- 1Israel Oceanographic and Limnological Research, National Institute of Oceanography, Haifa, Israel
- 2Institute of Marine Science, University of California Santa Cruz, Santa Cruz, CA, USA
This study explores the potential impacts of microbes deposited into the surface seawater of the southeastern Mediterranean Sea (SEMS) along with atmospheric particles on marine autotrophic and heterotrophic production. We compared in situ changes in autotrophic and heterotrophic microbial abundance and production rates before and during an intense dust storm event in early September 2015. Additionally, we measured the activity of microbes associated with atmospheric dry deposition (also referred to as airborne microbes) in sterile SEMS water using the same particles collected during the dust storm. A high diversity of prokaryotes and a low diversity of autotrophic eukaryotic algae were delivered to surface SEMS waters by the storm. Autotrophic airborne microbial abundance and activity were low, contributing ~1% of natural abundance in SEMS water and accounting for 1–4% to primary production. Airborne heterotrophic bacteria comprised 30–50% of the cells and accounted for 13–42% of bacterial production. Our results demonstrate that atmospheric dry deposition may supply not only chemical constitutes but also microbes that can affect ambient microbial populations and their activity in the surface ocean. Airborne microbes may play a greater role in ocean biogeochemistry in the future in light of the expected enhancement of dust storm durations and frequencies due to climate change and desertification processes.
Introduction
Aerosols, including mineral-dust, are regularly transported across marine systems, supplying nutrients and trace metals to the surface water (Prospero et al., 2005). Aerosols may also contain a wide array of microorganisms (reviewed in Griffin, 2007; Després et al., 2012; Polymenakou, 2012), which can be transported thousands of kilometers from their place of origin within a few days (Prospero et al., 2005; Kellogg and Griffin, 2006). These aerosol-associated (airborne) microbes may include heterotrophic bacteria (e.g., Seifried et al., 2015), fungi (e.g., Dannemiller et al., 2014), cyanobacteria, chemolitoptophic bacteria, and other autotrophic algae (e.g., Marshall and Chalmers, 1997; Lang-Yona et al., 2014; Gat et al., 2016), as well as viruses (e.g., Chow and Suttle, 2015). The diversity and viability of airborne microbes depends on the aerosol's route prior deposition (Rahav et al., 2016a).
Several studies have examined the effect desert dust and aerosols have on ocean productivity and microbial biomass, via on-board microcosm or mesocosm experiments that simulated atmospheric nutrient addition (e.g., Mills et al., 2004; Herut et al., 2005; Mackey et al., 2007; Pulido-Villena et al., 2008; Christaki et al., 2011). Overall, the impacts observed following desert dust or aerosol additions are diverse and cannot all be explained by the inducement of a “fertilization response” (Guieu et al., 2014). Although variability in aerosol composition and changes in ocean hydrography and ecosystem structure at the time of deposition have been invoked in order to explain the diverse responses (Paytan et al., 2009), another possible explanation is the impact of the airborne microbes delivered with the added dust/dry aerosol deposition. Such microbes, if viable, may interact with ambient microbial populations in the receiving environment. A recent study conducted across the North Atlantic Ocean measured the abundance of microbes in the lower atmosphere and estimated that airborne microbes cross over 10,000 Km in several days and that millions of microbes are being exchanged on a daily basis between the atmosphere and the ocean's surface layer (Mayol et al., 2014). Further, studies performed in freshwater (Reche et al., 2009; Peter et al., 2014) and marine (Rahav et al., 2016a) environments suggest that airborne microorganisms may remain viable after deposition and thus may play an important role in the receiving aquatic system. For example, Peter et al. (2014) reported viable airborne bacteria following dust deposition into sterile lake water, and Rahav et al. (2016a) showed activity of heterotrophic airborne bacteria in sterile seawater and measured both carbon and nitrogen fixation by these microbes. Airborne microbes can remain viable for decades (Gorbushina et al., 2007), and yet, the full extent of this “biological” addition and the ecological importance of airborne microbes in natural environments are unclear (Hervas et al., 2009; Rahav et al., 2016a). This is because our knowledge about the viability and functionality of airborne microorganisms upon deposition in the ocean is scant (Polymenakou et al., 2008).
The southeastern Mediterranean Sea (SEMS) is an ideal marine environment for studying the role of aerosols and associated microbes on surface ocean microbial production for multiple reasons. First, it is subjected to relatively high aerosol deposition throughout the year (Guerzoni et al., 1999; Ganor et al., 2010). Secondly, it is an oligotrophic environment with low inorganic nutrients (Herut et al., 2000; Kress and Herut, 2001; Kress et al., 2014) and low autotrophic and heterotrophic activity (Raveh et al., 2015). Thus, any external input of micro/macronutrients, along with aerosols-associate microbiota, can have a substantial effect upon interaction with the ambient microbial populations.
In this study, we followed the in situ temporal dynamics of autotrophic and heterotrophic microbial abundances and production rates in the SEMS surface waters during an intense natural dust storm event that lasted a few days. Specifically, we evaluated the role that the autotrophic and heterotrophic microbial communities associated with atmospheric dry deposition particles play in the SEMS surface water following this event.
Materials and Methods
Sampling Strategy
Surface SEMS water (~1 m depth) was sampled every 12 h at a coastal station near the National Institute of Oceanography (Haifa, Israel, Lat. 32.28N, Lon. 34.95E), from the 8th to the 13th of September 2015, during an intense storm event (Figures 1A,B). Seawater temperatures were measured using an in situ HOBO Pendant Temperature data logger (model UA-002-64, Onset Computer Corporation) mounted on the rocky bottom at a depth of ~4 m. Salinity was measured using a Yellow Spring Instruments YSI-6000. In order to quantify dry deposition during the dust storm event, atmospheric suspended particles were collected on a Whatman 41 filter (125 mm, ~20 μm pore size) using a high volume total suspended particles (TSP) sampler (located on a headland pointing into the sea, 22 m above sea level) at a flow rate of 60 m3 h−1 for 24 h (Figure 1), as described in Herut et al. (2002). Dry deposition rates were calculated based on Al concentration in the collected aerosol (measured by XRF, 7.6% dry wt. Table S1), a settling velocity of 1.8 cm s−1 (Kocak et al., 2005), and the particles weight collected on the filter for the volume pumped during the collection time (1.77 mg m−3 air). This yielded a deposition of 1.05 mg of dry deposition L−1 in seawater when integrated over the upper 5 m mixed layer, similar to values reported for other intense dust storm events in this area (Herut et al., 2005). Seawater was sampled twice a day for chlorophyll-a (acetone extraction), cyanobacterial abundance, pico-eukaryotic abundance and heterotrophic bacterial abundance (flow-cytometry), primary production (NaH14CO3 incorporation), and bacterial production (3H-Leucine incorporation) measurements.
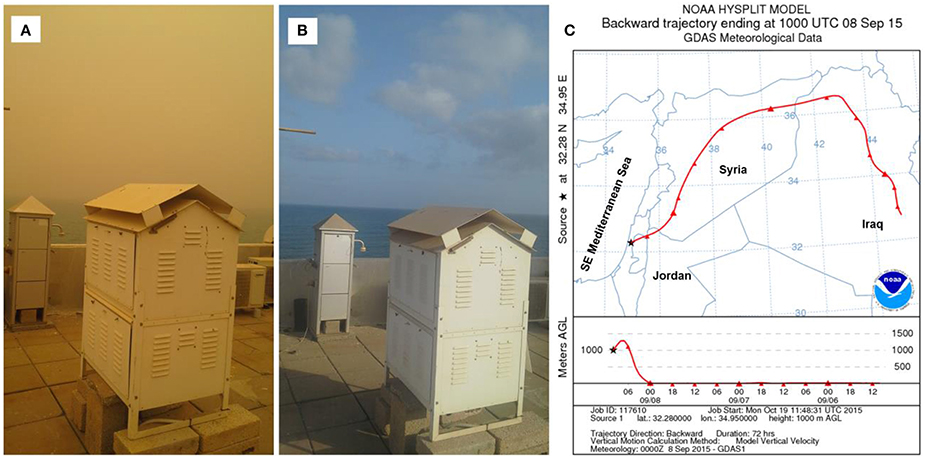
Figure 1. Dusty (A) and clear (B) skies off the SE Mediterranean coast between the 8th (A) and 13th (B) of September 2015. Air mass back trajectory analysis shows the dust's origin (C).
Aerosol Collection and Bioassay Experiment
Dry deposited material was collected on September 8th 2015 during a major dust storm event using a pre-cleaned glass deposition plate. The deposited particles were collected from the plate using a clean plastic knife, transferred into prewashed (10% hydrochloric acid) sterile 2 ml plastic tubes and stored at −20°C until further analyses. Three-day back trajectories arriving at 500 and 1000 m altitude levels were calculated, commencing at 10.00 UTC using the HYSPLIT model from the Air-Resources Laboratory, NOAA (Figure 1C). A few days after the aerosol deposition event (between the 16th and 20th of September 2015), an aerosol-enrichment microcosm bioassay experiment was carried out in triplicate using 4.6-L acid-washed polycarbonate Nalgene bottles and sterile (0.2 μm filtered and autoclaved-killed) surface SEMS water. The collected aerosol was added to each of the bottles (~1.5 mg dust L−1 of sterile surface seawater), and the bottles were incubated in an outdoor pool with seawater flow-through in order to maintain ambient temperatures. The pool was covered with a neutral density screening mesh to simulate ambient light and the experiment lasted for 4 days. Blank treatments of sterile SEMS water without added aerosol were carried out in parallel and sampled at the beginning (0.5 h) and at the end of the experiment (96 h). Subsamples of seawater from each incubation bottle were collected for chlorophyll-a, cyanobacterial abundance, pico-eukaryote abundance, heterotrophic bacteria abundance, primary production, and bacterial production measurements, at 0.5, 9, 24, 48, 57, 72, and 96 h after aerosol addition. The differences between in situ values (measured during September 8–13, 2015, and representing the ambient community's response to the dust storm event) and those measured during the enrichment experiments (September 16–20, 2015) performed in sterile seawater using the same particles that were collected during the dust storm, were used to estimate the potential contribution of the autotrophic and heterotrophic microbes associated with the event (hereafter airborne microbes) to the overall in situ microbial abundance and production rates following aerosol deposition during the storm.
Inorganic Nutrients and Trace Metals Leached from the Aerosol
These micro- and macro-nutrients from the aerosol particles were extracted according to Buck et al. (2012) and analyzed using a flow injection autoanalyzer (FIA, Lachat Instruments model QuickChem 8000) as describe in Chen et al. (2006). A detailed description of the method can be found in the supporting information.
X-Ray Fluorescence (XRF)
An elemental analysis of the collected aerosol particles was carried out using an ED-XRF spectrometer (SPECTROSCOUT) in a vacuum chamber. A detailed description of this method can be found in the supporting information.
DNA Extractions and High-Throughput Phylogenetic and Sequence Analyses
DNA was extracted from the aerosol particles that were collected on the clean glass deposition plates during the atmospheric deposition event. The DNA was extracted using the phenol–chloroform method, modified from Massana et al. (1997). A detailed description of this method can be found in the supporting information.
Chlorophyll-a Extraction
Autotrophic biomass was determined using the non-acidification method (Welschmeyer, 1994). A detailed description of this method can be found in the supporting information.
Pico-Phytoplankton and Bacterial Abundance
Water samples (1.8 mL) were analyzed using an Attune® Acoustic Focusing Flow Cytometer (Applied Biosystems) equipped with a syringe-based fluidic system and 488 and 405 nm lasers (Vaulot and Marie, 1999). A detailed description of this method can be found in the supporting information.
Primary Production
Photosynthetic carbon fixation rates were estimated using the 14C incorporation method (Steemann-Nielsen, 1952). A detailed description of this method can be found in the supporting information.
Bacterial Production
Rates were estimated using the 3H-leucine (Amersham, specific activity: 160 Ci mmol−1) incorporation method (Simon et al., 1990). A detailed description of this method can be found in the supporting information.
Statistical Analyses
The different variables presented in the figures and tables are averages and standard deviation (biological replicates, n = 3). Changes in chl-a, production rates, and abundance of cyanobacteria, pico-eukaryotes, and bacteria throughout each of the experiments (4–5 days) were evaluated using a one-way analysis of variance (ANOVA), followed by a Fisher LSD multiple comparison post-hoc test with a confidence level of 95% (α = 0.05). The difference between samples collected in situ at the SEMS during the dust storm event (8–13 September 2015) and those from the sterile seawater bioassay experiments (16–20 September 2015) were evaluated using a student t-test with a confidence level of 95% (α = 0.05). These statistical analyses were carried out using the XLSTAT software. The Shannon-Weiner diversity index (Margalef, 1958) was calculated using the primer software.
Results
The initial pre-storm physiochemical characteristics of the surface SEMS are shown in Table 1. The ambient surface seawater depicts a high temperature (~30°C) and high salinity (39.8). The algal biomass, derived from chl-a levels, was low overall (0.17 ± 0.02 mg m−3), as were the cyanobacterial and picophytoplankton abundances (1.9 × 104 and 8.0 × 102 cells mL−1, respectively). Concurrently, the primary production rates were low (1.99 ± 0.41 μg C L−1 d−1). In contrary to the low autotrophic biomass, bacterial heterotrophs were more abundant (8.5 × 105 cells mL−1), and active (5.18 ± 1.51 μg C L−1 d−1).
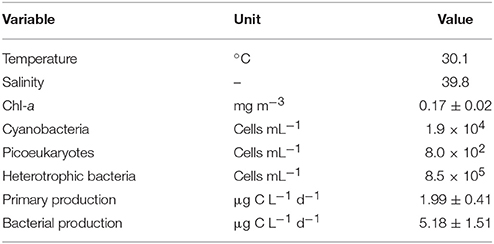
Table 1. The initial characteristics of the SE Mediterranean seawater 3 days prior to the dust storm event (5th September 2015).
The aerosols collected during early September 2015 had a very high fraction of Ca (20%) and were rich in Mg (4.3%), Fe (6.3%), Mn (945 ppm), Sr (450 ppm), Al (~67 ng mg−1), and Cu (~25 ng mg−1; Table 2, Table S1). They also had significant amounts of soluble NONO (~176 nmol mg−1) and relatively less NH (~5.4 nmol mg−1) and PO (~1.5 nmol mg−1), resulting in a high N:P ratio of ~120:1 (Table 2, Table S1).
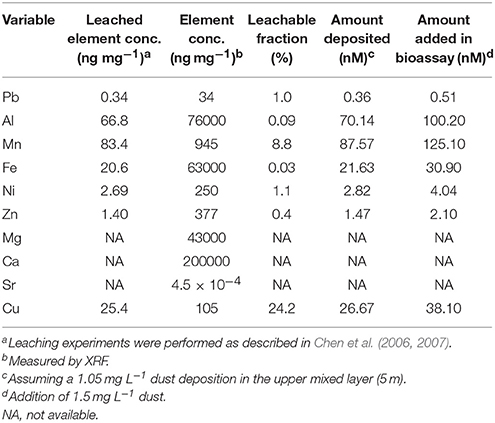
Table 2. The trace metals derived from the aerosols collected in September 8, 2015 and the subsequent values following the dust storm in the SEMS water.
Aerosol-derived autotrophic and heterotrophic microorganisms were also transported with the atmospheric particles (Figure 2). These included a wide array of prokaryotes (>100 families in 23 different phylum, ~650 species, Shannon-Weiner diversity index = 3.88) and a small number of autotrophic eukaryotic microbes (4 families in 2 phyla, 4 species, Shannon-Weiner diversity index = 1.03). Among the prokaryotes, the most dominant families (as relative operational taxonomic units, OTUs) were Cytophagaceae (10.3%), Chloroflexaceae (7.8%), Frankiales and Rhodobacteraceae (6.4% each), and Bacillaceae (4.3%), as well as other bacteria (Figure 2A). The autotrophic eukaryotic microorganisms contained within the aerosol particles belonged to Tracheophyta (61.5%), Chlorodendraceae (23.1%), Bryophyta (8.9%), and Pedinomonadaceae (6.5%) taxa (Figure 2B). Despite the low number of the different eukaryotic-autotrophic families, ~30% were of marine or freshwater origin (i.e., green algae).
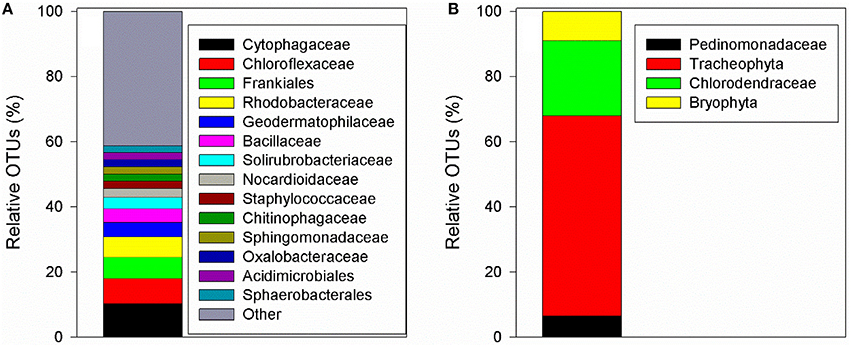
Figure 2. Distributions of bacterial 16S rRNA (A) and 18S rRNA (B) gene reads at a family level, retrieved from a dry deposition particles sample collected on September 8th 2015 off the SE Mediterranean Sea coast.
The In situ Temporal Dynamics of Autotrophic and Heterotrophic Microbial Communities during the Dust Storm Event
The in situ chl-a concentrations gradually increased from its background pre-storm levels (Table 1) to 0.24 mg m−3 within 55 h, corresponding to ~40% change, and decreased back to background levels at day 5 following the dust storm (0.19 mg m−3, Figure 3A, Figure S1). Contrary to the chl-a levels, cyanobacterial abundance exhibited a different yet insignificant temporal trend (P > 0.05), with an immediate 20% decrease in cell numbers (~1.3 × 104 cells mL−1), an increase back to the initial levels after 48 h (~1.7 × 104 cells mL−1), which was followed by another decrease (~1.3 × 104 cells mL−1; Figure 3B, Figure S1). The picoeukaryotes remained unchanged (~8.2 × 102 cells mL−1, P > 0.05; Figure 3C, Figure S1). Heterotrophic bacterial abundance increased by 30% within 10 h (8.7 × 105 cells mL−1, P = 0.05), an increase that lasted for 3 days (Figure 3D, Figure S1, P < 0.05). In situ primary production rates reached maximal values 48 h after deposition (a 25% increase, P > 0.05) and then decreased to background levels over the course of 5 days (Figure 4A, Figure S2). Bacterial production increased slightly (~15%, P > 0.05), however this increase lasted for only 48 h (Figure 4B, Figure S2).
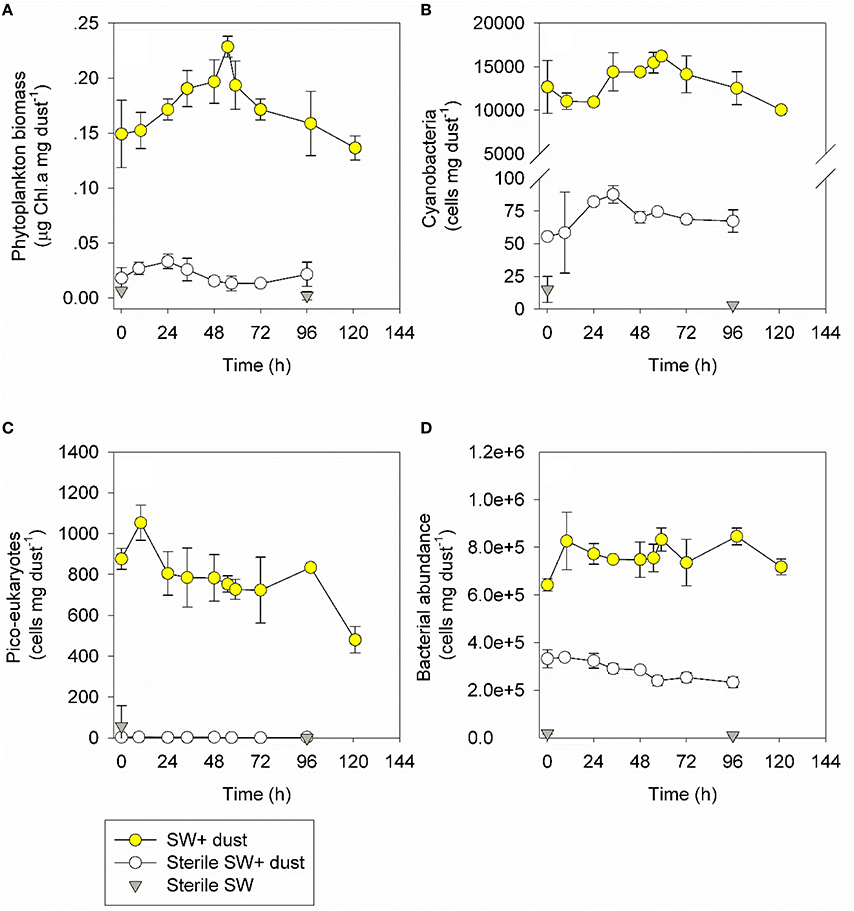
Figure 3. The temporal dynamics of chl-a (A), cyanobacterial abundance (B), pico-eukaryotes abundance (C), and heterotrophic bacterial abundance (D) during a dust storm event (in situ measurements, 1.05 mg L−1, yellow) off the SEMS between the 8th and 13th of September 2015. The contribution of airborne microbes was tested between the 16th and 20th of September 2015 in sterile seawater, either supplemented with 1.5 mg L−1 of the same atmospheric particles deposited the previous week (white) or without any addition (gray). Values (as averages and standard deviations, n = 3) are normalized to the amount of dust added. The un-normalized values are presented in Figure S1.
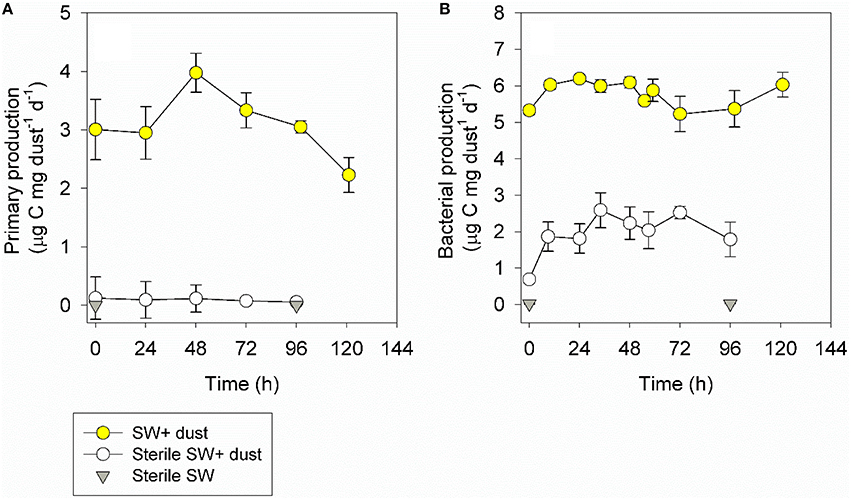
Figure 4. The temporal dynamics of primary production (A) and bacterial production (B) during a dust storm event (in situ measurements, 1.05 mg L−1, yellow) off the SEMS between the 8th and 13th of September 2015. The contribution of airborne microbes was tested between the 16th and 20th of September 2015 in sterile seawater, either supplemented with 1.5 mg L−1 of the same particles deposited the previous week (white) or without any aerosol addition (gray). Values (as averages and standard deviations, n = 3) are normalized to the amount of aerosols added. The un-normalized values are presented in Figure S2.
The Impact of Airborne Autotrophic and Heterotrophic Microorganisms
Airborne autotroph abundance (measured in the sterile seawater after aerosol addition) constituted only a small fraction of the overall photosynthetic biomass observed in situ (Figures 3A–C). Airborne cyanobacteria and picoeukaryotes comprised < 1% of the autotrophic microbial abundance (Figures 3B,C, Figure S1). Airborne heterotrophic bacteria were more significant (Figure S1), comprising 30–50% (abundance per gram of aerosol added) of the total heterotrophic bacteria in the SEMS water during the dust storm (Figure 3D).
Concurrent with the low airborne contribution to autotrophic biomass, the airborne primary production was low (Figure S2), comprising 1–4% of the rates measured in situ (activity per gram aerosol added) during the dust storm event (Figure 4A). The airborne bacterial production rates were higher (Figure S2), corresponding to 13–42% of the rates measured in situ during the dust storm event (Figure 4B). Taken together, the airborne heterotrophic cell specific activity (bacterial production per cell) was not different from the activity measured in the seawater during the dust storm event (~0.01 fg C d−1 cell−1, n = 6, P = 0.67). The airborne autotrophic cell specific activity (primary production per cell) was three-fold lower than in seawater (~19 vs. 6 μg C μg chl-a−1 d−1, n = 6, P < 0.001).
Discussion
The SEMS is constantly exposed to high levels of atmospheric deposition derived primarily from surrounding deserts and land sources (Herut et al., 2002; Lawrence and Neff, 2009). These atmospheric inputs provide a variety of nutrients and trace metals (Table S1 and reviewed in Guieu et al., 2014), which are required for microbial cellular metabolism, enzymatic activity and growth (e.g., Cvetkovic et al., 2010; Huertas et al., 2014). In addition to nutrients and trace metals, atmospheric deposition may also introduce a wide array of airborne microorganisms to surface seawater (reviewed in Griffin, 2007; Polymenakou, 2012). Some of these microbes can remain viable and fix carbon (C) and dinitrogen (N2) upon deposition in seawater (Rahav et al., 2016a).
The aerosol deposition event into the SEMS water in early September 2015 was an exceptional regional event. Previous works linked dust fallout over the Levantine Basin to Saharan origins (Ganor and Mamane, 1982; Herut et al., 1999, 2005), whereas the studied event originated from drylands in Eastern Syria (Figure 1C). This difference in origin is evident in the chemical composition of the Syrian aerosol particles when compared to the reported composition of Saharan dust particles (Table 2, Table S1). The most prominent difference was in Ca, which was much higher than the reported fraction in Saharan aerosols (Krom et al., 1999; Goudie and Middleton, 2001; Herut et al., 2001). This high Ca content in the Syrian aerosols likely reflects a source origin of calciorthid soils, which covers large areas in Syria (Ilaiwi, 1985). The Syrian aerosol was also richer in Mg, Fe, Mn, and Sr (normalized to Al) when compared to the reported concentrations in Saharan samples (Table S1, Krom et al., 1999; Goudie and Middleton, 2001; Herut et al., 2001). When calculating the soluble fraction of trace metals that were leached from the aerosols and added to seawater during this event (Table 2) concentrations were below the threshold for toxicity for phytoplankton in seawater (e.g., Sunda, 2012). In fact, some of the added trace metals, such as Fe or Zn, are key cofactors for many enzymatic reactions in the marine environment, including photosynthesis and N2 fixation (Falkowski, 1997; Sohm et al., 2011), and may contribute to enhancing production.
The studied Syrian aerosol particles released soluble NONO and PO (Table 3, Table S1), which may relieve nutrient stress for autotrophic and heterotrophic microbial biomass and activity in the surface SEMS (i.e., Kress et al., 2005; Zohary et al., 2005; Pitta et al., 2016). Assuming 1.05 mg L−1 of dust deposition into the upper SEMS 5 m mixed layer (see Materials and Methods), ~185 nM of NONO and ~1.5 nM PO were actually added into this water layer (Table 3), which constitutes ~50 and 5–10% of the nutrient concentration typically reported for this system during summer, respectively (Kress et al., 2014; Raveh et al., 2015). Due to the extremely oligotrophic nature of the SEMS, any amendment might prove to be important and may alter microbial dynamics via the release of scarce, key-limiting nutrients. For example, several authors showed that inorganic nitrogen (N) and phosphorus (P) may enhance algae biomass and growth rates in the SEMS water (Kress et al., 2005; Lagaria et al., 2011; Pitta et al., 2016), whereas P (Thingstad et al., 2005; Zohary et al., 2005) or dissolved organic carbon (Rahav et al., 2016b) can also stimulate heterotrophic bacterial activity. Thus, assuming a C to chl-a ratio of ~80 (e.g., Behrenfeld et al., 2005; Wang et al., 2009) and a 106:1 C:P ratio (Redfield, 1934), the addition of the dust-derived P could explain a chl-a enhancement of ~0.05 mg m−3 above the ambient levels. The chl-a enhancement calculated based on the added P is lower than the overall chl-a change measured following the dust examined here (~0.08 mg m−3, Figure S1 and see Discussion below). One of the possible explanations for this difference between the actual increase in chl-a (~0.08 mg m−3) and the dust-P derived chl-a (~0.05 mg m−3) may be chl-a associated with airborne microbes (algae and cyanobacteria).
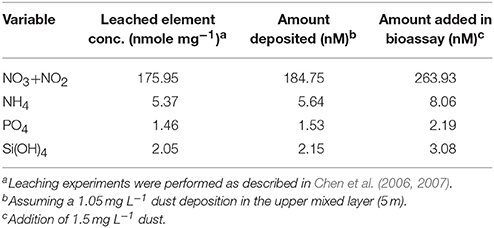
Table 3. Leached nutrients derived from the aerosols collected in September 8, 2015 and the subsequent values following the dust storm in the SEMS water.
Indeed, both autotrophic and heterotrophic microbes were delivered with the Syrian aerosol studied here (Figure 2). These include commonly isolated bacteria from marine, freshwater, and terrestrial environments (e.g., Hervas and Casamayor, 2009; Cho and Hwang, 2011; Seifried et al., 2015), as well as from the surroundings of the SEMS (Katra et al., 2014; Rahav et al., 2016a). It should be noted, that based on the 16S rRNA gene, we cannot rule out that in addition to heterotrophic microbes, chemolithotrophic bacteria were also part of the aerosol collection (Gat et al., 2016). More specific assays should be carried out in future studies to fully understand whether these chemolithotrophic microbes were actually transported with the aerosol particles. Such chemolithotrophic bacteria may play an important role e in the biogeochemistry of nitrogen, sulfur, and iron (e.g., Saeed and Sun, 2012). In contrast to this broad airborne microbial heterotroph biodiversity, only few algal families associated with the aerosol particles were observed based on the 18S rRNA gene. This may be due to the high settling velocities of large eukaryotic particles (such as those of microphytoplankton). Most of the large-size algae and other terrestrial plants were probably deposited along the dust's route (above land) rather than being suspended over long distances and, therefore, were rarely found in our sample. It is possible, however, that aerosols from different origins would contain different amounts of marine/freshwater autotrophic organisms, and particularly small-size cyanobacteria (Cho and Hwang, 2011; Lang-Yona et al., 2014; Seifried et al., 2015). Thus, a more typical atmospheric deposition event coming from the southwest (e.g., Herut et al., 2005) rather than from the northeast (Figure 1) would potentially carry more algae or small-size cyanobacteria, and, therefore, airborne autotrophs could be more abundant than what we have observed in this study.
In situ Dynamics of Autotrophic and Heterotrophic Microbial Populations Following Aerosol Deposition and the Relative Contribution of Airborne Microbes
A moderate change in the in situ chl-a level and picophytoplankton abundance was observed following the aerosol deposition event of early September 2015 (Figures 3A–C). This is consistent with the expected change derived from the PO addition (~1.5 nM) and its subsequent carbon addition based on the C to chl-a ratio (see above). The relatively limited change in picophytoplankton abundance implies a possible top-down control of small-size autotrophs by grazers (Billen, 1990), dominance of larger-size algae such as microphytoplankton that utilized most of the leached nutrients/trace metals and compete with the picophytoplankton (Duarte et al., 2000), or toxicity of small-size autotrophs (Mann et al., 2002; Paytan et al., 2009). It may also hint at interactions between the ambient population and the airborne microbes that affect the abundance of small-size autotrophs. In contrast, the heterotrophic bacterial abundance was immediately enhanced (Figure 3D), suggesting that the leached nutrients and trace metals, and possibly the addition of airborne microbes, favored heterotrophy. A similar response was recorded by Herut et al. (2005) in the SEMS water following a desert-dust storm event that triggered a slight increase in the chl-a and heterotrophic bacterial abundance and a decrease in cyanobacterial abundance (Prochlorococcus sp.). The similarity in trends observed during these two distinct events lends credibility to the observations.
The temporal dynamics of the in situ primary and bacterial production (Figure 4, Figure S2) are consistent with observed autotrophic and heterotrophic bacterial abundances (Figure 3, Figure S1). The overall low impact of the dust on autotrophic biomass and production is similar to the response reported from an onboard microcosm experiment in the open SEMS (Herut et al., 2005), and was most likely a result of the relatively low nutrient and trace metal amounts added from the dust (Tables 2, 3). It may also suggest the likely control of grazing pressure, and/or possible toxicity effects of the dust (Paytan et al., 2009). Alternatively, it is possible that airborne microbes delivered with the dust interacted with the ambient microbial populations and, in some circumstances, outcompeted them, resulting in the decline of abundance and production rates.
The airborne chl-a (up to 0.03 mg m−3) measured in sterile SEMS water reached 5–30% (biomass per gram of dry deposition particles added) of the total chl-a measured in situ during the event (Figure 3A, Figure S1). This airborne contribution to the total chl-a (~0.03 mg m−3), along with the increase in the chl-a level calculated based on that expected from the uptake of the leached P (~0.05 mg m−3, see above), is consistent with the overall increase measured in situ 55 h post-deposition (~0.08 mg m−3, Figure S1). The contribution of airborne heterotrophic bacteria measured here (abundance per gram of aerosol added) to the total bacterial abundance in the water is lower than the values recently reported for aerosols collected in the SEMS during 2006–2015, representing different source origins and seasons (Rahav et al., 2016a). This highlights the importance of biogeographical aspects in introducing not only a different diversity of airborne microbes but possibly also different quantities of cells with potentially different activities and functions. However, it should be noted that the presence of autotrophic and heterotrophic bacteria, regardless of their fraction of the total abundance measured in situ, is no evidence for their viability in the receiving seawater. It is possible that inactive/dormant cells were leached off the aerosol particles along with viable microbes and were counted using flow cytometry. This issue necessitates additional studies implementing techniques designed to estimate the microbial abundance of active cells.
Another method for estimating whether the retrieved cells are viable upon deposition in seawater is to measure metabolic parameters, such as primary production and bacterial production of the airborne microbes. Our results suggest that airborne primary production was low overall (Figure 4, Figure S2), which is consistent with the low contribution of airborne chl-a and the negligible fraction of small-size airborne photosynthetic microorganisms (Figures 3A–C). On the other hand, airborne bacterial production was more important and immediately responded to the aerosol addition, suggesting that airborne heterotrophs can rapidly interact with the ambient populations. The nature of such interactions can be diverse, depending on the species of the microbes that are associated with the aerosol particles (Rahav et al., 2016a). It is possible that under some circumstances airborne microbes can negatively affect certain microbial groups in seawater (competition, allelopathic affects, cell lysis, etc.), or that they can affect certain ambient populations positively (serving as unique food, relieving N-stress via N2 fixation, etc.). Rahav et al. (2016a) reported airborne heterotrophic bacterial production rates similar to changes observed following dry deposition aerosol amendments in seawater, potentially accounting for 100% of the change. In this study, however, the contribution of airborne heterotrophic bacterial production rates was lower than that reported by Rahav et al. (2016a) and accounted for a maximum of 42% of the ambient-typical production levels in the SEMS (e.g., Raveh et al., 2015), once again highlighting the importance of the aerosol's origin and associated numbers and taxa of airborne microbes.
To the best of our knowledge, this is one of only few attempts to estimate the role of airborne microbes in seawater following a major dust storm. If the results from this dust storm event are representative, the small contribution of airborne autotrophs (in terms of both abundance and C fixation) may suggest that the increase in primary production and chl-a usually observed following dust events (e.g., Herut et al., 2005; Ternon et al., 2011; Gallisai et al., 2014) results from the beneficial impacts of nutrient and/or trace metal additions from the aerosols. However, we postulate that the contribution of airborne microbes to the ambient autotrophic community's chl-a in the SEMS water may occasionally be higher if the dust's route prior to deposition went over marine areas of higher productivity rather than over land, which would be more likely to contain a higher abundance of viable autotrophic organisms that will be viable upon deposition in seawater.
Conclusions
We determine the role of autotrophic and heterotrophic airborne microbes in seawater during a specific dust storm event. Our results demonstrate that in this case, where the dust arrived from an atypical continental source (Figure 1), only a few groups of eukaryotic-autotrophic microbes were transported (Figure 2B) and their overall impact in the surface ocean was thus negligible (Figure 4). In contrast, a higher diversity of prokaryotes (both heterotrophs and possibly autotrophs such as cyanobacteria and chemolitotrophs) was contained within the dry deposition particles (Figure 2A), and at least some of these microbes exhibited immediate activity upon deposition (Figure 4). It should be noted that the open SEMS is an ultra-oligotrophic environment (Kress et al., 2014), much more than our coastal study site (Bar-Zeev and Rahav, 2015; Raveh et al., 2015). We postulate that airborne microbes (along with aerosol-derived nutrients) are likely to have a more profound affect in such oligotrophic provinces.
To date, we cannot say what mechanisms and strategies are used by airborne organisms once deposited in seawater to successfully compete with ambient microbes that are already acclimated in their habitat. It is possible that some chemical components derived from aerosols are toxic to specific groups of organisms (Paytan et al., 2009) or have negative biologically impacts via viral lysis (Vardi et al., 2012; Sharoni et al., 2015), allelopathy, or other mechanisms that may be at play. It is also possible that airborne microbes will synergistically interact with ambient populations. Dedicated studies aimed at filling these knowledge gaps are needed, including investigations of dust-derived allelopathic affects and a comparison between the nutrient uptake rates of airborne bacteria and those of in situ communities. Addressing these aspects will be particularly important in the near future, since climate and anthropogenic changes may increase aerosol deposition (including mineral dust). This, in turn, will also upsurge airborne microbes transfer and deposition in seawater and could, subsequently, impact surface ocean carbon and nitrogen cycles (Rahav et al., 2016a) as well as other biochemical and ecological aspects.
Author Contributions
Conceived and designed the experiment: ER and BH. Performed the experiment: ER, GO, CC, TK, and AP. Analyzed the data: ER, AP, TK, and BH. Contributed reagents/materials/analysis tools: ER, TK, and AP. Wrote the paper: ER, CC, AP, TK, and BH.
Conflict of Interest Statement
The authors declare that the research was conducted in the absence of any commercial or financial relationships that could be construed as a potential conflict of interest.
The reviewer CP and handling Editor declared their shared affiliation, and the handling Editor states that the process nevertheless met the standards of a fair and objective review.
Acknowledgments
We would like to thank Lilach Baumer and Kimberley Bitterwolf for English editing, Dr. Yana Yudkovsky for the XRF analysis and four reviewers that greatly improved the manuscript. This study was supported by a grants awarded by the Ministry of National infrastructures, Energy and Water Resources (grant 3-11519) to ER, by the Ministry of environmental Protection (145–1–2) to ER, by the PERSEUS project's (EC contract 287600) contribution to BH, partially by the ENVIMED MERMEX TRACOMED project's contribution to BH and by the NSF-OCE (grant 0850467) to AP. The authors gratefully acknowledge the NOAA Air Resources Laboratory (ARL) for the provision of the HYSPLIT transport and dispersion model used in this publication.
Supplementary Material
The Supplementary Material for this article can be found online at: http://journal.frontiersin.org/article/10.3389/fmars.2016.00127
References
Bar-Zeev, E., and Rahav, E. (2015). Microbial metabolism of transparent exopolymer particles during the summer months along a eutrophic estuary system. Front. Microbiol. 6:403. doi: 10.3389/fmicb.2015.00403
Behrenfeld, M. J., Boss, E., Siegel, D. A., and Shea, D. M. (2005). Carbon-based ocean productivity and phytoplankton physiology from space. Glob. Biogeochem. Cycles 19, 1–14. doi: 10.1029/2004GB002299
Billen, G. (1990). Dynamics of bacterioplankton in oligogtrophic and eutrophic aquatic environments: bottom-up or top-down control? Hydrobiologia 207, 37–42. doi: 10.1007/BF00041438
Buck, K. N., Moffett, J., Barbeau, K., Bundy, R., Kondo, Y., and Wu, J. (2012). The organic complexation of iron and copper : an intercomparison of competitive ligand exchange – adsorptive cathodic stripping voltammetry (CLE-ACSV) techniques. Limnol. Oceanogr. Methods 10, 496–515. doi: 10.4319/lom.2012.10.496
Chen, Y., Mills, S., Street, J., Golan, D., Post, A., Jacobson, M., et al. (2007). Estimates of atmospheric dry deposition and associated input of nutrients to Gulf of Aqaba seawater. J. Geophys. Res. Atmos. 112, 1–14. doi: 10.1029/2006JD007858
Chen, Y., Street, J., and Paytan, A. (2006). Comparison between pure-water- and seawater-soluble nutrient concentrations of aerosols from the Gulf of Aqaba. Mar. Chem. 101, 141–152. doi: 10.1016/j.marchem.2006.02.002
Cho, B. C., and Hwang, C. Y. (2011). Prokaryotic abundance and 16S rRNA gene sequences detected in marine aerosols on the East Sea (Korea). FEMS Microbiol. Ecol. 76, 327–341. doi: 10.1111/j.1574-6941.2011.01053.x
Chow, C.-E. T., and Suttle, C. A. (2015). Biogeography of viruses in the sea. Annu. Rev. Virol. 2, 41–66. doi: 10.1146/annurev-virology-031413-085540
Christaki, U., Van-Wambeke, F., Lefevre, D., Lagaria, A., Prieur, L., Pujo-Pay, M., et al. (2011). Microbial food webs and metabolic state across oligotrophic waters of the Mediterranean Sea during summer. Biogeosciences 8, 1839–1852. doi: 10.5194/bg-8-1839-2011
Cvetkovic, A., Menon, A. L., Thorgersen, M. P., Scott, J. W., Poole, F. L., Jenney, F. E. Jr., et al. (2010). Microbial metalloproteomes are largely uncharacterized. Nature 466, 779–782. doi: 10.1038/nature09265
Dannemiller, K. C., Reeves, D., Bibby, K., Yamamoto, N., and Peccia, J. (2014). Fungal high-throughput taxonomic identification tool for use with next-generation sequencing (FHiTINGS). J. Basic Microbiol. 54, 315–321. doi: 10.1002/jobm.201200507
Després, V. R., Alex Huffman, J., Burrows, S. M., Hoose, C., Safatov, A. S., Buryak, G., et al. (2012). Primary biological aerosol particles in the atmosphere: a review. Tellus Series B Chem. Phys. Meteorol. 64, 1–40. doi: 10.3402/tellusb.v64i0.15598
Duarte, C. M., Agustí, S., and Agawin, N. S. R. (2000). Response of a Mediterranean phytoplankton community to increased nutrient inputs: a mesocosm experiment. Mar. Ecol. Prog. Ser. 195, 61–70. doi: 10.3354/meps195061
Falkowski, P. (1997). Evolution of the nitrogen cycle and its influence on the biological sequestration of CO2 in the ocean. Nature 387, 272–275. doi: 10.1038/387272a0
Gallisai, R., Peters, F., Volpe, G., Basart, S., and Baldasano, J. M. (2014). Saharan dust deposition may affect phytoplankton growth in the Mediterranean Sea at ecological time scales. PLoS ONE 9:e110762. doi: 10.1371/journal.pone.0110762
Ganor, E., and Mamane, Y. (1982). Transport of Saharan dust across the eastern Mediterranean. Atmos. Environ. 16, 581–587. doi: 10.1016/0004-6981(82)90167-6
Ganor, E., Osetinsky, I., Stupp, A., and Alpert, P. (2010). Increasing trend of African dust, over 49 years, in the eastern Mediterranean. J. Geophys. Res. Atmos. 115, 1–7. doi: 10.1029/2009JD012500
Gat, D., Zeev, E., and Tsesarsky, M. (2016). Soil bacteria population dynamics following stimulation for ureolytic microbial-induced CaCO3 precipitation. Environ. Sci. Technol. 50, 616–624. doi: 10.1021/acs.est.5b04033
Gorbushina, A. A., Kort, R., Schulte, A., Lazarus, D., Schnetger, B., Brumsack, H. J., et al. (2007). Life in Darwin's dust: intercontinental transport and survival of microbes in the nineteenth century. Environ. Microbiol. 9, 2911–2922. doi: 10.1111/j.1462-2920.2007.01461.x
Goudie, A. S., and Middleton, N. J. (2001). Saharan dust storms: nature and consequences. Earth Sci. Rev. 56, 179–204. doi: 10.1016/S0012-8252(01)00067-8
Griffin, D. W. (2007). Atmospheric movement of microorganisms in clouds of desert dust and implications for human health. Clin. Microbiol. Rev. 20, 459–477. doi: 10.1128/CMR.00039-06
Guerzoni, S., Chester, R., Dulac, F., Herut, B., Loýe-Pilot, M. D., Measures, C., et al. (1999). The role of atmospheric deposition in the biogeochemistry of the Mediterranean Sea. Prog. Oceanogr. 44, 147–190. doi: 10.1016/S0079-6611(99)00024-5
Guieu, C., Aumont, O., Paytan, A., Bopp, L., Law, S. C., Mahowald, N., et al. (2014). Global biogeochemical cycles deposition to low nutrient low chlorophyll regions. Glob. Biogeochem. Cycles 28, 1179–1198. doi: 10.1002/2014GB004852
Herut, B., Almogi-Labin, A., and Jannink, N. (2000). The seasonal dynamics of nutrient and chlorophyll a concentrations on the SE Mediterranean shelf-slope. Oceanol. Acta 23, 771–782. doi: 10.1016/S0399-1784(00)01118-X
Herut, B., Kress, N., and Tibor, G. (2002). The use of hyper-spectral remote sensing in compliance monitoring of water quality (phytoplankton and suspended particles) at “hot spot” areas (Mediterranean coast of Israel). Fresenius Environ. Bull. 11, 782–787.
Herut, B., Krom, M. D., Pan, G., and Mortimer, R. (1999). Atmospheric input of nitrogen and phosphorus to the southeast Mediterranean: sources, fluxes, and possible impact, Limnology and Oceanography. 44, 1683–1692. doi: 10.4319/lo.1999.44.7.1683
Herut, B., Nimmo, M., Medway, A., Chester, R., and Krom, M. D. (2001). Dry atmospheric inputs of trace metals at the Mediterranean coast of Israel (SE Mediterranean): sources and fluxes. Atmos. Environ. 35, 803–813. doi: 10.1016/S1352-2310(00)00216-8
Herut, B., Zohary, T., Krom, M. D., Mantoura, R. F. C., Pitta, P., Psarra, S., et al. (2005). Response of East Mediterranean surface water to Saharan dust: on-board microcosm experiment and field observations. Deep Sea Res. Part II Top. Stud. Oceanogr. 52, 3024–3040. doi: 10.1016/j.dsr2.2005.09.003
Hervas, A., Camarero, L., Reche, I., and Casamayor, E. O. (2009). Viability and potential for immigration of airborne bacteria from Africa that reach high mountain lakes in Europe. Environ. Microbiol. 11, 1612–1623. doi: 10.1111/j.1462-2920.2009.01926.x
Hervas, A., and Casamayor, E. O. (2009). High similarity between bacterioneuston and airborne bacterial community compositions in a high mountain lake area. FEMS Microbiol. Ecol. 67, 219–228. doi: 10.1111/j.1574-6941.2008.00617.x
Huertas, M. J., López-Maury, L., Giner-Lamia, J., Sánchez-Riego, A. M., and Florencio, J. F. (2014). Metals in cyanobacteria: analysis of the copper, nickel, cobalt and arsenic homeostasis mechanisms. Life 4, 865–886. doi: 10.3390/life4040865
Katra, I., Arotsker, L., Krasnov, H., Zaritsky, A., Kushmaro, A., and Ben-Dov, E. (2014). Richness and diversity in dust stormborne biomes at the southeast Mediterranean. Sci. Rep. 4:5265. doi: 10.1038/srep05265
Kellogg, C. A., and Griffin, D. W. (2006). Aerobiology and the global transport of desert dust. Trends Ecol. Evol. 21, 638–644. doi: 10.1016/j.tree.2006.07.004
Kocak, M., Kubilay, N., Herut, B., and Nimmo, M. (2005). Dry atmospheric fluxes of trace metals (Al, Fe, Mn, Pb, Cd, Zn, Cu) over the Levantine Basin: a refined assessment. Atmos. Environ. 39, 7330–7341. doi: 10.1016/j.atmosenv.2005.09.010
Kress, N., Frede Thingstad, T., Pitta, P., Psarra, S., Tanaka, T., Zohary, T., et al. (2005). Effect of P and N addition to oligotrophic Eastern Mediterranean waters influenced by near-shore waters: a microcosm experiment. Deep Res. Part II Top. Stud. Oceanogr. 52, 3054–3073. doi: 10.1016/j.dsr2.2005.08.013
Kress, N., Gertman, I., and Herut, B. (2014). Temporal evolution of physical and chemical characteristics of the water column in the easternmost Levantine Basin (Eastern Mediterranean Sea) from 2002 to 2010. J. Mar. Syst. 135, 6–13. doi: 10.1016/j.jmarsys.2013.11.016
Kress, N., and Herut, B. (2001). Spatial and seasonal evolution of dissolved oxygen and nutrients in the southern Levantine Basin (Eastern Mediterranean Sea): chemical characterization of the water masses and inferences on the N:P ratios. Deep Sea Res. Part I Oceanogr. Res. Pap. 48, 2347–2372. doi: 10.1016/S0967-0637(01)00022-X
Krom, M. D., Cliff, R. A., Eijsink, L. M., Herut, B., and Chester, R. (1999). The characterisation of Saharan dusts and Nile particulate matter in surface sediments from the Levantine basin using Sr isotopes. Mar. Geol. 155, 319–330. doi: 10.1016/S0025-3227(98)00130-3
Lagaria, A., Psarra, S., Lefèvre, D., Van-Wambeke, F., Courties, C., Pujo-Pay, M., et al. (2011). The effects of nutrient additions on particulate and dissolved primary production in surface waters of three Mediterranean eddies. Biogeosciences 8, 2595–2607. doi: 10.5194/bg-8-2595-2011
Lang-Yona, N., Lehahn, Y., Herut, B., Burshtein, N., and Rudich, Y. (2014). Marine aerosol as a possible source for endotoxins in coastal areas. Sci. Total Environ. 499, 311–318. doi: 10.1016/j.scitotenv.2014.08.054
Lawrence, C. R., and Neff, J. C. (2009). The contemporary physical and chemical flux of aeolian dust: a synthesis of direct measurements of dust deposition. Chem. Geol. 267, 46–63. doi: 10.1016/j.chemgeo.2009.02.005
Mackey, K. R. M., Labiosa, R. G., Calhoun, M., Street, J. H., Post, A. F., and Paytan, A. (2007). Phosphorus availability, phytoplankton community dynamics, and taxon-specific phosphorus status in the Gulf of Aqaba, Red Sea. Limnol. Oceanogr. 52, 873–885. doi: 10.4319/lo.2007.52.2.0873
Mann, E. L., Ahlgren, N., Moffett, J. W., and Chisholm, S. W. (2002). Copper toxicity and cyanobacteria ecology in the Sargasso Sea. Limnol. Oceanogr. 47, 976–988. doi: 10.4319/lo.2002.47.4.0976
Marshall, W. A., and Chalmers, M. O. (1997). Airborne dispersal of antarctic terrestrial algae and cyanobacteria. Ecography 20, 585–594. doi: 10.1111/j.1600-0587.1997.tb00427.x
Massana, R., Murray, A. E., Preston, C. M., and Delong, E. (1997). Vertical distribution and phylogenetic characterization of marine planktonic archaea in the Santa Barbara Channel. Appl. Environ. Microbiol. 63, 50–56.
Mayol, E., Jiménez, M. A., Herndl, G. J., Duarte, C. M., and Arrieta, J. M. (2014). Resolving the abundance and air-sea fluxes of airborne microorganisms in the North Atlantic Ocean. Front. Microbiol. 5:557. doi: 10.3389/fmicb.2014.00557
Mills, M. M., Ridame, C., Davey, M., La-Roche, J., and Geider, R. (2004). Iron and phosphorus co-limit nitrogen fixation in the eastern tropical North Atlantic. Nature 429, 292–294. doi: 10.1038/nature02550
Paytan, A., Mackey, K. R. M., Chen, Y., Lima, I. D., Doney, S. C., and Mahowald, N. (2009). Toxicity of atmospheric aerosols on marine phytoplankton. Proc. Natl. Acad. Sci. U.S.A. 106, 4601–4605. doi: 10.1073/pnas.0811486106
Peter, H., Hörtnagl, P., Reche, I., and Sommaruga, R. (2014). Bacterial diversity and composition during rain events with and without Saharan dust influence reaching a high mountain lake in the Alps. Environ. Microbiol. Rep. 6, 618–624. doi: 10.1111/1758-2229.12175
Pitta, P., Nejstgaard, J. C., Tsagaraki, T. M., Zervoudaki, S., Egge, J. K., Frangoulis, C., et al. (2016). Confirming the “Rapid phosphorus transfer from microorganisms to mesozooplankton in the Eastern Mediterranean Sea” scenario through a mesocosm experiment. J. Plankton Res. 38, 502–521. doi: 10.1093/plankt/fbw010
Polymenakou, P. N. (2012). Atmosphere: a source of pathogenic or beneficial microbes? Atmosphere 3, 87–102. doi: 10.3390/atmos3010087
Polymenakou, P. N., Mandalakis, M., Stephanou, E. G., and Tselepides, A. (2008). Particle size distribution of airborne microorganisms and pathogens during an intense African dust event in the Eastern Mediterranean. Environ. Health Perspect. 116, 292–296. doi: 10.1289/ehp.10684
Prospero, J. M., Blades, E., Mathison, G., and Naidu, R. (2005). Interhemispheric transport of viable fungi and bacteria from Africa to the Caribbean with soil dust. Aerobiologia 21, 1–19. doi: 10.1007/s10453-004-5872-7
Pulido-Villena, E., Wagener, T., and Guieu, C. (2008). Bacterial response to dust pulses in the western Mediterranean: implications for carbon cycling in the oligotropic ocean. Glob. Biogeochem. Cycles 22, 1–12. doi: 10.1029/2007GB003091
Rahav, E., Giannetto, M., and Bar-Zeev, E. (2016b). Contribution of mono and polysaccharides to heterotrophic N2 fixation at the eastern Mediterranean coastline. Sci. Rep. 6:27858. doi: 10.1038/srep27858
Rahav, E., Ovadia, G., Paytan, A., and Herut, B. (2016a). Contribution of airborne microbes to bacterial production and N2 fixation in seawater upon aerosol deposition. Geophys. Res. Lett. 43, 1–9. doi: 10.1002/2015GL066898
Raveh, O., David, N., Rilov, G., and Rahav, E. (2015). The temporal dynamics of coastal phytoplankton and bacterioplankton in the Eastern Mediterranean Sea. PLoS ONE 10:e0140690. doi: 10.1371/journal.pone.0140690
Reche, I., Ortega-Retuerta, E., Romera, O., Pulido-Villena, E., Morales-Baquero, R., and Casamayor, E. O. (2009). Effect of Saharan dust inputs on bacterial activity and community composition in Mediterranean lakes and reservoirs. Limnol. Oceanogr. 54, 869–879. doi: 10.4319/lo.2009.54.3.0869
Redfield, A. C. (1934). “On the proportions of organic derivatives in sea water and their relation to the composition of plankton,” in James Johnstone Memorial Volume, ed R. J. Daniel (Liverpool: Liverpool University Press), 176–192.
Saeed, T., and Sun, G. (2012). A review on nitrogen and organics removal mechanisms in subsurface flow constructed wetlands: dependency on environmental parameters, operating conditions and supporting media. J. Environ. Manag. 112, 429–448. doi: 10.1016/j.jenvman.2012.08.011
Seifried, J. S., Wichels, A., and Gerdts, G. (2015). Spatial distribution of marine airborne bacterial communities. MicrobiologyOpen 25, 475–490. doi: 10.1002/mbo3.253
Sharoni, S., Trainic, M., Schatz, D., Lahahn, Y., Flores, M. J., Bidle, K. D., et al. (2015). Infection of phytoplankton by aerosolized marine viruses. Proc. Natl. Acad. Sci. U.S.A. 112, 6643–6647. doi: 10.1073/pnas.1423667112
Simon, M., Alldredge, A., and Azam, F. (1990). Bacterial carbon dynamics on marine snow. Mar. Ecol. Prog. Ser. 65, 205–211. doi: 10.3354/meps065205
Sohm, J. A., Webb, E. A., and Capone, D. G. (2011). Emerging patterns of marine nitrogen fixation. Nat. Rev. Microbiol. 9, 499–508. doi: 10.1038/nrmicro2594
Steemann-Nielsen, E. (1952). On the determination of the activity for measuring primary production. J. Cons. Int. Explor. Mer. 18, 117–140.
Sunda, W. G. (2012). Feedback interactions between trace metal nutrients and phytoplankton in the ocean. Front. Microbiol. 3:204. doi: 10.3389/fmicb.2012.00204
Ternon, E., Guieu, C., Ridame, C., L'Helguen, S., and Catala, P. (2011). Longitudinal variability of the biogeochemical role of Mediterranean aerosols in the Mediterranean Sea. Biogeosciences 8, 1067–1080. doi: 10.5194/bg-8-1067-2011
Thingstad, T. F., Krom, M. D., Mantoura, R. F. C., Flaten, G. A., Groom, S., Herut, B., et al. (2005). Nature of phosphorus limitation in the ultraoligotrophic eastern Mediterranean. Science 309, 1068–1071. doi: 10.1126/science.1112632
Vardi, A., Haramaty, L., Mooy, B. A. S., Van Fredricks, H. F., Kimmance, S. A., Larsen, A., et al. (2012). Host-virus dynamics and subcellular controls of cell fate in a natural coccolithophore population. Proc. Natl. Acad. Sci. U.S.A. 109, 19327–19332. doi: 10.1073/pnas.1208895109
Vaulot, D., and Marie, D. (1999). Diel variability of photosynthetic picoplankton in the equatorial Pacific. Appl. Environ. Microbiol. 104, 3297–3310. doi: 10.1029/98jc01333
Wang, X. J., Behrenfeld, M., Le-Borgne, R., Murtugudde, R., and Boss, E. (2009). Regulation of phytoplankton carbon to chlorophyll ratio by light, nutrients and temperature in the Equatorial Pacific Ocean : a basin-scale model. Biogeosciences 6, 391–404. doi: 10.5194/bg-6-391-2009
Welschmeyer, N. A. (1994). Fluorometric analysis of chlorophyll a in the presence of chlorophyll b and pheopigments. Limnol. Oceanogr. 39, 1985–1992. doi: 10.4319/lo.1994.39.8.1985
Keywords: dust storm, southeastern Mediterranean Sea, atmospheric dry deposition associated microbes, primary production, bacterial production
Citation: Rahav E, Paytan A, Chien C-T, Ovadia G, Katz T and Herut B (2016) The Impact of Atmospheric Dry Deposition Associated Microbes on the Southeastern Mediterranean Sea Surface Water following an Intense Dust Storm. Front. Mar. Sci. 3:127. doi: 10.3389/fmars.2016.00127
Received: 27 March 2016; Accepted: 07 July 2016;
Published: 21 July 2016.
Edited by:
Christos Dimitrios Arvanitidis, Hellenic Centre for Marine Research, GreeceReviewed by:
Christina Pavloudi, Hellenic Centre for Marine Research, Greece; University of Bremen, Germany; University of Ghent, BelgiumElena Tamburini, University of Cagliari, Italy
William J. Broughton, Bundesanstalt für Materialforschung und - prüfung (BAM), Germany
Copyright © 2016 Rahav, Paytan, Chien, Ovadia, Katz and Herut. This is an open-access article distributed under the terms of the Creative Commons Attribution License (CC BY). The use, distribution or reproduction in other forums is permitted, provided the original author(s) or licensor are credited and that the original publication in this journal is cited, in accordance with accepted academic practice. No use, distribution or reproduction is permitted which does not comply with these terms.
*Correspondence: Eyal Rahav, ZXlhbC5yYWhhdkBvY2Vhbi5vcmcuaWw=