- 1Hellenic Centre for Marine Research, Heraklion, Greece
- 2Institute of Estuarine and Coastal Studies, University of Hull, Hull, UK
- 3European Commission, Joint Research Centre (JRC), Directorate for Sustainable Resources, Ispra, Italy
- 4Marine Research Division, AZTI-Tecnalia, Pasaia, Spain
- 5School of Animal, Environmental and Rural Sciences, Nottingham Trent University, Southwell, UK
Conceptual models summarize, visualize and explain actual or predicted situations and how they might be tackled. In recent years, Pressure-State-Response (P-S-R) frameworks have been central to conceptualizing marine ecosystem issues and then translating those to stakeholders, environmental managers and researchers. Society is concerned about the risks to the natural and human system posed by those Pressures (thus needing risk assessment) and then needs to act to minimize or compensate those risks (as risk management). This research relates this to the DPSIR (Drivers-Pressure-State(change)-Impact-Response) hierarchical framework using standardized terminology/definitions and lists of impacting Activities and Pressures affecting ecosystem components, incorporating the European Marine Strategy Framework Directive (MSFD) legal decision components. This uses the example of fishing activity and the pressure of abrasion from trawling on the seabed and its effects on particular ecosystem components. The mechanisms of Pressure acting on State changes are highlighted here as an additional refinement to DPSIR. The approach moves from conceptual models to actual assessments including: assessment methodologies (interactive matrices, ecosystem modeling, Bayesian Belief Networks, Bow-tie approach, some assessment tools) data availability, confidence, scaling, cumulative effects and multiple simultaneous Pressures, which more often occur in multi-use and multi-user areas. In defining and describing the DPSIR Conceptual Framework we consider its use in real-world ecosystems affected by multiple pressures or multiple mechanisms of single pressures, and show how it facilitates management and assessment issues with particular relevance to the MSFD.
Introduction
Determining the cause and consequence of marine environmental problems entails risk assessment, and the responses entail risk management (Cormier et al., 2013). Conceptual models help to summarize, explain and address the identified risk by deconstructing each aspect being assessed, prioritized and addressed (Elliott, 2002). In risk management, these models communicate relevant knowledge to managers and developers as well as having an educational value (Mylopoulos, 1992), to increase awareness of the environmental risks through ocean literacy (Uyarra and Borja, 2016). This enables the development of quantitative and numerical models, hypothesis generation or for indicating the limitation of such models and the available scientific knowledge (Elliott, 2002).
Conceptual models are simple to complex diagrams which collate and summarize relevant information and so by their nature they may become increasingly complex, hence the term “horrendograms” (Elliott, 2002), but they are the pre-requisite for all numerical models.
A key current conceptual framework in widespread use, the Driver-Pressure-State-Impact-Response (DPSIR) framework (OECD, 1993), has developed over the last few decades and is used as the basis for many conceptual approaches addressing Pressure-State change links (Elliott, 2014; Gari et al., 2015). It structures and standardizes conceptualizing complex issues although at present it provides an overly simplistic representation of the relationship between Pressures and State changes, merely indicating that Pressure leads to State change (which may not necessarily be the case). It takes no account of the interaction between different Activities and their associated Pressures occurring simultaneously (Gari et al., 2015). Furthermore, it does not highlight the difference in the nature, severity, timescale or longevity of State changes in relation to pressure intensity, frequency or duration.
Today the DPSIR framework has produced many derivatives and refinements (e.g., Gari et al., 2015; Lewison et al., 2016) with the most extensive review undertaken by Patrício et al. (2016), covering some 152 studies and 27 major projects based around DPSIR, noting more than 23 derivative acronyms, with one further derivative recently being published (DAPSI(W)R(M)—Wolanski and Elliott (2015) and Scharin et al. (2016)). In this manuscript we use the terminology of the “DPSIR framework” rather than any one specific derivative, with emphasis on defining and clarifying components.
An improved understanding of the interactions between Drivers, Pressures and States (or, more particularly, the Pressure-State change (P-S) linkage) is important to help consider possible risk management responses. Pressures are the mechanisms that lead to State changes (and Impacts on human welfare). Hence a Pressure may be analogous to hazard as the cause of risk to an element. In turn, the risk is the probability of effect (likely consequences) causing a disaster or assets affected by the hazard (as human consequences) (Elliott et al., 2014). Smith and Petley (2009) consider that hazard, as a cause, and risk, as a likely consequence, relate especially to humans and their welfare. In the discussion here, the consequence may be regarded as relating to the Impact (on human Welfare) part of the DPSIR cycle (Cooper, 2013). Therefore, we can emphasize the links between the DPSIR approach and risk assessment and risk management.
European Union (EU) Member States must ensure no significant risks to, or impacts on marine biodiversity, marine ecosystems, human health or legitimate uses of the sea. This is enshrined in the Marine Strategy Framework Directive (MSFD; 2008/56/EC), an ambitious legislative instrument for the EU and indeed global marine environmental management which extends control of EU seas out to 200 nm (EC, 2008). Boyes and Elliott (2014) show its importance linking with other holistic and EU framework directives such as the Water Framework Directive (2000/60/EC), Habitats Directive (92/43/EEC), and the Maritime Spatial Planning Directive (2014/89/EU). The MSFD links the causes of marine environmental changes, human Activities and Pressures to their consequences leading to controlling and managing those causes and consequences. If successful, it will protect the natural system while also allowing the seas to produce ecosystem services and deliver societal benefits (Borja et al., 2013). The MSFD focuses on the assessment and monitoring of the functioning of marine ecosystems rather than just its structure. It aims to achieve Good Environmental Status (GES) by 2020 to ensure marine-related economic and social activities and via a roadmap for each Member State to develop an iterative strategy for its marine waters including assessments, determination of GES, establishment targets, indicators and monitoring with a programme of measures to achieve or maintain GES (EC, 2008, 2010; CSWP, 2011; CSWD, 2014). This structured approach allows each EU Member State to ensure there are no significant risks to marine ecosystems, human health or legitimate uses of the sea. Three Member States (Estonia, Denmark and Greece) used DPSIR in their MSFD initial assessments (CSWD, 2014), primarily in their socio-economic analyses.
This review focuses on the relevance of the DPSIR framework to the MSFD to organize and focus assessments in real marine situations including the linkages between multiple Activities exerting multiple Pressures and leading to State changes through multiple mechanisms (i.e., beyond simplistic single DPSIR chains). This ensures the DPSIR approach becomes more usable and a first choice starting approach to addressing marine issues. We standardize the approach incorporating ecosystem characteristics/components to allow ease of use in marine assessments, the movement from concepts to assessments and different assessment methodologies.
The DPSIR Framework
Rapport and Friend (1979) proposed the first Pressure-State-Response (PSR) framework which was then promoted by the Organization for Economic Cooperation and Development (OECD, 1993) for its environmental performance monitoring. This framework assumes causality that human Activities exert Pressures on the environment (marine and terrestrial), which can induce changes in the State/quality of natural resources. Society addresses these changes through environmental, governance, economic and sectoral responses (policies and programmes). Highlighting the cause-effect relationships can help decision makers and the public see how those issues are interconnected. The OECD (1993) re-evaluated the PSR model, whilst initiating work with environmental indicators. Its use has been extended widely and with many iterations (Patrício et al., 2016). The US Environmental Protection Agency (EPA, 1994) extended it to include the effects of changes in State on the environment (Pressure-State-Response/effects), UNEP (1994) further developed the Pressure-State-Impact-Response (PSIR) framework and the UN Commission on Sustainable Development proposed the Driving Force-State-Response framework (DSR). Here, Driving force replaced the term Pressure in order to accommodate more accurately the addition of social, economic and institutional indicators. Through agencies such as the European Environmental Agency and EUROSTAT, the EU adopted the Driving Force-Pressure-State-Impact-Response framework (DPSIR), as an overall mechanism for analyzing environmental problems (EC, 1999). The EU scheme (Figure 1A) shows that Driving forces (e.g., basic economic sectors) exert Pressures (e.g., carbon dioxide emissions), leading to changes in the State of the environment (e.g., changes in the physico-chemical and biological systems, nutrients, organic matter, etc.), which then lead to Impacts on humans and ecosystems (e.g., decreased fish production) that may in turn require a societal Response (e.g., research, building water treatment plants, energy taxes). The Response can feed back to Driving forces, Pressures, State or Impacts directly through adaptation or remedial action (e.g., policies, legislation, restrictions, etc.).
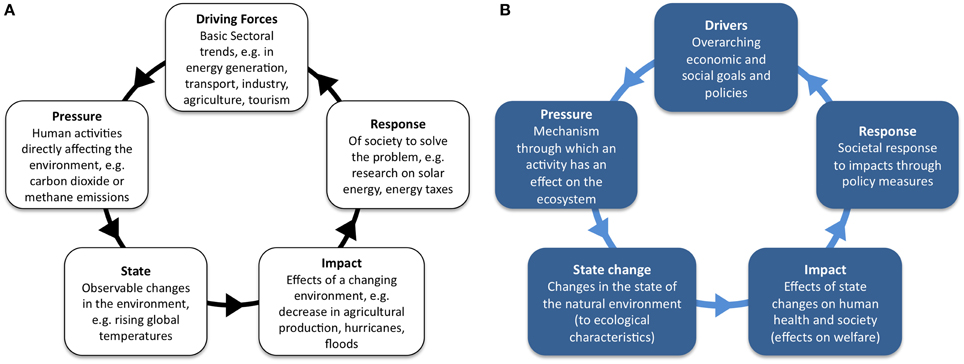
Figure 1. Driving forces-Pressure-State-Impact-Response evolution. (A) DPSIR redrawn from the original EU framework (EC, 1999). (B) DPSIR as used by the authors. Reproduction is authorised provided the source is acknowledged.
Interpretation of DPSIR has been variable and there has been the need to clarify terms which are often defined/used differently by natural and social scientists. For example, where either:
• State is the State of the Environment and Impacts are physical/chemical/biological changes to the state of the environment—natural science perspective, or
• State is State change (of the environment) and Impacts are the effects on human society and welfare—social science perspective
This lack of clarity has mostly led to further re-definition of one element of the model for example DPSWR where Impact has been replaced/clarified with Welfare (Cooper, 2013) or taking this further to DAPSI(W)R(M) [Driver-Activity-Pressure-State change-Impacts (on Welfare)-Responses (through Measures), (Wolanski and Elliott, 2015; Scharin et al., 2016)]. A clearer terminology (Figure 1B), is based on Borja et al. (2006), Robinson et al. (2008), and Atkins et al. (2011), for the DPSIR framework in natural ecosystems:
• Drivers: at the highest level, “Driving Forces” are the overarching economic and social policies of governments, and economic and social goals of those involved in industry. At a mid-level they may be considered to be Sectors in industry (e.g., fishing) and at a lower level, Activities in the Sector (e.g., demersal trawling).
• Pressure is considered as the mechanism through which an Activity has an actual or potential effect on any part of the ecosystem (e.g., for demersal trawling Activity, one Pressure would be abrasion to the seabed).
• State change refers to changes in the “State” of the natural environment which is effected by Pressures which cause State changes to ecological characteristics (environmental variables, habitats, species/groups structural or functional diversity) (e.g., abrasion may cause a decrease in macrofaunal diversity)
• Impacts are the effect of State changes on human health and society, sometimes referred to as Welfare, change in Welfare is affected by changes in use values and in non-use values (e.g., loss of goods and services from loss of biodiversity).
• Response is the societal response to Impacts through various policy measures, such as regulations, information, behavior change (e.g., ocean literacy), and taxes; these can be directed at any other part of the system (e.g., reduction in the number of bottom trawler licenses, the change to a less abrasive gear, or creation of no-fishing areas).
DPSIR Cycles
Whilst a single DPSIR model or cycle (Figure 1B) greatly over-simplifies the “real world,” it can conceptualize the relationships between environmental change, anthropogenic pressures and management options. However, to be of value, the model does need to be bounded (e.g., Svarstad et al., 2008), for example, by defining its spatial limits (usually the management unit such as a particular area of sea or length of coast). Furthermore, while a simple DPSIR cycle relates to the Activity or Sector to which it applies, the marine environment is a complex adaptive system (Gibbs and Cole, 2008) with areas subject to several Drivers. Accordingly, this requires to be visualized as several interlinked DPSIR cycles (each representing different interacting Activities or Sectors which compete for the available resources). Atkins et al. (2011) linked separate systems by the Response element, arguing that the effective management of anthropogenic impacts requires integrated actions (involving many types of response) affecting all relevant Activities; in contrast, Scharin et al. (2016) linked DAPSI(W)R(M) in similar cycles around State changes. Separate DPSIR cycles, each relating to a different Activity, can also be linked by Pressures and reflect the concept that several different Activities can create the same environmental pressure (Figure 2A). Following Atkins et al. (2011), Figure 2A illustrates how a single Pressure (the central blue circle) provides a common link between five separate DPSIR cycles, which represent five separate Activities. For clarity, the links within each individual DPSIR cycle have been simplified (e.g., by omitting the direct R-P link within each cycle and the links between other D, S, I, and R elements for different cycles a la Atkins et al., 2011). Linking separate DPSIR cycles in this way, and placing Pressure at the heart of the model, focuses attention on the Pressure as the system element that needs to be managed, thus supporting the assessment of Pressure-State change linkages. Hence, any such single Pressure may bring about a State change across a number of different ecological components. In essence, we assess State changes and Impacts but we manage the Drivers, the Activities and the Pressures, and in some cases State changes. Having a series of nested and linked DPSIR cycles, and linking these across ecosystems, accommodates many Pressures within one area (Atkins et al., 2011). Thus, a nested DPSIR cycle in a near-shore area, for example, has to link with those in the catchments, estuaries and at sea. This overcomes some of the difficulties in applying the framework to dynamic systems, cause-consequence relationships, multiple Drivers and only linear unidirectional causal chains.
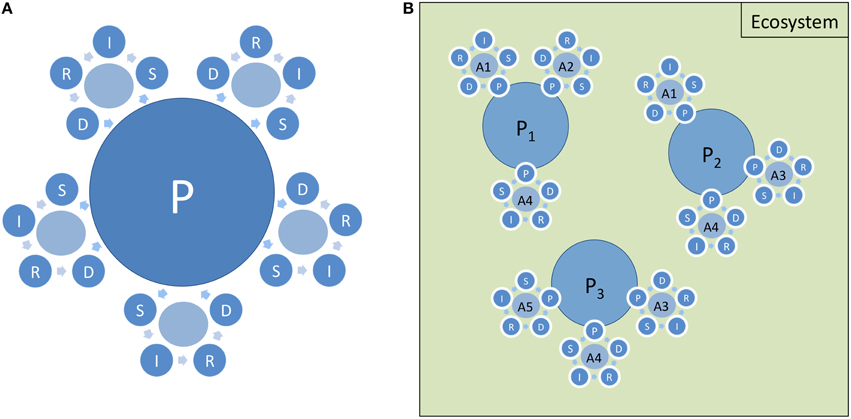
Figure 2. Multispace DPSIR cycles. (A) Separate DPSIR cycles linked through a common Pressure element (e.g., abrasion pressure from the activities of benthic trawling, anchoring, dredging, etc.). (B) Example of linked DPSIR cycles in a particular ecosystem with individual separate Pressures (P1-P3), each associated with discrete Activity types (A1-A4). It is important to acknowledge that, just as the same Pressure may be generated by more than one Activity, so the same Activity may give rise to a number of different Pressures. For example, (P1) might represent abrasion, and may link DPSIR cycles relating to three Activities—benthic trawling (A1), anchoring (A2), and dredging (A3); (P2) might represent marine litter, linking benthic trawling (A1), the development of both non-renewable energy facilities (A4) and renewable energy facilities (A5); whilst (P3) might represent substratum loss, linking DPSIR cycles relating to the development of both non-renewable energy facilities (A4), renewable energy facilities (A5), and dredging (A3). For simplicity and clarity, Responses are shown here as having limited, within-cycle effects. In practice however, Responses within one DPSIR cycle may affect one or more of those other DPSIR cycles that are linked by a common Pressure or, indeed, DPSIR cycles that are not directly linked.
It is necessary for the framework to accommodate multiple pressures and state changes which can lead to cumulative, synergistic or antagonistic impacts (Nõges et al., 2016; Teichert et al., 2016; Figure 2A). For example, the different cycles in Figure 2B representing different Pressures or classes of Pressure, P1, P2, and P3 acting on an ecosystem [for example, (P1) might represent abrasion, and may link DPSIR cycles for three Activities—benthic trawling (A1), anchoring (A2), and dredging (A3); (P2) might represent marine litter, linking benthic trawling (A1), the development of non-renewable energy facilities (A4) and renewable energy facilities (A5); whilst (P3) might represent substratum loss, linking DPSIR cycles relating to the development of non-renewable energy facilities (A4), renewable energy facilities (A5), and dredging (A3)]. Hence there are many links between DPSIR chains across the different levels; for example, where the Responses and Drivers for one Activity interact with or affect the Responses and Drivers for a different Activity.
DPS Chains in the MSFD
The MSFD lists indicative characteristics, pressures and impacts to be taken into account during assessments (EC, 2008). There is some ambiguity in terms where the Directive presents “pressures” and “impacts” together, when pressures (P, Pressures in the DPSIR framework) should be distinguished from Activities, and Pressures should be distinguished from adverse effects on the natural system (i.e., S, State changes in the DPSIR framework). These lists have evolved since first publication, for example, in DIKE (2011) and CSWP (2011), but some ambiguities still remain.
Activities
In addition to clarifying the terminology, we also advocate alternative tables that list Activities and Pressures based on the work of a number of MSFD-related EU funded projects, particularly ODEMM (https://www.liverpool.ac.uk/odemm), VECTORS (www.marine-vectors.eu) and DEVOTES (www.devotes-project.eu). A list of possible and/or existing Activities is needed from which a subset can be extracted that may contribute to a greater number and/or more detrimental pressures for risk assessment and risk management and used to fulfill programmes for monitoring and response measures. Table 1 shows a complete Activities list contributing to Pressures, refined from the ODEMM project (White et al., 2013) where Activities had been separated into Sector and sub-sectors. To avoid duplication with either Driver or Activity, we consider that the term “Sector” is unnecessary, meaning that only an Activity is required to produce Pressures. Overall 13 major Activities characterize the wide range of sea uses.
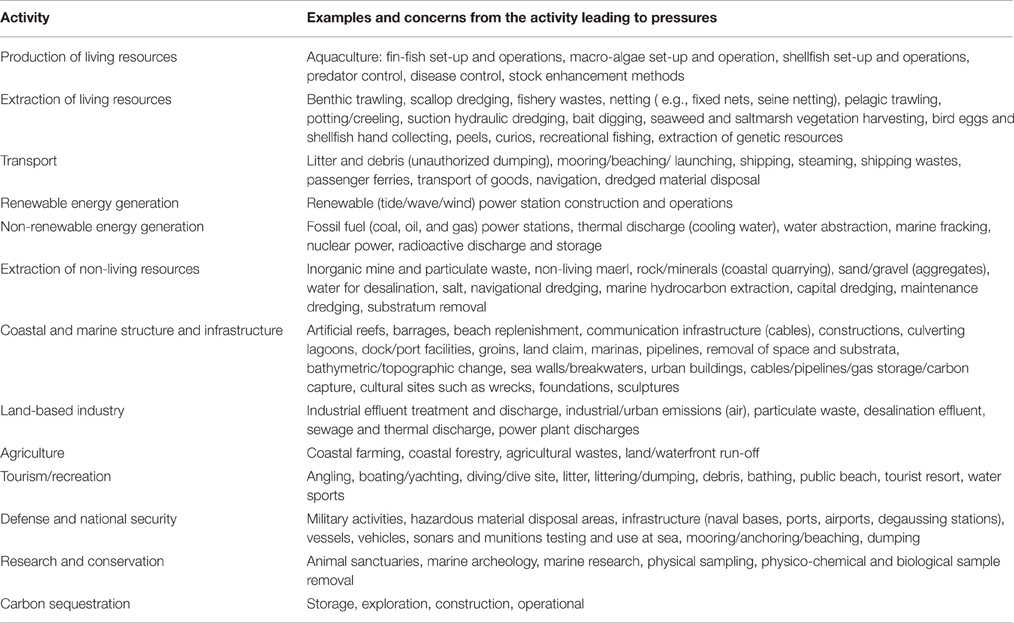
Table 1. Activities contributing to Pressures (modified extensively from White et al., 2013).
Pressures
The MSFD Pressures list (EC, 2008) identifies eight Pressure themes with 18 individual Pressures or mechanisms. Robinson et al. (2008) listed further Pressures, which were later updated by White et al. (2013). Except for Pressures from climate change, Pressures predominantly relate to anthropogenic Activity, also referred to as endogenic managed Pressures (Atkins et al., 2011; Elliott, 2011; Elliott et al., 2014), i.e., emanating from within the system to be managed. Exogenic unmanaged Pressures, in contrast, are from outside of the system and mostly relate to climate change, isostatic/eustatic change, or seismic activity. Elliott (2011) emphasizes that whereas the causes and consequences of endogenic managed Pressures are addressed within a management scheme for a marine area, only the consequences (as opposed to the causes) of exogenic unmanaged Pressures can be addressed at management scales; for example, the consequences of climate change can be addressed locally whereas the causes require global action.
As the MSFD only refers to an incomplete list of endogenic Pressures, we have revised both the MSFD and the White et al. (2013) lists to give 26 managed Pressures of which 18 were listed in the Directive (Table 2) and 7 are unmanaged Pressures (Table 3). The unmanaged Pressures allow climate change to be considered as it has been omitted in MSFD implementation and barely mentioned in the Directive (Elliott et al., 2015). The latter concluded that shifting baselines, resulting from climate change, need to be accommodated and revised during monitoring, environmental status assessment and in management actions (i.e., programmes of measures). The spatial and temporal variation in the response of the various biological components to climate change needs to be understood, as well as their ability (or lack of it) to adapt and reach equilibrium. Climate change may also exacerbate other Pressures and changes in the Descriptors (11 broad qualitative environmental descriptors for which GES must be assessed) for example the movement of non-indigenous species by increased shipping, but these effects may be indistinguishable from those arising from other anthropogenic Activities. Long-term, spatially extensive data sets will be needed to identify changes in ecological indicators. Although such data sets are not widely available for all Pressures, some efforts have been made to solve this gap. For example, for non-indigenous species, several databases hosting and sharing such information have been gathered in the European Alien Species Information Network (EASIN, http://easin.jrc.ec.europa.eu/) (Katsanevakis et al., 2015).
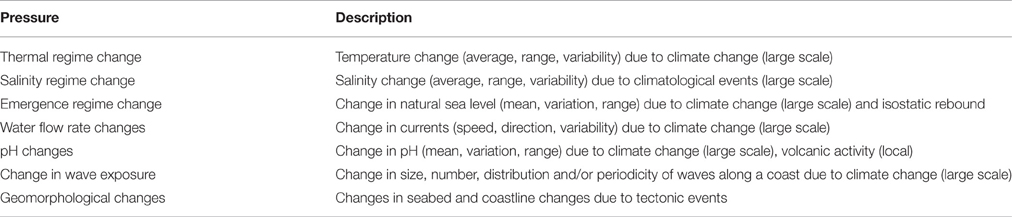
Table 3. Exogenic unmanaged Pressures in the marine environment (none originally or currently listed in the Marine Strategy Framework Directive).
Using the DPS
As a major example of the complexity of interactions we consider just one Activity, extraction of living resources from benthic trawling and its multiple individual Pressures affecting the seafloor environment (see Blaber et al., 2000, and conceptual models in Gray and Elliott, 2009). In terms of Pressures, benthic trawling targets and results in the selective extraction of species but also brings about the non-selective extraction of other living resources and causes abrasion, scouring and turning over the sediment as well as causing compaction and other changes in the seabed. Fishing vessels can also input various objects/elements into the marine environment (e.g., noise, synthetic compounds, non-synthetic compounds, other substances, litter), and cause death by collision. Benthic trawling includes some 12 individual primary and lesser Pressures (Table 2) each with differing effects.
In turn, the trawling Pressures may be site-specific, acting on specific habitats and ecosystems; Table 4 shows the European Commission MSFD-provided ecosystem components, with the first part highlighting habitats potentially impacted by benthic trawling—predominantly shallow to shelf sublittoral sedimentary habitats. The habitats in turn define and link with the potential biological components present (e.g., shallow sublittoral muddy sand supporting seagrass).
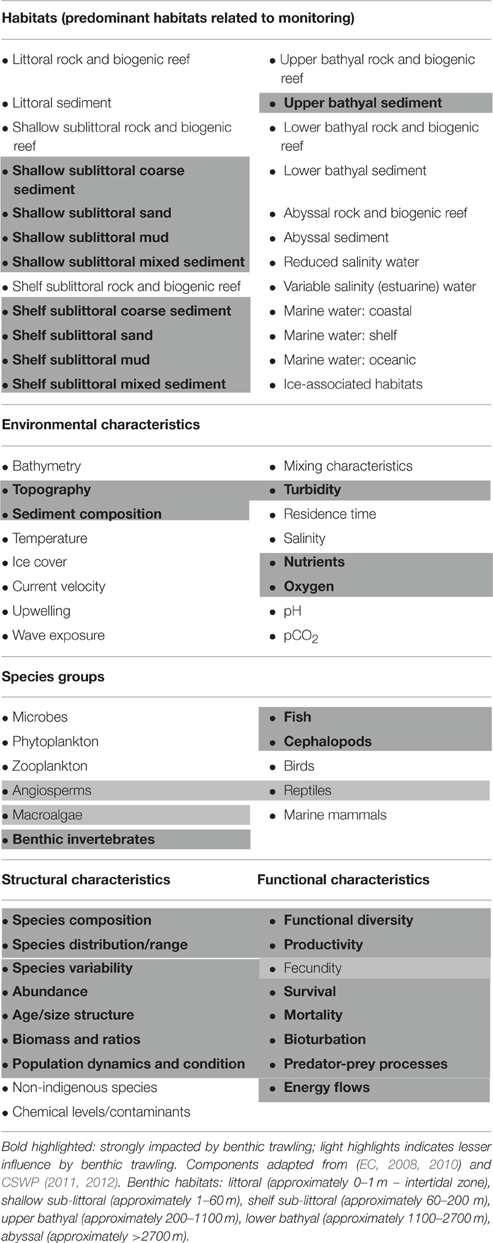
Table 4. Marine Strategy Framework Directive (MSFD) components, highlighted for those impacted by benthic trawling.
Within any one habitat, the different Pressures may affect several environmental characteristics (Table 4, highlighted) which also define/affect the niches of species groups (Table 4, highlighted) such that following a Pressure, the environmental characteristics may no longer be suitable for that species group. Each of those species groups has structural and functional characteristics (Table 4, highlighted) that may be affected to various extents. Although most of the effects that have been highlighted are direct, there are indirect effects for example through damage or habitat modification or changes to predator-prey relationships.
The situation is further complicated as different Pressure levels create different State change trajectories; for example, a Pressure causing large scale direct mortality will immediately reduce species, abundance, biomass, diversity, community structure, etc., and the duration of this depends on the nature of the habitat and its recovery potential (Duarte et al., 2015). The degree of Pressure then determines the severity and timescale of wider effects (e.g., at higher trophic levels) or on individuals (e.g., crushing, loss or damaged limbs or shells through collision with fishing gear) so that energy is allocated to individual recovery rather than growth/reproduction etc. In the long term, biomass, some components of population and community may be compromised with wider effects at the ecosystem level.
Refining DPSIR Pressure-State Change Relationships
Whilst it is well understood that Pressures on environmental systems can result in varying degrees of State change causing, for example, a loss of biodiversity and ecosystem services, the process by which those Impacts occur is complex. For a single, specific Pressure, the relationship between Pressure and Impact varies according to the degree of Pressure (e.g., spatial extent, duration and/or frequency, intensity), the habitat type upon which the Pressure is acting, the component species and those species in the wider ecosystem which they support. This produces many potential Pressure-State change trajectories that increase in complexity with concurrent potentially synergistic or antagonistic combinations of Activities and Pressures (Griffen et al., 2016). Hence the need to move from a conceptual framework to “nested horrendograms” to encompass the interlinked complexity (e.g., Elliott et al., 2015). Thus, generic processes leading to Impacts for a selection of Activities, Pressures, habitat types and biological components, then require specific, detailed trajectories that are site/system specific and specific to the nature of the Activities and their associated Pressures.
Current attempts to link Pressure with State change assume Pressure to act as a single mechanism leading to State change (Knights et al., 2011; Robinson and Knights, 2011; White et al., 2013). Hence, Pressure is the cause of physico-chemical and biological State changes which, through lethal or sub-lethal processes, compromise the performance or survival of one or more level of biological organization (cell, individual, population, community) (see Figure 3, overall organization). For example, the physical environment may be unsuitable to support the existing biological community, thus changing species composition and relative abundance (O'Neill and Ivanović, 2016).
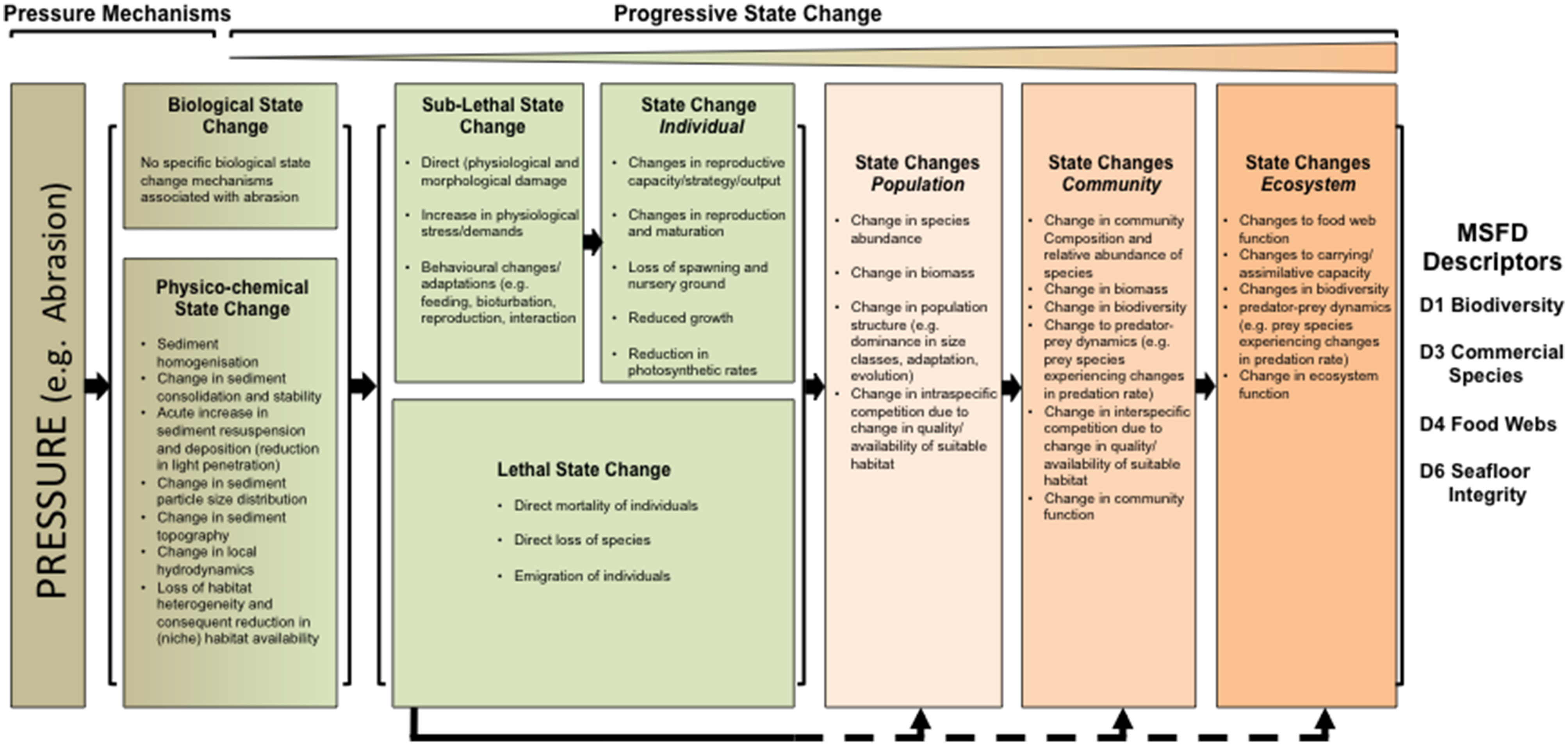
Figure 3. Conceptual model showing the progression of physico-chemical and biological State changes arising from Pressures in the marine environment. The black arrows under the diagram indicate the way in which Pressure can cause a biological State change at any level: either (1) progressively through a sub-lethal response at the individual level which, over time, can lead to State changes at higher levels or (2) directly by acting at a higher level, leading to more immediate community and ecosystem State changes. Example details are given for the Pressure of abrasion from benthic trawling in a subtidal sedimentary habitat and links to the MSFD descriptors (e.g., through physico-chemical, structural or functional indicators at different levels from individual to ecosystem for descriptors D1 biological diversity, D3 commercial fish species, D4 food webs and D6 seafloor integrity).
Achieving State change can be a progressive process and whilst changes to the physico-chemical and biological structure may be classed as State changes, paradoxically they may also be viewed as the mechanisms through which a Pressure acts to cause a biological State change (i.e., not mutually exclusive as the DPSIR model suggests). For example, a substratum change during an Activity is a physico-chemical State change and at the same time is a mechanism (and hence a Pressure) resulting in a biological State change in the benthos (see examples on trawling impacts in Clark et al., 2016). Hence, whilst most Pressures are associated with physical State changes (e.g., hydrodynamic changes, substratum changes), the direct removal of species, the introduction of non-indigenous species and the input of microbial contaminants represent biological mechanisms of change.
These physico-chemical and biological modifications to the environment lead to a series of biological State changes, which can occur at any level of biological organization (Solan and Whiteley, 2016). Responses may be lethal (referring to loss) as a result of direct mortality associated with the Pressure, direct removal (e.g., by fishing gear) or emigration, or sublethal. Lethal responses can have immediate, direct effects on an individual, population and community (and ultimately ecosystem) in terms of the species composition, their relative abundance and biomass, total population and community biomass, trophic interactions and other functional attributes such as primary and secondary production and biogeochemical cycling. Sublethal responses relate to physical, chemical or biological damage caused by the Pressure at an individual level, whereby the organism survives but its performance and, therefore, contribution to ecosystem processes is compromised. Hence, biological State changes to the lower levels of organization (individual, population) will, if unchecked, lead to higher level (community, ecosystem) changes (Borja et al., 2015). The ultimate degree of State change at a community or ecosystem level associated with lethal and sub-lethal mechanisms of State change may be broadly similar but their severity, extent and duration will differ (Amiard-Triquet et al., 2015).
Despite this, the inherent variability and complexity throughout the levels of biological organization may mean that an effect at a lower level does not necessarily manifest itself at higher levels, i.e., stressors at lower levels (e.g., cellular, individual) may get absorbed so that the higher levels (e.g., population, community, ecosystem) do not show any deleterious ecological effects. The ability to absorb that stress has been termed environmental homeostasis (Elliott and Quintino, 2007).
The severity and sequence of biological State changes will vary according to:
• type and degree of Pressure (spatial extent, intensity, duration, frequency) and whether it leads to lethal or sub-lethal effects;
• habitat sensitivity and the potential for disturbance and recovery of the physical attributes;
• sensitivity of the component species and communities and their recovery potential (resilience);
• sensitivity of the balance of interactions within and between habitats and biological components.
Using abrasion from benthic trawling as a specific worked example (Figure 3, fine detail), and assuming a sublittoral sedimentary (mud/sand) habitat, there are several physical State changes that may arise and which may, in turn, lead to a series of biological State changes (O'Neill and Ivanović, 2016).
The physical State changes associated with abrasion can be divided into those that cause immediate biological State change at higher biological levels (population/community/ecosystem), for example, by direct mortality, and those that cause a progressive State change over an extended time period (Eigaard et al., 2016; O'Neill and Ivanović, 2016). This leads to two different trajectories of State change (lethal and sub-lethal), which act over different timescales and may ultimately differ in severity and longevity (Gilkinson et al., 2005) or require a different intensity of stressor.
With respect to sub-lethal effects, “abrasion” can lead to various sedimentary changes (Figure 3, Physico-chemical State Change box). Since the benthic inhabitants are intimately linked to the substratum (Snelgrove and Butman, 1994), such changes, if of sufficient severity or duration, will physically impair biological community structure and its long term survival, larval settlement and recruitment (Alexander et al., 1993). Similarly, the removal of species will affect a feedback loop whereby the organisms modify the sedimentary conditions through bioturbation, bioengineering, biodeposition, etc. (e.g., Gray and Elliott, 2009). Additionally, those organisms that are more mobile may simply relocate to other areas. Whilst sedimentary changes can lead to species loss, it also presents opportunities for colonization by new species leading to an overall change in community structure. Coupled with this may be a change in community function, if species are replaced by functionally different species (Koutsidi et al., 2016). Abundance, biomass and secondary production would be influenced (and perhaps species richness and diversity), which may impact on wider ecosystem processes (Hiddink et al., 2006; Queiros et al., 2006). Whilst this impact would be more gradual than in the second (lethal effects) scenario, and may be partly counteracted by colonization by new species, overall community structure and function may nevertheless be altered.
Additionally, sub-lethal effects may arise through (for example) morphological damage (caused by interaction with fishing gear) and the associated physiological stress, changes in the physico-chemical parameters of the water column (e.g., dissolved oxygen, suspended solids), clogging of respiratory structures, inability to feed or burrow and behavioral modifications (Tillin et al., 2006). Subsequently, somatic growth and reproductive capacity may be compromised as a result of, for example, increased respiration rate, increased ammonia production in response to stress, re-allocation of resources to survival and recovery (e.g., Widdows et al., 1981) or evolutionary adaptations that enable accelerated maturation and early reproduction at the expense of ultimate body size (Mollet et al., 2007; Elliott et al., 2012). These effects may initially be apparent at the individual or population level but, if sustained, will ultimately change abundance, biomass and function at community and ecosystem levels (Thrush et al., 2016).
Lethal effects will create immediate State changes at the population and community level, including biomass and abundance declines in both target and non-target species (Hiddink et al., 2006; Koutsidi et al., 2016). In the longer term, and particularly with frequent benthic trawling, a sustained reduction in species richness and diversity may occur, coupled with changes to community structure and function (Bremner et al., 2003). Population structure in disturbed habitats may also be altered, particularly in longer-lived species, as certain size classes are selectively removed or where species of a more opportunistic nature allocate resources to reproductive output rather than somatic production resulting in a population dominated by small and or/young individuals. Ultimately, these State changes will reduce secondary production which, coupled with altered predator-prey interactions, will alter higher ecosystem processes (Thrush et al., 2016).
In terms of timescale, and regarding the ability of MSFD indicators to detect State change, such sub-lethal population and community level changes are likely to be relatively acute (and rapidly detectable) processes. The duration would depend on the sensitivity of the species and habitats, their resilience (or their potential to recover to an alternative state which supports wider ecosystem processes) and the intensity of the Pressure (or causative Activity). It also depends on the processes in the first (sub-lethal) scenario, since the two do not occur in isolation, whereby physical and biological changes to the environment will influence recovery rates and trajectories (Foden et al., 2010; Lambert et al., 2014).
The above changes in these scenarios (lethal and sub-lethal) have the potential to ultimately produce overall negative effects at higher trophic levels and wider ecosystem processes. The difference between the scenarios lies in the complexity/detail trajectory between the application of a Pressure and the resultant State change. Finally, the effects of trawling can result in human welfare being affected through the reduction in the provision of ecosystem services (Muntadas et al., 2015) and societal benefits (Atkins et al., 2011). The resulting changes compromise the performance or survival of an ecological component and so may bring about State change detected by MSFD descriptors [e.g., at the population, community or ecosystem level for descriptors D1 (biological diversity), D3 (commercial fish species), D4 (food webs) and D6 (seafloor integrity)].
Whilst the scenario above relates only to a single Pressure, abrasion, this Pressure may potentially arise as the result of a number of different Activities (Table 5).
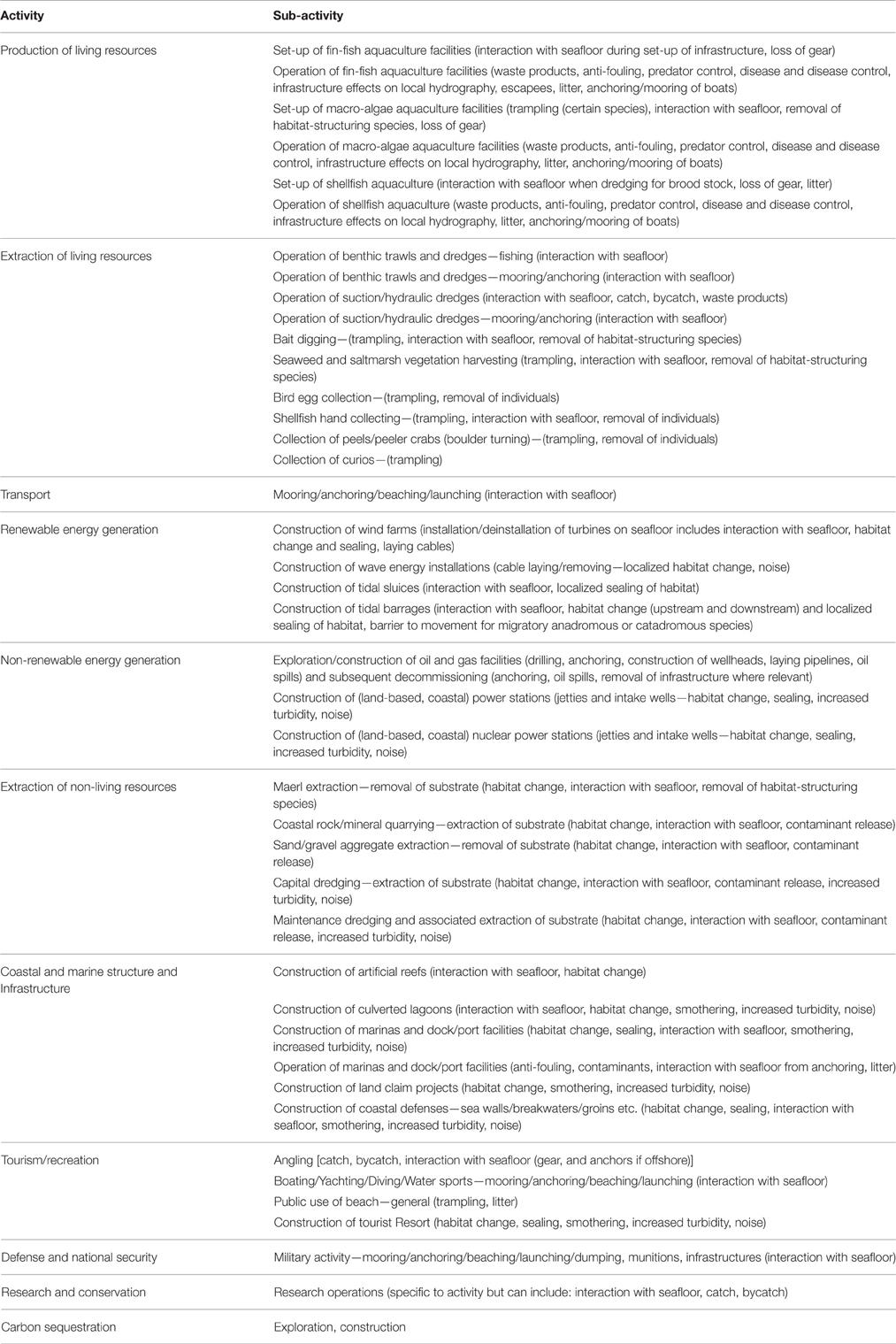
Table 5. Activities (related to Table 1) that may give rise to abrasion Pressures on the seabed.
Issues in Moving from Concepts to Assessments
Environmental management issues involve many challenges in moving from a conceptual framework to a data-based or expert judgment-based assessment. This involves identifying all the components and their linkages (e.g., D-P-S chains), and data/indicators and their quality or thresholds, etc.
Regional Seas
The European regional seas cover approximately 11,220,000 km2 (EEA, 2014) with a wide range of environmental conditions and different ecosystems, which vary in diversity and sensitivity. This affects the repercussions of human Activities and their resultant Pressures. Pressures in one regional area may not have the same footprint (type, extent, or duration) in another area because of differing conditions (see examples in the Baltic Andersen et al., 2015, Mediterranean Claudet and Fraschetti, 2010, and Black Seas Micheli et al., 2013). For example, the Mediterranean Sea is characterized by high salinity, high temperature, predominantly wind-driven or water mass difference-driven currents, deep water, oligotrophic conditions with a fauna exhibiting low abundance and biomass. In contrast northern waters have opposing characteristics where, for example, tidally-driven mixing may create a different footprint of a Pressure (Andersen et al., 2013). The regional seas also have contrasting developmental and socio-economic issues producing complex and fragmented governance systems (Raakjaer et al., 2014). Although each of the regional seas have their own conventions (North-East Atlantic, Oslo/Paris Convention; Baltic Sea, Helsinki Convention; Mediterranean Sea, Barcelona Convention; Black Sea, Bucharest Convention) with similar objectives and targets, there are differences in the cohesiveness of each regional seas EU Member States and state of developed/stability of the related bordered countries. Geographically differing stages of development influence the status, quality and quantity of monitoring programmes producing data for assessments of Drivers, Pressures and State change (Patrício et al., 2014).
Data Availability
Within a causal link framework and to provide the route for and efficacy of management, indicators and their component indices/metrics are needed to determine the level of Pressure, and changes in State and Impact (e.g., Aubry and Elliott, 2006). The trajectory of State change can be used to determine targets or reference conditions for the assessment of the indicators (see Borja et al., 2012) which requires developing assessment methods or indices such as those within the Water Framework Directive (Birk et al., 2012). However, they need to be validated and calibrated against independent abiotic datasets (Birk et al., 2013). As some of the MSFD descriptors are related to Pressures, whilst others are related to State change, data analysis is needed to assess the effects that Activities have on marine physical, chemical and biological quality. Consequently all the relevant Activities, Pressures, States and their indicators need to be identified together with the linkages (cause-effect interactions) between them. The ODEMM Project linkage framework (Knights et al., 2013; White et al., 2013), for example, provides a means to fully evaluate all components that can affect the achievement of GES in a fully integrated ecosystem assessment. Applying a framework relies on having not only indices of change but also baselines, thresholds and targets against which to judge that change. In addition, there is the need to define the inherent variability (“noise”) against which the “signal” of change is measured (Kennish and Elliott, 2011). Each of these requires a fit-for-purpose data background for each biological and physico-chemical component relevant to a particular stressor. Given that for many Activities, the amount of Pressure required to produce a given State change and thus Impact on human welfare is unknown, then the amount of data required to determine and assess the State change is also unknown. Furthermore, although this could be determined through power analysis, it cannot be used unless the inherent variability in the components is known. Hence, it is likely that the approaches advocated here will continue to be semi-qualitative at best and reliant on expert judgment (see below).
Cumulative/In-Combination Effects
As single Activities exert multiple Pressures and the marine ecosystem usually supports multiple Activities, we need to consider cumulative/co-occurring (within an Activity) and in-combination (between Activities) effects. The multiple Pressures will rarely be equal and will lead to cumulative and in-combination effects which may be synergistic or antagonistic (Griffen et al., 2016). To indicate some of difficulties in assessing cumulative impacts, Crain et al. (2008) analyzed 171 multiple stressors studies in marine and coastal environments and found effects to be 26% additive, 36% synergistic, and 38% antagonistic, while interaction type varied by response level, trophic level, and specific stressors. In another meta-analysis of 112 experimental studies Darling and Côté (2008) found similar combined effects of two stressors with 23% additive, 35% synergistic and 42% antagonistic. Despite the lack of knowledge at the community and ecosystem level elucidating or predicting effects of combinations of individual Pressure impacts, we can measure the status of an ecosystem that is impacted by multiple Pressures (Griffen et al., 2016; Nõges et al., 2016; Teichert et al., 2016). Hence, while we can identify some elements, we are unclear regarding the precise changes at a sub-species, species, population or community level.
Co-occurring multiple Activity/Pressure impacts, as cumulative and in-combination threats or impacts, have been investigated according to the footprints of a particular Driver/Activity and their overlap with habitats using spatial mapping/modeling (Nõges et al., 2016). Cumulative impacts (including both overlap and weighted cumulative methods) have been investigated at a global level by Halpern et al. (2008) producing global impacts maps but also at the European level, for example in the Baltic (Korpinen et al., 2013; Andersen et al., 2015), eastern North Sea (Andersen et al., 2013) and the Mediterranean-Black Seas (Claudet and Fraschetti, 2010; Coll et al., 2011; Micheli et al., 2013). These techniques may not be of direct use in assessing State changes, but may nevertheless be of value in spatial planning applications, for example, in identifying areas where high levels of protection may be necessary. It should be noted that an Activity does not always have to lead to an impact especially if mitigation measures are employed.
Assessment Scales and Scaling Up to Regional Seas
The connections between ecosystem features and human Activities (and their related Pressures) should determine the appropriate scale at which the ecosystem approach should be implemented (Borja et al., 2016). Defining these scales and their boundaries is imperative for any ecosystem-based management (EEA, 2014). For a well monitored small bay, a comprehensive assessment can be normally made, because the Drivers, Pressures, and State changes could be well understood, mapped and assessed. However, at a larger scale, not all issues may be well known; some areas have quantitative data, some have no data, and a more widespread range of very differing habitats may be included. Borja et al. (2013) suggest that the fundamental challenge of obtaining a regional quality status is by either having a broad approach and omitting or down-weighting point-source problems or summing the point-source problems (which may cover only a very small area) to indicate the quality status of the whole area. State change becomes much more complicated and diverse. An important issue is the mismatch between the quantitative information from the pressures and different descriptors and biodiversity components at a large scale (i.e., regional or sub-regional sea), making difficult the large-scale assessment of the response of indicators of change. During the first phase of MSFD implementation, the baseline assessment of the EU marine area in 2012 gave a very broad understanding of Pressures and impacts from human Activities. Although most Member States have reported on most descriptors, providing an overview of their marine environment, the quality of reporting varies widely between countries, and even within individual Member States, from one descriptor to another (EC, 2014; Palialexis et al., 2014). In addition, when different countries are involved in the assessment, the relevant information may come from many different sources, which each have their own assessment timescales, aims, indicators, criteria, targets and baseline values thus limiting not only direct comparison, but also coherence in implementation (Cavallo et al., 2016).
Levels of Confidence
A conceptual framework such as DPSIR aims to encompass all key components and interactions of an ecosystem problem. However, when moving to the next step of assessment, incorporating many types of data, confidence in the outcome becomes an issue for both the assessors and the users of the assessment. The level of confidence in an assessment depends on the degree of uncertainty associated with the method of assessment, data availability and adequacy, and knowledge and understanding. This requires distinguishing between the lack of knowledge and natural variability (Hoffman and Hammonds, 1994), and uncertainty in the future forecasted state (due to lack of long-term data sets and historical data and/or spatio-temporal variability of a biological indicator) as well as in the resulting ecosystem state post-management action, present challenges in target setting (Knights et al., 2014). Uncertainty is mostly addressed through monitoring programmes that have adequate spatio-temporal coverage (Borja et al., 2010), although the absence of reference conditions or clear targets makes it difficult to establish an accurate assessment (Borja et al., 2012). However, confidence can also be given through a range of methods from cumulative qualitative assessment of each metric and, for example, a traffic-light overall confidence assessment to a separate quantitative confidence metric (e.g., Andersen et al., 2010; Carstensen and Lindegarth, 2016). Despite this, most uncertainty is due to poor definition in the determination of deviation from that expected, in a physico-chemical or biological component. If the agreed targets against which indices and metrics are judged are not sufficiently well defined, then it is not possible to judge the efficacy of management measures.
Moving to the Next Step: Assessment
The intricacy and complexity of Driver-Pressure interactions, and the relationship of Pressures to State changes makes it difficult to undertake high level or quantitative assessments for management purposes. It requires knowledge of all the potential causal chains and State changes. The possible methodologies are broadly either a matrices approach or as a form of ecosystem modeling but the assessment is only as good as the knowledge and detail applied (Borja et al., 2016).
Simple Matrices Approach
Matrices are simple tables where Drivers (or, more specifically, the Activities resulting from them) can be related to Pressures, and where Pressures can be related to ecosystem components. These allow the identification of chains formed by particular causal links and permit linear analysis of the impact chain (Knights et al., 2013). The matrices record relationships between Activity and Pressures, and between Pressures and ecosystem components. The relationships that are represented are complex with, for example, any single Activity potentially causing many Pressures, and any single Pressure being caused by more than one Activity (i.e., a many-to-many relationship). The matrices can be linked simply by an overlap (Pressure X affects component Y) or through more detailed information on potential levels of interaction, for example showing high/low or increasing/decreasing changes to a component. The degree of State change caused by a Pressure on a habitat can be assessed in terms of: Activity area or footprint, frequency, persistence, and characteristics of the habitat/ecosystem component impacted, including sensitivity and resilience (ability for recovery) (Knights et al., 2015). Matrices and Pressure assessment approaches were used extensively in the ODEMM project (Knights et al., 2011; Robinson and Knights, 2011; White et al., 2013) and in the DEVOTES project (Barnard et al., 2015). They have also been used as standard tools for Pressure assessments by, for example, HELCOM and OSPAR (Johnson, 2008). Complex matrices and linkages can be compiled through databases where programming can be used to analyze and filter data, for example, to highlight Activities that need to be managed or sensitive ecological components that might be at risk of State change [e.g., the PRISM and PISA Access database tools developed through the U.K. Net Gain project (Net Gain, 2011)]. The accuracy and value of the matrix approach depends on identifying and parameterizing components and linkages for a particular area. They are valuable for assessments, and depending on how comprehensive they are, will show impacted components which may then allow prediction of the State changes under given circumstances.
Ecosystem Models
With the move toward ecosystem-based management, much attention has been devoted to ecosystem modeling. These models may be conceptual, deterministic (in which there is underlying theory or embedded mathematical relationships) or empirical in which the links are described statistically even when there is no apparent underlying theory. Some relate to the management of particular aspects of the ecosystem (e.g., Robinson and Frid, 2003; Plagányi, 2007) whilst other, more recent, models concern the whole natural ecosystem or socio-ecological system (i.e., “end-to-end” models) model development/application (e.g., Rose et al., 2010; Heath, 2012). Piroddi et al. (2015), in reviewing whole ecosystem models with respect to MSFD assessments, note that they are more relevant as they may better represent interactions with biodiversity components, for example, ECOPATH with ECOSIM, ATLANTIS or coupled lower trophic and high trophic models (Rose et al., 2010). The ability to apply models to Drivers and Pressure effects relies on knowledge of Activities/Pressures and being able to parameterize the elements accordingly. For example, if trawling causes a 30% reduction in suspension feeders in a modeled area, this figure can be applied to that biological component (according to temporal or spatial scales) (Petihakis et al., 2007). A specific model may not have the resolution to apply a precise mechanism or be applied at individual habitat scale. Whilst pelagic habitats may be defined by salinity, temperature, depth, nutrients, oxygen, etc., benthic habitats as different spatial entities (an important setting for all species groups) are generally not parameterized in models. Nevertheless, such models may well be able to accommodate indirect effects such as changes in predator-prey relations or be used in a predictive manner where climate change could be de-coupled from anthropogenic impacts.
Bayesian Belief Networks
Bayesian Belief Networks (BBNs; also referred to as belief networks, causal nets, causal probabilistic networks, probabilistic cause effect models, and graphical probability networks) offer a pragmatic and scientifically credible approach to modeling complex ecological systems and problems, where substantial uncertainties exist. A BBN is a graphical and probabilistic representation of causal and statistical relationships across a set of variables (McCann et al., 2006). It consists of graphically represented causal relationships (for example, the DPSIR D-P-S chain links) comprised of nodes that represent component variables and causal dependencies or links based on an understanding of underlying processes/relationships/association. Each node is associated with a function that gives the probability of the variable dependent on the upstream/parent nodes. As each variable is set with best data available and can include expert opinion, simulation results or observed data, this is flexible and also allows the information to be easily updated with improved data (from Hamilton et al., 2005; Pollino et al., 2007). Notwithstanding their potential, BBNs represent a relatively new modeling approach. They have only been applied to marine assessments in a limited way (e.g., Langmead et al., 2007; Stelzenmuller et al., 2015; Uusitalo et al., 2015). However, BBNs are becoming an increasingly popular modeling tool, particularly in ecology and environmental management. This is largely because they can be used in a predictive capacity and also, because they use probabilities to quantify relationships between model variables, while explicitly allowing uncertainty and variability to be accommodated in model predictions (Barnard and Boyes, 2013). They show high promise in adaptive management being iterative and especially in being able to combine both empirical data and expert knowledge, a necessary feature given the often poor data for those empirical relationships.
The Bow-Tie Approach
The Bow-tie method was initially presented as a conceptual model; whilst its original application was mostly in relation to industrial risk assessment and management (de Ruijter and Guldenmund, 2016), it is now increasingly being used in a qualitatively manner to explore the natural and anthropogenic causes of change, and the associated consequences and responses (e.g., Cormier et al., 2013; Smyth and Elliott, 2014; Burdon et al., in press). It facilitates analysis or assessment of a defined problem by focusing attention onto the areas of a system where the consequences of a potentially damaging event can be proactively managed. The Bow-tie method provides for a graphical representation of the expansion of the initial DPSIR environmental cause-and-effect pathway (Cormier et al., 2013). More specifically, it can be used to focus on the pathway between Pressure and State change, and provides a means of identifying where controls can be put in place either to control the occurrence of a particular event, or to mitigate for the effects of the event should it occur (see Figure 4). It comprises several components:
• The start of any Bow-tie is the identification of a “Hazard”—which is defined as a part of the system under consideration that has the potential to cause damage (e.g., benthic trawling).
• A Top Event is identified, representing the point where control would be lost over the Hazard. The Top Event is defined so as to be occurring just before events start causing actual damage.
• There are usually a number of “Threats” that might give rise to a given Top Event; if these threats are not prevented from occurring, or are not mitigated in some way, the realization of the Top Event could then cause a set of one or more “Consequences.” There are usually several or many Threats and Consequences for every Top Event.
• The final stage of building a basic Bow-tie model is to identify potential barriers which can be placed either between the Threat and the Top Event as prevention measures, or alternatively as recovery, mitigation or compensation mechanisms that either prevent the Top Event from escalating into actual Consequences, or reduce the severity of the Consequences. Preventative measures can take several forms, including economic, governance, societal, political or technological devices, based on the 10-tenets of adaptive management and sustainability (Barnard and Elliott, 2015).
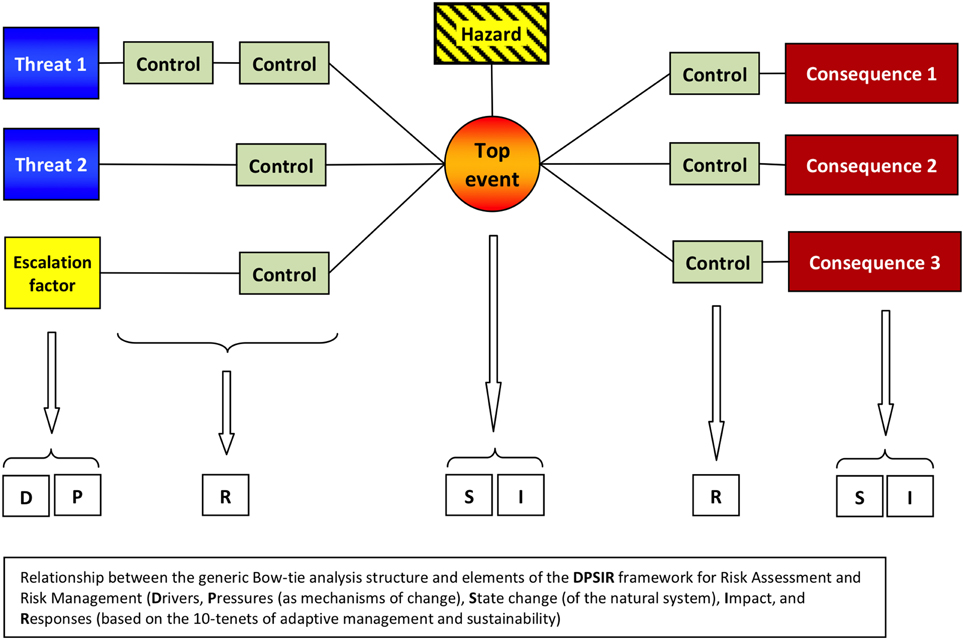
Figure 4. Example of a Bow-tie structure and how it relates to DPSIR. This shows the Threats (left) and Consequences (right) relating to the Top Event (e.g., loss/decrease in marine wind resource/production ability, lower center) that is associated with a Hazard (environmental change due to climate change impacts, upper center). It also details the related Prevention Measures (left of center), Mitigation Measures (right of center), and Escalation Factors.
There may be several top events in any one area as the result of the Drivers and hazards such that nested Bow-ties are required in any assessment of cumulative impacts (Cormier, 2015). Similarly, the consequence of the loss of control in one Bow-tie sequence may become the top event in another (Smyth and Elliott, 2014). For example, the threat of the introduction of non-indigenous species may be a top event, the consequence of which may be that an area fails GES under the MSFD. In turn, the failure to meet GES will then become the Top Event which has legal and financial consequences, each requiring mitigation (Smyth and Elliott, 2014). It is of note that ICES (2014) has recommended that the Bow-tie framework be used to address cumulative and in-combination Pressures and their consequences.
The DPSIR framework can be superimposed on the Bow-tie structure given that the threats to the top-event will be Drivers and/or Pressures and the top-event and consequences are likely to be the State changes and/or Impacts (Figure 4). The barriers both as prevention measures and as mitigation or compensation measures, constitute the Response within DPSIR. As such, this links to a risk assessment and then risk management (RARM) framework as the need for responses to human Pressures. Burdon et al. (in press) have directly linked the DAPSI(W)R(M) concept with Bow-tie in integrating natural and social sciences in a case study for the management of the Dogger Bank in the North Sea.
Nested Environmental Status Assessment Tool
The Nested Environmental status Assessment Tool (NEAT) is a recent tool for biodiversity assessments based on State indicators (Borja et al., 2016; and see therein for older, similar assessment tools). NEAT is a specialized user-friendly desktop application developed recently within the EU DEVOTES project (Berg et al., 2016) specifically targeted toward MSFD biodiversity assessments for defined spatial areas. It does not relate to Activities or specific Pressures, rather levels of State in relation to targets/thresholds. Assessments are indicator based with a large library of available indicators, habitats and ecosystem components. It allows different rules to be used for aggregating indicators, is fully customizable and will determine uncertainty values based on data inputs. The environmental status of a spatial assessment unit is obtained by choosing the marine region, entering the assessment values for the indicators chosen (along with an uncertainty measure and the classification scale) allowing the software to calculate and show the resulting status assessment. The algorithms and intermediate calculations are based on weighted average normalized indicators within specific groups. The NEAT weighting procedure avoids the dominance of certain indicators or habitats or spatial units. Thus, no bias is introduced into the assessment by the choice of the indicators. The tool is being trialed with many different user groups and national authorities. It is freely available for a number of different platforms at http://www.devotes-project.eu/neat/.
Concluding Remarks
In defining and describing the DPSIR Conceptual Framework, we show how it facilitates management and assessment issues and, through the detailed worked examples, show its particular use with respect to the MSFD. By showing the predominant use of the DPSIR framework and its derivatives as a generic approach to risk assessment and risk management, we emphasize the practical limits of conceptual models and diagrams. Whilst they are of value in an abstract or generic application, the underlying complexity of marine systems means that specific applications cannot be easily shown diagrammatically. Hence, following simple Pressure-Impact linkages, the most straightforward option for assessing specific examples of this conceptual model is to record relationships between successive stages by means of matrices. Subsequently, matrices and linkages can be compiled within a database and interrogated and analyzed by means of interactive data filters. Such an approach facilitates the extraction of information for specific stages of the overall process, which can then be used as the input to other techniques, such as Bow-tie analysis.
In emphasizing the complexity of the marine system, here we show that although creating a system which covers all eventualities (all Activities, Pressures, State changes and Impacts on human welfare and the links between these) is a laudable aim, it is more profitable to focus on a problem-based approach. Hence for any specific area (e.g., a Regional Sea, eco-region, or sub-ecoregion) to determine the ranked priority Pressures based on the number of Activities. Each of these can then be addressed through the proposed DPSIR-Bow-tie linked approach in which we can address the main risks and hazards creating Pressures, and thus the Main Event of concern (Smyth and Elliott, 2014). The challenge for marine management, as shown here, is to apply a linked DPSIR approach for the area being managed. By focusing on the risk assessment approach, i.e., the Pressures as mechanisms causing the State changes and Impacts on Human Welfare (and so ultimately impacting on Ecosystem Services and Societal Benefits, sensu Atkins et al. (2011)), then by definition management measures for prevention and mitigation/compensation can be implemented; hence the latter being the Responses under DPSIR and the means by which the Responses address the Drivers and Pressures (and State changes) becomes the risk management framework (see Elliott, 2014).
A further challenge, again given the complexity of the marine system, its uses and users, is its ability to respond to exogenic unmanaged Pressures as well as the endogenic managed Pressures where current assessments rarely consider climate changes, although its effects may be implicit in the measurement of indicators. Hence management not only has to provide the Responses to the causes and consequences of change due to system internal Pressures but also the Responses to the consequences of external Pressures. Because of this, the application of the proposed scheme to cumulative and in-combination Pressures, as discussed here, is also an imminent challenge.
Author Contributions
CS led the work on this manuscript. CS, KP, SB, KM, ME comprised the core writing team with inputs from JP, OS, SL, NB, AB. All authors were involved in reviewing and editing the manuscript.
Conflict of Interest Statement
The authors declare that the research was conducted in the absence of any commercial or financial relationships that could be construed as a potential conflict of interest.
The handling Editor declared a collaboration with the authors and states that the process nevertheless met the standards of a fair and objective review.
Acknowledgments
This study was undertaken as part of the DEVOTES (DEVelopment Of innovative Tools for understanding marine biodiversity and assessing good Environmental Status) project, funded by the European Union under the 7th Framework Programme, “The Ocean of Tomorrow” Theme (grant agreement no. 308392), www.devotes-project.eu. A preliminary version of this work was available in Smith et al. (2014) found on-line at www.devotes-project.eu/wp-content/uploads/2014/06/DEVOTES-D1-1-ConceptualModels.pdf. The authors would also like to thank two referees for helping to improve the manuscript.
References
Alexander, R. R., Stanton, R. J. Jr., and Dodd, J. R. (1993). Influence of sediment grain size on the burrowing of bivalves: correlation with distribution and stratigraphic persistence of selected Neogene clams. Palaios 8, 289–303. doi: 10.2307/3515151
Amiard-Triquet, C., Amiard, J. C., and Mouneyrac, C. (2015). Aquatic Ecotoxicology. Advancing Tools for Dealing with Emerging Risks. London: Academic Press, Elsevier.
Andersen, J. H., Halpern, B. S., Korpinen, S., Murray, C., and Reker, J. (2015). Baltic Sea biodiversity status vs. cumulative human pressures. Estuar. Coast. Shelf S. 161, 88–92. doi: 10.1016/j.ecss.2015.05.002
Andersen, J. H., Murray, C., Kaartokallio, H., Axe, P., and Molvaer, J. (2010). A simple method for confidence rating of eutrophication status classifications. Mar. Pollut. Bull. 60, 919–924. doi: 10.1016/j.marpolbul.2010.03.020
Andersen, J. H., Stock, A., Mannerla, M., Heinänen, S., and Vinther, M. (2013). Human Uses, Pressures and Impacts in the Eastern North Sea. Aarhus University, DCE – Danish Centre for Environment and Energy. 136. Technical Report from DCE – Danish Centre for Environment and Energy No. 18. Available online at: http://www.dmu.dk/Pub/TR18.pdf
Atkins, J. P., Burdon, D., Elliott, M., and Gregory, A. J. (2011). Management of the marine environment: integrating ecosystem services and societal benefits with DPSIR framework in a systems approach. Mar. Pollut. Bull. 62, 215–226. doi: 10.1016/j.marpolbul.2010.12.012
Aubry, A., and Elliott, M. (2006). The use of environmental integrative indicators to assess seabed disturbance in estuaries and coasts: application to the Humber Estuary, UK. Mar. Pollut. Bull. 53, 175–185. doi: 10.1016/j.marpolbul.2005.09.021
Barnard, S., and Boyes, S. J. (2013). Review of Case Studies and Recommendations for the Inclusion of Expert Judgement in Marine Biodiversity Status Assessments - A report for the Joint Nature Conservation Committee. JNCC Report No. 490 by the Institute of Estuarine and Coastal Studies, University of Hull.
Barnard, S., and Elliott, M. (2015). The 10-tenets of adaptive management and sustainability: An holistic framework for understanding and managing the socio-ecological system. Environ. Sci. Policy 51, 181–191. doi: 10.1016/j.envsci.2015.04.008
Barnard, S., Kryvenko, O., Churilova, T., Suslin, V., Papadopoulou, N., Moncheva, S., et al. (2015). Report on Sea-Specific Matrices of Pressure-Impact Links. DEVOTES FP7 Project Deliverable 1.2. 133. Available online at: http://www.devotes-project.eu/wp-content/uploads/2015/10/DEVOTES-D1.2.pdf
Berg, T., Murray, C., Carstensen, J., and Andersen, J. H. (2016). Manual, Guidelines and Software for Biodiversity Assessment. Deliverable D6.3. DEVOTES Project. 41. Available online at: http://www.devotes-project.eu/download/25439/
Birk, S., Bonne, W., Borja, Á., Brucet, S., Courrat, A., Poikane, S., et al. (2012). Three hundred ways to assess Europe's surface waters: an almost complete overview of biological methods to implement the Water Framework Directive. Ecol. Indic. 18, 31–41. doi: 10.1016/j.ecolind.2011.10.009
Birk, S., Willby, N. J., Kelly, M. G., Bonne, W., Borja, A., Poikane, S., et al. (2013). Intercalibrating classifications of ecological status: Europe's quest for common management objectives for aquatic ecosystems. Sci. Total Environ. 454–455, 490–499. doi: 10.1016/j.scitotenv.2013.03.037
Blaber, S. J. M., Albaret, J.-J., Ching, C. V., Cyrus, D. P., Day, J. W., Elliott, M., et al. (2000). Effects of fishing on the structure and functioning of estuarine and nearshore ecosystems. ICES J. Mar. Sci. 57, 590–602. doi: 10.1006/jmsc.2000.0723
Borja, A., Bremner, J., Muxika, I., and Rodríguez, J. G. (2015). “Biological responses at supraindividual levels,” in Aquatic Ecotoxicology. Advancing Tools for Dealing with Emerging Risks, eds C. Amiard-Triquet, J. C. Amiard, and C. Mouneyrac (London: Academic Press, Elsevier), 333–353.
Borja, Á., Dauer, D. M., and Grémare, A. (2012). The importance of setting targets and reference conditions in assessing marine ecosystem quality. Ecol. Indic. 12, 1–7. doi: 10.1016/j.ecolind.2011.06.018
Borja, A., Elliott, M., Andersen, J. H., Berg, T., Carstensen, J., Halpern, B. S., et al. (2016). Overview of integrative assessment of marine systems: the Ecosystem Approach in practice. Front. Mar. Sci. 3:20. doi: 10.3389/fmars.2016.00020
Borja, Á., Elliott, M., Andersen, J. H., Cardoso, A. C., Carstensen, J., Ferreira, J. G., et al. (2013). Good Environmental Status of marine ecosystems: what is it and how do we know when we have attained it? Mar. Pollut. Bull. 76, 16–27. doi: 10.1016/j.marpolbul.2013.08.042
Borja, Á., Elliott, M., Carstensen, J., Heiskanen, A.-S., and van de Bund, W. (2010). Marine management – Towards an integrated implementation of the European Marine Strategy Framework and the Water Framework Directives. Mar. Pollut. Bull. 60, 2175–2186. doi: 10.1016/j.marpolbul.2010.09.026
Borja, Á., Galparsoro, I., Solaun, O., Muxika, I., Tello, E. M., Uriarte, A., et al. (2006). The European Water Framework Directive and the DPSIR, a methodological approach to assess the risk of failing to achieve good ecological status. Estuar. Coast. Shelf S. 66, 88–96. doi: 10.1016/j.ecss.2005.07.021
Boyes, S. J., and Elliott, M. (2014). Marine legislation – The ultimate ‘horrendogram’: International law, European directives & national implementation. Mar. Pollut. Bull. 86, 39–47. doi: 10.1016/j.marpolbul.2014.06.055
Bremner, J., Frid, C. L. J., and Rogers, S. I. (2003). Assessing marine ecosystem health: the long-term effects of fishing on functional biodiversity in North Sea benthos. Aquat. Ecosyst. Health Manag. 6, 131–137. doi: 10.1080/14634980301470
Burdon, D., Boyes, S. J., Elliott, M., Smyth, K., Atkins, J. P., Barnes, R. A., et al. (in press). Integrating natural and social sciences to manage sustainably vectors of change in the marine environment: Dogger Bank transnational case study. Estuar. Coast. Shelf S. doi: 10.1016/j.ecss.2015.09.012
Carstensen, J., and Lindegarth, M. (2016). Confidence in ecological indicators: a framework for quantifying uncertainty components from monitoring data. Ecol. Indic. 67, 306–317. doi: 10.1016/j.ecolind.2016.03.002
Cavallo, M., Elliott, M., Touza-Montero, J., and Quintino, V. (2016). The ability of regional coordination and policy integration to produce coherent marine management: implementing the Marine Strategy Framework Directive in the North-East Atlantic. Mar. Policy 68, 108–116. doi: 10.1016/j.marpol.2016.02.013
Clark, M. R., Althaus, F., Schlacher, T. A., Williams, A., Bowden, D. A., and Rowden, A. A. (2016). The impacts of deep-sea fisheries on benthic communities: a review. ICES J. Mar. Sci. 73, i51–i69. doi: 10.1093/icesjms/fsv123
Claudet, J., and Fraschetti, S. (2010). Human-driven impacts on marine habitats: a regional meta-analysis in the Mediterranean Sea. Biol. Conserv. 143, 2195–2206. doi: 10.1016/j.biocon.2010.06.004
Coll, M., Piroddi, C., Albouy, C., Ben Rais Lasram, F., Cheung, W. W. L., Christensen, V., et al. (2011). The Mediterranean Sea under siege: spatial overlap between marine biodiversity, cumulative threats and marine reserves. Global Ecol. Biogeogr. 21, 465–480. doi: 10.1111/j.1466-8238.2011.00697.x
Cooper, P. (2013). Socio-ecological accounting: DPSWR, a modified DPSIR framework, and its application to marine ecosystems. Ecol. Econ. 94, 106–115. doi: 10.1016/j.ecolecon.2013.07.010
Cormier, R. (2015). “A comprehensive framework for characterizing cumulative effects in aquatic ecosystems to support regional environmental assessments and integrated planning and management. contribution to section III: governance experience and issues for managing impacts,” in Managing the Impacts of Human Activities on Fish Habitat: The Governance, Practices, and Science, eds N. Fisher, P. LeBlanc, C. A. Rose, and B. Sadler (Bethesda, MD: American Fisheries Society), 264. ISBN-13: 978-1-934874-41-7.
Cormier, R., Kannen, A., Elliott, M., Hall, P., and Davies, I. M. (eds.) (2013). Marine and Coastal Ecosystem-based Risk Management Handbook. ICES Cooperative Research Report No. 317, 60.
Crain, C. M., Kroeker, K., and Halpern, B. S. (2008). Interactive and cumulative effects of multiple human stressors in marine systems. Ecol. Lett. 11:1304. doi: 10.1111/j.1461-0248.2008.01253.x
CSWD (2014). Annex Accompanying the Document Commission Report to the Council and the European Parliament. The First Phase of Implementation of the Marine Strategy Framework Directive (2008/56/EC). The European Commission's Assessment and Guidance. Brussels, 20.2.2014 COM(2014) 97 final.
CSWP (2011). Commission Staff Working Paper – Relationship between the Initial Assessment of Marine Waters and the Criteria for Good Environmental Status. European Commission, Brussels, 14.10.2011. SEC(2011) 1255 final.
CSWP (2012). European Commission Staff Working Paper (CSWP) Guidance for 2012 Reporting Under the Marine Strategy Framework Directive, using the MSFD Database Tool. Version 1.0. DG Environment, Brussels, 164.
Darling, E. S., and Côté, I. M. (2008). Quantifying the evidence for ecological synergies. Ecol. Lett. 11, 1278–1286. doi: 10.1111/j.1461-0248.2008.01243.x
de Ruijter, A., and Guldenmund, F. (2016). The bowtie method: a review. Safety Sci. 88, 211–218. doi: 10.1016/j.ssci.2016.03.001
DIKE (2011). Approach to Reporting for the MSFD. Marine Strategy Framework Working Group on Data, Information and Knowledge Exchange (WG DIKE). DIKE 4/2011/02. DG Environment. EC. 23.
Duarte, C. M., Borja, A., Carstensen, J., Elliott, M., Krause-Jensen, D., and Marbà, N. (2015). Paradigms in the recovery of estuarine and coastal ecosystems. Estuar. Coast. 38, 1202–1212. doi: 10.1007/s12237-013-9750-9
EC (1999). Towards Environmental Pressure Indicators for the EU. 1st Edn. Luxembourg: Office for Official Publications of the European Communities.
EC (2008). Directive 2008/56/EC of the European Parliament and of the Council of 17 June 2008 establishing a framework for community action in the field of marine environment policy (Marine Strategy Framework Directive). Off. J. Eur. Union 164, 19–40.
EC (2010). EU Commission Decision of 1 September 2010 on criteria and methodological standards on good environmental status of marine waters (2010/477/EU). Off. J. Eur. Union 232, 14–24.
EC (2014) COM(2014) 97 Final. Report from the Commission to the Council and the European Parliament. The First Phase of Implementation of the Marine Strategy Framework Directive (2008/56/EC). The European Commission's Assessment and Guidance {SWD(2014) 49 final}. Available online at: http://eur-lex.europa.eu/legal-content/EN/TXT/PDF/?uri=CELEX:52014DC0097&from=EN
EEA (2014). Marine Messages. Our Seas, our Future – Moving Towards a New Understanding. Copenhagen: European Environment Agency. Available online at: http://www.eea.europa.eu/publications/marine-messages
Eigaard, O. R., Bastardie, F., Breen, M., Dinesen, G. E., Hintzen, N. T., Laffargue, P., et al. (2016). Estimating seabed pressure from demersal trawls, seines, and dredges based on gear design and dimensions. ICES J. Mar. Sci. 73, i27–i43. doi: 10.1093/icesjms/fsv099
Elliott, M. (2002). The role of the DPSIR approach and conceptual models in marine environmental management: an example for offshore wind power. Mar. Pollut. Bull. 44, iii–vii. doi: 10.1016/s0025-326x(02)00146-7
Elliott, M. (2011). Marine Science and management means tackling exogenic unmanaged pressures and endogenic managed pressures - a numbered guide. Mar. Pollut. Bull. 62, 651–655. doi: 10.1016/j.marpolbul.2010.11.033
Elliott, M. (2014). Integrated marine science and management: wading through the morass. Mar. Pollut. Bull. 86, 1–4. doi: 10.1016/j.marpolbul.2014.07.026
Elliott, M., Borja, Á., McQuatters-Gollop, A., Mazik, K., Birchenough, S., Andersen, J. H., et al. (2015). Force majeure: will climate change affect our ability to attain Good Environmental Status for marine biodiversity? Mar. Pollut. Bull. 95, 7–27. doi: 10.1016/j.marpolbul.2015.03.015
Elliott, M., Burdon, D., Callaway, R., Franco, A., Hutchinson, T., Longshaw, M., et al. (2012). Burry Inlet Cockle Mortalities Investigation 2009-2011. Final Technical Report to Environment Agency (Wales), 17 January 2012. Available online at: http://a0768b4a8a31e106d8b0-50dc802554eb38a24458b98ff72d550b.r19.cf3.rackcdn.com/gewa0112bwap-e-e.pdf
Elliott, M., Cutts, N. D., and Trono, A. (2014). A typology of marine and estuarine hazards and risks as vectors of change: a review for vulnerable coasts and their management. Ocean Coast. Manage. 93, 88–99. doi: 10.1016/j.ocecoaman.2014.03.014
Elliott, M., and Quintino, V. (2007). The Estuarine Quality Paradox, Environmental Homeostasis and the difficulty of detecting anthropogenic stress in naturally stressed areas. Mar. Pollut. Bull. 54, 640–645. doi: 10.1016/j.marpolbul.2007.02.003
EPA (1994). A Conceptual Framework to Support the Development and Use of Environmental Information. Environmental Statistics and Information Division. Office of Policy, Planning and Evaluation. EPA 230-R-94-012, USEPA, Washington, DC.
Foden, J., Rogers, S. I., and Jones, A. P. (2010). Recovery of UK seabed habitats from benthic fishing and aggregate extraction: towards a cumulative impact assessment. Mar. Ecol. Progr. Ser. 411, 259–270. doi: 10.3354/meps08662
Gari, S. R., Newton, A., and Icely, J. D. (2015). A review of the application and evolution of the DPSIR framework with an emphasis on coastal social-ecological systems. Ocean Coast. Manage. 103, 63–77. doi: 10.1016/j.ocecoaman.2014.11.013
Gibbs, M., and Cole, A. (2008). “Oceans and coasts as complex adaptive systems,” in Ecological Economics of the Oceans and Coasts, eds M. G. Patterson and B. C. Glavovic (Cheltenham: Edward Elgar Publishing), 74–91.
Gilkinson, K. D., Gordon, D. C. Jr., MacIsaac, K. G., McKeown, D. L., Kenchington, E. L. R., Bourbonnais, C., et al. (2005). Immediate impacts and recovery trajectories of macrofaunal communities following hydraulic clam dredging on Banquereau, eastern Canada. ICES J. Mar. Sci. 62, 925–947. doi: 10.1016/j.icesjms.2005.03.009
Gray, J. S., and Elliott, M. (2009). Ecology of Marine Sediments – From Science to Management, 2nd Edn. Oxford: Oxford University Press.
Griffen, B. D., Belgrad, B. A., Cannizzo, Z. J., Knotts, E. R., and Hancock, E. R. (2016). Rethinking our approach to multiple stressor studies in marine environments. Mar. Ecol. Progr. Ser. 543, 273–281. doi: 10.3354/meps11595
Halpern, B. S., Walbridge, S., Selkoe, K. A., Kappel, C. V., Micheli, F., D'Agrosa, C., et al. (2008). A global map of human impact on marine ecosystems. Science 319, 948–952. doi: 10.1126/science.1149345
Hamilton, G., Alston, C., Chiffings, T., Abal, E., Hart, B., and Mengersen, K. (2005). “Integrating science through bayesian belief networks: case study of lyngbya in moreton bay,” in Proceedings of International Congress on Modelling and Simulation 2005, Modelling and Simulation Society of Australia and New Zealand Inc., eds A. Zerger and R. Argent, (Melbourne, VIC), 392–399.
Heath, M. R. (2012). Ecosystem limits to food web fluxes and fisheries yields in the North Sea simulated with an end-to-end food web model. Prog. Oceanogr. 102, 42–66. doi: 10.1016/j.pocean.2012.03.004
Hiddink, J. G., Jennings, S., Kaiser, M. J., Queirós, A. M., Duplisea, D. E., and Piet, G. J. (2006). Cumulative impacts of seabed trawl disturbance on benthic biomass, production, and species richness in different habitats. Can. J. Fish. Aquat. Sci. 63, 721–736. doi: 10.1139/f05-266
Hoffman, F. O., and Hammonds, J. S. (1994). Propagation of uncertainty in risk assessments: the need to distinguish between uncertainty due to lack of knowledge and uncertainty due to variability. Risk Anal. 14, 707–712. doi: 10.1111/j.1539-6924.1994.tb00281.x
ICES (2014). Report of the Joint RIJKSWATERSTAAT/DFO/ICES workshop: Risk Assessment for Spatial Management (WKRASM), 24-28 February 2014, Amsterdam. ICES CM 2014 /SSGHIE:07. 35.
Johnson, D. (2008). Environmental indicators: their utility in meeting the OSPAR Convention's regulatory needs. ICES J. Mar. Sci. 65, 1387–1391. doi: 10.1093/icesjms/fsn154
Katsanevakis, S., Deriu, I., D'Amico, F., Nunes, A. L., Peláez-Sánchez, S., Crocetta, F., et al. (2015). European Alien Species Information Network (EASIN): supporting European policies and scientific research. Manag. Biol. Invasions 6, 147–157. doi: 10.3391/mbi.2015.6.2.05
Kennish, M. J., and Elliott, M. (2011). “Volume 8. Human-induced problems (uses and abuses) in Estuaries and Coasts,” in Treatise on Estuarine and Coastal Science, eds E. Wolanski and D. S. McLusky (Amsterdam: Elsevier), 315.
Knights, A. M., Culhane, F., Hussain, S. S., Papadopoulou, K. N., Piet, G. J., Raakær, J., et al. (2014). A step-wise process of decision-making under uncertainty when implementing environmental policy. Environ. Sci. Policy 39, 56–64. doi: 10.1016/j.envsci.2014.02.010
Knights, A. M., Koss, R. S., Papadopoulou, N., Cooper, L. H., and Robinson, L. A. (2011). Sustainable use of European Regional Seas and the Role of the Marine Strategy Framework Directive. Deliverable 1, EC FP7 Project (244273) ‘Options for Delivering Ecosystem-based Marine Management’. University of Liverpool. 165, ISBN: 978-0-906370-63-6.
Knights, A. M., Koss, R. S., and Robinson, L. A. (2013). Identifying common pressure pathways from a complex network of human activities to support ecosystem-based management. Ecol. Appl. 23, 755–765. doi: 10.1890/12-1137.1
Knights, A. M., Piet, G. J., Jongbloed, R. H., Tamis, J. E., White, L., Akoglu, E., et al. (2015). An exposure-effect approach for evaluating ecosystem-wide risks from human activities. ICES J. Mar. Sci. 72, 1105–1115. doi: 10.1093/icesjms/fsu245
Korpinen, S., Meidinger, M., and Laamanen, M. (2013). Cumulative impacts on seabed habitats: an indicator for assessments of good environmental status. Mar. Pollut. Bull. 74, 311–319. doi: 10.1016/j.marpolbul.2013.06.036
Koutsidi, M., Tzanatos, E., Machias, A., and Vassilopoulou, V. (2016). Fishing for function: the use of biological traits to evaluate the effects of multispecies fisheries on the functioning of fisheries assemblages. ICES J. Mar. Sci. 73, 1091–1103. doi: 10.1093/icesjms/fsw006
Lambert, G. I., Jennings, S., Kaiser, M. J., Davies, T. W., and Hiddink, J. G. (2014). Quantifying recovery rates and resilience of seabed habitats impacted by bottom fishing. J. Appl. Ecol. 51, 1326–1336. doi: 10.1111/1365-2664.12277
Langmead, O., McQuatters-Gollop, A., and Mee, L. D. (2007). European Lifestyles and Marine Ecosystems: Exploring Challenges for Managing Europe's Seas. Plymouth: University of Plymouth Marine Institute.
Lewison, R. L., Rudd, M. A., Al-Hayek, W., Baldwin, C., Beger, M., Lieske, S. N., et al. (2016). How the DPSIR framework can be used for structuring problems and facilitating empirical research in coastal systems. Environ. Sci. Policy 56, 110–119. doi: 10.1016/j.envsci.2015.11.001
McCann, R. K., Marcot, B. G., and Ellis, R. (2006). Bayesian belief networks: applications in ecology and natural resource management. Can. J. For. Res. 36, 3053–3062. doi: 10.1139/x06-238
Micheli, F., Halpern, B. S., Walbridge, S., Ciriaco, S., Ferretti, F., Fraschetti, S., et al. (2013). Cumulative human impacts on mediterranean and black sea marine ecosystems: assessing current pressures and opportunities. PLoS ONE 8:e79889. doi: 10.1371/journal.pone.0079889
Mollet, F. M., Kraak, S. B. M., and Rijnsdorp, A. D. (2007). Fisheries-induced evolutionary changes in maturation norms in the North Sea sole Solea solea. Mar. Ecol. Progr. Ser. 351, 189–199. doi: 10.3354/meps07138
Muntadas, A., de Juan, S., and Demestre, M. (2015). Integrating the provision of ecosystem services and trawl fisheries for the management of the marine environment. Sci. Total Environ. 506–507, 594–603. doi: 10.1016/j.scitotenv.2014.11.042
Mylopoulos, J. (1992). “Conceptual modeling and Telos1,” in Conceptual Modeling, Databases, and Case: An Integrated View of Information Systems Development, eds P. Loucopoulos and R. Zicari (New York, NY: Wiley), 49–68.
Net Gain (2011). Net Gain Final Recommendations Submission to Natural England and JNCC. V 1.1. Hull: The North Sea Marine Conservation Zones Project.
Nõges, P., Argillier, C., Borja, Á., Garmendia, J. M., Hanganu, J., Kodeš, V., et al. (2016). Quantified biotic and abiotic responses to multiple stress in freshwater, marine and ground waters. Sci. Total Environ. 540, 43–52. doi: 10.1016/j.scitotenv.2015.06.045
OECD (1993). OECD Core Set of Indicators for Environmental Performance Reviews. A Synthesis Report by the Group on the State of the Environment. Paris: OECD.
O'Neill, F. G., and Ivanović, A. (2016). The physical impact of towed demersal fishing gears on soft sediments. ICES J. Mar. Sci. 73, i5–i14. doi: 10.1093/icesjms/fsv125
Palialexis, A., Tornero, V., Barbone, E., Gonzalez, D., Hanke, G., Cardoso, A. C., et al. (2014). In-Depth Assessment of the EU Member States' Submissions for the Marine Framework Strategy Framework Directive under Articles 8, 9 and 10 JRC Scientific and Technical Reports. EUR 26473. Publications Office of the European Union. 153.
Patrício, J., Little, S., Mazik, K., Papadopoulou, K., Smith, C. J., Teixeira, H., et al. (2016). European Marine Biodiversity Monitoring Networks: strengths, weaknesses, opportunities and threats. Front. Mar. Sci. 3:161. doi: 10.3389/fmars.2016.00161
Patrício, J., Little, S., Mazik, K., Thomson, S., Zampoukas, N., Teixeira, H., et al. (2014). Report on SWOT Analysis of Marine Monitoring Networks. DEVOTES FP7 Project Deliverable 1.4 (JRC89561). 100, Annexes. Available online at: http://www.devotes-project.eu/report-on-swot-analysis-of-monitoring/
Petihakis, G., Smith, C. J., Triantafyllou, G., Sourlantzis, G., Papadopoulou, K.-N., Pollani, A., et al. (2007). Scenario testing of fisheries management strategies using a high resolution ERSEM–POM ecosystem model. ICES J. Mar. Sci. 64, 1627–1640. doi: 10.1093/icesjms/fsm161
Piroddi, C., Teixeira, H., Lynham, C., Smith, C., Alvarez, M., Mazik, K., et al. (2015). Using ecosystem models to assess ecosystem status in support of the European Marine Strategy Framework Directive. Ecol. Indic. 58, 175–191. doi: 10.1016/j.ecolind.2015.05.037
Plagányi, É. E. (2007). Models for an Ecosystem Approach to Fisheries. FAO Fisheries Technical Paper. No. 477, Rome: FAO, 108.
Pollino, C. A., Woodberry, O., Nicholson, A., Korb, K., and Hart, B. T. (2007). Parameterisation and evaluation of a Bayesian network for use in an ecological risk assessment. Environ. Model. Softw. 22, 1140–1152. doi: 10.1016/j.envsoft.2006.03.006
Queiros, A. M., Hiddink, J. G., Kaiser, M. J., and Hinz, H. (2006). Effects of chronic bottom trawling disturbance on benthic biomass, production and size spectra in different habitats. J. Exp. Mar. Biol. Ecol. 335, 91–103. doi: 10.1016/j.jembe.2006.03.001
Raakjaer, J., van Leeuwen, J., van Tatenhove, J., and Hadjimichael, M. (2014). Ecosystem-based marine management in European regional seas calls for nested governance structures and coordination — A policy brief. Mar. Policy 50, 373–381. doi: 10.1016/j.marpol.2014.03.007
Rapport, D., and Friend, A. (1979). Towards a Comprehensive Framework for Environmental Statistics: A Stress-Response Approach. Statistics Canada Catalogue 11-510. Ottawa, ON: Minister of Supply and Services Canada.
Robinson, L. A., and Frid, C. L. J. (2003). Dynamic ecosystem models and the evaluation of ecosystem effects of fishing: can we make meaningful predictions? Aquat. Conserv. 13, 5–20. doi: 10.1002/aqc.506
Robinson, L. A., and Knights, A. M. (2011). ODEMM Pressure Assessment Userguide. ODEMM Guidance Document Series No. 2. EC FP7 Project (244272) ‘Options for Delivering Ecosystem-based Marine Management’. University of Liverpool. ISBN: 978-0-906370-62-9.
Robinson, L. A., Rogers, S., and Frid, C. L. J. (2008). A Marine Assessment and Monitoring Framework for Application by UKMMAS and OSPAR – Assessment of Pressures and Impacts. Phase II: Application for regional assessments. Joint Nature Conservation Committee contract No. C-08-0007-0027.
Rose, K. A., Allen, J. I., Artiol, Y., Barange, M., Blackford, J., Carlotti, F., et al. (2010). End-to-end models for the analysis of marine ecosystems: challenges, issues, and next steps. Mar. Coast. Fish. Dyn. Manage. Ecosyst. Sci 2, 115–130. doi: 10.1577/c09-059.1
Scharin, H., Ericsdotter, S., Elliott, M., Turner, R. K., Niiranen, S., Blenckner, T., et al. (2016). Processes for the sustainable stewardship of marine environments. Ecol. Econ. 128, 55–67. doi: 10.1016/j.ecolecon.2016.04.010
Smith, C., Papadopoulou, K.-N., Barnard, S., Mazik, K., Patrício, J., Elliott, M., et al. (2014). Conceptual Models for the Effects of Marine Pressures on Biodiversity. DEVOTES Deliverable 1.1. Devotes FP7 Project. 80. Available online at: www.devotes-project.eu/wp-content/uploads/2014/06/DEVOTESD1-1-ConceptualModels.pdf
Smith, K., and Petley, D. N. (2009). Environmental Hazards: Assessing Risk and Reducing Disaster, 5th Edn. London: Routledge.
Smyth, K., and Elliott, M. (2014). Develop Risk assessments leading to Best Practice: Resource Exploitation – Renewable Energy. Deliverable 60.5, VECTORS FP7 project, IECS, University of Hull, 65.
Snelgrove, P. V. R., and Butman, C. A. (1994). Animal-sediment relationships revisited: cause versus affect. Oceanogr. Mar. Biol. Ann. Rev. 32, 111–177.
Solan, M., and Whiteley, N. (2016). Stressors in the Marine Environment: Physiological and Ecological Responses; Societal Implications. Oxford: Oxford University Press. doi: 10.1093/acprof:oso/9780198718826.001.0001
Stelzenmuller, V., Fock, H. O., Gimpel, A., Rambo, H., Diekmann, R., Probst, W. N., et al. (2015). Quantitative environmental risk assessments in the context of marine spatial management: current approaches and some perspectives. ICES J. Mar. Sci. 72, 1022–1042. doi: 10.1093/icesjms/fsu206
Svarstad, H., Petersen, L. K., Rothman, D., Siepel, H., and Wätzold, F. (2008). Discursive biases of the environmental research framework DPSIR. Land Use Policy 25, 116–125. doi: 10.1016/j.landusepol.2007.03.005
Teichert, N., Borja, A., Chust, G., Uriarte, A., and Lepage, M. (2016). Restoring fish ecological quality in estuaries: implication of interactive and cumulative effects among anthropogenic stressors. Sci. Total Environ. 542(Pt A), 383–393. doi: 10.1016/j.scitotenv.2015.10.068
Thrush, S. F., Ellingsen, K. E., and Davis, K. (2016). Implications of fisheries impacts to seabed biodiversity and ecosystem-based management. ICES J. Mar. Sci. 73, i44–i50. doi: 10.1093/icesjms/fsv114
Tillin, H. M., Hiddink, J. G., Jennings, S., and Kaiser, M. J. (2006). Chronic bottom trawling alters the functional composition of benthic invertebrate communities on a sea-basin scale. Mar. Ecol. Prog. Ser. 318, 31–45. doi: 10.3354/meps318031
UNEP (1994). World environment outlook: brainstorming session. ENEP/EAMR. 94-5. Environ. Assessm. Prog. Nairobiol. 18 p.
Uusitalo, L., Korpinen, S., Andersen, J. H., Niiranen, S., Valanko, S., Heiskanen, A.-S., et al. (2015). Exploring methods for predicting multiple pressures on ecosystem recovery: a case study on marine eutrophication and fisheries. Cont. Shelf Res. 121, 48–60. doi: 10.1016/j.csr.2015.11.002
Uyarra, M. C., and Borja, Á. (2016). Ocean literacy: a ‘new’ socio-ecological concept for a sustainable use of the seas. Mar. Pollut. Bull. 104, 1–2. doi: 10.1016/j.marpolbul.2016.02.060
White, L. J., Koss, R. S., Knights, A. M., Eriksson, A., and Robinson, L. A. (2013). ODEMM Linkage Framework Userguide (Version 2). ODEMM Guidance Document Series No.3. EC FP7 project (244273) ‘Options for Delivering Ecosystem-based Marine Management’. University of Liverpool. ISBN: 978-0-906370-87-6, 14. Available online at: http://odemm.com/content/linkage-framework
Widdows, J., Bayne, B. L., Donkin, P., Livingstone, D. R., and Lowe, D. M. (1981). Measurement of the responses of mussels to environmental stress and pollution in Sullom Voe: a baseline study. Proc. R. Soc Edinb. B 80, 323–338. doi: 10.1017/s0269727000006631
Keywords: DPSIR, risk, pressure mechanisms, exogenic pressures, endogenic pressures, assessment, benthic trawling
Citation: Smith CJ, Papadopoulou K-N, Barnard S, Mazik K, Elliott M, Patrício J, Solaun O, Little S, Bhatia N and Borja A (2016) Managing the Marine Environment, Conceptual Models and Assessment Considerations for the European Marine Strategy Framework Directive. Front. Mar. Sci. 3:144. doi: 10.3389/fmars.2016.00144
Received: 03 June 2016; Accepted: 28 July 2016;
Published: 25 August 2016.
Edited by:
Jacob Carstensen, Aarhus University, DenmarkReviewed by:
Katherine Dafforn, University of New South Wales, AustraliaMichelle McCrackin, Stockholm University, Sweden
Copyright © 2016 Smith, Papadopoulou, Barnard, Mazik, Elliott, Patrício, Solaun, Little, Bhatia and Borja. This is an open-access article distributed under the terms of the Creative Commons Attribution License (CC BY). The use, distribution or reproduction in other forums is permitted, provided the original author(s) or licensor are credited and that the original publication in this journal is cited, in accordance with accepted academic practice. No use, distribution or reproduction is permitted which does not comply with these terms.
*Correspondence: Christopher J. Smith, Y3NtaXRoQGhjbXIuZ3I=