- Department of Immunology, Mayo Clinic, Scottsdale, AZ, United States
Tissue-resident memory T cells (TRM cells) are vital for the promotion of barrier immunity. The lung, a tissue constantly exposed to foreign pathogenic or non-pathogenic antigens, is not devoid of these cells. Lung TRM cells have been considered major players in either the protection against respiratory viral infections or the pathogenesis of lung allergies. Establishment of lung TRM cells rely on intrinsic and extrinsic factors. Among the extrinsic regulators of lung TRM cells, the magnitude of the impact of factors such as the route of antigen entry or the antigen natural tropism for the lung is not entirely clear. In this perspective, we provide a summary of the literature covering this subject and present some preliminary results on this potential dichotomy between antigen location versus antigen type. Finally, we propose a hypothesis to synthesize the potential contributions of these two variables for lung TRM cell development.
Introduction
The lung is a respiratory organ specialized in gas exchange: within its alveoli, oxygen is extracted from the air and exchanged by carbon dioxide. A consequence of this fundamental role is the constant exposure to airborne antigens, innocuous or pathogenic. Many of these antigens elicit strong T cell responses, and understanding how those responses form is crucial to define how these immune responses can either eliminate pathogenic threats or promote unwanted responses to innocuous agents. CD4+ and CD8+ T cells are primed by antigen-presenting cells in secondary lymphoid organs, and effector cells migrate towards antigen-rich sites to perform their function (1, 2). After antigen clearance, a portion of T cells survive long-term, forming memory populations which are important to mount quick, efficient responses against secondary antigen exposure (3). Memory T cells can be divided by their migratory characteristics. Circulating memory T cells (TCIRCM) recirculate between blood, secondary lymphoid organs, and tissues, without taking up residency; these cells can be further subdivided into central memory (TCM), effector memory (TEM) and, in the case of CD8+ T cells, long-lived effector cells (LLEC) (3, 4). In contrast, resident memory T cells (TRM) establish long-term residency in tissues, mostly barrier tissues (5). The lung is, naturally, one of these tissues. Prior evidence strongly suggests that lung TRM cells are pivotal in promoting local immune responses which can either be protective against pathogens (6, 7) or deleterious – for example, in response to allergens (8).
Since their discovery (9, 10), several studies aimed to define how TRM cells form in the lung, as well as their specific function. From these studies, a few notions are relatively well-established. First, both CD4+ and CD8+ T cells can form lung TRM or TRM-like populations, and this is true in response to infections (11, 12) and to allergens (8). Second, while CD4+ lung TRM cells are somewhat stable over time (7, 8), CD8+ lung TRM cells are notoriously short-lived, with a faster rate of decay if compared to CD8+ TRM cells in other tissues (13, 14). Finally, both CD8+ and CD4+ T cells form heterogeneous lung TRM populations, with distinct transcriptional and functional characteristics (7, 14, 15). There are, however, several unanswered questions. One of the most important unsolved puzzles in the biology of lung TRM cells lies on the nature of the signals that educate T cells to acquire a resident memory phenotype. While much evidence points out that the routes of infection (or sensitization) are paramount in defining the magnitude of the lung TRM response, other works suggest that, at least partially, the type of antigen can dictate the homeostasis of lung TRM cells. In this perspective article, we will briefly review previous research, provide preliminary data, and propose a hypothesis for this outstanding question.
Where am I from? How the route of antigen priming can affect lung TRM cell establishment
TRM cell development occurs through a series of processes where initial priming, T cell sensing of peripheral tissue-derived signals, and tissue microenvironmental factors play a role in the acquisition of a TRM signature (16, 17). Because of their residency establishment inside the lung parenchyma, lung TRM cells must acquire certain transcriptional and protein expression characteristics. Among these characteristics, T cells (a) downregulate molecules associated with tissue egress (e.g., CCR7, S1PR1 and S1PR5), as well as upregulate molecules associated with tissue retention (e.g., TGF-βRII, CD69 and/or CD103) (17–19), and (b) express chemokine receptors such as CXCR3, which sense CCL9 and/or CCL10 released in the lung parenchyma during local immune responses (12, 20). The need for sensing of lung-derived chemokines means that optimal alterations in the lung microenvironment are paramount for the formation of lung TRM cells. These changes, such as production of CCL9 or CCL10 or of IL-33, a danger signal associated with heightened lung inflammation (21), are associated with local tissue antigen recognition. Indeed, airway infections or immunizations are very effective in the generation of lung TRM cells, and persistent antigen in the lungs promote long-term survival of lung TRM cells (22–26). The notion that lung initial antigen encounter is necessary for optimal lung-resident T cell responses is relatively well-established (27). In response to murine influenza, local antigen encounter is needed for CD8+ TRM cell establishment (28), and the same is true for TRM cells forming in response to Bacillus Calmette-Guerin (BCG) vaccination (23, 29).
Lung mucosal sites are often the first barrier encountered by pathogens or allergens. These sites are composed of a complex network of heterogeneous epithelial cells, peripheral nervous cells, innate and adaptive immune cells, covered by a mucous layer. Each one of these components can harness the tissue inflammation following local infections or allergen sensitization. IL-33 production by epithelial cells (21), nervous system regulation of immune responses (30), and the capture of antigens by local dendritic cells (31, 32) all play a role in the initiation and sustenance of lung T cell responses. The lung, due to its physiological role, must balance the induction of such responses with maintenance of its function of gas exchange. During viral infections, for example, the balance between pathogen clearance and immune modulation is tightly regulated by the epithelial cell-immune cell axis, and dysregulation of this balance can lead to severe tissue damage (33). Consequently, the production and release of effector molecules is likely regulated even within the lung tissue.
Due to the highly controlled immune environment in the lung, the presence of adjuvants to elucidate immune responses is widely used to enhance immunogenicity in the lung. Adjuvants (which are common components of vaccines) can increase the magnitude and durability of antiviral immunity, impacting the phenotype of recruited innate cells (34). In response to infections or airway allergen sensitization, natural adjuvants are pathogen-associated molecular patterns (PAMPs) present on viruses, bacteria, fungi, protozoans, recognized by pattern recognition receptors (PRRs) expressed by epithelial and resident immune cells. The establishment of an appropriate resistant or tolerant environment, the engagement of distinct PRR combinations, results in recruitment of immune cell types and cytokines produced (35). This, associated with a combination of cytokines, chemokines, and danger signals, offer evidence that the lung microenvironment is critical to promote lung TRM cell establishment.
Studies on TRM cells have focused on identification of tissue-derived signals, while understanding how priming of committed precursors in distinct secondary lymphoid organs has been less explored (36). Dendritic cells, for instance, are responsible for the imprinting of specific migratory patterns in the T cells during activation. DCs in skin-draining lymph nodes induce the preferential expression of homing molecules for entry into skin, whereas DCs in mesenteric lymph nodes elicit tropism for the small intestine (37). This indicates that TRM cell preconditioning in an organ-dependent way already occurs during homeostasis, whereas imprinting for tissue-selective homing occurs during T cell priming. In addition, migratory DCs from different tissues might have varying capacities for TGF-β activation in draining lymph nodes, since preconditioning was less pronounced in mediastinal lymph nodes, even though these tissues had comparable induction of CD103 in naïve T cells at both sites (38). This adds another layer on how the route of antigen priming can regulate the quality and/or magnitude of lung TRM cell establishment.
Other routes of antigen entry, such as intramuscular immunizations, can also induce lung TRM cells, but the phenotype of these cells seems to be heterogenic, with lower proportion of cells located in the lung parenchyma (39). Thus, intramuscular immunizations have traditionally been considered poor inducers of mucosal TRM cell responses (40, 41). Intranasal vaccination strategies can induce strong protection, as evidenced by past studies on RSV, Mtb and influenza (23, 26, 27, 42–44). However, this route has potential issues in antigen delivery to dendritic cells in the respiratory tract, perhaps due to physical barriers such as nasal clearing or mucus (45–47). A combination of intramuscular (i.e., distal antigen priming) and intranasal immunization approaches has been suggested as a candidate to enhance lung TRM cell development in response to vaccines (41, 48, 49). Another evidence from vaccination also challenges the notion that lung antigen priming is the sole factor inducing optimal lung TRM cells: the recent revolution in mRNA vaccines to combat SARS-CoV-2 (50) and, more recently, influenza (51). These immunizations, which are intramuscular, lead to robust lung CD4+ and CD8+ TRM-like cell responses, as studies in mice suggest (48, 51). Future studies will be necessary to identify how mRNA vaccines promote lung TRM cells even without intranasal priming, and whether long-lived lung TRM cells are generated in humans. It is important to note that, although these studies suggest that intranasal immunization is not strictly necessary for lung TRM cell responses, intranasal priming is still sufficient to improve lung TRM cell establishment in these cases (23, 26, 27).
Who am I? How antigen nature and tropism can influence lung TRM cell establishment
In contrast with evidence for route of antigen priming, other factors may also dictate the generation of lung TRM cells, for example antigen (pathogen) load, pathogen life cycle characteristics, or the strength of TCR-MHC interaction. In mouse models of viral infection, CD8+ TRM cells in brain and kidney express higher affinity to MHC class I tetramers (> 20x) than TCIRCM cells (52). We observed a similar trend in preliminary experiments comparing influenza-specific lung TRM cells with TCIRCM cells (Figure 1A), suggesting that a selection of high-affinity clones may also happen for lung CD8+ TRM cells.
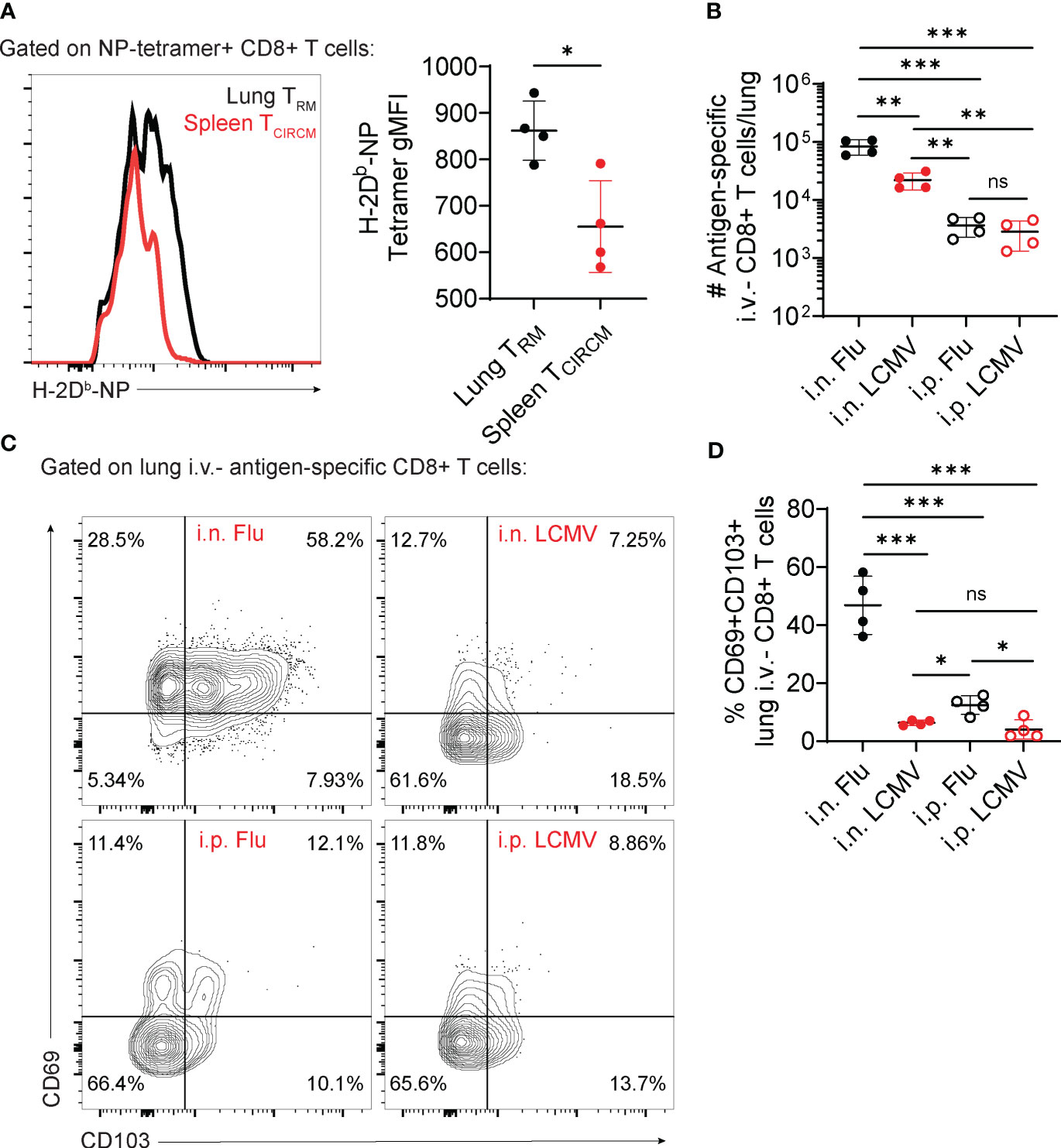
Figure 1 Contribution of antigen nature versus route of priming for lung CD8+ TRM cell establishment. (A–D) C57BL/6 mice were infected with influenza, PR8 strain (Flu) or LCMV-Armstrong (LCMV) through the indicated infection routes (intranasal – i.n; intraperitoneal – i.p.). At day 28 post-infection, the number and phenotype of antigen-specific CD8+ T cells were assessed. (A) Representative histogram (left) and average gMFI values (right) of H-2Db-NP tetramer staining in NP tetramer+ lung versus spleen CD8+ T cells. (B) Average numbers of antigen-specific lung i.v.- CD8+ T cells (CD44+ H-2Db-tetramer+). (C) Representative flow cytometry plots showing expression of CD69 and CD103 in antigen-specific lung i.v.- CD8+ T cells. (D) Average percentages of CD69+CD103+ antigen-specific lung i.v.- CD8+ T cells. Data from two independent experiments, n=4. (A) Unpaired t-test (B, D) One-way ANOVA with Tukey’s post-test, *p<0.05, **p<0.01, ***p<0.001. ns, not significant.
A preferential selection of lung TRM cells with high TCR affinity for antigen could either occur at the effector stage, after T cells migrated to the lung tissue, or at the priming and activation stage, in secondary lymphoid organs. Although these two hypothetical scenarios would suggest a major effect of the route of priming as a selector of lung TRM cells, different lung viral infections induce distinct magnitudes of a lung TRM cell response. In mice, while in response to influenza >50% of lung memory CD8+ T cells express the TRM markers CD69 and CD103 (14), in response to RSV or BCG these numbers are much lower (23, 53). These differences may suggest that distinct antigen types or differences in TCR affinity regulate the establishment and phenotype of lung TRM cells. Alternatively, they could also be explained by differences in how distinct pathogens interact with the lung immune system, for example differences in induction of cytokine production.
To test the potential contributions of route of priming versus antigen type, we infected mice with LCMV, Armstrong strain (a systemic virus with no tropism for the mouse lung) or influenza, using intraperitoneal versus intranasal infection routes. Intranasal infection with LCMV or influenza led to significantly increased numbers of lung parenchymal antigen-specific CD8+ T cell accumulation (Figure 1B). Per se, these results are indicative of the importance of airway antigen entry in the formation of lung TRM cells. However, the magnitude of lung TRM cell accumulation is higher in response to intranasal influenza if compared to intranasal LCMV (Figure 1B). A more detailed characterization of these lung TRM cells also show that intranasal influenza is unique in promoting upregulation of CD69 and CD103, in comparison to intranasal LCMV (Figures 1C, D). Confirming previous findings (27, 54), intraperitoneal influenza, despite failing to promote the numerical accumulation of lung TRM cells (Figure 1B), was sufficient to induce a consistent upregulation of CD69 and CD103 in a small proportion of lung TRM cells (Figures 1C, D). These preliminary findings suggest that, despite an important role for the intranasal route of immunization, the acquisition of a classic lung TRM phenotype may strongly rely on the antigen type, more specifically their natural lung tropism.
Conclusions and a proposed hypothesis
Most past studies strongly suggest that airway exposure to antigens is an important factor in the establishment of lung TRM cells, but additional evidence from us and others also point to the antigen type, more specifically its natural lung tropism, as another regulating factor. We believe that optimal lung TRM cell generation will take advantage of these two variables, and the magnitude of lung TRM cell responses obeys a continuum (Figure 2). In response to airway exposure to lung allergens or to respiratory infections, both lung antigen tropism and airway route of exposure are present, and consequently a strong lung TRM cell response is mounted. On the other end of the spectrum, systemic infections with pathogens lacking lung tropism do not elicit lung TRM cell responses. In “hybrid” scenarios, such as intraperitoneal exposure to influenza or intranasal exposure to LCMV, lung TRM cell generation will be partial, with the magnitude of the response relying on other factors induced by either lung inflammatory responses or antigen persistence.
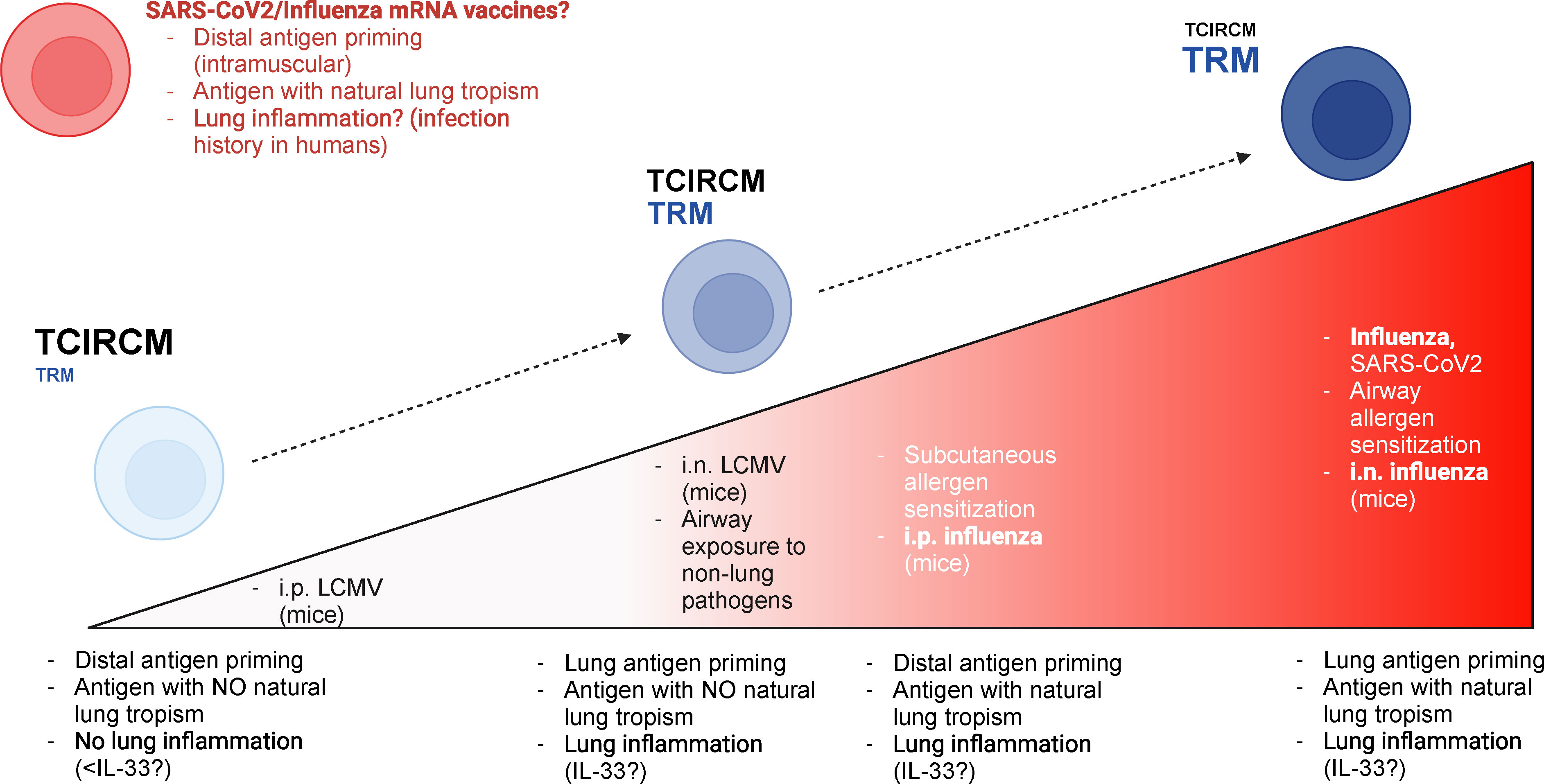
Figure 2 Hypothetical model for the relative contributions of antigen priming location versus antigen nature for lung TRM generation. Based on past research and on our preliminary results, we postulate that the TRM education of lung T cells relies on both local antigen priming and the lung tropism of relevant antigens. The magnitude of lung TRM responses is at its peak when lung-tropic antigens are recognized locally (i.e., in the lung); this is the case, for example, in response to respiratory infections such as influenza or SARS-CoV-2 or to intranasal allergen sensitization. On the other hand, recruitment of lung TRM cells is minimal when antigens with no lung tropism are recognized distally (e.g., systemic, or subcutaneous). When antigens with lung tropism are introduced through non-airway routes, the magnitude of lung TRM cell accumulation is greatly reduced, but some accumulation may still occur; lung inflammatory responses may play an essential role in this scenario, by creating chemotactic signals for lung T cell infiltration. An example of this situation in real life is subcutaneous exposure to lung allergens. Finally, local recognition of antigens with no lung tropism, at least in experimental models, can induce a relevant accumulation of lung parenchymal T cells; however, these cells still fail to acquire a bona-fide TRM phenotype, perhaps due to diminished lung inflammation – since the lack of lung tropism hinders the ability of such antigens to establish in the lung tissue. A possible exception to this rule is the product of mRNA vaccines to SARS-CoV-2 or influenza (which are in clinical trials). These immunizations, which are distal (i.e., intramuscular), would theoretically lead to intermediate lung TRM responses based on our model; however, current evidence suggests a strong lung T cell accumulation upon immunization. It is possible that a heightened state of basal lung inflammation (due to past infection history in humans) could tip the balance in favor of stronger lung TRM cell responses, even in the absence of local priming. This figure was generated using BioRender.
Some questions, however, are still unanswered. First, what is the exact influence of lung inflammatory responses (such as the ones induced by innate immune cells or epithelial cells) in this “TRM continuum”? When considering our intranasal LCMV system, for example, the lack of a CD69/CD103 phenotype can be due to changes during T cell priming, but the lack of lung tropism of LCMV possibly also translates in decreased infectivity in the lungs. Consequently, inflammatory responses during the acute phase are expected to be lower in lungs, which could influence the local release of signals such as TGF-β, which are necessary to educate nascent TRM cells for CD103 expression (55). CD8+ TRM cells can be generated in tissues without antigen if sterile inflammation is administered to such tissues simultaneously to systemic antigen immunization. This is true for skin TRM cells (56, 57) and female reproductive tract TRM cells (58). Future systematic investigations on whether such “prime and pull” strategies are sufficient for lung TRM cell generation will be important.
Another important point to consider is the fact that CD4+ and CD8+ TRM cells, despite sharing common pathways and molecular requirements, are not the same. CD4+ TRM cells typically locate outside of epithelial sites, partly due to their inability to respond to TGF-β – which is controlled by their downregulation of Runx3 (59). This is also true in the lungs, where CD4+ TRM cells are mostly concentrated within the lung parenchyma, with some of them in close contact with B cells and other immune cells (7, 15). Our model heavily takes into consideration our findings with lung CD8+ TRM cells, as well as the abundant literature on these cells (6, 13, 14, 28). It will be interesting to assess the relative contributions of the route of priming versus antigen tropism (versus local inflammation) for lung CD4+ TRM cell establishment. In conclusion, in this perspective we provided a short review of the known literature on how lung TRM cells form, and how lung tissue versus antigen type can influence their formation. Understanding the relative roles of each one of these variables will lead, in our opinion, to the discovery of more efficient approaches to boost the generation of lung TRM cells that can provide protection against infections – or to block undesirable lung TRM cell formation in response to lung allergens.
Materials and methods
Mice
Male and female 6- to 8-week-old adult C57BL/6 (B6) mice were purchased from Jackson and were allowed to acclimate to our housing facilities for at least one week. Animals were maintained under specific-pathogen-free conditions at Mayo Clinic Arizona. In all experiments, mice were randomly assigned to experimental groups. All experimental procedures were approved by the institutional animal care and use committee at Mayo Clinic Arizona (IACUC A00005542-20).
Viral strains
LCMV (Armstrong strain) was maintained at −80°C until infection and diluted to 2x106 PFU/ml in PBS. Influenza (PR8 strain) was maintained at −80°C and diluted to 7x104 PFU/ml in PBS (intranasal infection) or 7x107 PFU/ml in PBS (intraperitoneal infection) at the time of infection studies.
Infection studies
Mice were infected with LCMV-Armstrong (2x105 PFU, intraperitoneally or intranasally). Other mice were infected with Influenza-PR8 (100 PFU, intranasally or 1x106 PFU, intraperitoneally).
Flow cytometry
Lymphocytes were isolated from spleen or lungs as previously described (60, 61). Lungs were removed and cut in small pieces into Erlenmeyer flasks containing 30 mL of 0.5 mg/ml Collagenase type IV. During isolation of lymphocytes from lungs, in all experiments, 50 μg of Treg-Protector (anti-ARTC2.2) nanobodies (BioLegend) were injected i.v. 30 minutes prior to mouse sacrifice (62). Direct ex vivo staining was performed as described (60). To identify LCMV-specific or Flu-specific CD8+ T cells, tetramers were obtained from the Yerkes NIH Tetramer Core: Db-gp33 and Db-NP-flu tetramers conjugated with APC- or PE-Streptavidin were used. For detection of vascular-associated lymphocytes in non-lymphoid organs, in vivo i.v. injection of PerCP-Cy5.5-conjugated CD8α antibody was performed (63). Among LCMV- or Flu-specific CD8+ T cells, the following markers were used to distinguish lung TRM cells: i.v.CD8α-CD69+/−CD103hi/int/lo. In all flow cytometry experiments, Live/Dead Near-IR was used to distinguish between live and dead cells. Flow cytometric analyses were performed on FACS Symphony (BD Biosciences) and data was analyzed using FlowJo software (Treestar).
Statistical analyses
Data were subjected to the Kolmogorov-Smirnov test to assess normality of samples. Statistical differences were calculated by using unpaired two-tailed Student’s t-test (or one-way ANOVA with Tukey post-test, where indicated). All experiments were analyzed using Prism 9 (GraphPad Software). Graphical data was shown as mean values with error bars indicating the SD. P values of < 0.05 (*), < 0.01 (**), < 0.001 (***) indicated significant differences between groups.
Data availability statement
The raw data supporting the conclusions of this article will be made available by the authors, without undue reservation.
Ethics statement
The animal study was approved by IACUC, project number A00005542-20. The study was conducted in accordance with the local legislation and institutional requirements.
Author contributions
BM: Conceptualization, Writing – original draft, Writing – review & editing. MM: Data curation, Formal analysis, Investigation, Writing – original draft, Writing – review & editing. HB: Conceptualization, Data curation, Formal analysis, Funding acquisition, Project administration, Resources, Supervision, Writing – original draft, Writing – review & editing.
Funding
The author(s) declare financial support was received for the research, authorship, and/or publication of this article. HB was supported by the National Institutes of Health, National Institute of Allergy and Infectious Diseases (R01AI170649).
Acknowledgments
We thank the Borges da Silva lab for intellectual support.
Conflict of interest
The authors declare that the research was conducted in the absence of any commercial or financial relationships that could be construed as a potential conflict of interest.
Publisher’s note
All claims expressed in this article are solely those of the authors and do not necessarily represent those of their affiliated organizations, or those of the publisher, the editors and the reviewers. Any product that may be evaluated in this article, or claim that may be made by its manufacturer, is not guaranteed or endorsed by the publisher.
References
1. Ruterbusch M, Pruner KB, Shehata L, Pepper M. In Vivo CD4(+) T cell differentiation and function: Revisiting the Th1/Th2 Paradigm. Annu Rev Immunol (2020) 38:705–25. doi: 10.1146/annurev-immunol-103019-085803
2. Williams MA, Bevan MJ. Effector and memory CTL differentiation. Annu Rev Immunol (2007) 25:171–92. doi: 10.1146/annurev.immunol.25.022106.141548
3. Mueller SN, Gebhardt T, Carbone FR, Heath WR. Memory T cell subsets, migration patterns, and tissue residence. Annu Rev Immunol (2013) 31:137–61. doi: 10.1146/annurev-immunol-032712-095954
4. Omilusik KD, Nadjsombati MS, Shaw LA, Yu B, Milner JJ, Goldrath AW. Sustained Id2 regulation of E proteins is required for terminal differentiation of effector CD8(+) T cells. J Exp Med (2018) 215:773–83. doi: 10.1084/jem.20171584
5. Schenkel JM, Masopust D. Tissue-resident memory T cells. Immunity (2014) 41:886–97. doi: 10.1016/j.immuni.2014.12.007
6. Laidlaw BJ, Zhang N, Marshall HD, Staron MM, Guan T, Hu Y, et al. CD4+ T cell help guides formation of CD103+ lung-resident memory CD8+ T cells during influenza viral infection. Immunity (2014) 41:633–45. doi: 10.1016/j.immuni.2014.09.007
7. Swarnalekha N, Schreiner D, Litzler LC, Iftikhar S, Kirchmeier D, Kunzli M, et al. T resident helper cells promote humoral responses in the lung. Sci Immunol (2021) 6(55):eabb6808. doi: 10.1126/sciimmunol.abb6808
8. Hondowicz BD, An D, Schenkel JM, Kim KS, Steach HR, Krishnamurty AT, et al. Interleukin-2-dependent allergen-specific tissue-resident memory cells drive asthma. Immunity (2016) 44:155–66. doi: 10.1016/j.immuni.2015.11.004
9. Masopust D, Vezys V, Marzo AL, Lefrancois L. Preferential localization of effector memory cells in nonlymphoid tissue. Science (2001) 291:2413–7. doi: 10.1126/science.1058867
10. Gebhardt T, Wakim LM, Eidsmo L, Reading PC, Heath WR, Carbone FR. Memory T cells in nonlymphoid tissue that provide enhanced local immunity during infection with herpes simplex virus. Nat Immunol (2009) 10:524–30. doi: 10.1038/ni.1718
11. Wein AN, McMaster SR, Takamura S, Dunbar PR, Cartwright EK, Hayward SL, et al. CXCR6 regulates localization of tissue-resident memory CD8 T cells to the airways. J Exp Med (2019) 216:2748–62. doi: 10.1084/jem.20181308
12. Sakai S, Kauffman KD, Schenkel JM, McBerry CC, Mayer-Barber KD, Masopust D, et al. Cutting edge: control of Mycobacterium tuberculosis infection by a subset of lung parenchyma-homing CD4 T cells. J Immunol (2014) 192:2965–9. doi: 10.4049/jimmunol.1400019
13. Slutter B, Van Braeckel-Budimir N, Abboud G, Varga SM, Salek-Ardakani S, Harty JT. Dynamics of influenza-induced lung-resident memory T cells underlie waning heterosubtypic immunity. Sci Immunol (2017) 2(7):eaag2031. doi: 10.1126/sciimmunol.aag2031
14. Takamura S, Yagi H, Hakata Y, Motozono C, McMaster SR, Masumoto T, et al. Specific niches for lung-resident memory CD8+ T cells at the site of tissue regeneration enable CD69-independent maintenance. J Exp Med (2016) 213:3057–73. doi: 10.1084/jem.20160938
15. Son YM, Cheon IS, Wu Y, Li C, Wang Z, Gao X, et al. Tissue-resident CD4(+) T helper cells assist the development of protective respiratory B and CD8(+) T cell memory responses. Sci Immunol (2021) 6(55):eabb6852. doi: 10.1126/sciimmunol.abb6852
16. Kok L, Masopust D, Schumacher TN. The precursors of CD8(+) tissue resident memory T cells: From lymphoid organs to infected tissues. Nat Rev Immunol (2022) 22:283–93. doi: 10.1038/s41577-021-00590-3
17. Mueller SN, Mackay LK. Tissue-resident memory T cells: local specialists in immune defence. Nat Rev Immunol (2016) 16:79–89. doi: 10.1038/nri.2015.3
18. Evrard M, Wynne-Jones E, Peng C, Kato Y, Christo SN, Fonseca R, et al. Sphingosine 1-phosphate receptor 5 (S1PR5) regulates the peripheral retention of tissue-resident lymphocytes. J Exp Med (2022) 219(1):e20210116. doi: 10.1084/jem.20210116
19. Skon CN, Lee JY, Anderson KG, Masopust D, Hogquist KA, Jameson SC. Transcriptional downregulation of S1pr1 is required for the establishment of resident memory CD8+ T cells. Nat Immunol (2013) 14:1285–93. doi: 10.1038/ni.2745
20. Seung E, Cho JL, Sparwasser T, Medoff BD, Luster AD. Inhibiting CXCR3-dependent CD8+ T cell trafficking enhances tolerance induction in a mouse model of lung rejection. J Immunol (2011) 186:6830–8. doi: 10.4049/jimmunol.1001049
21. Thwaites RS, Uruchurtu ASS, Negri VA, Cole ME, Singh N, Poshai N, et al. Early mucosal events promote distinct mucosal and systemic antibody responses to live attenuated influenza vaccine. Nat Commun (2023) 14:8053. doi: 10.1038/s41467-023-43842-7
22. Uddback I, Cartwright EK, Scholler AS, Wein AN, Hayward SL, Lobby J, et al. Long-term maintenance of lung resident memory T cells is mediated by persistent antigen. Mucosal Immunol (2021) 14:92–9. doi: 10.1038/s41385-020-0309-3
23. Perdomo C, Zedler U, Kuhl AA, Lozza L, Saikali P, Sander LE, et al. Mucosal BCG vaccination induces protective lung-resident memory T cell populations against tuberculosis. mBio (2016) 7(6):e01686-16. doi: 10.1128/mBio.01686-16
24. Armitage E, Quan D, Florido M, Palendira U, Triccas JA, Britton WJ. CXCR3 provides a competitive advantage for retention of mycobacterium tuberculosis-specific tissue-resident memory T cells following a mucosal tuberculosis vaccine. Vaccines (Basel) (2023) 11(10):1549. doi: 10.3390/vaccines11101549
25. Ogongo P, Tezera LB, Ardain A, Nhamoyebonde S, Ramsuran D, Singh A, et al. Tissue-resident-like CD4+ T cells secreting IL-17 control Mycobacterium tuberculosis in the human lung. J Clin Invest (2021) 131(10):e142014. doi: 10.1172/JCI142014
26. Ogongo P, Porterfield JZ, Leslie A. Lung tissue resident memory T-cells in the immune response to mycobacterium tuberculosis. Front Immunol (2019) 10:992. doi: 10.3389/fimmu.2019.00992
27. Zens KD, Chen JK, Farber DL. Vaccine-generated lung tissue-resident memory T cells provide heterosubtypic protection to influenza infection. JCI Insight (2016) 1(10):e85832. doi: 10.1172/jci.insight.85832
28. McMaster SR, Wein AN, Dunbar PR, Hayward SL, Cartwright EK, Denning TL, et al. Pulmonary antigen encounter regulates the establishment of tissue-resident CD8 memory T cells in the lung airways and parenchyma. Mucosal Immunol (2018) 11:1071–8. doi: 10.1038/s41385-018-0003-x
29. Basile JI, Liu R, Mou W, Gao Y, Carow B, Rottenberg ME. Mycobacteria-specific T cells are generated in the lung during mucosal BCG immunization or infection with mycobacterium tuberculosis. Front Immunol (2020) 11:566319. doi: 10.3389/fimmu.2020.566319
30. Wang W, Cohen JA, Wallrapp A, Trieu KG, Barrios J, Shao F, et al. Age-related dopaminergic innervation augments T helper 2-type allergic inflammation in the postnatal lung. Immunity (2019) 51:1102–1118.e7. doi: 10.1016/j.immuni.2019.10.002
31. Si Y, Wang Y, Tian Q, Wang Q, Pollard JM, Srivastava PK, et al. Lung cDC1 and cDC2 dendritic cells priming naive CD8(+) T cells in situ prior to migration to draining lymph nodes. Cell Rep (2023) 42:113299. doi: 10.1016/j.celrep.2023.113299
32. Jenkins MM, Bachus H, Botta D, Schultz MD, Rosenberg AF, Leon B, et al. Lung dendritic cells migrate to the spleen to prime long-lived TCF1(hi) memory CD8(+) T cell precursors after influenza infection. Sci Immunol (2021) 6:eabg6895. doi: 10.1126/sciimmunol.abg6895
33. Mettelman RC, Allen EK, Thomas PG. Mucosal immune responses to infection and vaccination in the respiratory tract. Immunity (2022) 55:749–80. doi: 10.1016/j.immuni.2022.04.013
34. Li Z, Jackson RJ, Ranasinghe C. Vaccination route can significantly alter the innate lymphoid cell subsets: a feedback between IL-13 and IFN-gamma. NPJ Vaccines (2018) 3:10. doi: 10.1038/s41541-018-0048-6
35. Moldoveanu B, Otmishi P, Jani P, Walker J, Sarmiento X, Guardiola J, et al. Inflammatory mechanisms in the lung. J Inflammation Res (2009) 2:1–11.
36. Enamorado M, Khouili SC, Iborra S, Sancho D. Genealogy, dendritic cell priming, and differentiation of tissue-resident memory CD8(+) T cells. Front Immunol (2018) 9:1751. doi: 10.3389/fimmu.2018.01751
37. Dudda JC, Martin SF. Tissue targeting of T cells by DCs and microenvironments. Trends Immunol (2004) 25:417–21. doi: 10.1016/j.it.2004.05.008
38. Mani V, Bromley SK, Aijo T, Mora-Buch R, Carrizosa E, Warner RD, et al. Migratory DCs activate TGF-beta to precondition naive CD8(+) T cells for tissue-resident memory fate. Science (2019) 366(6462):eaav5728. doi: 10.1126/science.aav5728
39. Thompson EA, Darrah PA, Foulds KE, Hoffer E, Caffrey-Carr A, Norenstedt S, et al. Monocytes acquire the ability to prime tissue-resident T cells via IL-10-mediated TGF-beta release. Cell Rep (2019) 28:1127–1135.e4. doi: 10.1016/j.celrep.2019.06.087
40. Knight FC, Wilson JT. Engineering vaccines for tissue-resident memory T cells. Adv Ther (Weinh) (2021) 4(4):2000230. doi: 10.1002/adtp.202000230
41. Hassan AO, Kafai NM, Dmitriev IP, Fox JM, Smith BK, Harvey IB, et al. and lower respiratory tracts against SARS-CoV-2. Cell (2020) 183:169–184.e13. doi: 10.1016/j.cell.2020.08.026
42. Nelson SA, Dileepan T, Rasley A, Jenkins MK, Fischer NO, Sant AJ. Intranasal nanoparticle vaccination elicits a persistent, polyfunctional CD4 T cell response in the murine lung specific for a highly conserved influenza virus antigen that is sufficient to mediate protection from influenza virus challenge. J Virol (2021) 95:e0084121. doi: 10.1128/JVI.00841-21
43. Hartwell BL, Melo MB, Xiao P, Lemnios AA, Li N, Chang JYH, et al. Intranasal vaccination with lipid-conjugated immunogens promotes antigen transmucosal uptake to drive mucosal and systemic immunity. Sci Transl Med (2022) 14:eabn1413. doi: 10.1126/scitranslmed.abn1413
44. Spearman P, Jin H, Knopp K, Xiao P, Gingerich MC, Kidd J, et al. Intranasal parainfluenza virus type 5 (PIV5)-vectored RSV vaccine is safe and immunogenic in healthy adults in a phase 1 clinical study. Sci Adv (2023) 9:eadj7611. doi: 10.1126/sciadv.adj7611
45. Lycke N. Recent progress in mucosal vaccine development: potential and limitations. Nat Rev Immunol (2012) 12:592–605. doi: 10.1038/nri3251
46. Li M, Wang Y, Sun Y, Cui H, Zhu SJ, Qiu HJ. Mucosal vaccines: Strategies and challenges. Immunol Lett (2020) 217:116–25. doi: 10.1016/j.imlet.2019.10.013
47. Lobaina Mato Y. Nasal route for vaccine and drug delivery: Features and current opportunities. Int J Pharm (2019) 572:118813. doi: 10.1016/j.ijpharm.2019.118813
48. Kunzli M, O'Flanagan SD, LaRue M, Talukder P, Dileepan T, Stolley JM, et al. Route of self-amplifying mRNA vaccination modulates the establishment of pulmonary resident memory CD8 and CD4 T cells. Sci Immunol (2022) 7:eadd3075. doi: 10.1126/sciimmunol.add3075
49. Kong HJ, Choi Y, Kim EA, Chang J. Vaccine strategy that enhances the protective efficacy of systemic immunization by establishing lung-resident memory CD8 T cells against influenza infection. Immune Netw (2023) 23:e32. doi: 10.4110/in.2023.23.e32
50. Corbett KS, Edwards DK, Leist SR, Abiona OM, Boyoglu-Barnum S, Gillespie RA, et al. SARS-CoV-2 mRNA vaccine design enabled by prototype pathogen preparedness. Nature (2020) 586:567–71. doi: 10.1038/s41586-020-2622-0
51. McMahon M, O'Dell G, Tan J, Sarkozy A, Vadovics M, Carreno JM, et al. Assessment of a quadrivalent nucleoside-modified mRNA vaccine that protects against group 2 influenza viruses. Proc Natl Acad Sci U.S.A. (2022) 119:e2206333119. doi: 10.1073/pnas.2206333119
52. Frost EL, Kersh AE, Evavold BD, Lukacher AE. Cutting edge: Resident memory CD8 T cells express high-affinity TCRs. J Immunol (2015) 195:3520–4. doi: 10.4049/jimmunol.1501521
53. Malloy AMW, Lu Z, Kehl M, Pena DaMata J, Lau-Kilby AW, Turfkruyer M. Increased innate immune activation induces protective RSV-specific lung-resident memory T cells in neonatal mice. Mucosal Immunol (2023) 16:593–605. doi: 10.1016/j.mucimm.2023.05.012
54. Wu T, Hu Y, Lee YT, Bouchard KR, Benechet A, Khanna K, et al. Lung-resident memory CD8 T cells (TRM) are indispensable for optimal cross-protection against pulmonary virus infection. J Leukoc Biol (2014) 95:215–24. doi: 10.1189/jlb.0313180
55. Zhang N, Bevan MJ. Transforming growth factor-beta signaling controls the formation and maintenance of gut-resident memory T cells by regulating migration and retention. Immunity (2013) 39:687–96. doi: 10.1016/j.immuni.2013.08.019
56. Mohammed J, Beura LK, Bobr A, Astry B, Chicoine B, Kashem SW, et al. Stromal cells control the epithelial residence of DCs and memory T cells by regulated activation of TGF-beta. Nat Immunol (2016) 17:414–21. doi: 10.1038/ni.3396
57. Davies B, Prier JE, Jones CM, Gebhardt T, Carbone FR, Mackay LK. Cutting edge: Tissue-resident memory T cells generated by multiple immunizations or localized deposition provide enhanced immunity. J Immunol (2017) 198:2233–7. doi: 10.4049/jimmunol.1601367
58. Shin H, Iwasaki A. A vaccine strategy that protects against genital herpes by establishing local memory T cells. Nature (2012) 491:463–7. doi: 10.1038/nature11522
59. Fonseca R, Burn TN, Gandolfo LC, Devi S, Park SL, Obers A, et al. Runx3 drives a CD8(+) T cell tissue residency program that is absent in CD4(+) T cells. Nat Immunol (2022) 23:1236–45. doi: 10.1038/s41590-022-01273-4
60. Borges da Silva H, Beura LK, Wang H, Hanse EA, Gore R, Scott MC, et al. The purinergic receptor P2RX7 directs metabolic fitness of long-lived memory CD8(+) T cells. Nature (2018) 559:264–8. doi: 10.1038/s41586-018-0282-0
61. Santiago-Carvalho I, Almeida-Santos G, Macedo BG, Barbosa-Bomfim CC, Almeida FM, Pinheiro Cione MV, et al. T cell-specific P2RX7 favors lung parenchymal CD4(+) T cell accumulation in response to severe lung infections. Cell Rep (2023) 42:113448. doi: 10.1016/j.celrep.2023.113448
62. Borges da Silva H, Wang H, Qian LJ, Hogquist KA, Jameson SC. ARTC2.2/P2RX7 signaling during cell isolation distorts function and quantification of tissue-resident CD8(+) T cell and invariant NKT subsets. J Immunol (2019) 202:2153–63. doi: 10.4049/jimmunol.1801613
Keywords: lung, tissue-resident memory CD8(+) T cells, tissue-resident memory CD4(+) T cells, T cells, route of immunization, antigen tropism
Citation: Macedo BG, Masuda MY and Borges da Silva H (2024) Location versus ID: what matters to lung-resident memory T cells? Front. Immunol. 15:1355910. doi: 10.3389/fimmu.2024.1355910
Received: 14 December 2023; Accepted: 16 January 2024;
Published: 05 February 2024.
Edited by:
Lalit K. Beura, Brown University, United StatesReviewed by:
Kyra Zens, University of Zurich, SwitzerlandCopyright © 2024 Macedo, Masuda and Borges da Silva. This is an open-access article distributed under the terms of the Creative Commons Attribution License (CC BY). The use, distribution or reproduction in other forums is permitted, provided the original author(s) and the copyright owner(s) are credited and that the original publication in this journal is cited, in accordance with accepted academic practice. No use, distribution or reproduction is permitted which does not comply with these terms.
*Correspondence: Henrique Borges da Silva, borgesdasilva.henrique@mayo.edu