- Gansu Key Laboratory of Herbivorous Animal Biotechnology, College of Animal Science and Technology, Gansu Agricultural University, Lanzhou, China
Long-chain fatty acyl-CoA synthase 1 (ACSL1) plays a vital role in the synthesis and metabolism of fatty acids. The proportion of highly unsaturated fatty acids in beef not only affects the flavor and improves the meat’s nutritional value. In this study, si-ACSL1 and NC-ACSL1 were transfected in bovine preadipocytes, respectively, collected cells were isolated on the fourth day of induction, and then RNA-Seq technology was used to screen miRNAs related to unsaturated fatty acid synthesis. A total of 1,075 miRNAs were characterized as differentially expressed miRNAs (DE-miRNAs), of which the expressions of 16 miRNAs were upregulated, and that of 12 were downregulated. Gene ontology analysis indicated that the target genes of DE-miRNAs were mainly involved in biological regulation and metabolic processes. Additionally, KEGG (Kyoto Encyclopedia of Genes and Genomes) pathway analysis identified that the target genes of DE-miRNAs were mainly enriched in metabolic pathways, fatty acid metabolism, PI3K-Akt signaling pathway, glycerophospholipid metabolism, fatty acid elongation, and glucagon signaling pathway. Combined with the previous mRNA sequencing results, several key miRNA-mRNA targeting relationship pairs, i.e., novel-m0035-5p—ACSL1, novel-m0035-5p—ELOVL4, miR-9-X—ACSL1, bta-miR-677—ACSL1, miR-129-X—ELOVL4, and bta-miR-485—FADS2 were screened via the miRNA-mRNA interaction network. Thus, the results of this study provide a theoretical basis for further research on miRNA regulation of unsaturated fatty acid synthesis in bovine adipocytes.
Introduction
Fat deposits and unsaturated fatty acid (UFA) content in beef muscle not only affect meat quality and flavor but also help improve the nutritional value of meat. Polyunsaturated fatty acids (PUFAs) play a significant role in chronic diseases, including cardiovascular disorders, cancers, and diabetes mellitus (Sijben and Calder, 2007; Marventano et al., 2015). Such as, long-chain n−3 polyunsaturated fatty acids, eicosapentaenoic acid (EPA, 20:5n−3) and docosahexaenoic acid (DHA; 22:6n−3) can reduce cardiovascular disease, the risks of cancer and type 2 diabetes have been widely recognized (Simopoulos, 1991; Barcelo-Coblijn and Murphy, 2009; Lopez-Huertas, 2010). Long-chain fatty acyl-CoA synthetases (ACSLs) are essential for fatty acid (FA) activation and catalysis. ACSL1 converts long-chain FAs to fatty acyl-CoA (Paul et al., 2014). Previous studies have shown that ACSL1 plays an important role in the activation of fatty acid synthesis of triglyceride (Li et al., 2009). In addition, ACSL1 gene variants are also known to affect the content of unsaturated omega-3 FA, PUFAs, long-chain omega-3 FA, and docosapentaenoic acid in bovine skeletal muscle (Widmann et al., 2011). The results of follow-up studies have also shown that interference with the ACSL1 gene caused reduced levels of MUFAs and PUFAs in bovine adipocytes. In contrast, the overexpression of the ACSL1 gene was found to significantly upregulate the content of PUFAs (Tian et al., 2020; Zhao et al., 2020). Related studies have also found that miRNAs regulate lipid metabolism in milk (Lian et al., 2016), liver cancer cells (Cui et al., 2014), laying hens (Tian et al., 2019), and pig subcutaneous fat (Shan et al., 2022) by targeting ACSL1. Based on these results, ACSL1 plays a key regulatory role in the synthesis of unsaturated fatty acids.
MicroRNAs (miRNAs) are endogenous non-coding small RNAs (approximately 22 nt long), which are widely found in eukaryotic cells (Shukla et al., 2011). They bind to the specific complementary site of the target mRNA at the post-transcriptional level, thereby degrading the mRNA or inhibiting its translation and regulating the protein expression (Bartel, 2009). Previous studies found that miRNAs play an important regulatory role in cell proliferation and differentiation, early animal development, apoptosis, oncogene expression inhibition, and fat metabolism (Tufekci et al., 2014; Deb et al., 2018). Many miRNAs are known to regulate the differentiation and deposition of adipocytes by regulating PPAR, C/EBPs, and other transcription factor families (Shukla et al., 2011). For example, bta-miR-130a/b is known to affect the differentiation of bovine adipocytes by targeting PPARG and CYP2U1 (Ma et al., 2018). Also, miR-27a inhibits the differentiation of sheep preadipocytes by specifically binding to RXRα 3′UTR (Deng et al., 2020). Han et al. also found that miR-193a-5p inhibited the proliferation and differentiation of sheep preadipocytes by targeting the ACAA2 gene (Han et al., 2021). A recent study found that miRNAs were involved in the cascade of genes and transcription factors to regulate fatty acid desaturation and fat deposition (Ren et al., 2020). These studies indicated that miRNAs exhibited a critical regulatory effect on fat metabolism.
This study aimed to further explore the molecular mechanism of miRNAs regulating the synthesis of unsaturated fatty acids in bovine adipocytes. High-throughput sequencing was used to systematically identify miRNAs and mRNAs (Bai et al., 2021) that were differentially expressed in bovine adipocytes after interference with the ACSL1 gene and linked the mRNAs related to fatty acid metabolism with miRNAs to construct a miRNA-mRNA interaction network. The results provide new ideas and directions for the regulation of unsaturated fatty acid metabolism in bovine adipocytes.
Materials and methods
Sample collection
This animal study was reviewed and approved by the Faculty Animal Policy and Welfare Committee of Gansu Agricultural University (Ethic approval file No. GSAU-Eth-AST-2021-25). Calves (aged 1 day) from the livestock ranch of Gansu Agricultural University (Lanzhou, China) were selected. After sacrificing the calf, the perirenal adipose tissue was immediately collected for the isolation of bovine preadipocytes. The specific method of cell isolation is the same as our previous research (Tian et al., 2020).
Bovine pre-adipocyte culture and cell transfection
The bovine preadipocytes were cultured to the F3 generation, and then differentiation was induced in vitro. The test operation process and method followed the methods used in our previous studies (Tian et al., 2020). Finally, the cells on the fourth day of differentiation were collected in a 1.5 ml centrifuge tube without ribonuclease and were rapidly frozen in liquid nitrogen for subsequent RNA sequence analysis.
Construction and sequencing of small ribonucleic acid library
Total RNA was extracted from six adipocyte samples (3 with ACSL1 gene interference, si group; the other three without ACSL1 gene interference, NC group) using the Trizol kit (Invitrogen, Carlsbad, CA, USA). RNA degradation and contamination were analyzed via 1% agarose gel electrophoresis; RNA purity (OD260/280 ratio) was determined by Nanodrop analysis; RNA concentration was accurately quantified via Qubit; RNA integrity was determined using Agilent 2,100. After quantification, the NEBNext® Multiplex Small RNA Library Prep Set for Illumina® (NEB, USA) was used to build the library. Using total RNA as the starting sample, polyacrylamide gel (PAGE) electrophoresis was run, select bands in the range of 18–30 nt were isolated, and recovers small RNA. Connect the 3′ adaptor and the 5’ adaptor, respectively, and then perform reverse transcription and PCR amplification on the small RNAs connected to the adaptors on both sides. Finally, the PAGE gel was used to recover and purify the 140 bp band and dissolve it in EB solution to complete the library construction. The constructed library was tested for quality and yield using Agilent 2,100 and ABI StepOnePlus Real-Time PCR System (Life Technologies). After passing the quality inspection, the sequencing was performed by Novogene Bioinformatics Institute (Beijing, China) on Illumina HiSeq 2,500.
Identification of differential miRNAs
Raw reads were further filtered according to the following rules: 1) low quality reads containing more than one low quality (Q-value≤20) base or containing unknown nucleotides (N) were removed; 2) reads without 3′ adapters were removed; 3) reads containing 5′ adapters were removed; 4) reads containing 3′ and 5’ adapters but no small RNA fragment between them were removed; 5) reads containing ployA in small RNA fragment were removed. After the quality control process, the clean tag sequence was aligned to the reference genome of the known cattle (Bos_taurus_Ensembl_94), and classification was performed by aligning it with the GeneBank (version 209.0), Rfam (11.0), and miRBase (22.0) databases. The sequence matching miRBase was considered as the known miRNA. According to software mirdeep2, new miRNA candidates were identified. The miRNA expression level was calculated and normalized to transcripts per million (TPM). For differentially expressed miRNA analysis, DESeq2 (V1.20.0) software was used. The screening criteria for differential miRNAs were the | fold change | > 2.0 and p-value < 0.05. The differential expression of all miRNAs, existing miRNAs, known miRNAs, and new miRNAs were analyzed simultaneously.
Functional enrichment analysis of differential miRNAs
Miranda (v3.3a) and TargetScan (Version: 7.0), two prediction software, were used to predict the target gene of miRNA, and the intersection of the target gene prediction results was used as the result of miRNA target gene prediction. The miRNA target genes were mapped to each term of the GO database (http://www.geneontology.org/), and the number of miRNA target genes was calculated for each term to obtain a list of miRNA target genes with a GO function miRNA target statistics of the number of genes. The hypergeometric test was used to find GO entries that were significantly enriched in miRNA target genes compared with the background. KEGG (Kyoto Encyclopedia of Genes and Genomes) enrichment analysis used hypergeometric testing to find pathways that were significantly enriched in miRNA target genes compared with the entire background. Pathway significant enrichment was used to determine the most important biochemical metabolic pathways and signal transduction pathways involved in miRNA target genes. Finally, GO and KEGG pathways with Q-value < 0.05 were selected as the ones that were significantly enriched.
Real-time PCR verification
Ten miRNAs were selected for qRT-PCR to verify the differential expression results of sequencing. For miRNA expression, a miRNA first-strand cDNA synthesis kit (Accurate Biology, Hunan, China) was used to perform real-time fluorescent quantitative PCR. U6 snRNA was used as an internal reference. All qRT-PCR reactions were performed in the ABI 7500 real-time PCR system (Applied Biosystems, California, USA), with three reactions/samples. The relative expression of miRNA was calculated using the 2−ΔΔCt method (Livak and Schmittgen, 2001).
Construction of miRNA-mRNA interaction network
We selected nine signaling pathways for miRNA target gene enrichment, which are related to lipid metabolism. Then we intersect the genes enriched in the signaling path with the mRNAs expressed differently in our previous sequencing results (Bai et al., 2021), and use these mRNAs and miRNAs to build a miRNA-mRNA interaction network.
Results
Overview of small RNA sequencing
After high-throughput sequencing of six bovine adipocytes, the average clean reads of NC and si were 11, 368, 893 and 11,869,071, respectively, and the average clean tags obtained after quality control were 10,845,625 (95.40%) and 11,302,810 (95.23%) (Table 1). The raw reads obtained in the study were submitted to GenBank Temporary Submission ID: SUB11745359. The length of the smallest RNAs was in the range of 18–23 nt. The miRNAs of 22 nt were the longest, followed by miRNAs with a length of 20 nt, 21 nt, and 23 nt (Figure 1A). After comparing with GenBank, Rfam, and the reference genome, 93.59% of clean reads were identified as miRNA, and the remaining part included rRNA, scRNA, snRNA, snoRNA, tRNA, miRNA editing, and unann (Figure 1B). Approximately 96.15% of the tags were aligned to the reference genome (Bos_taurus_Ensembl_94). There were 491 bovine miRNAs and 462 known miRNAs. In addition, 122 new miRNAs were also predicted (Supplementary File S1).
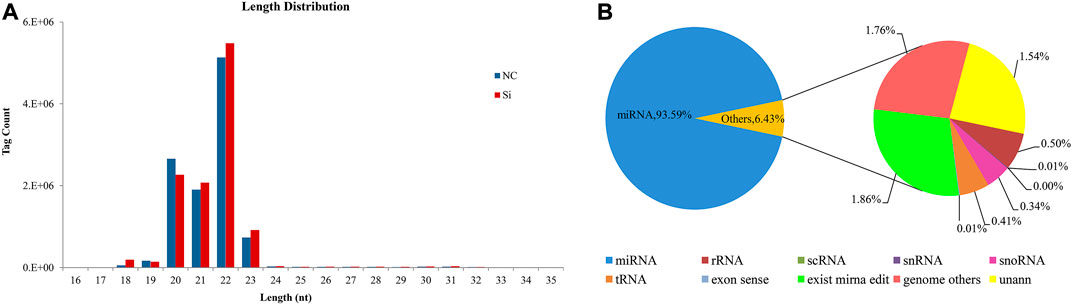
FIGURE 1. Correlation analysis of small RNA sequencing data. (A) Statistical analysis of small RNA fragment size. (B) Statistical analysis of the types of small RNA fragments after comparison with the database, including miRNA (existing miRNAs, known miRNAs and novel miRNAs), rRNA, scRNA, snRNA, snoRNA, tRNA, exon sense, miRNA editing, other genome, and unann.
Analysis of differentially expressed miRNAs
The DESeq2 (V1.20.0) software was used to compare the miRNAs between the si group and the NC group, and 28 DE-miRNAs were obtained, including 25 known and three new miRNAs. Among them, 16 upregulated miRNAs and 12 downregulated miRNAs were in the si group (Figure 2A). In general, the difference between the interference ACSL1 gene (si group) and the control group (NC group) was highly correlated with the expression of these miRNAs. The clustering patterns of these 28 DE-miRNAs are shown in (Figure 2B).
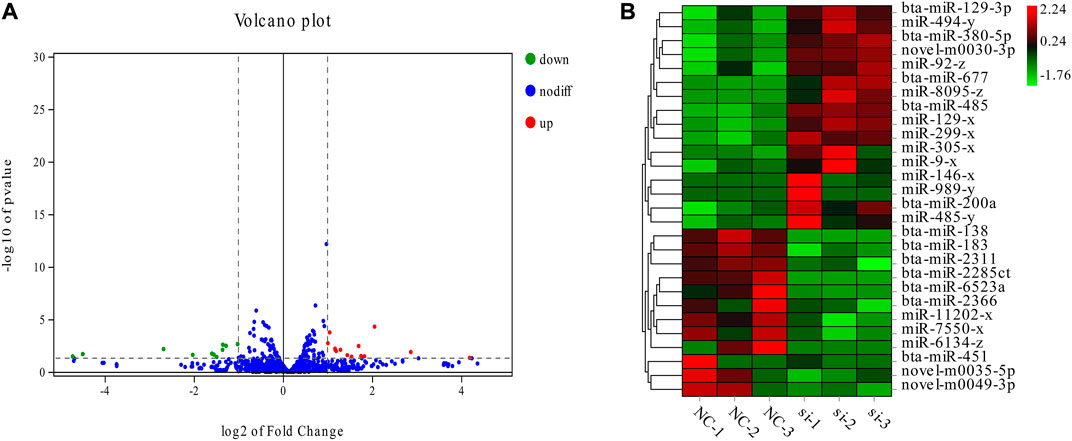
FIGURE 2. Statistical analysis of DE-miRNA. (A) The expression of miRNA volcano. The green dots on the left represent miRNAs that are significantly downregulated; the blue dots represent miRNAs that were not significantly different; and the red dots on the right represent miRNAs that were significantly upregulated. (B) Cluster map of differentially expressed miRNAs. Red indicates elevated expression and green indicates downregulated expression.
GO and KEGG enrichment analysis of differentially expressed miRNA
TargetScan (Version: 7.0) and miRanda (v3.3a) was used to predict DE-miRNA target genes to determine their biological functions. The 28 DE-miRNAs predicted a total of 6,828 target genes (Supplementary File S2). GO enrichment analysis showed that these target genes participated in 1,111 significantly enriched functional classifications (Q-value < 0.05). Biological processes contained the most enriched genes with 817 GO terms, followed by cell components with 203 GO terms and molecular functions with 91 GO terms (Supplementary File S3). The enriched GO terms were mainly related to cellular processes, biological regulation, metabolic processes, cellular parts, binding, and catalytic activity (Figure 3A). KEGG results indicated that the target genes of DE-miRNAs were significantly enriched in 81 signaling pathways (Q-value < 0.05) (Supplementary File S4). The enriched pathways included MAPK signaling pathway, metabolic pathway, AMPK signaling pathway, fatty acid metabolism, PI3K-Akt signaling pathway, glycerophospholipid metabolism, fatty acid elongation, steroid biosynthesis, and glucagon signaling pathway (Figure 3B).
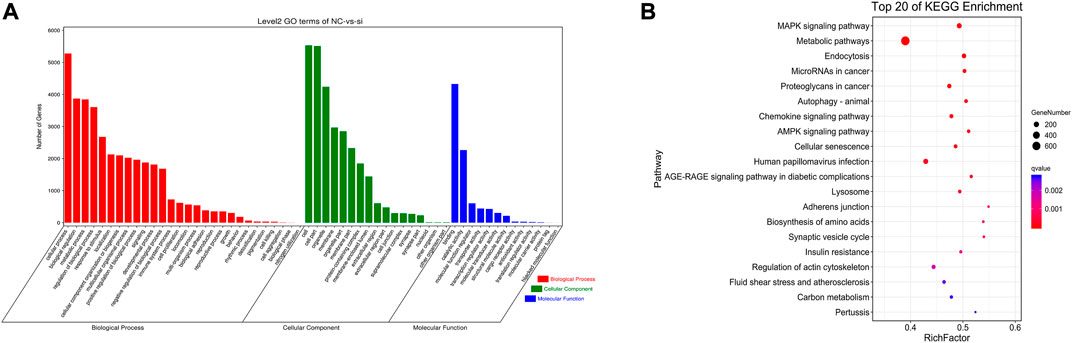
FIGURE 3. Functional enrichment analysis of DE-miRNAs. (A) GO enrichment analysis of target genes of DE-miRNAs. (B) Top 20 KEGG signaling pathways enriched by DE-miRNAs target genes.
Validation of differentially expressed miRNAs by qRT-PCR
The relative expression of 10 miRNAs was quantified by qRT-PCR to verify the differentially expressed miRNA (Figure 4). All selected DE-miRNAs showed consistent expression patterns between RNA-seq and qRT-PCR results, confirming the sequencing results.
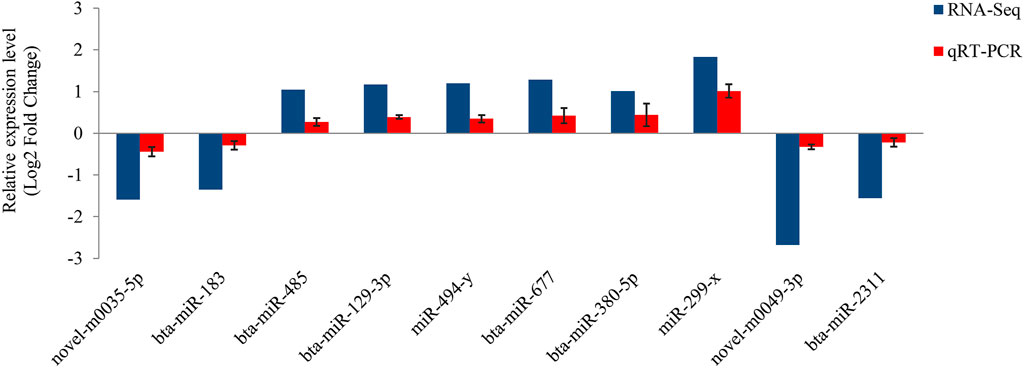
FIGURE 4. Validation of the miRNA-Seq results. The qRT-PCR validation was performed on 10 DE-miRNAs. These data show the mean ± SD for three replicates. Error bars indicate the standard deviation.
Construction of the miRNA-mRNA interaction network
The background genes related to fatty acid metabolism signaling pathways were screened to further understand and visualize the interaction between fat-related differentially expressed mRNAs (DEMs) and differentially expressed miRNAs (DE-miRNAs) (Figure 5A). Then these genes were used as target genes to construct a miRNA-mRNA interaction network (Figure 5B). In the network, 60 interactions were identified, and several of the most important DE-miRNAs were novel-m0035-5p, bta-miR-485, bta-miR-200a, bta-miR-677, miR-9-x, and miR-129-x.
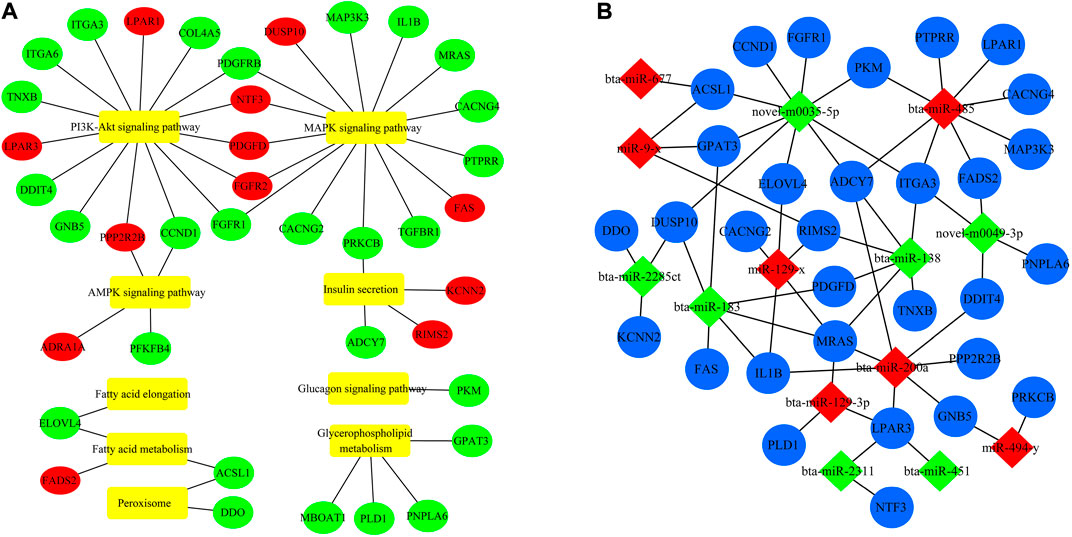
FIGURE 5. Interaction network of DE-miRNAs. (A) Network analysis of DE-miRNA target genes and their enrichment pathways. Red shows the upregulated mRNA. Green represents downregulated mRNA, and yellow represents the enriched signaling pathways. (B) Co-expression network of DE-miRNA and its target genes. Red is the miRNA whose expression is upregulated. Green represents downregulated miRNA, and blue represents mRNA.
Discussion
Fat deposition in muscle involves a series of processes, such as preadipocyte proliferation, preadipocyte differentiation, and lipid metabolism, and is regulated by related functional genes (Cai et al., 2018). MicroRNAs, as an important regulatory element after gene transcription, have been widely reported for their role in regulating lipid metabolism and synthesis (Du J et al., 2018; Jin et al., 2019; Khan et al., 2020; Zhang et al., 2020; Zhou et al., 2020). Therefore, it is necessary to understand the molecular mechanism of miRNAs in regulating the synthesis of unsaturated fatty acids (UFAs)-related genes to improve the nutritional value of beef.
In this study, 1,075 miRNAs were identified, of which 122 miRNAs were novel. Compared with the NC group, 28 miRNAs were identified as differentially expressed miRNAs. The DE-miRNAs included known lipid metabolism-related miRNAs such as miR-200a (Zhang et al., 2018), as well as newly discovered miRNAs novel-m0035-5p and novel-m0049-3p. GO and KEGG pathway enrichment analyses of miRNA target genes were carried out to reveal the potential function of DE-miRNAs. GO enrichment results showed that DE-miRNAs were mainly involved in cell processes, biological regulation, metabolic process, cellular parts, and binding. The results of KEGG analysis showed that DE-miRNAs target genes were mainly significantly enriched in 81 signal pathways (Q < 0.05). Nine pathways (Table 2) related to the synthesis of UFAs were selected for the follow-up research. The PI3K-Akt signaling pathway is a signaling pathway related to insulin (Pessin and Saltiel, 2000). Insulin regulates lipogenesis, fatty acid oxidation, VLDL-TG assembly, and secretion in goose liver cells through PI3K-Akt-mTOR to mediate lipid deposition (Han et al., 2015). Also, insulin and other hormones, such as glucagon, coordinate and regulate multiple biological responses. Insulin stimulates glucose transport in surrounding tissues, such as muscle and adipose tissue, inhibits glycogen synthesis and gluconeogenesis in the liver, and stimulates protein synthesis and lipogenesis (Wang and Sul, 1998). The MAPK signaling pathway can control biological processes via various cellular mechanisms involving the activation/inhibition of related factors (Yue and Lopez, 2020). Studies have found that miR-145 inhibits the lipid production of bovine preadipocytes by reducing the activity of PI3K/Akt and MAPK signaling pathways (Wang et al., 2020). Wu et al. found that miR-29a targeting CTRP6 inhibited the proliferation of pig muscle and subcutaneous adipocytes via the MAPK signaling pathway but promoteed differentiation (Wu et al., 2017). AMPK is one of the important enzymes that regulate cell energy metabolism and participates in cell metabolism. Activating AMPK in 3T3-L1 cells can inhibit the expression of key genes C/EBPβ, PPARγ, and C/EBPα in adipogenesis (Lee et al., 2012). We also found that more genes related to fat differentiation, such as PPARγ, FASN, and SCD5, are enriched in the AMPK signaling pathway. The enzymes required for β-oxidation of fatty acyl-CoA are present in peroxisomes and mitochondria. These enzymes promote the β-oxidation of long-chain and ultra-long-chain fatty acids in peroxisomes (Latruffe et al., 2001). They may also play a key role in regulating cellular senescence in related biochemical processes (Titorenko and Terlecky, 2011). An miRNA-mRNA interaction network was constructed to screen out miRNAs and mRNAs that may be related to UFA synthesis more intuitively and accurately. Here nine signaling pathways related to lipid metabolism were selected and then the background genes in the pathways were intersected with the differential mRNA sequenced from the previous transcriptome (Bai et al., 2021). These intersecting mRNAs and differential miRNAs were used to construct an interaction network. In the interaction network, the novel-m0035-5p and bta-miR-485 were found to simultaneously target multiple differential mRNAs. Most of the research on miR-485 is related to human cancer. A recent report discovered that miR-485 affected the content of triglycerides (TGs) and cholesterol (CHOL) in milk fat by targeting the DTX4 gene (Liu et al., 2021).
In addition, several pairs of miRNA-mRNA targeting relationships of interest were identified, such as novel-m0035-5p—ACSL1, novel-m0035-5p—ELOVL4, bta-miR-677—ACSL1, bta-miR-485—FADS2, miR-129-x—ELOVL4, and miR-9-x—ACSL1. Studies have reported that variants of human fatty acid desaturase 1 (FADS1, encoding delta-5 desaturase) and fatty acid desaturase 2 (FADS2, encoding delta-6 desaturase) are associated with PUFAs (PUFA) in the blood and long-chain (LC) PUFA levels have a strong correlation (Glaser et al., 2010). FADS2 gene polymorphism affects the content of unsaturated fatty acids (Gol et al., 2018; Proskura et al., 2019). In addition, studies have found that > C20 PUFAs synthesized by FADS2 play an important role in regulating liver triacylglycerol and cholesterol accumulation during PUFA deficiency (Hayashi et al., 2021). As the key rate-limiting enzyme for the synthesis of >20 PUFAs, FADS2 plays an important role in regulating the content of arachidonic acid (Stoffel et al., 2014). Hepatic steatosis was observed in FADS2-knockout mice that were fed a diet containing C18 PUFA but no C20 PUFA, and hepatic steatosis was reduced by administering arachidonic acid. Related studies have found that interference or overexpression of the ACSL1 gene affects the expression of the downstream gene COX2 and consequently the content of arachidonic acid (Cao et al., 2020).
In the study of ACSL1 gene promoter transcription regulation, several important transcription factors, were identified among which Sp1, the main negative transcription regulator, significantly reduced the activity of the ACSL1 promoter (Zhao et al., 2016). Interestingly, Sp1 could bind to the promoter region of FADS2 to increase the promoter activity of FADS2 (Li et al., 2019). Thus, it was hypothesized that ACSL1 acted as a regulatory switch between the FADS2 and COX2 genes, which worked together to maintain the homeostasis of arachidonic acid content in the body. Very long chain fatty acid extension-4 (ELOVL4) is a fatty acid condensing enzyme that mediates the biosynthesis of very long chain PUFAs (VLC-PUFA; ≥ C28) in a limited number of tissues (Agbaga et al., 2014). Previous studies have shown that ELOVL4 is required for the synthesis of C28 and C30 saturated fatty acids (VLC-FA) and C28-C38 very long-chain PUFAs (VLC-PUFA) (Agbaga et al., 2008), which are present in retinal photoreceptors, skin, sperm, and testis, which play an important role in normal and long-term function (McMahon et al., 2011; Harkewicz et al., 2012). Similar to fatty acid synthesis, the activity of enzymes involved in fatty acid extension and desaturation appears to be regulated primarily at the transcriptional level rather than through post-translational protein modification (Guillou et al., 2010). Therefore, it is necessary to understand the regulation of ELOVL4 by miRNAs as post-transcriptional regulators.
MicroRNAs bind to specific complementary sites of the target mRNA, thereby degrading mRNA or inhibiting its translation and regulating protein expression (Bartel, 2009). This study showed that novel-m0035-5p—ACSL1, novel-m0035-5p—ELOVL4, and bta-miR-485—FADS2 had the same differential trend. However, this did not imply that the key role of these miRNAs had been overlooked. The expression of miRNAs varied in different tissues and at different stages. In addition, miRNA coordinated biological functions by targeting genes. Therefore, the mutual targeting effects of different tissues and different stages were also different (Wienholds and Plasterk, 2005; Bushati and Cohen, 2007). In addition, ACSL1, FADS2, and ELOVL4 were significantly enriched in fatty acid metabolism and fatty acid extension pathways. The three aspects of differential genes, signal pathways, and gene function were comprehensively considered to determine the miRNAs related to the synthesis of unsaturated fatty acids. Future studies would verify the functional regulation of unsaturated fatty acid synthesis by these miRNAs.
Conclusion
RNA-Seq technology was used to identify miRNAs associated with UFAs synthesis by interfering with and none-interfering the ACSL1 gene. Functional enrichment results indicated that DE-miRNAs were mainly involved in unsaturated fatty acid synthesis-related signaling pathways. Through the miRNA-mRNA interaction network, several key miRNA-mRNA targeting relationships were screened, including novel-m0035-5p—ACSL1, novel-m0035-5p—ELOVL4, miR-9-x—ACSL1, bta-miR-677—ACSL1, miR-129-x—ELOVL4 and bta-miR-485—FADS2. These miRNAs might regulate unsaturated fatty acid synthesis in bovine adipocytes by targeting these genes.
Data availability statement
The data presented in the study are deposited in the NCBI repository, https://www.ncbi.nlm.nih.gov/bioproject/PRJNA856126 and the accession number: PRJNA856126.
Ethics statement
This animal study was reviewed and approved by the Faculty Animal Policy and Welfare Committee of Gansu Agricultural University (Ethic approval file No. GSAU-Eth-AST-2021-25).
Author contributions
Conceptualization, XL and ZZ; methodology, XL, YB, BS, and ZZ; validation, XL, YB, JL, YM, and ZC; formal analysis, XL, BS, YB, and SL; investigation, ZZ and XL; resources, YL and JW; writing—original draft preparation, XL; writing—review and editing, XL and ZZ; supervision, ZZ and XH; project administration, ZZ; funding acquisition, ZZ and JH All authors have read and agreed to the published version of the manuscript. Authorship have read and agreed to the published version of the manuscript.
Funding
This research was funded by the National Natural Science Foundation of China (NO. 31860631); Young Doctor Fund Project of Gansu Provincial Department of Education (2021QB-027); Beef Cattle Genome Research (GSAU-ZL2015-031-01).
Acknowledgments
We thank all the members of Gansu Key Laboratory of Herbivorous Animal Biotechnology of the College of Animal Science and Technology, Gansu Agricultural University who contributed their efforts to these experiments. We also thank LetPub (www.letpub.com) for its linguistic assistance during the preparation of this manuscript.
Conflict of interest
The authors declare that the research was conducted in the absence of any commercial or financial relationships that could be construed as a potential conflict of interest.
Publisher’s note
All claims expressed in this article are solely those of the authors and do not necessarily represent those of their affiliated organizations, or those of the publisher, the editors and the reviewers. Any product that may be evaluated in this article, or claim that may be made by its manufacturer, is not guaranteed or endorsed by the publisher.
Supplementary material
The Supplementary Material for this article can be found online at: https://www.frontiersin.org/articles/10.3389/fgene.2022.994806/full#supplementary-material
Supplementary File S1 | Details of differentially expressed miRNAs.
Supplementary File S2 | Details of differentially expressed miRNAs target genes.
Supplementary File S3 | GO analysis of differentially expressed miRNAs target genes.
Supplementary File S4 | KEGG analysis of differentially expressed miRNAs target genes.
References
Agbaga, M. P., Brush, R. S., Mandal, M. N., Henry, K., Elliott, M. H., and Anderson, R. E. (2008). Role of Stargardt-3 macular dystrophy protein (ELOVL4) in the biosynthesis of very long chain fatty acids. Proc. Natl. Acad. Sci. U. S. A. 105, 12843–12848. doi:10.1073/pnas.0802607105
Agbaga, M. P., Logan, S., Brush, R. S., and Anderson, R. E. (2014). Biosynthesis of very long-chain polyunsaturated fatty acids in hepatocytes expressing ELOVL4. Adv. Exp. Med. Biol. 801, 631–636. doi:10.1007/978-1-4614-3209-8_79
Bai, Y., Li, X., Chen, Z., Li, J., Tian, H., Ma, Y., et al. (2021). Interference with ACSL1 gene in bovine adipocytes: Transcriptome profiling of mRNA and lncRNA related to unsaturated fatty acid synthesis. Front. Vet. Sci. 8, 788316. doi:10.3389/fvets.2021.788316
Barcelo-Coblijn, G., and Murphy, E. J. (2009). Alpha-linolenic acid and its conversion to longer chain n-3 fatty acids: Benefits for human health and a role in maintaining tissue n-3 fatty acid levels. Prog. Lipid Res. 48, 355–374. doi:10.1016/j.plipres.2009.07.002
Bartel, D. P. (2009). MicroRNAs: Target recognition and regulatory functions. Cell 136, 215–233. doi:10.1016/j.cell.2009.01.002
Bushati, N., and Cohen, S. M. (2007). microRNA functions. Annu. Rev. Cell Dev. Biol. 23, 175–205. doi:10.1146/annurev.cellbio.23.090506.123406
Cai, H., Li, M., Sun, X., Plath, M., Li, C., Lan, X., et al. (2018). Global transcriptome analysis during adipogenic differentiation and involvement of transthyretin gene in adipogenesis in cattle. Front. Genet. 9, 463. doi:10.3389/fgene.2018.00463
Cao, Y., Wang, S., Liu, S., Wang, Y., Jin, H., Ma, H., et al. (2020). Effects of long-chain fatty acyl-CoA synthetase 1 on diglyceride synthesis and arachidonic acid metabolism in sheep adipocytes. Int. J. Mol. Sci. 21, E2044. doi:10.3390/ijms21062044
Cui, M., Wang, Y., Sun, B., Xiao, Z., Ye, L., and Zhang, X. (2014). MiR-205 modulates abnormal lipid metabolism of hepatoma cells via targeting acyl-CoA synthetase long-chain family member 1 (ACSL1) mRNA. Biochem. Biophys. Res. Commun. 444, 270–275. doi:10.1016/j.bbrc.2014.01.051
Deb, B., Uddin, A., and Chakraborty, S. (2018). miRNAs and ovarian cancer: An overview. J. Cell. Physiol. 233, 3846–3854. doi:10.1002/jcp.26095
Deng, K., Ren, C., Fan, Y., Liu, Z., Zhang, G., Zhang, Y., et al. (2020). miR-27a is an important adipogenesis regulator associated with differential lipid accumulation between intramuscular and subcutaneous adipose tissues of sheep. Domest. Anim. Endocrinol. 71, 106393. doi:10.1016/j.domaniend.2019.106393
Du, J., Xu, Y., Zhang, P., Zhao, X., Gan, M., Li, Q., et al. (2018). MicroRNA-125a-5p affects adipocytes proliferation, differentiation and fatty acid composition of porcine intramuscular fat. Int. J. Mol. Sci. 19, E501. doi:10.3390/ijms19020501
Glaser, C., Heinrich, J., and Koletzko, B. (2010). Role of FADS1 and FADS2 polymorphisms in polyunsaturated fatty acid metabolism. Metabolism. 59, 993–999. doi:10.1016/j.metabol.2009.10.022
Gol, S., Pena, R. N., Rothschild, M. F., Tor, M., and Estany, J. (2018). A polymorphism in the fatty acid desaturase-2 gene is associated with the arachidonic acid metabolism in pigs. Sci. Rep. 8, 14336. doi:10.1038/s41598-018-32710-w
Guillou, H., Zadravec, D., Martin, P. G., and Jacobsson, A. (2010). The key roles of elongases and desaturases in mammalian fatty acid metabolism: Insights from transgenic mice. Prog. Lipid Res. 49, 186–199. doi:10.1016/j.plipres.2009.12.002
Han, C., Wei, S., He, F., Liu, D., Wan, H., Liu, H., et al. (2015). The regulation of lipid deposition by insulin in goose liver cells is mediated by the PI3K-AKT-mTOR signaling pathway. PLoS One 10, e0098759. doi:10.1371/journal.pone.0098759
Han, F., Zhou, L., Zhao, L., Wang, L., Liu, L., Li, H., et al. (2021). Identification of miRNA in sheep intramuscular fat and the role of miR-193a-5p in proliferation and differentiation of 3T3-L1. Front. Genet. 12, 633295. doi:10.3389/fgene.2021.633295
Harkewicz, R., Du, H., Tong, Z., Alkuraya, H., Bedell, M., Sun, W., et al. (2012). Essential role of ELOVL4 protein in very long chain fatty acid synthesis and retinal function. J. Biol. Chem. 287, 11469–11480. doi:10.1074/jbc.M111.256073
Hayashi, Y., Lee-Okada, H. C., Nakamura, E., Tada, N., Yokomizo, T., Fujiwara, Y., et al. (2021). Ablation of fatty acid desaturase 2 (FADS2) exacerbates hepatic triacylglycerol and cholesterol accumulation in polyunsaturated fatty acid-depleted mice. FEBS Lett. 595, 1920–1932. doi:10.1002/1873-3468.14134
Jin, Y., Wang, J., Zhang, M., Zhang, S., Lei, C., Chen, H., et al. (2019). Role of bta-miR-204 in the regulation of adipocyte proliferation, differentiation, and apoptosis. J. Cell. Physiol. 234, 11037–11046. doi:10.1002/jcp.27928
Khan, R., Raza, S., Junjvlieke, Z., Wang, X., Wang, H., Cheng, G., et al. (2020). Bta-miR-149-5p inhibits proliferation and differentiation of bovine adipocytes through targeting CRTCs at both transcriptional and post-transcriptional levels. J. Cell. Physiol. 235, 5796–5810. doi:10.1002/jcp.29513
Latruffe, N., Cherkaoui, M. M., Nicolas-Frances, V., Jannin, B., Clemencet, M. C., Hansmannel, F., et al. (2001). Peroxisome-proliferator-activated receptors as physiological sensors of fatty acid metabolism: Molecular regulation in peroxisomes. Biochem. Soc. Trans. 29, 305–309. doi:10.1042/0300-5127:0290305
Lee, S. K., Lee, J. O., Kim, J. H., Kim, N., You, G. Y., Moon, J. W., et al. (2012). Coenzyme Q10 increases the fatty acid oxidation through AMPK-mediated PPARα induction in 3T3-L1 preadipocytes. Cell. Signal. 24, 2329–2336. doi:10.1016/j.cellsig.2012.07.022
Li, L. O., Ellis, J. M., Paich, H. A., Wang, S., Gong, N., Altshuller, G., et al. (2009). Liver-specific loss of long chain acyl-CoA synthetase-1 decreases triacylglycerol synthesis and beta-oxidation and alters phospholipid fatty acid composition. J. Biol. Chem. 284, 27816–27826. doi:10.1074/jbc.M109.022467
Li, Y., Zhao, J., Dong, Y., Yin, Z., Li, Y., Liu, Y., et al. (2019). Sp1 is involved in vertebrate LC-PUFA biosynthesis by upregulating the expression of liver desaturase and elongase genes. Int. J. Mol. Sci. 20, E5066. doi:10.3390/ijms20205066
Lian, S., Guo, J. R., Nan, X. M., Ma, L., Loor, J. J., and Bu, D. P. (2016). MicroRNA Bta-miR-181a regulates the biosynthesis of bovine milk fat by targeting ACSL1. J. Dairy Sci. 99, 3916–3924. doi:10.3168/jds.2015-10484
Liu, J., Jiang, P., Iqbal, A., Ali, S., Gao, Z., Pan, Z., et al. (2021). MiR-485 targets the DTX4 gene to regulate milk fat synthesis in bovine mammary epithelial cells. Sci. Rep. 11, 7623. doi:10.1038/s41598-021-87139-5
Livak, K. J., and Schmittgen, T. D. (2001). Analysis of relative gene expression data using real-time quantitative PCR and the 2(-Delta Delta C(T)) Method. Methods 25, 402–408. doi:10.1006/meth.2001.1262
Lopez-Huertas, E. (2010). Health effects of oleic acid and long chain omega-3 fatty acids (EPA and DHA) enriched milks. A review of intervention studies. Pharmacol. Res. 61, 200–207. doi:10.1016/j.phrs.2009.10.007
Ma, X., Wei, D., Cheng, G., Li, S., Wang, L., Wang, Y., et al. (2018). Bta-miR-130a/b regulates preadipocyte differentiation by targeting PPARG and CYP2U1 in beef cattle. Mol. Cell. Probes 42, 10–17. doi:10.1016/j.mcp.2018.10.002
Marventano, S., Kolacz, P., Castellano, S., Galvano, F., Buscemi, S., Mistretta, A., et al. (2015). A review of recent evidence in human studies of n-3 and n-6 PUFA intake on cardiovascular disease, cancer, and depressive disorders: Does the ratio really matter? Int. J. Food Sci. Nutr. 66, 611–622. doi:10.3109/09637486.2015.1077790
McMahon, A., Butovich, I. A., and Kedzierski, W. (2011). Epidermal expression of an Elovl4 transgene rescues neonatal lethality of homozygous Stargardt disease-3 mice. J. Lipid Res. 52, 1128–1138. doi:10.1194/jlr.M014415
Paul, D. S., Grevengoed, T. J., Pascual, F., Ellis, J. M., Willis, M. S., and Coleman, R. A. (2014). Deficiency of cardiac Acyl-CoA synthetase-1 induces diastolic dysfunction, but pathologic hypertrophy is reversed by rapamycin. Biochim. Biophys. Acta 1841, 880–887. doi:10.1016/j.bbalip.2014.03.001
Pessin, J. E., and Saltiel, A. R. (2000). Signaling pathways in insulin action: Molecular targets of insulin resistance. J. Clin. Invest. 106, 165–169. doi:10.1172/JCI10582
Proskura, W. S., Liput, M., Zaborski, D., Sobek, Z., Yu, Y. H., Cheng, Y. H., et al. (2019). The effect of polymorphism in the FADS2 gene on the fatty acid composition of bovine milk. Arch. Anim. Breed. 62, 547–555. doi:10.5194/aab-62-547-2019
Ren, H., Xiao, W., Qin, X., Cai, G., Chen, H., Hua, Z., et al. (2020). Myostatin regulates fatty acid desaturation and fat deposition through MEF2C/miR222/SCD5 cascade in pigs. Commun. Biol. 3, 612. doi:10.1038/s42003-020-01348-8
Shan, B., Yan, M., Yang, K., Lin, W., Yan, J., Wei, S., et al. (2022). MiR-218-5p affects subcutaneous adipogenesis by targeting ACSL1, a novel candidate for pig fat deposition. Genes (Basel) 13, 260. doi:10.3390/genes13020260
Shukla, G. C., Singh, J., and Barik, S. (2011). MicroRNAs: Processing, maturation, target recognition and regulatory functions. Mol. Cell. Pharmacol. 3, 83–92.
Sijben, J. W., and Calder, P. C. (2007). Differential immunomodulation with long-chain n-3 PUFA in health and chronic disease. Proc. Nutr. Soc. 66, 237–259. doi:10.1017/S0029665107005472
Simopoulos, A. P. (1991). Omega-3 fatty acids in health and disease and in growth and development. Am. J. Clin. Nutr. 54, 438–463. doi:10.1093/ajcn/54.3.438
Stoffel, W., Hammels, I., Jenke, B., Binczek, E., Schmidt-Soltau, I., Brodesser, S., et al. (2014). Obesity resistance and deregulation of lipogenesis in Δ6-fatty acid desaturase (FADS2) deficiency. EMBO Rep. 15, 110–120. doi:10.1002/embr.201338041
Tian, H. S., Su, X. T., Han, X. M., Zan, L. S., H, J., Luo, Y. Z., et al. (2020). Effects of silencing ACSL1 gene by siRNA on the synthesis of unsaturated fatty acids in adipocytes of qinchuan beef cattle. J. Agric. Biotechnol. 10, 1722–1732. doi:10.3969/j.issn.1674-7968.2020.10.002
Tian, W. H., Wang, Z., Yue, Y. X., Li, H., Li, Z. J., Han, R. L., et al. (2019). miR-34a-5p increases hepatic triglycerides and total cholesterol levels by regulating ACSL1 protein expression in laying hens. Int. J. Mol. Sci. 20, E4420. doi:10.3390/ijms20184420
Titorenko, V. I., and Terlecky, S. R. (2011). Peroxisome metabolism and cellular aging. Traffic 12, 252–259. doi:10.1111/j.1600-0854.2010.01144.x
Tufekci, K. U., Meuwissen, R. L., and Genc, S. (2014). The role of microRNAs in biological processes. Methods Mol. Biol. 1107, 15–31. doi:10.1007/978-1-62703-748-8_2
Wang, D., and Sul, H. S. (1998). Insulin stimulation of the fatty acid synthase promoter is mediated by the phosphatidylinositol 3-kinase pathway. Involvement of protein kinase B/Akt. J. Biol. Chem. 273, 25420–25426. doi:10.1074/jbc.273.39.25420
Wang, L., Zhang, S., Cheng, G., Mei, C., Li, S., Zhang, W., et al. (2020). MiR-145 reduces the activity of PI3K/Akt and MAPK signaling pathways and inhibits adipogenesis in bovine preadipocytes. Genomics 112, 2688–2694. doi:10.1016/j.ygeno.2020.02.020
Wienholds, E., and Plasterk, R. H. (2005). MicroRNA function in animal development. FEBS Lett. 579, 5911–5922. doi:10.1016/j.febslet.2005.07.070
Widmann, P., Nuernberg, K., Kuehn, C., and Weikard, R. (2011). Association of an ACSL1 gene variant with polyunsaturated fatty acids in bovine skeletal muscle. BMC Genet. 12, 96. doi:10.1186/1471-2156-12-96
Wu, W., Zhang, J., Zhao, C., Sun, Y., Pang, W., and Yang, G. (2017). CTRP6 regulates porcine adipocyte proliferation and differentiation by the AdipoR1/MAPK signaling pathway. J. Agric. Food Chem. 65, 5512–5522. doi:10.1021/acs.jafc.7b00594
Yue, J., and Lopez, J. M. (2020). Understanding MAPK signaling pathways in apoptosis. Int. J. Mol. Sci. 21, E2346. doi:10.3390/ijms21072346
Zhang, Q., Ma, X. F., Dong, M. Z., Tan, J., Zhang, J., Zhuang, L. K., et al. (2020). MiR-30b-5p regulates the lipid metabolism by targeting PPARGC1A in Huh-7 cell line. Lipids Health Dis. 19, 76. doi:10.1186/s12944-020-01261-3
Zhang, Y., Wu, X., Liang, C., Bao, P., Ding, X., Chu, M., et al. (2018). MicroRNA-200a regulates adipocyte differentiation in the domestic yak Bos grunniens. Gene 650, 41–48. doi:10.1016/j.gene.2018.01.054
Zhao, Z., Abbas, R. S., Tian, H., Shi, B., Luo, Y., Wang, J., et al. (2020). Effects of overexpression of ACSL1 gene on the synthesis of unsaturated fatty acids in adipocytes of bovine. Arch. Biochem. Biophys. 695, 108648. doi:10.1016/j.abb.2020.108648
Zhao, Z. D., Zan, L. S., Li, A. N., Cheng, G., Li, S. J., Zhang, Y. R., et al. (2016). Characterization of the promoter region of the bovine long-chain acyl-CoA synthetase 1 gene: Roles of E2F1, Sp1, KLF15, and E2F4. Sci. Rep. 6, 19661. doi:10.1038/srep19661
Keywords: micrornas, ACSL1, bovine adipocytes, unsaturated fatty acids, RNA-seq
Citation: Li X, Bai Y, Li J, Chen Z, Ma Y, Shi B, Han X, Luo Y, Hu J, Wang J, Liu X, Li S and Zhao Z (2022) Transcriptional analysis of microRNAs related to unsaturated fatty acid synthesis by interfering bovine adipocyte ACSL1 gene. Front. Genet. 13:994806. doi: 10.3389/fgene.2022.994806
Received: 15 July 2022; Accepted: 06 September 2022;
Published: 26 September 2022.
Edited by:
Hongyu Liu, Anhui Agricultural University, ChinaCopyright © 2022 Li, Bai, Li, Chen, Ma, Shi, Han, Luo, Hu, Wang, Liu, Li and Zhao. This is an open-access article distributed under the terms of the Creative Commons Attribution License (CC BY). The use, distribution or reproduction in other forums is permitted, provided the original author(s) and the copyright owner(s) are credited and that the original publication in this journal is cited, in accordance with accepted academic practice. No use, distribution or reproduction is permitted which does not comply with these terms.
*Correspondence: Zhidong Zhao, zhaozd@gsau.edu.cn