- 1Citrus Research and Education Center, Institute of Food and Agricultural Sciences (IFAS), University of Florida, Lake Alfred, FL, United States
- 2Entomology and Nematology Department, Institute of Food and Agricultural Sciences (IFAS), University of Florida, Gainesville, FL, United States
The Asian citrus psyllid, Diaphorina citri, vectors the bacterial causative agent of citrus greening disease, which has severely impacted citrus production on a global scale. As the current repeated application of chemical insecticides is unsustainable for management of this insect and subsequent protection of groves, we investigated the potential use of the bacteria-derived pesticidal protein, Cry1Ba1, when delivered via transgenic citrus plants. Having demonstrated transformation of the Indian curry leaf tree, Bergera koenigii, for Cry1Ba1 expression for use as a trap plant, we produced transgenic plants of Duncan grapefruit, Citrus paridisi, Valencia sweet orange, Citrus sinensis, and Carrizo citrange, C. sinensis x Poncirus trifoliata, for expression of Cry1Ba1. The presence of the cry1ba1 gene, and cry1ba1 transcription were confirmed. Western blot detection of Cry1Ba1 was confirmed in most cases. When compared to those from wild-type plants, leaf discs from transgenic Duncan and Valencia expressing Cry1Ba1 exhibited a “delayed senescence” phenotype, similar to observations made for transgenic B. koenigii. In bioassays, significant reductions in the survival of adult psyllids were noted on transgenic B. koenigii and Valencia sweet orange plants expressing Cry1Ba1, but not on transgenic Duncan grapefruit or Carrizo citrange. In contrast to psyllids fed on wild type plants, the gut epithelium of psyllids fed on transgenic plants was damaged, consistent with the mode of action of Cry1Ba1. These results indicate that the transgenic expression of a bacterial pesticidal protein in B. koenigii and Valencia sweet orange offers a viable option for management of D. citri, that may contribute to solutions that counter citrus greening disease.
1 Introduction
The Asian citrus psyllid (ACP), Diaphorina citri (L.) Kuwayama is the vector of the plant pathogenic proteobacterial species ‘Candidatus Liberibacter asiaticus’ (CLas) and ‘Candidatus Liberibacter americanus’ (CLam), the presumed causative agents of citrus greening (also known as huanglongbing) in Asia and the Americas (1, 2). Trees infected with these bacteria have high mortality and dramatic decreases in fruit yield with dire consequences in Florida and other citrus growing regions (3–5). Management of ACP populations has been a primary strategy against the spread of citrus greening into groves with newly planted citrus trees. However, the cost of citrus grove management significantly increased with the repeated application of chemical insecticides, which also selected for insecticide resistance in ACP (6, 7). Environmental issues associated with the potential impacts of insecticides on non-target organisms is an additional area of concern (8), that warrants consideration of alternative strategies for psyllid management in citrus.
The successful use of transgenic crops engineered to produce bacteria-derived pesticidal proteins for suppression of pestiferous insects has been demonstrated in multiple cropping systems, including cotton, maize and forest trees such as poplar (9–13). These structurally diverse bacterial proteins are derived from Bacillus thuringiensis (Bt) (14). In citrus, such pesticidal proteins could be deployed via transgenic fruit-yielding citrus, or via transgenic trap plants, i.e. an alternative host plant that is highly attractive to the psyllid but is not a host for CLas or CLam. As psyllids move into citrus groves from neighboring smaller habitats (15), trap plants surrounding the grove would function to intercept ACP prior to grove entry. We and others have previously reported on B. thuringiensis-derived pesticidal proteins with toxicity against ACP (16, 17), and the production of transgenic Indian curry leaf plant, Bergera koenigii expressing one of the psyllid-active proteins, Cry1Ba1 (18). Transgenic B. koenigii could be used as a trap plant when planted in or around citrus groves to attract psyllids away from fruit-yielding citrus. In this scenario, psyllids that feed on the trap plant would die from ingestion of Cry1Ba1 before reaching the citrus grove. Transgenic citrus trees or trap plants that suppress ACP populations would benefit citrus production in conjunction with other management tools through reducing the need for frequent insecticide applications thereby decreasing both management costs and potential negative impacts on the environment. Transgenic citrus or trap plants may also contribute to reducing the spread of citrus greening when used in conjunction with other strategies that target CLas or CLam.
Following transformation of B. koenigii for potential use as a trap plant for psyllid management (18), our goal for this project was first to produce transgenic plants of three different citrus cultivars, Duncan grapefruit, Valencia sweet orange, and Carrizo citrange, expressing the cry1ba1 gene. Having confirmed the presence of the cry1ba1 gene, transcription, and translation, we then sought to determine the impact of transgenic plants for which protein expression was confirmed on the physiology, behavior and survival of ACP. Based on the results of this study, the potential for use of transgenic citrus plants and alternative approaches for their deployment for ACP management are discussed.
2 Materials and methods
2.1 Vector construct and plant transformation
Construction of the pCAMBIA2301-derived binary vector pBa1 for expression of Cry1Ba1 has been described previously (18). This vector includes sequences encoding kanamycin resistance (neomycin phosphotransferase II; nptII), green fluorescent protein (GFP) and the citrus codon-optimized active core of Cry1Ba1 along with a GNA precursor leader sequence (19). Cry1Ba1 expression is driven by the constitutive CaMV 35S promoter for expression in all tissues including phloem, the site of psyllid feeding (20, 21). The pBa1 vector was mobilized into Agrobacterium tumefaciens EHA105 competent cells. Previously described protocols (18, 22) were employed for the genetic transformation of Duncan grapefruit (Citrus paradisi Macf.), Valencia sweet orange [Citrus sinensis (L.) Osbeck], and Carrizo citrange [Citrus sinensis Washington Navel sweet orange X Poncirus trifoliata (L.) Raf.]. In brief, etiolated seedlings of these cultivars were cut into 10-15 mm long explants and incubated in freshly prepared suspension of A. tumefaciens. After co-incubation with Agrobacterium, explants were placed on co-cultivation medium, CCM (23), for two days and moved to regeneration medium, RM, for shoot induction and elimination of Agrobacterium. After five weeks, shoots that sprouted from seedling explants were inspected for fluorescence of GFP under a fluorescence stereomicroscope (Leica MZ16 FA; Leica, Bannockburn, IL) with a 480 nm excitation blue light. Shoots that exhibited GFP fluorescence were micro-grafted on Carrizo rootstock plants. The plantlets made of grafted shoots and rootstocks were transferred to cubic pots (5 cm3) covered with inverted magenta boxes to maintain high humidity and left on a light bench with constant white light (fluence rate = 50 μmol/m2s). After two months in the laboratory, these plantlets were moved to the greenhouse, where they were acclimated to grow in larger pots.
2.2 Confirmation of Cry1Ba1 presence and transcription by quantitative real-time PCR analysis
Quantitative PCR (qPCR) was used to assess the presence of cry1ba1 in the plant genome (24). Sample preparation and qPCR were conducted as described previously (18). qPCR was performed using three different primer pairs targeting different regions of cry1ba1 (18) in a 7500 Thermal Cycler (Applied Biosystems, Waltham, MA, USA) using Power SYBR™ Green RNA-to-CT1 step kit (Applied Biosystems Catalog # 4391178) without the RT-step. The quality of DNA isolated from transgenic and control plants was confirmed by amplification of the actin gene (18). The qPCR reaction volume of 10 µl was comprised of 5 µl 2XSYBR mix, 0.5 µl 10 mM forward primer, 0.5 µl 10 mM reverse primer, 0.1 µl SYBR enzyme and 1 µl template DNA diluted 1 to 50 to negate the effect of PCR inhibitors present in the resuspended DNA. The program was run at 95°C for 10 min, 40 cycles of 95°C for 15 sec, and 60°C for 1 min. A melting curve was run at the end of the qPCR program.
The transgenic plants generated were first screened by RT-qPCR for the production of cry1ba1 transcripts. Total RNA was extracted from leaves of transgenic or wild type (WT) citrus plants with the RNeasy Plant Mini Kit (Qiagen, Germantown, MD). cDNA was synthesized from 1μg of RNA using the iScript cDNA Synthesis kit (Bio-Rad Labs, Hercules, CA). ACT primers were used to amplify an actin fragment as a reference for normalization of transcripts, and the cry1ba1 target site was amplified using primers Ba1(f) 5’-GCCTATTCACGGAGTTCCAA-3’ (nucleotides 1122 to 1142 within the cry1ba1 sequence) and Ba1(r) 5’-CAACTGAAGACCTGGTGATTC-3’ (nucleotides 1216 to 1235) (18). Appropriate amplification of cry1ba1 with these primers was confirmed prior to their use in these assays. The qPCR validations were carried out in duplicate using the iTaq Universal SYBR Green mix (Bio-Rad Labs) in an Eco Real-Time thermocycler (Illumina) with the following program: 95°C for 2 min, 42 cycles at 95°C for 5 s, 57°C for 10 s and 72°C for 15 s. The specificity of PCR amplification was verified by melt curve analysis (from 55°C to 95°C). cry1ba1 transcript levels in transgenic plants relative to WT were calculated using the 2−ΔΔCt method (25).
2.3 Assessment of Cry1Ba1 expression by western blot
Having confirmed cry1ba1 transcription by transgenic plants, western blot analysis was conducted on tissues from each plant to test whether Cry1Ba1 was produced. For production of Cry1Ba1 protein for use as a positive control in western blots, the pMH19 plasmid expressing Cry1Ba1 [bases 1 to 2037 (26)] was transformed into Escherichia coli XL-1. The positive colonies were grown on Terrific Broth (TB)-medium containing ampicillin (100 μg/ml) and 2% glucose at 37°C for 3 days. The cells were harvested and the pellet suspended in lysis buffer (50 mM Tris-HCl pH8, 5 mM EDTA, 100 mM NaCl, 8 μl 50 mM PMSF/gram pellet). The pellet was further washed 2–3 times with buffer (20 mM TrisHCl, pH 7.5, 1 M NaCl, 1% Triton X-100) and crystals were solubilized in 50 mM NaHCO3 20 mM NaCl, 10 mM DTT pH10. The solubilized Cry1Ba1 was activated by treating with bovine trypsin (Sigma, St. Louis, MO) at a 1:10 ratio of trypsin: protein in buffer at pH 7.5, with incubation at 37°C for 3 h. Trypsin was removed by incubating the sample with benzamidine sepharose (GE health care, Chicago, IL) at RT for 30 min by shaking slowly and collecting the clear supernatant by centrifuging at 4,000 rpm at RT for 10 min. Total protein in the solubilized fractions was estimated using Bradford’s Reagent. Cry1Ba1 was separated on 4–20% gradient polyacrylamide gels (Bio-Rad) and transferred to the PVDF membrane using standard procedures. IgG purification from polyclonal anti-Cry1Ba1 raised in rabbits (PRF&L, Canadensis, PA, USA) was conducted as described previously (27). To assess the sensitivity of the purified IgG fraction, 0.02 µg to 2 µg of activated Cry1Ba1, were transferred to a PVDF membrane (Amersham International PLC, Amersham, UK). The membrane was blocked with 1X TBS 0.2% Tween 20 and 5% BSA. Cry1Ba was detected with the purified Cry1Ba IgG (dilution 1:250) and an HRP-coupled secondary antibody (Thermo Fisher Scientific, Waltham, MA, USA: dilution 1:5,000) followed by a Pierce chemiluminescent substrate (Thermo Fisher Scientific).
For western blot analysis, the soluble protein fraction was prepared from plant tissues as follows: 100 mg of leaf tissues was pulverized into powder with liquid nitrogen in a mortar and pestle. The powdered tissue was then solubilized overnight in 500 μL of extraction buffer (0.1% IGEPAL, 50 mM Tris-HCl pH 7.5, 10 mM MgCl2 and 150 mM NaCl) supplemented with 1X protease inhibitor (cOmplete™, Mini, EDTA-free Protease Inhibitor Cocktail: Roche, Basel, Switzerland) at 4°C. The solution was centrifuged at 16,100 g for 15 minutes at 4°C with soluble proteins remaining in the aqueous supernatant layer. Protein concentrations for solubilized leaf tissue samples were determined by Bradford assay (28).
Solubilized proteins from WT and transgenic citrus plants were separated in 4–20% SDS PAGE gels (15 or 20 µg protein per lane). Proteins from the gels were transferred to a PVDF membrane (Amersham). The blocking and detection of Cry1Ba1 was as described above for assessment of purified IgG sensitivity. Following analysis for detection of Cry1Ba1, the membrane was stripped with a mild stripping buffer (15 g glycine, 1 g SDS and 10 ml Tween-20 pH 2.2 for 1 L) and re-probed with anti-GFP antibody (Invitrogen anti-GFP polyclonal; A-11122, Thermo Fisher; dilution 1:5000) and anti-rabbit HRP secondary antibody (dilution 1:5000).
The production of transgenic Bergera koenigii and confirmation of cry1ba1 transcription were as described by Ravanfar et al. (18).
2.4 Impact of Cry1Ba1 expression on plant phenotype
Four Valencia and four Duncan plants for which cry1ba1 transcription and translation had been confirmed, were used to determine whether the “delayed senescence” phenotype described for transgenic B. koenigii expressing Cry1Ba1 (18) would be observed. For each genotype, transgenic plants were paired with four WT controls of the same genotype, size, and age. A single detached leaf per plant was used to create 15 mm diameter leaf discs cut with a cork hole borer. Discs were placed into Petri dishes (35 mm; Fisher brand, Thermo Fisher Scientific, Waltham, MA), one disc per dish containing a 1.5% agar bed. The agar in the dishes maintained moisture (and leaf turgor) for approximately 2 weeks. The phenotype of the leaf discs taken from each of four plants from each of four treatments was recorded on day 3, day 7 and every 7 days thereafter until 60 d after leaf harvest and scored as 1-green, succulent; 2- green, dry; or 3- brown, wilted, dead or dying in appearance. Due to the destructive nature of this approach, a single replicate of four plants per citrus variety was conducted for observation of the “delayed senescence” phenotype.
2.5 Impact of transgenic citrus on Asian citrus psyllid
Asian citrus psyllids, Diaphorina citri, from an insecticide susceptible and CLas/CLam-free culture maintained in a greenhouse at the Citrus Research and Education Center, University of Florida, Lake Alfred, FL were used in bioassays to assess the survival of adult ACP on test transgenic Indian curry leaf and citrus plants. The insects were reared on various host plants (described above) for at least two generations prior to conducting biological evaluations on those same hosts to control for possible effects of host adaptation. Rearing occurred at 27–28°C, with 60–65% relative humidity, and a 14:10 h (light: dark) photoperiod.
Transgenic B. koenigii, Duncan grapefruit, as well as Carrizo citrange and Valencia orange plants (T0 generation) at ~50 cm in height for which transcription of cry1ba1 had been confirmed were used for bioassays. These were B. koenigii 2, 6, 16a (18); Valencia 15, 16, and 22; Duncan 3, 5, and 23; and Carrizo 2, 4, 7, 8, 10, and 14. Prior to initiation of bioassays, plants leaves were washed twice per week using a 2% Dawn dish detergent spray solution (Procter and Gamble, Cincinnati, OH). Twenty to 30 minutes after detergent was applied, plants were rinsed with clean water. Subsequently, plants were repositioned into individual cages and maintained at 23 ± 3°C, 60% RH, and a 16:8 h (Light: Dark) photoperiod with a maximum photosynthetic radiation of 215 µmol s−1 m−2. Plants were watered twice per week and fertilized twice per month with an alternating schedule of a 24-8-16 NPK solution at 4 g L−1 (Miracle-Gro All Purpose Plant Food; Scotts Miracle-Gro Products, Marysville, OH) and a 6-4-6 (N–P–K) granular fertilizer at 1 g per pot (Expert gardener Gro Tec. Inc. Madison, GA).
After withholding food from D. citri for 4 hours, 10 adults of mixed sex and age were released into bags sealed tightly around stems to prevent insect escape. Mortality was observed every other day for 8 days. For all Duncan and Valencia plants, flush, feather-like structures were apparent in both Cry1Ba1 transgenic and control plants. Plant bioassays were replicated independently three times for transgenic cry1ba1 Valencia, Duncan, and Indian curry leaf transgenic plants alongside wild type plants and six times with trangenic Carrizo plants and respective controls.
The settling preference of adult D. citri between transgenic Duncan grapefruit plants with cry1ba1 versus control plants was evaluated with an open-air choice assay. Control plants were of the same variety and approximate age and size. Newly emerged adult psyllids were collected and host preference was assessed using a choice assay as described elsewhere (29) with slight modifications. Briefly, plants were inserted into a 1.8×1.8×1.0 m cage custom made from 0.5 cm thick, white, and opaque acrylic. Plants were positioned at opposite sides of the cage 1 m apart. Position of treatment and control plants was randomized prior to each replicate. Afterward, 25 psyllids were vacuum‐suctioned into separate 50-mL vials. Subsequently, collection vials were introduced into the center of the cage containing two plants (a plant with cry1ba1 to one side and the control plant to the other side). The cap was removed from the collection vial allowing D. citri to move freely. The experiment was conducted under light conditions generated by a fluorescent 900-lux light bulb under temperature-controlled conditions of 27 ± 1°C, 63 ± 2% RH, and under a L14∶D10 h photoperiod. Each run of the host preference experiment contained three independent replicates with different treatment and control plants that were conducted on different days. The entire experiment was repeated twice. Psyllid preference was determined 24 hours after release by counting all psyllids alighting on each plant. Psyllids were considered settled when found in a feeding position with their abdomen at a 45° to the plant surface. Approximately 75% of all released psyllids were found on either of the two plants presented for settling choice. Assays were conducted with two control plants that determined no positional bias within the assay cage as evidenced by a 50:50 psyllid settling response between two control plants of similar size and age.
2.6 Transmission electron microscopy of ACP fed on transgenic and control plants
To look for potential physiological damage associated with Cry1Ba1-mediated toxicity, transmission electron microscopy (TEM) was used to examine the gut epithelium of ACP fed on Cry1Ba1 transformed Valencia, Duncan grapefruit and B. koenigii (Indian curry leaf), or control plants. The plants used here were the same as those used for toxicity bioassays described above, with a total of three plants each for Valencia, Duncan, B. koenigii or control (wild type). Adult psyllids (20 per plant) were maintained on the plants for 8 days. Adult ACP were collected for TEM analysis with >40 live insects pooled for each treatment. The head and tip of the abdomen was removed from each psyllid, and the torsos fixed for 16 h at 4°C in 1X PBS buffer containing 3% (v/v) glutaraldehyde. Samples were then postfixed for 4 h at room temperature in 2% (v/v) osmium tetroxide, dehydrated in acetone, and embedded in Spurr’s Resin (30). Cross sections were examined by light microscopy for location of the midgut and blocks sectioned from the anterior or posterior ends of 8 to 10 psyllids per treatment. Ultrathin sections (90 nm) mounted on copper grids were stained with 2% (w/v) aqueous uranyl acetate and lead citrate (31) and observed using an FEI Morgagni 268 Transmission Electron Microscope (FEI Company, Hillsboro, OR, USA).
2.7 Statistical analysis
Data collected for psyllid bioassays comparing psyllid survival on transgenic plants with cry1ba1 and control plants were analyzed using a Kaplan-Meier survival analysis (32), and a Log-Rank test for pairwise comparisons using the RStudio environment version 3.6.3 (33). Behavioral response by ACP between the two host outcomes was analyzed using chi-square contingency tables also in R (33).
3 Results
3.1 Citrus transformation and confirmation of cry1ba1 insertion
The citrus cultivars Valencia sweet orange, Duncan grapefruit and Carrizo citrange were transformed with cry1ba1, nptii and gfp. Antibiotic resistance was used as the basis for selection of transformants and green fluorescence used to visualize heterologous protein expression in tissues of the transformants and to determine transformation rates. For transformation of Duncan grapefruit we used 12,832 explants that were co-incubated with Agrobacterium. The number of shoots and buds that sprouted from Duncan explants was 17,598, for which GFP fluorescence was only detected in 71 shoots resulting in a transformation success rate of 0.40% (Table 1). Of these, 49 shoots were chimeric and 22 were fully transformed. To produce transgenic Valencia plants, 10,016 explants were co-incubated with Agrobacterium and 6,242 shoots that sprouted from those explants were examined for GFP fluorescence. Fluorescence was detected in 17 shoots comprised of seven that were fully transformed and 10 that were chimeric. The transformation rate for Valencia was 0.27%. Compared to Valencia and Duncan, the transformation rate for Carrizo citrange was the highest at 0.69%. For Carrizo, 1,920 explants were co-incubated with Agrobacterium, and 2,023 shoots sprouted from those explants. Of these, 14 were transgenic with GFP fluorescence. Three of the 14 shoots were chimeric and 11 fully transformed. A total of seven Carrizo citrange plants were produced and survived the transition to soil. In all cases, only fully transformed shoots were grafted on rootstock plants. Some of the grafted plants grew poorly and some did not survive the transition from in vitro culture to soil. A total of 27 transgenic plants of different citrus cultivars expressing cry1ba1 gene were produced: Fourteen Duncan grapefruit cultivar plants, six Valencia sweet orange and seven Carrizo citrange. These plants acclimated well to grow in pots. The presence of the transgene in the plants was assessed by use of qPCR with three sets of cry1ba1 primers (Table 2). The 1:1:1 ratio of qPCR Ct values amplified using three different sets of primers from the same isolated DNA indicates that the full cry1ba1 gene was present as part of the T-DNA.
3.2 Confirmation of cry1ba1 transcription and translation
Transcription of cry1ba1 was confirmed for all transgenic plants with variable levels of transcript accumulation detected (Table 3). Using the purified Cry1Ba1 IgG that was sufficiently sensitive for detection of 0.02 µg Cry1Ba1 (i.e. a detection limit of 100 ppm in western blots based on loading 20 µg plant sample per lane; Figure S1), translation of the cry1ba1 gene in transgenic plants was confirmed in most instances (Table 3; Figure S1). The goal of the western blots was to confirm Cry1Ba1 production and not to compare relative protein levels between lines. For some samples, although a protein band of the size expected for the toxic protein (~ 50kDa) was not observed, a strong immunoreactive band of lower molecular mass was apparent. These plants were scored as “negative” (Table 3). Cry1Ba1 detection was not consistent with multiple blots required to confirm expression in some cases (Table S1). Western blots for detection of GFP that were used as controls for the western blotting procedure, confirmed GFP expression by all transgenic Duncan, Valencia, and Carrizo plants (Figure S1).
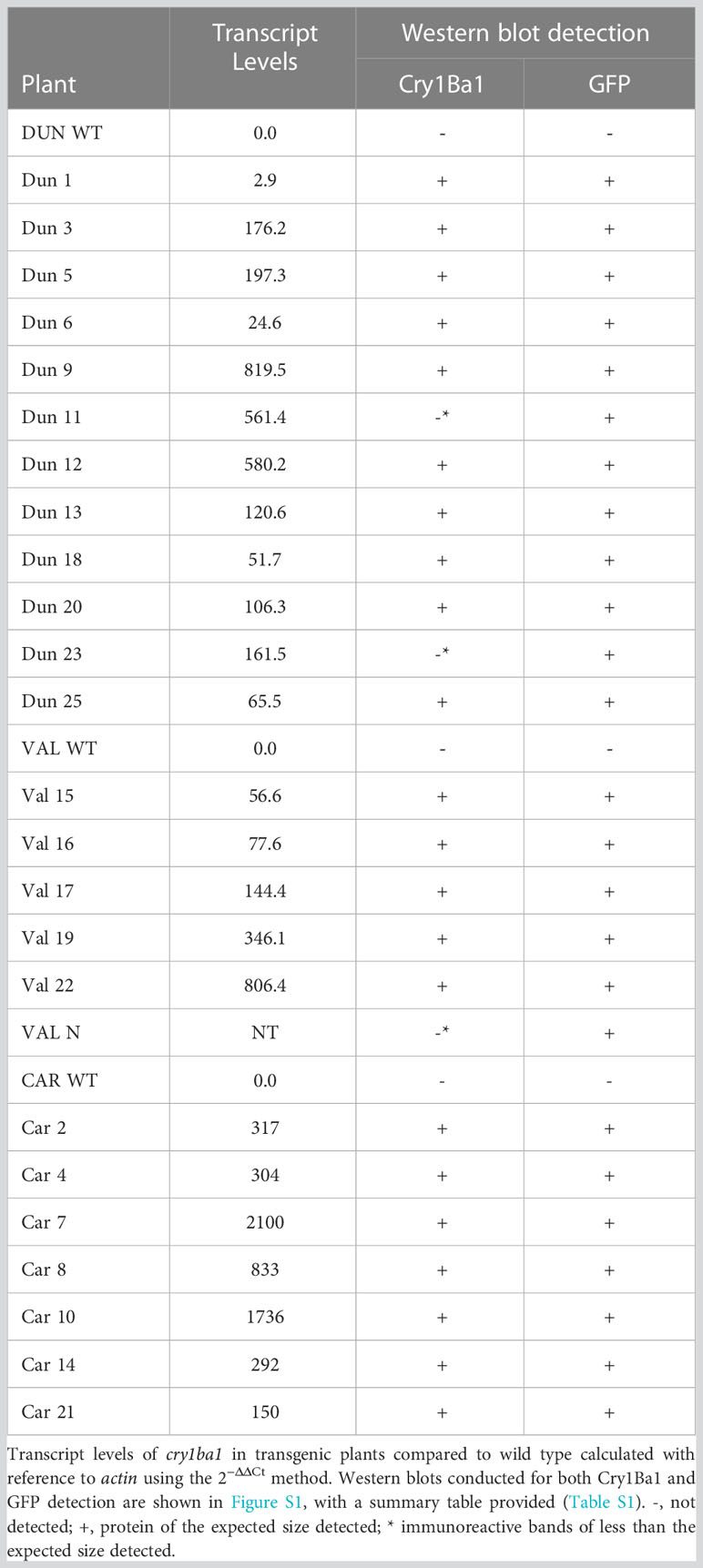
Table 3 Confirmation of transcription and translation for plants transformed for expression of Cry1Ba1.
3.3 Impact of Cry1Ba1 production on transgenic plants and ACP plant preference
Leaf discs taken from transgenic Valencia and Duncan plants expressing Cry1Ba1 exhibited a reduced tendency to dry out and turn brown in appearance relative to control plants. While discs from control plants had all dried and turned brown by day 21 (Figure 1), those from only one transgenic Valencia plant turned brown by day 35. Discs from the other seven transgenic plants (Valencia and Duncan) remained green and living in appearance but dry to the touch until the end of the experiment (day 60). To assess whether psyllids would avoid Cry1Ba1-expressing transgenic plants, behavioral assays were conducted. Adult psyllids exhibited no preference (χ2 = 1.07, P = 0.459) in choice test experiments, settling with equal frequency on transgenic Duncan or control plants (Figure S2).
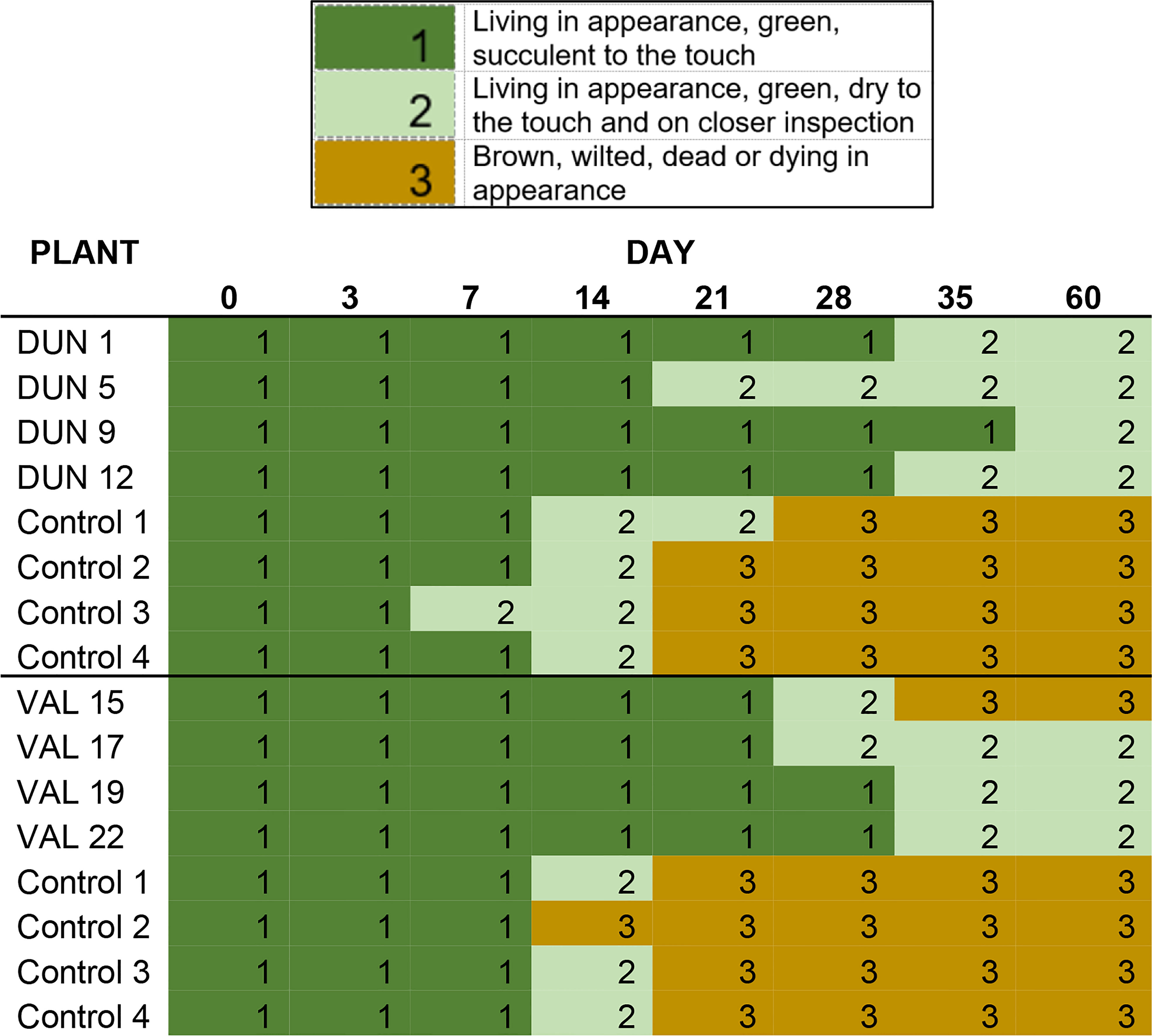
Figure 1 “Delayed senescence” phenotype of Cry1Ba1 transgenic plants. Graphic shows the delayed desiccation and browning of leaf discs maintained on agar plates from Cry1Ba1-expressing Duncan or Valencia compared to control plants. In contrast to those from transgenic plants, discs from the control plants had dried and mostly turned brown by day 21.
3.4 Impact of transgenic plants on ACP survival
Bioassays using whole plants showed that survival of ACP adults was significantly reduced on Indian curry leaf plant and Valencia sweet orange expressing Cry1Ba1 relative to control plants without transformation (Figure 2). Although a drop in ACP survival on transgenic Duncan by day 8 was noted, it was not statistically significant from survival on control plants (p = 0.079). No impact on psyllid survival was seen for transgenic Carrizo compared to control plants (p > 0.05).
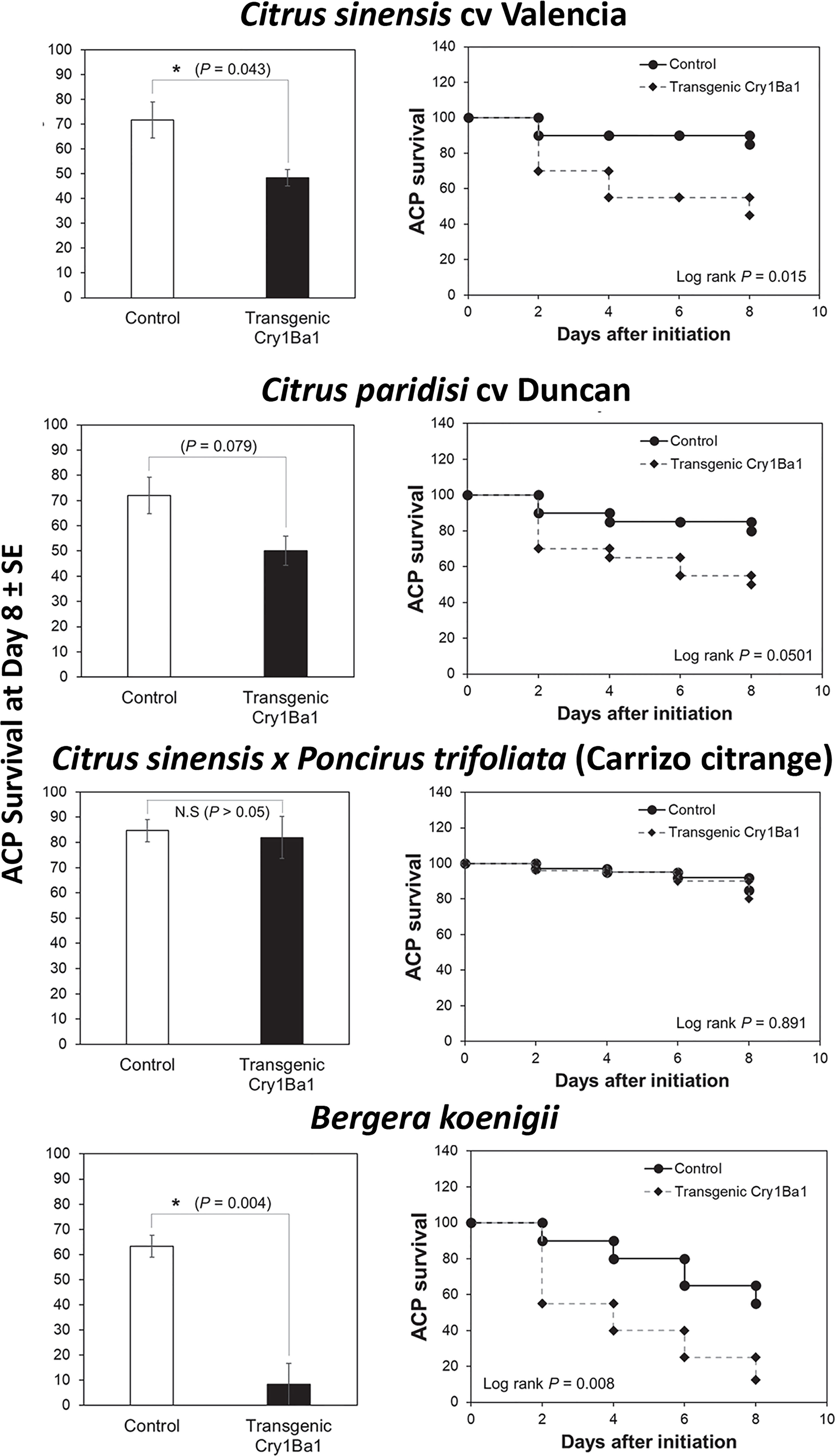
Figure 2 Reduced survival of Asian citrus psyllids on transgenic B. koenigii and C. sinensis expressing Cry1Ba1. Bars represent the mean Asian citrus psyllid (ACP) survival ± standard error (SE), with statistical differences indicated (*, significantly different; N.S, not significantly different; Log-Rank test for pairwise comparisons). ACP survival during the course of the bioassay is also shown for one of three independent replicates per treatment. Curves depict the Kaplan-Meier survival probabilities of ACP adults after access to transgenic plants.
The guts of surviving psyllids fed on Cry1Ba1 transgenic Valencia or Duncan or wild type plants for 8 days were dissected and examined by TEM for comparison with those of psyllids fed on transgenic or wild type B. koenigii (18). Cry1Ba1-mediated damage to the psyllid gut was noted in all cases for psyllids fed on transgenic plants. In all instances, the midgut microvilli of psyllids fed on Cry1Ba1 plants were swollen or deformed with extensive lesions exposing the gut epithelial cell membrane (Figure 3; Figure S3). The lesions are associated with thinning of the cytoplasm and cell organelles in the gut epithelium, and disruption or bursting of the cell. Breakdown of the basement membrane overlying the gut epithelium was not observed. These observations are consistent with damage associated with the toxicity of Bt-derived pesticidal proteins, such as Cry1Ba1. The impacts of bacterially expressed Cry1Ba1 on the psyllid gut epithelium, which are similar to those illustrated here are shown in Fernandez-Luna et al. (16).
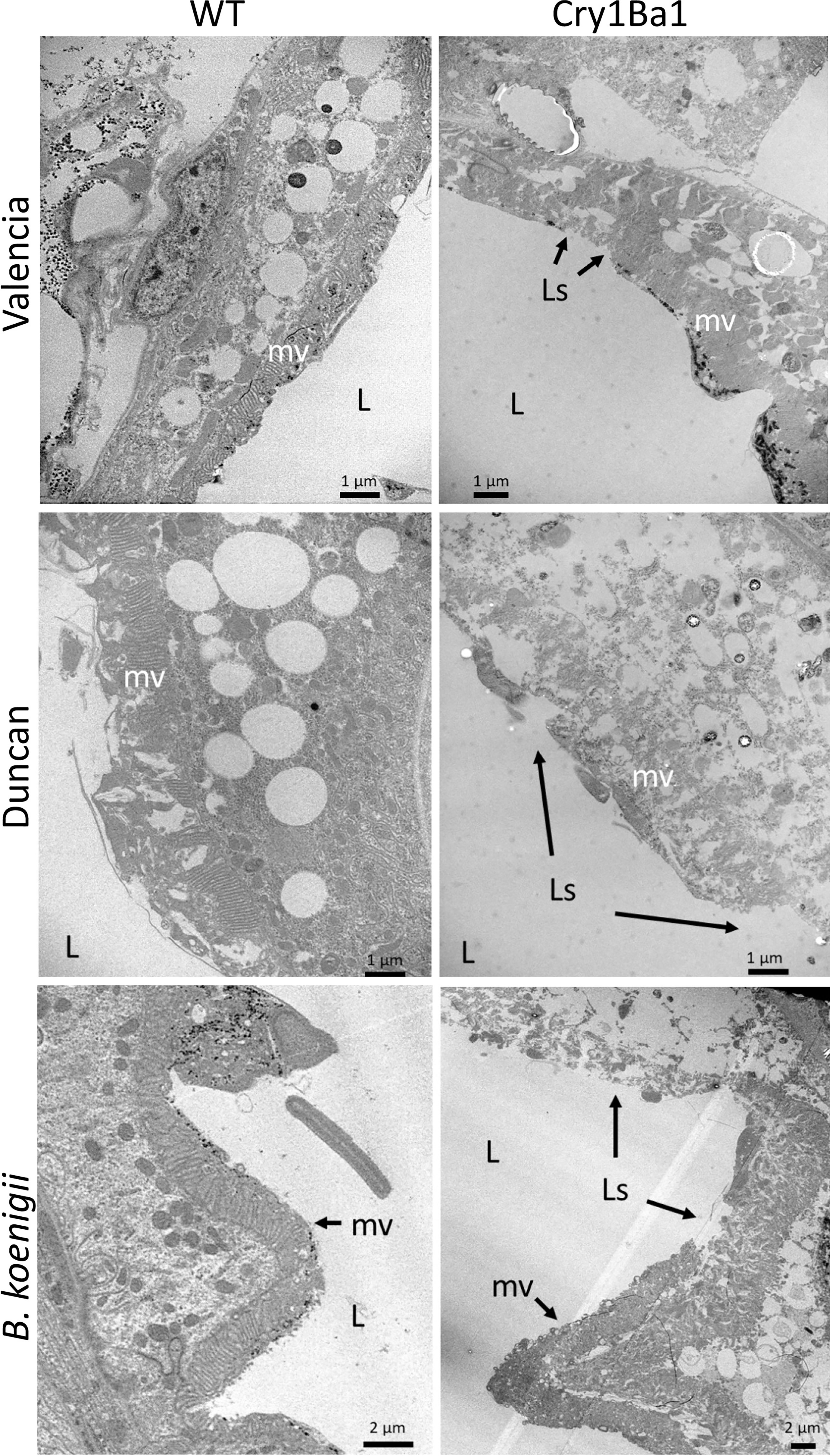
Figure 3 Damage to gut epithelial tissues of Asian citrus psyllid consistent with Cry1Ba1-mediated toxicity. Representative transmission electron micrographs showing the gut epithelia of ACP fed on WT or transgenic Cry1Ba1-expressing plants. While the microvilli lining the gut epithelium of ACP fed on WT plants are intact, the microvilli of ACP fed on transgenic plants were severely disrupted with multiple lesions apparent. L, gut lumen; mv, microvilli; Ls, lesion in microvilli. Scale bars as indicated. Additional micrographs are provided in Figure S3.
4 Discussion
The primary goal for this research was to assess the impact of transgenic citrus expressing Cry1Ba1 on the survival, physiology and behavior of the Asian citrus psyllid, D. citri. This work provides the foundation for the potential use of such transgenic plants as part of a multi-pronged strategy for management of citrus greening. Having confirmed cry1ba1 gene presence, transcription and translation in different lines of transgenic Indian curry leaf plants (18), Duncan grapefruit, Valencia sweet orange and Carrizo citrange, bioassays were conducted to examine the impact of transgenic Cry1Ba1 plants on ACP. The most dramatic reductions in ACP survival were seen on transgenic Indian curry leaf plants, with significant reductions in psyllid survival also seen on Valencia. Damage to the midgut epithelium typical of Bt pesticidal protein-mediated toxicity was observed, which supports that psyllid mortality was caused by Cry1Ba1 toxicity.
4.1 Production of transgenic citrus plants
The expression of Cry1Ba1 in transgenic Valencia and Duncan plants resulted in physiological changes to the plant observed as a “delayed senescence” phenotype. Similar changes in transgenic B. koenigii were associated with alterations in plant hormone levels. The abundance of senescence inhibitors increased whereas the abundance of most factors that promote senescence decreased (18). It is unclear whether these physiological changes result from general stress to the plant associated with Cry1Ba1 expression, or from a specific impact of this protein on plant metabolic pathways. The unexpectedly low transformation rates for all of these citrus varieties in comparison to previous reports (23, 34) could also be explained if Cry1Ba1 deleteriously affected the physiology of the regenerating shoots. As noted previously (18), the commercial utility of some bacterial pesticidal proteins has been limited by such impacts on the physiology of the plant.
In contrast to B. koenigii where high levels of phenolics interfered with the transfer of soluble proteins to membranes for western blot analysis (Figure S1) (18), sufficient soluble plant protein was extracted and transferred for detection of Cry1Ba1 in tissues of transgenic Duncan, Valencia and Carrizo by western blot (Figure S1). The predominant immunoreactive band from transgenic plants was ~ 50 kDa, the expected size for the protein for which toxicity has been confirmed by Fernandez-Luna et al. (16) (Figure S1) (18). In some cases, replicate blots were warranted to confirm expression of Cry1Ba1 of the expected size. However, the immunoreactive band of lower molecular mass detected for some plants could result from degradation of the 50 kDa protein in planta or during processing for western blot (Figure S1; Table S1). The stability of expressed Cry1Ba1 within the plant and comparison of Cry1Ba1 levels with plant age warrant further investigation.
4.2 Impact of plant susceptibility to psyllid feeding on ACP mortality
It is important to note that Cry1Ba1 levels in transgenic plants were not quantified in the current study. Therefore, it is possible that differences in levels of Cry1Ba1 expression between different citrus varieties could account for the differences in psyllid mortality observed in bioassays. In this scenario, Cry1Ba1 expression by the six Carrizo plants used in bioassays, for example, could have been too low for psyllids to acquire a toxic dose within the eight-day bioassay period. (It is notable in this context that Cry1Ba1 detection in the Carrizo plants was more consistent than in the other citrus varieties; Table S1).
A second factor that could impact the amount of Cry1Ba1 ingested by the psyllid is the susceptibility of each citrus variety to psyllid feeding. The Indian curry leaf plant is highly attractive to ACP, in large part due to continuous flushing, i.e. the production of new, bright green succulent leaves. Psyllids are highly attracted to such new leaves, which are the favored sites for egg laying and nymph development (35, 36). In contrast, citrus cultivars such as Duncan, Valencia and Carrizo flush intermittently. These differences in plant growth support the use of Indian curry leaf plants as trap plants to intercept psyllids away from fruit-yielding trees, and may also explain the lower psyllid survival in whole plant bioassays on transgenic Indian curry leaf plants compared to that on transgenic Valencia, Duncan or Carrizo plants. As psyllids feed more efficiently on immature leaves (37), psyllids in the B. koenigii treatment may have ingested more Cry1Ba1 from the continuous flush than psyllids in other transgenic treatments resulting in the higher mortality levels observed. Conversely, the lack of mortality observed for D. citri on transgenic Cry1Ba1 Carrizo plants may have been caused by known tolerance of trifoliate citrus varieties, such as Carrizo, to psyllid feeding as compared with non-trifoliate citrus such as Valencia (38). By using electrical penetration graph recording, Willett et al. (39) demonstrated significantly reduced phloem feeding behaviors by ACP on all Poncirus accessions tested, including Carrizo, compared to a wide variety of non-Poncirus citrus genotypes, including Valencia. The fibrous ring (sclerenchyma) around the phloem of xCitroncirus accessions (hybrids of Poncirus trifoliata and Citrus spp.) is thicker than that in other citrus genotypes, which is associated with reduced penetration of the vascular bundle and phloem feeding by ACP on Poncirus hybrids, such as Carrizo (40). Taken together, the impact of a transgenic citrus variety expressing a bacterial pesticidal protein will be influenced by the susceptibility of that variety to psyllid feeding and the amount of pesticidal protein ingested. Consequently, the use of bacteria-derived pesticidal proteins, or indeed other bioactives for transgenic tolerance to ACP, may be limited to a subset of citrus varieties such as Valencia sweet orange. The potential importance of plant susceptibility to psyllid feeding to psyllid mortality outcomes requires further investigation (41).
In choice test assays, psyllids did not discriminate between transgenic and control plants, which is to be expected. Differences may be observed following onset of feeding however: on acquisition of sufficient pesticidal protein to cause damage to the gut epithelium, insects commonly stop feeding and may die due to starvation. Analysis of feeding behavior using the electrical penetration graph system would shed light on the impact of Cry1Ba1 on psyllid feeding behavior, and potential differences between citrus varieties (36).
4.3 Possible applications of transgenic trees in citrus
The successful deployment on a commercial scale of transgenic forest trees expressing one or more bacterial pesticidal proteins (13, 42) provides a precedent for the use of transgenic perennial citrus trees, which differ markedly from traditional Bt crops that are planted annually. Bacterial pesticidal proteins have been combined with insecticidal agents with different modes of action (e.g. protease inhibitors) in these trees for broader suppression of pest species and to delay the onset of insect resistance.
We envision the possibility of applying genetically engineered citrus trees in citrus greening management that extends beyond sole cultivation of fully transgenic crops. For example, the Cry1Ba1-expressing Indian curry leaf plant could be planted adjacent to non-transgenic citrus and serve as a toxic trap plant. A logical next step would be to combine the Bt-expressing trap crop with a psyllid deterrent, such as kaolin (43, 44), applied to fruit-yielding citrus. The concept of combining a pest deterrent (“push”) with an alternative, more attractive host plant modified or engineered to produce an insecticidal agent (“pull”) was introduced in a prescient paper by Miller and Cowles in 1990 (45) and has been suggested as a strategy for management of D. citri (46). Furthermore, the efficacy of this tactic has now been confirmed in multiple field studies employing B. koenigii given that this host is: 1) more attractive to D. citri than Citrus genotypes and thus outcompetes the cultivated crop when planted so as to surround the cultivated crop (47–49) and 2) is immune to the pathogen causing citrus greening (50). The use of a transgenic trap plant rather than transgenic fruit-yielding trees may also align more readily with public sentiment regarding bioengineered food products (51).
4.4 Considerations for deployment in the field
Insect resistance management is a primary concern for the sustainable use of transgenic crops that express pesticidal proteins such as Cry1Ba1 (52, 53). One important component for successful resistance management has been the deployment of transgenic plants expressing multiple bioactives with different modes of action (e.g. bacterial pesticidal proteins from different structural classes (14); referred to as pyramiding) as the use of combined bioactives delays the onset of insect resistance (54). With such multiple traits targeting the same pest, if resistance develops to one, the target pest would succumb to the toxicity of a second bioactive. Even with multiple traits however, additional resistance management measures are needed for long-term pest management.
Insect resistance has been successfully managed using a high-dose/refuge strategy. In this case plants express a dose sufficient to kill insects that are heterozygous for resistance, and a refuge is used for maintenance of susceptible insect genotypes in the pest population. Following mating of these susceptible insects from the refuge with resistant insects, the heterozygous progeny are unable to survive on the transgenic plants. Key factors pertaining to the success of this approach are sufficient dispersal of the pest insect to facilitate mating between individuals from refuges with those from the transgenic crop, and a fitness cost associated with the resistance phenotype (52). In the case of ACP, while psyllids are capable of moving 1–2 km within a week (55), these long-distance migrations are relatively rare and most likely when resources (i.e. new leaf flush) become limiting (55, 56). Having settled on appropriately flushing host plants, psyllid populations typically move < 15 m (57). Such limited dispersal behavior would not be favorable for resistance management in groves planted entirely to transgenic citrus. This insect behavior would be appropriate however for effective resistance management on deployment of transgenic trap plants. Such trap plants would be planted around the grove or interspersed among non-transgenic fruit-bearing citrus trees. These resistance management considerations provide an additional argument for the use of trap plants for psyllid control in citrus rather than use of transgenic fruit-bearing citrus trees. Taken together, the use of a pyramid of bioactives from trap plants with proximal nontransgenic trees serving as refuge, within an integrated pest management approach may offer the optimal strategy for use of pesticidal proteins in citrus. The fact that ACP has not previously been exposed to bacteria-derived pesticidal proteins to any extent is an additional benefit due to the absence of pre-existing resistance.
4.5 Major conclusions and future research
The results from this study support the concept for use of transgenic citrus or trap plants for psyllid management as one component in an integrated and multipronged approach for citrus greening management. The bioassay data for Indian curry leaf plants along with resistance management considerations are particularly compelling for a push-pull, trap plant-based strategy. Several research avenues will allow for refinement of this transgenic plant approach including peptide- or mutagenesis-mediated optimization of Cry1Ba1 toxicity as previously demonstrated against other hemipteran pests (58–61), and screening for bacterial pesticidal proteins with greater natural toxicity to D. citri toward ready attainment of a high-dose approach. As pyramiding of bioactives has proved to be a successful approach for the deployment of insect resistant transgenic plants (52), the combined action of multiple pesticidal proteins or pesticidal proteins in combination with other bioactives such as gene silencing RNAs (62) against D. citri warrants investigation. Laboratory-based selection of D. citri for resistance to Cry1Ba1 and other traits selected for use in a pyramid approach would provide insight into the potential fitness costs associated with resistance should it arise in the field. In the long term, whether transgenic plants are adopted for D. citri management in citrus will be determined by industry.
Data availability statement
The original contributions presented in the study are included in the article/Supplementary Material. Further inquiries can be directed to the corresponding author.
Author contributions
SR, VO, FI-C, LS, DA, TS, CE-M, RB performed the experiments. VO, FI-C, LS, BB designed the experiments. VO and BB wrote the manuscript. All authors contributed to the article and approved the submitted version.
Funding
This project was supported by the Citrus Diseases Research and Extension grants program, Award number 2017-70016-26755 and by the Emergency Citrus Diseases Research and Extension grants program, Award number 2020-70029-33177 from the USDA National Institute of Food and Agriculture.
Acknowledgments
The authors thank Cecile Robertson for maintaining and propagating transgenic plants.
Conflict of interest
The authors declare that the research was conducted in the absence of any commercial or financial relationships that could be construed as a potential conflict of interest.
Publisher’s note
All claims expressed in this article are solely those of the authors and do not necessarily represent those of their affiliated organizations, or those of the publisher, the editors and the reviewers. Any product that may be evaluated in this article, or claim that may be made by its manufacturer, is not guaranteed or endorsed by the publisher.
Supplementary material
The Supplementary Material for this article can be found online at: https://www.frontiersin.org/articles/10.3389/finsc.2023.1125987/full#supplementary-material
References
1. Bove JM. Huanglongbing: a destructive, newly-emerging, century-old disease of citrus. J Plant Pathol (2006) 88:7–37. Available at: https://www.jstor.org/stable/41998278.
2. Manjunath KL, Halbert SE, Ramadugu C, Webb S, Lee RF. Detection of ‘Candidatus Liberibacter asiaticus’ in Diaphorina citri and its importance in the management of citrus huanglongbing in Florida. Phytopathology (2008) 98(4):387–96. doi: 10.1094/PHYTO-98-4-0387
3. Gottwald TR. Current epidemiological understanding of citrus huanglongbing. Annu Rev Phytopathol (2010) 48:119–39. doi: 10.1146/annurev-phyto-073009-114418
4. Singerman A, Rogers ME. The economic challenges of dealing with citrus greening: the case of Florida. J Integr Pest Manage (2020) 11:1–7. doi: 10.1093/jipm/pmz037
5. Li S, Wu F, Duan Y, Singerman A, Guan Z. Citrus greening: management strategies and their economic impact. Hort Sci (2020) 55:604–12. doi: 10.21273/HORTSCI14696-19
6. Tiwari S, Mann RS, Rogers ME, Stelinski LL. Insecticide resistance in field populations of Asian citrus psyllid in Florida. Pest Manag Sci (2011) 67(10):1258–68. doi: 10.1002/ps.2181
7. Tiwari S, Stelinski LL, Rogers ME. Biochemical basis of organophosphate and carbamate resistance in Asian citrus psyllid. J Econ Entomol (2012) 105(2):540–8. doi: 10.1603/EC11228
8. Byrne FJ, Visscher PK, Leimkuehler B, Fischer D, Grafton-Cardwell EE, Morse JG. Determination of exposure levels of honey bees foraging on flowers of mature citrus trees previously treated with imidacloprid. Pest Manag Sci (2014) 70(3):470–82. doi: 10.1002/ps.3596
9. Fleming D, Musser F, Reisig D, Greene J, Taylor S, Parajulee M, et al. Effects of transgenic Bacillus thuringiensis cotton on insecticide use, heliothine counts, plant damage, and cotton yield: a meta-analysis, 1996-2015. PloS One (2018) 13(7):e0200131. doi: 10.1371/journal.pone.0200131
10. Calles-Torrez V, Knodel JJ, Boetel MA, Doetkott CD, Podliska KK, Ransom JK, et al. Transgenic Bt corn, soil insecticide, and insecticidal seed treatment effects on corn rootworm (Coleoptera: chrysomelidae) beetle emergence, larval feeding injury, and corn yield in North Dakota. J Econ Entomol (2017) 111:348–60. doi: 10.1093/jee/tox297
11. Li Y, Zhang Q, Liu Q, Meissle M, Yang Y, Wang Y, et al. Bt rice in China – focusing the nontarget risk assessment. Plant Biotechnol J (2017) 15(10):1340–5. doi: 10.1111/pbi.12720
12. Shelton AM, Tang JD, Roush RT, Metz TD, Earle ED. Field tests on managing resistance to Bt-engineered plants. Nat Biotechnol (2000) 18(3):339–42. doi: 10.1038/73804
13. Yin Y, Wang C, Xiao D, Liang Y, Wang Y. Advances and perspectives of transgenic technology and biotechnological application in forest trees. Front Plant Sci (2021) 12:786328. doi: 10.3389/fpls.2021.786328
14. Crickmore N, Berry C, Panneerselvam S, Mishra R, Connor TR, Bonning BC. A structure-based nomenclature for Bacillus thuringiensis and other bacteria-derived pesticidal proteins. J Invertebr Pathol (2021) 186:107438. doi: 10.1016/j.jip.2020.107438
15. Setamou M, Tarshis Moreno A, Patt JM. Source or sink? the role of residential host plants in Asian citrus psyllid infestation of commercial citrus groves. J Econ Entomol (2022) 115(2):438–45. doi: 10.1093/jee/toab249
16. Fernandez-Luna MT, Kumar P, Hall DG, Mitchell AD, Blackburn MB, Bonning BC. Toxicity of Bacillus thuringiensis-derived pesticidal proteins Cry1Ab and Cry1Ba against Asian citrus psyllid, Diaphorina citri (Hemiptera). Toxins (Basel) (2019) 11(3):1–13. doi: 10.3390/toxins11030173
17. Dorta SO, Balbinotte J, Monnerat R, Lopes JRS, da Cunha T, Zanardi OZ, et al. Selection of Bacillus thuringiensis strains in citrus and their pathogenicity to Diaphorina citri (Hemiptera: liviidae) nymphs. Insect Sci (2020) 27(3):519–30. doi: 10.1111/1744-7917.12654
18. Ravanfar R, Achor DS, Killiny N, Shilts T, Chen Y, El-Mohtar C, et al. Genetic modification of Bergera koenigii for expression of the bacterial pesticidal protein Cry1Ba1. Front Plant Sci (2022) 13:899624. doi: 10.3389/fpls.2022.899624
19. Fouquaert E, Hanton SL, Brandizzi F, Peumans WJ, Van Damme EJ. Localization and topogenesis studies of cytoplasmic and vacuolar homologs of the galanthus nivalis agglutinin. Plant Cell Physiol (2007) 48(7):1010–21. doi: 10.1093/pcp/pcm071
20. Dutt M, Ananthakrishnan G, Jaromin MK, Brlansky RH, Grosser JW. Evaluation of four phloem-specific promoters in vegetative tissues of transgenic citrus plants. Tree Physiol (2012) 32(1):83–93. doi: 10.1093/treephys/tpr130
21. Miyata LY, Harakava R, Stipp LC, Mendes BM, Appezzato-da-Gloria B, de Assis Alves Mourao Filho F. GUS expression in sweet oranges (Citrus sinensis l. osbeck) driven by three different phloem-specific promoters. Plant Cell Rep (2012) 31(11):2005–13. doi: 10.1007/s00299-012-1312-2
22. Orbović V, Grosser JW. Citrus transformation using juvenile tissue explants. In: Wang K, editor. Agrobacterium protocols-methods in molecular biology. USA: Humana Press Inc (2015). p. 245–57.
23. Orbović V, Grosser JW. Citrus: sweet orange (Citrus sinensis L. osbeck ‘Valencia’) and carrizo citrange [Citrus sinensis (L.) osbeck x Poncirus trifoliata (L.) raf.]. In: Wang K, editor. Agrobacterium protocols-methods in molecular biology. USA: Humana Press Inc (2006). p. 177–89.
24. Dutt M, Li ZT, Dhekney SA, Gray DJ. Co-Transformation of grapevine somatic embryos to produce transgenic plants free of marker genes. Methods Mol Biol (2012) 847:201–13. doi: 10.1007/978-1-61779-558-9_17
25. Livak KJ, Schmittgen TD. Analysis of relative gene expression data using real-time quantitative PCR and the 2(-delta delta C(T)) method. Methods (2001) 25(4):402–8. doi: 10.1006/meth.2001.1262
26. de Maagd RA, Weemen-Hendriks M, Stiekema W, Bosch D. Bacillus thuringiensis Delta-endotoxin Cry1C domain III can function as a specificity determinant for Spodoptera exigua in different, but not all, Cry1-Cry1C hybrids. Appl Environ Microbiol (2000) 66(4):1559–63. doi: 10.1128/AEM.66.4.1559-1563.2000
27. Bassham DC, Raikhel NV. An arabidopsis VPS45p homolog implicated in protein transport to the vacuole. Plant Physiol (1998) 117:407–15. doi: 10.1104/pp.117.2.407
28. Bradford MM. A rapid and sensitive method for the quantitation of microgram quantities of protein utilizing the principle of protein-dye binding. Anal Biochem (1976) 72:248–54. doi: 10.1016/0003-2697(76)90527-3
29. Johnston N, Stansly P, Stelinski LL. Secondary hosts of Asian citrus psyllid, Diaphorina citri kuwayama: survivorship and preference. J Appl Entomol (2019) 143:921–8. doi: 10.1111/jen.12673
30. Spurr AR. A low-viscosity epoxy resin embedding medium for electron microscopy. J Ultrastruct Res (1969) 26(1):31–43. doi: 10.1016/S0022-5320(69)90033-1
31. Reynolds ES. The use of lead citrate at high pH as an electron-opaque stain in electron microscopy. J Cell Biol (1963) 17:208–12. doi: 10.1083/jcb.17.1.208
32. Kaplan EL, Meier P. Nonparametric estimation from incomplete observations. J Am Stat Assoc (1958) 53:457–81. doi: 10.1080/01621459.1958.10501452
33. Team R. RStudio: integrated development for R. PBC, Boston, MA: RStudio (2020). Available at: https://wwwrstudiocom/.
34. Pasquali G, Orbovic V, Grosser JW. Transgenic grapefruit plants expressing the PAPETALA-IPTgp gene exhibit altered expression of PR genes. Plant Cell Tissue Organ Cult (2009) 97:215–23. doi: 10.1007/s11240-009-9517-y
35. Tsai JH, Liu YH. Biology of Diaphorina citri (Homoptera: psyllidae) on four host plants. J Econ Entomol (2000) 93(6):1721–5. doi: 10.1603/0022-0493-93.6.1721
36. Wenninger EJ, Stelinski LL, Hall DG. Roles of olfactory cues, visual cues, and mating status in orientation of Diaphorina citri kuwayama (Hemiptera: psyllidae) to four different host plants. Environ Entomol (2009) 38(1):225–34. doi: 10.1603/022.038.0128
37. Ebert TA, Backus EA, Shugart HJ, Rogers ME. Behavioral plasticity in probing by Diaphorina citri (Hemiptera, liviidae): ingestion from phloem versus xylem is influenced by leaf age and surface. J Insect Behav (2018) 31(2):119–37. doi: 10.1007/s10905-018-9666-0
38. Adams JR, McClintock JT. Nuclear polyhedrosis viruses of insects. In: Adams JR, Bonami JR, editors. Atlas of invertebrate viruses. Boca Raton: CRC Press (1991). p. 87–204.
39. Willett DS, George J, Willett NS, Stelinski LL, Lapointe SL. Machine learning for characterization of insect vector feeding. PloS Comput Biol (2016) 12(11):e1005158. doi: 10.1371/journal.pcbi.1005158
40. Ammar el D, Richardson ML, Abdo Z, Hall DG, Shatters RG Jr. Differences in stylet sheath occurrence and the fibrous ring (sclerenchyma) between xCitroncirus plants relatively resistant or susceptible to adults of the Asian citrus psyllid Diaphorina citri (Hemiptera: liviidae). PloS One (2014) 9(10):e110919. doi: 10.1371/journal.pone.0110919
41. Stelinski LL. Ecological aspects of the vector-borne bacterial disease, citrus greening (Huanglongbing): dispersal and host use by Asian citrus psyllid. Diaphorina citri Kuwayama. Insects. (2019) 10(7):1–11. doi: 10.3390/insects10070208
42. Wang G, Dong Y, Liu X, Yao G, Yu X, Yang M. The current status and development of insect-resistant genetically engineered poplar in China. Front Plant Sci (2018) 9:1408. doi: 10.3389/fpls.2018.01408
43. Pierre MO, Salvatierra-Miranda J, Rivera MJ, Etxeberria E, Gonzalez P, Vincent CI. White and red-dyed kaolin particle films reduce Asian citrus psyllid populations, delay huanglongbing infection, and increase citrus growth. Crop Prot (2021) 150:105792. doi: 10.1016/j.cropro.2021.105792
44. Hall DG, Lapointe SL, Wenninger EJ. Effects of a particle film on biology and behavior of Diaphorina citri (Hemiptera: psyllidae) and its infestations in citrus. J Econ Entomol (2007) 100(3):847–54. doi: 10.1603/0022-0493(2007)100[847:EOAPFO]2.0.CO;2
45. Miller JR, Cowles RS. Stimulo-deterrent diversion: a concept and its possible application to onion maggot control. J Chem Ecol (1990) 16(11):3197–212. doi: 10.1007/BF00979619
46. Yan H, Zeng J, Zhong G. The push-pull strategy for citrus psyllid control. Pest Manag Sci (2015) 71(7):893–6. doi: 10.1002/ps.3915
47. Beloti VH, Santos F, Alves GR, Bento JMS, Yamamoto PT. Curry leaf smells better than citrus to females of Diaphorina citri (Hemiptera: liviidae). Arthropod-Plant Interact (2017) 11:709–16. doi: 10.1007/s11829-017-9524-6
48. Tomaseto AF, Krugner R, Lopes JRS. Effect of plant barriers and citrus leaf age on dispersal of Diaphorina citri (Hemiptera: liviidae). J Appl Entomol (2016) 140:91–102. doi: 10.1111/jen.12249
49. Eduardo WI, Silva AC, Volpe HXL, Alquezar B, Pena L, Miranda MP. Push-pull and kill strategy for Diaphorina citri control in citrus orchards. Entomologia Experimentalis Applicata (2023) 71:287–99. doi: 10.1111/eea.13273
50. Beloti VH, Alves GR, Coletta-Filho HD, Yamamoto PT. The Asian citrus psyllid host Murraya koenigii is immune to citrus huanglongbing pathogen ‘Candidatus Liberibacter asiaticus’. Phytopathology (2018) 108(9):1089–94. doi: 10.1094/PHYTO-01-18-0012-R
51. Wunderlich S, Gatto KA. Consumer perception of genetically modified organisms and sources of information. Adv Nutr (2015) 6(6):842–51. doi: 10.3945/an.115.008870
52. Gassmann AJ, Reisig DD. Management of insect pests with Bt crops in the United States. Annu Rev Entomol (2023) 68:31–49. doi: 10.1146/annurev-ento-120220-105502
53. Tabashnik BE, Fabrick JA, Carriere Y. Global patterns of insect resistance to transgenic Bt crops: the first 25 years. J Econ Entomol (2023). doi: 10.1093/jee/toac183
54. Ni M, Ma W, Wang X, Gao M, Dai Y, Wei X, et al. Next-generation transgenic cotton: pyramiding RNAi and Bt counters insect resistance. Plant Biotechnol J (2017) 15(9):1204–13. doi: 10.1111/pbi.12709
55. Lewis-Rosenblum H, Martini X, Tiwari S, Stelinski LL. Seasonal movement patterns and long-range dispersal of Asian citrus psyllid in Florida citrus. J Econ Entomol (2015) 108(1):3–10. doi: 10.1093/jee/tou008
56. Hall DG, Hentz MG. Seasonal flight activity by the Asian citrus psyllid in east central Florida. Entomol Exp Appl (2011) 139:75–85. doi: 10.1111/j.1570-7458.2011.01108.x
57. Kobori Y, Nakata T, Ohto Y, Takasu F. Dispersal of adult Asian citrus psyllid, Diaphorina citri kuwayama (Homoptera: psyllidae), the vector of citrus greening disease, in artificial release experiments. Appl Entomol Zool (2011) 46:27–30. doi: 10.1007/s13355-010-0004-z
58. Mishra R, Guo Y, Kumar P, Canton PE, Tavares CS, Banerjee R, et al. Streamlined phage display library protocols for identification of insect gut binding peptides highlight peptide specificity. Curr Res Insect Sci (2021) 1:100012. doi: 10.1016/j.cris.2021.100012
59. Baum JA, Sukuru UR, Penn SR, Meyer SE, Subbarao S, Shi X, et al. Cotton plants expressing a hemipteran-active Bacillus thuringiensis crystal protein impact the development and survival of Lygus hesperus (Hemiptera: miridae) nymphs. J Econ Entomol (2012) 105(2):616–24. doi: 10.1603/EC11207
60. Chougule NP, Li H, Liu S, Linz LB, Narva KE, Meade T, et al. Retargeting of the Bacillus thuringiensis toxin Cyt2Aa against hemipteran insect pests. Proc Natl Acad Sci USA (2013) 110(21):8465–70. doi: 10.1073/pnas.1222144110
61. Banerjee R, Flores-Escobar B, Chougule NP, Canton PE, Dumitru R, Bonning BC. Peptide mediated, enhanced toxicity of a bacterial pesticidal protein against southern green stink bug. Microb Biotechnol (2022) 15(7):2071–82. doi: 10.1111/1751-7915.14030
Keywords: genetic transformation, pesticidal protein, Bacillus thuringiensis, Bt toxin, insect resistant transgenic plants
Citation: Orbović V, Ravanfar SA, Achor DS, Shilts T, Ibanez-Carrasco F, Banerjee R, El-Mohtar C, Stelinski LL and Bonning BC (2023) Cry1Ba1-mediated toxicity of transgenic Bergera koenigii and Citrus sinensis to the Asian citrus psyllid Diaphorina citri. Front. Insect Sci. 3:1125987. doi: 10.3389/finsc.2023.1125987
Received: 16 December 2022; Accepted: 06 April 2023;
Published: 24 April 2023.
Edited by:
Peng He, Guizhou University, ChinaReviewed by:
Jeffrey A. Fabrick, Agricultural Research Service (USDA), United StatesXingliang Wang, Nanjing Agricultural University, China
Copyright © 2023 Orbović, Ravanfar, Achor, Shilts, Ibanez-Carrasco, Banerjee, El-Mohtar, Stelinski and Bonning. This is an open-access article distributed under the terms of the Creative Commons Attribution License (CC BY). The use, distribution or reproduction in other forums is permitted, provided the original author(s) and the copyright owner(s) are credited and that the original publication in this journal is cited, in accordance with accepted academic practice. No use, distribution or reproduction is permitted which does not comply with these terms.
*Correspondence: Bryony C. Bonning, YmJvbm5pbmdAdWZsLmVkdQ==
†Present address: Seyed Ali Ravanfar, Research and Development, InnerPlant, Davis, CA, United States
Freddy Ibanez-Carrasco, Department of Entomology, Texas A&M AgriLife Research, Weslaco, TX, United States