- 1Centre for Molecular Therapeutics, Australian Institute of Tropical Health and Medicine, James Cook University, Cairns, QLD, Australia
- 2College of Medicine and Dentistry, James Cook University, Cairns, QLD, Australia
- 3Institute for Molecular Bioscience, The University of Queensland, St Lucia, QLD, Australia
The collection, cryopreservation, thawing, and culture of peripheral blood mononuclear cells (PBMCs) can profoundly influence T cell viability and immunogenicity. Gold-standard PBMC processing protocols have been developed by the Office of HIV/AIDS Network Coordination (HANC); however, these protocols are not universally observed. Herein, we have explored the current literature assessing how technical variation during PBMC processing can influence cellular viability and T cell immunogenicity, noting inconsistent findings between many of these studies. Amid the mounting concerns over scientific replicability, there is growing acknowledgement that improved methodological rigour and transparent reporting is required to facilitate independent reproducibility. This review highlights that in human T cell studies, this entails adopting stringent standardised operating procedures (SOPs) for PBMC processing. We specifically propose the use of HANC’s Cross-Network PBMC Processing SOP, when collecting and cryopreserving PBMCs, and the HANC member network International Maternal Pediatric Adolescent AIDS Clinical Trials (IMPAACT) PBMC Thawing SOP when thawing PBMCs. These stringent and detailed protocols include comprehensive reporting procedures to document unavoidable technical variations, such as delayed processing times. Additionally, we make further standardisation and reporting recommendations to minimise and document variability during this critical experimental period. This review provides a detailed overview of the challenges inherent to a procedure often considered routine, highlighting the importance of carefully considering each aspect of SOPs for PBMC collection, cryopreservation, thawing, and culture to ensure accurate interpretation and comparison between studies.
Introduction
T-lymphocytes (T cells) are integral components of adaptive immunity (1), and are essential for clearing infections (2), responding to vaccinations (3) or emergent tumorigenesis (4), and maintaining immune system homeostasis (5). Given these broad and critical effector functions, T cells have been the focus of intensive research which predominantly aims to: i) characterise and compartmentalise T cell phenotypes (6, 7), and ii) understand and modulate T cell immunogenicity (8, 9). T cells can be broadly characterised as either CD4+ Helper T cells, or CD8+ cytotoxic T cells (1). CD4+ T cells are crucial for regulating the immune response by releasing signalling molecules that activate, modulate, or direct other immune cells against a particular pathogen (3, 5). Cytotoxic CD8+ T Cells are primarily responsible for killing dangerous self-cells, such as those infected with intracellular pathogens or cells undergoing tumorigenesis (3, 4). Both CD4+ and CD8+ T cell immunogenicity is commonly studied through functional immunoassays that investigate the activation and behaviour of T cells in response to specific stimuli (10). These experiments generally involve the in-vitro stimulation of peripheral blood mononuclear cells (PBMCs) with antigenic peptide epitopes or non-specific mitogens. This mimics the in-vivo activation of T cells, which initiates various responses including cytokine production (11), proliferation (12), or apoptosis (13). Optimal assessment of T cell phenotypes and immunogenicity requires PBMCs that are viable, and which retain their natural in-vivo immunogenic capabilities.
The in-vitro loss of PBMC viability or in-vivo T cell immunogenicity can critically impact immunological research and clinical trials (14, 15). A notable example is the differential response of T cells when stimulated either in-vitro or in-vivo with the CD28 agonist antibody TGN1412 (16). In 2006, a phase I clinical study triggered life threatening cytokine release syndrome in patients infused with TGN1412 (17). Further studies revealed that T-cell activation by TGN1412 was dependent upon co-stimulatory signals (18), which were restored to in-vivo conditions in-vitro when PBMCs were pre-cultured (rested) in high densities (14, 19). More broadly, persistent controversies in T cell research, such as inconsistencies between vaccine antigen testing and vaccine clinical trial immunogenicity (20–22), disagreements on immunodominant antigen or epitopes (23, 24), or other debated aspects of immunological responses (25), may be partly explainable by technical variation during PBMC processing.
PBMC processing involves collecting, storing, thawing, and culturing PBMCs from human donors (26). This protocol typically involves: i) collecting peripheral blood using venepuncture, ii) separating PBMCs from other blood components, iii) immediately experimenting on, or cryopreserving the cells, iv) thawing cryopreserved cells, and v) in-vitro cell culture. Following the outbreak of the HIV/AIDS epidemic there was a heightened need for a globally coordinated T cell clinical trial network with a standardised PBMC processing protocol (27, 28). In response, the Office of HIV/AIDS Network Coordination (HANC) established gold-standard PBMC processing standard operating procedures (SOPs), which were widely, but not universally adopted (29, 30). The current SOPs include the HANC’s Cross-Network PBMC Processing SOP, for collecting and cryopreserving PBMCs (31), and the HANC member network International Maternal Pediatric Adolescent AIDS Clinical Trials (IMPAACT) PBMC Thawing SOP when thawing PBMCs (32). This review will demonstrate even relatively minor deviations from these SOPs can have profound consequences for PBMC viability and T cell immunogenicity, and indeed that many studies investigating the effect of technical variation during PBMC processing have reported contradictory results (Supplementary Table 1). The objective of this review is to briefly discuss the key research that has demonstrated technical variation during this process can profoundly influence cellular viability and immunogenicity (Figure 1), highlight the challenges of comparing immunogenicity across samples exposed to variant protocols, and emphasize the need for stringent protocol standardization.
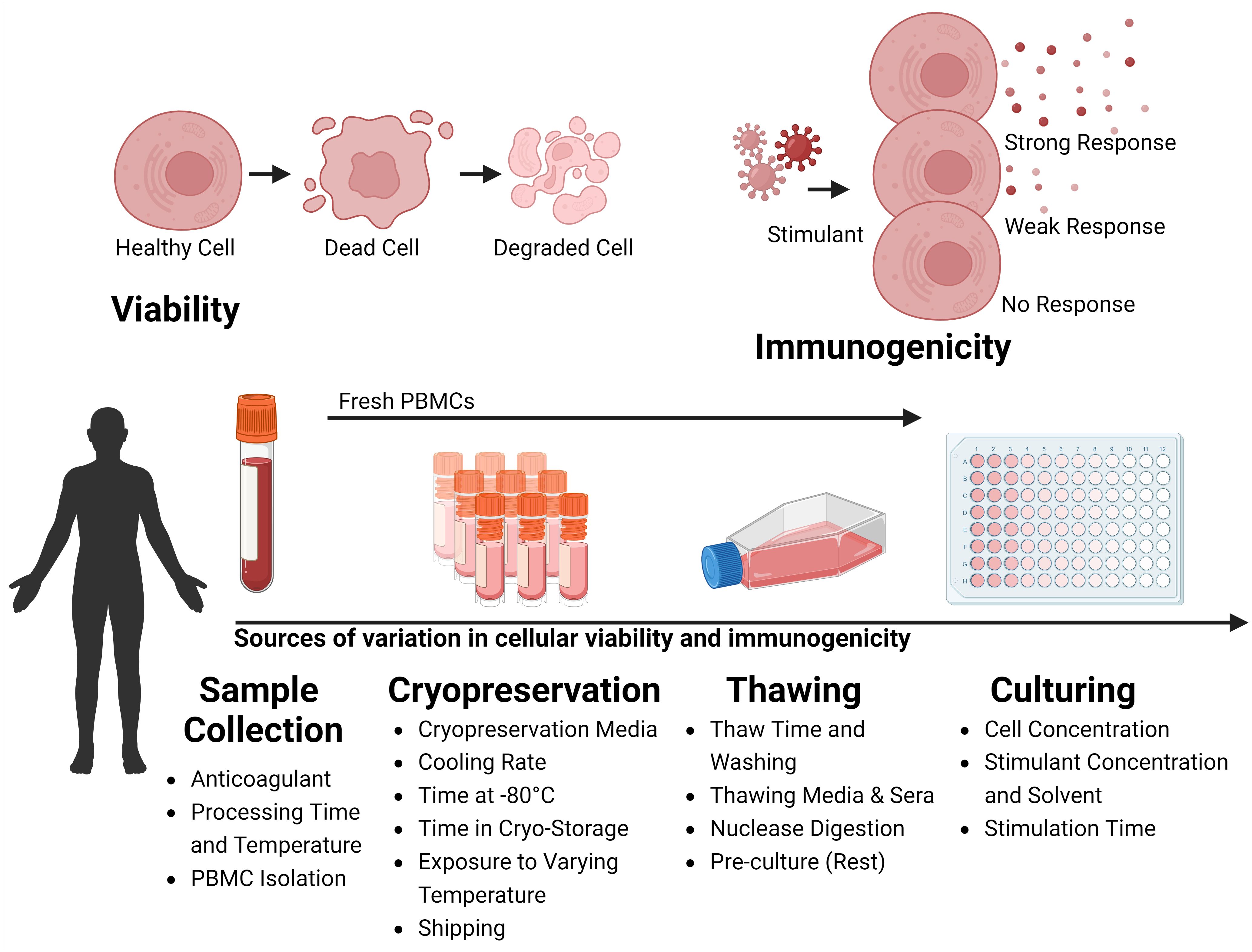
Figure 1 Variability Factors in Cellular Viability and Immunogenicity in Sample Processing. The process of collection, storage, thawing, and culturing cells collected from the peripheral blood of human donors can influence the viability and the immunogenicity of the sample. During Sample Collection, variability arises from the type of anticoagulant used, the time and temperature of processing, and the strategy for isolating Peripheral Blood Mononuclear Cells (PBMCs). During Cryopreservation variability is influenced by the choice of cryopreservation media, cooling rate, duration at -80°C before long-term storage, storage duration, temperature fluctuations during storage, and conditions during shipping. During Thawing, factors include thawing time, wash strategies, thawing media and sera, nuclease digestion, and whether cells are rested before experiments. During Culturing, the cell concentration, stimulant dose, and duration of stimulation affect outcomes. Viability is marked by a transition from healthy to dead cells, increasing cellular debris. Immunogenicity, is the ability of cells to react to stimulants like antigenic peptides or mitogens, is typically assessed through functional immunoassays measuring the immune response via the production of surrogate markers of immunity. Created with BioRender.com.
Sources of variation in cellular viability and immunogenicity
Sample collection
Choice of anticoagulant
When collecting peripheral blood for PBMC isolation, clinically-convenient anticoagulant-lined vacuum-tubes are commonly used for venepuncture. Different anticoagulants, typically Ethylenediaminetetraacetic acid (EDTA), heparin, or citrate, serve specific purposes and have advantages and disadvantages relative to others (Supplementary Table 2) (33). According to the HANC-SOP, it is mandatory to document the type of anticoagulant used for each sample (31). Use of EDTA rather than heparin has been linked to diminished immunogenicity following PBMC stimulation (34). Conversely, other studies have found no significant change of functionality between EDTA and sodium-heparin isolated PBMCs (35), nor between sodium-heparin and lithium-heparin collection (36). Anticoagulant has been shown to not be associated with PBMC viability (35), however this study did note viability was statistically associated with anticoagulant when the cryopreservation of PBMCs was delayed. Taken together, these studies report a potential connection between anticoagulant and PBMC viability and functionality and highlight the first potential technical pitfall when studying T cells: the absence of standardised or intentional anticoagulant selection.
Processing time and temperature
It is generally accepted the post-venepuncture processing time and temperature are critical parameters affecting cellular viability and T cell immunogenicity (35, 37). However, PBMCs are routinely isolated from peripheral blood well beyond 24 hours after venepuncture, especially in clinical trials (26, 38). The HANC-SOP recommends that processing time should not exceed 8 hours (31). Processing delays of 24 hours or more have been associated with reduced cell viability (35), and ambient temperatures less than 22°C reduced PBMC viability and immunogenicity (39). Nucleic acid recovery from whole blood was profoundly reduced following exposure to suboptimal processing times and temperatures (40). However, these results are challenged by another study which found a 24-hour delay in blood sample processing did not affect the viability of PBMCs, nor the amount of mitogen-induced protein secretion (37). Although conflicting studies exist, discrepancies in the timing and temperature conditions of PBMC processing clearly pose a significant challenge for T cell research. The HANC-SOP requires the collection, processing, and freezing date and time to be documented (31). We propose the ambient temperature should also be reported.
PBMC isolation
PBMCs are typically isolated from peripheral blood using density-gradient centrifugation methods, such as Ficoll-Paque, or in clinically-convenient cell preparation tubes (CPTs), including SepMate™ and Vacutainer® CPTs (41). The HANC-SOP requires the isolation method and processing technician to be documented (31). Ficoll-processed PBMCs were found to have higher viability when compared to CPT-processed PBMCs (42). Another study reported that differences in viability due to isolation method were detected in one laboratory but not another (43). In contrast, others have reported no significant differences in cell viability and recovery when isolating PBMCs with either Ficoll-Paque or CPTs (35, 44). Immunogenicity has also been associated with isolation method, as PBMCs isolated using Ficoll-Paque were found to secrete lower levels of the cytokine Interferon-gamma (IFN-γ) compared to those isolated with CPTs (41). However, transcriptomic profiles were not found to be influenced by isolation method (44). These results may be confounded by technician experience, which has been estimated to contribute to approximately 60% of the variability of cell recovery (41). The findings of these studies suggest that standardising PBMC isolation procedures and technician training is likely to enhance the reproducibility and reliability of T cell research.
Cryopreservation
Fresh PBMCs
PBMCs are used in immunoassays either immediately (fresh) or following cryopreservation. Cryopreservation can profoundly influence the viability and immunogenicity of T cells. Indeed, the kinetics of cytokine expression, proliferation, cell viability, and immunophenotypes were demonstrated to differ between freshly isolated and matched cryopreserved PBMCs (45). However, many studies have demonstrated minimal post-cryopreservation differences. A multi-site study across nine laboratories was able to recover similar PBMC numbers following cryopreservation without significant loss of viability (46), while others have demonstrated full functionality of cryopreserved T cells (47). Nevertheless, results from these single studies have not significantly influenced typical PBMC cryopreservation protocols, and reporting the status of cells, whether fresh or cryopreserved, is generally expected.
Cryopreservation media
The first stage of cryopreservation is typically to suspend PBMCs in a cryoprotective agent, such as dimethylsulfoxide (DMSO) (48). The HANC-SOP specifies PBMCs should be gently resuspended to 107/mL in a 10% DMSO 90% Foetal Calf Serum (FCS) cryopreservation media cooled to 2 to 8°C with continuous swirling (31). The concentration of DMSO has been found to be usually the most important factor determining cellular viability (49) and is generally 10% (50, 51). However, one study has reported PBMC recovery was significantly improved when using 5% DMSO (52). A cell concentration greater than 6x106 PBMC/mL has been associated with improved viability (53) and sera in the cryopreservation media has been found to improve viability (54) and immunogenicity (55). Sera is typically either FCS or ‘normal’ human AB serum, and although human sera may be more physiologically relevant (56), one study reported the use of FCS improved human PBMC viability (55), while another reported no significant difference between the two (57). Other studies have reported only a minimal cell viability (58), or immunogenicity (35, 58) improvement when supplementing cryopreservation media with sera, or noted heightened background immunoreactivity when supplementing with bovine serum albumin (BSA) (57). Gradually resuspending PBMCs in cooled cryopreservation media, such as by a drip-wise method, may improve PBMC viability and immunogenicity by minimising toxic shock or cell membrane damage (48, 59). However, the impact of cryoprotectant addition rate on PBMC viability has not been specifically contrasted, unlike in spermatozoa cryopreservation studies, which have yielded inconsistent findings (60, 61). Cooling the cryopreservation media to 4°C has been associated with preserving T cell immunogenicity (62), although others have shown that cooling temperature did not show any significant effects (49). Taken together, these studies demonstrate that cryopreservation media can significantly influence T cell viability and immunogenicity.
Cooling rate
Once cells are suspended in the cryopreservation media, they must be cooled to their storage temperature, generally aiming to reduce cell temperature by -1°C min-1. Cryopreservation of highly concentrated PBMCS using an automated controlled-rate freezer is the gold-standard, having been found to enhance T cell activation (63). However, typically, cryopreservation is achieved with two-stage cooling, where cells are cooled in ultra-low freezers (ULFs) to -80°C, then in vapor phase liquid nitrogen (LN2) to below -150°C in commercial freezing containers, such as a Mr. Frosty™. The HANC-SOP requires samples to be immediately frozen in commercial controlled rate freezing containers, first in ULF then LN2 to reduce temperature by -1°C min-1 (31). Strategies have been developed which avoid cell viability loss during rapid freezing, such as media ice seeding (nucleation) which allowed PBMCs to be cooled at -90°C min-1 (64). Another study found cooling rate did not influence viability, as long as thawing rates were high (113°C min-1 and 45°C min-1) (65). Others have reported no change in cell viability when cells were initially cooled in LN2 (66). Despite these reports from single studies, cooling rate is generally accepted to significantly influence T cell viability and immunogenicity. We propose that the freezing container brand should be recorded.
Time at -80°C
Cells may be left briefly in ULFs before long-term storage in LN2. The HANC-SOP requires samples to be transferred to LN2 within 72 hours of freezing (31). Studies have demonstrated storage at -80°C can influence gene expression (67), and significantly reduce PBMC viability and immunogenicity (68). Others have demonstrated a linear relationship between decreasing viability and time on dry ice (-70°C) over 12 weeks (69). Another study found that viability had been lost with as little as 48 hours of storage at -80°C (55). Conversely, others found PBMCs stored on dry ice for three weeks had no significant difference in viability or immunogenicity compared to those immediately cryo-stored (70), while others have reported storing PBMCs on dry ice for three weeks did not reduce the T cell immunogenicity (35). Although some studies found relatively short-term storage at -80°C had a minimal influence on PBMC viability and immunogenicity, the consensus on its potential effect highlights the need to record and standardise the date and time of transfer to LN2.
Time in cryo-storage
Cells stored in liquid nitrogen can remain viable and functional for very long periods of time and the HANC-SOP states cells may be stored in LN2 indefinitely (31). Several studies which directly investigated cryo-storage viability found no clinically significant variation in cellular viability over 15 months (70), or viability and hematopoietic stem cell populations over 60 months (71). However, immunogenicity may be influenced by small but statistically significant variations in the populations of lymphocytes, which have been found to vary following 3-to-6-months of cryo-storage (72, 73). Despite a limited number of studies which have demonstrated an impact of LN2 storage duration on PBMC viability or immunogenicity, we nevertheless propose the length of time in cryo-storage should be reported.
Exposure to varying temperatures
During long-term cryo-storage, cells are often transiently exposed to briefly varying temperatures as other co-stored aliquots are added or removed from the facility. One study found reduced cell viability and immunogenicity when cyclically exposing cryo-stored PBMCs briefly to room temperature (74). However, another study found viability was not influenced when aliquots of PBMCs went through repeated rounds of temperature cycling (75), while another study investigating gene expression profiles found no significant change of PBMC gene expression following brief but repetitive temperature cycling (67). The HANC-SOP states PBMCs are not to be transferred back to ULF storage (31). Additional studies are required to precisely assess the impact of brief but potentially repetitive temperature fluctuations on the viability and immunogenicity of cryopreserved PBMCs.
Shipping
The gold-standard practise to ship cryo-stored PBMCs is with LN2-dry shippers, however, dry ice is also used. The HANC-SOP requires PBMCs stored in LN2 to be shipped in cold-shippers which maintain LN2 temperatures (31). Both viability and immunogenicity were influenced by cold-shipping strategy (69). However, one study found that the shipping method influenced viability, but did not influence immunogenicity (35), while another found that viability was not influenced but lymphocyte populations (i.e., the ratio of CD4+/CD8+ T cells) were affected (76). It is unclear why one population of T cell would be more sensitive to shipping than another. Significant temperature changes during shipping certainly will impact PBMC viability and immunogenicity. However, similarly to the potential fluctuations which can occur during long-term cryo-storage, exposure to varying temperatures during shipping may be brief but recurrent, and more research is required on the effects of smaller or repeated fluctuations.
Thawing
Thaw time and washing
The IMPAACT-SOP calls for cells to be thawed rapidly at 37°C, then added to the thawing media in a drip-wise action (32). When returning cryo-preserved cells to physiological conditions PBMC viability and immunogenicity has been improved with rapid thawing (65, 77), and therefore, rapid thawing is widely recognized as the preferred method to thaw cryo-preserved cells. However, this effect has been found to be minimal in a single study when the cells were cooled at the rate required by the HANC-SOP (-1°C min−1) (65). Once cells are thawed, they are routinely immediately washed to minimise contact with the cryoprotectant. However, one study has controversially reported no change in cell viability following either immediate washing or leaving in the water bath for five minutes (78). Cells and washing medium may be combined by swiftly diluting the cells into the thawing media or by adding in a drip-wise action. No difference in the absolute count of live PBMCs was reported when cells were added rapidly (78). While further studies may identify protocols with more refined thawing rates and washing steps, standardising the current SOP would likely improve the reproducibility and reliability of T cell research.
Thawing media & sera
The media used during the thawing process can include various salt-balanced and buffered solutions such as phosphate buffered saline (PBS) or culture media, typically RPMI 1640 medium (RPMI) supplemented with additives including FCS. Sera can vary significantly between batches (79), and the HANC group reserve lots of FCS for batched experiments (32). Several studies investigating various combinations of washing medias have found PBMC viability was highest following washing with media including sera (55, 78), and noted improved cell viability (55, 78), and functionality (80), when thawing with media at a temperature of 37°C rather than 4°C. No significant difference in viability was observed when thawing cells in either 50mL or 15mL of thawing media (55). Collectively, these studies indicate that both thawing media and sera significantly affect T cell viability and immunogenicity, underscoring the importance of standardizing these components to the greatest extent feasible. The IMPAACT-SOP requires the use of 10% approved lot FCS in RPMI (32), we furthermore recommend the media is warmed to 37°C. The optimal sera for human T cell assays are human sera, which although impractical, would ideally be autologously matched (26). FCS is a popular alternative, especially in clinical trials due to its greater consistency, scalability, and cost efficiency.
Nuclease digestion
To prevent cell clumping due to nucleic material released from lysed cells, post-thawed and washed cells may be incubated with a nuclease. The use of a DNase endonuclease was reported to have little effect on cell morphology, function, or viability (81). However, a flow cytometry-based study found changes in cell populations with varying forward and side scatter profiles following DNase treatment (82), but no detectable changes in cell viability, expression of standard lymphocyte surface markers, nor intracellular cytokine expression. Benzonase is another commonly used nuclease which allows efficient degradation of all types of DNA and RNA. The use of Benzonase® during PBMC processing has been reported to not influence T cell immunogenicity (83). The IMPAACT-SOP includes the optional inclusion of Benzonase® during the first wash (32), as the enzyme’s properties suggest that it could potentially influence PCR outcomes (84). Further research will be required to provide clearer guidelines for the use of nuclease digestion; however, as no study has demonstrated Benzonase® influences PCR outcomes when used during PBMC thawing, we therefore recommend the routine inclusion of Benzonase®.
Pre-culture (rest)
Post-thawing, cells can be experimented upon immediately or undergo preculture (or resting). The IMPAACT-SOP includes an optional rest in culture for 14-18 hours (32). Overnight resting has been found to be optimal to increase the immunogenicity of PBMCs (85), while even 1 hour of pre-culture can replicate the surface marker expression of fresh cells (86). However, others have reported preculture had no statistically significant influence on PBMC immunogenicity (87). The concentration of cells during the rest period has also been found to influence cellular immunoreactivity. Short term pre-culture of PBMCs at high concentrations has been found to improve immunogenicity (19), and others reported the immunogenic response varied relative to PBMC preculture concentration (10). Longer term incubation, upwards of 48 hours in extremely high densities (1×107 cells/mL), has been reported to greatly improve the immunogenicity of CD8+ T cell responses (14), without influencing viability. The results from these combined studies demonstrate that pre-culture conditions, especially cellular density, can significantly influence T cell immunogenicity, and therefore, we propose that the rest period and cell density should be standardised and recorded.
Cell culture
Cell concentration
During functional immunoassays cell density is typically between 1-4×106 cells/mL to facilitate inter-cellular contact and antigen presentation, which is a concentration not expected to influence cell viability (10, 88). The immunogenic response of PBMCs is profoundly influenced by the concentration of cells in the stimulation reaction, particularly when stimulating with weakly immunoreactive antigenic peptides (10). More highly reactive stimulants are also influenced by cell concentration, with one study determining a PBMC concentration of 2.5×106 cells/mL was optimal to detect cytokine responses following mitogen stimulation (89). There are, however, a relatively limited number of studies which have optimised stimulation cell concentrations. Such optimisation may be impractical, particularly when considering experiments involving antigenic T cell peptide epitopes which may involve thousands of stimulatory conditions. Nevertheless, standardising and reporting cell density during culture is generally expected.
Stimulant concentration and solvent
Stimulant concentration is a critical determinant of T-cell functionality. For example, increasing the concentration of antigen is recognised to generally increase the number of IFN-γ+ PBMCs (14). Titrated antigen experiments have identified ranges between 1 μg/ml (90) to 10 μg/ml (36) as optimal for MHC-class I peptide epitope-induced immunogenicity. However, a donor-specific effect on the optimal antigen stimulant concentration to induce immunoreactivity has been described (36). While these studies cumulatively confirm that, as expected, stimulant concentration does influence T cell immunoreactivity, there are limited studies optimising stimulant concentrations. Identifying universally optimal stimulant concentrations is impractical, especially for T cell peptide epitope screening studies. Lyophilized peptide epitopes are typically resuspended in DMSO, which has several key advantages and disadvantages relative to other common solvents (Supplementary Table 3) (91). Even relatively low concentrations of DMSO in cell culture have been found to induce changes to cellular phenotypes (92), with as little as 0.25% DMSO influencing immunogenicity (93), and marginal toxicity reported at 2-5% DMSO (93). While reporting the stimulant concentration is common practise, we further recommend reporting the concentration and type of solvent in the stimulation.
Stimulation time
The length of time cells are incubated in the presence of the stimulant can influence the number and intensity of responsive cells. Six-hour long incubations are frequently reported (94, 95), however, longer incubations are also common (96). Overnight incubations have been reported to increase antigen immunogenicity (97). The optimal timepoint to measure mitogen stimulations has been reported to be between 72 and 96 hours (98). Similarly, kinetic studies investigating whole-blood stimulations found 72 hours as optimal for mitogen stimulants (99). While antigenic peptide stimulation time length has been found to not decrease cell viability, longer mitogen stimulations have been associated with increased numbers of non-viable cells (45). Interestingly, a recent study over a 12 hour time course noted peak cytokine mRNA expression occurred between 3-6 hours post peptide-epitope stimulation, and occurred in a peptide- and donor-specific fashion (95).
Discussion
Taken together these studies demonstrate that technical variation during PBMC collection, cryopreservation, thawing, and culture may profoundly impact cell viability and immunogenicity. Notably, many of the conclusions of these studies are contradictory, suggesting the influence and interaction of underreported factors. Although several of the results discussed in this review are derived from single studies, these results from well-controlled, high-quality studies nevertheless underscore the importance of maintaining stringently consistent protocols and reporting guidelines when analysing human T cells. Therefore, we propose the use of the HANC’s-SOP when collecting and cryopreserving PBMCs (31); and the IMPAACT networks-SOP when thawing PBMCs (32). Furthermore, we have made additional recommendations to standardise the protocol during PBMC collection, storage, thawing, and culture (Table 1).
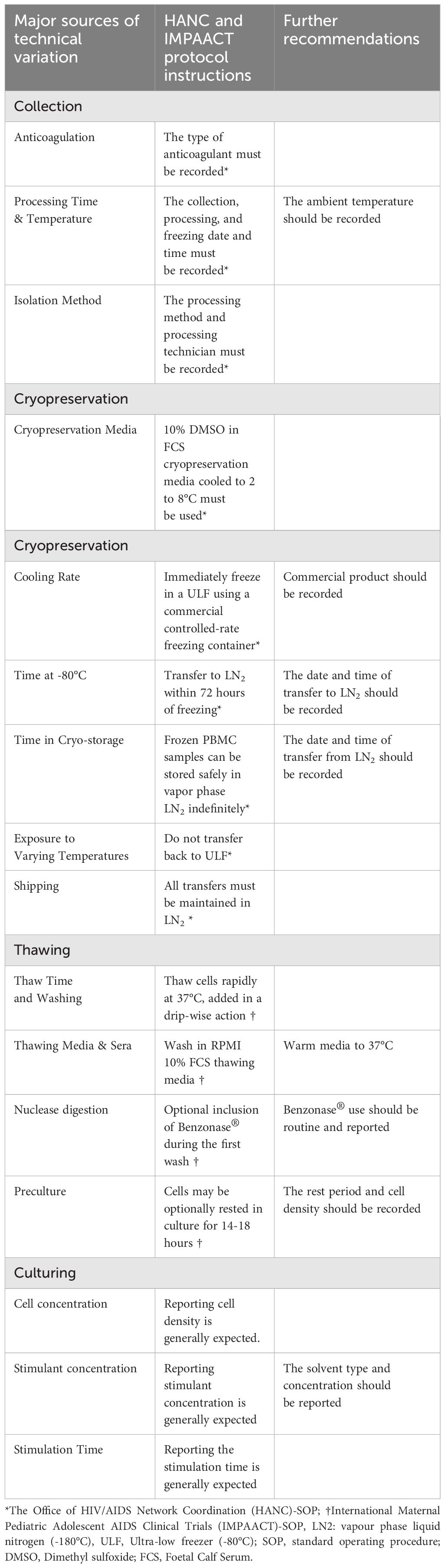
Table 1 Standardised protocol for the collection, cryopreservation, thawing and culturing of human PBMCs for T cell studies.
The biological mechanisms underlying variations in cell viability and immunogenicity during PBMC processing are complex and incompletely understood. The various reagents used during processing may significantly influence T cells by impacting cellular integrity, agonistically or antagonistically influencing cellular activation, or changing media chemistry. For example, the anticoagulant EDTA can impair T cell activation by disrupting cellular calcium levels (100), while heparin may interfere with cell-to-cell interactions (101). The more profound influences which have been found following delays in processing and fluctuations in temperature during storage can be attributed to induced metabolic stress which may have activated apoptosis pathways (102, 103). Techniques like density-gradient centrifugation and cryopreservation introduce stress through physical forces (104), and if cryoprotectants are not completely removed after thawing, they may further alter cell functions (105). This complexity highlights the critical need for strict standardisation and detailed documentation in T cell research to avoid the technical pitfalls when collecting, cryopreserving, thawing, and stimulating human T-cells.
The development of PBMC processing SOPs by HANC and its affiliated members such as IMPAACT were driven by an awareness that technical variation during this critical experiment window may reduce the reproducibility of experimental findings. There is a growing concern that the social, behavioural, and biomedical sciences are facing a ‘reproducibility crisis’ (106), as many influential published findings have failed reproducibility testing (107, 108). Indeed, a relatively recent large meta-analysis proposed that, at best, around 50% of preclinical biomedical research was reproducible (109). The cause of this low reproducibility is likely complex, ranging from poor statistical literacy (110) and noise discovery (111), to unconscious or conscious bias induced by a pressure to publish (112). While a low rate of reproducibility is certainly not ideal, it has been argued that some irreproducibility is expected (113), even potentially beneficial (114), when cutting-edge science is investigating competing hypotheses. Nevertheless, there is a growing appreciation that insufficient communication of experimental methods is a major contributing factor (115–118). We expect that strict adherence to HANC’s and HANC affiliates SOPs during PBMC collection, cryopreservation, thawing, and culture will greatly improve the replicability of human T cell research.
Author contributions
DB: Writing – original draft, Writing – review & editing. CM: Writing – review & editing. DD: Writing – review & editing.
Funding
The author(s) declare financial support was received for the research, authorship, and/or publication of this article. DB is supported by James Cook University Prestige Research Training Program Stipend (RTPS).
Conflict of interest
The authors declare that the research was conducted in the absence of any commercial or financial relationships that could be construed as a potential conflict of interest.
The author(s) declared that they were an editorial board member of Frontiers, at the time of submission. This had no impact on the peer review process and the final decision.
Publisher’s note
All claims expressed in this article are solely those of the authors and do not necessarily represent those of their affiliated organizations, or those of the publisher, the editors and the reviewers. Any product that may be evaluated in this article, or claim that may be made by its manufacturer, is not guaranteed or endorsed by the publisher.
Supplementary material
The Supplementary Material for this article can be found online at: https://www.frontiersin.org/articles/10.3389/fimmu.2024.1382192/full#supplementary-material
References
1. Boehm T, Swann JB. Origin and evolution of adaptive immunity. Annu Rev Anim Biosci. (2014) 2:259–83. doi: 10.1146/annurev-animal-022513-114201
2. Appay V, Papagno L, Spina CA, Hansasuta P, King A, Jones L, et al. Dynamics of T cell responses in HIV infection. J Immunol. (2002) 168:3660–6. doi: 10.4049/jimmunol.168.7.3660
3. Tarke A, Coelho CH, Zhang Z, Dan JM, Yu ED, Methot N, et al. SARS-CoV-2 vaccination induces immunological T cell memory able to cross-recognize variants from Alpha to Omicron. Cell. (2022) 185:847–59. e11. doi: 10.1016/j.cell.2022.01.015
4. Raskov H, Orhan A, Christensen JP, Gögenur I. Cytotoxic CD8+ T cells in cancer and cancer immunotherapy. Br J cancer. (2021) 124:359–67. doi: 10.1038/s41416-020-01048-4
5. Beissert S, Schwarz A, Schwarz T. Regulatory T cells. J Invest Dermatol. (2006) 126:15–24. doi: 10.1038/sj.jid.5700004
6. Crotty S. A brief history of T cell help to B cells. Nat Rev Immunol. (2015) 15:185–9. doi: 10.1038/nri3803
7. Masopust D, Vezys V, Wherry EJ, Ahmed R. A brief history of CD8 T cells. Eur J Immunol. (2007) 37:S103–S10. doi: 10.1002/(ISSN)1521-4141
8. Sterner RC, Sterner RM. CAR-T cell therapy: current limitations and potential strategies. Blood Cancer J. (2021) 11:69. doi: 10.1038/s41408-021-00459-7
9. Li K, Zhang Q, Zhang Y, Yang J, Zheng J. T-cell-associated cellular immunotherapy for lung cancer. J Cancer Res Clin Oncol. (2015) 141:1249–58. doi: 10.1007/s00432-014-1867-0
10. Browne DJ, Brady JL, Waardenberg AJ, Loiseau C, Doolan DL. An analytically and diagnostically sensitive RNA extraction and RT-qPCR protocol for peripheral blood mononuclear cells. Front Immunol. (2020) 11. doi: 10.3389/fimmu.2020.00402
11. Boaz MJ, Hayes P, Tarragona T, Seamons L, Cooper A, Birungi J, et al. Concordant proficiency in measurement of T-cell immunity in human immunodeficiency virus vaccine clinical trials by peripheral blood mononuclear cell and enzyme-linked immunospot assays in laboratories from three continents. Clin Vaccine Immunol. (2009) 16:147–55. doi: 10.1128/CVI.00326-08
12. Crough T, Beagley L, Smith C, Jones L, Walker DG, Khanna R. Ex vivo functional analysis, expansion and adoptive transfer of cytomegalovirus-specific T-cells in patients with glioblastoma multiforme. Immunol Cell Biol. (2012) 90:872–80. doi: 10.1038/icb.2012.19
13. Vella AT, Dow S, Potter TA, Kappler J, Marrack P. Cytokine-induced survival of activated T cells in vitro and in vivo. Proc Natl Acad Sci. (1998) 95:3810–5. doi: 10.1073/pnas.95.7.3810
14. Wegner J, Hackenberg S, Scholz C-J, Chuvpilo S, Tyrsin D, Matskevich AA, et al. High-density preculture of PBMCs restores defective sensitivity of circulating CD8 T cells to virus- and tumor-derived antigens. Blood. (2015) 126:185–94. doi: 10.1182/blood-2015-01-622704
15. Yi PC, Zhuo L, Lin J, Chang C, Goddard A, Yoon OK. Impact of delayed PBMC processing on functional and genomic assays. J Immunol Methods. (2023) 519:113514. doi: 10.1016/j.jim.2023.113514
16. Tabares P, Berr S, Romer PS, Chuvpilo S, Matskevich AA, Tyrsin D, et al. Human regulatory T cells are selectively activated by low-dose application of the CD28 superagonist TGN1412/TAB08. Eur J Immunol. (2014) 44:1225–36. doi: 10.1002/eji.201343967
17. Suntharalingam G, Perry MR, Ward S, Brett SJ, Castello-Cortes A, Brunner MD, et al. Cytokine storm in a phase 1 trial of the anti-CD28 monoclonal antibody TGN1412. N Engl J Med. (2006) 355:1018–28. doi: 10.1056/NEJMoa063842
18. Dennehy KM, Elias F, Na SY, Fischer KD, Hünig T, Lühder F. Mitogenic CD28 signals require the exchange factor Vav1 to enhance TCR signaling at the SLP-76-Vav-Itk signalosome. J Immunol. (2007) 178:1363–71. doi: 10.4049/jimmunol.178.3.1363
19. Römer PS, Berr S, Avota E, Na S-Y, Battaglia M, ten Berge I, et al. Preculture of PBMCs at high cell density increases sensitivity of T-cell responses, revealing cytokine release by CD28 superagonist TGN1412. Blood. (2011) 118:6772–82. doi: 10.1182/blood-2010-12-319780
20. Mwangoka G, Ogutu B, Msambichaka B, Mzee T, Salim N, Kafuruki S, et al. Experience and challenges from clinical trials with malaria vaccines in Africa. Malar J. (2013) 12:86. doi: 10.1186/1475-2875-12-86
21. Burchill MA, Tamburini BA, Pennock ND, White JT, Kurche JS, Kedl RM. T cell vaccinology: exploring the known unknowns. Vaccine. (2013) 31:297–305. doi: 10.1016/j.vaccine.2012.10.096
22. Flemming A. Why have T cell-inducing vaccines for HIV failed so far? Nat Rev Immunol. (2024) 24:89–. doi: 10.1038/s41577-024-00989-8
23. Hamed SM, Sakr MM, El-Housseiny GS, Wasfi R, Aboshanab KM. State of the art in epitope mapping and opportunities in COVID-19. Future Sci OA. (2023) 16:Fso832. doi: 10.2144/fsoa-2022-0048
24. Li Pira G, Ivaldi F, Moretti P, Manca F. High throughput T epitope mapping and vaccine development. J BioMed Biotechnol. (2010) 2010:325720. doi: 10.1155/2010/325720
25. Pang Z, Lu M-m, Zhang Y, Gao Y, Bai J-j, Gu J-y, et al. Neoantigen-targeted TCR-engineered T cell immunotherapy: current advances and challenges. biomark Res. (2023) 11:104. doi: 10.1186/s40364-023-00534-0
26. Mallone R, Mannering SI, Brooks-Worrell BM, Durinovic-Belló I, Cilio CM, Wong FS, et al. Isolation and preservation of peripheral blood mononuclear cells for analysis of islet antigen-reactive T cell responses: position statement of the T-Cell Workshop Committee of the Immunology of Diabetes Society. Clin Exp Immunol. (2010) 163:33–49. doi: 10.1111/j.1365-2249.2010.04272.x
27. Xiao D, Ling KHJ, Custodio J, Majeed SR, Tarnowski T. Quantitation of intracellular triphosphate metabolites of antiretroviral agents in peripheral blood mononuclear cells (PBMCs) and corresponding cell count determinations: review of current methods and challenges. Expert Opin Drug Metab Toxicol. (2018) 14:781–802. doi: 10.1080/17425255.2018.1500552
28. Spicer N, Aleshkina J, Biesma R, Brugha R, Caceres C, Chilundo B, et al. National and subnational HIV/AIDS coordination: are global health initiatives closing the gap between intent and practice? Global Health. (2010) 6:3. doi: 10.1186/1744-8603-6-3
29. Sarzotti-Kelsoe M, Needham LK, Rountree W, Bainbridge J, Gray CM, Fiscus SA, et al. The Center for HIV/AIDS Vaccine Immunology (CHAVI) multi-site quality assurance program for cryopreserved human peripheral blood mononuclear cells. J Immunol Methods. (2014) 409:21–30. doi: 10.1016/j.jim.2014.05.013
30. Ducar C, Smith D, Pinzon C, Stirewalt M, Cooper C, McElrath MJ, et al. Benefits of a comprehensive quality program for cryopreserved PBMC covering 28 clinical trials sites utilizing an integrated, analytical web-based portal. J Immunol Methods. (2014) 409:9–20. doi: 10.1016/j.jim.2014.03.024
31. Coordination. HAN. Procedures for the Isolation and Cryopreservation of PBMC [Standard Operating Procedure (SOP)] (2023). Available online at: https://www.hanc.info/resources/sops-guidelines-resources/laboratory/cross-network-pbmc-processing-sop.html
32. Coordination. HAN. Peripheral Blood Mononuclear Cell (PBMC) Thawing Standard Operating Procedure [Standard Operating Procedure (SOP)] (2023). Available online at: https://www.hanc.info/content/dam/hanc/documents/laboratory/actg-impaact-laboratory-manual/PBMC-Thawing.pdf
34. Kumar P, Satchidanandam V. Ethyleneglycol-bis-(beta-aminoethylether)tetraacetate as a blood anticoagulant: preservation of antigen-presenting cell function and antigen-specific proliferative response of peripheral blood mononuclear cells from stored blood. Clin Diagn Lab Immunol. (2000) 7:578–83. doi: 10.1128/CDLI.7.4.578-583.2000
35. Bull M, Lee D, Stucky J, Chiu YL, Rubin A, Horton H, et al. Defining blood processing parameters for optimal detection of cryopreserved antigen-specific responses for HIV vaccine trials. J Immunol Methods. (2007) 322:57–69. doi: 10.1016/j.jim.2007.02.003
36. Hoffmeister B, Bunde T, Rudawsky IM, Volk HD, Kern F. Detection of antigen-specific T cells by cytokine flow cytometry: the use of whole blood may underestimate frequencies. Eur J Immunol. (2003) 33:3484–92. doi: 10.1002/eji.200324223
37. Navas A, Giraldo-Parra L, Prieto MD, Cabrera J, Gómez MA. Phenotypic and functional stability of leukocytes from human peripheral blood samples: considerations for the design of immunological studies. BMC Immunol. (2019) 20:5. doi: 10.1186/s12865-019-0286-z
38. Langat RK, Farah B, Indangasi J, Ogola S, Omosa-Manyonyi G, Anzala O, et al. Performance of International AIDS Vaccine Initiative African clinical research laboratories in standardised ELISpot and peripheral blood mononuclear cell processing in support of HIV vaccine clinical trials. Afr J Lab Med. (2021) 10:1056. doi: 10.4102/ajlm.v10i1.1056
39. Olson WC, Smolkin ME, Farris EM, Fink RJ, Czarkowski AR, Fink JH, et al. Shipping blood to a central laboratory in multicenter clinical trials: effect of ambient temperature on specimen temperature, and effects of temperature on mononuclear cell yield, viability and immunologic function. J Trans Med. (2011) 9:26. doi: 10.1186/1479-5876-9-26
40. Sarathkumara YD, Browne DJ, Kelly AM, Pattinson DJ, Rush CM, Warner J, et al. The effect of tropical temperatures on the quality of RNA extracted from stabilized whole-blood samples. Int J Mol Sci. (2022) 23(18):10609. doi: 10.21203/rs.3.rs-1721397/v1
41. Hendrika W, Grievink TL, Kluft C, Moerland M, Malone KE. Comparison of three isolation techniques for human peripheral blood mononuclear cells: cell recovery and viability, population composition, and cell functionality. Biopreserv Biobank. (2016) 14:410–5. doi: 10.1089/bio.2015.0104
42. Ruitenberg JJ, Mulder CB, Maino VC, Landay AL, Ghanekar SA. VACUTAINER® CPT™ and Ficoll density gradient separation perform equivalently in maintaining the quality and function of PBMC from HIV seropositive blood samples. BMC Immunol. (2006) 7:11. doi: 10.1186/1471-2172-7-11
43. Nilsson C, Aboud S, Karlén K, Hejdeman B, Urassa W, Biberfeld G. Optimal blood mononuclear cell isolation procedures for gamma interferon enzyme-linked immunospot testing of healthy Swedish and Tanzanian subjects. Clin Vaccine Immunol. (2008) 15:585–9. doi: 10.1128/CVI.00161-07
44. Chen H, Schürch CM, Noble K, Kim K, Krutzik PO, O’Donnell E, et al. Functional comparison of PBMCs isolated by Cell Preparation Tubes (CPT) vs. Lymphoprep Tubes. BMC Immunol. (2020) 21:15. doi: 10.1186/s12865-020-00345-0
45. Jeurink PV, Vissers YM, Rappard B, Savelkoul HFJ. T cell responses in fresh and cryopreserved peripheral blood mononuclear cells: Kinetics of cell viability, cellular subsets, proliferation, and cytokine production. Cryobiology. (2008) 57:91–103. doi: 10.1016/j.cryobiol.2008.06.002
46. Reimann KA, Chernoff M, Wilkening CL, Nickerson CE, Landay AL. Preservation of lymphocyte immunophenotype and proliferative responses in cryopreserved peripheral blood mononuclear cells from human immunodeficiency virus type 1-infected donors: implications for multicenter clinical trials. Clin Diagn Lab Immunol. (2000) 7:352–9. doi: 10.1128/CDLI.7.3.352-359.2000
47. Kreher CR, Dittrich MT, Guerkov R, Boehm BO, Tary-Lehmann M. CD4+ and CD8+ cells in cryopreserved human PBMC maintain full functionality in cytokine ELISPOT assays. J Immunol Methods. (2003) 278:79–93. doi: 10.1016/S0022-1759(03)00226-6
48. Raju R, Bryant SJ, Wilkinson BL, Bryant G. The need for novel cryoprotectants and cryopreservation protocols: Insights into the importance of biophysical investigation and cell permeability. Biochim Biophys Acta (BBA) - Gen Subj. (2021) 1865:129749. doi: 10.1016/j.bbagen.2020.129749
49. Nazarpour R, Zabihi E, Alijanpour E, Abedian Z, Mehdizadeh H, Rahimi F. Optimization of human peripheral blood mononuclear cells (PBMCs) cryopreservation. Int J Mol Cell Med. (2012) 1:88–93.
50. Makino M, Baba M. A cryopreservation method of human peripheral blood mononuclear cells for efficient production of dendritic cells. Scandinavian J Immunol. (1997) 45:618–22
51. Barcelo H, Faul J, Crimmins E, Thyagarajan B. A practical cryopreservation and staining protocol for immunophenotyping in population studies. Curr Protoc Cytom. (2018) 84:e35. doi: 10.1002/cpcy.35
52. Kaiser D, Otto NM, McCallion O, Hoffmann H, Zarrinrad G, Stein M, et al. Freezing Medium Containing 5% DMSO Enhances the Cell Viability and Recovery Rate After Cryopreservation of Regulatory T Cell Products ex vivo and in vivo. Front Cell Dev Biol. (2021) 9:750286. doi: 10.3389/fcell.2021.750286
53. Hope CM, Huynh D, Wong YY, Oakey H, Perkins GB, Nguyen T, et al. Optimization of blood handling and peripheral blood mononuclear cell cryopreservation of low cell number samples. Int J Mol Sci. (2021) 22(17):9129. doi: 10.3390/ijms22179129
54. Bogoslovsky T, Wang D, Maric D, Scattergood-Keepper L, Spatz M, Auh S, et al. Cryopreservation and enumeration of human endothelial progenitor and endothelial cells for clinical trials. J Blood Disord Transfus. (2013) 4(5):158. doi: 10.4172/2155-9864.1000158
55. Disis ML, dela Rosa C, Goodell V, Kuan L-Y, Chang JCC, Kuus-Reichel K, et al. Maximizing the retention of antigen specific lymphocyte function after cryopreservation. J Immunol Methods. (2006) 308:13–8. doi: 10.1016/j.jim.2005.09.011
56. Alipour R, Fatemi A, Alsahebfosul F, Andalib A, Pourazar A. Autologous plasma versus fetal calf serum as a supplement for the culture of neutrophils. BMC Res Notes. (2020) 13:39. doi: 10.1186/s13104-020-4902-z
57. Filbert H, Attig S, Bidmon N, Renard BY, Janetzki S, Sahin U, et al. Serum-free freezing media support high cell quality and excellent ELISPOT assay performance across a wide variety of different assay protocols. Cancer Immunol Immunother. (2013) 62:615–27. doi: 10.1007/s00262-012-1359-5
58. Anja Germann JCS, Kemp-Kamke B, Zimmermann H, von Briesen H. Standardized serum-free cryomedia maintain peripheral blood mononuclear cell viability, recovery, and antigen-specific T-cell response compared to fetal calf serum-based medium. Biopreserv Biobank. (2011) 9:229–36. doi: 10.1089/bio.2010.0033
59. Kopeika J, Thornhill A, Khalaf Y. The effect of cryopreservation on the genome of gametes and embryos: principles of cryobiology and critical appraisal of the evidence. Hum Reprod Update. (2015) 21:209–27. doi: 10.1093/humupd/dmu063
60. Behnamifar A, Bernal B, Torres O, Luis-Chincoya H, Ggil M, García-Casado P, et al. Research Note: evaluation of two methods for adding cryoprotectant to semen and effects of bovine serum albumin on quality characteristics of cryopreserved rooster spermatozoa. Poult Sci. (2021) 100:101093. doi: 10.1016/j.psj.2021.101093
61. Fabozzi G, Starita MF, Rega E, Alteri A, Colicchia A, Piscitelli C, et al. Evaluation of the efficiency of two different freezing media and two different protocols to preserve human spermatozoa from cryoinjury. Int J Reprod Med. (2016) 2016:6059757. doi: 10.1155/2016/6059757
62. Tree T, Roep BO, Peakman M. Enhancing the sensitivity of assays to detect T cell reactivity: the effect of cell separation and cryopreservation media. Ann New York Acad Sci. (2004) 1037:26–32. doi: 10.1196/annals.1337.005
63. Buhl T, Legler TJ, Rosenberger A, Schardt A, Schön MP, Haenssle HA. Controlled-rate freezer cryopreservation of highly concentrated peripheral blood mononuclear cells results in higher cell yields and superior autologous T-cell stimulation for dendritic cell-based immunotherapy. Cancer Immunol Immunother. (2012) 61:2021–31. doi: 10.1007/s00262-012-1262-0
64. Huang Z, Liu W, Liu B, He X, Guo H, Xue S, et al. Cryopreservation of human T lymphocytes under fast cooling with controlled ice nucleation in cryoprotective solutions of low toxicity. Cryobiology. (2021) 103:92–100. doi: 10.1016/j.cryobiol.2021.09.002
65. Baboo J, Kilbride P, Delahaye M, Milne S, Fonseca F, Blanco M, et al. The impact of varying cooling and thawing rates on the quality of cryopreserved human peripheral blood T cells. Sci Rep. (2019) 9:3417. doi: 10.1038/s41598-019-39957-x
66. Mrowiec ZR, Fernandez-DeLeon M, Marchioni M, Cieszynska B. Comparison of controlled vs non-controlled rate freezing of umbilical cord blood units. Blood. (2004) 104:5011. doi: 10.1182/blood.V104.11.5011.5011
67. Yang J, Diaz N, Adelsberger J, Zhou X, Stevens R, Rupert A, et al. The effects of storage temperature on PBMC gene expression. BMC Immunol. (2016) 17:6. doi: 10.1186/s12865-016-0144-1
68. Valeri CR, Pivacek LE. Effects of the temperature, the duration of frozen storage, and the freezing container on in vitro measurements in human peripheral blood mononuclear cells. Transfusion. (1996) 36:303–8. doi: 10.1046/j.1537-2995.1996.36496226141.x
69. Weinberg A, Song LY, Wilkening CL, Fenton T, Hural J, Louzao R, et al. Optimization of storage and shipment of cryopreserved peripheral blood mononuclear cells from HIV-infected and uninfected individuals for ELISPOT assays. J Immunol Methods. (2010) 363:42–50. doi: 10.1016/j.jim.2010.09.032
70. Weinberg A, Song LY, Wilkening C, Sevin A, Blais B, Louzao R, et al. Optimization and limitations of use of cryopreserved peripheral blood mononuclear cells for functional and phenotypic T-cell characterization. Clin Vaccine Immunol. (2009) 16:1176–86. doi: 10.1128/CVI.00342-08
71. McCullough J, Haley R, Clay M, Hubel A, Lindgren B, Moroff G. Long-term storage of peripheral blood stem cells frozen and stored with a conventional liquid nitrogen technique compared with cells frozen and stored in a mechanical freezer. Transfusion. (2010) 50:808–19. doi: 10.1111/j.1537-2995.2009.02482.x
72. Tollerud DJ, Brown LM, Clark JW, Neuland CY, Mann DL, Pankiw-Trost LK, et al. Cryopreservation and long-term liquid nitrogen storage of peripheral blood mononuclear cells for flow cytometry analysis: effects on cell subset proportions and fluorescence intensity. J Clin Lab Anal. (1991) 5:255–61. doi: 10.1002/jcla.1860050406
73. Li B, Yang C, Jia G, Liu Y, Wang N, Yang F, et al. Comprehensive evaluation of the effects of long-term cryopreservation on peripheral blood mononuclear cells using flow cytometry. BMC Immunol. (2022) 23:30. doi: 10.1186/s12865-022-00505-4
74. Germann A, Oh Y-J, Schmidt T, Schön U, Zimmermann H, von Briesen H. Temperature fluctuations during deep temperature cryopreservation reduce PBMC recovery, viability and T-cell function. Cryobiology. (2013) 67:193–200. doi: 10.1016/j.cryobiol.2013.06.012
75. Cosentino LM, Corwin W, Baust JM, Diaz-Mayoral N, Cooley H, Shao W, et al. Preliminary report: evaluation of storage conditions and cryococktails during peripheral blood mononuclear cell cryopreservation. Cell Preserv Technol. (2007) 5:189–204. doi: 10.1089/cpt.2007.9987
76. Posevitz-Fejfár A, Posevitz V, Gross CC, Bhatia U, Kurth F, Schütte V, et al. Effects of blood transportation on human peripheral mononuclear cell yield, phenotype and function: implications for immune cell biobanking. PloS One. (2014) 9:e115920. doi: 10.1371/journal.pone.0115920
77. Xu Y, Zou Q, Gao F, Wang D, Xue S, Lin H, et al. Effect of warming process on the survival of cryopreserved human peripheral blood mononuclear cells. Biopreserv Biobank. (2021) 19:318–23. doi: 10.1089/bio.2020.0058
78. Hønge BL, Petersen MS, Olesen R, Møller BK, Erikstrup C. Optimizing recovery of frozen human peripheral blood mononuclear cells for flow cytometry. PloS One. (2017) 12:e0187440. doi: 10.1371/journal.pone.0187440
79. Yao T, Asayama Y. Animal-cell culture media: History, characteristics, and current issues. Reprod Med Biol. (2017) 16:99–117. doi: 10.1002/rmb2.12024
80. Ramachandran H, Laux J, Moldovan I, Caspell R, Lehmann PV, Subbramanian RA. Optimal thawing of cryopreserved peripheral blood mononuclear cells for use in high-throughput human immune monitoring studies. Cells. (2012) 1:313–24. doi: 10.3390/cells1030313
81. Darzynkiewicz Z, Li X, Gong J. Chapter 2 assays of cell viability: discrimination of cells dying by apoptosis. In: Darzynkiewicz Z, Paul Robinson J, Crissman HA, editors. Methods in cell biology, vol. 41. Amsterdam, Netherlands: Academic Press (1994). p. 15–38.
82. García-Piñeres AJ, Hildesheim A, Williams M, Trivett M, Strobl S, Pinto LA. DNAse treatment following thawing of Cryopreserved PBMC is a procedure suitable for lymphocyte functional studies. J Immunol Methods. (2006) 313:209–13. doi: 10.1016/j.jim.2006.04.004
83. Smith JG, Liu X, Kaufhold RM, Clair J, Caulfield MJ. Development and validation of a gamma interferon ELISPOT assay for quantitation of cellular immune responses to varicella-zoster virus. Clin Diagn Lab Immunol. (2001) 8:871–9. doi: 10.1128/CDLI.8.5.871-879.2001
84. Liao Q, Chiu NHL, Shen C, Chen Y, Vouros P. Investigation of enzymatic behavior of benzonase/alkaline phosphatase in the digestion of oligonucleotides and DNA by ESI-LC/MS. Anal Chem. (2007) 79:1907–17. doi: 10.1021/ac062249q
85. Santos R, Buying A, Sabri N, Yu J, Gringeri A, Bender J, et al. Improvement of IFNg ELISPOT performance following overnight resting of frozen PBMC samples confirmed through rigorous statistical analysis. Cells. (2015) 4:1–18. doi: 10.3390/cells4010001
86. Lemieux J, Jobin C, Simard C, Néron S. A global look into human T cell subsets before and after cryopreservation using multiparametric flow cytometry and two-dimensional visualization analysis. J Immunol Methods. (2016) 434:73–82. doi: 10.1016/j.jim.2016.04.010
87. Kuerten S, Batoulis H, Recks MS, Karacsony E, Zhang W, Subbramanian RA, et al. Resting of cryopreserved PBMC does not generally benefit the performance of antigen-specific T cell ELISPOT assays. Cells. (2012) 1:409–27. doi: 10.3390/cells1030409
88. Lozano-Ojalvo D, Lopez-Fandino R, Lopez-Exposito I. PBMC-derived T cells. In: Verhoeckx K, Cotter P, Lopez-Exposito I, Kleiveland C, Lea T, Mackie A, et al, editors. The Impact of Food Bioactives on Health: in vitro and ex vivo models. Cham CH: Springer International Publishing (2015). p. 169–80.
89. Sävendahl L, Underwood LE. Decreased interleukin-2 production from cultured peripheral blood mononuclear cells in human acute starvation*. J Clin Endocrinol Metab. (1997) 82:1177–80. doi: 10.1210/jc.82.4.1177
90. Kern F, Bunde T, Faulhaber N, Kiecker F, Khatamzas E, Rudawski IM, et al. Cytomegalovirus (CMV) phosphoprotein 65 makes a large contribution to shaping the T cell repertoire in CMV-exposed individuals. J Infect di. (2002) 185:1709–16. doi: 10.1086/340637
91. Houghten RA, Li CH. Reduction of sulfoxides in peptides and proteins. Anal Biochem. (1979) 98:36–46. doi: 10.1016/0003-2697(79)90702-4
92. Tunçer S, Gurbanov R, Sheraj I, Solel E, Esenturk O, Banerjee S. Low dose dimethyl sulfoxide driven gross molecular changes have the potential to interfere with various cellular processes. Sci Rep. (2018) 8:14828. doi: 10.1038/s41598-018-33234-z
93. Elisia I, Nakamura H, Lam V, Hofs E, Cederberg R, Cait J, et al. DMSO represses inflammatory cytokine production from human blood cells and reduces autoimmune arthritis. PloS One. (2016) 11:e0152538. doi: 10.1371/journal.pone.0152538
94. Kaveh DA, Whelan AO, Hogarth PJ. The duration of antigen-stimulation significantly alters the diversity of multifunctional CD4 T cells measured by intracellular cytokine staining. PloS One. (2012) 7:e38926. doi: 10.1371/journal.pone.0038926
95. Browne DJ, Kelly AM, Brady JL, Doolan DL. A high-throughput screening RT-qPCR assay for quantifying surrogate markers of immunity from PBMCs. Front Immunol. (2022) 13. doi: 10.3389/fimmu.2022.962220
96. Portmann K, Linder A, Oelgarth N, Eyer K. Single-cell deep phenotyping of cytokine release unmasks stimulation-specific biological signatures and distinct secretion dynamics. Cell Rep Methods. (2023) 3:100502. doi: 10.1016/j.crmeth.2023.100502
97. Bourguignon P, Clément F, Renaud F, Le Bras V, Koutsoukos M, Burny W, et al. Processing of blood samples influences PBMC viability and outcome of cell-mediated immune responses in antiretroviral therapy-naïve HIV-1-infected patients. J Immunol Methods. (2014) 414:1–10. doi: 10.1016/j.jim.2014.09.001
98. Listvanova S, Temmerman S, Stordeur P, Verscheure V, Place S, Zhou L, et al. Optimal kinetics for quantification of antigen-induced cytokines in human peripheral blood mononuclear cells by real-time PCR and by ELISA. J Immunol Methods. (2003) 281:27–35. doi: 10.1016/S0022-1759(03)00267-9
99. Godoy-Ramirez K, Franck K, Mahdavifar S, Andersson L, Gaines H. Optimum culture conditions for specific and nonspecific activation of whole blood and PBMC for intracellular cytokine assessment by flow cytometry. J Immunol Methods. (2004) 292:1–15. doi: 10.1016/j.jim.2004.04.028
100. Mellau LS, Jørgensen RJ. Does EDTA-infusion affect calcium homeostatis leading to increased resistance to challenge? Acta Vet Scand Suppl. (2003) 97:29–34.
101. Xu X, Dai Y. Heparin: an intervenor in cell communication. J Cell Mol Med. (2010) 14:175–80. doi: 10.1111/j.1582-4934.2009.00871.x
102. Gu ZT, Wang H, Li L, Liu YS, Deng XB, Huo SF, et al. Heat stress induces apoptosis through transcription-independent p53-mediated mitochondrial pathways in human umbilical vein endothelial cell. Sci Rep. (2014) 4:4469. doi: 10.1038/srep04469
103. Hikim APS, Lue Y, Yamamoto CM, Vera Y, Rodriguez S, Yen PH, et al. Key apoptotic pathways for heat-induced programmed germ cell death in the testis. Endocrinology. (2003) 144:3167–75. doi: 10.1210/en.2003-0175
104. Whaley D, Damyar K, Witek RP, Mendoza A, Alexander M, Lakey JR. Cryopreservation: an overview of principles and cell-specific considerations. Cell Transpl. (2021) 30:963689721999617. doi: 10.1177/0963689721999617
105. Ekpo MD, Xie J, Liu X, Onuku R, Boafo GF, Tan S. Incorporating cryopreservation evaluations into the design of cell-based drug delivery systems: an opinion paper. Front Immunol. (2022) 13:967731. doi: 10.3389/fimmu.2022.967731
106. Romero F. Philosophy of science and the replicability crisis. Philos Compass. (2019) 14:e12633. doi: 10.1111/phc3.12633
107. Errington TM, Denis A, Perfito N, Iorns E, Nosek BA. Challenges for assessing replicability in preclinical cancer biology. eLife. (2021) 10:e67995. doi: 10.7554/eLife.67995
108. Open Science C. Estimating the reproducibility of psychological science. Science. (2015) 349:aac4716. doi: 10.1126/science.aac4716
109. Freedman LP, Cockburn IM, Simcoe TS. The economics of reproducibility in preclinical research. PloS Biol. (2015) 13:e1002165. doi: 10.1371/journal.pbio.1002165
110. Ioannidis JP. Microarrays and molecular research: noise discovery? Lancet. (2005) 365:454–5. doi: 10.1016/S0140-6736(05)70249-X
111. Branch MN. The “Reproducibility crisis:” Might the methods used frequently in behavior-analysis research help? Perspect Behav Sci. (2019) 42:77–89. doi: 10.1007/s40614-018-0158-5
112. Fanelli D. Do pressures to publish increase scientists’ Bias? An empirical support from US states data. PloS One. (2010) 5:e10271. doi: 10.1371/journal.pone.0010271
113. Ioannidis JPA. Why most published research findings are false. PloS Med. (2005) 2:e124. doi: 10.1371/journal.pmed.0020124
114. Hunter P. The reproducibility “crisis”: Reaction to replication crisis should not stifle innovation. EMBO Rep. (2017) 18:1493–6. doi: 10.15252/embr.201744876
115. Scudamore CL, Soilleux EJ, Karp NA, Smith K, Poulsom R, Herrington CS, et al. Recommendations for minimum information for publication of experimental pathology data: MINPEPA guidelines. J Pathol. (2016) 238:359–67. doi: 10.1002/path.4642
116. Bustin SA, Benes V, Garson JA, Hellemans J, Huggett J, Kubista M, et al. The MIQE guidelines: minimum information for publication of quantitative real-time PCR experiments. Clin Chem. (2009) 55:611–22. doi: 10.1373/clinchem.2008.112797
117. Brazma A, Hingamp P, Quackenbush J, Sherlock G, Spellman P, Stoeckert C, et al. Minimum information about a microarray experiment (MIAME)—toward standards for microarray data. Nat Genet. (2001) 29:365–71. doi: 10.1038/ng1201-365
Keywords: PBMC, epitopes, stimulation, human, T cell
Citation: Browne DJ, Miller CM and Doolan DL (2024) Technical pitfalls when collecting, cryopreserving, thawing, and stimulating human T-cells. Front. Immunol. 15:1382192. doi: 10.3389/fimmu.2024.1382192
Received: 05 February 2024; Accepted: 29 April 2024;
Published: 15 May 2024.
Edited by:
Zhengguo Xiao, University of Maryland, United StatesReviewed by:
Shareni Jeyamogan, Northwestern University, United StatesCopyright © 2024 Browne, Miller and Doolan. This is an open-access article distributed under the terms of the Creative Commons Attribution License (CC BY). The use, distribution or reproduction in other forums is permitted, provided the original author(s) and the copyright owner(s) are credited and that the original publication in this journal is cited, in accordance with accepted academic practice. No use, distribution or reproduction is permitted which does not comply with these terms.
*Correspondence: Denise L. Doolan, d.doolan@imb.uq.edu.au
†These authors have contributed equally to this work and share senior authorship