- 1Department of Biology, University of Waterloo, Waterloo, ON, Canada
- 2Canadian Centre for Human Microbiome and Probiotics Research, Lawson Health Research Institute, London, ON, Canada
- 3Departments of Microbiology and Immunology, and Surgery, The University of Western Ontario, London, ON, Canada
- 4Yellow Island Aquaculture, Heriot Bay, BC, Canada
- 5Department of Pathobiology, University of Guelph, Guelph, ON, Canada
- 6Department of Surgery, The University of Western Ontario, St. Joseph’s Health Care London, London, ON, Canada
Microbial management is central to aquaculture’s efficiency. Pediococcus acidilactici MA18/5M has shown promising results promoting growth, modulation of the immune response, and disease resistance in many fishes. However, the mechanisms through which this strain confers health benefits in fish are poorly understood, particularly in Pacific salmonid models. Briefly, the aims of this study were to i) assess the protective effects of P. acidilactici MA18/5M by examining gut barrier function and the expression of tight junction (TJ) and immune genes in vitro and in vivo, and ii) to determine the protective effects of this strain against a common saltwater pathogen, Vibrio anguillarum J382. An in vitro model of the salmonid gut was employed utilizing the cell line RTgutGC. Barrier formation and integrity assessed by TEER measurements in RTgutGC, showed a significant decrease in resistance in cells exposed only to V. anguillarum J382 for 24 h, but pre-treatment with P. acidilactici MA18/5M for 48 h mitigated these effects. While P. acidilactici MA18/5M did not significantly upregulate tight junction and immune molecules, pre-treatment with this strain protected against pathogen-induced insults to the gut barrier. In particular, the expression of ocldn was significantly induced by V. anguillarum J382, suggesting that this molecule might play a role in the host response against this pathogen. To corroborate these observations in live fish, the effects of P. acidilactici MA18/5M was evaluated in Chinook salmon reared in real aquaculture conditions. Supplementation with P. acidilactici MA18/5M had no effect on Chinook salmon growth parameters after 10 weeks. Interestingly, histopathological results did not show alterations associated with P. acidilactici MA18/5M supplementation, indicating that this strain is safe to be used in the industry. Finally, the expression pattern of transcripts encoding TJ and immune genes in all the treatments suggest that variation in expression is more likely to be due to developmental processes rather than P. acidilactici MA18/5M supplementation. Overall, our results showed that P. acidilactici MA18/5M is a safe strain for use in fish production, however, to assess the effects on growth and immune response previously observed in other salmonid species, an assessment in adult fish is needed.
1 Introduction
In finfish aquaculture, the incorporation of healthy functional constituents into aquafeeds has become a potential tool to improve fish growth, stress tolerance, and disease resistance (1–3). Functional food components have been demonstrated to increase the respiratory burst activity, cytokine activity, complement system, phagocytosis, among others (4–7). To date, several immunostimulants and functional feed ingredients have been tested in aquaculture species, among which prebiotics, probiotics, vitamins, and synbiotics are notable (6, 8–11). Probiotic strains have been broadly studied in terrestrial and aquatic species and have shown promising results for the pork, poultry, and aquaculture industry (7, 12–15). This non‐polluting and efficient biological alternative to antibiotics is commercially available often at reasonable prices, making them a potential alternative for large-scale production (7–9, 14).
Probiotics are defined as “live microorganisms that, when administered in adequate amounts, confer a health benefit on the host” (16). In fish, some probiotic strains have been shown to improve fish growth and feed conversion rates, modulate the gastrointestinal microbial community, prevent bacterial diseases, and contribute to the digestive and nutritional processes of the host (15, 17–19). The fish gut constitutes the largest interface for host-microbe interactions and harbors the most abundant and diverse microbial community that can modulate overall host physiology (12). Therefore, modulation of host health at this interface may prove advantageous in aquaculture systems where optimal fish health is directly associated with profits in this highly margin-sensitive industry.
Recent reports have pointed at the potential of using Pediococcus acidilactici MA18/5M in terrestrial livestock as well as marine and freshwater fish, particularly salmonid species such as Atlantic salmon (20). P. acidilactici MA18/5M is a homofermentative Gram-positive cocci and is a member of the lactic acid bacteria (LAB) group. This strain has shown promising results promoting growth performance, modulation of both innate and adaptive immunity, improved survival rates, and resistance to infection (12, 17–20). To date, available research has demonstrated that P. acidilactici increases growth, blood leucocyte levels, immunoglobulin (Ig) levels, serum lysozyme activity, and transcripts encoding il1b, il8, il10, and tnfa, among others, in salmonids species utilized in aquaculture such as rainbow trout (Oncorhynchus mykiss) (21–23) and Atlantic salmon (Salmo salar) (1, 24–26).
The European Union has approved the use of this probiotic for aquaculture applications. Still, data supporting the use in North American species has yet to be recognized by the local regulatory agencies (18, 21). Interestingly, the Canadian Food Inspection Agency (CFIA) recently conducted consultations to evaluate the use of dehydrated P. acidilactici in gut modifier products for livestock species, including fish (27). In salmonids, the administration of this probiotic strain has been associated with a robust nonspecific immune response (both at the mucosal interface and at a systemic level), improving growth performance, modifying microbiota composition, and increasing disease resistance (20, 22, 23). The applicability of this strain for health enhancement of Pacific salmonid species has yet to be understood.
Salmonids constitute the most economically important family of finfish. In particular, Chinook salmon (Oncorhynchus tshawytscha) is the largest species of this family and presents potential economic and environmental advantages compared to farming of its Atlantic counterpart on the Pacific Northwest (28–31). However, Chinook salmon farming is hampered by the risk of escapees diluting the genetic diversity of wild populations and their poor tolerance to commercial production conditions (25, 26). Sterile triploid salmon effectively circumvents escapee issues, however these fish exhibit 10-30% higher mortality rate due to infectious disease compared to diploid fish (Data provided by Yellow Island Aquaculture Ltd.).
The fish gut is thought to be a primary site for pathogen attachment, proliferation, and entry into the bloodstream (32, 33). It serves as the primary site for digestion and nutrient absorption, immune modulation, osmoregulation, and acts as a barrier against pathobionts in the gut (33, 34). Notably, Vibrio anguillarum is a common aquatic pathogen that afflicts farmed fish worldwide, causing substantial financial losses to the industry (35). Therefore, investigating mechanisms that can prevent pathogen expansion, bolster gut barrier function and integrity will potentially prevent infections and sustainably improve productivity. There is currently no evidence for the effect of probiotic strain MA18/5M or other beneficial microbes in Chinook salmon.
Recent reports on a salmonid intestinal epithelial cell line derived from rainbow trout (O. mykiss), RTgutGC, have established the usefulness of this cell line as a model for functional studies on fish feed development based on gut barrier function and immune competence, as well as host-microbe interactions with viruses (36–40). Furthermore, by employing semipermeable membrane supports (Transwell®), it is possible to recapitulate the intestinal environment in vitro and conduct studies on the permeability and integrity of the cell monolayer (41).
The objectives of the present study are to i) investigate the effect of P. acidilactici MA18/5M supplementation on gut barrier function and the expression of tight junction and immune molecules, ii) determine the extent to which V. anguillarum disrupts barrier function, iii) assess whether P. acidilactici MA18/5M can protect monolayer integrity against pathogen-induced insults to the barrier, and iv) examine the effects of P. acidilactici MA18/5M on several physiological parameters of juvenile Chinook salmon.
2 Materials and methods
2.1 In vitro P. acidilactici MA18/5M trials
2.1.1 Tissue culture maintenance
The rainbow trout intestinal epithelial cell line RTgutGC was cultured in Leibovitz’s 15 media (HyClone, Cytiva), supplemented with 10% heat-inactivated fetal bovine serum (HI-FBS; Life Technologies), and incubated in plates or T75 flasks (Corning, Millipore Sigma) sealed with Parafilm (Bemis) at 22°C and atmospheric conditions. The medium was replaced every 3-4 days and cells were passaged when ≥80% confluent in a 1:2 to 1:4 sub-cultivation ratio, depending on the downstream experimental application. Cells were washed with 4 mL sterile PBS at room temperature, and residual buffer was aspirated with a glass Pasteur pipette (Fisher Scientific) connected to a vacuum line. Four mL of trypsin (0.05% w/v; Thermo Fisher Scientific) was then added and incubated for 10 min at room temperature on a flask vortex to facilitate detachment.
Cells were monitored periodically using a Nikon inverted microscope to ensure detachment from the plastic. Upon detachment, 8 mL of complete culture medium (L-15 + 10% FBS) was added to quench the trypsin protease activity. The suspension was then vigorously pipetted to break up clumps of cells, before the transfer of the cells to a 15 mL sterile conical tube from which a sample was taken for viable counting using a trypan blue (0.04%; Thermo Fisher Scientific) exclusion assay and the automated Countess cell counter (Invitrogen) prior to seeding into the cell culture dishes. The medium renewal was performed 24-48 h following trypsinization and seeding into new culture dishes to remove residual trypsin.
2.1.2 Bacterial strains and culture conditions
To determine the probiotic effect on RTgutGC, P. acidilactici MA18/5M (BioPower® PA, registration number 982989) was utilized. This strain was generously provided as a lyophilized powder by Lallemand Animal Nutrition Incorporated. P. acidilactici MA18/5M was routinely cultured anaerobically at 37°C in Mann, Rogosa, and Sharpe (MRS) medium (BD Difco) for each of the trials.
For RTgutGC cell stimulation trials, the Gram-negative pathogen V. anguillarum J382 (serotype O1) (42) isolated from winter Steelhead trout was obtained from Little Campbell River (British Columbia, Canada) and utilized for cell stimulation. Briefly, a single colony of V. anguillarum was grown in 2.5 mL of trypticase soy broth 2% sodium chloride (TSB 2% NaCl; Multicell Wisent, Quebec, Canada) at 20°C in a 16 mm diameter glass tube and placed in a shaker for 24 h at 200 rpm. After growth, 150 μL of the overnight culture were added to 150 mL of TSB 2% NaCl media using a 250 mL flask and incubated for 24 h at 20°C with aeration (200 rpm). After the overnight culture, the bacterial inoculum was centrifuged at 6,000 rpm at room temperature for 10 min. The pellet was washed thrice with PBS and centrifuged at 6,000 rpm at room temperature for 10 min, and finally resuspended in 25 mL of PBS (~8.6 × 108 CFU mL−1). The concentrated bacterial inoculum was serial diluted and quantified by plating onto TSA 2% NaCl for 48 h. Heat-killed V. anguillarum was prepared by transferring 1 mL of this inoculum to a 1.7 mL tube, which was then centrifuged at 10,000 rpm for 8 min at room temperature. The supernatant was discarded, and the bacterial pellet was resuspended in 1 mL of sterile PBS and incubated at 100°C for 30 min. Then, 100 μL of the heat-killed suspension was plated in TSA + 2% NaCl in triplicates to ensure sterility.
2.1.3 Coculture experiments
RTgutGC cells were cultured in 6- or 12-well plates (BD Falcon) for at least 3 weeks prior to the experiments to ensure that the cells established the brush border membrane and tight junction complexes. Frozen stocks of P. acidilactici MA18/5M and V. anguillarum J382 were streaked onto agar plates of the appropriate medium and incubated for 24 h. Single colonies were then re-streaked and incubated for another 24 h. Fresh single colonies were used to inoculate 3 mL of the appropriate growth medium and cultures were incubated for 48 h. Assuming that the concentration per area of cells at confluency is approximately 1.3 x 105 cells/cm2, the P. acidilactici MA18/5M was diluted to a final multiplicity of bacteria (MOB) of 1:100 gut cells to bacteria, while V. anguillarum was diluted to a final concentration of 2:1 MOB. Heat-killed V. anguillarum was diluted in like manner. The bacterial suspensions were mixed in the cell culture growth medium, and the spent cell culture medium was aspirated using a sterile glass Pasteur pipette connected to a vacuum line. The bacteria were then added to the RTgutGC cells and incubated for various durations (Figures 1–4). Cells were then harvested at specific time-points by aspirating the culture medium and adding 1 or 0.5 mL (for 6- or 12-well plates, respectively) of TRIzol reagent (Invitrogen), and removed by vigorously pipetting the RTgutGC cell lysate, which was then transferred to a 1.7 mL tube and stored at 4°C until further processing.
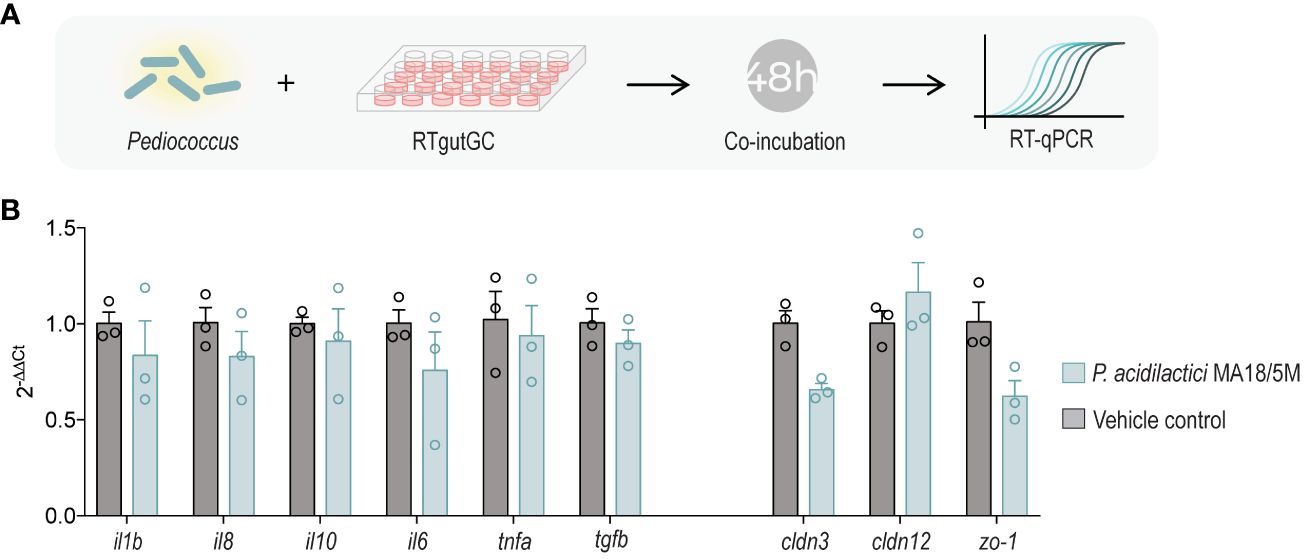
Figure 1 Coincubation of P. acidilactici MA18/5M with RTgutGC to evaluate expression of immune and barrier genes (A). Relative gene expression of interleukin 1 beta (il1b), interleukin 8 (il8), interleukin 10 (il10), interleukin 6 (il6), tumor necrosis factor alpha (tnfa), transforming growth factor beta (tgfb), claudin 3 (cldn3), claudin 12 (cldn12), and zonula occludens 1 (zo-1) in RTgutGC unstimulated (control) or inoculated with P. acidilactici MA18/5M for 48 h (B). All data are expressed as mean values ± S.E.M (n=3). Differences were not statistically significant (Kruskal-Wallis non-parametric test).
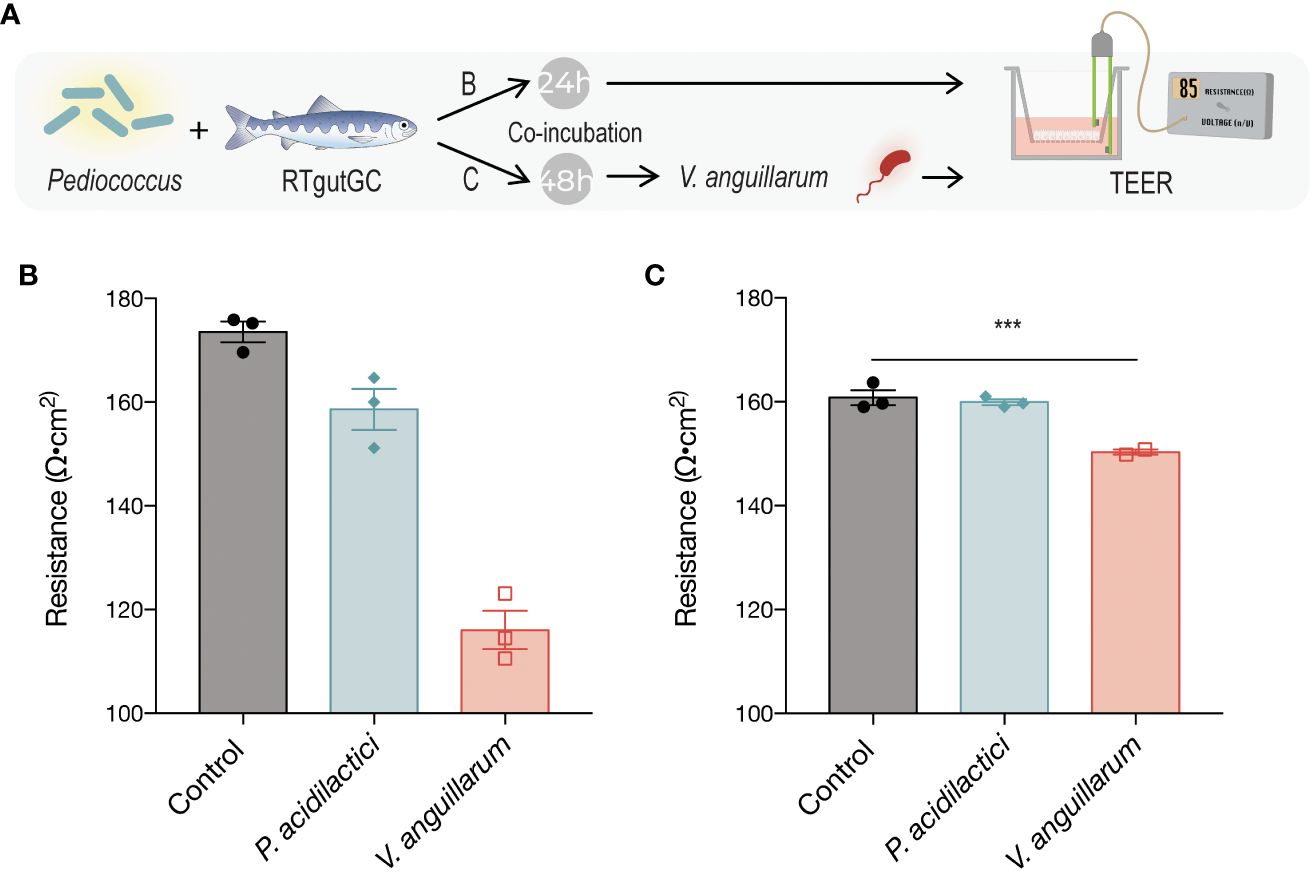
Figure 2 Transepithelial electrical resistance (TEER) in response to exposure to P. acidilactici MA18/5M or V. anguillarum J382 (A). RTgutGC cells were seeded on Transwell semipermeable transmembrane supports and P. acidilactici MA18/5M was added to the apical compartment for either 24 h (B) or 48 h (C). The control group was not exposed to bacteria at any time, and the V. anguillarum J382 group was incubated only with the bacterium for the latter 24 h of the experiment (B, C). TEER was determined based on the resistance given by the monolayer per area, normalized to the blank measurement. Bars indicate statistically significantly differences among treatments (*P < 0.05, ** P < 0.01, *** P < 0.001).
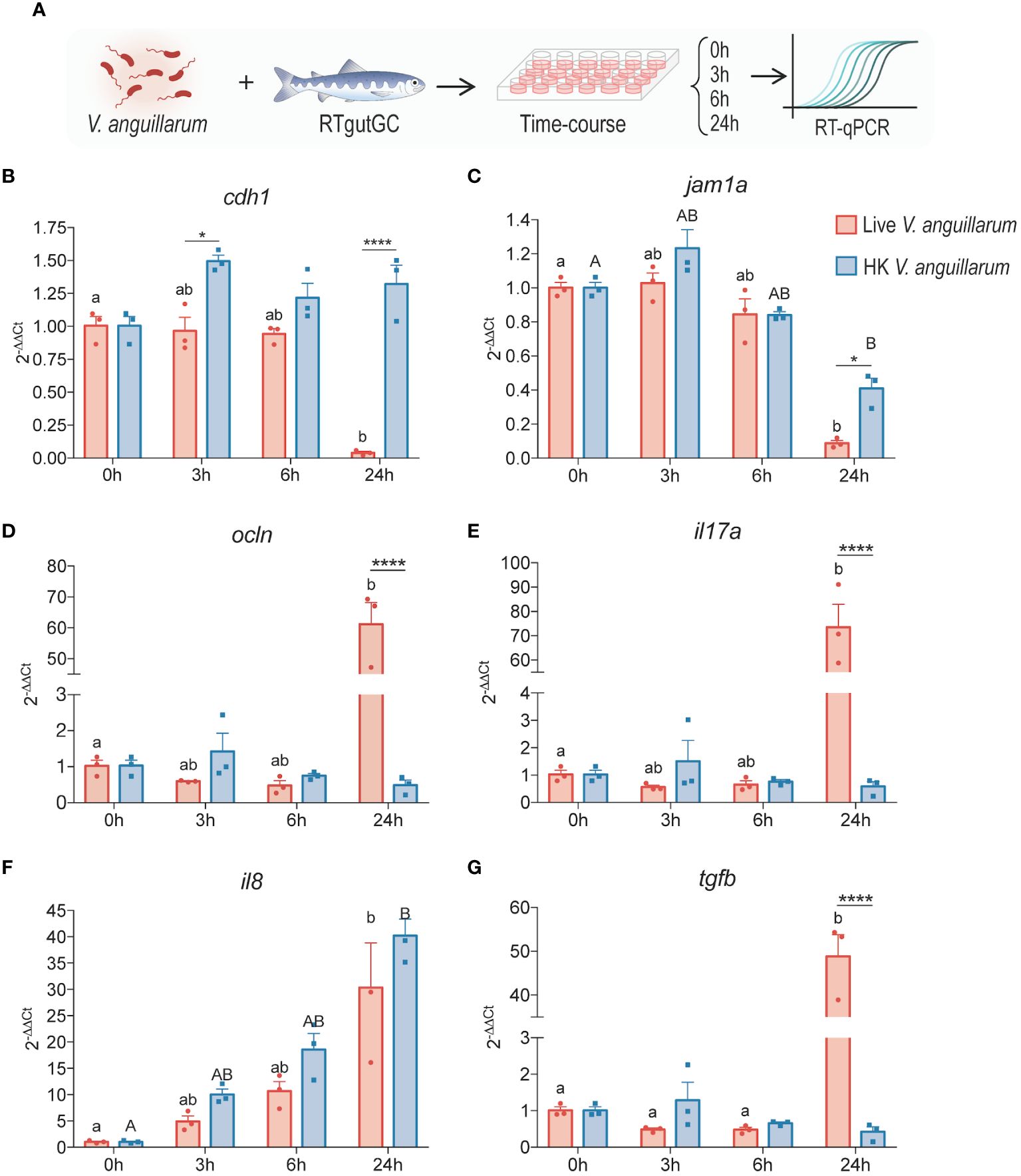
Figure 3 Time-course analysis of salmonid intestinal cells to live or heat-killed V. anguillarum. RTgutGC cells were exposed to either live or HK bacteria (2:1 MOB at the time of inoculation) (A) and gene expression of (B) e-cadherin (cdh1), (C) junctional adhesion molecule 1 alpha (jam1a), (D) occludin (ocln), (E) interleukin 17 (il17a), (F) interleukin 8 (il8), and (G) transforming growth factor β (tgfb) were measured using RT-qPCR. All data are expressed as mean values ± S.E.M (n=3). Bars represent significant differences between treatments at the same time-points. Different letters (Live bacteria: lower cases; HK bacteria: Upper cases) represent significant differences in each treatments at different times (*P < 0.05, ** P < 0.01, *** P < 0.001).
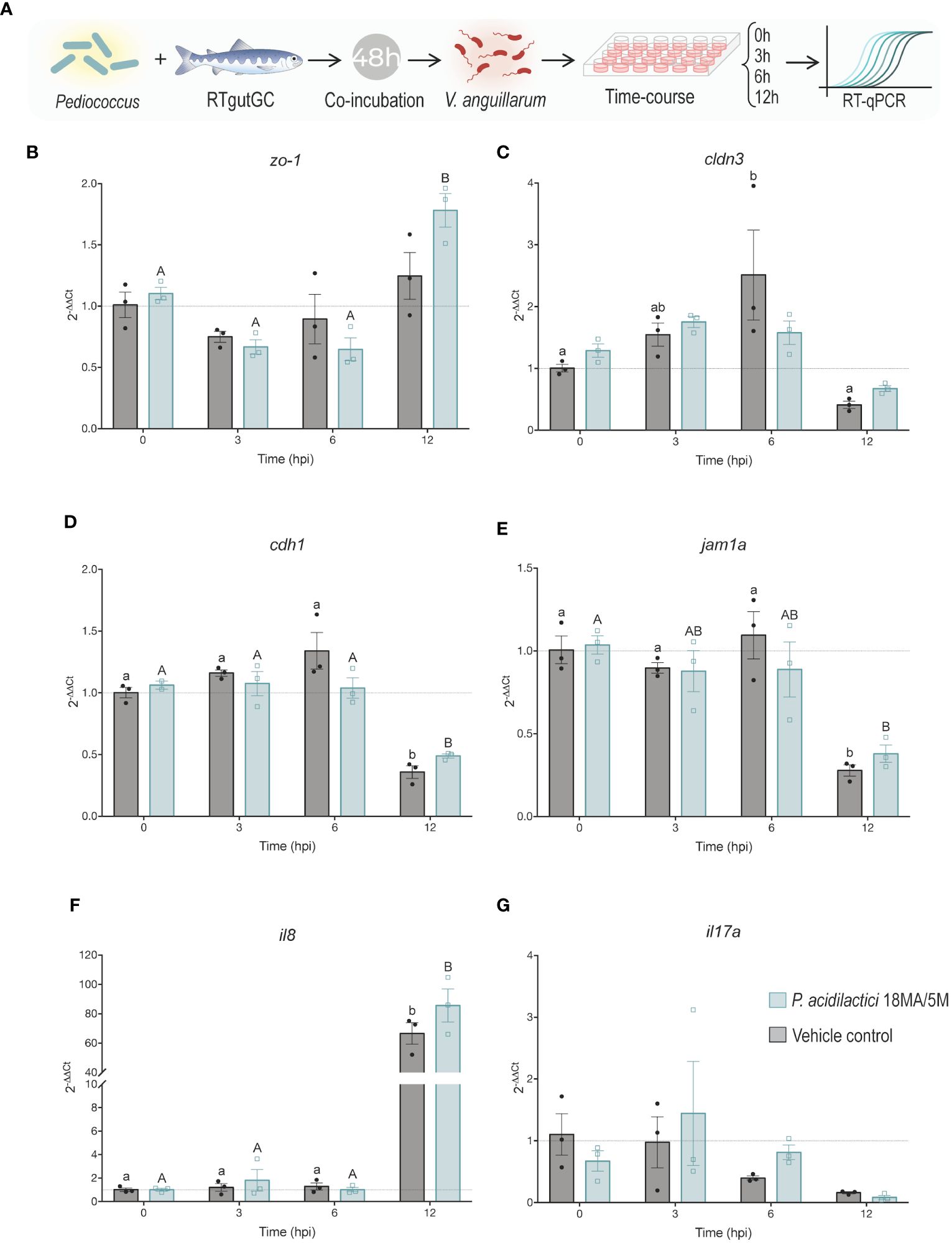
Figure 4 Effect of pre-treatment with P. acidilactici MA18/5M followed by V. anguillarum inoculation on the expression of key tight junction and immune molecules. (A) RTgutGC intestinal epithelial cells were incubated with P. acidilactici MA18/5M for 48 h (MOB 1:100; ~7.5 x 108 CFU/mL), then infected with V. anguillarum (2:1 MOB at the time of inoculation) and samples were collected at 0, 3, 6, and 12 h post-infection. The control group was not pretreated with P. acidilactici MA18/5M at any time and was only exposed to V. anguillarum for the latter 12 h of the experiment. Gene expression of (B) zonula occludens-1 (zo-1), (C) claudin 3 (cldn3), (D) e-cadherin (cdh1), (E) junctional adhesion molecule 1 alpha (jam1a), (F) interleukin 8 (il8), and (G) interleukin 17 (il17a) were assessed using RT-qPCR. All data are expressed as mean values ± S.E.M (n=3). Different letters (control: lower cases; P. acidilactici MA18/5M: Upper cases) represent significant differences in each treatments at different times (P < 0.05).
2.1.4 RTgutGC RNA extraction and cDNA synthesis
Total RNA was isolated from RTgutGC cell lysates using 0.3 volumes of chloroform per 1 volume of TRIzol (Invitrogen, 2020). After this, 0.7 volumes of 100% isopropanol per 1 volume of the sample were added, vortexed briefly, and incubated at room temperature for 5 min. Samples were then centrifuged at 16,000 rpm for 15 min at 4°C. The supernatant was discarded, and residual isopropanol was removed. Then, 1 mL of 70% ethanol in nuclease-free water (Invitrogen) was added and samples were centrifuged at 16,000 rpm for 15 min at 4°C. The residual ethanol was carefully removed, and pellets were air-dried for 15 to 20 min. The RNA was then resuspended in 30 μL of warm (56°C) nuclease-free water and DNase treated with Ambion DNase I (RNase free) (Ambion™ DNase I, Invitrogen) following the manufacturer’s instructions to degrade any residual genomic DNA. Then, DNase treated RNA samples were quantified using a NanoDrop spectrophotometer. Purified RNA samples had A260/280 ratios between 1.9 and 2.1 and A260/230 ratios between 1.9 and 2.2.
First-strand cDNA templates for qPCR were synthesized from 1 μg of the freshly isolated RNA using a High-Capacity cDNA Reverse Transcription Kit, following the manufacturer’s instructions (Applied Biosystems). Each sample was incubated at 25°C for 10 min, at 37°C for 120 min, and at 85°C for 2 min. Samples with a total volume of 40 μL per reaction were stored at -80°C until utilization.
2.1.5 qPCR analysis of RTgutGC samples
All qPCR reactions were performed in a 10 μL reaction, 4.58 μL of 1:10 diluted cDNA, 0.42 μL of primers (forward and reverse primer mix; 14.4 μM), and 5 μL of Power SYBR Green 2x Master Mix. All samples were amplified and detected using the QuantStudio5 Real-Time PCR System (Thermo Fisher Scientific) and analyzed using the associated cloud-based Design and Analysis software (Thermo Fisher Scientific; version 2.5.1). The reaction conditions were 50°C for 2 min, then 95°C for 10 min, followed by 40 cycles of 95°C for 15 s, then 60°C for 1 min. The melt curve was assessed every 15 s between 60-95°C.
The primer sequences of interleukin 1 beta (il1b), interleukin 6 (il6), interleukin 8 (il8), interleukin 10 (il10), interleukin 17a (il17a), tumor necrosis factor alpha (tnfa), transforming growth factor β (tgfb), E-cadherin (cdh1), claudin 3 (cldn3), claudin 12 (cldn12), occludin (ocln), junctional adhesion molecule 1 alpha (jam1a), zonula occludens-1 (zo-1), used in this study are listed in Table 1. Gene nomenclature abbreviations were obtained from the Zebrafish Information Network database (www.zfin.org). Since the reagents, cycling conditions and samples were different from previous studies, primer efficiencies were measured. Briefly, a 7-point 1:3 dilution series starting with cDNA representing 5 ng/µL (12.5 ng per reaction) of input total RNA was generated, and efficiencies then calculated using the formula E = 10(−1/slope).
Transcripts levels of the genes of interest (il1b, il6, il8, il10, il17a, tnfa, tgfb, cdh-1, cldn3, cldn12, ocln, jam1a, and zo-1) were normalized to transcript levels of the endogenous control gene cytochrome c oxidase subunit 6C-1 (cx6c1). Levels of five candidate normalizers [cx6c1, elongation factor 1 alpha (ef1a), beta actin (actb), glyceraldehyde-3-phosphate dehydrogenase (gapdh), and internal transcribed spacer 2 locus (its2)] were assessed. After normalizer testing was completed, transcript levels of the genes of interest were analyzed in the individual study samples, with normalization to cx6c1. On each gene a no RT control was included. Gene expression was determined using the comparative 2−ΔΔCt method (50).
2.1.6 Transepithelial electrical resistance
To determine the change in epithelial electrical resistance given by the effect of different treatments on the cell monolayer, a TEER experiment was carried out (Figure 2A). Prior to seeding cells onto the Corning Transwell polyester membrane cell culture inserts (6.5 mm and 0.4 μm pore size), baseline resistance was determined to be 107/0.33 cm2 using a STX2 chopstick electrode connected to a voltmeter. RTgutGC cells (passage number 20-30) were grown in T75 flasks, trypsinized when maximally (>90%) confluent, and cell counts were performed using the trypan blue (0.04%; Thermo Fisher Scientific) exclusion assay and the automated Countess cell counter (Invitrogen) prior to seeding into the cell culture dishes. The cells were seeded on semipermeable Transwell membrane supports (Corning Costar Transwell, Millipore Sigma) at a density of approximately 2.6 x 105 cells/cm2 or a final number of about 8.6 x 104 cells per insert (cell growth aera of 0.33 cm2). The cells were cultured for at least 3 weeks prior to the experiment to ensure that they established the brush border membrane and tight junction complexes. To the apical and basolateral compartments 100 μL or 500 μL, respectively, of L-15 media supplemented with 10% FBS were added, and the medium was replaced every 4-5 days. Periodic inspection of the cell monolayers was carried out using a Nikon inverted light microscope.
Bacterial cultures were prepared as outlined in section 2.1.2. and bacteria containing cell culture growth medium was added to the RTgutGC cells and incubated for 24 h. At the end of the incubation time, culture medium was carefully removed so not to disturb the cell monolayer and the Transwell inserts were transferred to a new 24-well plate containing sterile PBS on the basolateral compartment. To the apical compartment, 100 μL of PBS were added. Cell monolayers were likewise washed two more times, 100 mL of PBS was added to the apical compartment and 500 μL to the base of the electrode, and measurements were recorded using a cup electrode collected to a voltmeter. The baseline reading (membrane only) was subtracted from the measurements and the resistance per cm2 was determined. Statistical analyses were performed on the resistance values per area.
2.2 In vivo P. acidilactici MA18/5M supplementation trial
2.2.1 Experimental design
Chinook salmon (O. tshawytscha) juveniles (n = 280; 8.168 ± 0.721 g) were obtained from Yellow Island Aquaculture Ltd. (www.yellowislandaquaculture.ca, Quadra Island, British Columbia, Canada) and the experiments were conducted there. Before the infection trial, fish were kept in freshwater (FW, 14 ± 2°C) at a density of 2.3 kg/m3 using a flow-through system under natural photoperiod (12:12 h dark:light). After this, fish were randomly distributed between eight 120 L barrels (2 barrels per treatment, 35 fish per barrel) one-day prior the starting of feeding trial. During the transfer, the initial fish weight (g) was recorded (Figure 5A). To minimize growth differences related to the initial fish size and not to the diet supplementation, only fish around 8 ± 1 g were selected (Figure 5A). The experimental diets (treatments) utilized in this experiment were: i) Control diet, ii) P. acidilactici (MA18/5M probiotic strain), iii) Control diet intraperitoneally injected with heat-killed V. anguillarum, and iv) P. acidilactici intraperitoneally injected with heat-killed V. anguillarum (Please referrer to Supplementary Material 1 for formulation details). During the trial, fish were fed commercial dry pellet (EWOS Harmony 2 mm: 47% protein, 18% fat, 0.7% fiber, 2.9% calcium, 1.2% phosphorus, and 0.6% sodium) twice a day with a ration of 2% body weight.
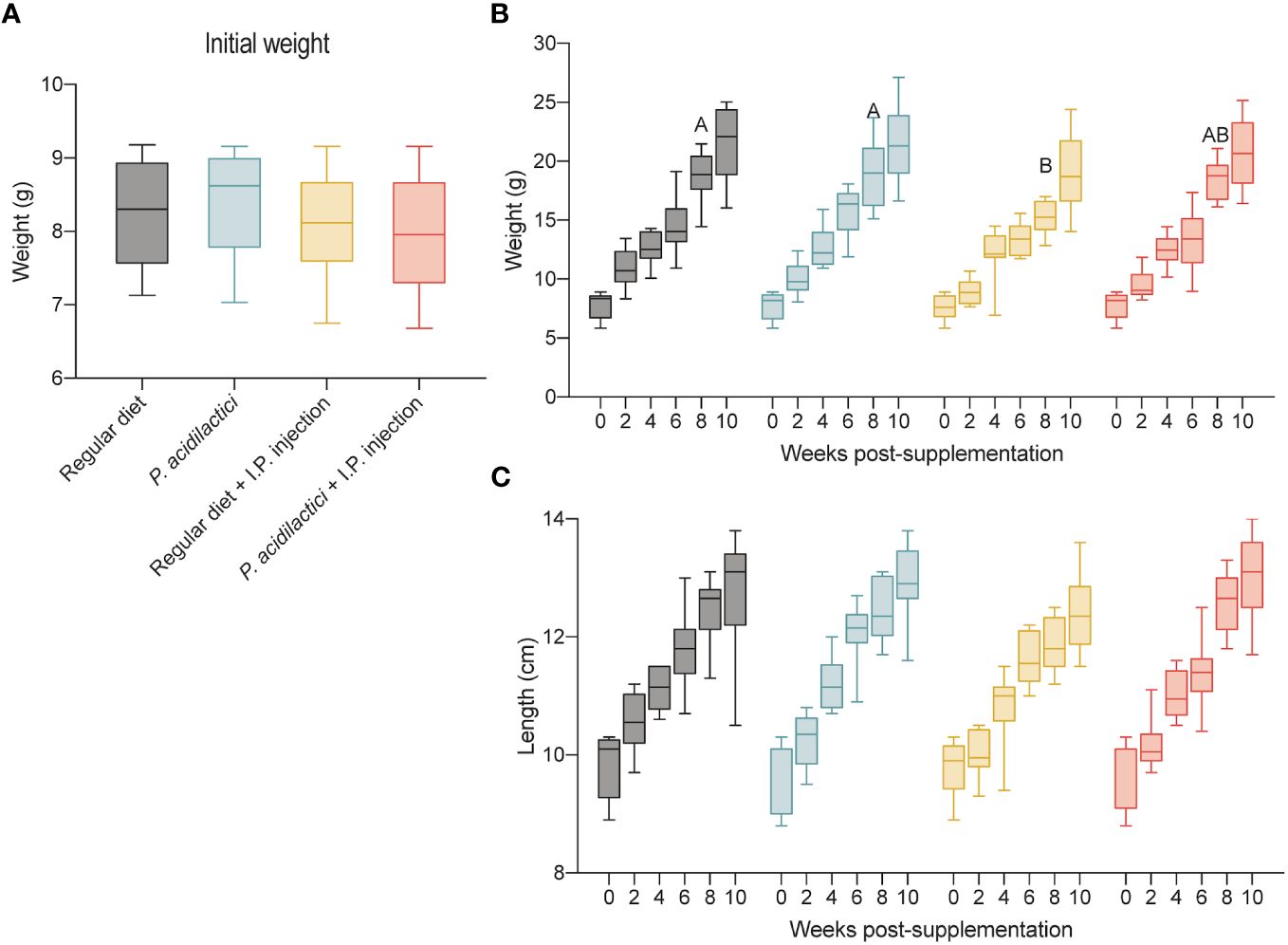
Figure 5 In vivo trial in juvenile Chinook salmon with diet supplementation of P. acidilactici MA18/5M followed by intraperitoneal challenge with V. anguillarum J382. Initial weight (g) of fish transferred to test the different supplementation groups (A). Each column represents an average of 35 fish. Weight (g) increase recorded for a total of 10 weeks post-supplementation (B). Length (cm) increase recorded for a total of 10 weeks post-supplementation (C). All data are expressed as mean values ± S.E.M. Different letters represent significant differences among treatments at the same time (P < 0.05).
2.2.2 Chinook salmon probiotic and heat-killed V. anguillarum stimulation trial
To determine the effect of P. acidilactici MA18/5M on Chinook salmon juveniles, both Control diet groups (Control diet and Control diet intraperitoneally injected with heat-killed V. anguillarum) and both P. acidilactici groups (P. acidilactici (MA18/5M probiotic strain) and P. acidilactici intraperitoneally injected with heat-killed V. anguillarum) were fed with dry pellet or dry pellet supplemented with the probiotic strain respectively for four weeks (Figure 6A). After this, to evaluate the effect of probiotic supplementation after inactivated-bacteria stimulation, one control group and one P. acidilactici group was injected with heat-killed V. anguillarum grown as mentioned in section 2.1.2. The respective diets remained during the total duration of the study.
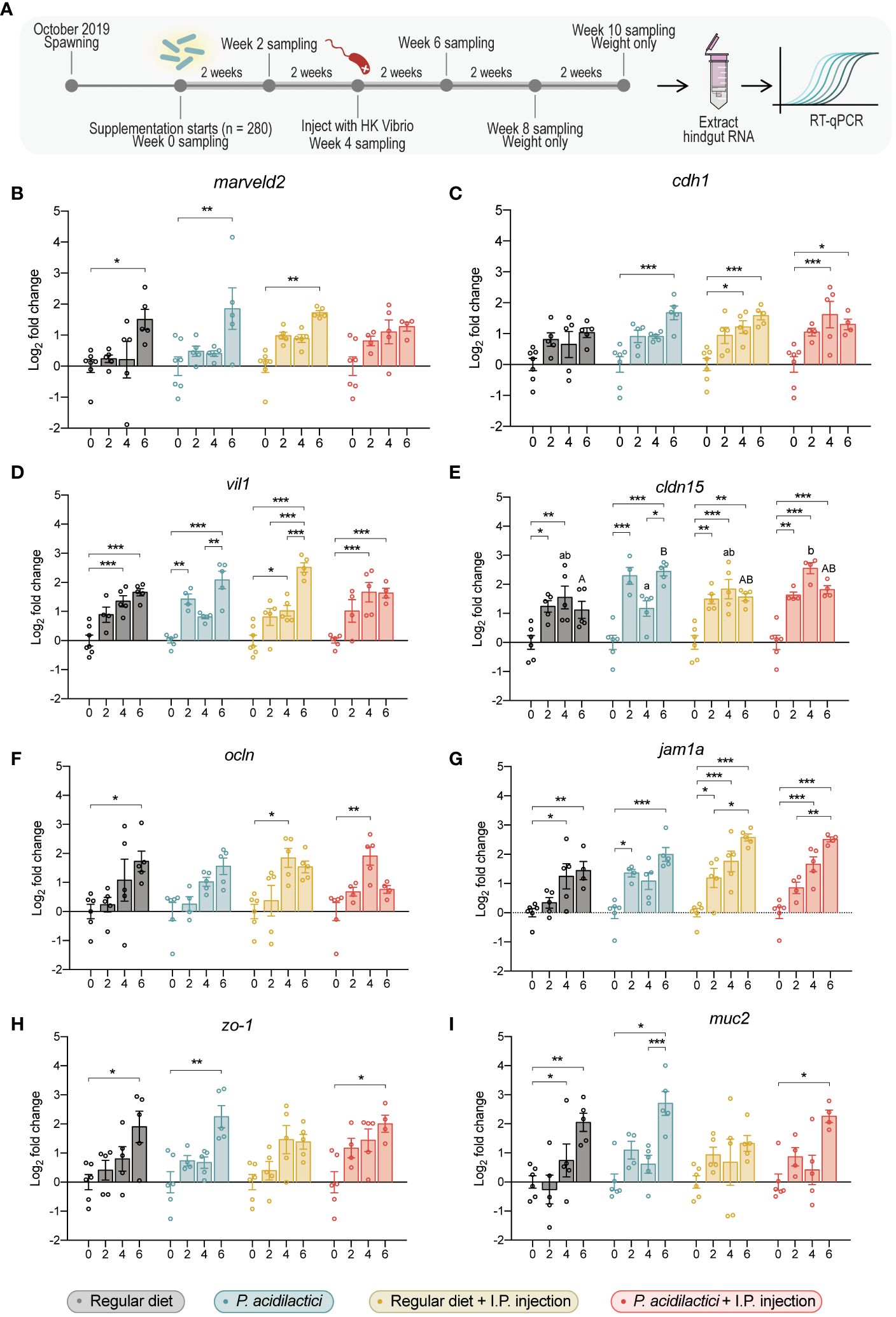
Figure 6 Gene expression analysis of relevant gut barrier genes in the hindgut of juvenile Chinook salmon fed with regular diet or supplemented with P. acidilactici MA18/5M and challenged with V. anguillarum J382 (A). Transcripts for (B) tricellulin (marveld2), (C) e-cadherin (cdh1), (D) villin 1 (vil1), (E) claudin 15 (cldn15), (F) occludin (ocln), (G) junctional adhesion molecule 1 alpha (jam1a), (H) zonula occludens-1 (zo-1), and (I) mucin 2 (muc2) were assessed. All data are expressed as mean values ± S.E.M (n = 6). Bars represent significant differences between time-points at the same treatment. Different letters represent significant differences between treatments at the same time-point (*P < 0.05, ** P < 0.01, *** P < 0.001).
The timing of sampling for this experiment was 0-, 2-, 4-, and 6-weeks post-diet supplementation (Figure 6A). For sampling, fish were exposed to a non-lethal dose of clove oil, and one half of the hindgut tissue was isolated immediately, placed in RNAlater, and stored at -20°C for RNA extraction and qPCR analysis. The other half of the hindgut was placed in 10% neutral buffered formalin (Azer Scientific, Fisher Scientific) at 4°C for further histopathology analysis. Additionally, weight (g) and length (cm) were also recorded at 8-, and 10-weeks post-diet supplementation (Figure 6A).
2.2.3 Hindgut RNA extraction and cDNA synthesis
Total RNA was isolated from Chinook salmon hindgut samples using 1 mL of TRIZOL reagent following the manufacturers protocol (Invitrogen, 2020). After extraction, the RNA was treated with Ambion DNase I (RNase free) (Ambion™ DNase I, Invitrogen) following the manufacturer’s instructions to degrade any residual genomic DNA. Briefly, 5 μg of RNA was treated with 2 μL of Ambion DNase I, 4 μL of DNase buffer 10x, and DEPC water complete to 40 μL. Then, samples were incubated at 37°C for 30 min, washed twice with Wash solution A, centrifuged at 3,500 x g for 1 min and purified in an RNA/Protein Purification Column. The supernatant containing the RNA was carefully transferred to a new tube. DNase treated RNA samples were quantified and evaluated for purity (A260/280 and A260/230 ratios) using a Take3 plate of a Synergy H1 Hybrid plate Reader (Biotek Instruments, Inc., USA). Column purified RNA samples had A260/280 ratios between 1.9 and 2.1 and A260/230 ratios between 1.9 and 2.2.
First-strand cDNA templates for qPCR were synthesized from 250 ng of DNaseI-treated, column-purified total RNA using qScript cDNA Supermix (Quanta Biosciences) following the manufacturer’s instructions. Each sample was incubated at 25°C for 5 min, at 42°C for 30 min, and at 85°C for 5 min. Samples in a concentration of 25 ng/µL were stored at -20°C until utilization.
2.2.4 qPCR analysis of Chinook salmon hindgut
All qPCR reactions were performed in a 10 μL reaction, 5 µL of 2x WISENT ADVANCED™ qPCR master mix (Wisent, Quebec, Canada), 2.5 µL of forward and reverse primer mix (Sigma Aldrich, USA) at a final concentration of 0.5 µM each, and 2.5 µL of cDNA (2.5 ng/µL, 6.25 ng per reaction). All samples were amplified and detected using the LightCycler® 480 II (Roche, USA). The reaction mixtures were pre-incubated for 2 min at 95°C, followed by 40 cycles of denaturation for 5 s at 95°C, annealing for 30 s at 60°C, and finally extension for 8 s at 72°C. The melt curve was completed for each run every 5 s from 65°C to 97°C.
The primer sequences of interleukin 1 beta (il1b), interleukin 6 (il6), interleukin 8 (il8), interleukin 10 (il10), interleukin 17a (il17a), tumor necrosis factor alpha (tnfa), myeloid differentiation factor 88 (myd88), transforming growth factor β (tgfb), tricellulin (marveld2), E-cadherin (cdh1), villin 1 (vil1), claudin 3 (cldn3), claudin 12 (cldn12), claudin 15 (cldn15), occludin (ocln), junctional adhesion molecule 1 alpha (jam1a), zonula occludens-1 (zo-1), and mucin 2 (muc2) used in this study are listed in Table 1. Gene discovery, qPCR primer design, initial quality testing, and primer efficiencies were assessed following the methods mentioned in section 2.1.5.
Transcripts levels of the genes of interest (il1b, il6, il8, il10, il17a, tnfa, myd88, tgfb, marveld2, cdh1, vil1, cldn3, cldn12, cldn15, ocln, jam1a, zo-1, and muc2) were normalized to transcript levels of two endogenous control genes. Levels of five candidate normalizers [elongation factor 1 alpha (ef1a), beta actin (actb), glyceraldehyde-3-phosphate dehydrogenase (gapdh), 18S ribosomal RNA (18S), and internal transcribed spacer 2 locus (its2)] were assessed in 50% of the samples (i.e., in 3 random samples per treatment) using cDNA representing 6.25 ng of input total RNA. Reference gene stability was assessed using geNorm, NormFinder, BestKeeper, and Delta Ct comparison, through the bioinformatic open-access portal RefFinder (51–55). Most stable genes assessed were ef1a and actb.
After normalizer testing was completed, transcript levels of the genes of interest were analyzed in the individual study samples, with normalization to both ef1a and actb. In all cases, levels were assessed (in triplicate) in 7 individual for day 0 and five individuals per treatment per time-point for 2-, 4-, and 6-weeks post-diet time-points using cDNA representing 6.25 ng of input total RNA. On each gene a no RT control was included. Gene expression was determined using the comparative 2−ΔΔCt method (50).
2.2.5 Semi-quantitative and qualitative histological analysis
For Chinook salmon samples collected at 0-, 2-, 4-, and 6-weeks post-diet supplementation, five individuals per treatment were processed further for histology. Each 10% formalin-fixed hindgut sample was cut into 5 equal-sized pieces, dehydrated in an alcohol gradient, cleared in two changes of xylene and sequentially embedded into a single paraffin block. For each fish, 5 μm-thick cross-sections of all five pieces of hindgut were mounted onto a glass slide, stained with hematoxylin and eosin and sealed under a coverslip. All the samples were processed at the Animal Health Laboratory, the University of Guelph (Guelph, ON, Canada).
All analyses were performed using bright field light microscopy on a Leica DMR light microscope (Leica Microsystems Inc., Concord, ON, Canada) equipped with Openlab imaging software (Openlab 5.5, PerkinElmer, Waltham, MA, USA). To visualize the presence of edema, and inflammation of the serosa, submucosa, and lamina propria, samples were observed using a 4x objective (40x magnification). To assess their suitability, the criteria and scales utilized were the following: 0 = none, 1 = mild, 2 = moderate, 3= severe or more extensive than 2. To determine the epithelial vacuolization (vacuolization) and numbers of goblet cells, a 10x objective was used (100x magnification). For epithelial vacuolization, the scores utilized were: 0 = none, 1 = mild vacuolization, 2 = moderate, 3= more extensive than 2 and sometimes involved folding of the epithelium and distortion of the cells and/or folds. For goblet cells, the criteria utilized were: 0 = none visible, 1 = small numbers present as a minority of cells in the epithelium, 2 = greater numbers often grouped together, 3= large numbers or more extensive than 2. Finally, to evaluate the mitotic figures (mitoses), epithelial cell death (apoptosis/necrosis), and multifocal inflammation, a 20x objective was employed (200x magnification). To assess mitoses and apoptosis/necrosis, the numbers were counted for the five intestinal folds.
2.3 Statistical analyses
For all RTgutGC in vitro trials, data are expressed as mean values ± SEM (n=3). Nonparametric data were statistically compared with a one-way ANOVA (Kruskal-Wallis) and Dunn’s multiple comparisons test. Experiments with two factors were compared with a two-way ANOVA and Tukey’s multiple comparisons test. All statistical analyses were done using GraphPad Prism software v9.0 (GraphPad Software, La Jolla California USA).
For Chinook salmon in vivo trials, data are shown as the mean ± SEM (n=5). Assumptions of variance, normality, and homogeneity were tested. A two‐way ANOVA was performed using the different dietary treatments and time-points as factors of variance, followed by a Tukey’s multiple comparisons test to identify differences between groups. All statistical analyses were performed using STATISTICA v7.0 (StatSoft software, Tulsa, USA) and graphs performed using GraphPad Prism v9.0 (GraphPad Software, La Jolla California USA).
Histopathological data were analyzed using a two-way ANOVA using GraphPad Prism v9.0 (GraphPad Software, La Jolla California USA). Different dietary treatments and time-points as factors of variance were considered. A Bonferroni correction was applied, and statistical significance was declared at P ≤ .05 for all dependent variables.
3 Results
3.1 Effect of coincubation with P. acidilactici MA18/5M on the expression of tight junction and immune molecules in RTgutGC cells
To examine whether P. acidilactici could promote gut barrier integrity and modulate immune-related genes in the established RTgutGC in vitro model, a 48 h endpoint coincubation experiment was performed using differentiated RTgutGC cells (Figure 1A) (56). However, P. acidilactici did not cause a significant change in the expression of the tight junction genes assessed (cldn3, cldn12, and zo-1) nor did it induce changes in expression of key proinflammatory cytokines (Figure 1B).
3.2 Changes in transepithelial electrical resistance in response to P. acidilactici MA18/5M and V. anguillarum coincubation
Differentiated RTgutGC cells cultured on semipermeable Transwell polyester membrane supports (pore size 0.4µm) were exposed to suspensions of the LAB (MOB 1:100; ~4 x 106 CFU/mL) or V. anguillarum (MOB 2:1; ~2 x 104 CFU/mL) in L-15 cell culture media for 24h (Figure 2A). The V. anguillarum, but not P. acidilactici, caused an appreciable decrease in resistance relative to the vehicle control (Figure 2B).
To determine whether pre-treatment with the candidate probiotic could protect the cell monolayer against the pathogen-induced damages to the intercellular tight junctions, the same strain was grown and added to the apical compartment of the membrane inserts in like manner for 48h. Then, V. anguillarum (MOB 2:1; ~2 x 104 CFU/mL) was added for 24 h and TEER measurements were taken at the end of the incubation period (Figure 2C). There were no statistically significant differences in resistance in either of the LAB-pretreated group, despite the addition of the pathogen. However, a statistically significant decrease in resistance (P = 0.0265) was observed in the group incubated with V. anguillarum only (Figure 2C).
3.3 Effect of exposure to V. anguillarum on the expression of tight junction and immune molecules
To characterize the response of RTgutGC cells to live or heat-killed (HK) V. anguillarum, a time-course coincubation experiment was carried out (Figure 3A). Samples were collected at 0, 3, 6, and 24 h (Figure 3A). Of the TJ-related molecules assessed, the live pathogen elicited a significant downregulation of cdh1 and jam1a, but not ocln, which had a puzzling upregulation by the 24 h timepoint (Figures 3B–D). Of the cytokines assessed, all exhibited a time-dependent upregulation, which was statistically significant at the 24 h timepoint relative to the 0 h control group (Figures 3E–G). In the case of il8, but not il17a or tgfb, the upregulation was observed in cells exposed to both live and HK bacteria (Figures 3E–G). In an independent replicate of this experiment, a similar time-dependent response was observed, in which there was a significant upregulation of il1b, il8, and tnfa at the 24 h timepoint for groups exposed to live bacteria (data not shown).
3.4 Effect of pre-treatment with LAB and exposure to V. anguillarum on the expression of immune and tight junction molecules
To examine the potential use of the LAB as a disease prevention strategy by stimulating immunity and gut barrier function, a time-course coincubation experiment was performed. Briefly, differentiated RTgutGC cells were pre-treated with P. acidilactici for 48 h and then exposed to V. anguillarum. Samples were taken at 0 h, 3 h, 6 h, and 12 h after infection with the pathogen and the relative expression of key TJ and immune molecules was assessed through RT-qPCR (Figure 4A).
The expression of zo-1 was significantly (p < 0.05) upregulated in the P. acidilactici at the 12 h timepoint relative to the expression level at 0, 3, and 6 h (Figure 4B). In contrast, a significant upregulation of cldn3 was observed 6 h post-exposure with V. anguillarum compared to 0 and 12 h timepoints (Figure 4C). There was a statistically significant (p < 0.05) difference in the expression of cdh1 for both treatment groups after 12 hpi (hours post-infection) compared to the other timepoints (Figure 4D). Lastly, there was also a significant (p < 0.05) downregulation of the jam1a molecule 12 hpi in the control and P. acidilactici MA18/5M groups compared to 0, 3, and 6 hpi, and 0 hpi respectively (Figure 4E). The expression of the pro-inflammatory cytokines il8 and il17a was also assessed. There was an extremely significant (p < 0.05) upregulation of il8 at the 12 h timepoint in both groups relative to the baseline control and the 3 and 6 hpi timepoints (Figure 4F). In contrast, no differences were observed in the expression of il17a between treatments and timepoints (Figure 4G).
3.5 Growth analysis
To minimize growth differences related to the initial fish size and not to the diet supplementation, fish weight (g) was recorded during the transfer to each of the treatments (Figure 5A). Overall, no statistically significant differences were observed in weight between the treatments tested in this study (Figure 5A).
Results collected during the trial showed significant differences in weight (g) of fish belonging to the regular diet and P. acidilactici treatments compared to the regular diet + I.P. injected treatment 8 wps (weeks post-supplementation) (Figure 5B). In contrast, no significant differences in weight (g) among treatments were observed at 0-, 2-, 4-, 6-, and 10-wps (Figure 5B). Length (cm) data collected during the supplementation trial did not show statistically significant differences between treatments (Figure 5C).
3.6 Chinook salmon gut-specific relative expression
Transcript levels of gut-specific genes were evaluated by qPCR in hindgut samples (Figure 6A). A significant increase in the transcript expression of marveld2 6 wps was found in fish from the regular diet, P. acidilactici, and regular diet + I.P. injection treatments compared to their respective time 0 (Figure 6B). An upregulation on the relative expression of cdh1 gene was observed at 4 and 6 wps in the regular diet + I.P. injection and P. acidilactici + I.P. injection treatments compared to their respective control timepoint (Figure 6C), meanwhile, an upregulation of cdh1 was noted in P. acidilactici treated fish at 6 wps compared to time 0 (Figure 6C).
The relative expression of the vil1 encoding gene showed different upregulation patterns among treatments (Figure 6D). After 4- and 6- wps, an increase of vil1 in the regular diet treatment was observed compared to 0 wps (Figure 6D). In P. acidilactici treatment, vil1 encoding transcripts in 2 and 6-wps fish showed an upregulation compared to 0 weeks supplemented fish (Figure 6D). Moreover, fish supplemented with P. acidilactici for 6 weeks showed an upregulation in the vil1 gene compared to 4 wps (Figure 6D). At 4 wps, regular diet + I.P. injection fish showed an increase in vil1 compared to the time 0 of supplementation (Figure 6D). Moreover, 6 wps fish showed an increase in the vil1 expression compared to 0-, 2-, and 4 wps (Figure 6D). Finally, individuals from the P. acidilactici + I.P. injection treatment showed an up-regulation after 4- and 6 wps on the expression of the vil1 gene compared to time 0 (Figure 6D).
An increased relative expression of cldn15 was observed in fish supplemented for 2- and 4 weeks with a regular diet compared to day 0 (Figure 6E). In P. acidilactici treated fish, the cldn15 gene showed an upregulation at 2- and 6-wps compared to 0-weeks supplemented fish (Figure 6E), whereas, 6-weeks supplemented fish also showed a statistically significant upregulation compared to 4-weeks P. acidilactici supplemented fish (Figure 6E). For regular diet + I.P. injection and P. acidilactici + I.P. injection treatments, a similar pattern of upregulation of the cldn15 gene was observed at 2-, 4-, and 6-wps compared to their respective time 0 (Figure 6E). Interestingly, cldn15 was the only gene that showed significant differences between diet treatments at the same time point. For instance, a statistically significant upregulation of cldn15 was observed in P. acidilactici + I.P. injection treatment compared to the P. acidilactici treatment at 4 wps (Figure 6E). Moreover, upregulation of cldn15 was observed after 6 wps with P. acidilactici compared to the regular diet treatment (p < 0.05) (Figure 6E).
Transcripts encoding the expression of ocln showed statistically significant differences in regular diet treatment between 0 and 6 wps (Figure 6F). Also, an upregulation after 4 weeks of supplementation in the relative expression of ocln was observed in the regular diet + I.P. injection and P. acidilactici + I.P. injection treatments compared to 0 wps (Figure 6F). The relative expression of jam1a in regular diet treated fish was upregulated after 4 and 6 wps compared to time 0 (Figure 6G). In P. acidilactici treatment, an upregulation at 2 and 6 wps was found compared to time 0 (Figure 6G). For regular diet + I.P. injection treated fish, significant differences were found in the expression of jam1a after 2 wps compared to 0 wps (Figure 6G). An increased relative expression of jam1a was observed in fish under regular diet + I.P. injection or P. acidilactici + I.P. injection treatments for 4 and 6 weeks compared to their respectively time 0 (Figure 6G). Additionally, an upregulation of this gene after 6 weeks of either regular diet + I.P. injection or P. acidilactici + I.P. injection treatments was found (p < 0.05) compared to fish 2 wps (Figure 6G).
The relative expression of zo-1 encoding gene was upregulated after 6 weeks of either regular diet, P. acidilactici, or P. acidilactici + I.P. injection treatment compared to their correspondingly time 0 (Figure 6H). Similarly, an upregulation in the transcripts encoding muc2 was observed in fish from the regular diet, P. acidilactici, and P. acidilactici + I.P. injection treatments (Figure 6I). In the regular diet treatment, muc2 relative expression was upregulated after 4 and 6 wps compared to time 0 (Figure 6I). For P. acidilactici-treated fish, this gene showed a statistically significant increase at 6 wps compared to 0 and 4 wps (Figure 6I). Finally, a significant increase in the expression of muc2 was determined in fish from the P. acidilactici + I.P. injection treatment after 6 weeks compared to baseline fish (0 wps; Figure 6I).
3.7 Chinook salmon gut immune relative expression
The effect of the P. acidilactici MA18/5M strain and of the inactivated pathogen stimulation on Chinook salmon immune genes were evaluated by qPCR (Figure 7). In our study, an upregulation of the pro-inflammatory cytokine il1b was only observed in the regular diet + I.P. injection treatment at 2 wps compared to time 0 (Figure 7A). In contrast, a statistically significant increase in the expression of the pro-inflammatory chemokine il8 was observed in each treatment (Figure 7B). For instance, an upregulation in il8 was observed after 4- and 6 wps compared to 0-weeks of regular diet treatment (Figure 7B). Also, a significant increase in the expression of il8 was determined after 2-, 4-, and 6 wps with P. acidilactici compared to day 0 (Figure 7B). In fish sampled from the regular diet + I.P. injection and P. acidilactici + I.P. injection treatments, a similar upregulation was observed after 4 wps compared to their respectively time 0 (Figure 7B). In contrast, the relative expression of il10 and tnfa was not modulated by the treatments utilized in this study (Figures 7C, D).
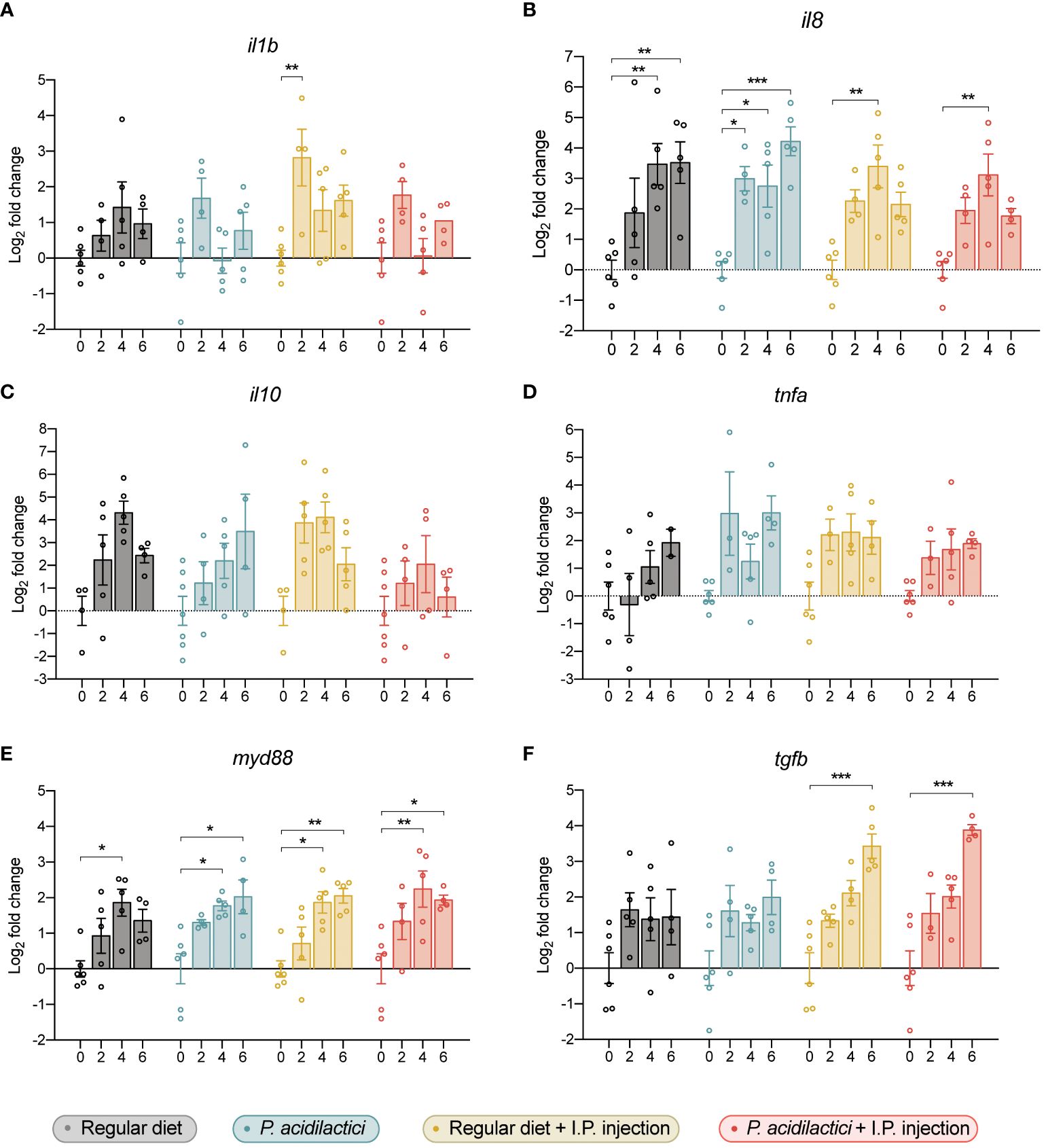
Figure 7 Gene expression analysis of relevant immune genes in the hindgut of juvenile Chinook salmon fed with regular diet or supplemented with P. acidilactici MA18/5M and challenged with V. anguillarum J382. Transcripts for interleukin (A) 1b (il1b), (B) interleukin 8 (il8), (C) interleukin 10 (il10), (D) tumor necrosis factor alpha (tnfa), (E) myeloid differentiation factor 88 (myd88), and (F) transforming growth factor β (tgfb) were assessed. All data are expressed as mean values ± S.E.M (n = 6). Bars represent significant differences between time-points within treatments (*P < 0.05, ** P < 0.01, *** P < 0.001).
Transcript levels of myd88 were upregulated in all conditions after 4 wps compared to time 0 in each treatment (Figure 7E). Moreover, an upregulation in the expression of myd88 compared to time 0 was observed in the P. acidilactici, regular diet + I.P. injection, and P. acidilactici + I.P. injection treatments (Figure 7E). Finally, a statistically significant increase in the expression of tgfb was observed in fish sampled from the regular diet + I.P. injection and P. acidilactici + I.P. injection treatments at 6 wps compared to time 0 (Figure 7F).
3.8 Histopathological effects after P. acidilactici supplementation and heat-killed pathogen stimulation
To examine the effect of P. acidilactici supplementation on Chinook salmon hindgut integrity, a histopathological analysis was conducted (Figure 8). Moreover, to determine whether P. acidilactici could promote hindgut integrity in presence of an immunomodulator, fish from both feeding treatments were I.P. injected with heat-killed V. anguillarum (Figure 8).
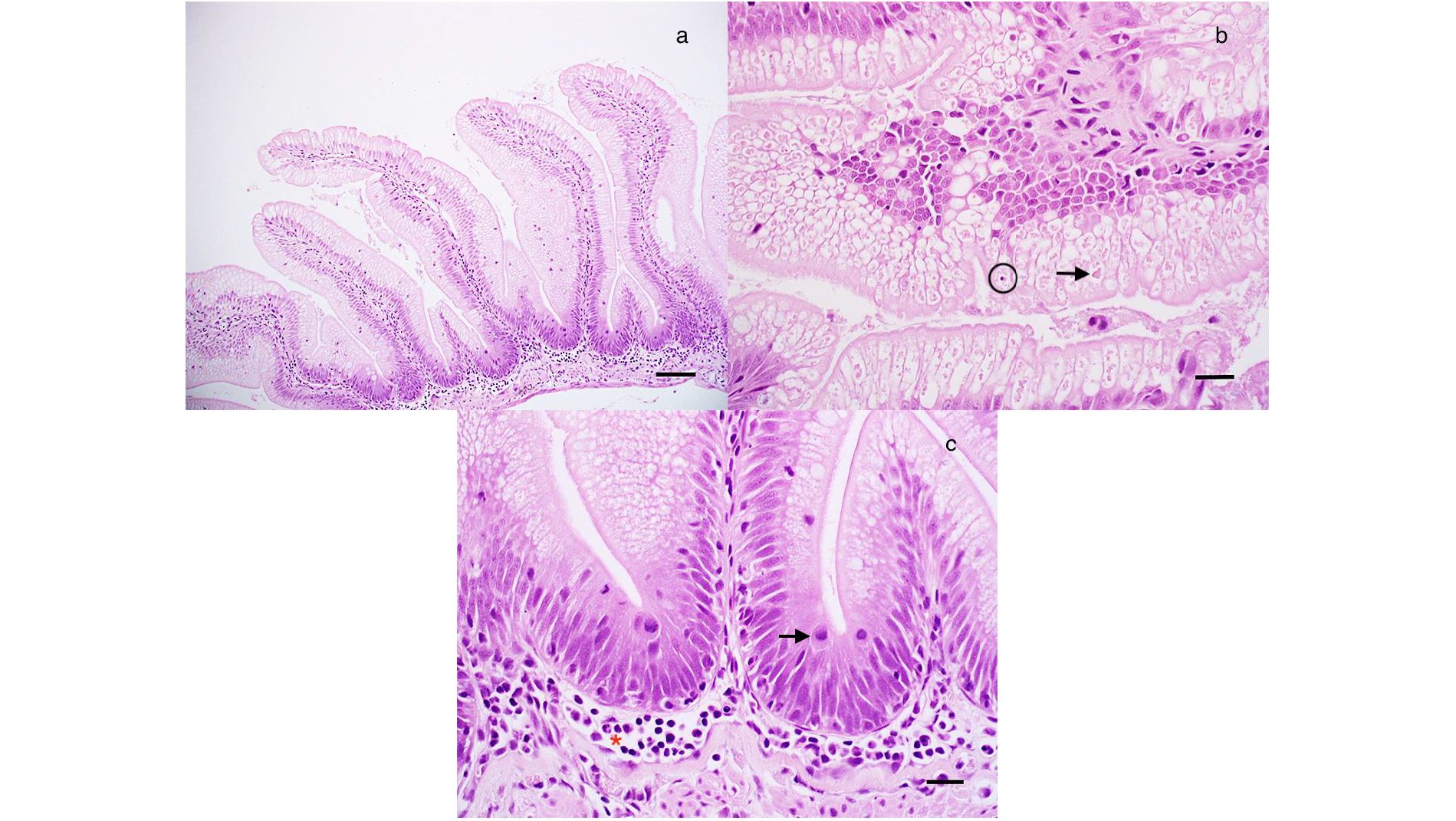
Figure 8 Histological analysis of juvenile Chinook salmon intestine fed with regular diet or supplemented with P. acidilactici MA18/5M and challenged with V. anguillarum J382. (A) Simple intestinal folds with epithelial cells filled with absorptive vacuoles. Bar = 120 mm. (B) Atypical intestinal fold with eosinophilic material (arrow) in absorptive vacuoles along with an example of epithelial apoptosis/necrosis, which was uncommon. Bar = 30 mm. (C) Detail of a, with numerous mitotic figures in the epithelium (arrow) and a mild increase in inflammatory cells in the submucosa (Red asterisk). Bar = 30 mm.
Our results showed that fish feed with the regular diet or P. acidilactici that were or not stimulated with heat-killed V. anguillarum, did not show evidence of edema, inflammation of the serosa, submucosa and lamina propria, and multifocal inflammation after 0, 4, and 6 weeks (Table 2, Figure 8). In the case of epithelial vacuolization, although scores associated with moderate (up to half of the cell is filled with absorptive vacuoles) and extensive (involved folding of the epithelium and distortion of the cells and/or folds) vacuolization were reported, no significant differences were observed among treatments and time points (Table 2, Figure 8). Small numbers of goblet cells were noted in the epithelium and no statistically significant differences were associated to the treatments and time points variables, as well as the interaction of both (Table 2, Figure 8). In this analysis, the numbers of mitotic figures counted in the five intestinal folds did not show differences among the regular diet, P. acidilactici, regular diet + I.P. injection, and P. acidilactici + I.P. injection after 0, 4, and 6 weeks of study (Table 2, Figure 8). There was evidence of epithelial cell death (apoptosis/necrosis) in Chinook salmon hindgut, however, this was not associated with probiotic supplementation and/or stimulation with heat-killed V. anguillarum (Table 2, Figure 8).
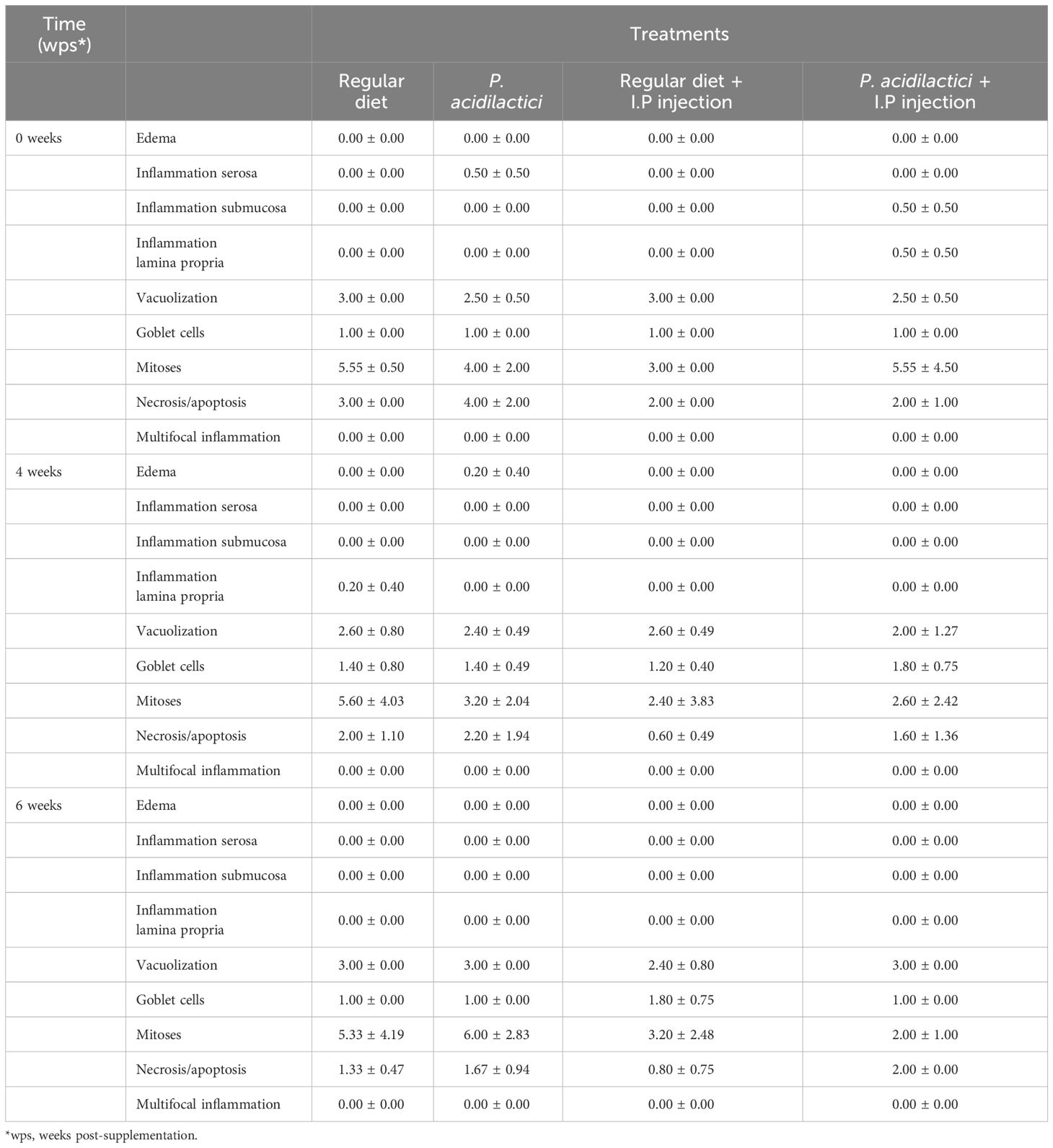
Table 2 Histopathological analysis of Chinook salmon hindgut (n = 5 per treatment per time-point) during six weeks of dietary and/or stimulation treatment (data is expressed as mean values ± S.E.M).
4 Discussion
In elucidating the intricacies of salmonid gut dynamics, this study pioneers a distinctive methodology employing transmembrane plates to model the gut environment. Our primary objective was to dissect the impact of P. acidilactici MA18/5M on the nuanced aspects of barrier function and integrity within this system. Moving beyond an examination of these fundamental parameters, we probed the expression of tight junctions and immune molecules upon exposure to the LAB. Furthermore, we explore the molecular mechanism through which V. anguillarum J382 exerts its disruptive potential on barrier function, unraveling the interplay between pathogens and the gut milieu. We also investigated the protective attributes of P. acidilactici MA18/5M against pathogen-induced assaults on the barrier, employing the in vitro model RTgutGC. Complementing these in vitro analyses, we extend our inquiry into the broader physiological, immunological, and histopathological landscape of juvenile Chinook salmon, broadening our understanding of the intricate relationship between P. acidilactici and the salmonid host organism.
Our results suggest that the sole interaction between P. acidilactici MA18/5M and RTgutGC cells did not elicit a statistically significant upregulation of immune (il1b, il8, il10, il6, tnfa, and tgfb) and TJ transcripts (cldn3, cldn12, zo-1; Figure 1). Moreover, pre-stimulation with P. acidilactici MA18/5M dampened the observed upregulation of il17a that occurred in presence of V. anguillarum J382 (Figure 4G), suggesting that P. acidilactici MA18/5M is a good candidate to evaluate in Chinook salmon.
Barrier formation and integrity was assessed by TEER measurements, which were in line with levels reported previously (37, 39, 57). Since the expression of barrier-forming TJ molecules was unchanged, an increase in resistance was not expected. A significant decrease in resistance was observed in cells exposed only to V. anguillarum J382 for 24 h, but pre-treatment with P. acidilactici MA18/5M for 48 h prior to exposure to the pathogen mitigated these effects (Figure 2). These results suggest that P. acidilactici MA18/5M can protect the epithelial barrier against pathogen-induced insults. The significant decrease in resistance given by V. anguillarum J382 is expected since the arsenal of virulence factors expressed by this bacterium are believed to degrade the epithelial barrier to gain access to the circulation (58). Improvements in barrier function have been reportedly associated with increased levels of related tight junction gene (cldn3 and cdh1) and protein (Claudin-3) levels (37, 39, 57). Although the data in the present study are in apparent contrast with these findings, the effect observed is modest and incubation with the probiotic strain protected against pathogen-induced damage but did not increase resistance after a 48-h incubation period. Further investigation of this phenomenon through orthogonal methods (such as Lucifer Yellow dye translocation studies) would be useful to ensure that the changes observed are physiologically relevant.
The effects of the fish pathogen V. anguillarum J382 were assessed in RTgutGC cells grown on conventional culture plates. Following a time-course coincubation experiment with live or heat-killed V. anguillarum J382, it was found that e-cadherin and jam-1a were significantly downregulated by 24 h post-infection in the live group relative to the heat-killed group and the baseline control (Figure 3). Interestingly, the expression of gut barrier protein occludin was extremely significantly increased in the live group at the 24 h timepoint. These data seem to suggest that V. anguillarum J382 not only impairs the barrier integrity, but the pathogen can also inhibit the expression of key barrier-forming TJ molecules.
The role of occludin in fish is not well understood, but studies in other organisms suggest that this protein is not only an integral component of tight junctions in various tissues, but that it can also participate in tight junction remodeling in response to cytokines (59, 60). High levels of proinflammatory cytokines, such as TNFA and IFNG, promote the endocytosis of OCCLUDIN in the tight junction complexes, which coincides with increases in tight junction permeability (61). Moreover, cytokine-induced changes in TEER and flux are directly proportional to OCCLUDIN levels (60). Paradoxically, the results in the present study appear to be at odds with the observations previously reported, in which the increased expression of occludin given by live V. anguillarum J382 exposure is associated with a decrease in resistance. Tight junctions have complex regulatory networks that dynamically respond to physiological stimuli (59). Therefore, post-transcriptional and post-translational modifications can impact the biological function of the junctions. Analyses that consider not only the molecular phenomena impacting barrier function, but also the dynamic nature of these intercellular junctions, would be instrumental in understanding how the gut epithelium responds to threats and activates immune defense mechanisms.
There was a robust upregulation of il17a and tgfb assessed by the 24 h timepoint for cells incubated with live V. anguillarum J382. Additionally, there was a time-dependent increase in the expression of il8 for both live and heat-killed groups, and these levels were significantly higher by 24 h (Figure 3). These results seem to indicate that il17a and tgfb are involved in the response to secreted virulence factors, whereas il8 might be more involved in the response to cell wall components such as LPS. These results are in line with the proposed mechanism of il8 induction given by LPS in other organisms (62). The role of il17a secreted by intestinal epithelial cells is less clear. In mammals, il17a is produced by a subset of T helper cells that induce the production of antimicrobial peptides, among other proinflammatory molecules (63). Host stimulation by LPS, peptidoglycans, and other antigens through pattern recognition receptors enables antigen-presenting cells to activate naïve T cells that mediate the adaptive immune response to the threat (63). Increased expression of il17a is also related to increased permeability of the blood brain barrier and small intestinal epithelial barrier (64). In the context of the present study, pathogen-induced upregulation of il17a can potentially enhance the damage to the epithelial barrier and thus contribute to the establishment of the infection.
A coculture experiment was employed to simulate a scenario in which LAB supplementation precedes pathogen exposure. Pre-treatment with LAB has been associated with protection from pathogen-induced injury to the intestinal epithelium in vitro (65). The data presented here suggest that the LAB strain tested has a mild effect in preventing pathogen-induced changes in the expression of key barrier proteins (Figure 4). Slight differences in trends of expression of zo-1 were observed in cells pre-treated with P. acidilactici 18MA/5M relative to the pre-treatment control group (i.e. Vibrio only), indicating that this strain can potentially induce the expression of this key tight junction molecule despite the presence of the pathogen. The expression of il-8 was highly increased by the 12h timepoint, indicating that the LAB strain tested was unable to dampen the excessive immune activation caused by V. anguillarum J382 that can lead to epithelial injury and further contribute to the infection.
To determine the effect of P. acidilactici MA18/5M on live fish health parameters, juvenile Chinook salmon reared under aquaculture conditions were supplemented for four months to determine the effect of this strain at physiological and immunological level. Fish growth is a key variable for the aquaculture industry, so it was imperative to start the study with similar size fish for every treatment. No differences associated to the candidate probiotic supplementation were seen during the trial. A previous P. acidilactici MA18/5M supplementation study performed in Atlantic salmon, showed that a 12-week supplementation with P. acidilactici MA18/5M did not improve the growth, specific growth rate (SGR), and thermal growth coefficient (TGC) (20). This, in addition to our findings in Chinook salmon, suggest that a longer supplementation time might be required to positively impact the fish growth desired for production. Confirmation that the strain is present and metabolically active would also be useful to rule out it simply passing through the gut due to rapid transit or too low a temperature for rehydration.
In the past, inflammation associated with the presence of antinutritional factors in plant ingredients used to feed salmonids (e.g. soybean meal products, soy protein concentrate) has increased the focus in evaluating possible inflammatory processes induced by external products in the fish intestine (66–68). Although it is unknown whether enteritis can be induced by probiotic supplementation, a histopathological analysis is of great importance to determine the safety of P. acidilactici MA18/5M supplementation in Chinook salmon. When P. acidilactici MA18/5M was added to the diet, there were no histological alterations, indicating that probiotic supplementation did not change the gut morphology in comparison to the regular pellet. Overall, in addition to the in vitro data indicating that P. acidilactici MA18/5M pre-treatment can protect the epithelial barrier against pathogen-induced damage, our study demonstrated that P. acidilactici MA18/5M supplementation does not substantially affect the gut epithelial barrier, making it a great candidate for future supplementation in Canadian aquaculture.
The fish pathogen, V. anguillarum, is well known for inducing immune transcripts of the inflammatory response when infecting salmonids (26, 69). Therefore, it is not surprising to see an increase in the expression of il1b, il8, and myd88 after inoculation with heat-killed V. anguillarum J382. Following this, upregulation of tgfb, a suppressor of the activation, proliferation, and function of T-cells to protect the organisms from inflammation is expected (70). However, we observed increases in the expression of these genes in non-injected fish. Even though more evidence is needed to confirm the results, we hypothesize that variation in the transcript encoding the genes seen here, is more likely to be associated with an ontogenetic process during the parr/smolt transition, instead of the treatments. A study conducted in coho salmon (Oncorhynchus kisutch) proposed that variations in the hepatic gene expression profiles observed in smolts and adults might be associated to complex physiological transformations as the fish start preparing to migrate towards seawater (71). Also, Johansen et al. (72) obtained differential expression of chemokines and antiviral genes in uninfected Atlantic salmon parr and smolts.
Similar to the immune genes evaluated in this study, TJ genes marveld2, cdh1, vil1, cldn15, ocln, jam1a, zo-1, and muc2 did not show a modulation pattern associated with probiotic supplementation, the heat-killed V. anguillarum J382 stimulation, or both. As hypothesized above, the parr/smolt transition represents an important physiological change in Chinook salmon, therefore, for genes with a complex regulatory network, such as TJ, variations can be extensive during this process. Since parr/smolt transformation represents a stressful stage that affects the intestinal homoeostasis of salmonids (20, 43), expected effects on gut barrier function after P. acidilactici MA18/5M supplementation might be masked by ontogenetic changes. To avoid this, future research should focus in determining the effect of P. acidilactici in adult Chinook salmon already transferred to sea pens.
5 Conclusion
The in vitro coculture system is a powerful and cost-effective tool for the investigation of host-microbe interactions. This study is the first of its kind to employ a tissue culture of the salmonid intestine in a semipermeable membrane system (Transwell) for investigating host-microbe interactions and evaluating the potential of P. acidilactici MA18/5M as a fish probiotic. Gene expression of immune and TJ genes and histopathological analysis supported the findings obtained in RTgutGC, showing that P. acidilactici MA18/5M supplementation does not negatively impact Chinook salmon homeostasis. Future immunostimulant research should focus in evaluating an increased number of TJ genes in addition to canonical immune response transcripts, since during probiotic supplementation, the gut plays a primary role in the host-microbe interaction.
In the context of this study, the P. acidilactici strain examined did not exhibit a strong ability to modulate barrier function in a salmonid intestinal cell line or enhance health parameters in live juvenile Chinook salmon. Importantly, since intraperitoneal infection does not simulate what occurs in natural environments, studies would benefit from using a different delivery method for infection, such as bath immersion, to compare the results obtained by our group. Although no deleterious effects were observed, the benefits provided to fish must be of sufficiently large magnitude to offset the costs in sourcing and administering the beneficial microbes and therefore make probiotic supplementation a viable, sustainable, and affordable solution for the industry.
Data availability statement
The datasets presented in this study can be found in online repositories. The names of the repository/repositories and accession number(s) can be found in the article/Supplementary Material.
Ethics statement
The animal study was approved by University of Waterloo Animal Care Committee. The study was conducted in accordance with the local legislation and institutional requirements.
Author contributions
MS-D: Writing – original draft, Methodology, Investigation, Formal analysis, Conceptualization. LL: Writing – original draft, Methodology, Investigation, Formal analysis, Conceptualization. JH: Writing – review & editing, Resources, Methodology, Funding acquisition. JL: Writing – review & editing, Methodology, Investigation. GR: Writing – review & editing, Supervision, Methodology, Funding acquisition, Formal analysis. BD: Writing – review & editing, Supervision, Resources, Project administration, Methodology, Funding acquisition, Conceptualization.
Funding
The author(s) declare financial support was received for the research, authorship, and/or publication of this article. The authors are grateful for the funding support provided by the NSERC Strategic Grant STPGP-521253-2018 to BD and GR. Additionally, LL was awarded an NSERC CGS-M scholarship to support this research.
Acknowledgments
The authors would also like to give special thanks to Drs. John Heath and Ann Heath from Yellow Island Aquaculture and their staff for providing and maintaining the fish, for hosting the research team year-round, and for the logistical support. Additionally, we thank Dr. Gediminas Cepinskas and Eric K. Patterson for their logistical and technical support in the TEER experiments. We would like to thank Maureen Latimer, Gillian McDonald, Ivan Cadonic, and Earl Heath for the invaluable support provided in each trial performed for this study. Finally, the authors would like to thank Shannon Seney and Nadeem Akhtar for their technical support with probiotic growth and for developing the probiotic-supplementation protocol utilized in this trial.
Conflict of interest
The authors declare that the research was conducted in the absence of any commercial or financial relationships that could be construed as a potential conflict of interest.
Publisher’s note
All claims expressed in this article are solely those of the authors and do not necessarily represent those of their affiliated organizations, or those of the publisher, the editors and the reviewers. Any product that may be evaluated in this article, or claim that may be made by its manufacturer, is not guaranteed or endorsed by the publisher.
Supplementary material
The Supplementary Material for this article can be found online at: https://www.frontiersin.org/articles/10.3389/fimmu.2024.1306458/full#supplementary-material
References
1. Oliva-Teles A. Nutrition and health of aquaculture fish. J Fish Dis. (2012) 35:83–108. doi: 10.1111/j.1365-2761.2011.01333.x
2. Teles M, Mackenzie S, Vallejos-Vidal E, Reyes-l F. Fish & Shellfish Immunology The response of fish to immunostimulant diets. Fish Shellfish Immunol. (2016) 56:34–69. doi: 10.1016/j.fsi.2016.06.028
3. Sakai M. Current research status of fish immunostimulants. Aquaculture (1999) 172:63–92. doi: 10.1016/S0044-8486(98)00436-0
4. Bridle AR, Carter CG, Morrison RN, Nowak BF. The effect of β-glucan administration on macrophage respiratory burst activity and Atlantic salmon, Salmo salar L., challenged with amoebic gill disease – evidence of inherent resistance. J Fish Dis. (2005) 28:347–56. doi: 10.1111/j.1365-2761.2005.00636.x
5. Song SK, Beck BR, Kim D, Park J, Kim J, Kim HD, et al. Prebiotics as immunostimulants in aquaculture: A review. Fish Shellfish Immunol. (2014) 40:40–8. doi: 10.1016/j.fsi.2014.06.016
6. Nayak SK. Probiotics and immunity: A fish perspective. Fish Shellfish Immunol. (2010) 29:2–14. doi: 10.1016/j.fsi.2010.02.017
7. Jamal MT, Sumon AA, Pugazhendi A, Al Harbi M, Hussain A, Haque F. Use of probiotics in commercially important finfish aquaculture. Int J Probiotics Prebiotics. (2020) 15:7–21. doi: 10.37290/ijpp2641-7197.15:7-21
8. Barman D, Tech H, Mandal SC, Kumar V. Immunostimulants for aquaculture health management. Journal of Marine Science: Research and Development (2013) 3:1000134. doi: 10.4172/2155-9910
9. Wang W, Sun J, Liu C, Xue Z. Application of immunostimulants in aquaculture: current knowledge and future perspectives. Aquac Res. (2017) 48:1–23. doi: 10.1111/are.13161
10. Lieke T, Meinelt T, Hoseinifar SH, Pan B, Straus DL. Sustainable aquaculture requires environmental-friendly treatment strategies for fish diseases. Reviews in Aquaculture (2019) 121–23. doi: 10.1111/raq.12365
11. Akhter N, Wu B, Memon AM, Mohsin M. Probiotics and prebiotics associated with aquaculture: A review. Fish Shellfish Immunol. (2015) 45:733–41. doi: 10.1016/j.fsi.2015.05.038
12. Langlois L, Akhtar N, Tam KC, Dixon B, Reid G. Fishing for the right probiotic: host–microbe interactions at the interface of effective aquaculture strategies. FEMS Microbiol Rev. (2021) 45:fuab030. doi: 10.1093/femsre/fuab030
13. Dawood MAO, Koshio S. Recent advances in the role of probiotics and prebiotics in carp aquaculture: A review. Aquaculture. (2016) 454:243–51. doi: 10.1016/j.aquaculture.2015.12.033
14. Hoseinifar SH, Sun Y-Z, Wang A, Zhou Z. Probiotics as means of diseases control in aquaculture, a review of current knowledge and future perspectives. Front Microbiol. (2018) 9:2429. doi: 10.3389/fmicb.2018.02429
15. Balcázar JL, de Blas I, Ruiz-Zarzuela I, Cunningham D, Vendrell D, Múzquiz JL. The role of probiotics in aquaculture. Vet Microbiol. (2006) 114:173–86. doi: 10.1016/j.vetmic.2006.01.009
16. Hill C, Guarner F, Reid G, Gibson GR, Merenstein DJ, Pot B, et al. Expert consensus document: The International Scientific Association for Probiotics and Prebiotics consensus statement on the scope and appropriate use of the term probiotic. Nat Rev Gastroenterol Hepatol. (2014) 11:506–14. doi: 10.1038/nrgastro.2014.66
17. Wang YB, Li JR, Lin J. Probiotics in aquaculture: Challenges and outlook. Aquaculture. (2008) 281:1–4. doi: 10.1016/j.aquaculture.2008.06.002
18. Pérez-Sánchez T, Ruiz-Zarzuela I, de Blas I, Balcázar JL. Probiotics in aquaculture: a current assessment. Rev Aquac. (2014) 6:133–46. doi: 10.1111/raq.12033
19. Soto-Dávila M, Latimer MF, Dixon B. “Chapter 4 - Enhancing immune function and fish health in aquaculture,”. In: Benfey TJ, Farrell AP, Brauner CJ, editors. Aquaculture. San Diego: Academic Press. (2020) p. 123–61. doi: 10.1016/bs.fp.2020.09.003
20. Jaramillo-Torres A, Rawling MD, Rodiles A, Mikalsen HE, Johansen L-H, Tinsley J, et al. Influence of dietary supplementation of probiotic Pediococcus acidilactici MA18/5M during the transition from freshwater to seawater on intestinal health and microbiota of Atlantic salmon (Salmo salar L.). Front Microbiol. (2019) 10:2243. doi: 10.3389/fmicb.2019.02243
21. Hoseinifar SH, Hosseini M, Paknejad H, Safari R, Jafar A, Yousefi M, et al. Enhanced mucosal immune responses, immune related genes and growth performance in common carp (Cyprinus carpio) juveniles fed dietary Pediococcus acidilactici MA18/5M and raffinose. Dev Comp Immunol. (2019) 94:59–65. doi: 10.1016/j.dci.2019.01.009
22. Abid A, Davies SJ, Waines P, Emery M, Castex M, Gioacchini G, et al. Dietary synbiotic application modulates Atlantic salmon (Salmo salar) intestinal microbial communities and intestinal immunity. Fish Shellfish Immunol. (2013) 35:1948–56. doi: 10.1016/j.fsi.2013.09.039
23. Merrifield DL, Bradley G, Harper GM, Baker RTM, Munn CB, Davies SJ. Assessment of the effects of vegetative and lyophilized Pediococcus acidilactici on growth, feed utilization, intestinal colonization and health parameters of rainbow trout (Oncorhynchus mykiss Walbaum). Aquac Nutr. (2011) 17:73–9. doi: 10.1111/anu.2010.17.issue-1
24. Chi C, Jiang B, Yu X-B, Liu T-Q, Xia L, Wang G-X. Effects of three strains of intestinal autochthonous bacteria and their extracellular products on the immune response and disease resistance of common carp. Cyprinus carpio Fish Shellfish Immunol. (2014) 36:9–18. doi: 10.1016/j.fsi.2013.10.003
25. Olson DE, Paiya M. An evaluation of rearing densities to improve growth and survival of hatchery spring Chinook salmon. J Fish Wildl Manag. (2013) 4:114–23. doi: 10.3996/042010-JFWM-009
26. Semple SL, Heath G, Filice CT, Heath DD, Dixon B. The impact of outbreeding on the immune function and disease status of eight hybrid Chinook salmon crosses after Vibrio Anguillarum challenge. Aquac Res. (2022) 53:957–73. doi: 10.1111/are.15636
27. Canadian Food Inspection Agency C. Share your thoughts: Proposed new livestock feed ingredient – Pediococcus acidilactici culture dehydrated Current status: Closed Proposed SIF description. (2023).
28. Houde ALS, Wilson CC, Neff BD. Competitive interactions among multiple non-native salmonids and two populations of Atlantic salmon. Ecol Freshw Fish. (2015) 24:44–55. doi: 10.1111/eff.12123
29. Toews SD, Wellband KW, Dixon B, Heath DD. Variation in juvenile Chinook salmon (Oncorhynchus tshawytscha) transcription profiles among and within eight population crosses from British Columbia, Canada. Mol Ecol. (2019) 28:1890–903. doi: 10.1111/mec.15025
30. Semeniuk CAD, Capelle PM, Dender MGE, Devlin R, Dixon B, Drown J, et al. Domestic-wild hybridization to improve aquaculture performance in Chinook salmon. Aquaculture. (2019) 511:734255. doi: 10.1016/j.aquaculture.2019.734255
31. Christensen KA, Leong JS, Sakhrani D, Biagi CA, Minkley DR, Withler RE, et al. Chinook salmon (Oncorhynchus tshawytscha) genome and transcriptome. PloS One. (2018) 13:e0195461. doi: 10.1371/journal.pone.0195461
32. Olsson JC, Joborn A, Westerdahl A, Blomberg L, Kjelleberg S, Conway PL. Is the turbot, Scophthalmus maximus (L.), intestine a portal of entry for the fish pathogen Vibrio Anguillarum? J Fish Dis. (1996) 19:225–34. doi: 10.1111/j.1365-2761.1996.tb00129.x
33. Lee P-T, Yamamoto FY, Low C-F, Loh J-Y, Chong C-M. Gut immune system and the implications of oral-administered immunoprophylaxis in finfish aquaculture. Front Immunol. (2021) 12:773193. doi: 10.3389/fimmu.2021.773193
34. Dawood MAO. Nutritional immunity of fish intestines: important insights for sustainable aquaculture. Rev Aquac. (2021) 13:642–63. doi: 10.1111/raq.12492
35. Lafferty KD, Harvell CD, Conrad JM, Friedman CS, Kent ML, Kuris AM, et al. Infectious diseases affect marine fisheries and aquaculture economics. Ann Rev Mar Sci. (2015) 7:471–96. doi: 10.1146/annurev-marine-010814-015646
36. Kawano A, Haiduk C, Schirmer K, Hanner R, Lee LEJ, Dixon B, et al. Development of a rainbow trout intestinal epithelial cell line and its response to lipopolysaccharide. Aquac Nutr. (2011) 17:241–52. doi: 10.1111/anu.2011.17.issue-2
37. Minghetti M, Drieschner C, Bramaz N, Schug H, Schirmer K. A fish intestinal epithelial barrier model established from the rainbow trout (Oncorhynchus mykiss) cell line, RTgutGC. Cell Biol Toxicol. (2017) 33:539–55. doi: 10.1007/s10565-017-9385-x
38. Langan LM, Harper GM, Owen SF, Purcell WM, Jackson SK, Jha AN. Application of the rainbow trout derived intestinal cell line (RTgutGC) for ecotoxicological studies: molecular and cellular responses following exposure to copper. Ecotoxicology. (2017) 26:1117–33. doi: 10.1007/s10646-017-1838-8
39. Wang J, Lei P, Gamil AAA, Lagos L, Yue Y, Schirmer K, et al. Rainbow trout (Oncorhynchus mykiss) intestinal epithelial cells as a model for studying gut immune function and effects of functional feed ingredients. Front Immunol. (2019) 10:152. doi: 10.3389/fimmu.2019.00152
40. Løkka G, Gamil AAA, Evensen Ø, Kortner TM. Establishment of an in vitro model to study viral infections of the fish intestinal epithelium. Cells. (2023) 12:1351. doi: 10.3390/cells12111531
41. Hubatsch I, Ragnarsson EGE, Artursson P. Determination of drug permeability and prediction of drug absorption in Caco-2 monolayers. Nat Protoc. (2007) 2:2111–9. doi: 10.1038/nprot.2007.303
42. Machimbirike VI, Vasquez I, Cao T, Chukwu-Osazuwa J, Onireti O, Segovia C, et al. Comparative genomic analysis of virulent Vibrio (Listonella) Anguillarum serotypes revealed genetic diversity and genomic signatures in the O-antigen biosynthesis gene cluster. Microorganisms. (2023) 11:792. doi: 10.3390/microorganisms11030792
43. Wang J, Kortner TM, Chikwati EM, Li Y, Jaramillo-Torres A, Jakobsen JV, et al. Gut immune functions and health in Atlantic salmon (Salmo salar) from late freshwater stage until one year in seawater and effects of functional ingredients: A case study from a commercial sized research site in the Arctic region. Fish Shellfish Immunol. (2020) 106:1106–19. doi: 10.1016/j.fsi.2020.09.019
44. Schug H, Yue Y, Krese R, Fischer S, Kortner TM, Schirmer K. Time- and concentration-dependent expression of immune and barrier genes in the RTgutGC fish intestinal model following immune stimulation. Fish Shellfish Immunol. (2019) 88:308–17. doi: 10.1016/j.fsi.2019.02.036
45. Semple SL, Kellendonk CJ, Al-Hussinee L, MacInnes JI, Lumsden JS, Dixon B. Serum IgM, MH class IIβ genotype and respiratory burst activity do not differ between rainbow trout families displaying resistance or susceptibility to the coldwater pathogen, Flavobacterium psychrophilum. Aquaculture. (2018) 483:131–40. doi: 10.1016/j.aquaculture.2017.10.020
46. Ma F, Liu Z, Huang J, Kang Y, Wang J. Evaluation of reference genes for quantitative real-time PCR analysis of messenger RNAs and microRNAs in rainbow trout Oncorhynchus mykiss under heat stress. J Fish Biol. (2019) 95:540–54. doi: 10.1111/jfb.13986
47. Rajanbabu V, Chen J-Y. Antiviral function of tilapia hepcidin 1–5 and its modulation of immune-related gene expressions against infectious pancreatic necrosis virus (IPNV) in Chinook salmon embryo (CHSE)-214 cells. Fish Shellfish Immunol. (2011) 30:39–44. doi: 10.1016/j.fsi.2010.09.005
48. Giroux M, Gan J, Schlenk D. The effects of bifenthrin and temperature on the endocrinology of juvenile Chinook salmon. Environ Toxicol Chem. (2019) 38:852–61. doi: 10.1002/etc.4372
49. Eder KJ, Leutenegger CM, Köhler H-R, Werner I. Effects of neurotoxic insecticides on heat-shock proteins and cytokine transcription in Chinook salmon (Oncorhynchus tshawytscha). Ecotoxicol Environ Saf. (2009) 72:182–90. doi: 10.1016/j.ecoenv.2008.04.020
50. Livak KJ, Schmittgen TD. Analysis of relative gene expression data using real-time quantitative PCR and the 2(-Delta Delta C(T)) Method. Methods. (2001) 25:402–8. doi: 10.1006/meth.2001.1262
51. Pfaffl MW. A new mathematical model for relative quantification in real-time RT-PCR. Nucleic Acids Res. (2001) 29:e45. doi: 10.1093/nar/29.9.e45
52. Andersen CL, Jensen JL, Ørntoft TF. Normalization of real-time quantitative reverse transcription-PCR data: A model-based variance estimation approach to identify genes suited for normalization, applied to bladder and colon cancer data sets. Cancer Res. (2004) 64:5245–50. doi: 10.1158/0008-5472.CAN-04-0496
53. Silver N, Best S, Jiang J, Thein SL. Selection of housekeeping genes for gene expression studies in human reticulocytes using real-time PCR. BMC Mol Biol. (2006) 7:1–9. doi: 10.1186/1471-2199-7-33
54. Vandesompele J, De Preter K, Pattyn F, Poppe B, Van Roy N, De Paepe A, et al. Accurate normalization of real-time quantitative RT-PCR data by geometric averaging of multiple internal control genes (2002). Available online at: http://genomebiology.com/2002/3/7/research/0034.1Correspondence:.rankSpeleman.
55. Xie F, Xiao P, Chen D, Xu L, Zhang B. miRDeepFinder: a miRNA analysis tool for deep sequencing of plant small RNAs. Plant Mol Biol. (2012) 80:75–84. doi: 10.1007/s11103-012-9885-2
56. Pumputis PG, Dayeh VR, Lee LEJ, Pham PH, Liu Z, Viththiyapaskaran S, et al. Responses of rainbow trout intestinal epithelial cells to different kinds of nutritional deprivation. Fish Physiol Biochem. (2018) 44:1197–214. doi: 10.1007/s10695-018-0511-3
57. Geppert M, Sigg L, Schirmer K. A novel two-compartment barrier model for investigating nanoparticle transport in fish intestinal epithelial cells. Environ Sci Nano. (2016) 3:388–95. doi: 10.1039/C5EN00226E
58. Frans I, Michiels CW, Bossier P, Willems KA, Lievens B, Rediers H. Vibrio Anguillarum as a fish pathogen: virulence factors, diagnosis and prevention. J Fish Dis. (2011) 34:643–61. doi: 10.1111/j.1365-2761.2011.01279.x
59. Sawada N. Tight junction-related human diseases. Pathol Int. (2013) 63:1–12. doi: 10.1111/pin.12021
60. Van Itallie CM, Fanning AS, Holmes J, Anderson JM. Occludin is required for cytokine-induced regulation of tight junction barriers. J Cell Sci. (2010) 123:2844–52. doi: 10.1242/jcs.065581
61. Yu D, Turner JR. Stimulus-induced reorganization of tight junction structure: The role of membrane traffic. Biochim Biophys Acta - Biomembr. (2008) 1778:709–16. doi: 10.1016/j.bbamem.2007.07.027
62. Yan Y, Wang Z, Greenwald J, Kothapalli KSD, Park HG, Liu R, et al. BCFA suppresses LPS induced IL-8 mRNA expression in human intestinal epithelial cells. Prostaglandins Leukot Essent Fat Acids. (2017) 116:27–31. doi: 10.1016/j.plefa.2016.12.001
63. Iwakura Y, Nakae S, Saijo S, Ishigame H. The roles of IL-17A in inflammatory immune responses and host defense against pathogens. Immunol Rev. (2008) 226:57–79. doi: 10.1111/j.1600-065X.2008.00699.x
64. Rahman MT, Ghosh C, Hossain M, Linfield D, Rezaee F, Janigro D, et al. IFN-γ, IL-17A, or zonulin rapidly increase the permeability of the blood-brain and small intestinal epithelial barriers: Relevance for neuro-inflammatory diseases. Biochem Biophys Res Commun. (2018) 507:274–9. doi: 10.1016/j.bbrc.2018.11.021
65. Karimi S, Jonsson H, Lundh T, Roos S. Lactobacillus reuteri strains protect epithelial barrier integrity of IPEC-J2 monolayers from the detrimental effect of enterotoxigenic. Escherichia coli Physiol Rep. (2018) 6:1–12. doi: 10.14814/phy2.13514
66. Djordjevic B, Morales-Lange B, Øverland M, Mercado L, Lagos L. Immune and proteomic responses to the soybean meal diet in skin and intestine mucus of Atlantic salmon (Salmo salar L.). Aquac Nutr. (2021) 27:929–40. doi: 10.1111/anu.13248
67. Król E, Douglas A, Tocher DR, Crampton VO, Speakman JR, Secombes CJ, et al. Differential responses of the gut transcriptome to plant protein diets in farmed Atlantic salmon. BMC Genomics. (2016) 17:156. doi: 10.1186/s12864-016-2473-0
68. Krogdahl Å, Dhanasiri AKS, Krasnov A, Aru V, Chikwati EM, Berge GM, et al. Effects of functional ingredients on gut inflammation in Atlantic salmon (Salmo salar L). Fish Shellfish Immunol. (2023) 134:108618. doi: 10.1016/j.fsi.2023.108618
69. Johnson KA, Flynn JK, Amend DF. Onset of immunity in salmonid fry vaccinated by direct immersion in Vibrio Anguillarum and Yersinia ruckeri bacterins. J Fish Dis. (1982) 5:197–205. doi: 10.1111/j.1365-2761.1982.tb00474.x
70. Zhang Q, Geng M, Li K, Gao H, Jiao X, Ai K, et al. TGF-β1 suppresses the T-cell response in teleost fish by initiating Smad3- and Foxp3-mediated transcriptional networks. J Biol Chem. (2023) 299:102843. doi: 10.1016/j.jbc.2022.102843
71. Gallagher EP, LaVire HM, Bammler TK, Stapleton PL, Beyer RP, Farin FM. Hepatic expression profiling in smolting and adult coho salmon (Onchorhynchus kisutch). Environ Res. (2008) 106:365–78. doi: 10.1016/j.envres.2007.10.001
Keywords: RTgutGC, Chinook salmon, Pediococcus acidilactici MA 18/5M, immune response, gut barrier function, tight junctions, histopathological analysis, Transwell
Citation: Soto-Dávila M, Langlois Fiorotto L, Heath JW, Lumsden JS, Reid G and Dixon B (2024) The effects of Pediococcus acidilactici MA18/5M on growth performance, gut integrity, and immune response using in vitro and in vivo Pacific salmonid models. Front. Immunol. 15:1306458. doi: 10.3389/fimmu.2024.1306458
Received: 04 October 2023; Accepted: 06 March 2024;
Published: 27 March 2024.
Edited by:
Tor Gjøen, University of Oslo, NorwayReviewed by:
Vikash Kumar, Central Inland Fisheries Research Institute (ICAR), IndiaPrabhugouda Siriyappagouder, Nord University, Norway
Eva Vallejos-Vidal, Universidad de las américas, Chile
Anusha Kirhanthi Shyama Dhanasiri, Norwegian University of Life Sciences, Norway
Copyright © 2024 Soto-Dávila, Langlois Fiorotto, Heath, Lumsden, Reid and Dixon. This is an open-access article distributed under the terms of the Creative Commons Attribution License (CC BY). The use, distribution or reproduction in other forums is permitted, provided the original author(s) and the copyright owner(s) are credited and that the original publication in this journal is cited, in accordance with accepted academic practice. No use, distribution or reproduction is permitted which does not comply with these terms.
*Correspondence: Brian Dixon, bdixon@uwaterloo.ca
†These authors have contributed equally to this work and share first authorship