- 1Faculty of Environmental and Earth Science, Hokkaido University, Sapporo, Japan
- 2Marine Science Program, University of South Carolina, Columbia, SC, USA
- 3Southeast Environmental Research Center, Department of Chemistry and Biochemistry, Florida International University, Miami, FL, USA
- 4Department of Biological Sciences, University of South Carolina, Columbia, SC, USA
Excitation emission matrix (EEM) fluorescence spectroscopy coupled with parallel factor analysis (PARAFAC) is commonly used to investigate the dynamics of dissolved organic matter (DOM). However, a lack of direct comparisons with known biomolecules makes it difficult to substantiate the molecular composition of specific fluorescent components. Here, coincident surface-water measurements of EEMs, dissolved lignin, and total dissolved amino acids (TDAA) acquired in the northern Gulf of Mexico were used to investigate the relationships between specific fluorescent components and DOM biomolecules. Two terrestrial humic-like components identified by EEM-PARAFAC using samples obtained from river to offshore waters were strongly linearly correlated with dissolved lignin concentrations. In addition, changes in terrestrial humic-like abundance were correlated with those in lignin phenol composition, suggesting such components are largely derived from lignin and its alteration products. By applying EEM-PARAFAC to offshore samples, two protein-like components were obtained. The tryptophan-like component was strongly correlated with TDAA concentrations, corroborating the suggested protein/peptide origin of this component. The ratios of tryptophan-like component to tyrosine-like component or dissolved organic carbon (DOC) concentrations were also correlated with DOC-normalized yields of TDAA, suggesting these proxies are useful indicators of the bioavailability of DOM in marine waters of the studied ecosystem.
Introduction
In natural waters, a fraction of dissolved organic matter (DOM, <0.2–0.7 μm pore-size filter) absorbs light (chromophoric DOM, CDOM), and a component of this light-absorbing fraction fluoresces (fluorescent DOM, FDOM) (Stedmon and Nelson, 2015). In the 1990s, Coble et al. (1990) introduced excitation emission matrix (EEM) spectroscopy as a means to characterize the fluorescence features of FDOM. The fluorescence properties of FDOM were categorized into three humic-like and two protein-like fluorophores (Coble, 1996). Although Coble's categorization has been well accepted and widely used in qualitative studies of FDOM composition, recent chemometric techniques for characterizing EEMs have enabled more quantitative evaluations of FDOM composition (Stedmon et al., 2003; Boehme et al., 2004; Murphy et al., 2014). Parallel factor analysis (PARAFAC) enables to decompose an EEM into statistically independent fluorescent components (Stedmon et al., 2003). This technique is often applied to gain insights about the environmental dynamics of these different fluorescent components (e.g., Cory and McKnight, 2005; Yamashita and Jaffé, 2008; Yamashita et al., 2008; Jaffé et al., 2014).
Despite the popularity of PARAFAC, there is still a severe lack of direct evidence linking PARAFAC fluorescent components to molecularly characterized organic molecules in DOM. Lignins (Hernes et al., 2009) and tannins (Maie et al., 2007) are measurable components in DOM that are thought to be important contributors to the humic-like fluorescence. Aromatic amino acids (Yamashita and Tanoue, 2003a) and phenolic structures (Maie et al., 2007; Stedmon and Nelson, 2015) within macromolecules such as lignins and tannins are potential contributors to the protein-like fluorescence. Tannins are highly reactive (Maie et al., 2006, 2008), thereby suggesting that lignins and aromatic amino acids are the major contributors to the humic-like and protein-like fluorescence, respectively.
Previous investigations relating PARAFAC components and major organic compounds have yielded inconsistent results. Walker et al. (2009) found linear relationships between terrestrial humic-like PARAFAC components and lignin phenol concentrations in the Canadian Archipelago and Beaufort Sea surface waters. Similar observations were made in the Baltic-North Sea transition zone (Osburn and Stedmon, 2011). In contrast, no significant correlation was found between any of the PARAFAC components and lignin concentrations within the Sacramento River/San Joaquin River Delta (Hernes et al., 2009). More recently, Walker et al. (2013) noted that there is no universal linear relationship between terrestrial humic-like PARAFAC components and lignin phenol concentrations among large Arctic rivers, and attributed it to microbial processing. Thus, it appears that both source and biogeochemical processing are important factors affecting the relationship between humic-like fluorophores and lignin concentrations.
A strong correlation between tyrosine-like and tryptophan-like fluorescence intensity in EEMs, and tyrosine and tryptophan concentration was found in samples from Ise Bay to offshore waters of the Kuroshio current (Yamashita and Tanoue, 2003a). Similar correlations were found in a vertical profile from Sagami Bay (Yamashita and Tanoue, 2004). These results indicated that protein-like fluorescence was derived from aromatic amino acids (e.g., tyrosine and tryptophan) in marine environments. Yamashita and Tanoue (2003a) also found that protein-like fluorescence intensities were linearly correlated with concentrations of total dissolved amino acids (TDAA), suggesting that protein-like fluorescence can be a useful indicator of TDAA concentration. In addition, relationships between the relative contribution (%) of protein-like PARAFAC components in total PARAFAC components and the biodegradable fraction (%) of dissolved organic carbon (DOC) were found in freshwater environments (Balcarczyk et al., 2009; Fellman et al., 2009; Hood et al., 2009). A correlation between degradation rates of protein-like fluorescence intensity and DOC was also observed in coastal environments (Lønborg et al., 2010). Since carbon-normalized yields of amino acids are known to be useful molecular indicators of biodegradable DOM (Davis and Benner, 2007; Benner and Kaiser, 2011), the linkage between bioavailable protein-like components and amino acids in DOM has been assumed based on empirical evidence.
Although the number of studies directly comparing DOM fluorescence and chemical composition remains limited, FDOM research performed over the past 15 years suggests that humic-like and protein-like fluorescence can be used as proxies for lignin and amino acids concentrations in DOM, respectively. If this is correct, FDOM and its corresponding organic molecules should change not only quantitatively but also qualitatively with biogeochemical processing. For example, the compositions of humic-like FDOM and lignin phenols are known to change with sunlight irradiation (e.g., Opshal and Benner, 1998; Stedmon and Markager, 2005), and composition of protein-like FDOM and amino acid parameters (carbon-normalized yields of amino acids) co-vary with microbial processing (e.g., Davis and Benner, 2005; Cory and Kaplan, 2012). However, direct compositional comparisons between FDOM and its molecular constituents have scarcely been evaluated (Hernes et al., 2009). Thus, establishing compositional as well as quantitative relationships between FDOM and corresponding biomolecules is needed for the application of FDOM as proxies of lignin and amino acid concentrations and as indicators of bioavailability and photodegradability of DOM.
Here, we investigated how fluorescent components determined by PARAFAC were related to the concentrations and compositions of lignin phenols and amino acids in surface waters of the northern Gulf of Mexico (NGoM), the largest river-influenced ocean margin in North America. We assessed whether terrestrial humic-like and protein-like fluorescence can be used as valid proxies for the concentrations of total dissolved lignin phenols and amino acids, respectively. In addition, compositional comparisons between FDOM components and molecular parameters derived from lignin phenols and amino acids were investigated.
Materials and Methods
Study Area and Sampling
Field samples and measurements were collected during four research cruises conducted in the northern Gulf of Mexico (NGoM) in April, July, and October-November 2009 and March 2010 (Fichot and Benner, 2012, 2014; Fichot et al., 2014). The NGoM is among the world's largest river-influenced ocean margins, where the Mississippi and Atchafalaya Rivers discharge about 6.6 × 1011 m3 yr−1 of freshwater and 2.70 Tg yr−1 of DOC into the margin (Shen et al., 2012).
Surface-water samples for DOC, CDOM, EEM-PARAFAC, and TDAA analyses were collected at about 50 stations during each cruise (Figure 1 and Table 1). Samples for DOC and TDAA analysis were gravity filtered from Niskin bottles using precombusted GF/F filters (0.7-μm pore size) and stored frozen (−20°C) immediately after collection in precombusted borosilicate glass vials. Samples for CDOM and EEMs analysis were gravity filtered from Niskin bottles using Whatman Polycap Aqueous Solution (AS) cartridges (0.2-μm pore size), collected in precombusted borosilicate glass vials, and stored immediately at 4°C until analysis in the laboratory. Samples for dissolved lignin analysis were collected at 20–23 of the ~50 stations during each cruise (Table 1).
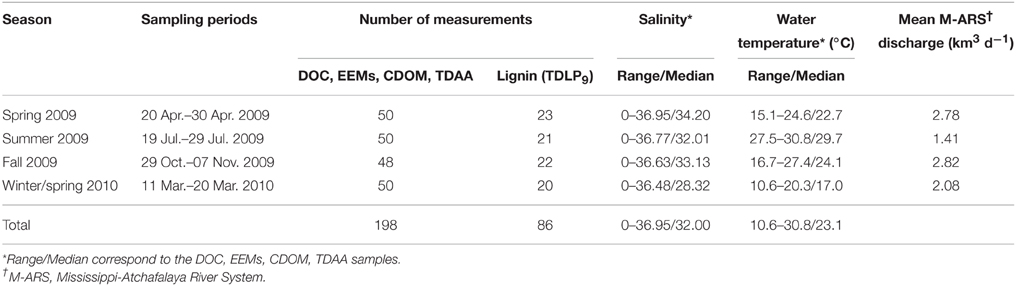
Table 1. Sampling information and environmental conditions during the four GulfCarbon cruises to the northern Gulf of Mexico.
Most samples were collected under well-mixed conditions from Niskin bottles mounted on a rosette with a conductivity–temperature–depth sensor. Samples were collected with a polypropylene bucket from the bow of the ship wherever a strong vertical salinity gradient was observed (e.g., river plume). Steep vertical salinity gradients like the ones often observed in the Mississippi River plume can be retained inside a Niskin bottle. The bucket sampling was therefore used to avoid any inconsistency that would result when collecting multiple samples from the same Niskin bottle. A total set of 198 samples for DOC/EEMs/CDOM/TDAA was collected along with a set of 86 dissolved lignin samples. The samples spanned a salinity range of 0–37, from nutrient-rich riverine waters to oligotrophic marine waters, and were collected during contrasting seasons and environmental conditions in terms of salinity, water temperature, and river discharge (Table 1). This data set is representative of the majority of water types and environmental conditions typically encountered in this river-influenced ocean margin.
DOC Analysis
DOC analysis was conducted within a month of collection by high temperature combustion using a Shimadzu total organic carbon (TOC) TOC-V analyzer equipped with an autosampler (Benner and Strom, 1993). Blanks (Milli-Q UV-Plus water) were negligible and the coefficient of variation between injections of a given sample was typically ±0.6%. Accuracy and consistency of measured DOC concentrations were checked by analyzing a deep seawater reference standard (University of Miami) every sixth sample.
CDOM Analysis and Calculation of S275–295
Samples were analyzed for EEM fluorescence within a week after the cruise. After the water samples reached room temperature, absorbance of the samples was measured from λ = 250–800 nm using a Shimadzu ultraviolet (UV)-visible UV-1601 dual-beam spectrophotometer and 10-cm cylindrical quartz cells. For highly absorbing samples, 5-cm cylindrical quartz cells, or 1-cm quartz cuvettes were used. An exponential fit of the absorbance spectrum over an optimal spectral range was used to derive an offset value that was subtracted from the absorbance spectrum (Johannessen and Miller, 2001; Fichot and Benner, 2011). Absorbance corrected for offset was then converted to Napierian absorption coefficient, ag(λ) (m−1). The dependence of ag(λ) on λ is described using Equation (1):
where λ0 < λ and S is the spectral slope coefficient in the λ0-λ nm spectral range. The spectral slope coefficient between 275 and 295 nm, S275–295, was calculated as the slope of the linear regression of ln(ag(λ)) on λ, between λ = 275 and 295 nm (Helms et al., 2008; Fichot and Benner, 2011). Here, S275–295 is reported with units of nm−1.
EEM-PARAFAC Analysis
Samples were analyzed for EEM fluorescence within 10 days after the cruise. After the water samples reached room temperature, EEM fluorescence was measured according to Yamashita et al. (2011). Briefly, emission scans from 290 to 600 nm at 2-nm intervals were acquired at excitation wavelengths between 250 and 450 nm at 5-nm intervals. Bandpass was set at 5 nm for excitation and emission. Fluorescence spectra were scanned with 0.25 s of integration time and acquired in S/R ratio mode. Inner filter effects were corrected using the absorbance spectrum according to McKnight et al. (2001), and the EEM of Milli-Q water was subtracted from sample EEMs. The excitation and emission correction files obtained every month using rhodamine b and supplied by the manufacturers, respectively, were applied for the correction of the specific instrument's components (Cory et al., 2010). Fluorescence intensities were corrected to the area under the water Raman peak (excitation = 350 nm) analyzed daily (Lawaetz and Stedmon, 2009), and were converted to quinine sulfate units.
Two PARAFAC modeling exercises were conducted using complete data set (n = 331) and samples with salinities above 30 (n = 145), respectively. Each EEM was not normalized to its total signal for both PARAFAC modeling. Thus, riverine samples with high fluorescence exert higher leverage in the first PARAFAC model. This procedure should be suitable to trace low abundance of terrigenous component in high salinity waters, but may fail to reveal minor marine-derived components. On the other hand, the gradients of fluorescence intensity is narrow in the second PARAFAC model, implying that while this model may not be best suited to capture terrigenous signals, it increases the chance for minor marine-derived components to be revealed. The modeling was carried out in MATLAB (Mathworks, Natick, MA) with the DOMFluor toolbox (Stedmon and Bro, 2008). The EEM of excitation wavelengths from 250 to 450 nm and emission wavelengths from 290 to 520 nm were used for PARAFAC modeling, and validation of the model was conducted by the split half validation and the random initialization according to Stedmon and Bro (2008).
Lignin Sampling, Extraction, and Analysis
Samples for lignin analysis (10-L) were gravity filtered from Niskin bottles using Whatman Polycap AS cartridges (0.2-μm pore size), acidified to pH ≃ 2.5–3 with sulfuric acid, and extracted onboard using C-18 cartridges (Louchouarn et al., 2000). Cartridges were stored at 4°C until elution in 30 mL of HPLC-grade methanol, and then stored at −20°C until analysis. Lignin was analyzed using the CuO oxidation method of Kaiser and Benner (2012). Concentrations of lignin phenols were measured as trimethylsilyl derivatives using an Agilent 7890 gas chromatograph equipped with a Varian DB5-MS capillary column and an Agilent 5975 mass selective detector. The concentrations of nine lignin phenols were measured in this study: p-hydroxybenzaldehyde (PAL), p-hydroxyacetophenone (PON), p-hydroxybenzoic acid (PAD), vanillin (VAL), acetovanillone (VON), vanillic acid (VAD), syringaldehyde (SAL), acetosyringone (SON), syringic acid (SAD). The sum of nine p-hydroxyl, vanillyl and syringyl lignin phenols (TDLP9) are reported in units of nmol L−1.
Amino Acid Hydrolysis and Analysis
The amino acids were analyzed using an Agilent 1100 high performance liquid chromatography system equipped with a fluorescence detector (Excitation: 330 nm; Emission: 450 nm). Hydrolysis and derivatization followed the approach of Kaiser and Benner (2005). In brief, water samples (100 μl) were dried with pure nitrogen gas and hydrolyzed using a vapor-phase technique with 6 mol L−1 hydrochloric acid at 150°C for 32.5 min in a CEM Mars 5000 microwave. After hydrolysis samples were neutralized and then separated as o-phthaldialdehyde (OPA) derivatives on a LiChrosphere RP18 column (4.6 × 150 mm, 5 μm). Sixteen amino acids were included in the analysis: aspartic acid + asparagine (Asx), glutamic acid + glutamine (Glx), serine (Ser), glycine (Gly), threonine (Thr), β-alanine (β-Ala), arginine (Arg), alanine (Ala), γ-aminobutyric acid (γ-Aba), tyrosine (Tyr), valine (Val), phenylalanine (Phe), isoleucine (Ile), and lysine (Lys).
Concentrations of total dissolved amino acids (TDAA) were the sum of the sixteen amino acids. DOC-normalized yields of TDAA were calculated as the percentage of DOC measured as amino acids using Equation (2):
where [DOC] and [TDAA-C] are concentrations of bulk DOC and carbon measured in total dissolved amino acids, respectively. β-Ala and γ-Aba are two non-protein amino acids thought to be products of diagenesis (Cowie and Hedges, 1994) and therefore they were not included in the calculation of TDAA yields.
Results and Discussion
Riverine inputs exert a strong influence on the surface distribution of DOM in the NGoM (Figure 2). The concentrations of DOC, TDLP9, and TDAA were much higher in the rivers than in ocean waters (salinity > 36.5), and displayed linear relationships with salinity (r2 = 0.82, 0.64, and 0.64, respectively). The strong influence of riverine inputs was also evident from the seasonal variability of DOC, TDLP9, and TDAA concentrations in the riverine end-members. Furthermore, the Atchafalaya River is also enriched in bioavailable DOM relative to the Mississippi River (Chen and Gardner, 2004; Conmy et al., 2004; Shen et al., 2012; Fichot and Benner, 2014), and this important difference between riverine end-members was also evident from the variability of DOC, TDLP9, and TDAA (Figure 2). This DOM enrichment has been shown to result from the interaction of the Atchafalaya River with its productive, cypress-dominated floodplain (Shen et al., 2012).
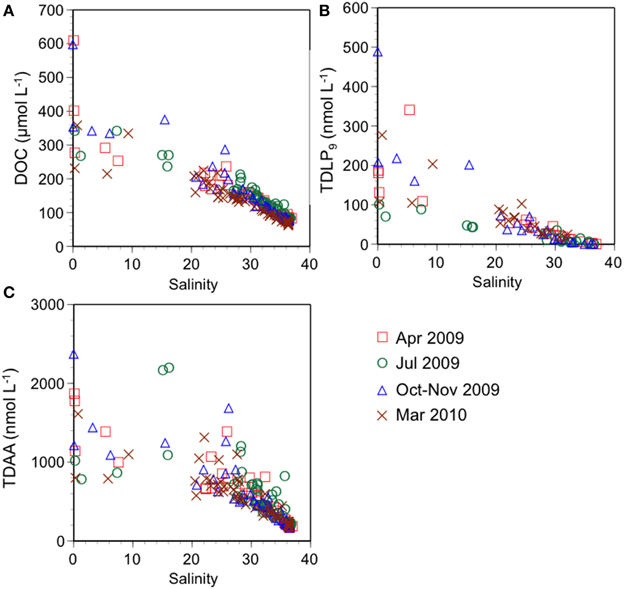
Figure 2. Relationships between (A) dissolved organic carbon (DOC), (B) total dissolved lignin phenols (TDLP9), (C) total dissolved amino acids (TDAA) and salinity.
PARAFAC Components
The significant contribution of terrigenous DOM was evident in the 0–30 salinity range of the NGoM. S275–295, a tracer of terrigenous DOM, exhibited a non-linear dependence on salinity, indicating DOC was dominated by marine DOC at salinities >30 (Fichot and Benner, 2012). Marine DOC concentrations were found to be highest at mid salinities (28–30) throughout the study period (Shen et al., pers. comm.). Two PARAFAC models were developed in the present study because optical parameters (e.g., fluorescence intensities and spectra) can be influenced by the physicochemical environment (Osburn et al., 2014), as well as biochemical components (e.g., lignin phenols and amino acids). The Gulf of Mexico (GoM) model was developed using all the data, and was used to evaluate the mixing of terrigenous and marine FDOM, and the photochemical and microbial processing of FDOM. The open ocean (OP) model was developed using high salinity (>30) samples to provide a more suitable evaluation of the dynamics of autochthonous (marine) FDOM.
A total of five and six PARAFAC components were obtained for the GoM and OP models, respectively (Figure 3; Supplemental Figure 1; Table 2). Two components from each model were similar (i.e., GoM3 and OP5; GoM4 and OP3; Figures 3C,D). GoM4 and OP3 had fluorescence maxima at longest emission wavelength among PARAFAC components. Similar components were previously found in riverine and coastal environments, and were categorized as terrigenous (Cory and McKnight, 2005; Yamashita et al., 2008, 2010; Kowalczuk et al., 2009). They were also found to be rich in fulvic acid fractions extracted from soils and estuarine sediments (Santín et al., 2009). GoM3 and OP5 corresponded to terrestrial humic-like peak A according to Coble (1996). Similar components have also been shown to be photo-resistant or products of photochemical processing (Stedmon et al., 2007; Chen et al., 2010; Cawley et al., 2012). Thus, GoM3 and OP5 are potential qualitative indicators of photooxidation of FDOM in the NGoM. OP2 was unlike other components derived in the GoM model, and was characterized as a humic-like PARAFAC component of microbial origin (Cory and McKnight, 2005; Stedmon and Markager, 2005; Yamashita et al., 2010).
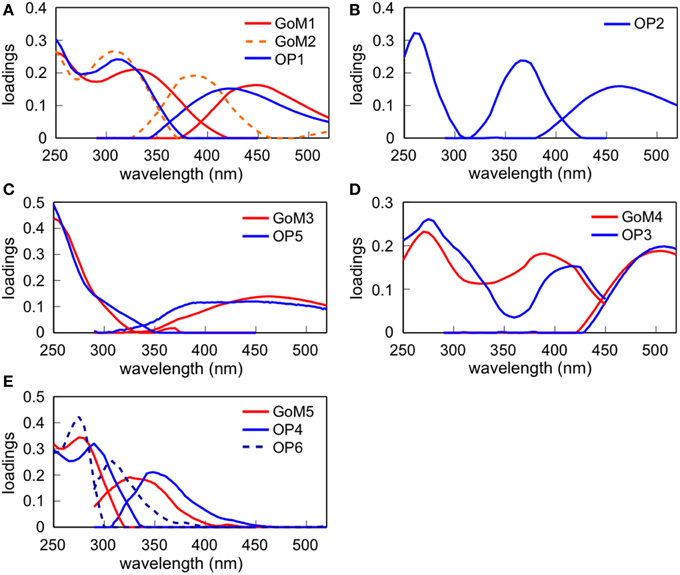
Figure 3. Excitation and emission spectra of 5 and 6 fluorescent components obtained from GoM and OP PARAFAC, respectively. (A) GoM1, GoM2, and OP1, (B) OP2, (C) GoM3 and OP5, (D) GoM4 and OP3, and (E) GoM5, OP4, and OP6.
Interestingly, GoM5 and OP1 seemed to be separated into independent components in the models. GoM5 resembles a combination of OP4 and OP6, and OP1 is likely mixture of GoM1 and GoM2. Spectral features suggest OP1 (GoM1 and GoM2) belong to the traditionally defined terrestrial humic-like peak C and/or marine (microbial) humic-like peak M (Coble, 1996). Considering the GoM model was developed using the complete data set (river, coastal, and offshore waters), the terrestrial humic-like component (GoM1) might have separated from the marine humic-like component (GoM2). The GoM5 component (OP4 and OP6) was found in the EEM region corresponding to protein-like fluorophores (Coble, 1996), and OP4 and OP6 can be categorized as tryptophan-like peak T and tyrosine-like peak B, respectively (Coble, 1996; Yamashita and Tanoue, 2003a). The OP model separated the protein-like fluorophores into tryptophan-like and tyrosine-like components, implying that spectral variability in protein-like fluorophores in offshore waters is larger than that of riverine and coastal waters.
Relationships between the Fluorescence Intensity of PARAFAC Components and the Concentrations of Lignin Phenols and Amino Acids
Fluorescence intensity of all PARAFAC components in the GoM model were positively correlated (r = 0.79–0.91, p < 0.001) with TDLP9 concentrations (Figure 4), implying the distributions of all PARAFAC components in the NGoM were mainly controlled by riverine inputs, especially on the shelf where concentrations of terrigenous DOC were high (Fichot et al., 2014). S275–295, an indicator of terrigenous DOM, decreased exponentially with increases in all PARAFAC components. The relationships between S275–295 and GoM2, GoM3, and GoM5 were variable between summer (Jul 2009) and winter/spring (Mar 2010) (Supplemental Figure 2).
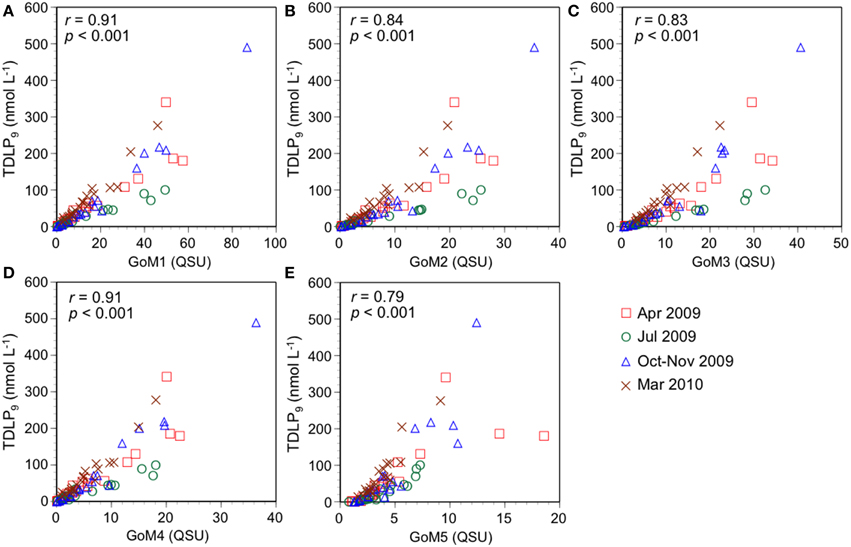
Figure 4. Relationships between lignin phenol concentrations (TDLP9) and fluorescence intensity of (A) terrestrial humic-like GoM1, (B) microbial humic-like GoM2, (C) terrestrial humic-like GoM3, (D) terrestrial humic-like GoM4, and (E) protein-like GoM5.
The correlation coefficients (r) between PARAFAC components and TDLP9 were different among PARAFAC components. The strongest correlations with TDLP9 were found for GoM1 (r = 0.91, p < 0.001, Figure 4A) and GoM4 (r = 0.91, p < 0.001, Figure 4D), which were categorized to be terrestrial humic-like components based on spectral features (Table 2). These spectral features with strongest correlation with TDLP9 suggest these components are mostly related to lignin among PARAFAC components obtained from GoM model. In addition, the strongest correlations imply that relationships between TDLP9 and GoM1 or GoM4 are less variable among seasons. Strongest correlations between terrestrial humic-like GoM1 and GoM4 agreed with previous findings in coastal Canadian Arctic surface waters (Walker et al., 2009), implying that GoM1 and GoM4 can be robust proxies for terrestrial humic-like DOM. The lowest correlation coefficient was found between GoM5 and TDLP9 (r = 0.79, p < 0.001, Figure 4E). GoM5 was categorized as a protein-like component from fluorescence characteristics (Table 2).
The correlation coefficients were of moderate strength for GoM2 vs. TDLP9 (r = 0.84, p < 0.001, Figure 4B) and GoM3 vs. TDLP9 (r = 0.83, p < 0.001, Figure 4C), suggesting that biogeochemical processing might affect the relationships. GoM2 was categorized as a marine (microbial) humic-like component based on spectral characteristics (Table 2). Likewise, addition of marine-derived components in shelf waters could affect the relationship between GoM2 vs. TDLP9. Non-conservative mixing of a marine (microbial) humic-like component was also observed in low- to mid-salinity waters in the estuaries of central Japan and southeastern Alaska (Yamashita et al., 2008; Fellman et al., 2010), suggesting that fluorescence of GoM2 in this study could originate from microbial products rather than lignin.
GoM3 was characterized as a terrestrial humic-like component (Table 2) but was weakly correlated with TDLP9, unlike GoM1 and GoM4. The relationship between GoM3 and TDLP9 in July 2009 was different from that in other seasons (Figure 4C). The ratio of GoM3 to TDLP9 (GoM3/TDLP9) in the river samples was higher during summer (0.32–0.39) than during other seasons (0.08–0.17) (Table 3), and likely affected the relationship between GoM3 and TDLP9 in the shelf waters. However, the GoM3/TDLP9 ratio increased from river to offshore waters (salinity > 30), irrespective of differences among seasons (Table 3). Photochemical alterations of lignin were enhanced during summer (Fichot and Benner, 2012), and the seasonal and spatial patterns of the GoM3/TDLP9 ratio indicate GoM3 could be more resistant to photodegradation compared with river and offshore TDLP9. The photo-resistant nature of GoM3 is consistent with previous observations for similar PARAFAC components (Table 2; Stedmon et al., 2007; Chen et al., 2010; Cawley et al., 2012). GoM3 values corresponding to S275–295 values in the 0.02–0.03 range were slightly higher in summer compared to winter/spring (Supplemental Figure 2), suggesting a photo-resistant characteristic of GoM3 compared with S275–295. Some DOM occurs as supramolecular assemblies of small molecules (Peuravuori and Pihlaja, 2004; Romera-Castillo et al., 2014), and the increase in S275–295 could be related to disruption of the DOM macrostructure during photooxidation. The molecular weight of lignin has been shown to decrease during photooxidation (Opshal and Benner, 1998). It is possible that the destruction of supramolecular assemblies reduces fluorescence self-quenching of fluorescent molecules with other molecules, without changing lignin phenol concentrations.
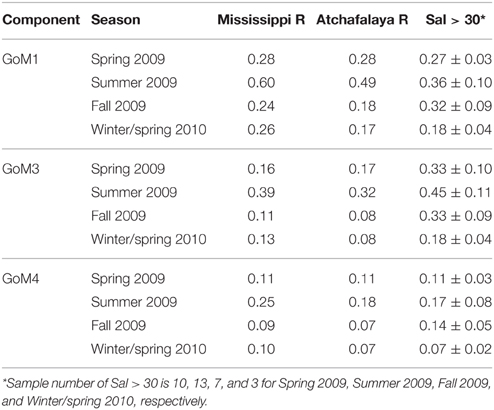
Table 3. Ratio of terrestrial humic-like components to TDLP9(GoMX / TDLP9) for Mississippi River, Atchafalaya River and offshore (Sal > 30) samples.
The relationships of GoM1 and GoM4 with TDLP9 were also slightly different in summer than during other seasons (Figures 4A,D). The GoM1/TDLP9 and GoM4/TDLP9 ratios in river waters were higher during summer (Table 3). However, these ratios did not increase consistently from river to offshore waters (Table 3), suggesting that seasonal differences in relationships among TDLP9 and GoM4 as well as GoM1 were due to variability among fluorophores rather than photooxidation. Interestingly, relationships between S275–295 and GoM4 as well as GoM1 were similar between summer and winter/spring (Supplemental Figure 2), implying that physical mixing and photooxidation were largely responsible for maintaining these relationships.
All PARAFAC components derived from the GoM model were positively correlated with TDAA (r = 0.81, p < 0.001 for GoM1; r = 0.84, p < 0.001 for GoM2; r = 0.85, p < 0.001 for GoM3; r = 0.82, p < 0.001 for GoM4; r = 0.83, p < 0.001 for GoM5, respectively). The correlation coefficient between TDAA and the corresponding fluorescent component, i.e., tryptophan-like GoM5, was similar to those between TDAA and the humic-like components GoM1-4. The relationship between TDAA and the tryptophan-like GoM5 component varied with salinity, with a stronger correlation (r = 0.80, p < 0.001) occurring in high salinity (>30) waters (Figure 5). This suggests that the physicochemical environment of the DOM (e.g., ionic strength, pH) could affect the TDAA-GoM5 relationship. Given the large inputs of terrigenous DOM in low-salinity regions, these variable relationships with salinity indicate that terrigenous DOM might obscure the autochthonous GoM5-TDAA relationship. Differences in the biochemical compositions of land plants and phytoplankton could also affect the GoM5-TDAA relationship across the salinity gradient. For example, land plants have a group of structural glycoproteins containing certain amino acids (e.g., hydroxyproline) that are largely absent from plankton (Kieliszewski and Lamport, 1994). Tyrosine is typically 4–10 mol%, whereas tryptophan is typically absent in glycoproteins (Roberts et al., 1985). In addition, terrestrial phenolic compounds such as tannins (Maie et al., 2007, 2008) might contribute to GoM5 in low salinity waters.
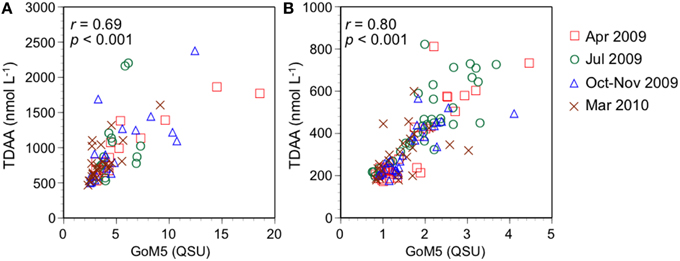
Figure 5. Relationships between TDAA concentration and fluorescence intensity of protein-like GoM5 for (A) low salinity (<30) waters and (B) high salinity (>30) waters.
The PARAFAC OP model used offshore samples (salinity > 30; Figure 3, Table 2) and identified two protein-like components (tryptophan-like OP4 and tyrosine-like OP6). Tyrosine-like OP6 was weakly correlated with concentrations of tyrosine (r = 0.46, p < 0.001; Figure 6A) and TDAA (r = 0.54, p < 0.001; Figure 6B). Yamashita and Tanoue (2003a) also found that tyrosine-like fluorescence intensity was not well correlated with tyrosine concentrations for offshore samples (tyrosine concentration is < 10 nmol L−1). Two factors can contribute to a weak correlation between tyrosine-like OP6 and tyrosine concentrations: (1) OP6 corresponds to a mixture of tyrosine and phenylalanine, another aromatic amino acid, (2) the fluorescence quantum yield of tyrosine in DOM is variable. The fluorescence characteristics of phenylalanine are similar to those of tyrosine (Lakowicz, 2006) and can potentially affect OP6. However, the fluorescence quantum yield of free phenylalanine is approximately one fifth of that of free tyrosine (Lakowicz, 2006), and therefore the influence of phenylalanine on OP6 is probably limited. As for the second factor, tyrosine fluorescence in peptides and proteins is often quenched due to energy transfer to tryptophan molecules, or due to interactions with the peptide chain (Lakowicz, 2006). Thus, fluorescence quenching of tyrosine molecules seems a likely cause for the weak relationship between tyrosine-like OP6 and either TDAA or tyrosine concentrations.
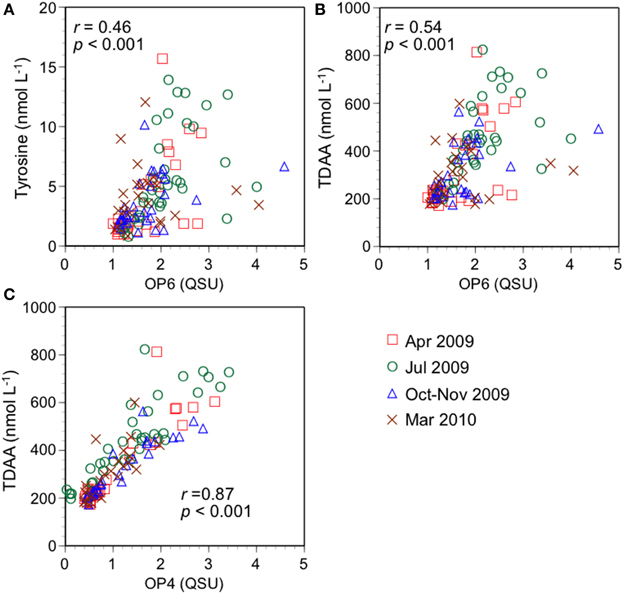
Figure 6. Relationships between quantitative amino acid parameters and fluorescence intensity of protein-like components. (A) Tyrosine vs. tyrosine-like OP6, (B) TDAA vs. tyrosine-like OP6, and (C) TDAA vs. tryptophan-like OP4.
A strong relationship was observed between tryptophan-like OP4 and concentrations of TDAA (r = 0.87, p < 0.001; Figure 6C), and bears similarities to the one observed between GoM5 and concentrations of TDAA at high salinity (Figure 5B). Proteins/peptides containing both tyrosine and tryptophan molecules generally emit only tryptophan fluorescence due to energy transfer (Lakowicz, 2006). Thus, all tryptophan molecules in DOM potentially emit fluorescence. The strong correlation between TDAA concentrations and tryptophan-like OP4 implies that mol percentages of tryptophan in TDAA are not highly variable in the NGoM, which is consistent with previous tryptophan analysis for the bay and offshore waters (Yamashita and Tanoue, 2003b). While Yamashita and Tanoue (2003a) did not use PARAFAC modeling, they found no correlation between tryptophan-like fluorescence intensity and concentrations of TDAA as well as tryptophan in offshore waters. The shoulder of the humic-like peak possibly overlaps with the peak position of tryptophan fluorescence. The PARAFAC analysis separates the tryptophan-like fluorescence from humic-like fluorescence, and the significant correlation between TDAA and OP4 found in this study appears to be due to the separation of OP4 from humic-like components by PARAFAC.
Compositional Relationships between Lignin and Humic-like Components
Lignin phenol concentrations have a major influence on the fluorescence intensity of the terrestrial humic-like components derived from PARAFAC modeling. Photochemical processes alter the composition and size of lignin and humic-like fluorophores, and these alterations are apparent in various chemical and optical parameters (e.g., Opshal and Benner, 1998; Stedmon and Markager, 2005). The ratios of GoM components to TDLP9 (Table 3), and relationships between S275–295 and the GoM components (Supplemental Figure 2) suggest that GoM3 is more resistant to photodegradation compared to GoM1. The ratio of two terrestrial humic-like components (GoM3/GoM1) was established as an indicator of the extent of photooxidation of terrestrial humic-like components. A high GoM3/GoM1 ratio indicates greater photochemical alteration of terrigenous humic-like fluorophores. The acid-to-aldehyde ratios of vanillyl, syringyl, and p-hydroxyl phenols typically increase during photooxidation of lignin (Opshal and Benner, 1998; Spencer et al., 2009; Benner and Kaiser, 2011). The GoM3/GoM1 ratio was strongly correlated with the acid-to-aldehyde ratios of p-hydroxy and vanillyl phenol (Figures 7B,C), but was weakly correlated with that of syringyl phenols (Figure 7A). The p-hydroxy phenols are the least photoreactive components of lignin, whereas vanillyl and syringyl phenols have similar reactivities, with syringyl phenols tending to be slightly more photoreactive (Opshal and Benner, 1998; Benner and Kaiser, 2011). The GoM3/GoM1 ratio was strongly correlated with the ratio of p-hydroxy and vanillyl phenols (P/V), but not with the ratio of syringyl and vanillyl phenols (S/V) (Figures 7D,E).
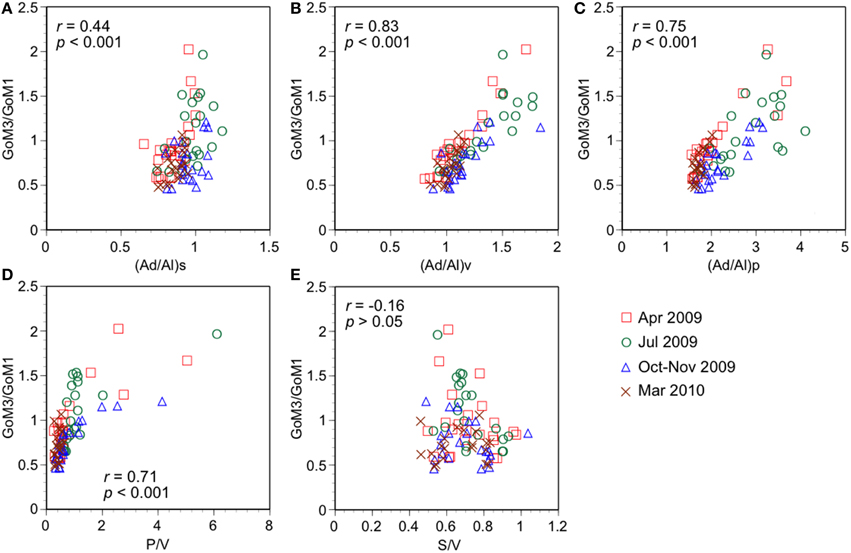
Figure 7. Relationships between ratio of terrestrial humic-like components and lignin phenol parameters. GoM3/GoM1 vs. (A) ratio of acid to aldehyde in syringyl phenols, (Ad/Al)s, (B) ratio of acid to aldehyde in vanillyl phenols, (Ad/Al)v, (C) ratio of acid to aldehyde in p-hydroxy phenols, (Ad/Al)p, (D) ratio of p-hydroxy and vanillyl phenols (P/V), and (E) ratio of syringyl and vanillyl phenols (S/V).
Compositional variations among source vegetation and mixing processes on the Louisiana margin could have influenced the observed relationships between optical and chemical parameters. The Atchafalaya River has greater contributions of lignin from floodplain vegetation, such as cypress, than the Mississippi River (Shen et al., 2012). Cypress is a gymnosperm and its lignin is composed of p-hydroxy and vanillyl phenols and no syringyl phenols (Hedges and Mann, 1979; Opshal and Benner, 1995). Thus, the weak correlations between GoM3/GoM1 and S/V and acid-to-aldehyde ratio of syringyl phenols could be related to the varying compositions of lignin from the primary sources of terrestrial humic-like components. In conclusion, photochemical alterations of terrestrial humic-like components are accompanied with changes of lignin phenols, even though variations in the source and composition of lignin phenols influence the observed relationships.
Compositional Relationships between Amino Acids and Protein-like Components
The DOC-normalized yields of amino acids (TDAA %DOC) have been demonstrated to be molecular indicators of DOM bioavailability in freshwater and marine environments (Davis and Benner, 2007; Benner and Kaiser, 2011; Shen et al., 2015). The use of this biochemical indicator in the NGoM was further validated by the shipboard bioassay experiments in spring 2010, which confirmed that amino acids were preferentially consumed and showed lower yield values in DOM of lower bioavailability (Shen et al. pers. comm.).
Amino acid yields (TDAA %DOC) were compared with protein-like components and related parameters obtained from the OP PARAFAC modeling using offshore samples (salinity > 30) on the Louisiana margin (Figure 8). Amino acid yields were mostly between 0.6 and 1.6% DOC, a range indicative of low concentrations of labile DOM (Davis and Benner, 2007). The relative contribution of protein-like PARAFAC components, which were found to be related to the bioavailable fraction of DOC in freshwater environments (Balcarczyk et al., 2009; Fellman et al., 2009; Hood et al., 2009), were negatively correlated with TDAA yields (r = −0.68, p < 0.001; Figure 8A). A possible reason for the negative correlation is the complex nature of humic-like components. In addition to autochthonous production of protein-like components, the photodegradation and dilution of terrestrial humic-like components are possibly important factors controlling the relative abundance of protein-like components. Thus, the relative contribution of protein-like components does not appear to be a sensitive indicator of biodegradable DOM in high salinity waters of the NGoM.
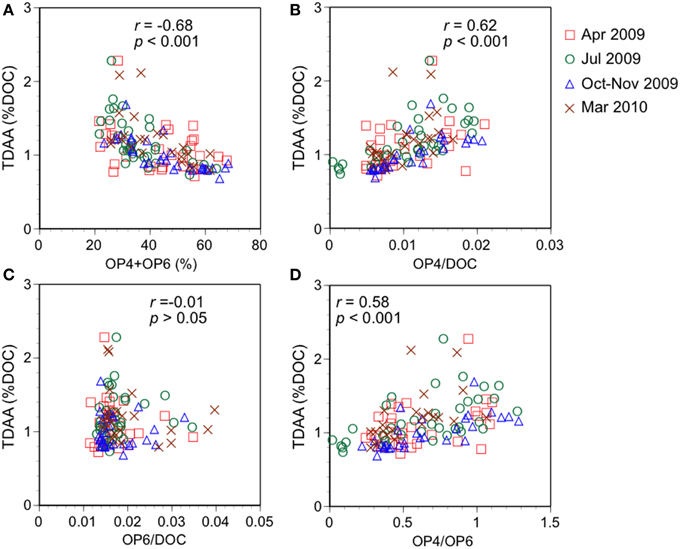
Figure 8. Relationships between TDAA yields (%DOC) and (A) relative abundance of protein-like components, (B) OP4/DOC, (C), OP6/DOC, and (D) ratio of protein-like components (OP4/OP6).
The ratios of protein-like components to DOC, which is conceptually similar to the DOC-normalized yields of amino acids (i.e., ratio of amino acid-C to DOC), were compared with amino acid yields (Figures 8B,C). The ratio of tryptophan-like OP4 to DOC concentrations (OP4/DOC) was weakly but significantly correlated with TDAA yields (r = 0.62, p < 0.001; Figure 8B). However, OP6/DOC did not correlate significantly with TDAA yields (p > 0.05; Figure 8C), which was possibly due to fluorescence quenching of tyrosine molecules. Previous analysis of fluorescence properties of marine DOM in the surface Sagami Bay (Japan) showed that only tryptophan-like fluorescence was found in high molecular weight fractions, while tyrosine-like fluorescence was dominant in low molecular weight fraction (Yamashita and Tanoue, 2004). High molecular weight DOM is more easily degraded by microbes and has higher amino acid yields than low molecular weight DOM (Amon and Benner, 1994, 1996; Benner and Amon, 2015). Thus, tryptophan-like OP4 could be related to reactive high-molecular-weight DOM, while tyrosine-like OP6 might be quenching or derived from less reactive tyrosine-containing low-molecular-weight DOM. The ratio of tryptophan-like OP4 to tyrosine-like OP6 (OP4/OP6) was weakly correlated with TDAA yields (r = 0.58, p < 0.001; Figure 8D), confirming different reactivities between tryptophan-like and tyrosine-like components. Overall, the relationships obtained from the spatial distributions of TDAA yields and protein-like components in high salinity waters of the NGoM suggest that DOC-normalized yields of the tryptophan-like component (i.e., OP4/DOC in this study) and the ratio of tryptophan-like to tyrosine-like component (i.e., OP4/OP6 in this study) are useful optical indicators of the bioavailability of marine DOM.
Conclusions and Implications
The transition from river water to seawater includes large changes in the physicochemical properties that can affect optical parameters without affecting biochemical parameters (Osburn et al., 2014). This study provides important insights about the fidelity of EEM-PARAFAC components as tracers of biochemical components of DOM in a river-influenced ocean margin.
• Terrestrial humic-like components, characterized as peak C type (GoM1) and fulvic acid type (GoM4), were useful tracers of lignin phenol concentrations.
• The ratio of terrestrial humic-like components, i.e., the ratio of peak A type (GoM3) to peak C type (GoM1), appeared to be indicative of the degree of photooxidation.
• Terrigenous DOM likely affected the relationship between the protein-like component (GoM5) and aromatic amino acids and total dissolved amino acid concentrations in riverine and coastal environments.
• The tryptophan-like component (OP4) was found to be a useful indicator of total dissolved amino acid concentrations in offshore environments.
• The ratios of tryptophan-like component to DOC (OP4/DOC) and tryptophan-like component to tyrosine-like component (OP4/OP6) are potentially useful indicators of DOM bioavailability in this system.
This study indicated that certain EEM-PARAFAC components are tightly linked with corresponding compounds, and suggested that other components are affected by variable sources and biogeochemical processing. Such influences need to be considered when evaluating the fidelity of EEM-PARAFAC for tracing fluorescent DOM in the coastal ocean. Further studies are needed to develop general optical parameters that represent the quantity and quality of lignin using variable samples that cover a wide range of aquatic environments. In particular, linkages between optical properties and lignins should be explored in the open ocean where lignin phenol concentrations are quite low (Opshal and Benner, 1997). In addition, most DOC-normalized yields of amino acids observed in offshore waters of the NGoM were < 1.6% DOC, indicating the concentrations of labile DOM were generally low. Further comparisons of florescence based indicators with amino acids yields in highly bioavailable DOM are necessary for expanding the application of optical parameters in a wide range of environments.
Author Contributions
RB, RJ, CF, and YY contributed to the design of the study. CF collected the water samples. YY conducted the EEM-PARAFAC analyses, CF analyzed samples for TDLP9 and DOC, and YS analyzed the TDAA. YY and CF performed data analyses with comments from other authors. YY wrote the initial draft of the manuscript and all authors contributed to its revision.
Conflict of Interest Statement
The authors declare that the research was conducted in the absence of any commercial or financial relationships that could be construed as a potential conflict of interest.
Acknowledgments
We thank Steven E. Lohrenz and Wei-Jun Cai for providing berths on the GulfCarbon cruises, and Leanne Powers, Kevin Martin, and the crews of the R/V Cape Hatteras and the R/V Hugh Sharp for their assistance with sample collection onboard. Funding for this work was provided by National Science Foundation grant 0850653 to RB. Additional funding was provided through the George Barley endowment to RJ. This is contribution #764 from the Southeast Environmental Research Center.
Supplementary Material
The Supplementary Material for this article can be found online at: http://journal.frontiersin.org/article/10.3389/fmars.2015.00092
References
Amon, R. M. W., and Benner, R. (1994). Rapid cycling of high-molecular-weight dissolved organic matter in the ocean. Nature 369, 549–552. doi: 10.1038/369549a0
Amon, R. M. W., and Benner, R. (1996). Bacterial utilization of different size classes of dissolved organic matter. Limnol. Oceanogr. 41, 41–51. doi: 10.4319/lo.1996.41.1.0041
Balcarczyk, K. L., Jones, J. B. Jr. Jaffe, R., and Maie, N. (2009). Stream dissolved organic matter bioavailability and composition in watersheds underlain with discontinuous permafrost. Biogeochemistry 94, 255–270. doi: 10.1007/s10533-009-9324-x
Benner, R., and Amon, R. M. W. (2015). The size-reactivity continuum of major bioelements in the ocean. Annu. Rev. Mar. Sci. 7, 185–205. doi: 10.1146/annurev-marine-010213-135126
Benner, R., and Kaiser, K. (2011). Biological and photochemical transformations of amino acids and lignin phenols in riverine dissolved organic matter. Biogeochemistry 102, 209–222. doi: 10.1007/s10533-010-9435-4
Benner, R., and Strom, M. (1993). A critical evaluation of the analytical blank associated with DOC measurements by high-temperature catalytic oxidation. Mar. Chem. 41, 153–160. doi: 10.1016/0304-4203(93)90113-3
Boehme, J., Coble, P., Conmy, R., and Stovall-Leonard, A. (2004). Examining CDOM fluorescence variability using principal component analysis: seasonal and regional modeling of three-dimensional fluorescence in the Gulf of Mexico. Mar. Chem. 89, 3–14. doi: 10.1016/j.marchem.2004.03.019
Cawley, K. M., Wolski, P., Mladenov, N., and Jaffé, R. (2012). Dissolved organic matter biogeochemistry along a transect of the Okavango delta, Botswana. Wetlands 32, 475–486. doi: 10.1007/s13157-012-0281-0
Chen, M., Price, R. M., Yamashita, Y., and Jaffé, R. (2010). Comparative study of dissolved organic matter from groundwater and surface water in the Florida coastal Everglades using multi-dimensional spectrofluorometry combined with multivariate statistics. Appl. Geochem. 25, 872–880. doi: 10.1016/j.apgeochem.2010.03.005
Chen, R. F., and Gardner, G. B. (2004). High-resolution measurements of chromophoric dissolved organic matter in the Mississippi and Atchafalaya River plume regions. Mar. Chem. 89, 103–125. doi: 10.1016/j.marchem.2004.02.026
Coble, P. G. (1996). Characterization of marine and terrestrial DOM in seawater using excitation-emission matrix spectroscopy. Mar. Chem. 51, 325–346. doi: 10.1016/0304-4203(95)00062-3
Coble, P. G., Green, S., Blough, N. V., and Gagosian, R. B. (1990). Characterization of dissolved organic matter in the Black Sea by fluorescence spectroscopy. Nature 348, 432–435. doi: 10.1038/348432a0
Conmy, R. N., Coble, P. G., Chen, R. F., and Gardner, G. B. (2004). Optical properties of colored dissolved organic matter in the Northern Gulf of Mexico. Mar. Chem. 89, 127–144. doi: 10.1016/j.marchem.2004.02.010
Cory, R. M., and Kaplan, L. A. (2012). Biological lability of streamwater fluorescent dissolved organic matter. Limnol. Oceanogr. 57, 1347–1360. doi: 10.4319/lo.2012.57.5.1347
Cory, R. M., and McKnight, D. M. (2005). Fluorescence spectroscopy reveals ubiquitous presence of oxidized and reduced quinones in dissolved organic matter. Environ. Sci. Technol. 39, 8142–8149. doi: 10.1021/es0506962
Cory, R. M., Miller, M. P., McKnight, D. M., Guerard, J. J., and Miller, P. L. (2010). Effect of instrument-specific response on the analysis of fulvic acid fluorescence spectra. Limnol. Oceanogr. Meth. 8, 67–78. doi: 10.4319/lom.2010.8.0067
Cowie, G. L., and Hedges, J. I. (1994). Biogeochemical indicators of diagenetic alteration in natural organic matter mixtures. Nature 369, 304–307. doi: 10.1038/369304a0
Davis, J., and Benner, R. (2005). Seasonal trends in the abundance, composition and bioavailability of particulate and dissolved organic matter in the Chukchi/Beaufort Seas and western Canada Basin. Deep-Sea Res. Part II 52, 3396–3410. doi: 10.1016/j.dsr2.2005.09.006
Davis, J., and Benner, R. (2007). Quantitative estimates of labile and semi-labile dissolved organic carbon in the western Arctic Ocean: a molecular approach. Limnol. Oceanogr. 52, 2434–2444. doi: 10.4319/lo.2007.52.6.2434
Fellman, J. B., Hood, E., D'Amore, D. V., Edwards, R. T., and White, D. (2009). Seasonal changes in the chemical quality and biodegradability of dissolved organic matter exported from soils to streams in coastal temperate rainforest watersheds. Biogeochemistry 95, 277–293. doi: 10.1007/s10533-009-9336-6
Fellman, J. B., Spencer, R. G. M., Hernes, P. J., Edwards, R. T., D'Amore, D. V., and Hood, E. (2010). The impact of glacier runoff on the biodegradability and biochemical composition of terrigenous dissolved organic matter in near-shore marine ecosystems. Mar. Chem. 121, 112–122. doi: 10.1016/j.marchem.2010.03.009
Fichot, C. G., and Benner, R. (2011). A novel method to estimate DOC concentrations from CDOM absorption coefficients in coastal waters. Geophys. Res. Lett. 38, L03610. doi: 10.1029/2010GL046152
Fichot, C. G., and Benner, R. (2012). The spectral slope coefficient of chromophoric dissolved organic matter (S275–295) as a tracer of terrigenous dissolved organic carbon in river-influenced ocean margins. Limnol. Oceanogr. 57, 1453–1466. doi: 10.4319/lo.2012.57.5.1453
Fichot, C. G., and Benner, R. (2014). The fate of terrigenous dissolved organic carbon in a river-influenced ocean margin. Global Biogeochem. Cycles 28, 300–318. doi: 10.1002/2013GB004670
Fichot, C. G., Lohrenz, S. E., and Benner, R. (2014). Pulsed, cross-shelf export of terrigenous dissolved organic carbon to the Gulf of Mexico. J. Geophys. Res. 119, 1176–1194. doi: 10.1002/2013JC009424
Hedges, J. I., and Mann, D. C. (1979). The characterization of plant issues by their lignin oxidation products. Geochim. Cosmoshim. Acta 43, 1803–1807. doi: 10.1016/0016-7037(79)90028-0
Helms, J. R., Stubbins, A., Ritchie, J. D., Minor, E. C., Kieber, D. J., and Mopper, K. (2008). Absorption spectral slopes and slope ratios as indicators of molecular weight, source, and photobleaching of chromophoric dissolved organic matter. Limnol. Oceanogr. 53, 955–969. doi: 10.4319/lo.2008.53.3.0955
Hernes, P. J., Bergamaschi, B. A., Eckard, R. S., and Spencer, R. G. M. (2009). Fluorescence-based proxies for lignin in freshwater dissolved organic matter. J. Geophys. Res. 114, G00F03. doi: 10.1029/2009jg000938
Hood, E., Fellman, J., Spencer, R. G. M., Hernes, P. J., Edwards, R., D'Amore, D., et al. (2009). Glaciers as a source of ancient and labile organic matter to the marine environment. Nature 462, 1044–1047. doi: 10.1038/nature08580
Jaffé, R., Cawley, K. M., and Yamashita, Y. (2014). “Applications of excitation emission matrix fluorescence with parallel factor analysis (EEM-PARAFAC) in assessing environmental dynamics of natural dissolved organic matter (DOM) in aquatic environments: a review,” in Physicochemical Characterization of Dissolved Organic Matter: Impact on Natural and Engineered Systems, ed F. Rosario-Ortiz (Washington, DC: ACS Publications), 27–73.
Johannessen, S. C., and Miller, W. L. (2001). Quantum yield for the photochemical production of dissolved inorganic carbon in seawater. Mar. Chem. 76, 271–283. doi: 10.1016/S0304-4203(01)00067-6
Kaiser, K., and Benner, R. (2005). Hydrolysis-induced racemization of amino acids. Limnol. Oceanogr.-Methods 3, 318–325. doi: 10.4319/lom.2005.3.318
Kaiser, K., and Benner, R. (2012). Characterization of lignin by gas chromatography and mass spectrometry using a simplified CuO oxidation method. Anal. Chem. 84, 459–464. doi: 10.1021/ac202004r
Kieliszewski, M. J., and Lamport, D. T. (1994). Extensin: repetitive motifs, functional sites, post−translational codes, and phylogeny. Plant J. 5, 157–172. doi: 10.1046/j.1365-313X.1994.05020157.x
Kowalczuk, P., Durako, M. J., Young, H., Kahn, A. E., Cooper, W. J., and Gonsior, M. (2009). Characterization of dissolved organic matter fluorescence in the South Atlantic Bight with use of PARAFAC model: interannual variability. Mar. Chem. 113, 182–196. doi: 10.1016/j.marchem.2009.01.015
Lawaetz, A. J., and Stedmon, C. A. (2009). Fluorescence intensity calibration using the Raman scatter peak of water. Appl. Spectrosc. 63, 936–940. doi: 10.1366/000370209788964548
Lønborg, C., Álvarez-Salgad, X. A., Davidson, K., Martínez-García, S., and Teira, E. (2010). Assessing the microbial bioavailability and degradation rate constants of dissolved organic matter by fluorescence spectroscopy in the coastal upwelling system of the Ría de Vigo. Mar. Chem. 119, 121–129. doi: 10.1016/j.marchem.2010.02.001
Louchouarn, P., Opsahl, S., and Benner, R. (2000). Isolation and quantification of dissolved lignin from natural waters using solid-phase extraction and GC/MS. Anal. Chem. 72, 2780–2787. doi: 10.1021/ac9912552
Maie, N., Jaffé, R., Miyoshi, T., and Childers, D. L. (2006). Quantitative and qualitative aspects of dissolved organic carbon leached from senescent plants in an oligotrophic wetland. Biogeochemistry 78, 285–314. doi: 10.1007/s10533-005-4329-6
Maie, N., Pisani, O., and Jaffé, R. (2008). Mangrove tannins in aquatic ecosystems: their fate and possible influence on dissolved organic carbon and nitrogen cycling. Lomnol. Oceanogr. 53, 160–171. doi: 10.4319/lo.2008.53.1.0160
Maie, N., Scully, N. M., Pisani, O., and Jaffé, R. (2007). Composition of a protein-like fluorophore of dissolved organic matter in coastal wetland and estuarine ecosystems. Water Res. 41, 563–570. doi: 10.1016/j.watres.2006.11.006
McKnight, D. M., Boyer, E. W., Westerhoff, P. K., Doran, P. T., Kulbe, T., and Andersen, D. T. (2001). Spectrofluorometric characterization of aquatic fulvic acids for determination of precursor organic material and general structural properties. Limnol. Oceanogr. 46, 38–48. doi: 10.4319/lo.2001.46.1.0038
Murphy, K. R., Bro, R., and Stedmon, C. A. (2014). “Chemometric analysis of organic matter fluorescence,” in Aquatic Organic Matter Fluorescence, eds P.G. Coble, J. Lead, A. Baker, D. M. Reynolds, and R. G. M Spencer (New York, NY: Cambridge University Press), 339–375.
Opshal, S., and Benner, R. (1995). Early diagenesis of vascular plant-tissues—lignin and cutin decomposition and biogeochemical implications. Geochim. Cosmochim. Acta 59, 4889–4904. doi: 10.1016/0016-7037(95)00348-7
Opshal, S., and Benner, R. (1997). Distribution and cycling of terrigenous dissolved organic matter in the ocean. Nature 386, 480–482. doi: 10.1038/386480a0
Opshal, S., and Benner, R. (1998). Photochemical reactivity of dissolved lignin in river and ocean waters. Limnol. Oceanogr. 43, 1297–1304. doi: 10.4319/lo.1998.43.6.1297
Osburn, C. L., Del Vecchio, R., and Boyd, T. J. (2014). “Physicochemical effects on dissolved organic matter fluorescence in natural waters,” in Aquatic Organic Matter Fluorescence, eds P. G. Coble, J. Lead, A. Baker, D. M. Reynolds and R. G. M. Spencer (New York, NY: Cambridge University Press), 233–277.
Osburn, C. L., and Stedmon, C. A. (2011). Linking the chemical and optical properties of dissolved organic matter in the Baltic–North Sea transition zone to differentiate three allochthonous inputs. Mar. Chem. 126, 281–294. doi: 10.1016/j.marchem.2011.06.007
Peuravuori, J., and Pihlaja, K. (2004). Preliminary study of Lake dissolved organic matter in light of nanoscale supramolecular assembly. Environ. Sci. Technol. 38, 5958–5967. doi: 10.1021/es040041l
Roberts, K., Grief, C., Hills, G. J., and Shaw, P. (1985). Cell wall glycoproteins: structure and function. J. Cell Sci. 2, 105–127. doi: 10.1242/jcs.1985.Supplement_2.6
Romera-Castillo, C., Chen, M., Yamashita, Y., and Jaffé, R. (2014). Fluorescence characteristics of size-fractionate dissolved organic matter: implications for a molecular assembly based structure? Water Res. 55, 40–51. doi: 10.1016/j.watres.2014.02.017
Santín, C., Yamashita, Y., Otero, X. L., Álvarez, M. Á., and Jaffé, R. (2009). Characterizing humic substances from estuarine soils and sediments by excitation-emission matrix spectroscopy and parallel factor analysis. Biogeochemistry 96, 131–147. doi: 10.1007/s10533-009-9349-1
Shen, Y., Chapelle, F. H., Strom, E. W., and Benner, R. (2015). Origins and bioavailability of dissolved organic matter in groundwater. Biogeochemistry 122, 61–78. doi: 10.1007/s10533-014-0029-4
Shen, Y., Fichot, C. G., and Benner, R. (2012). Floodplain influence on dissolved organic matter composition and export from the Mississippi–Atchafalaya River system to the Gulf of Mexico. Limnol. Oceanogr. 57, 1149–1160. doi: 10.4319/lo.2012.57.4.1149
Spencer, R. G. M., Stubbins, A., Hernes, P. J., Baker, A., Mopper, K., Aufdenkampe, A. K., et al. (2009). Photochemical degradation of dissolved organic matter and dissolved lignin phenols from the Congo River. J. Geophys. Res. 114, G03010. doi: 10.1029/2009jg000968
Stedmon, C. A., and Bro, R. (2008). Characterizing dissolved organic matter fluorescence with parallel factor analysis: a tutorial. Limnol. Oceanogr. Meth. 6, 572–579. doi: 10.4319/lom.2008.6.572
Stedmon, C. A., and Markager, S. (2005). Tracing the production and degradation of autochthonous fractions of dissolved organic matter by fluorescence analysis. Limnol. Oceanogr. 50, 1415–1426. doi: 10.4319/lo.2005.50.5.1415
Stedmon, C. A., Markager, S., and Bro, R. (2003). Tracing dissolved organic matter in aquatic environments using a new approach to fluorescence spectroscopy. Mar. Chem. 82, 239–254. doi: 10.1016/S0304-4203(03)00072-0
Stedmon, C. A., Markager, S., Tranvik, L., Kronberg, L., Slätis, T., and Martinsen, W. (2007). Photochemical production of ammonium and transformation of dissolved organic matter in the Baltic Sea. Mar. Chem. 104, 227–240. doi: 10.1016/j.marchem.2006.11.005
Stedmon, C. A., and Nelson, N. B. (2015). “The optical properties of DOM in the ocean,” in Biogeochemistry of Marine Dissolved Organic Matter, 2nd Edn., eds D. A. Hansell and C. A. Carlson (London: Academic Press), 481–508.
Walker, S. A., Amon, R. M. W., and Stedmon, C. A. (2013). Variations in high-latitude riverine fluorescent dissolved organic matter: a comparison of large Arctic rivers. J. Geophys. Res. 118, 1689–1702. doi: 10.1002/2013JG002320
Walker, S. A., Amon, R. M. W., Stedmon, C., Duan, S., and Louchouarn, P. (2009). The use of PARAFAC modeling to trace terrestrial dissolved organic matter and fingerprint water masses in coastal Canadian Arctic surface waters. J. Geophys. Res. 114, G00F06. doi: 10.1029/2009jg000990
Yamashita, Y., and Jaffé, R. (2008). Characterizing the interactions between trace metals and dissolved organic matter using excitation-emission matrix and parallel factor analysis. Environ. Sci. Technol. 42, 7374–7379. doi: 10.1021/es801357h
Yamashita, Y., Jaffé, R., Maie, N., and Tanoue, E. (2008). Assessing the dynamics of dissolved organic matter (DOM) in coastal environments by excitation emission matrix fluorescence and parallel factor analysis (EEM-PARAFAC). Limnol. Oceanogr. 53, 1900–1908. doi: 10.4319/lo.2008.53.5.1900
Yamashita, Y., Maie, N., Briceño, H., and Jaffé, R. (2010). Optical characterization of dissolved organic matter in tropical rivers of the Guayana Shield, Venezuela. J. Geophys. Res. 115, G00F10. doi: 10.1029/2009jg000987
Yamashita, Y., Panton, A., Mahaffey, C., and Jaffé, R. (2011). Assessing the spatial and temporal variability of dissolved organic matter in Liverpool Bay using excitation–emission matrix fluorescence and parallel factor analysis. Ocean Dyn. 61, 569–579. doi: 10.1007/s10236-010-0365-4
Yamashita, Y., and Tanoue, E. (2003a). Chemical characterization of protein-like fluorophores in DOM in relation to aromatic amino acids. Mar. Chem. 82, 255–271. doi: 10.1016/S0304-4203(03)00073-2
Yamashita, Y., and Tanoue, E. (2003b). Distribution and alteration of amino acids in bulk DOM along a transect from bay to oceanic waters. Mar. Chem. 82, 145–160. doi: 10.1016/S0304-4203(03)00049-5
Keywords: fluorescent dissolved organic matter, excitation-emission matrix, parallel factor analysis, lignin phenols, amino acids, northern Gulf of Mexico
Citation: Yamashita Y, Fichot CG, Shen Y, Jaffé R and Benner R (2015) Linkages among fluorescent dissolved organic matter, dissolved amino acids and lignin-derived phenols in a river-influenced ocean margin. Front. Mar. Sci. 2:92. doi: 10.3389/fmars.2015.00092
Received: 05 August 2015; Accepted: 19 October 2015;
Published: 05 November 2015.
Edited by:
Christopher Osburn, North Carolina State University, USAReviewed by:
Christian Lønborg, Australian Institute of Marine Science, AustraliaRobert Spencer, Woods Hole Research Center, USA
Copyright © 2015 Yamashita, Fichot, Shen, Jaffé and Benner. This is an open-access article distributed under the terms of the Creative Commons Attribution License (CC BY). The use, distribution or reproduction in other forums is permitted, provided the original author(s) or licensor are credited and that the original publication in this journal is cited, in accordance with accepted academic practice. No use, distribution or reproduction is permitted which does not comply with these terms.
*Correspondence: Youhei Yamashita, eWFtYXNoaXlAZWVzLmhva3VkYWkuYWMuanA=
†Present Address: Cédric G. Fichot, Jet Propulsion Laboratory, California Institute of Technology, Pasadena, USA