- 1Cooperative Institute for Marine and Atmospheric Studies, Rosenstiel School of Marine and Atmospheric Science, University of Miami, Miami, FL, USA
- 2Southeast Fisheries Science Center, National Marine Fisheries Service, Miami, FL, USA
- 3Wetland and Aquatic Research Center, US Geological Survey, Caribbean Field Station, St. John, VI, USA
- 4Center for Marine and Environmental Studies, University of the Virgin Islands, St. Thomas, VI, USA
- 5Coral Health and Disease Program, Mote Marine Laboratory and Aquarium, Sarasota, FL, USA
Disturbances such as tropical storms cause coral mortality and reduce coral cover as a direct result of physical damage. Storms can be one of the most important disturbances in coral reef ecosystems, and it is crucial to understand their long-term impacts on coral populations. The primary objective of this study was to determine trends in disease prevalence and snail predation on damaged and undamaged colonies of the threatened coral species, Acropora palmata, following an episode of heavy ocean swells in the US Virgin Islands (USVI). At three sites on St. Thomas and St. John, colonies of A. palmata were surveyed monthly over 1 year following a series of large swells in March 2008 that fragmented 30–93% of colonies on monitored reefs. Post-disturbance surveys conducted from April 2008 through March 2009 showed that swell-generated damage to A. palmata caused negative indirect effects that compounded the initial direct effects of physical disturbance. During the 12 months after the swell event, white pox disease prevalence was 41% higher for colonies that sustained damage from the swells than for undamaged colonies (df = 207, p = 0.01) with greatest differences in disease prevalence occurring during warm water months. In addition, the corallivorous snail, Coralliophila abbreviata, was 46% more abundant on damaged corals than undamaged corals during the 12 months after the swell event (df = 207, p = 0.006).
Introduction
Physical damage to coral reefs from major storms and ocean swells has both immediate and long-term consequences (Hughes and Connell, 1999). Storms can directly affect reef organisms by (i) causing physical breakage directly from high wave action, (ii) transporting loose reef rubble and damaging intact structure leading to fragmentation, (iii) resuspending sediments that physically abrade living organisms, and (iv) smothering colonies with sediments from run-off, all of which can lead to losses in coral cover (Woodley et al., 1981; Rogers et al., 1982). Yet, the potential for indirect effects following initial injury has received little attention. In terrestrial ecosystems, damage to trees from storms increases susceptibility to disease, fungi, and herbivores, including insects that may be vectors in disease transmission (Lautenschlager and Nielsen, 1999; Warillow and Mou, 1999; Gill et al., 2000). Little is known about the potential secondary effects of physical disturbance on coral reef ecosystems.
Increases in coral disease or “disease–like” mortality (Knowlton et al., 1990; Williams et al., 2008; Brandt et al., 2013) and predation (Knowlton et al., 1990) following damaging storms has been documented, suggesting that corals may become susceptible to secondary stressors following a physical disturbance. After injury, the colony presumably diverts energy to the repair and regenerating of tissue (D'Angelo et al., 2012). Tissue can be regenerated by an initial but limited amount of energetic resources (Van Woesik, 1998; Lirman, 2000) that are likely drawn from nearby, unaffected tissue (Meesters et al., 1994). The energetic costs of repair may increase susceptibility to disease by lowering immune responses of the coral (reviewed in Mullen et al., 2004). In fact, damaged corals exhibit reduced overall growth (Bak, 1983), reproduction (Rinkevich and Loya, 1979), and resistance to disease (Bak and Criens, 1981). Furthermore, lesions caused by fragmentation could be sites for the introduction of pathogens, increasing susceptibility to disease. Lesions may also attract corallivores (Brawley and Adey, 1982; Morton et al., 2002; Chong-Seng et al., 2011), which may be vectors of disease (Sussman et al., 2003; Williams and Miller, 2005; Gignoux-Wolfsohn et al., 2012).
Physical damage plays an important role in the life history of the threatened scleractinian coral, Acropora palmata. This branching coral is relatively fast-growing (Gladfelter et al., 1978) and adapted to repeated breakage, which leads to asexual reproduction through fragmentation and dispersion (Highsmith, 1982; Rogers et al., 1982). As sexual recruitment is limited in this species, likely a result of population declines and accelerating environmental challenges (Szmant, 1986; Williams et al., 2008), recovery following disturbance relies primarily on the continued growth of remaining attached colonies and successful attachment and growth of new fragments.
Despite the potential benefits of fragmentation, storm damage and disease are thought to have been the primary causes of the mass die-off of Acropora in the Caribbean beginning in the late 1970's (Gladfelter, 1982; Aronson and Precht, 2001). In Jamaica, acroporids dominated shallow reef zones before being decimated by two major hurricanes in the 1980's (Woodley et al., 1981; Woodley, 1992; Hughes, 1994). On surveyed reefs at Buck Island Reef National Monument, US Virgin Islands (USVI), A. palmata cover declined by ~90%, primarily as a result of white band disease (Anderson et al., 1986; Mayor et al., 2006). The Caribbean-wide population decline and lack of recovery of A. palmata and A. cervicornis were the impetus for their listing as “threatened” under the United States Endangered Species Act (ESA) in 2006 (Hogarth, 2006).
In late March 2008, a series of large swells generated by a winter cold front moving off the eastern coast of North America damaged many A. palmata colonies at long-term monitoring sites on the north side of St. Thomas and St. John, USVI. This event provided an opportunity to quantify the direct and indirect impacts of damage to coral colonies without confounding factors that can occur with storms, such as increased terrestrial run-off and thermal stress, as in the case of summer hurricanes. We tested the hypotheses that direct physical damage caused by a natural disturbance will be associated with an increase in disease and corallivorous snails on colonies of A. palmata.
Materials and Methods
Study Sites
Three reefs off the northern coasts of St. Thomas and St. John, USVI, were chosen as the focus for this study (Figure 1). Botany Bay is off St. Thomas (18° 21.43 N, 65° 02.03 W), and Hawksnest Bay (18° 20.84 N, 64° 46.86 W), and Haulover Bay (18° 20.99 N, 64° 40.74 W) are off St. John. An additional site, Flat Cay, located off the southwest coast of St. Thomas (18° 19.00 N, 64° 59.28 W) was protected from the swells, and served as a control site to assess the severity of swell related damage.
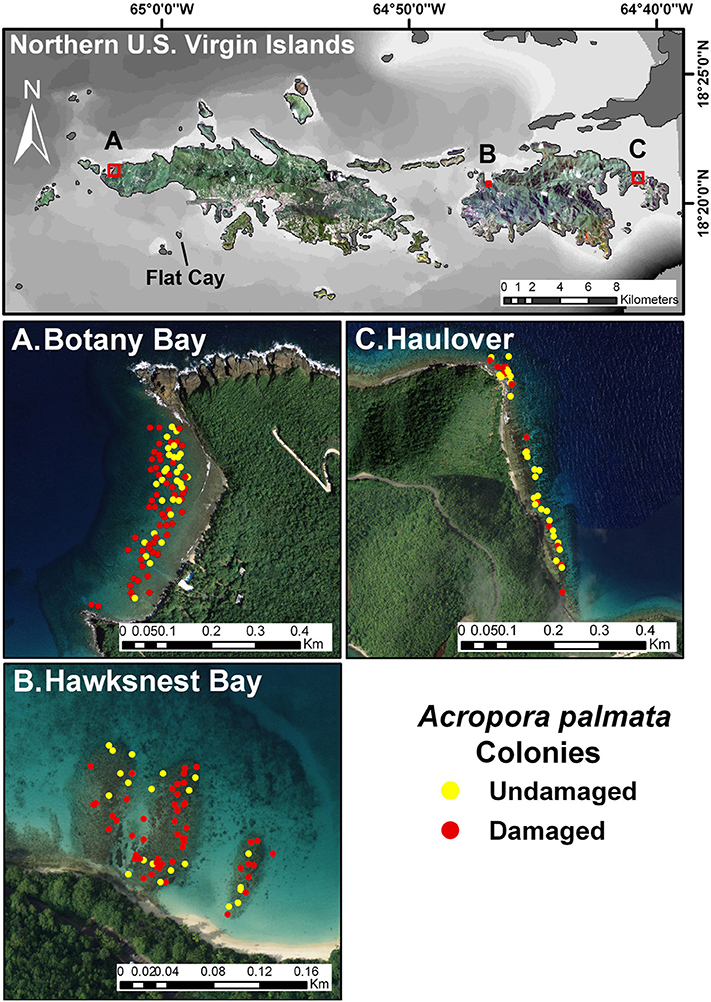
Figure 1. Map of three main research sites on the north side of St. John and St. Thomas, US Virgin Islands. Close-up views show aerial imagery of the sampling sites with the positions of damaged and undamaged colonies of Acropora palmata indicated. The southern reference site, Flat Cay, is also indicated on the map.
Long-Term Acropora palmata Monitoring
Haulover and Hawksnest were part of a long-term A. palmata monitoring program conducted by the US Geological Survey (USGS) starting in 2003 and continued by the US National Marine Fisheries Service (NMFS) beginning in 2007 (see Muller et al., 2008 and Rogers and Muller, 2012, for methods). This long-term monitoring study provided data that were compared with the findings in the current study. Monitoring began at Haulover in February 2003 and at Hawksnest in May 2004. A. palmata colonies were surveyed by University of the Virgin Islands scientists at Botany starting in September 2007 and at Flat Cay in October 2007. At each site, except Haulover, colonies were randomly selected as described below. Colony positions were marked with GPS, and colonies were surveyed monthly. Due to the lower numbers of colonies at Haulover (n = 52), every colony was surveyed monthly at this site.
Post-Disturbance Acropora palmata Sampling
After the March 2008 swell event, a subset of damaged and undamaged colonies at Botany and Hawksnest were randomly selected for repeated sampling. Individuals were identified using a random point generator in ESRI ArcView Geographic Information System (GIS) software version 9.2. Each study site was outlined using the ArcView polygon tool. Random GPS points were distributed within the polygon. The nearest A. palmata colony to the randomly generated GPS point was selected, and the colony was marked with a new GPS point to facilitate relocation. Fifty-one damaged and 42 undamaged colonies were surveyed at Botany (~1–5 m depth), and 43 damaged and 45 undamaged colonies were surveyed at Hawksnest (~1–3 m depth). At Haulover, all colonies observed on the western side of the bay were surveyed (16 damaged and 36 undamaged; ~1–4 m depth). At the control site, Flat Cay, two of the 45 colonies monitored since October 2007 were damaged by the swells (~1–6 m depth).
Coral colonies were monitored nearly monthly, except during a period of rough seas at Botany in September 2008 and March 2009. For this site, data from early April 2009 (5–7 April) were used in place of the missing March 2009 data. In situ observations of colony status (dead/alive), snail predation, physical damage, and disease were documented, and all colonies were photographed (7.1 megapixel digital camera). Predation by the corallivorous snail, Coralliophila abbreviata, was assessed as present or absent, and only recorded if snails were seen near the site of recent tissue loss (to be sure the lesion was a result of snail predation). Consistent with the long-term monitoring study referred to above, snails were removed when found and displaced from the colony ~5 to 10 m distance. Thus, snail populations assessed in each time period represented recolonization rates after removal in the previous month.
Colony size was measured for a subset of colonies (total n = 177; Botany, n = 46; Haulover, n = 49; Hawksnest, n = 82). Three colony dimensions (length, width, and height) were averaged to calculate a mean dimension (cm), and then squared to provide an index of live tissue area (cm2; see Williams and Miller, 2012). Colony dimensions were measured only for continuous spans of live tissue. If tissue isolates occurred, they were measured individually, and an index of live tissue area was calculated in the same manner. For colonies composed of multiple isolates, the sizes of individual isolates were summed to provide a measurement of total tissue area.
In situ water temperature was recorded at Hawksnest and Haulover at 1–2 m depth every 2 h and at 11 m depth at Botany every 15 min with a HOBO Water Temperature Pro v2® Data Logger - U22-001 (Onset Computer Corporation).
Colony Damage and Disease Estimates
Within 1 month after the swells in March 2008, corals were observed for signs of damage, e.g., broken branches and abrasions (examples of damage patterns are shown in Figure S1). The total percent of colony damage, including abraded areas and fracture zones where branches were broken, was estimated visually. Colonies with at least one broken branch and total recent tissue loss comprising 5% or more of the surface were categorized as damaged. Some damaged colonies were uprooted and overturned during the swells (n = 20 colonies across all sites). A colony was considered undamaged if it had no broken branches and abrasions comprising < 5% of its surface. In order to restrict the data to the effects of physical damage caused by the swells, undamaged colonies that were damaged after the March swells (n = 26) were excluded from analyses beginning with the month when damage occurred.
Formal standards for disease identification and nomenclature are lacking, resulting in inconsistencies when classifying coral diseases (Rogers, 2010). For the purpose of this study, the classification of disease was based on descriptions in Raymundo et al. (2008). Diseases were classified as either white pox disease (WPX; also referred to as white patch disease; Figure 2A) or white band disease type I (WBD; Figure 2B). An additional characteristic pattern of tissue loss was observed on overturned colonies and named “overturned syndrome” (OTS; Figure 2C) specifically for this study after colonies were observed with the condition in June 2008. This type of mortality was seen only on colonies that had been overturned by the swells and only in regions of a colony that were newly shaded after the colony was overturned or knocked free from the substrate. Some extensive dead areas associated with this condition appeared quickly (indicated by large areas of freshly exposed white skeleton) while others began as small patches and increased in size more slowly (indicated by thick algal growth in the middle of the dead area to less algal growth toward the perimeter eventually meeting a thin edge of freshly exposed white skeleton). Overturned syndrome may be an endogenous process resulting from newly shaded regions on overturned colonies, and by definition was only present on damaged colonies; therefore, no analyses were conducted for this condition. White pox disease, WBD, and OTS were noted as present or absent.
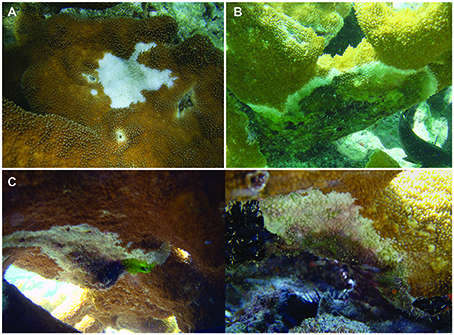
Figure 2. Photo examples for characteristic patterns of tissue loss. (A) white pox disease (B) white band disease (C) overturned syndrome (2 examples).
Wave Monitoring
Wave height, period, and direction were monitored with a 600 kHz Nortek Acoustic Wave and Current Profiler (AWAC) in a gimbaled spider mount positioned in 39.5 m depth. The current meter was located 22 km northwest of the Botany Bay study site near the northern Puerto Rican Shelf edge (18° 30.68 N, 65° 09.94 W). Waves were measured over two intervals. The first interval lasted from 7 February to 8 April 2008, and recorded waves hourly. The second interval lasted from 8 May to 18 November 2008, and recorded waves every 4 h. At each recording, waves were monitored for 1 min at a frequency of 1 Hz.
Analyses
Prevalence of WPX and WBD, expressed as a percentage of the surveyed population, was calculated as follows:
“Cumulative prevalence,” a single value for the 12-month survey period, is the proportion of colonies that had disease at least once during the survey period. “Cumulative presence” is the proportion of colonies that had snails at least once during the survey period. Differences in disease prevalence values and percent of colonies occupied by snails between damaged and undamaged corals were reported as the relative difference:
where Xa is the disease prevalence or percent of colonies with snails for damaged corals and Xb is the disease prevalence or percent of colonies with snails for undamaged corals.
Differences in colony size, expressed as an index of live tissue area, between damaged and undamaged corals was analyzed using a Mann-Whitney U test.
To test the hypotheses that damaged colonies would experience increased levels of disease and snail occupation, we compared damaged and undamaged colonies within sites and among all sites for each month following damage using a relative risk analysis. The relative risk of exposed (i.e., damaged) individuals was compared with the relative risk of non-exposed (i.e., undamaged) individuals. The relative risk, or risk ratio, was calculated as:
where the risk in exposed individuals was calculated as the prevalence of disease in damaged corals and the risk in non-exposed individuals was calculated as the prevalence of disease in undamaged corals. The relative risk was calculated using a Bayesian approach (Gelman et al., 2004; Lawson, 2013), and estimated using a binomial likelihood distribution and a uniform-Beta prior distribution. Markov Chain Monte Carlo simulations were used with Gibbs sampling in OpenBUGS to obtain an estimate of relative risk (MRC Biostatistics Unit, Cambridge, UK; Randall et al., 2014). Ninety-five percent credible intervals were calculated for each estimate of relative risk which was calculated for each month of monitoring. Credible intervals that did not include a value of one were considered significant, with a credible interval above one signifying an increased risk of disease because of damage. A credible interval below one signified a decreased risk of disease because of damage. Additionally, the range of the credible intervals provided a measurement of the confidence in each relative risk estimate, with a large range signifying high variability and low confidence in the estimate (Kruschke, 2011). A relative risk analysis was also conducted on corals that did not experience snail predation to remove the potential confounding factor of predation on disease prevalence.
To test the hypotheses that damaged colonies would have a greater frequency of disease and snail occupation, we compared damaged and undamaged colonies using a 2x2 contingency table (model II). We tested the cumulative prevalence of disease and snail occupation on damaged vs. undamaged colonies in the 12 months following physical damage from swells within sites and among all sites. Statistics were computed with a Fisher's Exact test (one-tailed), and data satisfied assumptions for a 2x2 contingency table. Regressions of monthly disease prevalence relative to average monthly water temperature are presented to describe the effect of temperature on disease prevalence for damaged and undamaged corals.
To test differences when corals initially showed signs of disease, a rank of 1 through 12 was given to each coral representing the month at which that coral first showed signs of disease during the 12-month monitoring period. Therefore, corals with higher rankings showed disease later in the monitoring period, and corals that did not show signs of disease were given the highest rank of 13. Ordinal logistic regression on these rankings was then used to test when damaged and undamaged corals first showed signs of disease.
Results
Impacts of the March 2008 Swells on Acropora palmata
Large north-northeast (~22°) swells started abruptly on 19 March 2008 0500 h, with mean wave heights quickly reaching >4 m at the AWAC sensor, and sustained wave heights above 3 m lasting 61 h until 21 March 1700 h (Figure S2). A second interval of waves above 3 m also occurred 10 days later starting on 1 April 2100 h, and lasted 38 h until 3 April 1100 h. Mean waves higher than 3 m had a longer period (mean = 13.8 s, n = 99) and a more northern component (mean = 29.4°) than typical northern swells impinging on the USVI (8.5 s, n = 2617; mean = 58°). Swells were sufficiently deep and severe to cause detectable rocking (recorded pitch/roll deviations of ± 3°) of the AWAC sensor at 39.5 m depth from 19 March 0600 h to 21 March 1900 h, whereas no rocking was detectable anywhere else in the record. In comparison, from 9 May to 18 November, wave heights reached a max of 2.7 m lasting no longer than 16 h (occurred on 26 October).
The amount of coral damage as a result of the March 2008 swells was the highest or nearly highest recorded during the present and long-term monitoring at study sites on the north coast of St. John and St. Thomas (Figure 3A). Physical damage was sustained by 62% of A. palmata colonies at the three study sites, and included broken branches, abrasions, and/or detachment from the substrate. Colonies at Botany received the greatest damage (93.3%) followed by those at Hawksnest (62.9%) and Haulover (30%). In contrast, the monitoring site off the south coast of St. Thomas (Flat Cay) had no detectable increase in damage after the March swells. Colony size, expressed as an index of live tissue area, did not differ significantly between damaged and undamaged colonies (Damaged = 6138.9 ± 735 cm2; Undamaged = 6687.3 ± 1501.2 cm2; p = 0.30; mean ± SE).
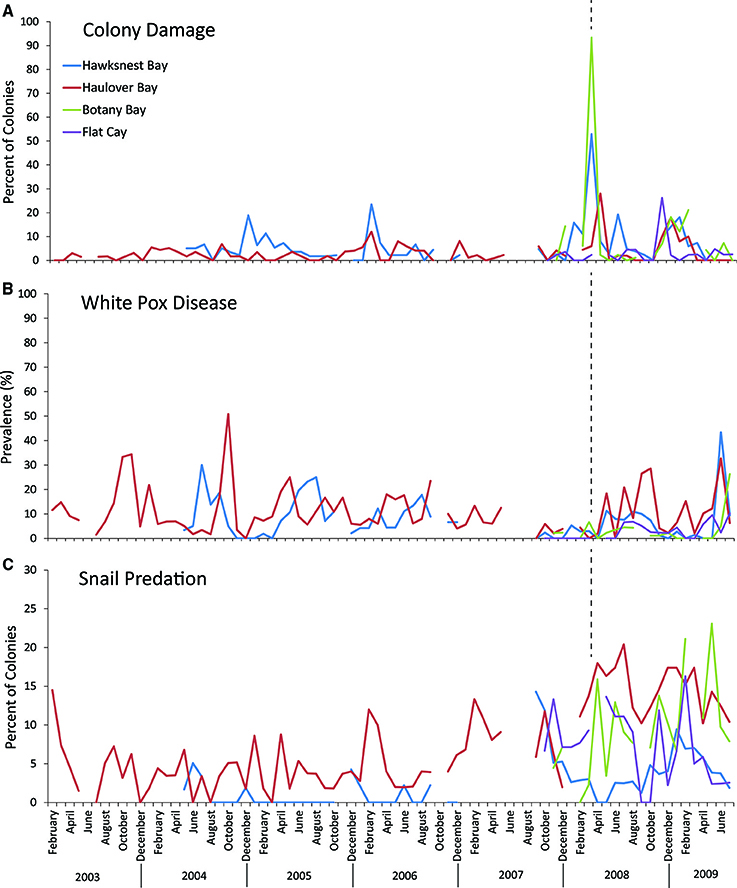
Figure 3. Long-term trends in (A) colony damage, (B) white pox disease, and (C) snail presence on selected Acropora palmata colonies from the three northern sites, Botany Bay (max. monthly n = 91 colonies, mean monthly n = 62.2 ± 6.1; mean ± SE), Haulover (max monthly n = 99, mean monthly n = 56.5 ± 1.6), Hawksnest (max. monthly n = 83, mean monthly n = 53.3 ± 2.2), and a reference site at Flat Cay (max. monthly n = 45, mean monthly n = 41.4 ± 0.9) on the south side of St. Thomas. Dotted lines represent the timing of the March 2008 swell event.
Average monthly water temperatures did not vary by more than half a degree Celsius among sites, with minimum temperatures occurring in March 2009 and maximum temperatures in August 2008 (Figure S3). Temperatures at Botany ranged from 24.7 to 29.6°C (minimum temperature at a single time point to maximum temperature at a single time point), from 24.6 to 30.2°C at Haulover, and from 24.5 to 30.7°C at Hawksnest. Cooler peak temperatures at Botany may be partly explained by the deeper position of the sensor.
Disease on Damaged and Undamaged Corals
Overall, disease prevalence after the March swells was not anomalous compared to the long-term monitoring before the March swells (Figure 3B). However, colonies damaged from the March swells had more disease in the following year than undamaged colonies (Table 1). Specifically, WPX was greater on damaged vs. undamaged colonies with average monthly prevalence values that were approximately twice as high on damaged colonies (Damaged = 9.6 ± 2.5%, Undamaged = 4.1 ± 0.8%; mean ± SE). At each site, WPX was observed on damaged colonies earlier than on undamaged colonies (Botany: L-R χ2 = 9.44, p < 0.01; Haulover: L-R χ2 = 4.08, p < 0.05; Hawksnest: L-R χ2 = 4.77, p < 0.05; Figure 4), and higher WPX prevalence on damaged colonies peaked in warmer months suggesting that temperature at least played a partial role in disease initiation (Figure 5). Monthly prevalence values showed a consistently higher risk for WPX on damaged corals during the first 8–9 months following the March swells at every monitored site. The relative risk analyses showed on average a 2-fold increase in median risk of disease within 9 months after experiencing physical damage (Figures 6A–D). However, a statistically significant increase in disease risk was found only at Haulover during the warm water months of July, September, and October (Figure 6C).
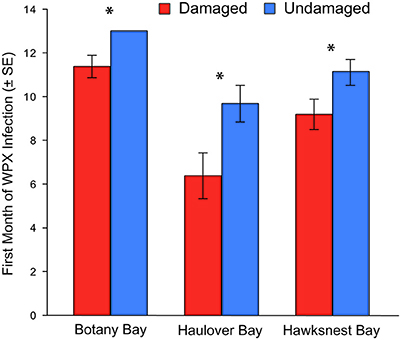
Figure 4. Month of first infection of white pox disease for damaged and undamaged corals at each site. Bars represent an average of all corals for each category. Corals that did not have white pox disease during the 12-month study were given a rank of 13. Asterisks indicate a statistically significant difference.
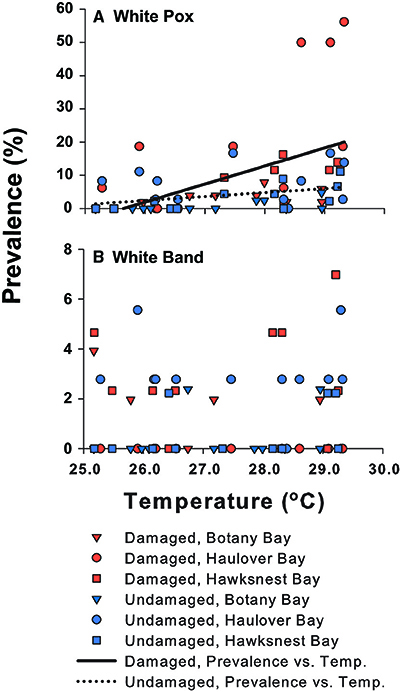
Figure 5. The prevalence of (A) white pox and (B) white band disease on Acropora palmata vs. temperature among damage states and sites. The relationship of white pox disease with temperature is shown as regression lines for damaged and undamaged colonies. White band disease was not associated with temperature.
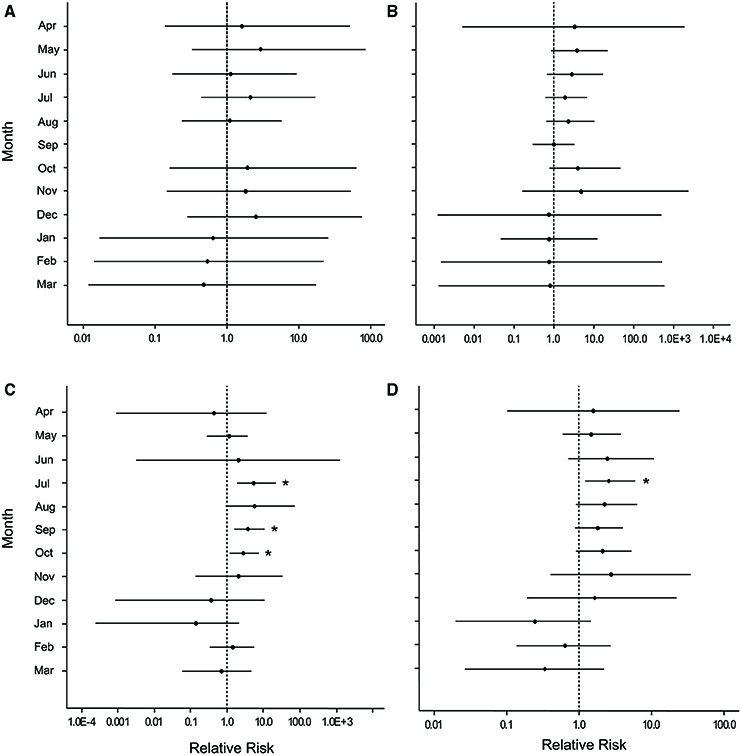
Figure 6. Caterpillar plots depicting the median (black dot) and 95% credible intervals for the relative risk of white pox disease after damage from the March swells at (A) Botany Bay, (B) Hawksnest Bay, (C) Haulover Bay, and (D) all sites combined. Asterisks indicate a statistically significant increase in disease risk.
Monthly WBD prevalence ranged from 0.5 to 3.1% (all sites and damage categories combined). White band disease prevalence did not differ between damaged and undamaged corals (Table 1), and no relationship with temperature was found (Figure 5). Overturned syndrome occurred on 90% of damaged colonies that were overturned by the March 2008 swells, and was observed on colonies beginning in June 2008. Swells occurring after March 2008 knocked free and overturned four undamaged colonies. All four soon developed signs similar to OTS. Overturned syndrome affected colonies for ~3.5 ± 2 months (mean ± SE), and showed no trend with temperature. No lesions completely healed, and tissue regeneration was minimal.
During the course of this study, five colonies died entirely: one damaged colony at Botany, one undamaged colony at Hawksnest, and three undamaged colonies at Haulover. Complete coral mortality was attributed to unidentified lesions.
Corallivorous Snails on Damaged and Undamaged Corals
After the March swells, the proportion of colonies occupied by the snail, C. abbreviata, increased by 2- to 3-fold when compared with values for colonies monitored before the March swells, and showed no temporal pattern (Figure 3C). After March 2008, the cumulative presence of snail occupation was significantly greater for damaged colonies at Botany and when all sites were combined (Table 1). Monthly surveys show there was an overall consistently higher risk of snail occupation at Haulover and Botany (and all sites combined) when a coral was damaged by the March swells with an average 1.5-fold increase in snail predation risk (Figures 7A,C,D). However, a statistically significant higher risk of snail predation was found only at Botany for the months of November and February (Figure 7A). Differences were not observed between damaged and undamaged corals at Hawksnest where snail occupation was less common (Table 1; Figure 7B).
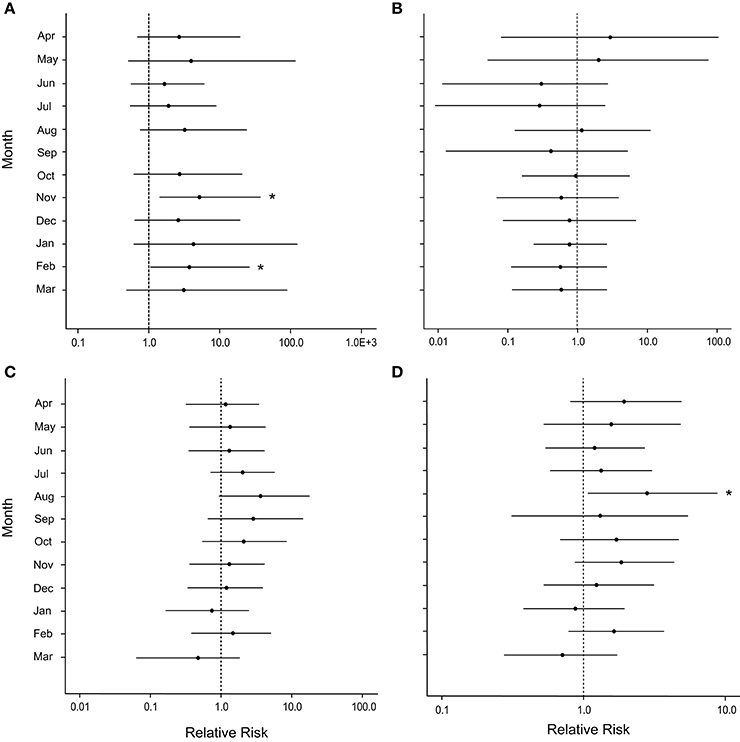
Figure 7. Caterpillar plots depicting the median (black dot) and 95% credible intervals for the relative risk of occupation by the snail, Coralliophila abbreviata, after damage from the March swells at (A) Botany Bay, (B) Hawksnest Bay, (C) Haulover Bay, and (D) all sites combined. Asterisks indicate a statistically significant increase in disease risk.
For all sites and months combined, disease was observed on more colonies with snails (55.2%) than colonies without snails (43.2%) either at the time of infestation or after (a relative difference of 21.7%). Hawksnest showed the greatest relative difference (31%) followed by Haulover (29.6%) and Botany (12.7%). To verify that snails were not the driving force in elevated disease on damaged corals, disease prevalence was calculated solely for damaged and undamaged colonies without snails. Damaged corals had approximately double the proportion of colonies with WPX as undamaged corals (Damaged = 37.1%; Undamaged = 16.5%). The median relative risk for disease occurrence was consistently higher on colonies that were damaged and not occupied by snails compared with those that were undamaged and not occupied by snails. There was an average 2-fold increase detected in the disease risk analysis for the first year after damage occurred, and showed low variability for ~7 months after damage with significant effects detected in May and July (Figure 8).
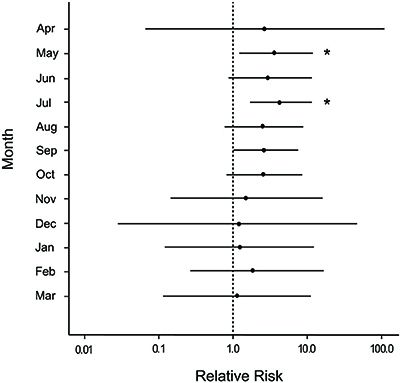
Figure 8. Caterpillar plots depicting the median (black dot) and 95% credible intervals for the relative risk of white pox disease after damage from the March swells for colonies not occupied by the snail, Coralliophila abbreviata, at all sites combined. Asterisks indicate a statistically significant increase in disease risk.
Discussion
Direct physical damage from storms can cause significant reductions in stony corals, and the long-term indirect effects of such damage have the potential to cause further decline. The present study showed that populations of A. palmata damaged directly by storm-generated swells were associated with increased susceptibility to disease, notably WPX, especially during periods with high seawater temperatures. In the year after the March 2008 swells, disease on damaged corals appeared earlier and was approximately twice as prevalent as on undamaged corals. These results were further supported by a doubling of disease risk evident within the first 9 months after damage occurred. Additionally, occupation by the corallivorous snail, C. abbreviata, was observed on 46% more damaged corals than undamaged corals, and overall predation risk increased 1.5-fold (or 50%) within a year after damage. These results suggest that the indirect effects of physical damage can be significant beyond the initial physical breakage.
While, overall, there was a pattern of increased disease on damaged corals for WBD and WPX, the most common ailment, WPX, was the only disease type to affect significantly more damaged corals. Coral tissue loss and injury have been shown to increase susceptibility to waterborne transmission of WBD (Gignoux-Wolfsohn et al., 2012), suggesting that WBD may be responsive to physiological stress. However, it is possible that the prevalence of WBD on surveyed reefs was low enough that differences in prevalence among damaged and undamaged colonies could not be detected.
Occupation by the snail, C. abbreviata, was associated with coral damage, but this association was site dependent. Low coral density sites such as Botany and Haulover had significantly more damaged corals with snails vs. undamaged corals; whereas, Hawksnest, a site with dense thicket-forming colonies (A. Bright, pers. obs.), showed no association between snail occupation and coral damage. This may be explained by generally lower snail predation pressure in high-density coral stands (Miller et al., 2002; Baums et al., 2003) as is the case at Hawksnest (monthly proportion of snail occupied colonies: 3.8 ± 0.9, mean ± SE) relative to Botany (10.2 ± 1.4) and Haulover (15.2 ± 0.8). This is particularly important, as coral predators may be relatively more abundant per coral host than in previous decades when coral populations persisted at higher densities (i.e., the Allee effect; Knowlton, 2001). In the Florida Keys, snails per area of live coral tissue increased significantly from 2004 to 2010 primarily as a result of reduced abundances of coral tissue (Williams and Miller, 2012). Considering the significant decline in coral cover Caribbean-wide (Jackson et al., 2014), these results suggest that, today, corals in low-density stands may be more susceptible to predation following damaging disturbance events.
Snail occupation also seemed to be associated with higher disease prevalence independent of colony damage. Colonies with snails had more disease either at the time of infestation or subsequently compared to colonies without snails. As C. abbreviata is a known vector in acroporid disease transmission (Williams and Miller, 2005; Gignoux-Wolfsohn et al., 2012), it is possible that disease was influenced by this corallivore. However, for colonies not occupied by snails, damaged corals had higher disease prevalence than undamaged corals, again with a doubled disease risk for damaged colonies, suggesting that, while also likely an important disease vector in this system, the presence of C. abbreviata was not the primary driver in high disease levels on swell-damaged colonies.
A few other studies have suggested that physical damage leads to increased disease and predation on acroporid corals. For example, in Jamaica, populations of A. cervicornis were reduced by ~60–85% as a direct result of Hurricane Allen in 1980 (Woodley et al., 1981), but continued to decline by more than 98% (of the original survivors) during the following 5 months, likely as a result of disease and predation (Knowlton et al., 1981). In Curacao, experimental plots of fragmented A. cervicornis had low tissue mortality until disease ravaged the fragments at about 12 weeks post-fragmentation (Bak and Criens, 1981). At 22 weeks post-fragmentation, all fragments in the experimental plot were dead (n = 1, 250), while, in control plots, unfragmented colonies had suffered some tissue loss from disease, but did not die entirely (Bak and Criens, 1981). In the Florida Keys, colonies and fragments of A. palmata experienced disease-like mortality up to several weeks following Hurricane Dennis in 2005 (Williams et al., 2008).
Disease events are common following damaging storms, but it is not known if post-storm disease is the result of an infectious mechanism (e.g., the coral is abraded and exposed to infection) or the physiological state of the coral (e.g., the coral is stressed from physical damage, increased water temperature, irradiance levels, or alteration of nutrient environment). The present study enables a finer resolution of the potential mechanisms involved in post-storm disease trends as it documented the fate of corals that were damaged during a cold water season when most stress factors associated with major storms were not present (e.g., high water temperature, high irradiance levels, increased sedimentation, etc.). In fact, this study supported others where disease manifested on corals affected by physical damage (Bak and Criens, 1981; Knowlton et al., 1981; Williams et al., 2008), but it was deferred until periods of higher sea water temperatures (when lesions caused by the swells had healed) suggesting that the autogenous physiological state of the colony plays an important role in disease initiation. This hypothesis is supported in studies where coral bleaching, a sign of physiological stress to the coral, was positively correlated with elevated levels of disease on Caribbean reefs, sometimes several months after bleaching (Muller et al., 2008; Brandt and McManus, 2009; Cróquer and Weil, 2009; Miller et al., 2009; Rogers and Muller, 2012).
In addition to physical damage, the March swells may have increased nutrients via water mixing and/or mobilization of sediments, both of which are also associated with major storms. Nutrient enrichment experiments suggest a change in ambient nutrient levels can negatively affect the physiology of the coral (particularly during heat and light stress; D'Angelo and Wiedenmann, 2014), potentially resulting in increased susceptibility to disease (Bruno et al., 2003; Vega Thurber et al., 2014). However, Vega Thurber et al. (2014) showed no delayed effects of nutrient enrichment on disease 10 months after enrichment was terminated. Since damaged and undamaged corals responded dissimilarly in the present study, a change in ambient environmental parameters can be ruled out as the primary factor affecting disease trends on monitored corals.
Little is known about the resistance of corals to disease. Some evidence suggest corals have an innate ability to resist disease via their genetic makeup (Vollmer and Kline, 2008), while other corals that are predisposed to disease may be affected when energy resources are depleted and host defenses are compromised. Physical damage to corals may result in physiological changes that promote pathogenic infestation. Corals respond to damage by initiating tissue regeneration, which is very energy intensive for polyps near the site of injury (Meesters et al., 1994) and possibly for the colony as a whole (Henry and Hart, 2005). The basic coral host defenses toward pathogen intrusion are a physical barrier (e.g., mucous layer) and intracellular defenses (e.g., phagocytosis and secretion of antimicrobial properties). Reduced energy levels may result in a lack of available resources (e.g., carbohydrates, proteins, amoebocytes, mucocytes, etc.) for these defenses (reviewed by Mullen et al., 2004) resulting in increased susceptibility to disease (Reed et al., 2010).
In the past, storm damage often promoted fragmentation leading to population expansion. Today, such damaging events may prove more detrimental than beneficial as corals are likely experiencing higher accumulated levels of stress due to factors associated with changing climate such as increased temperature, irradiance, and disease (Williams et al., 2008; Bright et al., 2013). Furthermore, most Caribbean reefs today are structurally degraded (Alvarez-Filip et al., 2009), providing less suitable habitat for fragment retention and attachment following physical disturbance. As changing climate is predicted to bring increases in storm frequency and intensity, thermal stress (e.g., McWilliams et al., 2005; IPCC, 2013), and disease (Harvell et al., 2002), it is critical that further research on the relationships among these stressors is conducted to provide a better understanding of how corals may recover from future disturbances.
Author Contributions
AB, TS, CR conceived and designed the research. AB collected the data. AB, TS, MB, EM analyzed the data. AB, TS, CR, MB, EM wrote and edited the manuscript.
Funding
This project was funded by VI EPSCoR (NSF#0814417) and the Lana Vento Charitable Trust. This is contribution #96 from the Center for Marine and Environmental Studies, UVI.
Conflict of Interest Statement
The authors declare that the research was conducted in the absence of any commercial or financial relationships that could be construed as a potential conflict of interest.
Acknowledgments
Thanks to Dr. Margaret Miller for project support and helpful critique of the manuscript. Dr. Diego Lirman (UM) provided constant support and guidance throughout this project. Kevin Brown provided processed Acoustic Wave and Current Profiler data. Gabriel Rivera, Anthony Spitzack, Ariane Frappier, Stephen Hale, and Christine Settar assisted in fieldwork. Thanks to The Preserve at Botany and the National Park Service (NPS) on St. John for access to sites. The National Oceanographic and Atmospheric Administration-Coral Reef Conservation Program provided financial assistance for this project with support from the US Geological Survey on St. John and the University of the Virgin Islands (UVI). The National Institutes of Health provided funding for undergraduate field research assistance to UVI (NIH MBRS-RISE 2 R25 GM061325).
Supplementary Material
The Supplementary Material for this article can be found online at: http://journal.frontiersin.org/article/10.3389/fmars.2016.00077
References
Alvarez-Filip, L., Dulvy, N. K., Gill, J., Cote, I. M., and Watkinson, A. R. (2009). Flattening of Caribbean coral reefs: region-wide declines in architectural complexity. Proc. R. Soc. London B. 276, 3019–3025. doi: 10.1098/rspb.2009.0339
Anderson, M., Lund, H., Gladfelter, E., and Davis, M. (1986). Ecological Community Type Maps and Biological Community Descriptions for Buck Island Reef National Monument and Proposed Marine Park Sites in the British Virgin Islands. Biosphere Reserve Research Report No. 4, Virgin Islands National Park, St. John, USVI.
Aronson, R. B., and Precht, W. F. (2001). White-band disease and the changing face of Caribbean coral reefs. Hydrobiologia 460, 25–38. doi: 10.1023/A:1013103928980
Bak, R. (1983). Neoplasia, regeneration and growth in the reef-building coral Acropora palmata. Mar. Biol. 77, 221–221. doi: 10.1007/BF00395810
Bak, R., and Criens, S. (1981). “Survival after fragmentation of colonies of Madracis mirabilis, Acropora palmata and A. cervicornis (scleractinia) and the subsequent impact of a coral disease,” in Proceedings of the 4th International Coral Reef Symposium, Vol. 2 (Manila), 221–228.
Baums, I. B., Miller, M. W., and Szmant, A. M. (2003). Ecology of a corallivorous gastropod, Coralliophila abbreviata, on two scleractinian hosts. I. Population structure of snails and corals. Mar. Biol. 142, 1083–1091. doi: 10.1007/s00227-003-1024-9
Brandt, M. E., and McManus, J. W. (2009). Disease incidence is related to bleaching extent in reef-building corals. Ecology 90, 2859–2867. doi: 10.1890/08-0445.1
Brandt, M. E., Smith, T. B., Correa, A. M. S., and Vega-Thurber, R. (2013). Disturbance driven colony fragmentation as a driver of a coral disease outbreak. PLoS ONE 8:e57164. doi: 10.1371/journal.pone.0057164
Brawley, S. H., and Adey, W. H. (1982). Coralliophila abbreviata: a significant corallivore! Bull. Mar. Sci. 32, 595–599.
Bright, A. J., Williams, D. E., Kramer, K. L., and Miller, M. W. (2013). Recovery of Acropora palmata in Curacao: a comparison with the Florida Keys. Bull. Mar. Sci. 89, 747–757. doi: 10.5343/bms.2012.1029
Bruno, J. F., Petes, L. E., Harvell, C. D., and Hettinger, A. (2003). Nutrient enrichment can increase the severity of coral diseases. Ecol. Lett. 6, 1056–1061. doi: 10.1046/j.1461-0248.2003.00544.x
Chong-Seng, K. M., Cole, A. J., Pratchett, M. S., and Willis, B. L. (2011). Selective feeding by coral reef fishes on coral lesions associated with brown band and black band disease. Coral Reefs 30, 473–481. doi: 10.1007/s00338-010-0707-1
Cróquer, A., and Weil, E. (2009). Changes in Caribbean coral disease prevalence after the 2005 bleaching event. Dis. Aquat. Org. 87, 33–43. doi: 10.3354/dao02164
D'Angelo, C., Smith, E. G., Oswald, F., Burt, J., Tchernov, D., and Wiedenmann, J. (2012). Locally accelerated growth is part of the innate immune response and repair mechanisms in reef-building corals as detected by green fluorescent protein (GFP)-like pigments. Coral Reefs 31, 1045–1056. doi: 10.1007/s00338-012-0926-8
D'Angelo, C., and Wiedenmann, J. (2014). Impacts of nutrient enrichment on coral reefs: new perspectives and implications for coastal management and reef survival. Curr. Opin. Environ. Sustainability 7, 82–93. doi: 10.1016/j.cosust.2013.11.029
Gelman, A. J., Carlin, B., Stern, H. S., and Rubin, D. B. (2004). Bayesian Data Analysis, 2nd Edn. Boca Raton, FL: CRC Texts/Champan and hall.
Gignoux-Wolfsohn, S. A., Marks, C. J., and Vollmer, S. V. (2012). White band disease transmission in the threatened coral, Acropora cervicornis. Sci. Rep. 2:804. doi: 10.1038/srep00804
Gill, R. M. A., Webber, J., and Peace, A. (2000). The Economic Implications of Deer Damage. Contract Report, The Deer Commission for Scotland.
Gladfelter, E. H., Monahan, R. K., and Gladfelter, W. B. (1978). Growth rates of five reef-building corals in the northeastern Caribbean. Bull. Mar. Sci. 28, 728–734.
Gladfelter, W. B. (1982). White band disease in Acropora palmata: implications for the structure and growth of shallow reefs. Bull. Mar. Sci. 32, 689–643.
Harvell, C. D., Mitchell, C. E., Ward, J. R., Altizer, A., Dobson, A. P., Ostfeld, R. S., et al. (2002). Climate warming and disease risks for terrestrial and marine biota. Science 296, 2158–2162. doi: 10.1126/science.1063699
Henry, L., and Hart, M. (2005). Regeneration from injury and resource allocation in sponges and corals – a review. Int. Rev. Hydrobiol. 90, 125–158. doi: 10.1002/iroh.200410759
Highsmith, R. C. (1982). Reproduction by fragmentation in corals. Mar. Ecol. Prog. Ser. 7, 207–226. doi: 10.3354/meps007207
Hogarth, W. T. (2006). Endangered and threatened species: final listing determinations for elkhorn and staghorn coral. Fed. Regist. 71, 26852–26861. Available online at: http://www.nmfs.noaa.gov/pr/pdfs/fr/fr71-26852.pdf
Hughes, T. P. (1994). Catastrophes, phase shifts, and large-scale degradation of a Caribbean coral reef. Science 265, 1547–1551. doi: 10.1126/science.265.5178.1547
Hughes, T. P., and Connell, J. H. (1999). Multiple stressors on coral reefs: a long-term perspective. Limnol. Oceanogr. 44, 932–940. doi: 10.4319/lo.1999.44.3_part_2.0932
IPCC (2013). “Climate Change 2013: the physical science basis,” in Contribution of Working Group I to the Fifth Assessment Report of the Intergovernmental Panel on Climate Change, eds T. F. Stocker, D. Qin, G. K. Plattner, M. Tignor, S. K. Allen, J. Boschung, A. Nauels, Y. Xia, V. Bex and P. M. Midgley (New York, NY; Cambridge: Cambridge University Press), 1535.
Jackson, J. B. C., Donovan, M., Cramer, K., and Lam, V. (eds.). (2014). Status and Trends of Caribbean Coral Reefs 1970–2012. Washington, DC: Global Coral Reef Monitoring Network, International Union for the Conservation of Nature Global Marine and Polar Program. Available online at: http://cmsdata.iucn.org/downloads/executive_summary_caribbean_status_report_eng.pdf
Knowlton, N. (2001). The future of coral reefs. Proc. Nat. Acad. Sci. U.S.A. 98, 5419–5425. doi: 10.1073/pnas.091092998
Knowlton, N., Lang, J. C., and Keller, B. D. (1990). Case study of natural population collapse: post-hurricane predation on Jamaican staghorn corals. Smithson. Contr. Mar. Sci. 31, 1–25. doi: 10.5479/si.01960768.31.1
Knowlton, N., Lang, J. C., Rooney, M. C., and Clifford, P. (1981). Evidence for delayed mortality in hurricane-damaged Jamaican staghorn corals. Nature 294, 251–252. doi: 10.1038/294251a0
Kruschke, J. K. (2011). Bayesian assessment of null values via parameter estimation and model comparison. Perspect. Psychol. Sci. 6, 299–312. doi: 10.1177/1745691611406925
Lautenschlager, R. A., and Nielsen, C. (1999). Ontario's forest science efforts following the 1998 ice storm. For. Chron. 75, 633–641. doi: 10.5558/tfc75633-4
Lawson, A. B. (2013). Bayesian Disease Mapping: Hierarchical Modeling in Spatial Epidemiology, 2nd Edn. Boca Raton, FL: Chapman and Hall/CRC.
Lirman, D. (2000). Lesion regeneration in the branching coral Acropora palmata: effects of colonization, colony size, lesion size, and lesion shape. Mar. Ecol. Prog. Ser. 197, 209–215. doi: 10.3354/meps197209
Mayor, P. A., Rogers, C. S., and Hillis-Star, Z. M. (2006). Distribution and abundance of elkhorn coral, Acropora palmata, and prevalence of white-band disease at Buck Island Reef National Monument, St. Croix, US Virgin Islands. Coral Reefs 25, 239–242. doi: 10.1007/s00338-006-0093-x
McWilliams, J. P., Cote, I. M., Gill, J. A., Sutherland, W. J., and Watkinson, A. R. (2005). Accelerating impacts of temperature-induced coral bleaching in the Caribbean. Ecology 86, 2055–2060. doi: 10.1890/04-1657
Meesters, E. H., Noordeloos, M., and Bak, R. P. M. (1994). Damage and regeneration: links to growth in the reef-building coral Montastrea annularis. Mar. Ecol. Prog. Ser. 112, 119–128. doi: 10.3354/meps112119
Miller, J., Muller, E., Rogers, C., Waara, R., Atkinson, A., Whelan, K., et al. (2009). Coral disease following massive bleaching in 2005 causes 60% decline in coral cover on reefs in the US Virgin Islands. Coral Reefs 28, 925–937. doi: 10.1007/s00338-009-0531-7
Miller, M. S., Baums, I. B., Williams, D. E., and Szmant, A. M. (2002). Status of Candidate Coral, Acropora palmata, and Its Snail Predator in the Upper Florida Keys National Marine Sanctuary: 1998-2001. Miami, FL: NOAA Technical Memorandum NMFS-SEFSC-479.
Morton, B., Blackmore, G., and Kwok, C. T. (2002). Corallivory and prey choice by Drupella rugosa (Gastropoda: Muricidae) in Hong Kong. J. Molluscan Stud. 68, 217–223. doi: 10.1093/mollus/68.3.217
Mullen, K., Peters, E. C., and Harvell, C. D. (2004). “Coral resistance to disease,” in Coral Health and Disease, eds E. Rosenberg and Y. Loya (New York, NY: Springer-Verlag), 377–399.
Muller, E. M., Rogers, C. S., Spitzack, A. S., and van Woesik, R. (2008). Bleaching increases likelihood of disease on Acropora palmata (Lamarck) in Hawksnest, St. John, US Virgin Islands. Coral Reefs 27, 191–195. doi: 10.1007/s00338-007-0310-2
Randall, C. J., Jordan-Garza, A. G., Muller, E. M., and van Woesik, R. (2014). Relationship between the history of thermal stress and the relative risk of diseases of Caribbean corals. Ecology 95, 1981–1994. doi: 10.1890/13-0774.1
Raymundo, L., Couch, C., and Harvell, C. (2008). Coral Disease Handbook: Guidelines for Assessment, Monitoring and Management. Melbourne, VIC: Currie Communications.
Reed, K. C., Muller, E. M., and van Woesik, R. (2010). Coral immunology and resistance to disease. Dis. Aquat. Org. 90, 85–92. doi: 10.3354/dao02213
Rinkevich, B., and Loya, Y. (1979). The reproduction of the Red Sea coral Stylophora pistillata. I. Gonads and planulae. Mar. Ecol. Prog. Ser. 1, 133–144. doi: 10.3354/meps001133
Rogers, C. S. (2010). Words matter: recommendations for clarifying coral disease nomenclature and terminology. Dis. Aquat. Org. 91, 167–175. doi: 10.3354/dao02261
Rogers, C. S., and Muller, E. M. (2012). Bleaching, disease and recovery in the threatened scleractinian coral Acropora palmata in St. John, US Virgin Islands: 2003-2010. Coral Reefs 31, 807–819. doi: 10.1007/s00338-012-0898-8
Rogers, C. S., Suchanek, T. H., and Pecora, F. A. (1982). Effects of hurricanes David and Frederic (1979) on shallow Acropora palmata reef communities: St. Croix, US Virgin Islands. Bull. Mar. Sci. 32, 532–548.
Sussman, M., Loya, Y., Fine, M., and Rosenberg, E. (2003). The marine fireworm Hermodice carunculata is a winter reservoir and spring-summer vector for the coral-bleaching pathogen Vibrio shiloi. Environ. Microbiol. 5, 250–255. doi: 10.1046/j.1462-2920.2003.00424.x
Szmant, A. M. (1986). Reproductive ecology of Caribbean reef corals. Coral Reefs 5, 43–54. doi: 10.1007/BF00302170
Van Woesik, R. (1998). Lesion healing on massive Porites spp. corals. Mar. Ecol. Prog. Ser. 164, 213–220. doi: 10.3354/meps164213
Vega Thurber, R. L., Burkepile, D. E., Fuchs, C., Shantz, A. A., McMinds, R., and Zaneveld, J. R. (2014). Chronic nutrient enrichment increases prevalence and severity of coral disease and bleaching. Glob. Change Biol. 20, 544–554. doi: 10.1111/gcb.12450
Vollmer, S. V., and Kline, D. I. (2008). Natural disease resistance in threatened staghorn corals. PLoS ONE 3:e3718. doi: 10.1371/journal.pone.0003718
Warillow, M., and Mou, P. (1999). Ice storm damage to forest tree species in the ridge and valley region of southwestern Virginia. J. Torrey. Bot. Club. 126, 147–158. doi: 10.2307/2997291
Williams, D. E., and Miller, M. W. (2005). Coral disease outbreak: pattern, prevalence and transmission in Acropora cervicornis. Mar. Ecol. Prog. Ser. 301, 119–128. doi: 10.3354/meps301119
Williams, D. E., and Miller, M. W. (2012). Attributing mortality among drivers of population decline in Acropora palmata in the Florida Keys (USA). Coral Reefs 31, 369–382. doi: 10.1007/s00338-011-0847-y
Williams, D. E., Miller, M. W., and Kramer, K. L. (2008). Recruitment failure in Florida Keys Acropora palmata, a threatened Caribbean coral. Coral Reefs 27, 697–705. doi: 10.1007/s00338-008-0386-3
Woodley, J., Chornesky, E., Clifford, P., Jackson, J., Kaufman, L., Knowlton, N., et al. (1981). Hurricane Allen's impact on Jamaican coral reefs. Science 214, 749–755. doi: 10.1126/science.214.4522.749
Keywords: coral disease, white pox disease, Coralliophila, physical damage, oceanic swell, fragmentation, relative risk
Citation: Bright AJ, Rogers CS, Brandt ME, Muller E and Smith TB (2016) Disease Prevalence and Snail Predation Associated with Swell-Generated Damage on the Threatened Coral, Acropora palmata (Lamarck). Front. Mar. Sci. 3:77. doi: 10.3389/fmars.2016.00077
Received: 10 December 2015; Accepted: 03 May 2016;
Published: 24 May 2016.
Edited by:
Yehuda Benayahu, Tel Aviv University, IsraelReviewed by:
Jörg Wiedenmann, University of Southampton, UKMelissa Garren, Pelagic Data Systems & California State University Monterey Bay, USA
Copyright © 2016 Bright, Rogers, Brandt, Muller and Smith. This is an open-access article distributed under the terms of the Creative Commons Attribution License (CC BY). The use, distribution or reproduction in other forums is permitted, provided the original author(s) or licensor are credited and that the original publication in this journal is cited, in accordance with accepted academic practice. No use, distribution or reproduction is permitted which does not comply with these terms.
*Correspondence: Allan J. Bright, YWxsYW4uYnJpZ2h0QG5vYWEuZ292