- 1Interuniversity Institute for Marine Sciences, Eilat, Israel
- 2The Fredy & Nadine Herrmann Institute of Earth Sciences, The Hebrew University of Jerusalem, Jerusalem, Israel
Ocean warming has exacerbated the severity of coral diseases, many of which are mediated by pathogenic bacteria. In response to the presence of pathogens various organisms activate an oxidative burst response, involving strong and rapid generation of reactive oxygen species (ROS) that serve both as bactericides and as signals for other defense systems. While many components of the coral immune systems are being unveiled, an oxidative burst response, triggered by bacteria proximity, has not yet been reported. Hydrogen Peroxide (H2O2), one of the ROS produced during the oxidative burst, is often monitored as an indicator of this pathway. Here we report a yet undescribed release of H2O2 from the coral Stylophora pistillata upon contact with bacteria. In a series of short term experiments we monitored H2O2 concentrations in the vicinity of S. pistillata prior to and following local administration of five bacteria isolates belonging to the genus Vibrio (obtained from healthy S. pistillata). In most experiments, rapid H2O2 release was recorded at the site of interaction within one min from Vibrio addition and persisted for the remaining 5 min of the experiment. In some experiments, discrete or continuous drop of H2O2 to below background levels were seen following Vibrio addition. This H2O2 loss was quantitatively accounted for by the bacterial antioxidants and implies that Vibrio may at times offset the coral released H2O2.The total H2O2 released by the coral from the small interaction site (~10 mm2) was 200–600 pmol, which may build up to a concentration of ~20 μM in the coral diffusive boundary layer. H2O2 concentrations above 10 μM resulted in significant mortality of the coral pathogen Vibrio coralliilyticus, suggesting that the released H2O2 may act as a bactericide. It is thus far unclear if this H2O2 release is part of an oxidative burst response, but it's occurrence strongly indicates that corals sense and chemically react toward bacteria.
Introduction
Coral reefs are highly diverse ecosystems, containing thousands of invertebrates and fish species that live in complex symbiotic relationships (Bellwood and Hughes, 2001). On top of these interactions, reef organisms also harbor complex associations with microorganisms that live in their tissue, on their surfaces, in the water surrounding them and in the sediments (DiSalvo and Gundersen, 1971; Moriarty, 1979; Rohwer et al., 2002).
During the last few decades, especially following major worldwide coral disease outbreaks, intense research has been conducted to explore the different factors controlling the onset of coral diseases. So far, half of the 18 recognized coral syndromes have been strongly linked to fungal and bacterial infection with six of them associated with bacteria from the genus Vibrio (Bourne et al., 2009; Arotsker and Kushmaro, 2015; Munn, 2015). Infectious coral diseases, caused by specific pathogen or microbial assemblages, can be transmitted from infected to susceptible corals by different mechanism such as, vector mediation (e.g., butterflyfish; Aeby and Santavy, 2006) and water-borne infection (Zvuloni et al., 2009). Due to global warming, infectious diseases are expected to become more prevalent with higher transmission rates (Zvuloni et al., 2015). This may result in a severe threat to coral reefs worldwide.
The search for causative agents of coral diseases has also revealed the huge diversity of coral associated microorganisms (Rohwer et al., 2002) and shed light on various coral-bacteria interactions (Ritchie, 2006). In addition to acting as pathogens, many coral associated bacteria were found to be beneficial to the coral (Ritchie, 2011), producing different organic and antibacterial compounds (Shnit-Orland and Kushmaro, 2009) and assisting in nutrient recycling (Raina et al., 2009; Rädecker et al., 2015). Bacterial populations associated with corals are now thought to play important roles in the stability of the coral animal as a whole (termed holobiont; Reshef et al., 2006).
The community composition of the coral associated bacteria often changes along with changes in environmental conditions (Webster et al., 2016), physiological state of the coral (Ng et al., 2015), and exposure to anthropogenic impacts (Ziegler et al., 2016). These changes might generate open spaces for virulent bacteria to proliferate and establish pathology, taking advantage of the unstable state of the coral holobiont (Mydlarz et al., 2010). Severe pathology will appear if bacteria are capable of carrying out a few critical stages including: first interaction, adhesion, penetration, proliferation in the coral tissue, and damage to physiological functions (Rosenberg and Ben-Haim, 2002).
To prevent the pathology caused by bacterial infection, corals make use of different mechanisms; many of which rely on the coral associated bacteria (Shnit-Orland and Kushmaro, 2009). The coral external mucus layer is densely populated with dynamic and rich microbial communities. These microbial populations compete for resources and space and produce antibiotics to fight each other. This competitive behavior eventually reduces the chances of new, potentially pathogenic, species to establish in the coral tissue (Rypien et al., 2010). The corals themselves combat invading bacteria with immune defenses (Mydlarz et al., 2009), cilia-mediated water flow that prevent bacteria adhesion (Shapiro et al., 2014), or by secreting chemicals that inhibit microbial growth, attachment, and behavior (Ritchie, 2006). Such chemicals could include compounds that regulate bacteria communication or have antimicrobial activity (Geffen et al., 2009).
Reactive oxygen species (ROS) have been assigned some roles in antibacterial defense in corals. Banin et al. (2003) showed that the bacterium Vibrio shiloi, which causes bleaching in the stony coral Oculina patagonica, cannot establish itself in the coral during winter. They suggested that the coral protects itself from invading bacteria with the aid of high levels of ROS in the coral tissue. In winter when the bacterial superoxide dismutase activity is low, the pathogen is highly susceptible to ROS. Mydlarz and Jacobs (2006) found that injury by sonic sound cavitations and heat stresses were capable of inducing the release of ROS in four gorgonian species. They suggested that ROS release may be the first line of defense in gorgonian corals as well as acting as a signal to induce additional immune and wound healing responses.
Indeed, rapid release of ROS, known as “oxidative burst” (transient ROS generation within time periods from several minutes up to hours), is activated in plants and mammalian immune cells under stress conditions and as a defense against microorganisms (Lamb and Dixon, 1997). The steep increase in internal and often external ROS is mediated by enzymatic ROS production as well as the inhibition of the ROS depletion systems (Schmitt et al., 2014). The elevated ROS, including superoxide, hydrogen peroxide, hydroxyl radicals and nitrous oxide, are harmful to the invading bacteria but also act as signals for activating stress-response and defense pathways (Schmitt et al., 2014). In plants the oxidative burst is part of the hypersensitive response, where rapid death of cells occurs in the local region surrounding a microbial infection to prevent it from spreading. The H2O2 burst play multiple roles in the hypersensitive response, damaging the plant cell membrane and acting as a diffusible signal that activates the programmed cell death pathway (Levine et al., 1994).
H2O2 is moderately reactive and is a relatively long-lived molecule with a half-life of 1 ms within cells and ~1 d in coastal water (Shaked et al., 2010; Sharma et al., 2012). As the only ROS that can diffuse through aquaporins in the membranes and over larger distances within the cell, H2O2 received particular attention as a signal molecule which induces molecular, biochemical, and physiological responses (Gough and Cotter, 2011). The cytotoxic activity of H2O2, involving oxidative damage to proteins and lipids is based on the highly reactive hydroxyl radical produced during H2O2 interactions with superoxide (Haber-Weiss's reaction) or Fe(II) (Fenton's reaction; Sharma et al., 2012). Oxidative damage to DNA by H2O2 is thought to occur through ferryl radical formed when H2O2 reacts with DNA-associated iron (Linley et al., 2012). H2O2 may also initiate lethal cellular cascades such as lipid peroxidation and apoptotic cell death (Apel and Hirt, 2004; Repetto et al., 2012), and is often used in interactions between organisms as a grazer and pathogen deterrent (McDowell et al., 2014).
Recently we identified and characterized a rapid release of H2O2 from the stony coral Stylophora pistillata, which occur on time-scales of minutes in response to various triggers (Armoza-Zvuloni et al., 2016). In incubation experiments, with healthy and bleached coral fragments, we documented rapid H2O2 release during feeding on zooplankton prey and in response to physical and chemical stimuli. By applying localized stimuli and a frequent sampling procedure we found that the response is limited to the site of stimulus (rather than whole colony response) and its duration is limited to a few minutes.
Here, in a follow-up study, we applied the same setup as in Armoza-Zvuloni et al. (2016) to test whether corals sense the presence of bacteria and respond to it by locally releasing H2O2. We used Stylophora pistillata coral fragments and cultures of five Vibrio isolates obtained from healthy S. pistillata. We characterized H2O2 release kinetics by measuring H2O2 concentrations next to the coral surface before and after local administration of concentrated bacteria, and detected a significant, persistent and local release of H2O2. The bacteria did not contribute to this H2O2 production; on the contrary, their antioxidants degraded some of the H2O2 released by the coral. By probing for H2O2 toxicity to the coral pathogen Vibrio coralliilyticus, and estimating H2O2 accumulation in its site of release, we obtained comparable concentrations, suggesting it may act as a bactericide.
Materials and Methods
Coral Handling and Preconditioning
Thirty similarly sized S. pistillata coral fragments (with a surface area of ~20 cm2) were collected from the Interuniversity Institute for Marine Sciences (IUI) coral nursery located in the Gulf of Aqaba (29°30′6.84″N, 34°55′4.09″E). Coral sampling and handling was carried out in accordance with the recommendations of the Israeli Nature and Park Authority. The fragments were glued to plastic lids facing upright using underwater epoxy glue (Propoxy 20, Hercules Chemicals), and underwent an acclimation period of 6 months in shaded water tables with running seawater at ambient temperatures of 21–27°C.
Vibrio Culturing
Non-pathogenic Vibrios were isolated from mucus of three healthy S. pistillata coral fragments. The coral fragments were collected from the coral nursery, brought to the lab, washed shortly with filtered seawater and centrifuged for 1 min at maximal speed to remove mucus. The mucus was then diluted and plated on Vibrio-selective TCBS agar (4.5% Difco TCBS agar, 0.9% marine broth, 0.45% NaCl, and 1.2% Difco Bacto agar). After serial dilution and plating, five phenotypically different isolates were screened and isolated. A pure culture was obtained by sub-cultivation under the same conditions. The Vibrio isolates were assigned numbers from 1 to 5 and were maintained as viable cultures on plates at room temperature or stored as 20% (v/v) glycerol suspensions at −80°C. Prior to the H2O2 release experiments, one Vibrio colony was inoculated in conical flasks containing 5 mL marine broth medium (1.8% marine broth, Difco MA 2216, and 0.9% NaCl), and incubated at 30°C under shaking (160 rpm) for 24–36 h. Growth was monitored by measuring OD at 590 nm. Cultures were collected by centrifugation at 3000 g for 5 min and re-suspended in 500 μl FSW. Centrifugation and re-suspension was repeated five more times to ensure the complete removal of culture media. Bacteria density was examined using hemocytometer and diluted to final concentration of 1 × 106 cells mL−1. We lack genetic information on the isolates due to failure of the freezer and sample loss.
H2O2 Measurements
H2O2 concentrations were measured using the POHPAA (4-hydroxyphenylacetic acid) technique detailed in Shaked and Armoza-Zvuloni (2013). The reagent stock, consisting of 0.25 mM POHPAA, 70 units mL−1 of horseradish peroxidase, and 0.25 M Tris at pH 8.8, was added to the samples at a 1:50 dilution. All stocks were kept on ice in the dark. The florescence of the dark-kept samples was read within 30 min of sampling using a Varian spectroflurometer (Cary Eclipse) set at an excitation of 315 ± 10 nm and an emission of 408 ± 10 nm. Calibration curves were run daily using filtered seawater spiked with freshly made H2O2 standards and catalase treated seawater as blanks.
H2O2 Release Experiments
The release of H2O2 from the stony coral S. pistillata in response to bacteria proximity was studied in high temporal resolution in localized experiments. We utilized a container fitted with a narrow Teflon tube (0.03 inch internal diameter). The tube carried water by gravitation from the coral surface to cuvettes for H2O2 analysis. The cuvettes, containing 20 μL of POHPAA reagent stock (see H2O2 measurements), were placed outside the container. The tube position was adjustable and the opening of the tube was set at ~0.2 cm from the coral surface, adjacent to the site of stimulus. No stirring was applied during the experiment and care was taken to minimize turbulence while administering the Vibrio culture. The tubes constantly transferred water at a rate of 2 mL min−1 from the coral surface to cuvettes that were replaced every 0.5 min. The experiments started with 2.5 min of background H2O2 measurements. Then, 100 μl Vibrio culture, containing 1 × 106 cells mL−1, was applied on the coral surface near the tube opening over a small area of 10 mm2. Following the addition of bacteria, H2O2 readings were taken every 0.5 min from 3.5 to 8 min. To rule out the possibility that the water motion rather than the bacteria addition induced the release of H2O2, these experiments were repeated with similar volumes of filtered seawater (FSW). All experiments were conducted under fluorescent laboratory lighting (~10 μmol quanta m−2 s−1) at a constant temperature (25 ± 1°C). Coral fragments were usually used for one experiment every week. Prior to the experiments initiation, corals were allowed to recover from their preparative handling for 30–60 min under running seawater. Experiments commenced once the coral polyps were extended. Coral fragments did not show any signs of pathology, tissue lysis or bleaching during the period of the experiments and afterwards.
Quantification of the Amount of H2O2 Released from the Corals
To convert the H2O2 concentrations measured next to the coral to amounts of H2O2 released by the coral we conducted the following steps. We first calculated the H2O2 released in each discrete time interval, by subtracting the minimal H2O2 concentration detected prior to stimulation from all measurements. These minimal values reflect the basal levels of H2O2 occurring naturally in seawater. We then converted the nM concentrations in to moles of H2O2 according to the collected volumes. In order to get the total H2O2 released before and after stimulation we summed up all the relevant time intervals. To account for the shorter duration of the experiment prior to stimulus we multiplied the amount of H2O2 released prior to stimulus by 2.5 (the time ratio between the two experimental sections). Note that our sampling procedure leads to removal of all the H2O2 released by the coral in each time interval. Therefore, any addition of H2O2 above background levels represents in fact a continuous release of H2O2 as explained below.
Sensitivity of Vibrio coralliilyticus to H2O2
H2O2 toxicity was studied according to the assay of Geffen and Rosenberg (2005) using the bacteria V. coralliilyticus YB2 (LMG 21348), a virulent coral pathogen (Ben-Haim and Rosenberg, 2002). Vibrio coralliilyticus cultures were donated by Prof. Eugene Rosenberg (Tel-Aviv University, Israel). The bacteria were incubated at a final density of 1 × 105 cells mL−1 with increasing concentrations of H2O2 for 30 min at 30°C and with filtered seawater as controls. Then 5 μl aliquots (n = 10) were spotted on TCBS agar at two dilutions (undiluted and 10-fold). Plates were incubated for 24 h at 30°C. The toxicity of H2O2 to V. coralliilyticus is evaluated based on the number of colonies growing in each spot on the plates (termed CFU -colony forming units). The experiments were repeated twice at different range of H2O2 concentrations using independently grown cultures.
Results
Release of H2O2 from Corals in Response to Vibrios
We probed for coral H2O2 release in response to a local administration of ~105 Vibrio cells suspended in 100 μl FSW, by monitoring H2O2 concentrations in the coral vicinity prior to and following the bacteria addition. We conducted 103 experiments using 5 different Vibrio isolates (Vibrios 1-5), with Vibrio 4 tested most frequently and hence shown first.
Average Changes in H2O2 Concentrations on Stylophora pistillata Surface in Response to Interaction with Vibrios
Figure 1 shows the mean H2O2 concentrations measured in all 48 experiments with Vibrio 4 and in 10 control experiments using Vibrio-free filtered seawater. Before the stimulus, the water next to the coral had stable H2O2 concentrations of 27 ± 2.2 nM (mean ± SE, n = 48). A significant increase in H2O2 was recorded at the site of stimulus following Vibrio addition reaching 42 ± 7.3 nM (p = 0.03, paired t-test). This localized H2O2 release occurred rapidly, within 1 min, and persisted for the remaining 5 min of the experiment. To assure that the coral responded specifically to Vibrio and not the jet of water from the pipette, we repeated the experiments with a similar volume (100 μl) of filtered seawater (FSW). Here, mean H2O2 concentrations on the coral surface were similar before (20 ± 2.7 nM, mean ± SE, n = 10; 1–2.5 min) and after (21 ± 3.8 nM; 3.5–8 min) FSW addition (p > 0.05, paired t-test; Figures 1A,B). Testing whether the bacteria themselves are a source of H2O2, we measured the H2O2 levels of the bacteria suspension used in the experiments. In all five Vibrios cell suspensions, H2O2 concentrations were similar or lower than in FSW (not shown). Mean H2O2 concentrations measured in similar experiments with Vibrios 1–3 and 5 are shown in Figure SI-1.
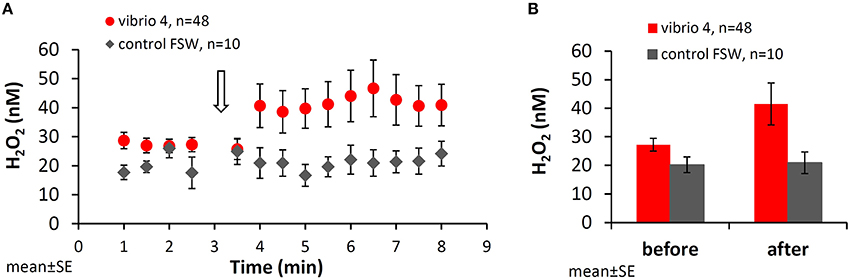
Figure 1. Changes in H2O2 concentrations in the vicinity of Stylophora pistillata following interaction with Vibrio. (A) H2O2 concentrations next to the coral surface prior to and following addition of bacteria (105 cells of Vibrio 4; red dots) or filtered seawater as a control (gray diamonds). Vibrio 4 culture, originally isolated from healthy S. pistillata, was added after 3 min (open arrow) and the coral response was examined for additional 5 min. (B) Mean H2O2 concentrations measured before (between 1.0 and 2.5 min) and after (between 3.5 and 8.0 min) interaction with Vibrio 4. All the data is averaged over the entire set of 48 experiments.
It is important to note that while sampling the water next to the coral with the collection tube, all the H2O2 released by the coral was also removed from the experiment. This is based on our recent study showing no diffusion of H2O2 from the site of stimulus to other areas of the beaker (Armoza-Zvuloni et al., 2016). Hence, the elevated concentrations measured in response to bacteria represent, in fact, a continuous and localized release of H2O2.
Variations in the Patterns of H2O2 Release in Response to Vibrio 4
Figure 1 shows the average behavior of all corals but hides information on variations in the H2O2 release patterns and amounts between individual experiments. While in many experiments rapid H2O2 accumulation was observed, in other experiments H2O2 concentrations did not change and even dropped below background levels (see Figure SI-2 for representative individual experiments).
To better understand patterns of H2O2 release we calculated the changes in H2O2 in individual experiments following stimulus in two ways and presented them on a scatter plot (Figure 2). The first calculation examines the immediate response to bacterial addition using the ratio of two single H2O2 measurements taken immediately after and before the stimulus (= Immediate after/before). The second calculation examines the longer term response to bacteria using the ratio of averaged H2O2 concentrations measured after and before the stimulus (= Averaged after/before). The resulting ratios conveniently demonstrate the net change in H2O2 and its degree following bacteria addition and allow us to split the experiments into four categories, as shown in Figure 2.
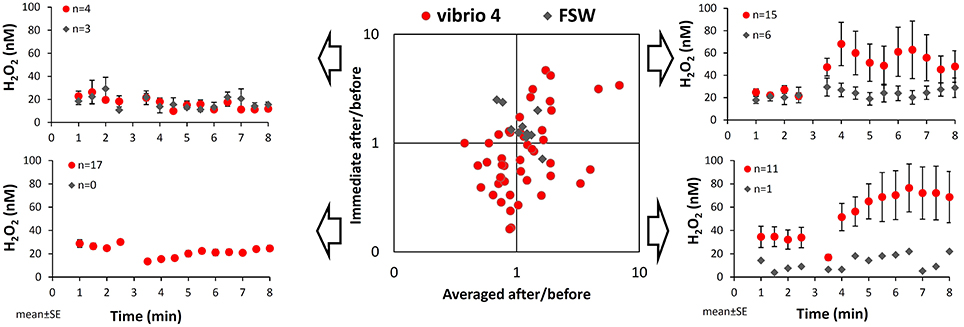
Figure 2. Variations among individual experiments in the pattern of H2O2 release from Stylophora pistillata following interaction with Vibrio. Taking a closer look at the changes in H2O2 concentrations over time in individual experiments we noted four distinct patterns, which are illustrated on the side panels. The scatter plot conveniently distinguishes between these patterns according to the ratio of H2O2 concentrations after and before the interaction with bacteria. These ratios are calculated separately for mean changes in H2O2 concentrations (x-axis) or for immediate changes in H2O2 concentrations (y-axis; i.e., at 3.5 min over 2.5 min). Here, values below 1 represent a drop in H2O2 concentrations following interaction, while values above 1 represent an increase in H2O2 concentrations. The red dots are all 48 experiments conducted with Vibrio 4 and the gray diamonds are the 10 seawater controls.
In our data set, 15 out of 48 experiments with Vibrio 4 plot on the upper right corner, indicating both an immediate and a longer term H2O2 accumulation (i.e., ratios > 1). The average increase in H2O2 concentration in response to bacteria of these 15 experiments (shown on the upper right corner of Figure 2), is naturally higher than the average change observed in all the experiments (Figure 1). Eleven of the remaining experiments plot on the lower right corner, indicating an immediate decrease in H2O2 but a longer term H2O2 increase (as shown also by their average changes in time). Together the 26 experiments with “averaged after/before” larger than 1 (the right hand side of the plot), represent overall H2O2 accumulation, despite an immediate drop in H2O2 in almost half of them. Interestingly, 17 of the 48 experiments plot on the lower left corner, showing both an immediate and a longer term H2O2 loss. The controls with Vibrio-free FSW addition had ratios close to 1 on both axes with 6 out of the 10 experiments showing mild positive H2O2 release (Figure 2; upper right corner).
H2O2 Degradation by Vibrios
The common pattern in 28 of 48 experiments of an immediate or a longer term drop in H2O2 following bacteria addition (i.e., the lower side of the plot with an Immediate after/before <1; Figure 2), may stem from bacterially mediated H2O2 degradation. We therefore measured the rates of H2O2 degradation in water sub-samples removed from the corals through the collection tube in a few of the experiments. We followed the procedure described in detail in Armoza-Zvuloni and Shaked (2014), of adding 1 μM H2O2 to the sample of interest and monitoring its loss over time (see Figure SI-3 for details on the methods). We found high H2O2 degradation rates in water collected from the coral surface immediately after bacteria addition, which dropped back to background within few minutes (Figure SI-3).
To further verify that the bacteria themselves and not the corals are responsible for H2O2 degradation we tested the rates of H2O2 degradation by diluted Vibrio 4 cell suspension. H2O2 degradation rates in the diluted cell suspension were high, ~6-fold higher than those measured in water collected from corals following the bacteria addition (Figure SI-3).
Quantifying the Amount of H2O2 Released from Corals in Response to Vibrios
The H2O2 concentrations presented so far do not accurately reflect the actual H2O2 release from corals in response to bacteria. This is due to our experimental setup where H2O2-enriched water from the coral surface is continuously removed through the collection tube. Hence, when estimating H2O2 release in each time interval, as presented below, we should take into account the fact that the coral repeatedly replenishes this H2O2. We calculated the number of H2O2 moles released in each time interval by multiplying the water volume collected in each fraction by the concentrations of H2O2 above that of ambient seawater (see Methods for calculation details). Here we present the experiments with all Vibrios but include only experiments with positive H2O2 release (i.e., Averaged after/before >1) to eliminate the H2O2 degradation effect and probe the maximal strength of this phenomenon. This analysis, presented in Figure 3 for the five Vibrio isolates and a control (A–F), reveals an active H2O2 release by the corals from the time of interaction and throughout the experiment.
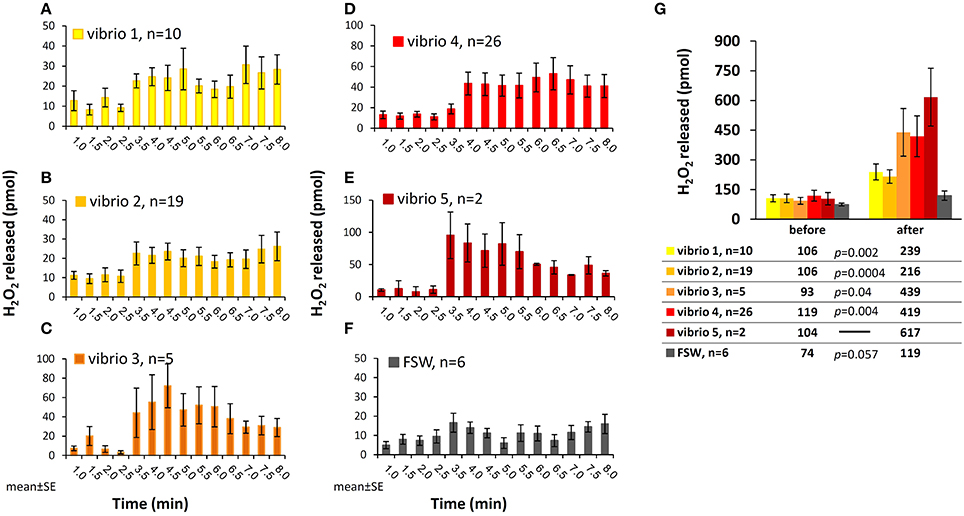
Figure 3. Moles of H2O2 released from S. pistillata following interaction with the five Vibrio isolates. (A–F) Calculated mols of H2O2 released by the corals in discrete time intervals during interactions with the five Vibrio isolates (A–E) and seawater control (F). The data set includes only experiments with positive H2O2 accumulation after bacteria addition (i.e., Averaged after/before >1, see Figure 2). (G) Total moles of H2O2 released prior to and following interaction with bacteria, averaged for each of the Vibrio isolates tested. This calculation takes into account the different experimental durations before and after stimulation. The results of the paired t-test are shown below the figure.
Summing up all these fractions and separately averaging the experiments with each of the Vibrios, we obtain an overall release of 200–600 pmol H2O2, with the highest release in the presence of Vibrio 5 and the lowest in the presence of Vibrio 2 (Figure 3G). When looking at individual experiments, the range of values increase and the overall H2O2 release vary between 100 and 1000 pmol after stimulus (data not shown). The FSW control experiments had lower and more moderate range of H2O2 release (Figure 3).
Sensitivity of the Pathogenic Vibrio Coralliilyticus to H2O2
The released H2O2 may play multiple roles in the coral recognition and defense against bacteria. Testing for its direct role as a bactericide, we conducted toxicity assays with a strain of pathogenic Vibrio–Vibrio coralliilyticus. We chose this strain over our Vibrio isolates due to its environmental significance and frequent use in toxicity assays (Munn et al., 2008; Geffen et al., 2009). Incubating V. coralliilyticus in FSW with different H2O2 concentrations we obtained the expected trend of decreasing bacterial survival with increasing H2O2 (Figure 4). In the first experiment, using a large range of H2O2 concentrations, we crudely established that 10 μM of H2O2 is highly toxic to V. coralliilyticus (Figure 4A). Then, using a finer concentration range we examined the toxicity of H2O2 at micromolar levels. The bacteria colonies decreased to 75, 30, and 10% of the controls at H2O2 concentrations of 10, 20, and 30 μM, respectively and at 60 μM H2O2 only 1% of the bacteria survived (Figure 4B).
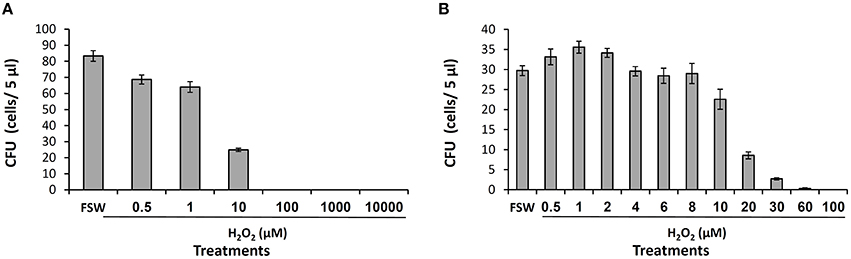
Figure 4. H2O2 toxicity to Vibrio coralliilyticus. The sensitivity of V. coralliilyticus to H2O2 was examined in two independent experiments (A,B) using different H2O2 concentration range. V. coralliilyticus diluted cultures were exposed to H2O2 for 30 min and spotted on TCBS agar. CFU refers to the number of colonies appearing on each spot after incubation for 24 h at 30°C.
Discussion
Corals Actively Release H2O2 during Interaction with Vibrio Cells
Our study reports a previously unstudied phenomenon of H2O2 release from the stony coral S. pistillata in response to interactions with different Vibrio isolates (previously obtained from healthy S. pistillata). The release occurred locally and rapidly, within 1 min from bacteria addition and persisted for the remaining 5 min of the experiment (Figures 1–3). Given our experimental system, which removes the coral released H2O2 through a collection tube, the elevated and relatively constant H2O2 concentrations observed following bacteria addition indicate that, in fact, an active and continuous release of H2O2 by the coral is required to replenish and maintain these concentrations. The rapid and persistent response suggests that the corals recognize the presence of the bacteria and actively release H2O2 at the site of interaction. As corals released H2O2 following stimulation with all five Vibrio isolates tested (Figure 3), we postulate that the coral response is general to interactions with Vibrios.
We can compare our current findings with H2O2 release patterns we recently reported for other stimuli using the same coral species and experimental system (Armoza-Zvuloni et al., 2016). When subjected to physical or chemical stimuli the corals rapidly released H2O2 within 1–2 min from the time of stimulation at comparable concentrations. However, the H2O2 release period was shorter in response to physical and chemical stimuli and H2O2 concentrations dropped to background levels within 2–3 min from the stimuli. Considering the ability of pathogenic Vibrios to adhere to the coral's surface layer before penetrating the coral tissue (Toren et al., 1998), it is possible that after stimulation, some bacteria remained attached to the coral surface and hence were recognized by the coral, leading to continues H2O2 release.
In coral focused literature, there are no previous reports of rapid H2O2 production or release in response to contact with bacteria. However, short term elevated production and release of H2O2 (and other ROS), referred to as an oxidative burst response, is well-documented in plants, marine micro- and macro algae and few marine invertebrates, when challenged with pathogens or various chemical elicitors (e.g., Levine et al., 1994; Coteur et al., 2002; Weinberger, 2007). The oxidative burst often involves the enzyme NADPH oxidase that generates superoxide in the cell or on its membrane (Schmitt et al., 2014). Since this superoxide is rapidly converted to the more stable H2O2, activity of a cell surface NADPH oxidase is a likely precursor for an external H2O2 flux. Our group has reported on continuous superoxide release from S. pistillata to its external milieu, which is inhibited by the NADPH oxidase inhibitor DPI (Diphenyleneiodonium chloride; Saragosti et al., 2010). Such a pathway, if activated upon contact with bacteria, may enable corals to generate an oxidative burst response. Indeed, an up-regulation of transcripts linked to ROS production, including three subunits of the enzymatic complex NADPH oxidase, were reported in the coral Acropora cervicornis infected with the pathogen related White Band Disease (Libro et al., 2013). Further research is required to identify the enzymes and ROS that play the major role in this pathway, promoting oxidative burst response in corals during interactions with bacteria.
Influence of Bacterial Antioxidants on Observed H2O2 Release
In many of the experiments, a sharp decline in H2O2 concentrations occurred immediately following the addition of bacteria and, in many cases, a net drop in H2O2 was observed throughout the whole experiment (Figure 2). Measuring the rates of H2O2 degradation in the experiment water and in Vibrio cell suspension (Figure SI-3), we assigned this effect to the bacteria themselves, holding a variety of antioxidant enzymes and molecules. This effect is lost in many of the experiments, where H2O2 does accumulate, since most of the bacteria are washed off of the coral surface and sucked into our collection tube. Similarly, Munn et al. (2008) found high catalase and superoxide dismutase activity in pathogenic and non-pathogenic Vibrio cultures. Considering the antioxidant activity of Vibrios and the drop in H2O2 levels following interaction, it is reasonable to assume that the actual H2O2 release by the coral is higher than measured here. On the other hand, the significant capability of bacteria to degrade H2O2 may imply that Vibrios in nature can offset the coral released H2O2. The actual ability of natural bacteria to counteract the coral released H2O2 will depend on their densities, antioxidant levels, H2O2 fluxes, and concentrations of trace metals that react with H2O2 to form the highly toxic hydroxyl and ferryl radicals (Linley et al., 2012).
Possible Role of the Released H2O2 in Coral Protection from Bacteria
Corals secrete various chemicals for preventing bacterial attachment, colonization and invasion (Ritchie, 2006). H2O2 and other ROS, which are co-released with it or form as it reacts with trace metals, may act as antimicrobial agents when released at high enough concentrations (Linley et al., 2012). In a rather crude calculation, we can estimate H2O2 concentrations in the diffusive boundary layer (DBL) at the coral–water interface prior to dilution in the medium. For this calculation we take the overall moles of H2O2 released following stimulus as presented in Figure 3G (340 ± 47 pmol, mean ± SE n = 63). We then divide the averaged moles of H2O2 by the volume into which H2O2 is released (20 mm3 = 10 mm2, site of stimulus × 2 mm DBL). This results in an average H2O2 concentration of 17 ± 2.4 μM at the site of stimulus. Considering all 103 experiments reported here, H2O2 concentrations of 10–20 μM are calculated for 15% of the experiments, and H2O2 concentrations higher than 20 μM are calculated for additional 14% of the experiments.
Are these concentrations high enough to be toxic for bacteria? In our assays with V. coralliilyticus, 10 and 20 μM H2O2 were found to induce 30 and 70% mortality, respectively (Figure 4B). Based on these assays, the estimated average value of 17 μM H2O2 released to the DBL is high enough to induce some bacterial mortality. Furthermore, H2O2 concentrations exceeding 17 μM, which were estimated in several individual experiments, are high enough to induce significant mortality. It is important to note however, that the bacterial sensitivity to H2O2 obtained in such assays vary substantially, depending on the bacterial density and growth phase, experimental medium, incubation length etc. For example, Munn et al. (2008) reported a higher resistance to H2O2 of various coral associated bacteria including V. coralliilyticus, with toxic effects seen only at mM H2O2 concentrations. Another complication of the H2O2 toxicity assays is that they fail to probe for the toxicity of other ROS (and possibly other antibacterial compounds) that may be released together with H2O2 to the coral surface. These ROS in combination with trace metals in the medium or in the bacterial cells can become lethal even at moderate concentrations (Sharma et al., 2012). Hence, while our data hints at the potential role of the released H2O2 as a bactericide, more research is required to determine if H2O2 indeed acts as a bactericide in situ.
In plants, the rapid ROS release in response to pathogens, often result in programmed cell death of the cells surrounding the pathogen, preventing it from penetrating into the plant tissue (Levine et al., 1994). In analogy to plants, we speculate that the rapid and local H2O2 release we measured in response to bacteria may result in a localized programmed cell death (apoptosis) of a single polyp. Such a mechanism may protect the whole colony from proliferation of the pathogen. Since each of the polyps in the coral colony is performing all life functions, a local damage to a single polyp may not reduce the coral fitness. Corals were reported to actively cut off nutritional supply to injured parts of the colony if the coral was suffering from bleaching (Fine et al., 2002). Moreover, since corals can stop apoptotic pathways (Kvitt et al., 2011) apoptosis can be contained within a small part of the colony. Nowadays, when infectious coral diseases are becoming more prevalent in concert with the rise in sea surface temperature; it is worth examining whether colonial corals can apply ROS induced programmed cell death in order to protect themselves from pathogens.
Conclusions
Our novel findings of local, rapid release of H2O2 by S. pistillata upon contact with Vibrio, implies that the corals chemically sense the bacteria and respond to their presence. The released H2O2 can build up to 10–20 μM in the coral diffusive boundary layer; high enough to induce mortality of the coral pathogen V. coralliilyticus. The H2O2 release observed here, together with our recent reports of H2O2 release by corals under flow (Armoza-Zvuloni and Shaked, 2014), during feeding, and in response to different chemical and physical stimuli (Armoza-Zvuloni et al., 2016), demonstrate the broad use of H2O2 during interactions of corals with their surroundings. The actual roles of H2O2 in these interactions remain to be elucidated.
Author Contributions
YS, RA, and AS designed the research; RA and AS performed the experiments; RA analyzed the data. YS and RA wrote the manuscript.
Conflict of Interest Statement
The authors declare that the research was conducted in the absence of any commercial or financial relationships that could be construed as a potential conflict of interest.
Acknowledgments
We wish to thank Murielle Dray for her assistance with the lab work and also to Daniel Sher, Eugene Rosenberg, Assaf Vardi, Orr Shapiro, and Ariel Kushmaro for their good advice and helpful discussions. This study was supported by Israel Science Foundation grant number 248/11 to YS (www.isf.org.il).
Supplementary Material
The Supplementary Material for this article can be found online at: http://journal.frontiersin.org/article/10.3389/fmars.2016.00124
References
Aeby, G. S., and Santavy, D. L. (2006). Factors affecting the susceptibility of the coral Montastrea faveolata to black-band disease. Mar. Ecol. Prog. Ser. 318, 103–110. doi: 10.3354/meps318103
Apel, K., and Hirt, H. (2004). Reactive oxygen species: metabolism, oxidative stress, and signal transduction. Annu. Rev. Plant. Biol. 55, 373–399. doi: 10.1146/annurev.arplant.55.031903.141701
Armoza-Zvuloni, R., Schneider, A., Sher, D., and Shaked, Y. (2016). Rapid Hydrogen Peroxide release from the coral Stylophora pistillata during feeding and in response to chemical and physical stimuli. Sci. Rep. 6:21000. doi: 10.1038/srep21000
Armoza-Zvuloni, R., and Shaked, Y. (2014). Release of hydrogen peroxide and antioxidants by the coral Stylophora pistillata to its external milieu. Biogeosciences 11, 4587–4598. doi: 10.5194/bg-11-4587-2014
Arotsker, L., and Kushmaro, A. (2015). “Vibriosis,” in Diseases of Coral, eds C. M. Woodley, C. A. Downs, A. W. Bruckner, J. W. Porter, Galloway, and S. B. John (Hoboken, NJ: Wiley & Sons, Inc.), 206–220.
Banin, E., Vassilakos, D., Orr, E., Martinez, R. J., and Rosenberg, E. (2003). Superoxide dismutase is a virulence factor produced by the coral bleaching pathogen Vibrio shiloi. Curr. Microbiol. 46, 418–422. doi: 10.1007/s00284-002-3912-5
Bellwood, D. R., and Hughes, T. P. (2001). Regional-scale assembly rules and biodiversity of coral reefs. Science 292, 1532–1535. doi: 10.1126/science.1058635
Ben-Haim, Y., and Rosenberg, E. (2002). A novel Vibrio sp. pathogen of the coral Pocillopora damicornis. Mar. Biol. 141, 47–55. doi: 10.1007/s00227-002-0797-6
Bourne, D. G., Garren, M., Work, T. M., Rosenberg, E., Smith, G. W., and Harvell, C. D. (2009). Microbial disease and the coral holobiont. Trends Microbiol. 17, 554–562. doi: 10.1016/j.tim.2009.09.004
Coteur, G., Warnau, M., Jangoux, M., and Dubois, P. (2002). Reactive oxygen species (ROS) production by amoebocytes of Asterias rubens (Echinodermata). Fish Shellfish Immunol. 12, 187–200. doi: 10.1006/fsim.2001.0366
DiSalvo, L., and Gundersen, K. (1971). Regenerative functions and microbial ecology of coral reefs. I. Assays for microbial population. Can. J. Microbiol. 17, 1081–1089. doi: 10.1139/m71-171
Fine, M., Oren, U., and Loya, Y. (2002). Bleaching effect on regeneration and resource translocation in the coral Oculina patagonica. Mar. Ecol. Prog. Ser. 234, 119–125. doi: 10.3354/meps234119
Geffen, Y., Ron, E. Z., and Rosenberg, E. (2009). Regulation of release of antibacterials from stressed scleractinian corals. FEMS Microbiol. Lett. 295, 103–109. doi: 10.1111/j.1574-6968.2009.01590.x
Geffen, Y., and Rosenberg, E. (2005). Stress-induced rapid release of antibacterials by scleractinian corals. Mar. Biol. 146, 931–935. doi: 10.1007/s00227-004-1505-5
Gough, D. R., and Cotter, T. G. (2011). Hydrogen peroxide: a Jekyll and Hyde signaling molecule. Cell Death Dis. 2:e213. doi: 10.1038/cddis.2011.96
Kvitt, H., Rosenfeld, H., Zandbank, K., and Tchernov, D. (2011). Regulation of apoptotic pathways by Stylophora pistillata (Anthozoa, Pocilloporidae) to survive thermal stress and bleaching. PLoS ONE 6:e28665. doi: 10.1371/journal.pone.0028665
Lamb, C., and Dixon, R. A. (1997). The oxidative burst in plant disease resistance. Annu. Rev. Plant. Phys. 48, 251–275. doi: 10.1146/annurev.arplant.48.1.251
Levine, A., Tenhaken, R., Dixon, R., and Lamb, C. (1994). H2O2 from the oxidative burst orchestrates the plant hypersensitive disease resistance response. Cell 79, 583–593. doi: 10.1016/0092-8674(94)90544-4
Libro, S., Kaluziak, S. T., and Vollmer, S. V. (2013). RNA-seq profiles of immune related genes in the staghorn coral Acropora cervicornis infected with white band disease. PLoS ONE 8:e81821. doi: 10.1371/journal.pone.0081821
Linley, E., Denyer, S. P., McDonnell, G., Simons, C., and Maillard, J. Y. (2012). Use of hydrogen peroxide as a biocide: new consideration of its mechanisms of biocidal action. J. Antimicrob. Chemother. 67, 1589–1596. doi: 10.1093/jac/dks129
McDowell, R. E., Amsler, C. D., McClintock, J. B., and Baker, B. J. (2014). Reactive oxygen species as a marine grazing defense: H2O2 and wounded Ascoseira mirabilis both inhibit feeding by an amphipod grazer. J. Exp. Mar. Biol. Ecol. 458, 34–38. doi: 10.1016/j.jembe.2014.04.012
Moriarty, D. J. W. (1979). Biomass of suspended bacteria over coral reefs. Mar. Biol. 53, 193–200. doi: 10.1007/BF00389189
Munn, C. B. (2015). The role of Vibrios in diseases of corals. Microbiol. Spectr. 3:4. doi: 10.1128/microbiolspec.VE-0006-2014
Munn, C. B., Marchant, H. K., and Moody, A. J. (2008). Defences against oxidative stress in vibrios associated with corals. FEMS Microbiol. Lett. 281, 58–63. doi: 10.1111/j.1574-6968.2008.01073.x
Mydlarz, L. D., Couch, C. S., Weil, E., Smith, G., and Harvell, C. D. (2009). Immune defenses of healthy, bleached and diseased Montastraea faveolata during a natural bleaching event. Dis. Aquat. Organ. 87, 67–78. doi: 10.3354/dao02088
Mydlarz, L. D., and Jacobs, R. S. (2006). An inducible release of reactive oxygen radicals in four species of gorgonian corals. Mar. Freshwater Behav. Physiol. 39, 143–152. doi: 10.1080/10236240600708512
Mydlarz, L. D., McGinty, E. S., and Harvell, D. C. (2010). What are the physiological and immunological responses of coral to climate warming and disease? J. Exp. Biol. 213, 934–945. doi: 10.1242/jeb.037580
Ng, J. C. Y., Chan, Y., Tun, H. M., Leung, F. C. C., Shin, P. K. S., and Chiu, J. M. Y. (2015). Pyrosequencing of the bacteria associated with Platygyra carnosus corals with skeletal growth anomalies reveals differences in bacterial community composition in apparently healthy and diseased tissues. Front. Microbiol. 6:1142. doi: 10.3389/fmicb.2015.01142
Rädecker, N., Pogoreutz, C., Voolstra, C. R., Wiedenmann, J., and Wild, C. (2015). Nitrogen cycling in corals: the key to understanding holobiont functioning? Trends Microbiol. 23, 490–497. doi: 10.1016/j.tim.2015.03.008
Raina, J. B., Tapiolas, D., Willis, B. L., and Bourne, D. G. (2009). Coral-associated bacteria and their role in the biogeochemical cycling of sulfur. Appl. Environ. Microbiol. 75, 3492–3501. doi: 10.1128/AEM.02567-08
Repetto, M., Semprine, J., and Boveris, A. (2012). “Lipid peroxidation: chemical mechanism, biological implications and analytical determinations,” in Lipid Peroxidation, ed A. Catala (Rijeka: InTechEurope), 1–28.
Reshef, L., Koren, O., Loya, Y., Zilber-Rosenberg, I., and Rosenberg, E. (2006). The coral probiotic hypothesis. Environ. Microbiol. 8, 2068–2073 doi: 10.1111/j.1462-2920.2006.01148.x
Ritchie, K. B. (2006). Regulation of microbial populations by coral surface mucus and mucus-associated bacteria. Mar. Ecol. Prog. Ser. 322, 1–14. doi: 10.3354/meps322001
Ritchie, K. B. (2011). “Bacterial symbionts of corals and Symbiodinium,” in Beneficial Microorganisms in Multicellular Life Forms, eds E. Rosenberg and U. Gophna (Berlin: Springer), 139–150.
Rohwer, F., Seguritan, V., Azam, F., and Knowlton, N. (2002). Diversity and distribution of coral-associated bacteria. Mar. Ecol. Prog. Ser. 243, 1–10. doi: 10.3354/meps243001
Rosenberg, E., and Ben-Haim, Y. (2002). Microbial diseases of corals and global warming. Environ. Microbiol. 4, 318–326. doi: 10.1046/j.1462-2920.2002.00302.x
Rypien, K. L., Ward, J. R., and Azam, F. (2010). Antagonistic interactions among coral-associated bacteria. Environ. Microbiol. 12, 28–39. doi: 10.1111/j.1462-2920.2009.02027.x
Saragosti, E., Tchernov, D., Katsir, A., and Shaked, Y. (2010). Extracellular production and degradation of superoxide in the coral Stylophora pistillata and cultured Symbiodinium. PLoS ONE 5:e12508. doi: 10.1371/journal.pone.0012508
Schmitt, F. J., Renger, G., Friedrich, T., Kreslavski, V. D., Zharmukhamedov, S. K., Los, D. A., et al. (2014). Reactive oxygen species: re-evaluation of generation, monitoring and role in stress-signaling in phototrophic organisms. BBA Bioenergetics 1837, 835–848. doi: 10.1016/j.bbabio.2014.02.005
Shaked, Y., and Armoza-Zvuloni, R. (2013). Dynamics of hydrogen peroxide in a coral reef: sources and sinks. J. Geophys. Res. Biogeo. 118, 1793–1801. doi: 10.1002/2013jg002483
Shaked, Y., Harris, R., and Klein-Kedem, N. (2010). Hydrogen peroxide photocycling in the Gulf of Aqaba, Red Sea. Environ. Sci. Technol. 44, 3238–3244. doi: 10.1021/es902343y
Shapiro, O. H., Vicente, I., Fernandez, B., Garrena, V. I., Guastoa, M., Kramarsky-Winter, E., et al. (2014). Vortical ciliary flows actively enhance mass transport in reef corals. Proc. Natl. Acad. Sci. U.S.A. 111, 13391–13396. doi: 10.1073/pnas.1323094111
Sharma, P., Jha, A. B., Dubey, R. S., and Pessarakli, M. (2012). Reactive oxygen species, oxidative damage, and antioxidative defense mechanism in plants under stressful conditions. J. Bot. 2012:217037. doi: 10.1155/2012/217037
Shnit-Orland, M., and Kushmaro, A. (2009). Coral mucus-associated bacteria: a possible first line of defense. FEMS Microbiol. Ecol. 67, 371–380. doi: 10.1111/j.1574-6941.2008.00644.x
Toren, A., Landau, L., Kushmaro, A., Loya, Y., and Rosenberg, E. (1998). Effect of temperature on adhesion of Vibrio strain AK-1 to Oculina patagonica and on coral bleaching. Appl. Environ. Microbiol. 64, 1379–1384.
Webster, N. S., Negri, A. P., Botté, E. S., Laffy, P. W., Flores, F., Noonan, S., et al. (2016). Host-associated coral reef microbes respond to the cumulative pressures of ocean warming and ocean acidification. Sci. Rep. 6:19324. doi: 10.1038/srep19324
Weinberger, F. (2007). Pathogen-induced defense and innate immunity in macroalgae. Biol. Bull. 213, 290–302. doi: 10.2307/25066646
Ziegler, M., Roik, A., Porter, A., Zubier, K., Mudarris, M. S., Ormond, R., et al. (2016). Coral microbial community dynamics in response to anthropogenic impacts near a major city in the central Red Sea. Mar. Pollut. Bull. 105, 629–640. doi: 10.1016/j.marpolbul.2015.12.045
Zvuloni, A., Artzy-Randrup, Y., Katriel, G., Loya, Y., and Stone, L. (2015). Modeling the impact of white-plague coral disease in climate change scenarios. PLoS Comput. Biol. 11:e1004151. doi: 10.1371/journal.pcbi.1004151
Keywords: hydrogen peroxide, Stylophora pistillata, coral–bacteria interactions, Vibrio, oxidative burst response, coral surface layer
Citation: Armoza-Zvuloni R, Schneider A and Shaked Y (2016) Rapid Hydrogen Peroxide Release during Coral-Bacteria Interactions. Front. Mar. Sci. 3:124. doi: 10.3389/fmars.2016.00124
Received: 20 March 2016; Accepted: 01 July 2016;
Published: 14 July 2016.
Edited by:
Colleen Hansel, Woods Hole Oceanographic Institution, USAReviewed by:
John Robert Helms, Morningside College, USADavid Waite, University of New South Wales, Australia
Copyright © 2016 Armoza-Zvuloni, Schneider and Shaked. This is an open-access article distributed under the terms of the Creative Commons Attribution License (CC BY). The use, distribution or reproduction in other forums is permitted, provided the original author(s) or licensor are credited and that the original publication in this journal is cited, in accordance with accepted academic practice. No use, distribution or reproduction is permitted which does not comply with these terms.
*Correspondence: Rachel Armoza-Zvuloni, cmFjaGVsYXJtb3phQGdtYWlsLmNvbQ==
Yeala Shaked, eWVhbGEuc2hha2VkQG1haWwuaHVqaS5hYy5pbA==