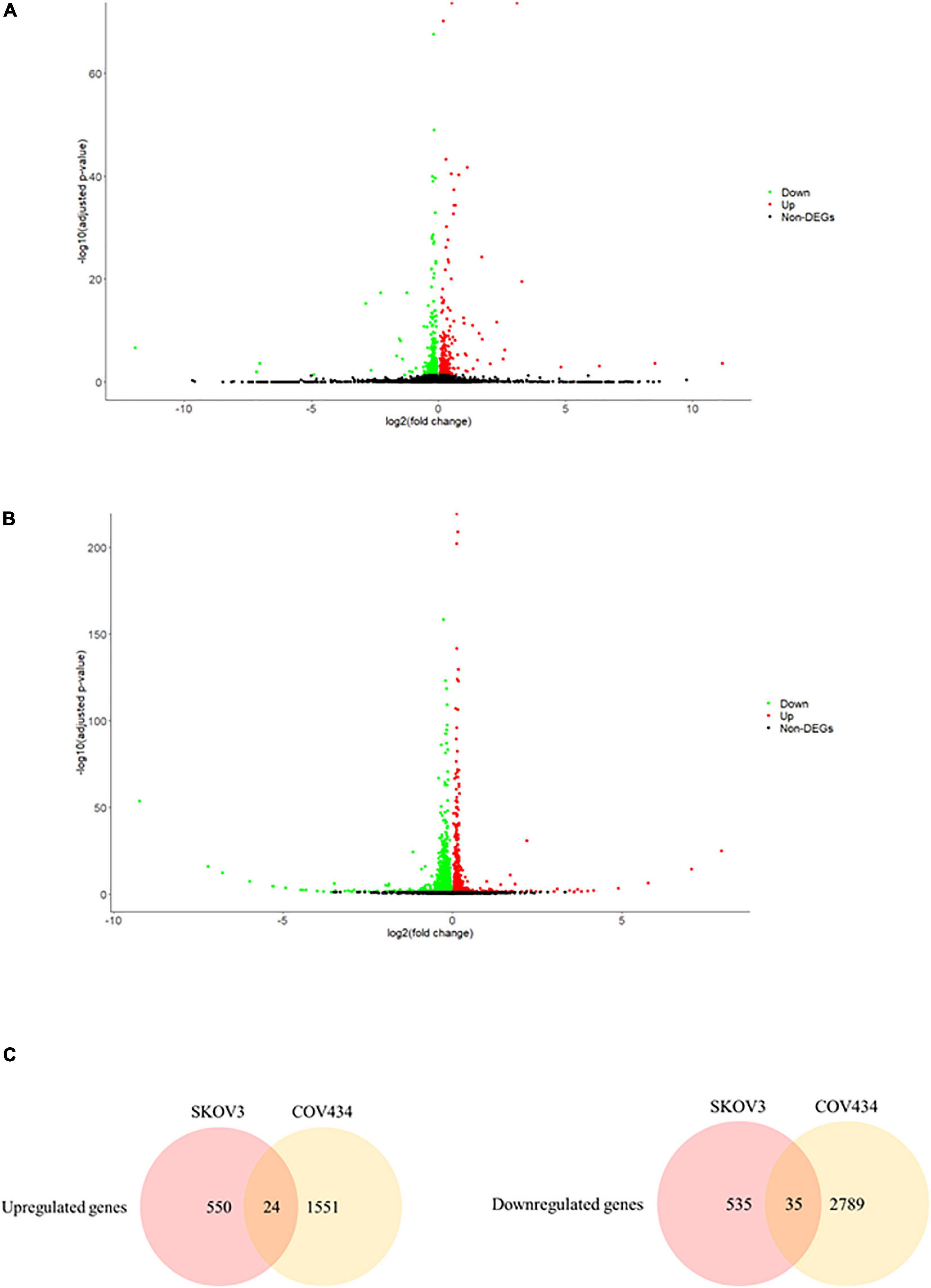
Figure 2. The ionizing radiation exposure caused the differential gene expression in male reproductive cells. (A) Volcano plot showed the differentially expressed genes in the TM4 cells. Green dots represented downregulated genes. Red dots represented upregulated genes. (B) Volcano plot showed the differentially expressed genes in the GC-1 cells. Green dots represented downregulated genes. Red dots represented upregulated genes. (C) Common deregulated genes in TM4 and GC-1 cells under the ionizing radiation exposure.
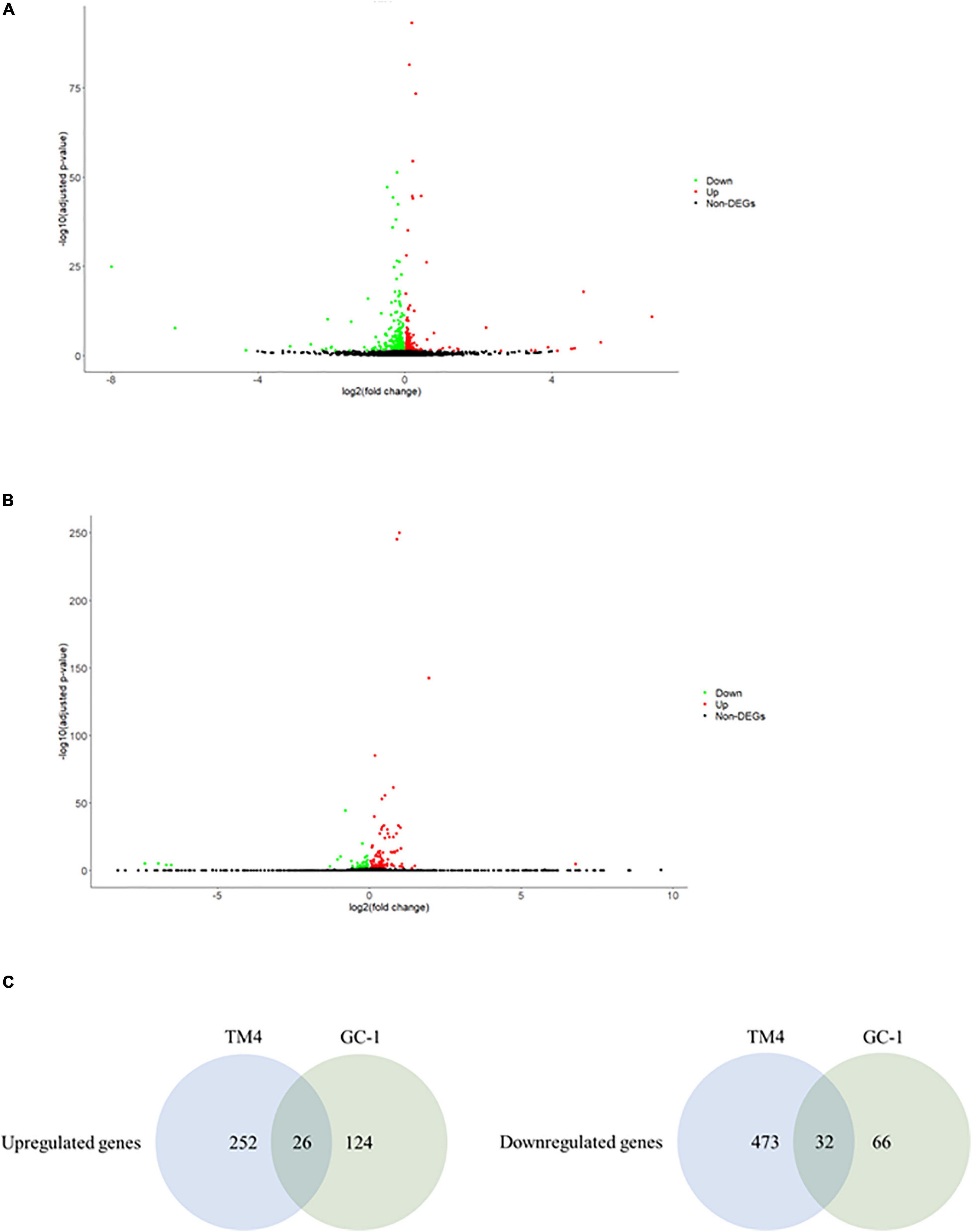
Figure 3. The ionizing radiation exposure caused the differential gene expression in female reproductive cells. (A) Volcano plot showed the differentially expressed genes in the SKOV3 cells. Green dots represented downregulated genes. Red dots represented upregulated genes. (B) Volcano plot showed the differential expressed genes in the COV434 cells. Green dots represented downregulated genes. Red dots represented upregulated genes. (C) Common deregulated genes in SKOV3 and COV434 cells under the ionizing radiation exposure.
Ionizing Radiation Altered Biological Processes and Signaling Pathways in the Reproductive Cells
The common DEGs were then subjected to GO and KEGG enrichment analysis, to elucidate the alteration of biological functions and signaling pathways in female and male reproductive cells caused by the ionizing radiation. The result of GO analysis in the female reproductive cells showed that the ionizing radiation would trigger DNA damage response, leading to the alteration of many biological processes related to cell apoptosis, cell growth, cell cycle arrest, protein stabilization and folding, cell-cell adhesion, gene expression, and in utero embryonic development (Figure 4A and Table 3). More importantly, our results showed that ionizing radiation can alter the biological processes closely related to epigenetic regulation such as establishment of protein localization to telomere, telomerase RNA localization to Cajal body, and heterochromatin assembly (Figure 4A and Table 3). Results of KEGG pathway analysis further highlighted that radiation causes alteration of MAPK signaling pathway, protein processing in endoplasmic reticulum, spliceosome, antigen processing and presentation, estrogen signaling pathway, and cell cycle (Figure 4B and Table 4). In the male reproductive cells, the ionizing radiation could similarly cause a DNA damage response (Figure 4C and Table 5), leading to alterations of different biological processes such as cell apoptosis, cell proliferation, cell cycle, and cell death (Figure 4C and Table 5). Although the responses were similar to those observed in female reproductive cells, the gene clusters involved in the processes were largely different (Table 6) and only shared the induction of MYC and CYR61 (Table 6). More importantly, the ionizing radiation resulted in the modulation of chromatin remodeling such as chromosome organization, meiotic chromosome segregation, and meiotic chromosome condensation, which was considered a major event leading to epigenetic modification in male reproductive cells (Figure 4C and Table 5). The chromatin remodeling is controlled by a family of structural maintenance of chromosome protein including SMC2, SMC3, and SMC4 and SJQ/SMB-related matrix-associated actin-dependent regulator of chromatin subfamily A member 5 (SMARCA5) (Table 5). The result of KEGG pathway analysis highlighted the alteration of focal adhesion, Hippo signaling pathway, ECM-receptor interaction, and MAPK signaling pathway (Figure 4D and Table 7). Taken together, our data suggested that ionizing radiation caused a similar biological alteration in both the female and male reproductive cells through the regulation of different gene clusters.
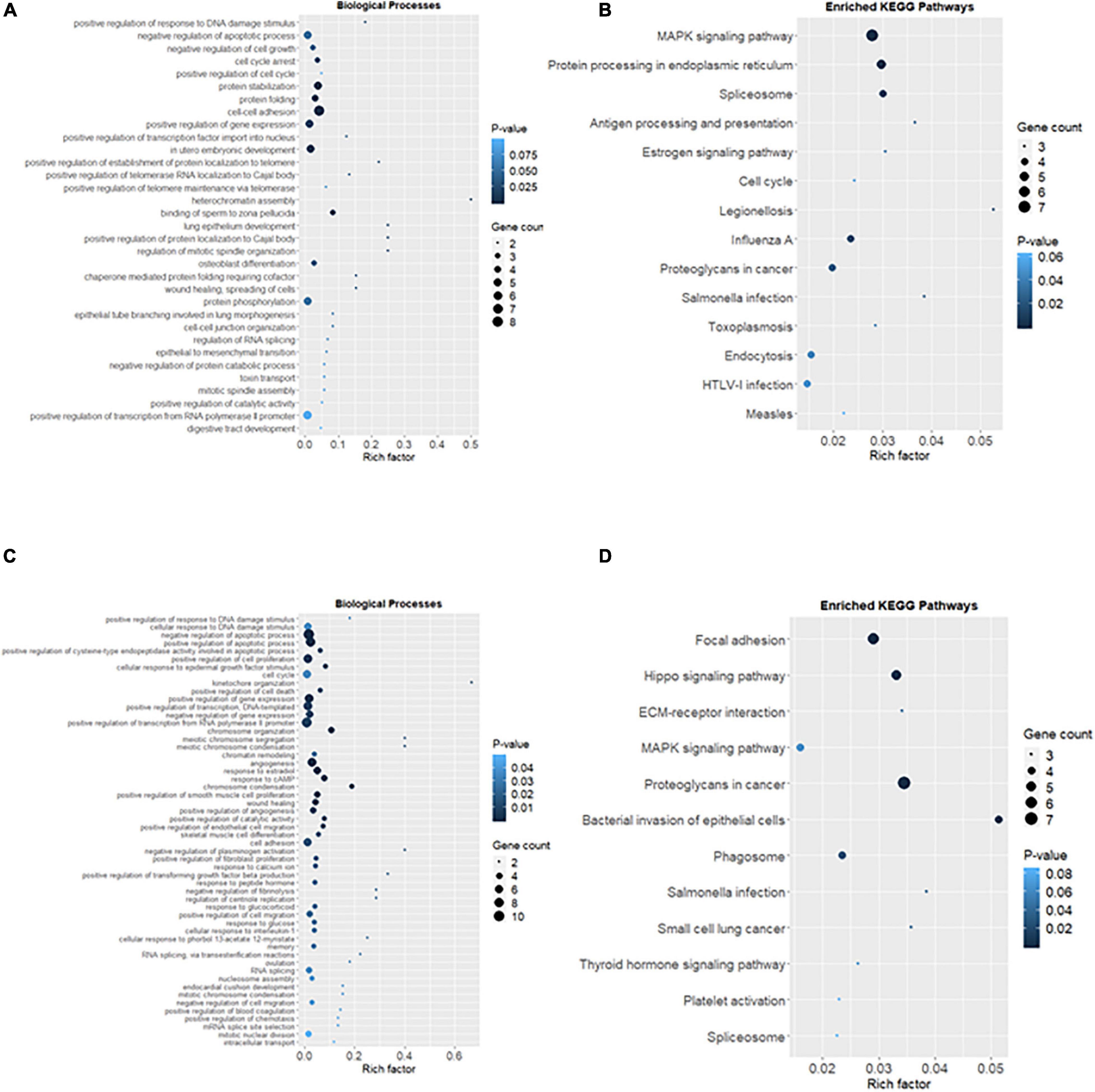
Figure 4. Alteration of biological processes and signaling pathways in the reproductive cells caused by the ionizing radiation. (A) Rich factor plot showed the altered biological processes related to DNA damage responses and cell apoptosis and telomere structure in female reproductive cells using Gene Ontology (GO) enrichment analysis. (B) Rich factor plot showed the altered cell signaling pathways in the female reproductive cells after the ionizing radiation exposure using Kyoto Encyclopedia of Genes and Genomes (KEGG) analysis. (C) Rich factor plot showed the altered biological processes related to DNA damage and chromosome organization in male reproductive cells using GO enrichment analysis. (D) Rich factor plot showed the altered cell signaling pathways in the male reproductive cells after the ionizing radiation exposure using KEGG analysis. The size of dot represented the number of gene. The color intensity of dot represented the significance of the biological processes and cell signaling pathways.
Ionizing Radiation Caused Deregulation of Gene Network and Reproductive Disorder
In order to understand the reproductive diseases and to delineate the gene networks involved in ionizing radiation-altered biological processes, IPA was conducted. The results of disease analysis showed that the radiation exposure could potentially lead to many female reproductive disorders and diseases such as ovary growth impairment, genital tract cancer, and ovarian cancer (Table 8). A similar finding was observed in the male reproductive system that radiation could cause tumorigenesis of reproductive tract and develop malignant neoplasm and endometriosis of the male genital organ (Table 9). Canonical pathway analysis of IPA further highlighted the involvement of many components from different cellular levels in radiation-mediated reproductive impairment. In the female reproductive cells, tight junction protein 1 (TJP1) was found to be downregulated and associated with the deregulation of filamin A, alpha (FLNA) and heat shock 70 kDa protein 1 (HSPA1A) (Figure 5A). Also, a group of enzymes such as lactate dehydrogenase A (LDHA), DNA polymerase Epsilon (POLE), and small nuclear ribonucleoprotein U5 Subunit 200 (SNRP200) (Figure 5A) were highlighted. More importantly, a cluster of transcription factors including DnaJ heat shock protein family (Hsp40) member B1 (DNAJB1), mothers against decapentaplegic homolog 3 (SMAD3) and MYC proto-oncogene, and BHLH transcription factor (MYC) were involved in the ionizing radiation-mediated reproductive impairment (Figure 5A). Our result showed that MYC is a key mediator that directly controls different kinases and enzymes (Figure 5A). In the male reproductive cells, more candidates were found to be involved in reproductive impairment caused by the ionizing radiation (Figure 5B). The gene networking showed the induction of thrombospondin-1 (THBS1), fibronectin 1 (FN1), collagen, type I, alpha 1 (COL1A1), and serpin family E member 1 (SERPINE1) in extracellular matrix (Figure 5B). Also, radiation exposure could lead to downregulation of membrane protein transient receptor potential cation channel subfamily M member 7 (TRPM7). Similar to the result in the female reproductive cells, MYC was the major mediator connecting different enzymes such as methionine adenosyltransferase 2A (MAT2), Ras homolog family member B (RHOB), ubiquitin C (UBC), prostaglandin-endoperoxide synthase 2 (PTGS2), and heat shock protein family A member 8 (HSPA8), as well as a large number of transcription factors including nucleophosmin (NPM1), Cbp/p300-interacting transactivator 2 (CITED2), and ATP-dependent helicase (ATRX) (Figure 5B). Taken together, our results suggested a gender-specific gene network involved in the ionizing radiation-mediated reproductive impairment.
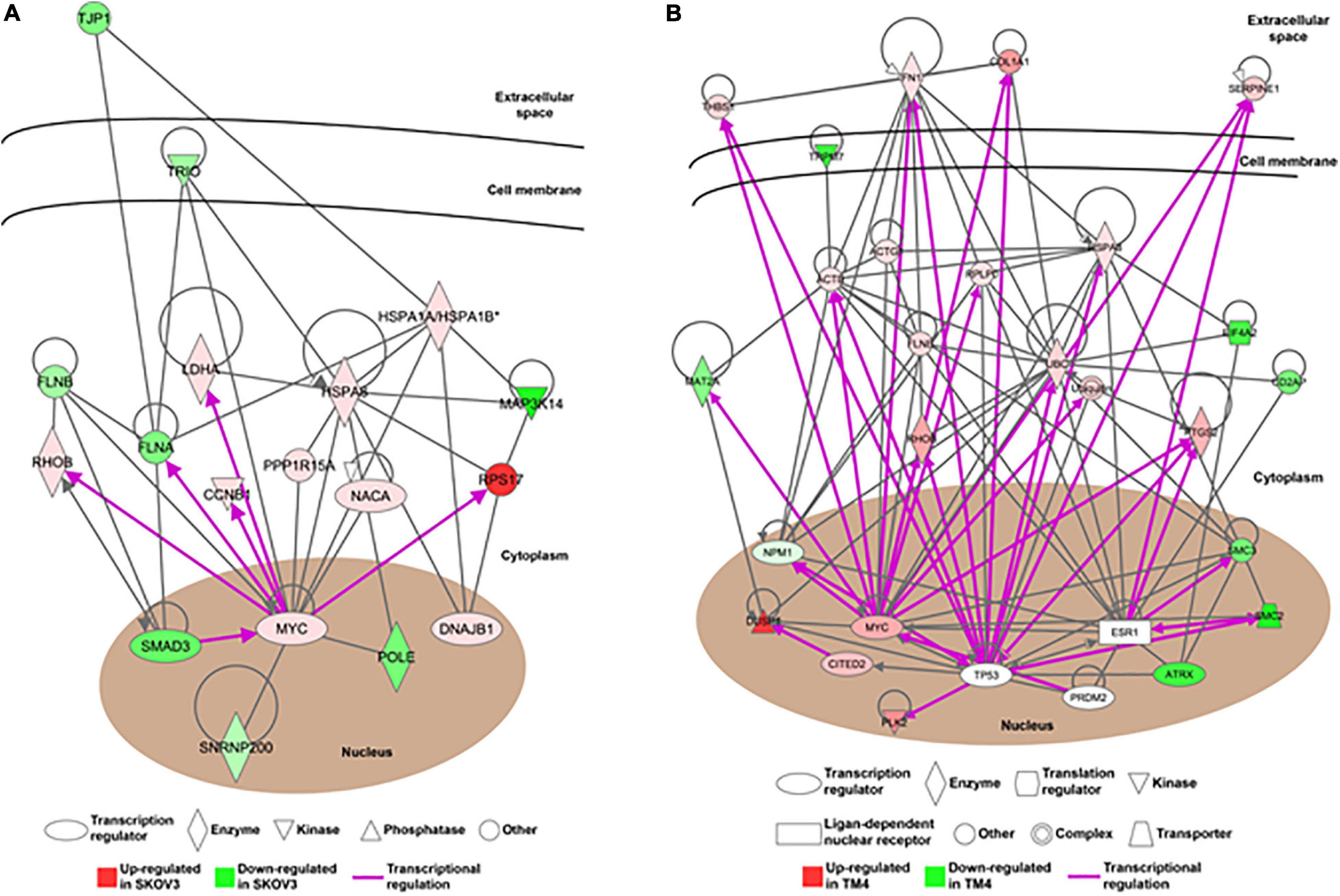
Figure 5. The alteration of gene networks is caused by the ionizing radiation. The gene networking of the Ingenuity Pathway Analysis (IPA) showed the gene networks in response to the ionizing radiation in (A) female reproductive cells and (B) male reproductive cells. Red color represented upregulated genes and green color represented downregulated genes. The purple arrow showed the direct transcriptional regulation.
Discussion
The present study unraveled that epigenetic changes associated with reproductive impairment can be elicited by a low dose of ionizing radiation (i.e., 100 mGy). The dose we used in this study is considered a low-dose radiation because fetal absorbed about 4.9 cGy per procedure during abdominal CT scan, and for pelvis it was as high as 7.9 cGy (Groen et al., 2012). A cohort study of diagnostic medical radiation workers in South Korea showed that the workers absorbed a mean cumulative badge dose of 7.20 mSv (Lee et al., 2021). While the environmental level of ionizing radiation is about 3 mGy/year, high levels of background radiation was commonly reported in some areas around the world (Hendry et al., 2009).
In this study, we determined and compared the effects of radiation on different types of male and female reproductive cells. GC-1 spg is an immortalized type B spermatogonia line and displays characteristics of a stage between type B spermatogonia and primary spermatocytes. TM4 is an immortalized Sertoli cell line and is a somatic cell-type essential for nurturing germ cell development. Due to the low availability of freshly isolated primary ovarian reproductive cells, two ovarian cancer lines derived from ovarian surface epithelium and granulosa cells, respectively, are used. Our comparative transcriptomic analysis revealed that environmental relevant level of ionizing radiation is sufficient to induced DNA damage, leading to cell cycle arrest and cell apoptosis in both female and male reproductive cells. It is not surprising that the genes involved were largely different according to cell types, and only MYC and CYR61 were shared in both female and male reproductive cells. MYC is a transcription factor and contributes to the development of many cancer types (Godwin et al., 2021). In addition, it plays an important role in DNA damage response repair. For instance, MYC formed foci with γ-H2AX to phosphorylate ATM and to mediate DNA-PKcs activity (Cui et al., 2015), leading to enhanced chromosomal and chromatid breaks in response to γ-ray ionizing radiation (Li et al., 2012). In addition, MYC was essential for DNA damage-induced apoptosis through the control of the p53 tumor suppressor protein (Phesse et al., 2014). The other deregulated gene, cysteine-rich angiogenic inducer 61 (CYR61), is an extracellular matrix-associated signaling protein of the CCN intercellular signaling protein family (Lau, 2011). CYR61 is a cell apoptosis and senescence inducer involved in DNA damage response through the regulation of p53 upon genotoxic stress (Feng et al., 2008; Morrison et al., 2009). In addition, CYR61 was a downstream effector of the Hippo signaling pathway that is one the important cell signaling pathways of DNA damage response (Shome et al., 2020). Other than the common DNA damage responses, our result also suggested that the ionizing radiation could cause gene deregulation related to impairment of utero embryonic development in the female reproductive system. Although it has been reported that abdominopelvic ionizing irradiation (>5 Gy) increased the risk of unfavorable neonatal outcomes such as fetal malformation and disturbances of growth or development (Kumar and DeJesus, 2021), our result showed that even a much lower level of ionizing radiation (10 cGy) could also pose adverse effect on embryonic development. In addition, our data highlighted that ionizing radiation could alter estrogen signaling pathway in female reproductive cells. Estrogen signaling pathway is one of the most important pathways involved in steroid hormones and reproductive regulation in mammals (Saito and Cui, 2018), and estrogens are also associated with tumor development, particularly breast and ovary cancers (Ferreira et al., 2009). Studies also demonstrated that estrogen is also related to the DNA repair pathways and DNA integrity (Jiménez-Salazar et al., 2021; Pescatori et al., 2021).
Our results further highlighted that ionized radiation could alter a spectrum of male reproductive function-related cell signaling such as ECM-receptor interaction, Hippo signaling pathway, and MAPK signaling pathway. Extracellular matrix (ECM) receptor interaction pathway has been reported to be associated with spermatogenesis (Gong et al., 2018). In addition, ECM remodeling is required for the testicular development and maturation (Slongo et al., 2002). Hippo signaling pathway is responsible for the controls of organ size through the regulation of cell proliferation and apoptosis (Zhang et al., 2009). Yes-associated protein, the downstream effector of Hippo signaling pathway was reported to modulate the decline of germline stem cells and niche cells (Francis et al., 2019). Also, Hippo signaling cascade was found to be associated with the pubertal development of male reproductive tract and spermatogenesis in sheep (Zhang et al., 2019). MAPK signaling pathway is the key mediator that controls the phosphorylation of many downstream effectors, leading to modulate different cellular functions, including cell proliferation, differentiation, and migration (Joerger-Messerli et al., 2021). Oxidative stress-mediated p38 MAPK signaling pathway was associated with the blood-testis barrier-related junction protein and promoting apoptosis in mice testes (Huang et al., 2021). Moreover, activation of the MAPK signaling pathway was involved in the molecular mechanism of apoptosis in spermatogonia cells (Park et al., 2020). As such, deregulation of these pathways found in the present study suggested different aspects of reproductive impairment caused by the ionizing radiation.
Beside the cell signaling pathways related to reproductive functions, our result also demonstrated that ionizing radiation may cause epigenetic modification in both female and male reproductive cells. In female reproductive cells, telomerase, telomere-binding proteins, and heterochromatin assembly were disturbed by ionizing radiation. In male reproductive cells, chromosome organization such as meiotic chromosome segregation and condensation was interfered. Telomere, a repetitive DNA sequencing at the end of chromosome, protects chromosome from progressive degradation. The length of telomere is controlled by a group of telomere-binding proteins and the enzymatic activity of telomerase. Recent research showed that dysregulated telomerase activation was associated with epigenetic, transcriptional, and posttranscriptional modifications (Dogan and Forsyth, 2021). Lister-Shimauchi et al. (2021) demonstrated that deficiency for telomerase in Caenorhabditis elegans resulted in transgenerational shortening of telomeres. In addition, histone modifications including activation markers (H3K4me1 and H3K4me3) and silencing marks (H3K27me3) at distal promoters were telomere length dependent, suggesting that the epigenetic state of telomere-distal promoters could be influenced by telomere length (Mukherjee et al., 2018). Moreover, meiotic chromosome segregation is essential for the maintenance of genomic integrity of gametes and requires functional centromeres that are required for a precise epigenetic inheritance (Mahlke and Nechemia-Arbely, 2020; Tan et al., 2020).
In conclusion, we show that environmental relevant dose of ionizing radiation can alter the expression of gene cluster related to DNA damage response through the control of MYC, which agreed with other studies reporting amplification of MYC gene in somatic cells and cancer cells (Sawey et al., 1987; Mothersill et al., 1991; Käcker et al., 2013; Ginter et al., 2014). The ionizing radiation-mediated reproductive impairment is not only gender specific but also connected with different gene networks and pathways. Due to the limited number of germ cells and their extended period of developmental processes, research on the radiation-induced effects of female germline has been hindered (Skrzypek et al., 2019). In this study, we have unraveled possible pathways in ovarian cells altered by radiation, thus providing insights on the mechanisms underlying the perturbed reproductive functions. More importantly, our findings suggested that ionizing radiation can interfere telomere and chromatin remodeling, leading to possible epigenetic changes. Further investigation is warranted to elucidate whether these induced modifications can lead to transgenerational reproductive impairments.
Data Availability Statement
Sequencing data of transcriptome sequencing that support the findings of this study have been deposited in the NCBI BioProject database (https://www.ncbi.nlm.nih.gov/bioproject) with the BioProject accession code PRJNA730377.
Ethics Statement
In accordance with the national legislation and the institutional requirements, the Human Research Ethics Committee of The University of Hong Kong waived the requirement for ethical approval and written informed consent for participants in this study.
Author Contributions
RW, AW, WL, KWY, RK, and JC contributed to conception and design of the study. CL and YY performed the experiments. KL organized the database. TC, XL, and NT performed the bioinformatics and statistical analysis. KL, RW, and KNY wrote the manuscript. All authors contributed to manuscript revision, read, and approved the submitted version.
Funding
This project was supported by the Hong Kong Branch of Southern Marine Science and Engineering Guangdong Laboratory (Guangzhou) (SMSEGL20SC02). KL was supported by the Hong Kong SAR, Macao SAR, and Taiwan Province Talented Young Scientist Program of Guangxi.
Conflict of Interest
The authors declare that the research was conducted in the absence of any commercial or financial relationships that could be construed as a potential conflict of interest.
Publisher’s Note
All claims expressed in this article are solely those of the authors and do not necessarily represent those of their affiliated organizations, or those of the publisher, the editors and the reviewers. Any product that may be evaluated in this article, or claim that may be made by its manufacturer, is not guaranteed or endorsed by the publisher.
Supplementary Material
The Supplementary Material for this article can be found online at: https://www.frontiersin.org/articles/10.3389/fgene.2021.710143/full#supplementary-material
Footnotes
References
Ahmed, E., Scherthan, H., and deRooij, D. (2015). DNA double strand break response and limited repair capacity in mouse elongated spermatids. Int. J. Mol. Sci. 16, 29923–29935. doi: 10.3390/ijms161226214
Clifton, D. K., and Bremner, W. J. (1983). The effect of testicular X-irradiation on spermatogenesis in man. J. Androl. 4, 387–392. doi: 10.1002/j.1939-4640.1983.tb00765.x
Critchley, H. O. D., Wallace, W. H., Shalet, S. M., Mamtora, H., Higginson, J., and Anderson, D. C. (1992). Abdominal irradiation in childhood; the potential for pregnancy. Br J Obstet Gynaecol. 99, 392–394.
Cui, F., Fan, R., Chen, Q., He, Y., Song, M., Shang, Z., et al. (2015). The involvement of c-Myc in the DNA double-strand break repair via regulating radiation-induced phosphorylation of ATM and DNA-PKcs activity. Mol. Cell. Biochem. 406, 43–51. doi: 10.1007/s11010-015-2422-2
Dogan, F., and Forsyth, N. R. (2021). Telomerase regulation: a role for epigenetics. Cancers (Basel) 13:1213. doi: 10.3390/cancers13061213
Dubrova, Y. E., and Sarapultseva, E. I. (2020). Radiation-induced transgenerational effects in animals. Int. J. Radiat. Biol. doi: 10.1080/09553002.2020.1793027 [Epub ahead of print].
Fajardo, L. F. (2005). The pathology of ionizing radiation as defined by morphologic patterns. Acta Oncol. (Madr.) 44, 13–22. doi: 10.1080/02841860510007440
Feng, P., Wang, B., and Ren, E. C. (2008). Cyr61/CCN1 is a tumor suppressor in human hepatocellular carcinoma and involved in DNA damage response. Int. J. Biochem. Cell Biol. 40, 98–109. doi: 10.1016/j.biocel.2007.06.020
Ferreira, A. M., Westers, H., Albergaria, A., Seruca, R., and Hofstra, R. M. W. (2009). Estrogens, MSI and lynch syndrome-associated tumors. Biochim. Biophys. Acta 1796, 194–200. doi: 10.1016/j.bbcan.2009.06.004
Filkowski, J. N., Ilnytskyy, Y., Tamminga, J., Koturbash, I., Golubov, A., Bagnyukova, T., et al. (2010). Hypomethylation and genome instability in the germline of exposed parents and their progeny is associated with altered miRNA expression. Carcinogenesis 31, 1110–1115. doi: 10.1093/carcin/bgp300
Francis, D., Chanana, B., Fernandez, B., Gordon, B., Mak, T., and Palacios, I. M. (2019). YAP/Yorkie in the germline modulates the age-related decline of germline stem cells and niche cells. PLoS One 14:e0213327. doi: 10.1371/journal.pone.0213327
García-Rodríguez, A., Gosálvez, J., Agarwal, A., Roy, R., and Johnston, S. (2018). DNA damage and repair in human reproductive cells. Int. J. Mol. Sci. 20:31. doi: 10.3390/ijms20010031
Ginter, P. S., Mosquera, J. M., MacDonald, T. Y., D’Alfonso, T. M., Rubin, M. A., and Shin, S. J. (2014). Diagnostic utility of MYC amplification and anti-MYC immunohistochemistry in atypical vascular lesions, primary or radiation-induced mammary angiosarcomas, and primary angiosarcomas of other sites. Hum. Pathol. 45, 709–716. doi: 10.1016/j.humpath.2013.11.002
Godwin, I., Anto, N. P., Bava, S. V., Babu, M. S., and Jinesh, G. G. (2021). Targeting K-Ras and apoptosis-driven cellular transformation in cancer. Cell Death Discov. 7:80.
Gong, J., Zhang, Q., Wang, Q., Ma, Y., Du, J., Zhang, Y., et al. (2018). Identification and verification of potential piRNAs from domesticated yak testis. Reproduction 155, 117–127.
Goodhead, D. T. (1994). Initial events in the cellular effects of ionizing radiations: clustered damage in DNA. Int. J. Radiat. Biol. 65, 7–17. doi: 10.1080/09553009414550021
Grandjean, V., Fourré, S., De Abreu, D. A. F., Derieppe, M.-A., Remy, J.-J., and Rassoulzadegan, M. (2016). RNA-mediated paternal heredity of diet-induced obesity and metabolic disorders. Sci. Rep. 5:18193.
Groen, R. S., Bae, J. Y., and Lim, K. J. (2012). Fear of the unknown: ionizing radiation exposure during pregnancy. Am. J. Obstet. Gynecol. 206, 456–462. doi: 10.1016/j.ajog.2011.12.001
Haines, G. A., Hendry, J. H., Daniel, C. P., and Morris, I. D. (2002). Germ cell and dose-dependent DNA damage measured by the comet assay in murine Spermatozoaa after testicular X-irradiation1. Biol. Reprod. 67, 854–861. doi: 10.1095/biolreprod.102.004382
Halimi, M., Asghari, S. M., Sariri, R., Moslemi, D., and Parsian, H. (2012). Cellular response to ionizing radiation: a microRNA story. Int. J. Mol. Cell. Med. 1, 178–184.
Hendry, J. H., Simon, S. L., Wojcik, A., Sohrabi, M., Burkart, W., Cardis, E., et al. (2009). Human exposure to high natural background radiation: what can it teach us about radiation risks? J. Radiol. Prot. 29, A29–A42.
Huang, W., Liu, M., Xiao, B., Zhang, J., Song, M., Li, Y., et al. (2021). Aflatoxin B1 disrupts blood-testis barrier integrity by reducing junction protein and promoting apoptosis in mice testes. Food Chem. Toxicol. 148:111972. doi: 10.1016/j.fct.2021.111972
Jenkins, T. G., and Carrell, D. T. (2012). The sperm epigenome and potential implications for the developing embryo. Reproduction 143, 727–734. doi: 10.1530/rep-11-0450
Jiménez-Salazar, J. E., Damian-Ferrara, R., Arteaga, M., Batina, N., and Damián-Matsumura, P. (2021). Non-genomic actions of estrogens on the DNA repair pathways are associated with chemotherapy resistance in breast cancer. Front. Oncol. 11:631007. doi: 10.3389/fonc.2021.631007
Joerger-Messerli, M. S., Thomi, G., Haesler, V., Keller, I., Renz, P., Surbek, D. V., et al. (2021). Human Wharton’s Jelly mesenchymal stromal cell-derived small extracellular vesicles drive oligodendroglial maturation by restraining MAPK/ERK and notch signaling pathways. Front. Cell Dev. Biol. 9:622539. doi: 10.3389/fcell.2021.622539
Käcker, C., Marx, A., Mössinger, K., Svehla, F., Schneider, U., Hogendoorn, P. C., et al. (2013). High frequency of MYC gene amplification is a common feature of radiation-induced sarcomas. Further results from EORTC STBSG TL 01/01. Genes Chromosomes Cancer 52, 93–98. doi: 10.1002/gcc.22009
Kamstra, J. H., Hurem, S., Martin, L. M., Lindeman, L. C., Legler, J., Oughton, D., et al. (2018). Ionizing radiation induces transgenerational effects of DNA methylation in zebrafish. Sci. Rep. 8:15373.
Kumar, D., Salian, S. R., Kalthur, G., Uppangala, S., Kumari, S., Challapalli, S., et al. (2013). Semen abnormalities, sperm DNA damage and global hypermethylation in health workers occupationally exposed to ionizing radiation. PLoS One 8:e69927. doi: 10.1371/journal.pone.0069927
Kumar, R., and DeJesus, O. (2021). “Radiation Effects on the Fetus,” in StatPearls [Internet], (Treasure Island, FL: StatPearls Publishing).
Kumar, R., Horikoshi, N., Singh, M., Gupta, A., Misra, H. S., Albuquerque, K., et al. (2013). Chromatin modifications and the DNA damage response to ionizing radiation. Front. Oncol. 2:214. doi: 10.3389/fonc.2012.00214
Lau, L. F. (2011). CCN1/CYR61: the very model of a modern matricellular protein. Cell. Mol. Life Sci. 68, 3149–3163. doi: 10.1007/s00018-011-0778-3
Lee, W. J., Ko, S., Bang, Y. J., Choe, S. A., Choi, Y., and Preston, D. L. (2021). Occupational radiation exposure and cancer incidence in a cohort of diagnostic medical radiation workers in South Korea. Occup. Environ. Med. doi: 10.1136/oemed-2021-107452 [Epub ahead of print].
Li, R., Huang, C., Ho, C. H., Leung, C. T., Kong, R. Y. C., Liang, X., et al. (2021). The use of glutathione to reduce oxidative stress status and its potential for modifying the extracellular matrix organization in cleft lip. Free Radic. Biol. Med. 164, 130–138. doi: 10.1016/j.freeradbiomed.2020.12.455
Li, Z., Owonikoko, T. K., Sun, S. Y., Ramalingam, S. S., Doetsch, P. W., Xiao, Z. Q., et al. (2012). c-Myc suppression of DNA double-strand break repair. Neoplasia 14, 1190–1202.
Lister-Shimauchi, E. H., Dinh, M., Maddox, P., and Ahmed, S. (2021). Gametes deficient for Pot1 telomere binding proteins alter levels of telomeric foci for multiple generations. Commun. Biol. 4:158.
Little, J. B. (2000). Radiation carcinogenesis. Carcinogenesis 21, 397–404. doi: 10.1093/carcin/21.3.397
Mahlke, M. A., and Nechemia-Arbely, Y. (2020). Guarding the genome: CENP-A-chromatin in health and cancer. Genes (Basel) 11:810. doi: 10.3390/genes11070810
Metheetrairut, C., and Slack, F. J. (2013). MicroRNAs in the ionizing radiation response and in radiotherapy. Curr. Opin. Genet. Dev. 23, 12–19. doi: 10.1016/j.gde.2013.01.002
Miousse, I. R., Kutanzi, K. R., and Koturbash, I. (2017). Effects of ionizing radiation on DNA methylation: from experimental biology to clinical applications. Int. J. Radiat. Biol. 93, 457–469. doi: 10.1080/09553002.2017.1287454
Morrison, J. P., Ton, T. V., Collins, J. B., Switzer, R. C., Little, P. B., Morgan, D. L., et al. (2009). Gene expression studies reveal that DNA damage, vascular perturbation, and inflammation contribute to the pathogenesis of carbonyl sulfide neurotoxicity. Toxicol. Pathol. 37, 502–511. doi: 10.1177/0192623309335631
Mothersill, C., Seymour, C. B., and O’Brien, A. (1991). Induction of c-myc oncoprotein and of cellular proliferation by radiation in normal human urothelial cultures. Anticancer Res. 11, 1609–1612.
Mukherjee, A. K., Sharma, S., Sengupta, S., Saha, D., Kumar, P., Hussain, T., et al. (2018). Telomere length-dependent transcription and epigenetic modifications in promoters remote from telomere ends. PLoS Genet. 14:e1007782. doi: 10.1371/journal.pgen.1007782
Paris, L., Giardullo, P., Leonardi, S., Tanno, B., Meschini, R., Cordelli, E., et al. (2015). Transgenerational inheritance of enhanced susceptibility to radiation-induced medulloblastoma in newborn Ptch1+/– mice after paternal irradiation. Oncotarget 6, 36098–36112. doi: 10.18632/oncotarget.5553
Park, H.-J., Lee, R., Yoo, H., Hong, K., and Song, H. (2020). Nonylphenol induces apoptosis through ROS/JNK signaling in a spermatogonia cell line. Int. J. Mol. Sci. 22:307. doi: 10.3390/ijms22010307
Pescatori, S., Berardinelli, F., Albanesi, J., Ascenzi, P., Marino, M., Antoccia, A., et al. (2021). A tale of ice and fire: the dual role for 17β-estradiol in balancing DNA damage and genome integrity. Cancers (Basel). 13:1583. doi: 10.3390/cancers13071583
Phesse, T. J., Myant, K. B., Cole, A. M., Ridgway, R. A., Pearson, H., Muncan, V., et al. (2014). Endogenous c-Myc is essential for p53-induced apoptosis in response to DNA damage in vivo. Cell Death Differ. 21, 956–966. doi: 10.1038/cdd.2014.15
Pogribny, I., Koturbash, I., Tryndyak, V., Hudson, D., Stevenson, S. M., Sedelnikova, O., et al. (2005). Fractionated low-dose radiation exposure leads to accumulation of DNA damage and profound alterations in DNA and histone methylation in the murine thymus. Mol. Cancer Res. 3, 553–561. doi: 10.1158/1541-7786.mcr-05-0074
Pujol, R., Cusidó, L., Rubio, A., Egozcue, J., and Garcia, M. (1996). Effect of X-rays on germ cells in female fetuses of Rattus norvegicus irradiated at three different times of gestation. Mutat. Res. Mol. Mech. Mutagen. 356, 247–253. doi: 10.1016/0027-5107(96)00067-x
Pujol, R., Cusidó, L., Rubio, A., Egozcue, J., and Garcia, M. (1997). X-ray-induced synaptonemal complex damage during meiotic prophase in female fetuses of Rattus norvegicus. Mutat. Res. Mol. Mech. Mutagen. 379, 127–134. doi: 10.1016/s0027-5107(97)00115-2
Robinson, M. D., McCarthy, D. J., and Smyth, G. K. (2010). EdgeR: a Bioconductor Package for Differential Expression Analysis of Digital Gene Expression Data. Bioinformatics. 26, 139–40. doi: 10.1093/bioinformatics/btp616
Rogakou, E. P., Pilch, D. R., Orr, A. H., Ivanova, V. S., and Bonner, W. M. (1998). DNA double-stranded breaks induce histone H2AX phosphorylation on serine 139. J. Biol. Chem. 273, 5858–5868. doi: 10.1074/jbc.273.10.5858
Rowley, M. J., Leach, D. R., Warner, G. A., and Heller, C. G. (1974). Effect of graded doses of ionizing radiation on the human testis. Radiat. Res. 59, 665–678. doi: 10.2307/3574084
Saito, K., and Cui, H. (2018). Emerging roles of estrogen-related receptors in the brain: potential interactions with estrogen signaling. Int. J. Mol. Sci. 19:1091. doi: 10.3390/ijms19041091
Sawey, M. J., Hood, A. T., Burns, F. J., and Garte, S. J. (1987). Activation of c-myc and c-K-ras oncogenes in primary rat tumors induced by ionizing radiation. Mol. Cell. Biol. 7, 932–935. doi: 10.1128/mcb.7.2.932
Sharma, U., Sun, F., Conine, C. C., Reichholf, B., Kukreja, S., Herzog, V. A., et al. (2018). Small RNAs are trafficked from the epididymis to developing mammalian sperm. Dev. Cell 46, 481.e–494.e.
Shome, D., vonWoedtke, T., Riedel, K., and Masur, K. (2020). The HIPPO transducer YAP and its targets CTGF and Cyr61 drive a paracrine signalling in cold atmospheric plasma-mediated wound healing. Oxid. Med. Cell. Longev. 2020:4910280.
Skrzypek, M., Wdowiak, A., Panasiuk, L., Stec, M., Szczygieł, K., Zybała, M., et al. (2019). Effect of ionizing radiation on the female reproductive system. Ann. Agric. Environ. Med. 26, 606–616.
Slongo, M. L., Zampieri, M., and Onisto, M. (2002). Expression of matrix metalloproteases (MMP-2, MT1 -MMP) and their tissue inhibitor (TIMP-2) by rat sertoli cells in culture: implications for spermatogenesis. Biol. Chem. 383, 235–239.
Szatmári, T., Persa, E., Kis, E., Benedek, A., Hargitai, R., Sáfrány, G., et al. (2019). Extracellular vesicles mediate low dose ionizing radiation-induced immune and inflammatory responses in the blood. Int. J. Radiat. Biol. 95, 12–22. doi: 10.1080/09553002.2018.1450533
Tamminga, J., Koturbash, I., Baker, M., Kutanzi, K., Kathiria, P., Pogribny, I. P., et al. (2008). Paternal cranial irradiation induces distant bystander DNA damage in the germline and leads to epigenetic alterations in the offspring. Cell Cycle 7, 1238–1245. doi: 10.4161/cc.7.9.5806
Tan, H. L., Zeng, Y. B., and Chen, E. S. (2020). N-terminus does not govern protein turnover of Schizosaccharomyces pombe CENP-A. Int. J. Mol. Sci. 21:6175. doi: 10.3390/ijms21176175
UNSCEAR (2010). Sources and Effects of Ionizing Radiation. Available online at: http://www.unscear.org/unscear/en/publications/2008_1.html (accessed January 2021).
Wallace, W. H. B., Thomson, A. B., and Kelsey, T. W. (2003). The radiosensitivity of the human oocyte. Hum. Reprod. 18, 117–121. doi: 10.1093/humrep/deg016
Ward, J. F. (1988). DNA damage produced by ionizing radiation in mammalian cells: identities, mechanisms of formation, and reparability. Prog. Nucleic Acid Res. Mol. Biol. 35, 95–125. doi: 10.1016/S0079-6603(08)60611-X
Ward, J. F. (1995). Radiation mutagenesis: the initial DNA lesions responsible. Radiat. Res. 142:362. doi: 10.2307/3579145
Wdowiak, A., Skrzypek, M., Stec, M., and Panasiuk, L. (2019). Effect of ionizing radiation on the male reproductive system. Ann. Agric. Environ. Med. 26, 210–216. doi: 10.26444/aaem/106085
Zhang, G.-M., Zhang, T. T., An, S. Y., El-Samahy, M. A., Yang, H., Wan, Y., et al. (2019). Expression of Hippo signaling pathway components in Hu sheep male reproductive tract and spermatozoa. Theriogenology 126, 239–248. doi: 10.1016/j.theriogenology.2018.12.029
Keywords: environmental radiation, epigenetic, reproductive impairments, testicular, ovarian
Citation: Leung CT, Yang Y, Yu KN, Tam N, Chan TF, Lin X, Kong RYC, Chiu JMY, Wong AST, Lui WY, Yuen KWY, Lai KP and Wu RSS (2021) Low-Dose Radiation Can Cause Epigenetic Alterations Associated With Impairments in Both Male and Female Reproductive Cells. Front. Genet. 12:710143. doi: 10.3389/fgene.2021.710143
Received: 31 May 2021; Accepted: 07 July 2021;
Published: 02 August 2021.
Edited by:
Pradyumna Kumar Mishra, ICMR-National Institute for Research in Environmental Health, IndiaReviewed by:
Kailash Manda, Institute of Nuclear Medicine & Allied Sciences (DRDO), IndiaRavindra M. Samartha, Bhopal Memorial Hospital & Research Centre, India
Copyright © 2021 Leung, Yang, Yu, Tam, Chan, Lin, Kong, Chiu, Wong, Lui, Yuen, Lai and Wu. This is an open-access article distributed under the terms of the Creative Commons Attribution License (CC BY). The use, distribution or reproduction in other forums is permitted, provided the original author(s) and the copyright owner(s) are credited and that the original publication in this journal is cited, in accordance with accepted academic practice. No use, distribution or reproduction is permitted which does not comply with these terms.
*Correspondence: Rudolf Shiu Sun Wu, rudolfwu@eduhk.hk; Keng Po Lai, kengplai@cityu.edu.hk