- 1Department of Critical Care Medicine, The First Medical Center, Chinese People’s Liberation Army (PLA) General Hospital, Beijing, China
- 2Graduate School of The People’s Liberation Army (PLA) General Hospital, Beijing, China
- 3Department of Emergency Medicine, The Second Hospital of Hebei Medical University, Shijiazhuang, China
- 4Department of Basic Medicine, Graduate School, Chinese PLA General Hospital, Beijing, China
- 5National Key Laboratory of Kidney Diseases, National Clinical Research Center for Kidney Diseases, Beijing Key Laboratory of Kidney Disease Research, Beijing, China
Sepsis is a clinical syndrome caused by uncontrollable immune dysregulation triggered by pathogen infection, characterized by high incidence, mortality rates, and disease burden. Current treatments primarily focus on symptomatic relief, lacking specific therapeutic interventions. The core mechanism of sepsis is believed to be an imbalance in the host’s immune response, characterized by early excessive inflammation followed by late immune suppression, triggered by pathogen invasion. This suggests that we can develop immunotherapeutic treatment strategies by targeting and modulating the components and immunological functions of the host’s innate and adaptive immune systems. Therefore, this paper reviews the mechanisms of immune dysregulation in sepsis and, based on this foundation, discusses the current state of immunotherapy applications in sepsis animal models and clinical trials.
1 Introduction
Sepsis is defined as a life-threatening organ dysfunction caused by a dysregulated host response to infection, which can progress to septic shock and/or multiple organ dysfunction or failure in severe cases (1). Recently, sepsis has exhibited characteristics of “three highs and one low,” namely high incidence, high mortality, high disease burden, and low recovery rates. Rudd et al. reported approximately 48.9 million cases of sepsis globally and 11 million sepsis-related deaths in 2017, accounting for 19.7% of all global deaths (2). Li et al. identified 9,455,279 registered hospital cases of sepsis in China from 2017 to 2019, with 806,728 related deaths, particularly among high-risk groups such as children under the age of 9 (incidence rate: 20.4%) and the elderly aged 65 and older (incidence rate: 57.5%) (3). Furthermore, a recent study estimated the annual direct and indirect economic costs associated with sepsis in the Netherlands to be between 3.8 and 6.5 billion euros (4). Moreover, survivors of sepsis from different demographics may experience various adverse outcomes, such as long-term neurodevelopmental abnormalities and physical dysfunctions in neonates, as well as cognitive impairments and psychological issues such as depression and anxiety (5–8). These phenomena are not only related to the rapid progression and unpredictable nature of the complex clinical picture but also due to the lack of specific therapeutic measures in current clinical practice. Undoubtedly, the early implementation of a sepsis bundle strategy, including fluid resuscitation, antibiotic therapy, and lung-protective ventilation, is necessary and crucial. These protective measures have a positive impact on patients with sepsis and have significantly reduced mortality rates to some extent (9–11). However, studies have also found that inappropriate fluid management (12) and uncontrolled use of broad-spectrum empirical antibiotics (13–15) may hinder the timely control of the patient’s condition. Additionally, the management of sepsis patients should also focus on modulating the host response, including but not limited to the use of corticosteroids and vasopressors (16).
As early as 1893, William Coley inadvertently discovered that postoperative infections with pyogenic Streptococcus could induce tumor regression in sarcoma patients (17), thereby unveiling the prelude to immunotherapy. With the rapid advancement of biomedical technologies, various autologous immunotherapies (such as dendritic cells (DCs), interleukin (IL)-2) (18–20), genetically engineered therapies (such as CAR-T therapy) (21, 22), and recombinant antibodies (such as bispecific antibodies, trispecific antibodies) (23–25) have been developed and introduced into clinical trial. Although immunotherapy was initially and most extensively applied in the field of oncology, as research has revealed that virtually all diseases have some form of direct or indirect relation with the immune system, the scope of immunotherapy has extended beyond cancer treatment. It now encompasses therapies aimed at inducing, enhancing, or suppressing the patient’s own immune response to treat a wide array of diseases related to immune molecules, immune cells, and the immune system itself, including infectious diseases (such as sepsis) (26), autoimmune diseases (such as systemic lupus erythematosus) (27, 28), and diseases related to immunosenescence and inflammaging (such as atherosclerosis, Alzheimer’s disease, and diabetes) (29–32). It is commonly accepted that the core pathophysiological mechanism of sepsis involves a dysregulated immune response characterized by acute-phase hyperinflammation followed by late-phase immune suppression, triggered by pathogens. This suggests the feasibility of immunotherapy (33). Compared to bundle therapies, immunotherapy can modulate disease through mechanisms such as cytokine level adjustment, targeting immune checkpoints/blocking programmed cell death, and supplementing immunoglobulins. This adjusts the components and functions of the patient’s innate and adaptive immune systems, thereby facilitating the rapid restoration of immune homeostasis. Additionally, with the continuous development of novel biomarkers (34, 35), medical professionals may soon be able to track and record changes in a patient’s immune function in real-time by analyzing immune components, metabolic products, differentially expressed proteins or genes in bodily fluids or tissues. This development could provide convenient management pathways and monitoring windows for immunotherapy. Of course, potential side effects and immune-related adverse events (IR-AEs) during treatment should not be overlooked. This underlines the importance of developing convenient and reliable markers to dynamically monitor patients’ immune statuses during immunotherapy.
Although recent research into the use of immunotherapy for treating sepsis has made preliminary progress, issues such as the therapy’s stability, long-term efficacy, and potential side effects remain to be validated. This article reviews the mechanisms of immunological dysregulation in sepsis hosts and summarizes the application of immunotherapy in both sepsis animal models and clinical patients based on this understanding. Additionally, we present perspectives on the prospects and challenges of applying immunotherapy in the treatment of sepsis.
2 Sepsis-induced immunological dysregulation
Initially, sepsis was believed to be primarily driven by an excessive systemic inflammatory response induced by exogenous and/or endogenous infections. However, increasing evidence suggests that during the progression of sepsis, the phenotype of the host’s immune cells can shift from pro-inflammatory to anti-inflammatory, with a rise in anti-inflammatory cytokine levels and a marked reduction in pro-inflammatory cytokines. In the later stages of the disease, there is even sustained apoptosis of immune cells, indicating that “sepsis is not merely an inflammatory response.” Furthermore, some clinical trial results have reported that immunosuppressive therapies have few therapeutic effect on sepsis patients, underscoring that sepsis is not simply a process of inflammatory response. Today, a multitude of preclinical and clinical studies have confirmed that sepsis involves a complex syndrome with multiple intrinsic mechanisms, including systemic inflammatory response syndrome (SIRS), compensatory anti-inflammatory response syndrome (CARS), immunoparalysis, inflammatory cytokine gene remodeling, and endotoxin tolerance (ET).
Current research supports the notion that both SIRS and CARS occur simultaneously at the onset of sepsis (36, 37). However, an imbalance in the intensity and duration of responses between these two phases leads to clinical manifestations characterized initially by an excessive inflammatory response and later by immune suppression or immune paralysis. During this period, factors such as the host’s age, ethnicity, genetic background, comorbidities, and the type of pathogen can influence the immune status and clinical features of sepsis patients. In terms of overall effects, the acute phase of the disease course in sepsis hosts is marked by excessive activation of immune cells, cytokine storm (CS), and SIRS, while the later stages are characterized by increased immune cell apoptosis or chronic exhaustion and functional impairment, as well as ET (Figure 1).
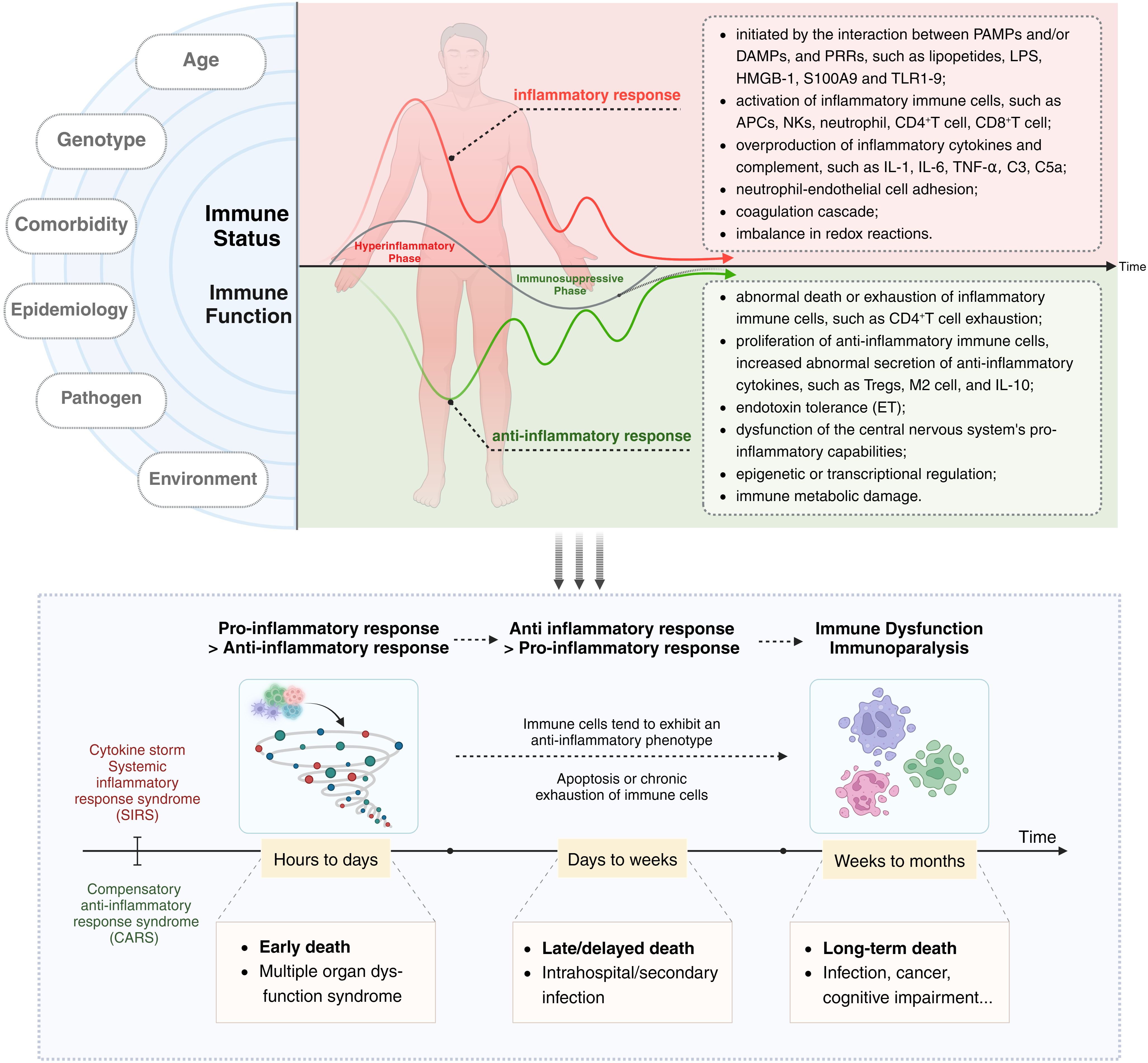
Figure 1 The immune dysregulation mechanism and related characteristics of sepsis: Current research supports the notion that both SIRS and CARS occur simultaneously at the onset of sepsis. However, an imbalance in the intensity and duration of responses between these two phases leads to clinical manifestations characterized initially by an excessive inflammatory response and later by immune suppression or immune paralysis. Acute excessive inflammatory response is associated with early death, while later immune paralysis/tolerance is an important cause of late or long-term death. The left side of the diagram delineates factors influencing the host’s immune status and function, such as age, genotype, comorbidities, epidemiology, pathogen type, and environmental conditions. The central and right portions of the diagram depict the immune status of a host with sepsis, with the upper half focusing on excessive inflammatory responses and their associated characteristics, and the lower half detailing secondary immune suppression and its related features. The black arrow (“Time”) indicates that the immune response and immune status of sepsis patients undergo dynamic and imbalanced changes over time/disease progression.
2.1 The acute phase is dominated by an excessive inflammatory response
During the acute phase of sepsis, both in animal models and in patients, a significant elevation is observed in white blood cell counts and levels of inflammatory cytokines, such as tumor necrosis factor (TNF)-α, interferon (IFN)-γ, and IL-6 (38–41). When the host is exposed to exogenous and/or endogenous pathogens’ pathogen associated molecular patterns (PAMPs), such as lipopolysaccharide (LPS) (42, 43) and mannose-binding lectin (MBL) (44), or to damage-associated molecular patterns (DAMPs) released from its own damaged tissue cells, such as histones (45), high-mobility group box-1 (HMGB-1) (46, 47), and heat shock proteins (HSPs) (48), these molecules are recognized and interact with pattern recognition receptors (PRRs) on the surface of antigen-presenting cells. This interaction marks the initiation of the acute inflammatory response in sepsis. Current research has categorized PRRs into five subfamilies: the Toll-like receptors (TLRs) (49, 50), the nucleotide oligomerization domain (NOD)-like receptors (NLRs) (51, 52), the retinoic acid-inducible gene-I (RIG-I)-like receptors (RLRs) (53), the C-type lectin receptors (CLRs) (54, 55), and the Absent in melanoma-2-like receptors (ALRs) (56). The effector domains of PRRs mediate the activation of downstream inflammatory signaling pathways by recognizing specific ligands. This activation leads to the release of pro-inflammatory cytokines, recruitment of innate immune cells, and induction of inflammatory responses. Specifically, to address intracellular infections caused by pathogens, certain cytoplasmic PRRs (typically from the NLRs or ALRs families) often serve as receptor proteins that participate in the assembly of inflammasomes. Inflammasomes recruit pro-caspase-1 and activate it to caspase-1, which in turn cleaves pro-interleukin-1β, pro-interleukin-18, and gasdermin (GDSMD) into their mature forms, triggering an inflammatory response and cell pyroptosis. Subsequently, these cytokines induce further proliferation and activation of immune cells, skewing them toward an inflammatory phenotype and elevating levels of various inflammatory mediators such as interleukins IL-1, IL-6, IL-17, TNF-α, IFN-γ, and chemotactic factors such as prostaglandins, histamine. For instance, neutrophils combat pathogens through direct actions such as phagocytosis, degranulation, and the release of neutrophil extracellular traps (NETs), as well as indirectly through the release of reactive oxygen species (ROS), reactive nitrogen species (RNS), and proteolytic enzymes during cell proliferation and migration (57–60). Macrophages polarize toward an M1 inflammatory phenotype and release large amounts of IL-1β, TNF-α, and IL-6 (61–63), while DCs mediate the activation of CD4+T (64). This syndrome is also accompanied by an imbalance of redox reactions (65, 66), neutrophil-endothelial cell adhesion (67), activation of the complement system (68, 69), and the coagulation cascade (70, 71). Additionally, intense complement activation (especially C3 and C5a) enhances vascular permeability, and increases the adhesiveness between leukocytes and vascular endothelial cells, further promoting the inflammatory response and damaging self-tissue organs (72, 73). A recent single-cell transcriptomic analysis revealed the inflammatory profile of peripheral blood mononuclear cells (PBMCs) in COVID-19 and sepsis patients, identifying ten highly inflammatory cell subtypes and their characteristics (74). The adaptive immune response typically lags behind the innate immune response, with antigen-presenting cells activating T lymphocytes through the dual signaling system of the major histocompatibility complex (MHC)/antigen peptide-T cell receptor (TCR) and CD80-CD28 (75, 76). Under the influence of various cytokines released by innate immune cells, tissue cells, and activated lymphocytes (77), T lymphocytes differentiate into multiple subgroups, including cytotoxic T cells (CTLs), helper T cells (Th), and regulatory T cells (Tregs), playing a crucial role in the acute phase inflammatory response in sepsis hosts. Additionally, B lymphocytes counteract pathogen invasion by secreting antigen-specific antibodies and inflammatory cytokines (78).
Unfortunately, during the acute phase of sepsis, the excessive activation of immune cells and pro-inflammatory cytokines is not adequately restrained by anti-inflammatory responses. Consequently, this uncontrolled and excessive inflammatory response not only fails to efficiently eradicate pathogens within the host but also leads to severe cellular death, tissue damage, and organ dysfunction, potentially resulting in the early death of the host (79).
2.2 The later stages are characterized by secondary immune suppression
Under normal circumstances, as infections are cleared and host compensatory anti-inflammatory responses are modulated, a patient’s heightened inflammatory response gradually subsides and returns to physiological levels, ultimately restoring the immune homeostasis of the internal environment. However, in the later stages of sepsis, the host often experiences a dysregulated anti-inflammatory response, which is not conducive to moderating inflammation but instead manifests as immune tolerance or paralysis, showing a low response to pathogens (80). These factors significantly increase the risk of secondary infections and adverse prognoses, including late or posthumous deaths (81, 82).
Lymphocyte apoptosis or reduction in their numbers is a significant factor contributing to immune paralysis. Preclinical studies using sepsis animal models indicate widespread apoptosis of parenchymal and immune cells across multiple organs, including the thymus, spleen, lungs, intestines, and skeletal muscle (83–86). More importantly, in mice undergoing cecal ligation and puncture (CLP), it has been observed that sepsis-induced impairment in T cells’ ability to combat pathogen infections can persist for several months (87). In clinical research, uncontrolled circulating immune cell apoptosis is considered a primary cause of impaired immune function (88–90). Additionally, transcriptomic analysis revealed that circulating lymphocytes in the later stages of the disease exhibit low inflammatory activity and immune suppression (91). There is a notable reduction in the numbers of CD4+ and CD8+T cells, with an increased proportion of Tregs (90, 92). This impaired proliferative capacity and sustained apoptosis of T lymphocytes may be associated with the upregulation of negative signaling pathways, such as the programmed death receptor-1/programmed death ligand-1 (PD-1/PD-L1) axis (93–96). The role of B lymphocytes in immune suppression remains unclear, yet studies have identified selective depletion or increased apoptosis of memory B cells in sepsis patients (97). These cells exhibit reduced MHC II expression and tend toward a CD21lowCD95high exhausted-like phenotype (98), with a significant reduction in antigen-specific antibody release. During the process of immune suppression in the host, innate immune cells are both victims and perpetrators (99–102), including neutrophils (103–105), DCs (106–108), and monocytes/macrophages (109–112). These cells commonly experience abnormal differentiation, functional impairments, and extensive tissue infiltration. Research has shown that an increased proportion of immature neutrophils (CD10lowCD16low cells) in the whole blood of sepsis patients is associated with an increased risk of early death within 48 hours after sepsis onset (113). This may be linked to the upregulation of myeloid-derived suppressor cells (MDSCs) subgroups (113) and the integrin Mac-1 (αMβ2) (114), which mediates suppression of T cell proliferation. Recent studies have reported a higher abundance of immature neutrophil subgroups expressing genes related to IL1R2, PADI4, and MPO in sepsis patients, and in vitro experiments suggest that these immature neutrophils can inhibit the proliferation and activation of CD4+T cells (115). Significant changes also occur in DCs, characterized by the acute phase’s systemic high-inflammatory microenvironment excessively activating the immature DCs stored in parenchymal tissues and lymphoid organs until they are exhausted. However, newly generated DCs are functionally immature, which includes acquiring the immunogenic phenotype of pathogens, capturing, processing, and/or presenting antigens, as well as the capacity to stimulate T cell activation (116). Recent studies have found that low expression of monocyte human leukocyte antigen DR (MHLA-DR) of in sepsis patients reduces activation of T lymphocytes (117). Increasing evidence suggests that low expression of MHLA-DR can serve as a biomarker for predicting immune paralysis or poor prognosis in sepsis (118–121). Concurrently with extensive apoptosis of immune cells, a class of immature myeloid cells collectively known as MDSCs—which include progenitors or precursors of neutrophils, DCs, and monocytes—proliferate abundantly and are released into the bloodstream (122, 123). These MDSCs exhibit significant immunosuppressive properties, including inhibiting the proliferation and activation of effector lymphocytes while activating Tregs, reducing the production of inflammatory cells and promoting the release of anti-inflammatory cytokines, as well as upregulating the expression of immune checkpoint molecules.
Endotoxin tolerance constitutes a critical aspect of immunosuppression (124), often resulting from the innate and adaptive immune cells of the host being persistently exposed to low levels of endotoxins or LPS, entering a transient “desensitized state.” This leads to an unresponsive state of the host immune system to sudden, high-dose endotoxin or LPS exposure. Numerous preclinical studies have shown that mouse monocytes continuously exposed to LPS in vitro can undergo ET, characterized by the downregulation of inflammatory cytokines (including TNF-α, IL-6, and IL-8) and the upregulation of anti-inflammatory cytokine (IL-10) expression (125). Macrophages from mice pretreated with LPS also exhibit a diminished response to subsequent LPS stimulation in vitro, with downregulated mRNA expression of genes encoding recombinant granulocyte-macrophage colony-stimulating factor (GM-CSF), IFN-γ-inducible protein-10, JE/monocyte chemoattractant protein-1, and macrophage-inflammatory protein-1β/2 (126). This reduced responsiveness and immune tolerance of monocytes/macrophages to LPS stimulation may be associated with the remodeling of NF-κB function, chromatin modifications, and reprogramming of inflammatory genes (127). Besides, tolerance-inducing DCs in mice, which express lower levels of MHC-II and CD86, can induce the proliferation and recruitment of CD4+Foxp3+ Tregs through the secretion of TGF-β (128). In clinical research, healthy volunteers continuously stimulated with LPS exhibited a downregulation of pro-inflammatory cytokine levels in vivo (129). In vitro experiments showed that under high levels of LPS stimulation, the expression of MHC class II, CD86, and MHC II class transactivator (CIITA) in human monocytes was significantly reduced, leading to impaired antigen presentation (130). Shalova et al. treated monocytes from sepsis patients with LPS in vitro, and their findings indicated that the expression of genes associated with pro-inflammatory cytokines (such as TNF-α, IL-1A, IL-1B, IL-6, IL-12A, IL-23A) and chemokines (such as CCL3, CCL4, CCL5, CCL20, CCL23, CXCL2, CXCL11) were not upregulated. Similarly, gene expression related to activation-associated surface molecules (such as CD80, CD44) and transcription factors (such as ATF5, NFKB1, NFKB2, REL, RELA) were also deficient. This suggests that monocytes in sepsis patients are unable to actively respond to LPS stimulation, indicative of an immune functional defect (131). Research has also reported that hypoxia-inducible factor-1α (HIF1-α) is overexpressed in human tolerant monocytes and targets the upregulation of PD-L1-related gene expression, thereby mediating the suppression of T lymphocyte proliferation and activation (132). Although there are currently no universally recognized biomarkers for ET, genomic and transcriptomic analyses can partially elucidate the genetic variations associated with the onset of immune tolerance in sepsis hosts, which is helpful in distinguishing patients with immune dysfunction (133–135).
In addition to the mechanisms mentioned above, various intrinsic mechanisms contribute to secondary immunosuppression, including dysfunction in the pro-inflammatory functions of the central nervous system (136), epigenetic or transcriptional regulation (137, 138), and immune metabolic dysfunction. For instance, PBMCs exhibit reduced cytokine release capacity, and monocytes display metabolic dysfunctions such as impaired glycolysis and lipid oxidation. Additionally, mitochondrial damage within tissue and immune cells is accompanied by decreased ATP and NAD+ levels, reduced lactate production, and diminished oxygen consumption (139–141).
3 Preclinical studies of immunotherapy in the treatment of sepsis
To date, a substantial body of research has shown that immunotherapy offers some protective effects against the severity of disease, organ dysfunction, and mortality in sepsis models in animals. Although the same experimental drugs have shown significant variability in effectiveness across different sepsis modeling techniques or types of sepsis animals, some drugs effective in animal models have not yet successfully transitioned to clinical trials. Overall, the positive results from animal studies provide preliminary indications for the potential of immunotherapy in clinical trials (Table 1).
3.1 Targeting cytokine
Blocking the activity of inflammatory cytokines is a fundamental strategy to inhibit acute-phase excessive inflammatory responses. For instance, in vivo experiments with Infliximab treatment (an anti-TNF-α antibody) have significantly reduced serum TNF-α levels in septic rats, markedly improving acute lung, liver, and kidney injuries, and increased the 7-day survival rate of rats from 0% to 37.5% (142). The IL-6 receptor antagonist Tocilizumab can inhibit NF-κB activation, significantly reducing inflammatory responses and oxidative stress levels in CLP rats, and offers protection against sepsis-induced acute lung and kidney injuries (143). Flierl and colleagues confirmed that anti-IL-17 treatment significantly increased the 7-day survival rate of CLP mice (control group: 10% vs. anti-IL-17 antibody group: 60%), and administering the treatment 12 hours later still offered some protection to the host (144). Intracerebral administration of anti-IL-17 or anti-IL-17R antibody also mitigated microglial activation and central nervous system inflammation in CLP mice by blocking the IL-17A/IL-17R pathway, alleviating sepsis-associated encephalopathy (SAE), and improving cognitive dysfunctions (145). Wan and others found that an anti-CCR6 antibody, by blocking the CCR6-CCL20 axis, inhibited the recruitment and migration of γδT cells and the release of IL-17A in CLP mice, reducing the infiltration of inflammatory cells in the liver induced by sepsis (146). In studies of immune suppression, Unsinger and colleagues tested the efficacy of recombinant human IL-7 (rhIL-7) in a peritonitis-induced sepsis model in mice. The results indicated that rhIL-7 mediated a reduction in apoptosis of CD4+ and CD8+T cells and promoted the production of IFN-γ by upregulating the expression of Bcl-2, thereby improving immune suppression in mice (147). Conversely, Murphey and others, using a combination of IFN-γ and anti-IL-10 antibody in CLP mice experiencing immune suppression, did not observe a significant improvement in bacterial clearance rates or survival rates (148). However, recent studies have shown that IFN-γ treatment in CLP mice can upregulate CD86 expression on DCs and reduce DC apoptosis, reversing the immune suppression caused by sepsis (149). These findings highlight that while supplementing or modulating cytokines—whether anti-inflammatory or pro-inflammatory—is a relatively straightforward immunomodulatory strategy, the application protocols and therapeutic outcomes require further study.
3.2 Targeting complements
The activated complement system plays a critical role in the transmission of inflammatory signals. For instance, studies have shown that using an anti-C5aR antibody in CLP mice significantly reduces the accumulation of inflammatory cytokines in plasma and decreases mortality (150). Another in vitro experiment observed that after administering an anti-C5a antibody, chemotaxis of neutrophils activated via the complement alternative pathway was significantly reduced in septic mice, helping to regulate excessive accumulation and abnormal infiltration of neutrophils in tissues (151). However, recent research indicates that C5a/C5aR also participates in anti-inflammatory signaling. Sommerfeld and colleagues observed that mice with a C5aR1 gene deficiency exhibited high levels of IFN-γ and low levels of IL-10 post-CLP (152). Beyond C5a/C5aR, other components of the complement system have also garnered attention. Chung and others discovered that hippocampal tissue expression of complement C1q was upregulated in SAE mice, mediating neuronal damage. Intracerebral injection of a specific C1q blocker significantly protected microglial cells, improving neurocognitive function impairments in mice (69).
3.3 Targeting immune checkpoints
Immune checkpoints (ICs) inhibit excessive activation and proliferation of immune cells, regulate inflammatory responses, prevent damage to self-tissues and organs, and promote the restoration of immune homeostasis. However, the continuous transmission of negative signaling pathways can also induce uncontrollable cell apoptosis and immune suppression. Common ICs, including PD-1/PD-L1, cytotoxic T-lymphocyte antigen-4 (CTLA-4)/CD80(CD86), B-and T-lymphocyte attenuator (BTLA)/herpes virus entry mediator (HVEM), and T-cell immunoglobulin and mucin-domain containing-3 (TIM-3)/Galectin-9 (Gal-9), are all potential therapeutic targets. Chang and colleagues reported that the individual use of anti-PD-1, anti-PD-L1, or anti-CTLA-4 antibodies could promote the release of IFN-γ by blocking lymphocyte apoptosis and upregulating the expression of MHC II on DCs, modulating the immune suppression state in mice with primary and secondary fungal sepsis (153). Similarly, Zhang and others observed that in CLP mice, the expression of PD-1/PD-L1 was upregulated on T cells, B cells, and monocytes, and that anti-PD-L1 antibodies could inhibit some lymphocyte apoptosis and exhaustion induced by sepsis, promote the release of TNF-α and IL-6, reduce the production of IL-10, and significantly improve the survival rate of the mice (154). Huang and colleagues discovered that the deletion of TIM-3 in CD4+T cells in septic mice could alleviate lymphocyte apoptosis and restore their proliferative activity, thus protecting organ function and reducing mortality (155). In the same year, Liu and others observed that treatment with anti-TIM-3 in CLP mice reduced lymphocyte apoptosis and significantly alleviated sepsis-induced acute lung and liver damage (156). However, the timing and dosage of administration can lead to significant differences in treatment effectiveness. For instance, Inoue and colleagues noted an increase in CTLA-4 expression in T cells in CLP mice, and administering anti-CTLA-4 antibodies reduced sepsis-induced lymphocyte apoptosis, but the 7-day survival rate of the mice showed a clear dose dependency; higher doses of anti-CTLA-4 antibodies decreased survival rates while lower doses increased them (157). Immune checkpoint inhibitors (ICIs) play a crucial role in inhibiting persistent apoptosis and exhaustion of lymphocytes. Other novel checkpoint inhibitors such as VISTA (158) and ICOS (159) are also being developed and validated.
3.4 Targeting mesenchymal stem cells
Mesenchymal stem cells (MSCs) are described as balancers of the inflammatory microenvironment and immune dysregulation due to their ability to modulate the activation, maturation, proliferation, differentiation, and effector functions of various immune cells (170, 171). This modulation occurs through direct contact with target cells, the release of bioactive factors (such as cytokines, growth factors, chemokines), and paracrine pathways involving the secretion of extracellular vesicles and exosomes that contain cytokines, miRNAs, and other soluble factors. Extensive in vivo and in vitro experimental results support that MSCs and their derivatives can regulate programmed cell death (including apoptosis (160–162), autophagy (163–165), pyroptosis (166–168), and ferroptosis (169)) in immune and tissue cells, maintaining homeostasis within the host environment. They can modulate imbalanced immune responses, alleviate tissue and organ damage, improve multi-organ dysfunction, and reduce mortality. Despite satisfactory results in rodent models, the efficacy of MSCs and their derivatives in larger animal models remains unclear. For instance, Horak and colleagues observed that in pigs with peritoneal sepsis treated with MSCs, there was no significant alleviation of hemodynamic abnormalities, the systemic overactivation of inflammatory responses was unmodulated, and organ failure assessment scores continued to increase (172). Thus, further preclinical experiments are needed to determine the appropriate pathways, dosages, indications, and potential adverse reactions for the use of MSCs and their derivatives in sepsis and related diseases.
4 Clinical studies of immunotherapy in the treatment of sepsis
The clinical course of sepsis in patients is not only a race between the pathogen and the host’s immune response but also a battle between the host’s own abnormally activated inflammatory response and the subsequent anti-inflammatory response. Pathogen infection is the trigger for the onset of sepsis, while the host’s uncontrolled and disordered immune response is the key mechanism driving the progression of sepsis. Increasingly, clinical trials are attempting to modulate the components and functions of the host’s immune system to promote the restoration of immune homeostasis, yet the outcomes of these trials vary widely. To date, there are no universally recognized effective or approved immune therapies or related products for the treatment of sepsis in clinical settings. Furthermore, sepsis is a highly heterogeneous disease, which suggests that differences in immune responses among individual hosts should also be considered. Of course, as our understanding of the pathophysiological mechanisms of sepsis deepens and as biomarkers related to sepsis are continuously developed, these immune components serve not only as important indicators to assist clinicians in assessing the severity of the condition and guiding treatment but also as potential targets for immunotherapy (Figure 2; Table 2).
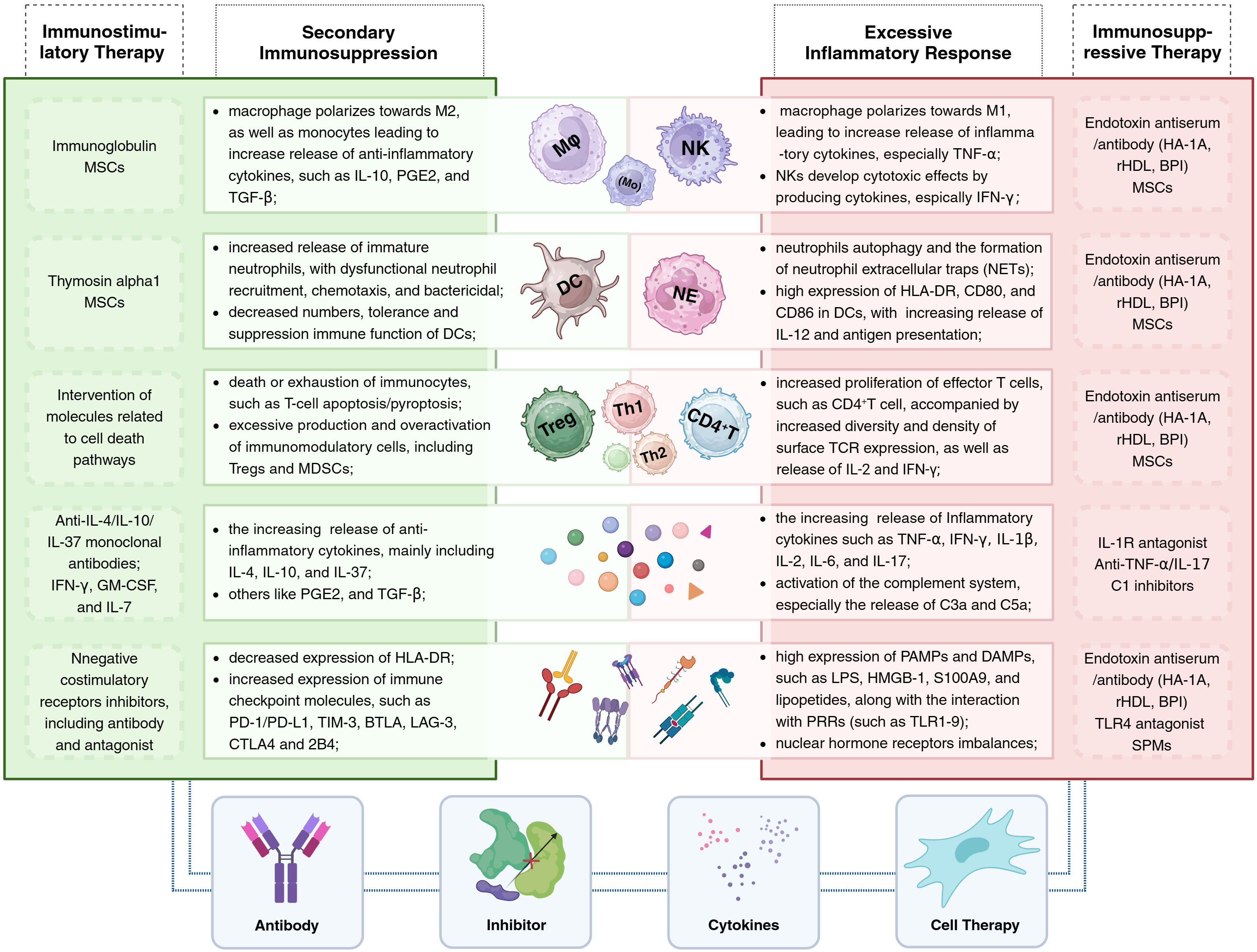
Figure 2 Immunotherapy for sepsis: The central portion of the illustration presents alterations in immune cells and components within the host under different immune states (with excessive inflammatory responses on the right and secondary immune suppression on the left), alongside corresponding immunotherapeutic strategies. The lower section of the illustration summarizes the four primary types of immunomodulatory agents, including antibodies, inhibitors, cytokines, and cellular therapies. BPI, bactericidal/permeability-increasing protein; HA-1A, human monoclonal anti-endotoxin antibody; rHDL, reconstituted high-density lipoprotein; SPMs, specialized pro-resolving mediators.
4.1 Immunosuppressive therapy
The acute inflammatory response in sepsis patients, coupled with the encouraging results from preclinical studies of immunotherapy, has provided a preliminary basis for conducting clinical trials on immunosuppressive therapies. Although some studies indicate that administering anti-inflammatory treatments within the first few hours after the onset of sepsis can somewhat mitigate the systemic inflammatory response and protect against organ dysfunction, overall, immunosuppressive strategies have not yielded satisfactory results in clinical trials for sepsis.
4.1.1 Anti-inflammatory cytokines
Numerous studies have reported that levels of pro-inflammatory cytokines such as TNF-α, IL-6, IL-18, and IFN-γ are associated with increased short-term or long-term mortality in sepsis patients, suggesting that blocking cytokine-related pathways could potentially improve host survival rates (41, 209–212). Currently, anti-IL-1 and anti-TNF-α antibodies are widely used in autoimmune diseases such as rheumatoid arthritis and ankylosing spondylitis, and their efficacy in sepsis patients has also been tested. For example, a Phase III randomized controlled trial (RCT) confirmed that Anakinra (recombinant interleukin-1 receptor antagonist/rIL-1Ra) significantly improved the 28-day survival rate of sepsis patients with concurrent hepatic and biliary dysfunction and disseminated intravascular coagulation (Anakinra group: 65.4% vs. placebo group: 35.3%). Patients with sepsis characterized by high inflammatory activity or macrophage activation syndrome (MAS) may benefit from this anti-inflammatory treatment strategy (173). Another ongoing RCT led by Kotsaki is exploring whether intravenous injection of Anakinra can improve the SOFA score and 28-day/90-day mortality rates in sepsis patients, with expected results to be published in 2025 (ClinicalTrials.gov identifier: NCT04990232) (174). However, studies by Opal and others have shown that 72 hours of continuous intravenous infusion of rhIL-1Ra or placebo did not significantly reduce the 28-day mortality rates in patients (175). As for anti-TNF-α treatment, the results are not promising. While such therapy can reduce the concentrations of IL-6 and TNF-α in the serum of sepsis patients, its effect on reducing mortality is very limited (176–178). Furthermore, a RCT involving a dimer consisting of the extracellular portion of the human TNF receptor and the Fc portion of IgG1 (TNFR: Fc) was conducted with 141 sepsis patients randomly assigned to receive a single intravenous infusion of TNFR: Fc at doses of 0.15, 0.45, or 1.5 mg per kilogram of body weight, or a placebo. The results indicated that TNFR: Fc treatment did not reduce mortality rates, and higher doses of TNFR: Fc might be associated with an increased risk of death (179). Allocetra™-OTS (early apoptotic cells) has been demonstrated to modulate immune response. A recent Phase I clinical trial evaluated the safety and efficacy of Allocetra™-OTS in patients with sepsis. The findings indicate that this formulation is safe for patients with mild to moderate sepsis and can facilitate the early resolution of cytokine storms, thereby improving organ dysfunction and reducing ICU length of stay (180). The complement system also represents an important target for anti-inflammatory strategies. For example, C1 esterase inhibitor treatment has been shown to mitigate the systemic inflammatory response and protect renal function in sepsis patients (181–183). However, a recent Phase III clinical trial (ALXN1210-COV-305) indicated that intravenous administration of Ravulizumab (a complement C5 inhibitor) combined with supportive care did not improve clinical outcomes in hospitalized patients with severe COVID-19. Instead, there were serious IR-AEs in five patients (ClinicalTrials.gov identifier: NCT04369469) (184).
4.1.2 Targeting PAMPs/DAMPs-related pathways
Since the 20th century, numerous clinical trials have focused on neutralizing endotoxins to block the activation of inflammatory responses. However, treatments like human monoclonal anti-endotoxin antibodies (HA-1A) are applicable to infections caused by Gram-negative bacteria, and their clinical efficacy has been unstable (185–187). In addition, bactericidal/permeability-increasing protein (BPI)-related formulations have also been reported in early studies to have antimicrobial activity and neutralizing effects on endotoxins, offering some protection in severe meningococcal sepsis in children (188, 189). Moreover, in 2013, Steven’s team reported that Eritoran (a TLR4 antagonist) did not significantly reduce the 28-day and 12-month mortality rates in sepsis patients (ClinicalTrials.gov identifier: NCT00334828) (190). Thus, immune therapies targeting PAMPs/DAMPs-related pathways have not yet demonstrated superiority in treatment effectiveness.
4.2 Immunostimulatory therapy
To date, clinical trials exploring immunosuppressive therapies for sepsis have indicated that the “theoretical” or “idealized” strategy of immunosuppression to mitigate the excessive inflammatory response in sepsis and restore host immune homeostasis is not always viable. Simultaneously, with advancing research into sepsis, a significant number of preclinical and clinical studies have observed that hosts often exhibit an excessive state of immunosuppression in the later stages of the disease. This severe immunoparalysis mediates secondary infections, subsequent deaths, or severe adverse prognoses (93, 213). In this context, there has been a shift in focus from “immunosuppressive therapy” to “immune enhancement therapy” or “immune stimulation therapy” in an effort to reverse the state of immune paralysis in sepsis hosts. The goal is to reduce apoptosis of immune cells and stimulate their proliferation and anti-inflammatory effects, enhance the release of inflammatory cells, and improve patients’ long-term survival rates.
4.2.1 Immunoglobulin supplementation
Previous research has shown that sepsis patients with low levels of IgG have a significantly higher mortality rate compared to those with normal IgG levels, and that low IgG levels in sepsis patients are associated with a higher 28-day mortality (214). This suggests that intravenous immunoglobulin (IVIG) could be a valuable immune-enhancing therapy. For example, a retrospective case-control study indicated that polyclonal IgM-enriched immunoglobulin (IgGAM) reduced the 28-day mortality rates in sepsis patients compared to the control group (IgGAM treatment group: 39% vs. control group: 58%) and was an independent protective factor against 28-day mortality (OR: 0.34; 95% CI: 0.17–0.67) (191). Additionally, numerous studies have systematically reviewed the efficacy of IVIG in sepsis. For instance, Turgeon and colleagues conducted a systematic review of 20 RCTs using IVIG to treat sepsis, which suggested that IVIG treatment was closely associated with patient survival benefits compared to placebo or no intervention (risk ratio: 0.74; 95% CI: 0.62–0.89). Sepsis or septic shock patients who received a total dose of 1 gram per kilogram body weight or more (risk ratio: 0.61; 95% CI: 0.40–0.94) and those treated for more than two days (risk ratio: 0.66; 95% CI: 0.53–0.82) showed a significant correlation with improved survival rates (192). A recent meta-analysis, which included 31 RCTs, found that IVIG treatment significantly improved APACHE II scores in sepsis patients (mean difference: -1.65; 95% CI: -2.89 to -0.63), reduced hospital stay (mean difference: -4.46 days; 95% CI: -2.57 to -6.35), and decreased mortality rates (RR: 0.86; 95% CI: 0.77–0.95), particularly playing a crucial role in reducing mortality rates among adult sepsis patients (RR: 0.70; 95% CI: 0.57–0.86) (193). Although there is significant heterogeneity among the clinical trials included in the meta-analysis, including differences in population characteristics, administration regimens, types of antibody formulations, and control interventions, and varying qualities of the studies, overall, IVIG treatment has been shown to reduce the mortality rates in sepsis patients.
4.2.2 Immune checkpoint inhibitors
Theoretically, in the later stages of disease marked by immune suppression, ICIs can restore T cell proliferative and effector functions by inhibiting ICs, thereby improving the host’s state of immune tolerance. For instance, a recent study found that non-surviving sepsis patients had significantly increased PD-1 expression on CD4+T cells, and an increased percentage of PD1+/CD4+T cells was an independent risk factor for 28-day mortality rates (OR: 1.368; 95%CI: 0.571–0.937) (215). Hotchkiss and colleagues evaluated the safety and efficacy of the PD-L1 inhibitor BMS-936559 in sepsis patients administered in single escalating doses. The results confirmed good tolerance to BMS-936559 with an overall mortality rate of 25% for all dose treatments, and significant increases in HLA-DR expression on monocytes (> 5,000 monoclonal antibodies per cell) that persisted for more than 28 days in patients receiving single doses of 300mg and 900mg (ClinicalTrials.gov identifier: NCT02576457) (194). Zhang and others treated lymphocytes from sepsis patients in vitro with anti-PD-L1 antibodies, showing that the antibody could block PD-1/PD-L1 mediated T cell apoptosis and inhibit monocyte production of IL-10 while enhancing LPS-induced levels of TNF-α and IL-6 (ClinicalTrials.gov identifier: NCT01161745) (94). Additionally, two studies preliminarily affirmed the safety and tolerability of Nivolumab (a PD-1 inhibitor) (ClinicalTrials.gov identifier: NCT02960854; JAPIC identifier: JapicCTI-173600) (195, 196). Wu and colleagues observed that upregulated expression of TIM-3 in sepsis patients mediated the apoptosis of natural killer T cells (NKTs) and was associated with disease severity and mortality, whereas in vitro administration of α-galactosylceramide could inhibit the apoptosis of NKTs derived from sepsis patients by blocking the TIM-3/Gal-9 pathway (197). In summary, although ICIs have shown considerable promise in preclinical studies, there is currently no direct clinical evidence to suggest that ICIs provide a definitive therapeutic effect in sepsis patients.
4.2.3 Inflammatory activity factors
Direct supplementation of inflammatory cytokines or administering cytokines with immune-stimulating properties are ideal means to enhance host immunity. For instance, a study by Francois et al. showed that septic shock patients and those with severe lymphocytopenia who received 4 weeks of rhIL-7 (CYT107) treatment experienced an increase in total lymphocyte count and circulating CD4+and CD8+T cells to three to four times the baseline levels, without triggering cytokine storms, exacerbation of inflammation, or organ dysfunction (ClinicalTrials.gov identifier: NCT02640807, NCT02797431) (198). Thymosin alpha 1 (Tα1) is a highly conserved peptide found in the thymus, playing a key role in T cell maturation and differentiation. Its synthetic form has been approved by various regulatory agencies for the treatment of cancer and infectious diseases (216). A multicenter RCT reported that treatment with Tα1 in severe sepsis patients upregulated the expression of MHLA-DR, improved SOFA scores, and reduced the 28-day mortality rates from 35.0% to 26.0%, indicating that Tα1 can enhance the immune function of severe sepsis patients and reduce the 28-day all-cause mortality rates (ClinicalTrials.gov identifier: NCT00711620) (199). Li and colleagues conducted a systematic review of 12 clinical trials related to Tα1, indicating that Tα1 treatment could reduce the all-cause mortality in sepsis patients (pooled risk ratio: 0.68; 95%CI: 0.59–0.78). However, given the poor quality of the included studies and the small number of participants, these positive results should be interpreted with caution (200). In vitro administration of GM-CSF has been proven to increase the expression of MHLA-DR and the production of TNF-α in human monocytes stimulated with LPS (201), suggesting GM-CSF as a potential tool to enhance host immunity and reverse immune paralysis. Meisel and colleagues reported that patients with severe sepsis or septic shock who were in the immunosuppressive phase of sepsis and received GM-CSF treatment for 8 days showed a significant increase in MHLA-DR levels within 24 hours, returning to normal levels compared to the placebo group. Additionally, there were improvements in APACHE II scores, and a reduction in mechanical ventilation duration (ClinicalTrials.gov identifier: NCT00252915) (202). Another clinical trial reported that children with sepsis who developed multiple organ dysfunction syndrome (MODS) and were treated with GM-CSF showed increased production of TNF-α and a reduced risk of nosocomial secondary infections (ClinicalTrials.gov identifier: NCT03769844) (203). It’s indeed intriguing that Bo and colleagues, after a systematic review of 12 RCTs, found no significant correlation between GM-CSF treatment and reductions in the 14-day or 28-day mortality rates or hospital mortality rates among sepsis patients (204). This suggests that routine use of GM-CSF in sepsis patients is not supported by direct clinical evidence as a standard treatment. Research findings indicate that IFN-γ treatment can partially restore immune function in sepsis patients. Döcke and colleagues have reported that treatment with recombinant IFN-γ led to an upregulation of MHLA-DR expression in monocytes of sepsis patients and an increase in TNF-α secretion upon LPS stimulation in vitro, thereby ameliorating monocyte dysfunction (205). Similarly, Leentjens and others observed that compared to the placebo group, the IFN-γ treatment group exhibited elevated levels of TNF-α and MHLA-DR, and a decrease in the concentration of IL-10, suggesting an improvement in the immune response capabilities of these patients (ClinicalTrials.gov identifier: NCT01374711) (206).
4.3 Immunomodulatory therapy
Numerous preclinical studies have demonstrated that MSCs and their derivatives can protect against organ dysfunction and improve survival rates in septic animals (217). MSCs and their derivatives have unique advantages such as low immunogenicity, multi-directional differentiation potential, and the ability to modulate immune responses, providing a new option for the treatment of sepsis. However, the ethics, safety, effectiveness, and strategies for application and therapeutic mechanisms still need to be confirmed through extensive clinical trials (207, 208).
4.4 Registered clinical trials
As of April 19, 2024, our team entered the following keywords into ClinicalTrials.gov: “sepsis,” “Immunotherapy,” “Immunosuppressive therapy,” “Immunomodulatory therapy,” “Immune checkpoint,” “Checkpoint inhibitor,” “immunoregulation,” “antibody,” “Anakinra,” “Complement inhibitor,” “GM-CSF,” “Thymosin,” “MSCs,” “MSCs-Exo.” After screening, we compiled 35 registered clinical trials. These clinical trials use similar or different immunotherapy strategies but are all aimed at exploring the safety and/or effectiveness of immunotherapy in sepsis patients (Table 3).
5 Discussion
Despite ongoing preclinical and clinical studies further clarifying the feasibility and rationale of immunotherapy, to date, the majority of clinical trials have ended in failure, and currently, there is no universally recognized and widely applicable effective immunotherapy. We still face many challenges, including but not limited to: 1) Identifying suitable molecular targets and their corresponding biomarkers, developing engineered molecular formulations, and finding tools to monitor patient immune function; 2) Determining the safe and effective doses of related formulations, including antagonists, antibodies, cytokines, and mesenchymal stem cells; 3) Developing intervention strategies for immunotherapy, including subcutaneous, intravenous, and inhaled routes of administration; 4) The procurement and clinical management of immunomodulatory drugs to ensure their safe and effective use by the human body, as well as their appropriate handling.
Sepsis is a highly heterogeneous disease, and standardized immunotherapy only has a certain therapeutic effect on a portion of the participants, failing to benefit the majority. Therefore, in addition to classic treatment targets, some teams have used omics-based markers such as differentially expressed genes and proteins to heterogeneously categorize sepsis patients. Subsequently, they have designed and initiated stratified immunotherapies with the aim of achieving classified and precision treatment for sepsis (174, 218). For instance, Seymour et al. incorporated 29 candidate variables, including demographic characteristics, basic vital signs, markers of inflammation, and biomarkers related to organ dysfunction or injury, and employed k-means clustering to perform phenotypic analysis on sepsis patients. Their findings delineated four derived phenotypes associated with patterns of host immune response, clinical features, and treatment outcomes, enhancing our understanding of the heterogeneity in treatment effects among sepsis hosts (219). This also suggests that novel tools such as machine learning and omics analyses could be utilized for subtype or phenotype analysis in sepsis. Furthermore, considering the potential role of genetic factors in the progression of sepsis, aspects like genetic susceptibility and epigenetic modifications are garnering significant attention. For example, the genetic polymorphism rs11536889 in TLR4 is linked to an increased risk of Gram-negative bacterial infections, as well as coagulation dysfunction, renal and hepatic dysfunction or organ failure in sepsis patients (220). Similarly, the genetic polymorphism rs11568821 in PD-1 is associated with poor prognosis and a higher 90-day mortality rate in sepsis patients (221). Other apoptosis-related genes, such as rs2093266 in SERPINA4, rs1955656 in SERPINA5 (222), and rs8094315 and rs12457893 in BCL2 (223), have also been reported to correlate with acute kidney injury in sepsis hosts.
Indeed, the progression of sepsis is distinctly time-dependent, accompanied by unstable and unbalanced pro-inflammatory and anti-inflammatory responses and other complex immune mechanisms. These factors must be carefully considered when developing immunotherapy strategies (224). Additionally, we must not overlook the occurrence of IR-AEs, such as pulmonary infiltration and acute kidney injury (225), which can occur at any time during or after treatment and are difficult to distinguish from the recurrent infections caused by the early excessive inflammatory state or late immune suppression in sepsis.
6 Conclusion
Immunotherapy undoubtedly harbors significant potential for advancement in the treatment of sepsis. However, extensive research is still required to elucidate the correlations between dysfunction of immune cells related to the host in sepsis, immune suppression, chronic inflammation, and outcomes such as short-term mortality and long-term adverse prognoses. This knowledge is crucial for the development and formulation of safe, effective, and widely applicable immunotherapeutic drugs and corresponding strategies.
Author contributions
YW: Conceptualization, Writing – review & editing, Writing – original draft. LW: Conceptualization, Writing – review & editing, Writing – original draft. HK: Writing – review & editing, Conceptualization, Supervision. YL: Project administration, Writing – review & editing. YC: Project administration, Writing – review & editing. MW: Project administration, Writing – review & editing. ZD: Supervision, Writing – review & editing.
Funding
The author(s) declare that no financial support was received for the research, authorship, and/or publication of this article.
Acknowledgments
Figures in this review are made by BioRender (https://app.biorender.com/).
Conflict of interest
The authors declare that the research was conducted in the absence of any commercial or financial relationships that could be construed as a potential conflict of interest.
Publisher’s note
All claims expressed in this article are solely those of the authors and do not necessarily represent those of their affiliated organizations, or those of the publisher, the editors and the reviewers. Any product that may be evaluated in this article, or claim that may be made by its manufacturer, is not guaranteed or endorsed by the publisher.
Glossary
References
1. Singer M, Deutschman CS, Seymour CW, Shankar-Hari M, Annane D, Bauer M, et al. The third international consensus definitions for sepsis and septic shock (Sepsis-3). Jama. (2016) 315:801–10. doi: 10.1001/jama.2016.0287
2. Rudd KE, Johnson SC, Agesa KM, Shackelford KA, Tsoi D, Kievlan DR, et al. Global, regional, and national sepsis incidence and mortality, 1990–2017: analysis for the Global Burden of Disease Study. Lancet. (2020) 395:200–11. doi: 10.1016/S0140-6736(19)32989-7
3. Weng L, Xu Y, Yin P, Wang Y, Chen Y, Liu W, et al. National incidence and mortality of hospitalized sepsis in China. Crit Care. (2023) 27:84. doi: 10.1186/s13054-023-04385-x
4. Luijks ECN, van der Slikke EC, van Zanten ARH, Ter Maaten JC, Postma MJ, Hilderink HBM, et al. Societal costs of sepsis in the Netherlands. Crit Care. (2024) 28:29. doi: 10.1186/s13054-024-04816-3
5. Wiens MO, Bone JN, Kumbakumba E, Businge S, Tagoola A, Sherine SO, et al. Mortality after hospital discharge among children younger than 5 years admitted with suspected sepsis in Uganda: a prospective, multisite, observational cohort study. Lancet Child Adolesc Health. (2023) 7:555–66. doi: 10.1016/S2352-4642(23)00052-4
6. Molloy EJ, Bearer CF. Paediatric and neonatal sepsis and inflammation. Pediatr Res. (2022) 91:267–9. doi: 10.1038/s41390-021-01918-4
7. Minogue J, Schlapbach LJ, Keogh S, Gibbons K, Long D. Long-term outcomes after paediatric sepsis (LOTUS)-A protocol for an Australian cohort study. Nurs Crit Care. (2024) 29:438–43. doi: 10.1111/nicc.12938
8. Pandolfi F, Brun-Buisson C, Guillemot D, Watier L. Care pathways of sepsis survivors: sequelae, mortality and use of healthcare services in France, 2015–2018. Crit Care. (2023) 27:438. doi: 10.1186/s13054-023-04726-w
9. Seymour CW, Gesten F, Prescott HC, Friedrich ME, Iwashyna TJ, Phillips GS, et al. Time to treatment and mortality during mandated emergency care for sepsis. N Engl J Med. (2017) 376:2235–44. doi: 10.1056/NEJMoa1703058
10. Zampieri FG, Bagshaw SM, Semler MW. Fluid therapy for critically ill adults with sepsis: A review. Jama. (2023) 329:1967–80. doi: 10.1001/jama.2023.7560
11. Evans L, Rhodes A, Alhazzani W, Antonelli M, Coopersmith CM, French C, et al. Surviving sepsis campaign: international guidelines for management of sepsis and septic shock 2021. Intensive Care Med. (2021) 47:1181–247. doi: 10.1007/s00134-021-06506-y
12. Obonyo NG, Olupot-Olupot P, Mpoya A, Nteziyaremye J, Chebet M, Uyoga S, et al. A clinical and physiological prospective observational study on the management of pediatric shock in the post-fluid expansion as supportive therapy trial era. Pediatr Crit Care Med. (2022) 23:502–13. doi: 10.1097/PCC.0000000000002968
13. Kadri SS, Lai YL, Warner S, Strich JR, Babiker A, Ricotta EE, et al. Inappropriate empirical antibiotic therapy for bloodstream infections based on discordant in-vitro susceptibilities: a retrospective cohort analysis of prevalence, predictors, and mortality risk in US hospitals. Lancet Infect Dis. (2021) 21:241–51. doi: 10.1016/S1473-3099(20)30477-1
14. Woods-Hill CZ, Colantuoni EA, Koontz DW, Voskertchian A, Xie A, Thurm C, et al. Association of diagnostic stewardship for blood cultures in critically ill children with culture rates, antibiotic use, and patient outcomes: results of the bright STAR collaborative. JAMA Pediatr. (2022) 176:690–8. doi: 10.1001/jamapediatrics.2022.1024
15. Russell NJ, Stöhr W, Plakkal N, Cook A, Berkley JA, Adhisivam B, et al. Patterns of antibiotic use, pathogens, and prediction of mortality in hospitalized neonates and young infants with sepsis: A global neonatal sepsis observational cohort study (NeoOBS). PloS Med. (2023) 20:e1004179. doi: 10.1371/journal.pmed.1004179
16. Vincent JL. Current sepsis therapeutics. EBioMedicine. (2022) 86:104318. doi: 10.1016/j.ebiom.2022.104318
18. Sanmarco LM, Rone JM, Polonio CM, Fernandez Lahore G, Giovannoni F, Ferrara K, et al. Lactate limits CNS autoimmunity by stabilizing HIF-1α in dendritic cells. Nature. (2023) 620:881–9. doi: 10.1038/s41586-023-06409-6
19. Humrich JY, Cacoub P, Rosenzwajg M, Pitoiset F, Pham HP, Guidoux J, et al. Low-dose interleukin-2 therapy in active systemic lupus erythematosus (LUPIL-2): a multicentre, double-blind, randomised and placebo-controlled phase II trial. Ann Rheum Dis. (2022) 81:1685–94. doi: 10.1136/ard-2022-222501
20. Dong S, Hiam-Galvez KJ, Mowery CT, Herold KC, Gitelman SE, Esensten JH, et al. The effect of low-dose IL-2 and Treg adoptive cell therapy in patients with type 1 diabetes. JCI Insight. (2021) 6:e147474. doi: 10.1172/jci.insight.147474
21. Granit V, Benatar M, Kurtoglu M, Miljković MD, Chahin N, Sahagian G, et al. Safety and clinical activity of autologous RNA chimeric antigen receptor T-cell therapy in myasthenia gravis (MG-001): a prospective, multicentre, open-label, non-randomised phase 1b/2a study. Lancet Neurol. (2023) 22:578–90. doi: 10.1016/S1474-4422(23)00194-1
22. Qin C, Tian DS, Zhou LQ, Shang K, Huang L, Dong MH, et al. Anti-BCMA CAR T-cell therapy CT103A in relapsed or refractory AQP4-IgG seropositive neuromyelitis optica spectrum disorders: phase 1 trial interim results. Signal Transduct Target Ther. (2023) 8:5. doi: 10.1038/s41392-022-01278-3
23. Shi N, Zhou Y, Liu Y, Zhang R, Jiang X, Ren C, et al. PD-1/LAG-3 bispecific antibody potentiates T cell activation and increases antitumor efficacy. Front Immunol. (2022) 13:1047610. doi: 10.3389/fimmu.2022.1047610
24. Liu D, Qi X, Wei X, Zhao L, Wang X, Li S, et al. A Novel Her2/VEGFR2/CD3 trispecific antibody with an optimal structural design showed improved T-cell-redirecting antitumor efficacy. Theranostics. (2022) 12:7788–803. doi: 10.7150/thno.75037
25. Wykoff CC, Abreu F, Adamis AP, Basu K, Eichenbaum DA, Haskova Z, et al. Efficacy, durability, and safety of intravitreal faricimab with extended dosing up to every 16 weeks in patients with diabetic macular oedema (YOSEMITE and RHINE): two randomised, double-masked, phase 3 trials. Lancet. (2022) 399:741–55. doi: 10.1016/S0140-6736(22)00018-6
26. Wallis RS, O'Garra A, Sher A, Wack A. Host-directed immunotherapy of viral and bacterial infections: past, present and future. Nat Rev Immunol. (2023) 23:121–33. doi: 10.1038/s41577-022-00734-z
27. Lee DSW, Rojas OL, Gommerman JL. B cell depletion therapies in autoimmune disease: advances and mechanistic insights. Nat Rev Drug Discovery. (2021) 20:179–99. doi: 10.1038/s41573-020-00092-2
28. Wei L, Xiang Z, Zou Y. The role of NKG2D and its ligands in autoimmune diseases: new targets for immunotherapy. Int J Mol Sci. (2023) 24:17545. doi: 10.3390/ijms242417545
29. Barbé-Tuana F, Funchal G, Schmitz CRR, Maurmann RM, Bauer ME. The interplay between immunosenescence and age-related diseases. Semin Immunopathol. (2020) 42:545–57. doi: 10.1007/s00281-020-00806-z
30. Smit V, de Mol J, Schaftenaar FH, Depuydt MAC, Postel RJ, Smeets D, et al. Single-cell profiling reveals age-associated immunity in atherosclerosis. Cardiovasc Res. (2023) 119:2508–21. doi: 10.1093/cvr/cvad099
31. Sim BC, Kang YE, You SK, Lee SE, Nga HT, Lee HY, et al. Hepatic T-cell senescence and exhaustion are implicated in the progression of fatty liver disease in patients with type 2 diabetes and mouse model with nonalcoholic steatohepatitis. Cell Death Dis. (2023) 14:618. doi: 10.1038/s41419-023-06146-8
32. Tramutola A, Abate G, Lanzillotta C, Triani F, Barone E, Iavarone F, et al. Protein nitration profile of CD3(+) lymphocytes from Alzheimer disease patients: Novel hints on immunosenescence and biomarker detection. Free Radic Biol Med. (2018) 129:430–9. doi: 10.1016/j.freeradbiomed.2018.10.414
33. Steinhagen F, Schmidt SV, Schewe JC, Peukert K, Klinman DM, Bode. Immunotherapy in sepsis - brake or accelerate? Pharmacol Ther. (2020) 208:107476. doi: 10.1016/j.pharmthera.2020.107476
34. Barichello T, Generoso JS, Singer M, Dal-Pizzol F. Biomarkers for sepsis: more than just fever and leukocytosis-a narrative review. Crit Care. (2022) 26:14. doi: 10.1186/s13054-021-03862-5
35. Kong C, Zhu Y, Xie X, Wu J, Qian M. Six potential biomarkers in septic shock: a deep bioinformatics and prospective observational study. Front Immunol. (2023) 14:1184700. doi: 10.3389/fimmu.2023.1184700
36. Cavaillon JM. During sepsis and COVID-19, the pro-inflammatory and anti-inflammatory responses are concomitant. Clin Rev Allergy Immunol. (2023) 65:183–7. doi: 10.1007/s12016-023-08965-1
37. Brands X, Haak BW, Klarenbeek AM, Otto NA, Faber DR, Lutter R, et al. Concurrent immune suppression and hyperinflammation in patients with community-acquired pneumonia. Front Immunol. (2020) 11:796. doi: 10.3389/fimmu.2020.00796
38. Chen LR, Hui Y, Lu L, Wang XX, Zhang XM, Wang HX. CD1d-dependent natural killer T-cells inactivation aggravates sepsis-induced myocardial injury via T lymphocytes infiltration and IL-6 production in mice. Int Immunopharmacol. (2023) 120:110256. doi: 10.1016/j.intimp.2023.110256
39. Zhang Y, Li B, Ning B. Evaluating IL-6 and IL-10 as rapid diagnostic tools for Gram-negative bacteria and as disease severity predictors in pediatric sepsis patients in the intensive care unit. Front Immunol. (2022) 13:1043968. doi: 10.3389/fimmu.2022.1043968
40. Dhudasia MB, Benitz WE, Flannery DD, Christ L, Rub D, Remaschi G, et al. Diagnostic performance and patient outcomes with C-reactive protein use in early-onset sepsis evaluations. J Pediatr. (2023) 256:98–104.e6. doi: 10.1016/j.jpeds.2022.12.007
41. Ling H, Chen M, Dai J, Zhong H, Chen R, Shi F. Evaluation of qSOFA combined with inflammatory mediators for diagnosing sepsis and predicting mortality among emergency department. Clin Chim Acta. (2023) 544:117352. doi: 10.1016/j.cca.2023.117352
42. Fux AC, Casonato Melo C, Michelini S, Swartzwelter BJ, Neusch A, Italiani P, et al. Heterogeneity of lipopolysaccharide as source of variability in bioassays and LPS-binding proteins as remedy. Int J Mol Sci. (2023) 24:8395. doi: 10.3390/ijms24098395
43. Cheng TL, Lin YS, Hong YK, Ma CY, Tsai HW, Shi GY, et al. Role of tumor endothelial marker 1 (Endosialin/CD248) lectin-like domain in lipopolysaccharide-induced macrophage activation and sepsis in mice. Transl Res. (2021) 232:150–62. doi: 10.1016/j.trsl.2021.03.009
44. Fischer S, Stegmann F, Gnanapragassam VS, Lepenies B. From structure to function - Ligand recognition by myeloid C-type lectin receptors. Comput Struct Biotechnol J. (2022) 20:5790–812. doi: 10.1016/j.csbj.2022.10.019
45. Xu J, Zhang X, Pelayo R, Monestier M, Ammollo CT, Semeraro F, et al. Extracellular histones are major mediators of death in sepsis. Nat Med. (2009) 15:1318–21. doi: 10.1038/nm.2053
46. Yu F, Tian W, Dong J. Anagliptin prevents lipopolysaccharide (LPS)- induced inflammation and activation of macrophages. Int Immunopharmacol. (2022) 104:108514. doi: 10.1016/j.intimp.2021.108514
47. Ueno K, Nomura Y, Morita Y, Kawano Y. Prednisolone suppresses the extracellular release of HMGB-1 and associated inflammatory pathways in kawasaki disease. Front Immunol. (2021) 12:640315. doi: 10.3389/fimmu.2021.640315
48. Murao A, Aziz M, Wang H, Brenner M, Wang P. Release mechanisms of major DAMPs. Apoptosis. (2021) 26:152–62. doi: 10.1007/s10495-021-01663-3
49. Zhang SY, Xu QP, Shi LN, Li SW, Wang WH, Wang QQ, et al. Soluble CD4 effectively prevents excessive TLR activation of resident macrophages in the onset of sepsis. Signal Transduct Target Ther. (2023) 8:236. doi: 10.1038/s41392-023-01438-z
50. Duan T, Du Y, Xing C, Wang HY, Wang RF. Toll-like receptor signaling and its role in cell-mediated immunity. Front Immunol. (2022) 13:812774. doi: 10.3389/fimmu.2022.812774
51. Ohto U. Activation and regulation mechanisms of NOD-like receptors based on structural biology. Front Immunol. (2022) 13:953530. doi: 10.3389/fimmu.2022.953530
52. Pei G, Dorhoi A. NOD-like receptors: guards of cellular homeostasis perturbation during infection. Int J Mol Sci. (2021) 22:6714. doi: 10.3390/ijms22136714
53. Jiang Y, Zhang H, Wang J, Chen J, Guo Z, Liu Y, et al. Exploiting RIG-I-like receptor pathway for cancer immunotherapy. J Hematol Oncol. (2023) 16:8. doi: 10.1186/s13045-023-01405-9
54. van der Donk LEH, Bermejo-Jambrina M, van Hamme JL, Volkers MMW, van Nuenen AC, Kootstra NA, et al. SARS-CoV-2 suppresses TLR4-induced immunity by dendritic cells via C-type lectin receptor DC-SIGN. PloS Pathog. (2023) 19:e1011735. doi: 10.1371/journal.ppat.1011735
55. Drouin M, Saenz J, Chiffoleau E. C-type lectin-like receptors: head or tail in cell death immunity. Front Immunol. (2020) 11:251. doi: 10.3389/fimmu.2020.00251
56. Kumar V. The trinity of cGAS, TLR9, and ALRs guardians of the cellular galaxy against host-derived self-DNA. Front Immunol. (2020) 11:624597. doi: 10.3389/fimmu.2020.624597
57. Sekheri M, El Kebir D, Edner N, Filep JG. 15-Epi-LXA(4) and 17-epi-RvD1 restore TLR9-mediated impaired neutrophil phagocytosis and accelerate resolution of lung inflammation. Proc Natl Acad Sci U S A. (2020) 117:7971–80. doi: 10.1073/pnas.1920193117
58. Rosa BA, Ahmed M, Singh DK, Choreño-Parra JA, Cole J, Jiménez-Álvarez LA, et al. IFN signaling and neutrophil degranulation transcriptional signatures are induced during SARS-CoV-2 infection. Commun Biol. (2021) 4:290. doi: 10.1038/s42003-021-01829-4
59. Zhang H, Wang Y, Qu M, Li W, Wu D, Cata JP, et al. Neutrophil, neutrophil extracellular traps and endothelial cell dysfunction in sepsis. Clin Transl Med. (2023) 13:e1170. doi: 10.1002/ctm2.1170
60. Metzler KD, Fuchs TA, Nauseef WM, Reumaux D, Roesler J, Schulze I, et al. Myeloperoxidase is required for neutrophil extracellular trap formation: implications for innate immunity. Blood. (2011) 117:953–9. doi: 10.1182/blood-2010-06-290171
61. Chen X, Liu Y, Gao Y, Shou S, Chai Y. The roles of macrophage polarization in the host immune response to sepsis. Int Immunopharmacol. (2021) 96:107791. doi: 10.1016/j.intimp.2021.107791
62. Tan F, Cao Y, Zheng L, Wang T, Zhao S, Chen J, et al. Diabetes exacerbated sepsis-induced intestinal injury by promoting M1 macrophage polarization via miR-3061/Snail1 signaling. Front Immunol. (2022) 13:922614. doi: 10.3389/fimmu.2022.922614
63. Wang A, Kang X, Wang J, Zhang S. IFIH1/IRF1/STAT1 promotes sepsis associated inflammatory lung injury via activating macrophage M1 polarization. Int Immunopharmacol. (2023) 114:109478. doi: 10.1016/j.intimp.2022.109478
64. Yao RQ, Li ZX, Wang LX, Li YX, Zheng LY, Dong N, et al. Single-cell transcriptome profiling of the immune space-time landscape reveals dendritic cell regulatory program in polymicrobial sepsis. Theranostics. (2022) 12:4606–28. doi: 10.7150/thno.72760
65. Yazal T, Lee PY, Chen PR, Chen IC, Liu PL, Chen YR, et al. Kurarinone exerts anti-inflammatory effect via reducing ROS production, suppressing NLRP3 inflammasome, and protecting against LPS-induced sepsis. BioMed Pharmacother. (2023) 167:115619. doi: 10.1016/j.biopha.2023.115619
66. Andrades M, Morina A, Spasić S, Spasojević I. Bench-to-bedside review: sepsis - from the redox point of view. Crit Care. (2011) 15:230. doi: 10.1186/cc10334
67. Wang L, Cao Y, Gorshkov B, Zhou Y, Yang Q, Xu J, et al. Ablation of endothelial Pfkfb3 protects mice from acute lung injury in LPS-induced endotoxemia. Pharmacol Res. (2019) 146:104292. doi: 10.1016/j.phrs.2019.104292
68. de Nooijer AH, Kotsaki A, Kranidioti E, Kox M, Pickkers P, Toonen EJM, et al. Complement activation in severely ill patients with sepsis: no relationship with inflammation and disease severity. Crit Care. (2023) 27:63. doi: 10.1186/s13054-023-04344-6
69. Chung HY, Wickel J, Hahn N, Mein N, Schwarzbrunn M, Koch P, et al. Microglia mediate neurocognitive deficits by eliminating C1q-tagged synapses in sepsis-associated encephalopathy. Sci Adv. (2023) 9:eabq7806. doi: 10.1126/sciadv.abq7806
70. Maneta E, Aivalioti E, Tual-Chalot S, Emini Veseli B, Gatsiou A, Stamatelopoulos K, et al. Endothelial dysfunction and immunothrombosis in sepsis. Front Immunol. (2023) 14:1144229. doi: 10.3389/fimmu.2023.1144229
71. Iba T, Levy JH, Warkentin TE, Thachil J, van der Poll T, Levi M. Diagnosis and management of sepsis-induced coagulopathy and disseminated intravascular coagulation. J Thromb Haemost. (2019) 17:1989–94. doi: 10.1111/jth.14578
72. Merle NS, Noe R, Halbwachs-Mecarelli L, Fremeaux-Bacchi V, Roumenina LT. Complement system part II: role in immunity. Front Immunol. (2015) 6:257. doi: 10.3389/fimmu.2015.00257
73. Karasu E, Nilsson B, Köhl J, Lambris JD, Huber-Lang M. Targeting complement pathways in polytrauma- and sepsis-induced multiple-organ dysfunction. Front Immunol. (2019) 10:543. doi: 10.3389/fimmu.2019.00543
74. Liu N, Jiang C, Cai P, Shen Z, Sun W, Xu H, et al. Single-cell analysis of COVID-19, sepsis, and HIV infection reveals hyperinflammatory and immunosuppressive signatures in monocytes. Cell Rep. (2021) 37:109793. doi: 10.1016/j.celrep.2021.109793
75. Hilligan KL, Ronchese F. Antigen presentation by dendritic cells and their instruction of CD4+ T helper cell responses. Cell Mol Immunol. (2020) 17:587–99. doi: 10.1038/s41423-020-0465-0
76. Muntjewerff EM, Meesters LD, van den Bogaart G. Antigen cross-presentation by macrophages. Front Immunol. (2020) 11:1276. doi: 10.3389/fimmu.2020.01276
77. Ruterbusch M, Pruner KB, Shehata L, Pepper M. In vivo CD4(+) T cell differentiation and function: revisiting the th1/th2 paradigm. Annu Rev Immunol. (2020) 38:705–25. doi: 10.1146/annurev-immunol-103019-085803
78. Ma C, Liu H, Yang S, Li H, Liao X, Kang Y. The emerging roles and therapeutic potential of B cells in sepsis. Front Pharmacol. (2022) 13:1034667. doi: 10.3389/fphar.2022.1034667
79. Bosmann M, Ward PA. The inflammatory response in sepsis. Trends Immunol. (2013) 34:129–36. doi: 10.1016/j.it.2012.09.004
80. Torres LK, Pickkers P, van der Poll T. Sepsis-induced immunosuppression. Annu Rev Physiol. (2022) 84:157–81. doi: 10.1146/annurev-physiol-061121-040214
81. Guignant C, Lepape A, Huang X, Kherouf H, Denis L, Poitevin F, et al. Programmed death-1 levels correlate with increased mortality, nosocomial infection and immune dysfunctions in septic shock patients. Crit Care. (2011) 15:R99. doi: 10.1186/cc10112
82. Heffernan DS, Monaghan SF, Thakkar RK, Machan JT, Cioffi WG, Ayala A. Failure to normalize lymphopenia following trauma is associated with increased mortality, independent of the leukocytosis pattern. Crit Care. (2012) 16:R12. doi: 10.1186/cc11157
83. Jiao Y, Zhang T, Zhang C, Ji H, Tong X, Xia R, et al. Exosomal miR-30d-5p of neutrophils induces M1 macrophage polarization and primes macrophage pyroptosis in sepsis-related acute lung injury. Crit Care. (2021) 25:356. doi: 10.1186/s13054-021-03775-3
84. Yuan Y, Hua L, Zhou J, Liu D, Ouyang F, Chen X, et al. The effect of artesunate to reverse CLP-induced sepsis immunosuppression mice with secondary infection is tightly related to reducing the apoptosis of T cells via decreasing the inhibiting receptors and activating MAPK/ERK pathway. Int Immunopharmacol. (2023) 124:110917. doi: 10.1016/j.intimp.2023.110917
85. Ma Y, Cheng Z, Zheng Y, Wang W, He S, Zhou X, et al. Low dose of esmolol attenuates sepsis-induced immunosuppression via modulating T-lymphocyte apoptosis and differentiatioN. Shock. (2023) 59:771–8. doi: 10.1097/SHK.0000000000002104
86. Tsuji N, Tsuji T, Yamashita T, Hayase N, Hu X, Yuen PS, et al. BAM15 treats mouse sepsis and kidney injury, linking mortality, mitochondrial DNA, tubule damage, and neutrophils. J Clin Invest. (2023) 133:e152401. doi: 10.1172/JCI152401
87. Condotta SA, Rai D, James BR, Griffith TS, Badovinac VP. Sustained and incomplete recovery of naive CD8+ T cell precursors after sepsis contributes to impaired CD8+ T cell responses to infection. J Immunol. (2013) 190:1991–2000. doi: 10.4049/jimmunol.1202379
88. Hotchkiss RS, Swanson PE, Freeman BD, Tinsley KW, Cobb JP, Matuschak GM, et al. Apoptotic cell death in patients with sepsis, shock, and multiple organ dysfunction. Crit Care Med. (1999) 27:1230–51. doi: 10.1097/00003246-199907000-00002
89. Hotchkiss RS, Schmieg RE Jr., Swanson PE, Freeman BD, Tinsley KW, Cobb JP, et al. Rapid onset of intestinal epithelial and lymphocyte apoptotic cell death in patients with trauma and shock. Crit Care Med. (2000) 28:3207–17. doi: 10.1097/00003246-200009000-00016
90. Cao C, Yu M, Chai Y. Pathological alteration and therapeutic implications of sepsis-induced immune cell apoptosis. Cell Death Dis. (2019) 10:782. doi: 10.1038/s41419-019-2015-1
91. Darden DB, Dong X, Brusko MA, Kelly L, Fenner B, Rincon JC, et al. A novel single cell RNA-seq analysis of non-myeloid circulating cells in late sepsis. Front Immunol. (2021) 12:696536. doi: 10.3389/fimmu.2021.696536
92. Inoue S, Suzuki-Utsunomiya K, Okada Y, Taira T, Iida Y, Miura N, et al. Reduction of immunocompetent T cells followed by prolonged lymphopenia in severe sepsis in the elderly. Crit Care Med. (2013) 41:810–9. doi: 10.1097/CCM.0b013e318274645f
93. Boomer JS, To K, Chang KC, Takasu O, Osborne DF, Walton AH, et al. Immunosuppression in patients who die of sepsis and multiple organ failure. Jama. (2011) 306:2594–605. doi: 10.1001/jama.2011.1829
94. Zhang Y, Li J, Lou J, Zhou Y, Bo L, Zhu J, et al. Upregulation of programmed death-1 on T cells and programmed death ligand-1 on monocytes in septic shock patients. Crit Care. (2011) 15:R70. doi: 10.1186/cc10059
95. Monaghan SF, Thakkar RK, Tran ML, Huang X, Cioffi WG, Ayala A, et al. Programmed death 1 expression as a marker for immune and physiological dysfunction in the critically ill surgical patient. Shock. (2012) 38:117–22. doi: 10.1097/SHK.0b013e31825de6a3
96. Lozano-Rodríguez R, Avendaño-Ortíz J, Montalbán-Hernández K, Ruiz-Rodríguez JC, Ferrer R, Martín-Quirós A, et al. The prognostic impact of SIGLEC5-induced impairment of CD8(+) T cell activation in sepsis. EBioMedicine. (2023) 97:104841. doi: 10.1016/j.ebiom.2023.104841
97. Shankar-Hari M, Fear D, Lavender P, Mare T, Beale R, Swanson C, et al. Activation-associated accelerated apoptosis of memory B cells in critically ill patients with sepsis. Crit Care Med. (2017) 45:875–82. doi: 10.1097/CCM.0000000000002380
98. Gustave CA, Gossez M, Demaret J, Rimmelé T, Lepape A, Malcus C, et al. Septic shock shapes B cell response toward an exhausted-like/immunoregulatory profile in patients. J Immunol. (2018) 200:2418–25. doi: 10.4049/jimmunol.1700929
99. Guisset O, Dilhuydy MS, Thiébaut R, Lefèvre J, Camou F, Sarrat A, et al. Decrease in circulating dendritic cells predicts fatal outcome in septic shock. Intensive Care Med. (2007) 33:148–52. doi: 10.1007/s00134-006-0436-7
100. Juskewitch JE, Abraham RS, League SC, Jenkins SM, Smith CY, Enders FT, et al. Monocyte HLA-DR expression and neutrophil CD64 expression as biomarkers of infection in critically ill neonates and infants. Pediatr Res. (2015) 78:683–90. doi: 10.1038/pr.2015.164
101. Cazalis MA, Friggeri A, Cavé L, Demaret J, Barbalat V, Cerrato E, et al. Decreased HLA-DR antigen-associated invariant chain (CD74) mRNA expression predicts mortality after septic shock. Crit Care. (2013) 17:R287. doi: 10.1186/cc13150
102. Joshi I, Carney WP, Rock EP. Utility of monocyte HLA-DR and rationale for therapeutic GM-CSF in sepsis immunoparalysis. Front Immunol. (2023) 14:1130214. doi: 10.3389/fimmu.2023.1130214
103. Qi X, Yu Y, Sun R, Huang J, Liu L, Yang Y, et al. Identification and characterization of neutrophil heterogeneity in sepsis. Crit Care. (2021) 25:50. doi: 10.1186/s13054-021-03481-0
104. Siemińska I, Węglarczyk K, Surmiak M, Kurowska-Baran D, Sanak M, Siedlar M, et al. Mild and asymptomatic COVID-19 convalescents present long-term endotype of immunosuppression associated with neutrophil subsets possessing regulatory functions. Front Immunol. (2021) 12:748097. doi: 10.3389/fimmu.2021.748097
105. Kim YS, Jeong YS, Bae GH, Kang JH, Lee M, Zabel BA, et al. CD200R(high) neutrophils with dysfunctional autophagy establish systemic immunosuppression by increasing regulatory T cells. Cell Mol Immunol. (2024) 21:349–61. doi: 10.1038/s41423-024-01136-y
106. Grimaldi D, Louis S, Pène F, Sirgo G, Rousseau C, Claessens YE, et al. Profound and persistent decrease of circulating dendritic cells is associated with ICU-acquired infection in patients with septic shock. Intensive Care Med. (2011) 37:1438–46. doi: 10.1007/s00134-011-2306-1
107. Lu ZQ, Zhang C, Zhao LJ, Dong W, Lv L, Lu Y, et al. Matrix metalloproteinase-8 regulates dendritic cell tolerance in late polymicrobial sepsis via the nuclear factor kappa-B p65/β-catenin pathway. Burns Trauma. (2024) 12:tkad025. doi: 10.1093/burnst/tkad025
108. Miao S, Chang Z, Gu B, Jiang J, Pei F, Liu Y, et al. GENERATION OF TOLEROGENIC DENDRITIC CELLS UNDER THE PERSISTENT INFLAMMATION STIMULATION. Shock. (2024) 61:454–64. doi: 10.1097/SHK.0000000000002318
109. Lapko N, Zawadka M, Polosak J, Worthen GS, Danet-Desnoyers G, Puzianowska-Kuźnicka M, et al. Long-term monocyte dysfunction after sepsis in humanized mice is related to persisted activation of macrophage-colony stimulation factor (M-CSF) and demethylation of PU.1, and it can be reversed by blocking M-CSF in vitro or by transplanting naïve autologous stem cells in vivo. Front Immunol. (2017) 8:401. doi: 10.3389/fimmu.2017.00401
110. Baudesson de Chanville C, Chousterman BG, Hamon P, Laviron M, Guillou N, Loyher PL, et al. Sepsis triggers a late expansion of functionally impaired tissue-vascular inflammatory monocytes during clinical recovery. Front Immunol. (2020) 11:675. doi: 10.3389/fimmu.2020.00675
111. Drewry AM, Ablordeppey EA, Murray ET, Dalton CM, Fuller BM, Kollef MH, et al. Monocyte function and clinical outcomes in febrile and afebrile patients with severe sepsis. Shock. (2018) 50:381–7. doi: 10.1097/SHK.0000000000001083
112. Fabri A, Kandara K, Coudereau R, Gossez M, Abraham P, Monard C, et al. Characterization of circulating IL-10-producing cells in septic shock patients: A proof of concept study. Front Immunol. (2020) 11:615009. doi: 10.3389/fimmu.2020.615009
113. Guérin E, Orabona M, Raquil MA, Giraudeau B, Bellier R, Gibot S, et al. Circulating immature granulocytes with T-cell killing functions predict sepsis deterioration*. Crit Care Med. (2014) 42:2007–18. doi: 10.1097/CCM.0000000000000344
114. Pillay J, Kamp VM, van Hoffen E, Visser T, Tak T, Lammers JW, et al. A subset of neutrophils in human systemic inflammation inhibits T cell responses through Mac-1. J Clin Invest. (2012) 122:327–36. doi: 10.1172/JCI57990
115. Kwok AJ, Allcock A, Ferreira RC, Cano-Gamez E, Smee M, Burnham KL, et al. Neutrophils and emergency granulopoiesis drive immune suppression and an extreme response endotype during sepsis. Nat Immunol. (2023) 24:767–79. doi: 10.1038/s41590-023-01490-5
116. Roquilly A, Villadangos JA. The role of dendritic cell alterations in susceptibility to hospital-acquired infections during critical-illness related immunosuppression. Mol Immunol. (2015) 68:120–3. doi: 10.1016/j.molimm.2015.06.030
117. Yao RQ, Zhao PY, Li ZX, Liu YY, Zheng LY, Duan Y, et al. Single-cell transcriptome profiling of sepsis identifies HLA-DR(low)S100A(high) monocytes with immunosuppressive function. Mil Med Res. (2023) 10:27. doi: 10.1186/s40779-023-00462-y
118. Quirant-Sánchez B, Plans-Galván O, Lucas E, Argudo E, Martinez-Cáceres EM, Arméstar F. HLA-DR expression on monocytes and sepsis index are useful in predicting sepsis. Biomedicines. (2023) 11:1836. doi: 10.3390/biomedicines11071836
119. Bourgoin P, Taspinar R, Gossez M, Venet F, Delwarde B, Rimmelé T, et al. Toward monocyte HLA-DR bedside monitoring: A proof-of-concept study. Shock. (2021) 55:782–9. doi: 10.1097/SHK.0000000000001673
120. Hagedoorn NN, Kolukirik P, Nagtzaam NMA, Nieboer D, Verbruggen S, Joosten KF, et al. Association of monocyte HLA-DR expression over time with secondary infection in critically ill children: a prospective observational study. Eur J Pediatr. (2022) 181:1133–42. doi: 10.1007/s00431-021-04313-7
121. Monneret G, Lepape A, Voirin N, Bohé J, Venet F, Debard AL, et al. Persisting low monocyte human leukocyte antigen-DR expression predicts mortality in septic shock. Intensive Care Med. (2006) 32:1175–83. doi: 10.1007/s00134-006-0204-8
122. Schrijver IT, Théroude C, Roger T. Myeloid-derived suppressor cells in sepsis. Front Immunol. (2019) 10:327. doi: 10.3389/fimmu.2019.00327
123. Wu Y, Yi M, Niu M, Mei Q, Wu K. Myeloid-derived suppressor cells: an emerging target for anticancer immunotherapy. Mol Cancer. (2022) 21:184. doi: 10.1186/s12943-022-01657-y
124. Cavaillon JM, Adib-Conquy M. Bench-to-bedside review: endotoxin tolerance as a model of leukocyte reprogramming in sepsis. Crit Care. (2006) 10:233. doi: 10.1186/cc5055
125. Biswas SK, Lopez-Collazo E. Endotoxin tolerance: new mechanisms, molecules and clinical significance. Trends Immunol. (2009) 30:475–87. doi: 10.1016/j.it.2009.07.009
126. Medvedev AE, Kopydlowski KM, Vogel SN. Inhibition of lipopolysaccharide-induced signal transduction in endotoxin-tolerized mouse macrophages: dysregulation of cytokine, chemokine, and toll-like receptor 2 and 4 gene expression. J Immunol. (2000) 164:5564–74. doi: 10.4049/jimmunol.164.11.5564
127. Chan C, Li L, McCall CE, Yoza BK. Endotoxin tolerance disrupts chromatin remodeling and NF-kappaB transactivation at the IL-1beta promoter. J Immunol. (2005) 175:461–8. doi: 10.4049/jimmunol.175.1.461
128. Jia L, Lu J, Zhou Y, Tao Y, Xu H, Zheng W, et al. Tolerogenic dendritic cells induced the enrichment of CD4(+)Foxp3(+) regulatory T cells via TGF-β in mesenteric lymph nodes of murine LPS-induced tolerance model. Clin Immunol. (2018) 197:118–29. doi: 10.1016/j.clim.2018.09.010
129. Draisma A, Pickkers P, Bouw MP, van der Hoeven JG. Development of endotoxin tolerance in humans in vivo. Crit Care Med. (2009) 37:1261–7. doi: 10.1097/CCM.0b013e31819c3c67
130. Wolk K, Döcke WD, von Baehr V, Volk HD, Sabat R. Impaired antigen presentation by human monocytes during endotoxin tolerance. Blood. (2000) 96:218–23. doi: 10.1182/blood.V96.1.218.013k04_218_223
131. Shalova IN, Lim JY, Chittezhath M, Zinkernagel AS, Beasley F, Hernández-Jiménez E, et al. Human monocytes undergo functional re-programming during sepsis mediated by hypoxia-inducible factor-1α. Immunity. (2015) 42:484–98. doi: 10.1016/j.immuni.2015.02.001
132. Avendaño-Ortiz J, Maroun-Eid C, Martín-Quirós A, Toledano V, Cubillos-Zapata C, Gómez-Campelo P, et al. PD-L1 overexpression during endotoxin tolerance impairs the adaptive immune response in septic patients via HIF1α. J Infect Dis. (2018) 217:393–404. doi: 10.1093/infdis/jix279
133. Davenport EE, Burnham KL, Radhakrishnan J, Humburg P, Hutton P, Mills TC, et al. Genomic landscape of the individual host response and outcomes in sepsis: a prospective cohort study. Lancet Respir Med. (2016) 4:259–71. doi: 10.1016/S2213-2600(16)00046-1
134. Scicluna BP, van Vught LA, Zwinderman AH, Wiewel MA, Davenport EE, Burnham KL, et al. Classification of patients with sepsis according to blood genomic endotype: a prospective cohort study. Lancet Respir Med. (2017) 5:816–26. doi: 10.1016/S2213-2600(17)30294-1
135. Antcliffe DB, Burnham KL, Al-Beidh F, Santhakumaran S, Brett SJ, Hinds CJ, et al. Transcriptomic signatures in sepsis and a differential response to steroids. From the VANISH randomized trial. Am J Respir Crit Care Med. (2019) 199:980–6. doi: 10.1164/rccm.201807-1419OC
136. Chavan SS, Pavlov VA, Tracey KJ. Mechanisms and therapeutic relevance of neuro-immune communication. Immunity. (2017) 46:927–42. doi: 10.1016/j.immuni.2017.06.008
137. Wu D, Shi Y, Zhang H, Miao C. Epigenetic mechanisms of Immune remodeling in sepsis: targeting histone modification. Cell Death Dis. (2023) 14:112. doi: 10.1038/s41419-023-05656-9
138. Stienstra R, Netea-Maier RT, Riksen NP, Joosten LAB, Netea MG. Specific and complex reprogramming of cellular metabolism in myeloid cells during innate immune responses. Cell Metab. (2017) 26:142–56. doi: 10.1016/j.cmet.2017.06.001
139. Cheng SC, Scicluna BP, Arts RJ, Gresnigt MS, Lachmandas E, Giamarellos-Bourboulis EJ, et al. Broad defects in the energy metabolism of leukocytes underlie immunoparalysis in sepsis. Nat Immunol. (2016) 17:406–13. doi: 10.1038/ni.3398
140. Zou R, Tao J, Qiu J, Lu H, Wu J, Zhu H, et al. DNA-PKcs promotes sepsis-induced multiple organ failure by triggering mitochondrial dysfunction. J Adv Res. (2022) 41:39–48. doi: 10.1016/j.jare.2022.01.014
141. Martínez-García JJ, Martínez-Banaclocha H, Angosto-Bazarra D, de Torre-Minguela C, Baroja-Mazo A, Alarcón-Vila C, et al. P2X7 receptor induces mitochondrial failure in monocytes and compromises NLRP3 inflammasome activation during sepsis. Nat Commun. (2019) 10:2711. doi: 10.1038/s41467-019-10626-x
142. Senousy SR, El-Daly M, Ibrahim ARN, Khalifa MMA, Ahmed AF. Effect of celecoxib and infliximab against multiple organ damage induced by sepsis in rats: A comparative study. Biomedicines. (2022) 10:1613. doi: 10.3390/biomedicines10071613
143. Ibrahim YF, Moussa RA, Bayoumi AMA, Ahmed AF. Tocilizumab attenuates acute lung and kidney injuries and improves survival in a rat model of sepsis via down-regulation of NF-κB/JNK: a possible role of P-glycoprotein. Inflammopharmacology. (2020) 28:215–30. doi: 10.1007/s10787-019-00628-y
144. Flierl MA, Rittirsch D, Gao H, Hoesel LM, Nadeau BA, Day DE, et al. Adverse functions of IL-17A in experimental sepsis. FASEB J. (2008) 22:2198–205. doi: 10.1096/fj.07-105221
145. Ye B, Tao T, Zhao A, Wen L, He X, Liu Y, et al. Blockade of IL-17A/IL-17R pathway protected mice from sepsis-associated encephalopathy by inhibition of microglia activation. Mediators Inflamm. (2019) 2019:8461725. doi: 10.1155/2019/8461725
146. Wan J, Zhang Q, Hao Y, Tao Z, Song W, Chen S, et al. Infiltrated IL-17A-producing gamma delta T cells play a protective role in sepsis-induced liver injury and are regulated by CCR6 and gut commensal microbes. Front Cell Infect Microbiol. (2023) 13:1149506. doi: 10.3389/fcimb.2023.1149506
147. Unsinger J, McGlynn M, Kasten KR, Hoekzema AS, Watanabe E, Muenzer JT, et al. IL-7 promotes T cell viability, trafficking, and functionality and improves survival in sepsis. J Immunol. (2010) 184:3768–79. doi: 10.4049/jimmunol.0903151
148. Murphey ED, Sherwood ER. Bacterial clearance and mortality are not improved by a combination of IL-10 neutralization and IFN-gamma administration in a murine model of post-CLP immunosuppression. Shock. (2006) 26:417–24. doi: 10.1097/01.shk.0000226343.70904.4f
149. Fu XZ, Wang Y. Interferon-γ regulates immunosuppression in septic mice by promoting the Warburg effect through the PI3K/AKT/mTOR pathway. Mol Med. (2023) 29:95. doi: 10.1186/s10020-023-00690-x
150. Rittirsch D, Flierl MA, Nadeau BA, Day DE, Huber-Lang M, Mackay CR, et al. Functional roles for C5a receptors in sepsis. Nat Med. (2008) 14:551–7. doi: 10.1038/nm1753
151. Xu G, Feng Y, Li D, Zhou Q, Chao W, Zou L. Importance of the complement alternative pathway in serum chemotactic activity during sepsis. Shock. (2018) 50:435–41. doi: 10.1097/SHK.0000000000001031
152. Sommerfeld O, Medyukhina A, Neugebauer S, Ghait M, Ulferts S, Lupp A, et al. Targeting complement C5a receptor 1 for the treatment of immunosuppression in sepsis. Mol Ther. (2021) 29:338–46. doi: 10.1016/j.ymthe.2020.09.008
153. Chang KC, Burnham CA, Compton SM, Rasche DP, Mazuski RJ, McDonough JS, et al. Blockade of the negative co-stimulatory molecules PD-1 and CTLA-4 improves survival in primary and secondary fungal sepsis. Crit Care. (2013) 17:R85. doi: 10.1186/cc12711
154. Zhang Y, Zhou Y, Lou J, Li J, Bo L, Zhu K, et al. PD-L1 blockade improves survival in experimental sepsis by inhibiting lymphocyte apoptosis and reversing monocyte dysfunction. Crit Care. (2010) 14:R220. doi: 10.1186/cc9354
155. Huang S, Liu D, Sun J, Zhang H, Zhang J, Wang Q, et al. Tim-3 regulates sepsis-induced immunosuppression by inhibiting the NF-κB signaling pathway in CD4 T cells. Mol Ther. (2022) 30:1227–38. doi: 10.1016/j.ymthe.2021.12.013
156. Liu S, Wang C, Jiang Z, Deng X, Bo L. Tim-3 blockade decreases the apoptosis of CD8(+) T cells and reduces the severity of sepsis in mice. J Surg Res. (2022) 279:8–16. doi: 10.1016/j.jss.2022.05.014
157. Inoue S, Bo L, Bian J, Unsinger J, Chang K, Hotchkiss RS. Dose-dependent effect of anti-CTLA-4 on survival in sepsis. Shock. (2011) 36:38–44. doi: 10.1097/SHK.0b013e3182168cce
158. Gray CC, Biron-Girard B, Wakeley ME, Chung CS, Chen Y, Quiles-Ramirez Y, et al. Negative immune checkpoint protein, VISTA, regulates the CD4(+) T(reg) population during sepsis progression to promote acute sepsis recovery and survival. Front Immunol. (2022) 13:861670. doi: 10.3389/fimmu.2022.861670
159. Alves GF, Stoppa I, Aimaretti E, Monge C, Mastrocola R, Porchietto E, et al. ICOS-Fc as innovative immunomodulatory approach to counteract inflammation and organ injury in sepsis. Front Immunol. (2022) 13:992614. doi: 10.3389/fimmu.2022.992614
160. Gao F, Zuo B, Wang Y, Li S, Yang J, Sun D. Protective function of exosomes from adipose tissue-derived mesenchymal stem cells in acute kidney injury through SIRT1 pathway. Life Sci. (2020) 255:117719. doi: 10.1016/j.lfs.2020.117719
161. Zhou Q, Xie M, Zhu J, Yi Q, Tan B, Li Y, et al. PINK1 contained in huMSC-derived exosomes prevents cardiomyocyte mitochondrial calcium overload in sepsis via recovery of mitochondrial Ca(2+) efflux. Stem Cell Res Ther. (2021) 12:269. doi: 10.1186/s13287-021-02325-6
162. Su Y, Song X, Teng J, Zhou X, Dong Z, Li P, et al. Mesenchymal stem cells-derived extracellular vesicles carrying microRNA-17 inhibits macrophage apoptosis in lipopolysaccharide-induced sepsis. Int Immunopharmacol. (2021) 95:107408. doi: 10.1016/j.intimp.2021.107408
163. Jin C, Cao Y, Li Y. Bone mesenchymal stem cells origin exosomes are effective against sepsis-induced acute kidney injury in rat model. Int J Nanomedicine. (2023) 18:7745–58. doi: 10.2147/IJN.S417627
164. Liu W, Hu C, Zhang B, Li M, Deng F, Zhao S. Exosomal microRNA-342–5p secreted from adipose-derived mesenchymal stem cells mitigates acute kidney injury in sepsis mice by inhibiting TLR9. Biol Proced Online. (2023) 25:10. doi: 10.1186/s12575-023-00198-y
165. Guo J, Wang R, Liu D. Bone marrow-derived mesenchymal stem cells ameliorate sepsis-induced acute kidney injury by promoting mitophagy of renal tubular epithelial cells via the SIRT1/parkin axis. Front Endocrinol (Lausanne). (2021) 12:639165. doi: 10.3389/fendo.2021.639165
166. Hua T, Yang M, Song H, Kong E, Deng M, Li Y, et al. Huc-MSCs-derived exosomes attenuate inflammatory pain by regulating microglia pyroptosis and autophagy via the miR-146a-5p/TRAF6 axis. J Nanobiotechnology. (2022) 20:324. doi: 10.1186/s12951-022-01522-6
167. Liu Y, Zhang S, Xue Z, Zhou X, Tong L, Liao J, et al. Bone mesenchymal stem cells-derived miR-223–3p-containing exosomes ameliorate lipopolysaccharide-induced acute uterine injury via interacting with endothelial progenitor cells. Bioengineered. (2021) 12:10654–65. doi: 10.1080/21655979.2021.2001185
168. Cai X, Zhang ZY, Yuan JT, Ocansey DKW, Tu Q, Zhang X, et al. hucMSC-derived exosomes attenuate colitis by regulating macrophage pyroptosis via the miR-378a-5p/NLRP3 axis. Stem Cell Res Ther. (2021) 12:416. doi: 10.1186/s13287-021-02492-6
169. Lin F, Chen W, Zhou J, Zhu J, Yao Q, Feng B, et al. Mesenchymal stem cells protect against ferroptosis via exosome-mediated stabilization of SLC7A11 in acute liver injury. Cell Death Dis. (2022) 13:271. doi: 10.1038/s41419-022-04708-w
170. Martí-Chillón GJ, Muntión S, Preciado S, Osugui L, Navarro-Bailón A, González-Robledo J, et al. Therapeutic potential of mesenchymal stromal/stem cells in critical-care patients with systemic inflammatory response syndrome. Clin Transl Med. (2023) 13:e1163. doi: 10.1002/ctm2.1163
171. Cheng Y, Cao X, Qin L. Mesenchymal stem cell-derived extracellular vesicles: A novel cell-free therapy for sepsis. Front Immunol. (2020) 11:647. doi: 10.3389/fimmu.2020.00647
172. Horak J, Nalos L, Martinkova V, Tegl V, Vistejnova L, Kuncova J, et al. Evaluation of mesenchymal stem cell therapy for sepsis: A randomized controlled porcine study. Front Immunol. (2020) 11:126. doi: 10.3389/fimmu.2020.00126
173. Shakoory B, Carcillo JA, Chatham WW, Amdur RL, Zhao H, Dinarello CA, et al. Interleukin-1 receptor blockade is associated with reduced mortality in sepsis patients with features of macrophage activation syndrome: reanalysis of a prior phase III trial. Crit Care Med. (2016) 44:275–81. doi: 10.1097/CCM.0000000000001402
174. Kotsaki A, Pickkers P, Bauer M, Calandra T, Lupse M, Wiersinga WJ, et al. ImmunoSep (Personalised Immunotherapy in Sepsis) international double-blind, double-dummy, placebo-controlled randomised clinical trial: study protocol. BMJ Open. (2022) 12:e067251. doi: 10.1136/bmjopen-2022-067251
175. Opal SM, Fisher CJ Jr., Dhainaut JF, Vincent JL, Brase R, Lowry SF, et al. Confirmatory interleukin-1 receptor antagonist trial in severe sepsis: a phase III, randomized, double-blind, placebo-controlled, multicenter trial. The Interleukin-1 Receptor Antagonist Sepsis Investigator Group. Crit Care Med. (1997) 25:1115–24. doi: 10.1097/00003246-199707000-00010
176. Panacek EA, Marshall JC, Albertson TE, Johnson DH, Johnson S, MacArthur RD, et al. Efficacy and safety of the monoclonal anti-tumor necrosis factor antibody F(ab')2 fragment afelimomab in patients with severe sepsis and elevated interleukin-6 levels. Crit Care Med. (2004) 32:2173–82. doi: 10.1097/01.CCM.0000145229.59014.6C
177. Reinhart K, Menges T, Gardlund B, Harm Zwaveling J, Smithes M, Vincent JL, et al. Randomized, placebo-controlled trial of the anti-tumor necrosis factor antibody fragment afelimomab in hyperinflammatory response during severe sepsis: The RAMSES Study. Crit Care Med. (2001) 29:765–9. doi: 10.1097/00003246-200104000-00015
178. Gallagher J, Fisher C, Sherman B, Munger M, Meyers B, Ellison T, et al. A multicenter, open-label, prospective, randomized, dose-ranging pharmacokinetic study of the anti-TNF-alpha antibody afelimomab in patients with sepsis syndrome. Intensive Care Med. (2001) 27:1169–78. doi: 10.1007/s001340100973
179. Fisher CJ Jr., Agosti JM, Opal SM, Lowry SF, Balk RA, Sadoff JC, et al. Treatment of septic shock with the tumor necrosis factor receptor:Fc fusion protein. The Soluble TNF Receptor Sepsis Study Group. N Engl J Med. (1996) 334:1697–702. doi: 10.1056/NEJM199606273342603
180. van Heerden PV, Abutbul A, Sviri S, Zlotnick E, Nama A, Zimro S, et al. Apoptotic cells for therapeutic use in cytokine storm associated with sepsis- A phase ib clinical trial. Front Immunol. (2021) 12:718191. doi: 10.3389/fimmu.2021.718191
181. Igonin AA, Protsenko DN, Galstyan GM, Vlasenko AV, Khachatryan NN, Nekhaev IV, et al. C1-esterase inhibitor infusion increases survival rates for patients with sepsis*. Crit Care Med. (2012) 40:770–7. doi: 10.1097/CCM.0b013e318236edb8
182. Dorresteijn MJ, Visser T, Cox LA, Bouw MP, Pillay J, Koenderman AH, et al. C1-esterase inhibitor attenuates the inflammatory response during human endotoxemia. Crit Care Med. (2010) 38:2139–45. doi: 10.1097/CCM.0b013e3181f17be4
183. Caliezi C, Zeerleder S, Redondo M, Regli B, Rothen HU, Zürcher-Zenklusen R, et al. C1-inhibitor in patients with severe sepsis and septic shock: beneficial effect on renal dysfunction. Crit Care Med. (2002) 30:1722–8. doi: 10.1097/00003246-200208000-00008
184. Annane D, Pittock SJ, Kulkarni HS, Pickering BW, Khoshnevis MR, Siegel JL, et al. Intravenous ravulizumab in mechanically ventilated patients hospitalised with severe COVID-19: a phase 3, multicentre, open-label, randomised controlled trial. Lancet Respir Med. (2023) 11:1051–63. doi: 10.1016/S2213-2600(23)00082-6
185. Ziegler EJ, Fisher CJ Jr., Sprung CL, Straube RC, Sadoff JC, Foulke GE, et al. Treatment of gram-negative bacteremia and septic shock with HA-1A human monoclonal antibody against endotoxin. A randomized, double-blind, placebo-controlled trial. The HA-1A Sepsis Study Group. N Engl J Med. (1991) 324:429–36. doi: 10.1056/NEJM199102143240701
186. Wortel CH, von der Möhlen MA, van Deventer SJ, Sprung CL, Jastremski M, Lubbers MJ, et al. Effectiveness of a human monoclonal anti-endotoxin antibody (HA-1A) in gram-negative sepsis: relationship to endotoxin and cytokine levels. J Infect Dis. (1992) 166:1367–74. doi: 10.1093/infdis/166.6.1367
187. McCloskey RV, Straube RC, Sanders C, Smith SM, Smith CR. Treatment of septic shock with human monoclonal antibody HA-1A. A randomized, double-blind, placebo-controlled trial. CHESS Trial Study Group. Ann Intern Med. (1994) 121:1–5. doi: 10.7326/0003-4819-121-1-199407010-00001
188. Levin M, Quint PA, Goldstein B, Barton P, Bradley JS, Shemie SD, et al. Recombinant bactericidal/permeability-increasing protein (rBPI21) as adjunctive treatment for children with severe meningococcal sepsis: a randomised trial. rBPI21 Meningococcal Sepsis Study Group. Lancet. (2000) 356:961–7. doi: 10.1016/S0140-6736(00)02712-4
189. Giroir BP, Quint PA, Barton P, Kirsch EA, Kitchen L, Goldstein B, et al. Preliminary evaluation of recombinant amino-terminal fragment of human bactericidal/permeability-increasing protein in children with severe meningococcal sepsis. Lancet. (1997) 350:1439–43. doi: 10.1016/S0140-6736(97)06468-4
190. Opal SM, Laterre PF, Francois B, LaRosa SP, Angus DC, Mira JP, et al. Effect of eritoran, an antagonist of MD2-TLR4, on mortality in patients with severe sepsis: the ACCESS randomized trial. Jama. (2013) 309:1154–62. doi: 10.1001/jama.2013.2194
191. Giamarellos-Bourboulis EJ, Tziolos N, Routsi C, Katsenos C, Tsangaris I, Pneumatikos I, et al. Improving outcomes of severe infections by multidrug-resistant pathogens with polyclonal IgM-enriched immunoglobulins. Clin Microbiol Infect. (2016) 22:499–506. doi: 10.1016/j.cmi.2016.01.021
192. Turgeon AF, Hutton B, Fergusson DA, McIntyre L, Tinmouth AA, Cameron DW, et al. Meta-analysis: intravenous immunoglobulin in critically ill adult patients with sepsis. Ann Intern Med. (2007) 146:193–203. doi: 10.7326/0003-4819-146-3-200702060-00009
193. Pan B, Sun P, Pei R, Lin F, Cao H. Efficacy of IVIG therapy for patients with sepsis: a systematic review and meta-analysis. J Transl Med. (2023) 21:765. doi: 10.1186/s12967-023-04592-8
194. Hotchkiss RS, Colston E, Yende S, Angus DC, Moldawer LL, Crouser ED, et al. Immune checkpoint inhibition in sepsis: A phase 1b randomized, placebo-controlled, single ascending dose study of antiprogrammed cell death-ligand 1 antibody (BMS-936559). Crit Care Med. (2019) 47:632–42. doi: 10.1097/CCM.0000000000003685
195. Hotchkiss RS, Colston E, Yende S, Crouser ED, Martin GS, Albertson T, et al. Immune checkpoint inhibition in sepsis: a Phase 1b randomized study to evaluate the safety, tolerability, pharmacokinetics, and pharmacodynamics of nivolumab. Intensive Care Med. (2019) 45:1360–71. doi: 10.1007/s00134-019-05704-z
196. Watanabe E, Nishida O, Kakihana Y, Odani M, Okamura T, Harada T, et al. Pharmacokinetics, pharmacodynamics, and safety of nivolumab in patients with sepsis-induced immunosuppression: A multicenter, open-label phase 1/2 study. Shock. (2020) 53:686–94. doi: 10.1097/SHK.0000000000001443
197. Wu H, Tang T, Deng H, Chen D, Zhang C, Luo J, et al. Immune checkpoint molecule Tim-3 promotes NKT cell apoptosis and predicts poorer prognosis in Sepsis. Clin Immunol. (2023) 254:109249. doi: 10.1016/j.clim.2023.109249
198. Francois B, Jeannet R, Daix T, Walton AH, Shotwell MS, Unsinger J, et al. Interleukin-7 restores lymphocytes in septic shock: the IRIS-7 randomized clinical trial. JCI Insight. (2018) 3:e98960. doi: 10.1172/jci.insight.98960
199. Wu J, Zhou L, Liu J, Ma G, Kou Q, He Z, et al. The efficacy of thymosin alpha 1 for severe sepsis (ETASS): a multicenter, single-blind, randomized and controlled trial. Crit Care. (2013) 17:R8. doi: 10.1186/cc11932
200. Li C, Bo L, Liu Q, Jin F. Thymosin alpha1 based immunomodulatory therapy for sepsis: a systematic review and meta-analysis. Int J Infect Dis. (2015) 33:90–6. doi: 10.1016/j.ijid.2014.12.032
201. Flohé S, Lendemans S, Selbach C, Waydhas C, Ackermann M, Schade FU, et al. Effect of granulocyte-macrophage colony-stimulating factor on the immune response of circulating monocytes after severe trauma. Crit Care Med. (2003) 31:2462–9. doi: 10.1097/01.CCM.0000089640.17523.57
202. Meisel C, Schefold JC, Pschowski R, Baumann T, Hetzger K, Gregor J, et al. Granulocyte-macrophage colony-stimulating factor to reverse sepsis-associated immunosuppression: a double-blind, randomized, placebo-controlled multicenter trial. Am J Respir Crit Care Med. (2009) 180:640–8. doi: 10.1164/rccm.200903-0363OC
203. Hall MW, Knatz NL, Vetterly C, Tomarello S, Wewers MD, Volk HD, et al. Immunoparalysis and nosocomial infection in children with multiple organ dysfunction syndrome. Intensive Care Med. (2011) 37:525–32. doi: 10.1007/s00134-010-2088-x
204. Bo L, Wang F, Zhu J, Li J, Deng X. Granulocyte-colony stimulating factor (G-CSF) and granulocyte-macrophage colony stimulating factor (GM-CSF) for sepsis: a meta-analysis. Crit Care. (2011) 15:R58. doi: 10.1186/cc10031
205. Döcke WD, Randow F, Syrbe U, Krausch D, Asadullah K, Reinke P, et al. Monocyte deactivation in septic patients: restoration by IFN-gamma treatment. Nat Med. (1997) 3:678–81. doi: 10.1038/nm0697-678
206. Leentjens J, Kox M, Koch RM, Preijers F, Joosten LA, van der Hoeven JG, et al. Reversal of immunoparalysis in humans in vivo: a double-blind, placebo-controlled, randomized pilot study. Am J Respir Crit Care Med. (2012) 186:838–45. doi: 10.1164/rccm.201204-0645OC
207. Schlosser K, Wang JP, Dos Santos C, Walley KR, Marshall J, Fergusson DA, et al. Effects of mesenchymal stem cell treatment on systemic cytokine levels in a phase 1 dose escalation safety trial of septic shock patients. Crit Care Med. (2019) 47:918–25. doi: 10.1097/CCM.0000000000003657
208. He X, Ai S, Guo W, Yang Y, Wang Z, Jiang D, et al. Umbilical cord-derived mesenchymal stem (stromal) cells for treatment of severe sepsis: aphase 1 clinical trial. Transl Res. (2018) 199:52–61. doi: 10.1016/j.trsl.2018.04.006
209. Lei W, Ren Z, Su J, Zheng X, Gao L, Xu Y, et al. Immunological risk factors for sepsis-associated delirium and mortality in ICU patients. Front Immunol. (2022) 13:940779. doi: 10.3389/fimmu.2022.940779
210. Yen SC, Wu CC, Tseng YJ, Li CH, Chen KF. Using time-course as an essential factor to accurately predict sepsis-associated mortality among patients with suspected sepsis. BioMed J. (2023) 17:100632. doi: 10.1016/j.bj.2023.100632
211. Davoudian S, Piovani D, Desai A, Piovani D, Desai A, Mapelli SN, Leone R, Sironi M, et al. A cytokine/PTX3 prognostic index as a predictor of mortality in sepsis. Front Immunol. (2022) 13:979232. doi: 10.3389/fimmu.2022.979232
212. Kyriazopoulou E, Leventogiannis K, Norrby-Teglund A, Dimopoulos G, Pantazi A, Orfanos SE, et al. Macrophage activation-like syndrome: an immunological entity associated with rapid progression to death in sepsis. BMC Med. (2017) 15:172. doi: 10.1186/s12916-017-0930-5
213. Barrios EL, Mazer MB, McGonagill PW, Bergmann CB, Goodman MD, Gould RW, et al. Adverse outcomes and an immunosuppressed endotype in septic patients with reduced IFN-γ ELISpot. JCI Insight. (2024) 9:e175785. doi: 10.1172/jci.insight.175785
214. Akatsuka M, Tatsumi H, Sonoda T, Masuda Y. Low immunoglobulin G level is associated with poor outcomes in patients with sepsis and septic shock. J Microbiol Immunol Infect. (2021) 54:728–32. doi: 10.1016/j.jmii.2020.08.013
215. Chen J, Wang H, Guo R, Li H, Cui N. Early expression of functional markers on CD4(+) T cells predicts outcomes in ICU patients with sepsis. Front Immunol. (2022) 13:938538. doi: 10.3389/fimmu.2022.938538
216. Mao L. Thymosin alpha 1 - Reimagine its broader applications in the immuno-oncology era. Int Immunopharmacol. (2023) 117:109952. doi: 10.1016/j.intimp.2023.109952
217. Sun XY, Ding XF, Liang HY, Zhang XJ, Liu SH, Bing H, et al. Efficacy of mesenchymal stem cell therapy for sepsis: a meta-analysis of preclinical studies. Stem Cell Res Ther. (2020) 11:214. doi: 10.1186/s13287-020-01730-7
218. Leventogiannis K, Kyriazopoulou E, Antonakos N, Kotsaki A, Tsangaris I, Markopoulou D, et al. Toward personalized immunotherapy in sepsis: The PROVIDE randomized clinical trial. Cell Rep Med. (2022) 3:100817. doi: 10.1016/j.xcrm.2022.100817
219. Seymour CW, Kennedy JN, Wang S, Chang CH, Elliott CF, Xu Z, et al. Derivation, validation, and potential treatment implications of novel clinical phenotypes for sepsis. Jama. (2019) 321:2003–17. doi: 10.1001/jama.2019.5791
220. Mansur A, von Gruben L, Popov AF, et al. The regulatory toll-like receptor 4 genetic polymorphism rs11536889 is associated with renal, coagulation and hepatic organ failure in sepsis patients. J Transl Med. (2014) 12:177. doi: 10.1186/1479-5876-12-177
221. Mansur A, Hinz J, Hillebrecht B, et al. Ninety-day survival rate of patients with sepsis relates to programmed cell death 1 genetic polymorphism rs11568821. J Investig Med. (2014) 62:638–43. doi: 10.2310/JIM.0000000000000059
222. Vilander LM, Kaunisto MA, Vaara ST, Pettilä V. Genetic variants in SERPINA4 and SERPINA5, but not BCL2 and SIK3 are associated with acute kidney injury in critically ill patients with septic shock. Crit Care. (2017) 21:47. doi: 10.1186/s13054-017-1631-3
223. Frank AJ, Sheu CC, Zhao Y, Chen F, Su L, Gong MN, et al. BCL2 genetic variants are associated with acute kidney injury in septic shock*. Crit Care Med. (2012) 40:2116–23. doi: 10.1097/CCM.0b013e3182514bca
224. Rienzo M, Skirecki T, Monneret G, Timsit JF. Immune checkpoint inhibitors for the treatment of sepsis:insights from preclinical and clinical development. Expert Opin Investig Drugs. (2022) 31:885–94. doi: 10.1080/13543784.2022.2102477
Keywords: sepsis, immunological dysregulation, immunotherapy, immunostimulatory therapy, immunosuppressive therapy
Citation: Wu Y, Wang L, Li Y, Cao Y, Wang M, Deng Z and Kang H (2024) Immunotherapy in the context of sepsis-induced immunological dysregulation. Front. Immunol. 15:1391395. doi: 10.3389/fimmu.2024.1391395
Received: 25 February 2024; Accepted: 06 May 2024;
Published: 21 May 2024.
Edited by:
Eizo Watanabe, Aichi Medical University, JapanReviewed by:
Susana Fernandes, Universidade de Lisboa, PortugalEduardo López-Collazo, University Hospital La Paz Research Institute (IdiPAZ), Spain
Copyright © 2024 Wu, Wang, Li, Cao, Wang, Deng and Kang. This is an open-access article distributed under the terms of the Creative Commons Attribution License (CC BY). The use, distribution or reproduction in other forums is permitted, provided the original author(s) and the copyright owner(s) are credited and that the original publication in this journal is cited, in accordance with accepted academic practice. No use, distribution or reproduction is permitted which does not comply with these terms.
*Correspondence: Zihui Deng, dzihui123@163.com; Hongjun Kang, doctorkang301@163.com
†These authors have contributed equally to this work and share first authorship