- 1Department of Biochemistry and Microbiology, University of Victoria, Victoria, BC, Canada
- 2Ocean Sciences Division, Institute of Ocean Sciences, Fisheries and Oceans Canada, Sidney, BC, Canada
Complexation by organic ligands dominates the speciation of iron (Fe), copper (Cu), and other bioactive trace metals in seawater, controlling their bioavailability and distribution in the marine environment. Several classes of high-affinity Fe-binding ligands (siderophores) have been identified in seawater but the chemical structures of marine Cu-complexing ligands remain unknown. Immobilized metal-ion affinity chromatography (IMAC) allows Cu ligands to be isolated from bulk dissolved organic matter (DOM) in seawater and separated into fractions, which can be characterized independently using electrochemical and spectroscopic techniques. Attempts have been made to combine IMAC with electrospray ionization mass spectrometry (ESI-MS) to characterize marine Cu ligands, but results have proven inconclusive due to the lack of tandem mass spectrometry (MS/MS) data to confirm ligand recovery. We used 8-hydroxyquinoline (8-HQ), a well-characterized model ligand that forms strong 1:2 metal:ligand complexes with Cu2+ at pH 8 (log β2 = 18.3), to evaluate Cu(II)-IMAC and ESI-MS/MS for recovery and identification of copper(II)-complexing ligands in seawater. One-liter samples of 0.45 μm-filtered surface seawater were spiked with 8-HQ at low concentrations (up to 100 nM) and fractionated by IMAC. Fractions eluted with acidified artificial seawater were desalted and re-suspended in methanol via solid-phase extraction (SPE) to obtain extracts suitable for ESI-MS analysis. Recovery of 8-HQ by Cu(II)-IMAC was confirmed unambiguously by MS/MS and found to average 81% based upon accurate quantitation via multiple reaction monitoring (MRM). Cu(II)-IMAC fractionation of unspiked seawater using multiple UV detection wavelengths suggests an optimal fraction size of 2 mL for isolating and analyzing Cu ligands with similar properties.
Introduction
Organic complexation of dissolved trace metals like iron (Fe) and copper (Cu) exerts a major influence on their mobility, toxicity, and bioavailability in the marine environment (Florence, 1982; Sunda, 1991; Donat and Bruland, 1995; Kraemer, 2004). Several classes of biogenic high-affinity Fe-binding ligands (siderophores) have been identified in seawater and are thought to facilitate iron uptake in marine bacterioplankton (Wilhelm, 1995; Hutchins et al., 1999; McCormack et al., 2003). Copper-carrying analogs (chalkophores) such as methanobactin (log KCuL ~20; El Ghazouani et al., 2011) are known to be produced by certain methanotrophic bacteria (Kim et al., 2004; Bandow et al., 2012). Culturing experiments have shown that marine phytoplankton are also capable of producing strong, extracellular Cu-binding ligands (McKnight and Morel, 1979, 1980; Moffett et al., 1990; Moffett and Brand, 1996; Leal et al., 1999; Croot et al., 2000; Dupont et al., 2004; Dupont and Ahner, 2005; Wiramanaden et al., 2008). Some of these exudates are thought to act like chalkophores (Semeniuk et al., 2015), promoting biological uptake and utilization of Cu for e.g., photosynthesis, radical detoxification, and iron acquisition (Maldonado et al., 2006). Others may be released to moderate concentrations of free, bioavailable Cu2+ ions (Dryden et al., 2004; Leão et al., 2007) that would otherwise be toxic to marine phytoplankton (Sunda, 1991). Although numerous studies have shown that Cu in seawater exists primarily in the form of organic complexes (see Donat and Bruland, 1995) no marine copper ligands have been positively identified.
Originally developed for protein isolation by Porath et al. (1975), immobilized metal-ion affinity chromatography (IMAC) was first applied to the study of marine Cu-complexing ligands by Gordon (1992). The principal advantage of Cu(II)-IMAC is that it enables compounds with an affinity for copper to be isolated from other organic compounds that could interfere with their analysis. IMAC is also capable of resolving compounds into fractions with differing affinities for copper, allowing electrochemical or spectroscopic analysis to be applied specifically to each fraction. Gordon (1992) successfully demonstrated concentration and partial purification of Cu-complexing organic compounds from the Sargasso Sea using IMAC with UV detection, resolving these compounds into two chromatographic peaks or fractions based on their relative affinities for copper. Vertical profiles showed that the lower-affinity (earlier-eluting) peak was invariant with depth whereas the higher-affinity (later-eluting) peak reached a maximum at 60 m. Subsequent applications of Cu(II)-IMAC to natural waters (Gordon et al., 1996, 2000; Midorikawa and Tanoue, 1996, 1998; Donat et al., 1997; Wu and Tanoue, 2001a,b; Paunovic et al., 2005, 2008) employed UV absorbance, fluorescence, chemical analysis, and electrochemical methods such as differential pulse anodic stripping voltammetry (DPASV) to characterize the recovered ligands. Two classes of marine Cu-complexing ligands have been distinguished: a stronger (L1) class (log K′ = 12 to 14) present at relative low concentrations (below 10 nM) and found primarily in surface waters, and a weaker (L2) class (log K′ = 9 to 12) found at higher concentrations (above 100 nM) and more broadly distributed in the water column (Vraspir and Butler, 2009). Donat et al. (1997) demonstrated removal of L1 and L2 from seawater by Cu(II)-IMAC and detection of L1 in pooled IMAC eluents. More recently, Cu(II)-IMAC has been used in combination with electrospray ionization mass spectrometry (ESI-MS) to isolate and identify intracellular copper-binding proteins from marine algal cells (Smith et al., 2014), some of which (e.g., chaperones) are thought to bind Cu+ in vivo.
Early attempts to characterize marine Cu-binding ligands using Cu(II)-IMAC and ESI-MS were successful in obtaining molecular weight information from seawater extracts (Ross et al., 2003; Vachet and Callaway, 2003), paving the way for structural analysis using tandem mass spectrometry (MS/MS) (Ross et al., 2000; Wiramanaden et al., 2008). However, results obtained using ESI-MS alone were insufficient to positively identify or optimize the recovery of marine Cu ligands. The latter is important given the typically low concentrations at which organic ligands are present in seawater, and the need for intermediate steps such as solid-phase extraction (SPE) to desalt and concentrate samples prior to MS analysis (Waska et al., 2015). We describe a method for recovery and structural characterization of Cu(II)-complexing ligands in seawater using IMAC, SPE, and ESI-MS/MS. We validate our approach by demonstrating recovery and unambiguous identification of a model organic Cu-binding ligand from seawater at low concentrations (below 100 nM). The compound chosen was 8-hydroxyquinoline (8-HQ), a well-characterized model ligand that is amenable to ESI-MS and forms strong 1:2 metal:ligand complexes with Cu2+ at pH 8 (log β2 = 18.3; Perrin, 1979; Ross et al., 1998). The ability of 8-HQ to form uncharged, lipophilic complexes makes it a viable model for biological metal-ion carriers (Gârban et al., 2014) as well as for marine Cu-complexing ligands. We also present Cu(II)-IMAC data for oceanic and coastal surface waters using multiple detection wavelengths, which enable fractionation of marine Cu ligands based on UV absorbance and IMAC retention times.
Materials and Methods
Reagents
All reagents and solvents were analytical and high-performance liquid chromatography (HPLC) grade, respectively, unless otherwise noted. Hydrochloric acid (12N) was obtained from Anachemia (Vancouver, BC), acetonitrile and methanol from VWR (Mississauga, ON), and ethylenediamine tetraacetic acid (EDTA) and ammonium acetate from Fisher (Pittsburgh, PA). Copper (II) sulfate (99.995% trace metal free), 8-hydroxyquinoline, glutathione, histidine, and tryptophan were obtained from Sigma-Aldrich (St. Louis, MO). Ultrapure deionized water (16–18 MΩ-cm) was prepared using an EMD Super-Q system from Millipore (Billerica, MA). Artificial seawater (ASW) was prepared by dissolving 32 g of Instant Ocean (Blacksburg, VA) in 1 L of ultrapure water, and acidified (aASW) by adding HCl to a concentration of 10 mM (pH 2.1).
Collection of Seawater Samples
Natural seawater was collected from inshore coastal and open ocean surface waters of the northeast Pacific. Oceanic surface seawater (OSW) was collected at a depth of 5 m near station P22 (49° 41.92′ N, 140° 39.98′ W) during the June 2015 Line-P cruise aboard the CCGS John P. Tully and transferred directly to a 50-gallon plastic drum using a high-volume Teflon® pump (model PFD1 210, Saint-Gobain ASTI, Nanterre, France). Coastal surface seawater (CSW) was collected in plastic tubs lowered by hand from the dock at the Institute of Ocean Sciences (IOS), Sidney, British Columbia during the spring and summer of 2015. Samples from both sources were stored unfiltered in the dark at room temperature, and were pumped through a 0.45-μm filter (GWV high-capacity groundwater sampling capsule, Pall Corporation, Port Washington, NY) prior to any treatment or analysis.
Immobilized Copper(II)-Ion Affinity Chromatography
Cu(II)-IMAC involves passing filtered aqueous samples through a chelating column charged with Cu2+ ions, which are immobilized by tethered functional groups such as iminodiacetate (IDA) or nitrilotriacetate (NTA). Compounds with an affinity for divalent copper are selectively retained on the column, then eluted and recovered using an acidic or competing ligand solution. We performed Cu(II)-IMAC fractionations using two systems. The first was a manually operated dual-column system built in-house during previous studies (Ross et al., 2003) utilizing a single wavelength UV detector (LKB Bromma Uvicord S, Pharmacia Biotech, Uppsala, Sweden), henceforth referred to as the LKB system. The second system was an automated single-column commercial unit developed primarily for protein purification (AKTA PrimePlus, GE Healthcare, Mississauga, ON), which we refer to as the AKTA system. This system was supplied with multiple UV filters and software (PrimeView v5.31) for chromatographic analysis. Both systems were fitted with 5-mL Hi-Trap Chelating Sepharose HP columns (part no. 17-0409-03, GE Healthcare).
The same experimental protocol was applied to each system, all reagents and samples being delivered at a flow rate of 0.9 mL/min (LKB) or 1.0 mL/min (AKTA). Seawater samples and the solutions used to condition, charge, elute and regenerate the IMAC columns were prepared in 1-L acid-washed high-density polyethylene (Nalgene®) bottles. After rinsing with 30 mL of deionized water the Hi-Trap columns were charged with 50 μmoles of Cu2+ ions by passing 5 mL of a 10 mM copper(II) sulfate solution through each column. The columns were rinsed with 5 mL of ultrapure water and equilibrated with 20–30 mL of acidified artificial seawater (aASW). One-liter samples of natural or artificial seawater, with or without added model ligands, were then passed through each column. When the sample containers were nearly empty, desorption of retained compounds was achieved by eluting the columns with aASW for 20–30 min, during which the UV absorbance of the eluent was monitored at 254 nm (both systems), 214 or 280 nm (AKTA only). Eluent fractions corresponding to major chromatographic peaks were collected in glass scintillation vials (Wheaton, Millville, NJ) and stored at 5°C. The absorbance spectra of selected IMAC fractions were obtained using a UV-Vis spectrophotometer (model 8453, HP Agilent, Mississauga, ON) to confirm the extraction of model ligands. Following elution the IMAC columns were regenerated by passing 50 mM EDTA in ultrapure water through each column for 15 min to remove the Cu2+ ions before repeating the procedure, at all times taking care to avoid entraining air into the system.
Solid-Phase Extraction
We used SPE for off-line desalting of IMAC fractions as this method has the potential to recover unknown organic compounds of varying chemical composition. SPE was performed using a RapidTrace Workstation (Caliper Life Science, Hopkinton, MA) and Oasis 3 cc HLB (hydrophilic-lipophilic) cartridges (Waters Limited, Mississauga, ON). The cartridges contained a polymeric reversed-phase sorbent designed to extract a wide range of acidic, basic, and neutral compounds. HLB cartridges were conditioned with one volume (3 mL) of methanol and rinsed with 3 mL ultrapure water and 3 mL aASW (the IMAC eluent) before loading 2–4 mL of IMAC fraction. Loaded cartridges were rinsed with 3 mL aASW and 3 mL of 5% (v/v) methanol in ultrapure water before eluting with 2 mL methanol, which provided the highest concentration of recovered ligand. The cartridges were then regenerated with 3 mL of methanol and 5 mL ultrapure water, followed by a blank extraction using unspiked aASW to minimize carryover between IMAC fractions (see Section Characterization of Natural Ligands below). Extracts were collected in polypropylene culture tubes (VWR) and stored at 5°C prior to ESI-MS analysis. Selected extracts were also analyzed by ESI-MS to confirm and measure the recovery of model ligands using standards prepared in methanol.
Mass Spectrometry
Model ligand standard solutions and SPE extracts were analyzed by flow-injection (FI) ESI-MS using a tandem quadrupole mass spectrometer (model API 5000, AB Sciex) fitted with an electrospray ion source (Turbo Ionspray; AB Sciex) connected to a high-performance liquid chromatography (HPLC) pump and autosampler (model P680 and ASI-100, Dionex). ESI-MS parameters were optimized using the Taguchi method (Taguchi and Yokoyama, 1994) while infusing a 0.68 μM solution of 8-HQ in the FI carrier solvent at 100 μL/min using a syringe pump (Harvard Instruments). The carrier solvent consisted of 1 mM ammonium acetate in 70% (v/v) aqueous acetonitrile (pH 7.6; Ross et al., 2003). Optimal ESI-MS conditions were: DP 120, EP 10, CAD 5, CUR 30, GS1 60, GS2 30, and IS 5500. Fragmentation of the 8-HQ molecular or precursor ion of mass-to-charge ratio (m/z) 146.2 to produce diagnostic fragment or product ions via collision induced dissociation (CID) tandem mass spectrometry (MS/MS) was optimized using the same solution. Two precursor-to-product ion transitions corresponding to loss of water (m/z 146.2 > 128.2) and formation of the tropylium ion (m/z 146.2 > 91.2) from 8-HQ were selected for quantification and confirmation, respectively, via multiple reaction monitoring (MRM) using the instrument software (Analyst v1.6.2). MRM provides specific, sensitive, and accurate quantification of target compounds during FI (or on-line HPLC) analysis. Optimal MRM conditions were CE 40, CXP 19 (146.2 > 91.2), and CE 35, CXP 24 (146.2 > 128.2). Standard solutions containing 0.5–50 μM 8-HQ were prepared by serial dilution of a stock solution containing 100 μM 8-HQ in methanol (the SPE eluent). The system was calibrated for FI-ESI-MS/MS analysis of SPE extracts using the HPLC pump and autosampler to deliver 10 μL of each 8-HQ standard to the ESI source at flow rate of 100 μL/min using the FI carrier solvent. Refrigerated SPE extracts were allowed to equilibrate at room temperature, filtered through a 0.22-μm syringe filter (part no. 179718, Canadian Life Science, Peterborough ON), transferred to 2-mL glass vials (Sigma), loaded into the autosampler, and analyzed by MRM under the same conditions as the calibration standards.
Treatment of Seawater Samples
The properties of natural copper-binding compounds were investigated using data from replicate Cu(II)-IMAC experiments. Retention times and peak areas for coastal and oceanic surface seawater samples were compared using different UV detection wavelengths (214, 254, and 280 nm) before and after different treatments. The photochemical and thermal stabilities of natural ligands were examined by placing oceanic seawater samples in water-cooled 1-L PTFE containers within an artificial sun chamber (~700 W/m2; Suntest CPS, Atlas, Mount Prospect, IL) designed to reproduce the spectrum of natural sunlight, with minimal transmission below 280 nm, or immersing and heating them in a water bath (~60°C; Isotemp 3016D, Fisher Scientific) for up to 24 h prior to IMAC. The effects of ionic strength and prior complexation on the recovery of natural ligands were also examined by decreasing salinity with ultrapure deionized water, increasing it to approximately 38‰ with artificial sea salt (Instant Ocean), or spiking the seawater sample with copper(II) sulfate to a concentration of 100 nM and allowing it to equilibrate for several hours before IMAC fractionation.
Results
Immobilized Copper(II)-Ion Affinity Chromatography
UV spectrophotometric analysis of 8-HQ standards and OSW samples spiked with 8-HQ showed that the model ligand absorbs strongly at 254 nm, as noted previously (Zhang et al., 1996), and that the absorbance at this wavelength is proportional to ligand concentration. Using this wavelength to monitor the elution of Cu(II)-IMAC extracts from OSW samples spiked with up to 100 nM 8-HQ produced chromatograms with two significant peaks (Figure 1). The earlier-eluting peak remained essentially unchanged whereas the later-eluting peak increased in area with increasing 8-HQ concentration (r2 = 0.9927). Chromatograms obtained during Cu(II)-IMAC of ASW spiked with 8-HQ contained only the later-eluting peak, while UV absorbance spectra of collected fractions corresponding to this peak matched those of standards contained 8-HQ in aASW (the IMAC eluent).
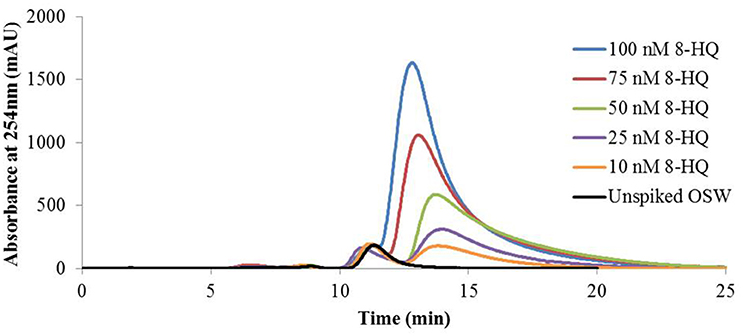
Figure 1. Immobilized copper(II)-ion affinity chromatography (Cu(II)-IMAC) of oceanic surface seawater (OSW) spiked with the model ligand 8-hydroxyquinoline (8-HQ). One-liter OSW samples containing up to 100 nmoles of added 8-HQ were fractionated by Cu(II)-IMAC while monitoring UV absorbance of the eluent at 254 nm using the LKB system (see text). All samples gave rise to a peak at 11 min, attributed to natural copper ligands present in OSW, whereas samples spiked with 8-HQ gave rise to a second elution peak at 12–14 min with area proportional to the amount of ligand added to the OSW sample (r2 = 0.9927).
Our decision to elute the IMAC column with acidified seawater (Midorikawa and Tanoue, 1996) was based on the need to avoid introducing large concentrations of a competing ligand such as glycine, which would interfere with subsequent ESI-MS analysis, while ensuring that our results could be used to validate those of earlier experiments performed using the same eluent (Ross et al., 2003). Previous studies (Lohan et al., 2005; Milne et al., 2010) suggest that eluting Cu(II)-IMAC columns with acidic solution may displace copper from the column, making it available to form complexes with ligands recovered in the eluent. Because this may affect the quantification of 8-HQ in IMAC fractions using UV absorbance spectrophotometry we relied upon specific, accurate quantification by MRM (see Section Electrospray-Ionization Mass Spectrometry below) to estimate the recovery of model ligands during IMAC.
Solid-Phase Extraction
Since marine Cu ligands have not yet been identified we chose to evaluate a type of SPE cartridge (Waters Oasis HLB) designed to recover as broad a range of organic compounds as possible. SPE conditions were optimized using FI-ESI-MS/MS with MRM to quantify 8-HQ in methanol extracts collected following SPE of 10 μM 8-HQ standards prepared in 2 mL of aASW (the IMAC eluent). The dependence of SPE recovery on elution volume was investigated by using different amounts of methanol (0.5–3.0 mL) to elute the HLB cartridge, and MRM to quantify the amount of 8-HQ in the resulting extracts. Recoveries ranged from less than 10% using 0.5 mL to 90% using 3 mL of methanol. However, the highest concentration of 8-HQ in the SPE extract was obtained using the recommended elution volume of 2 mL (Waters Corporation, 2014), which gave an average recovery (n = 4) of 74.4 ± 2.3 %. Rinsing the eluted HLB cartridge with methanol did not appear to retrieve any residual 8-HQ. During preliminary experiments, however, recoveries obtained after extracting several samples using the same cartridge were found to be greater than those obtained using a new cartridge. Carryover was henceforth minimized by performing blank extractions with 2 mL of unspiked aASW between samples. Cu(II)-IMAC fractions were desalted and concentrated for FI-ESI-MS/MS analysis using the optimized SPE protocol, applying a value of 74.4% to correct for recovery during SPE (Table 1).
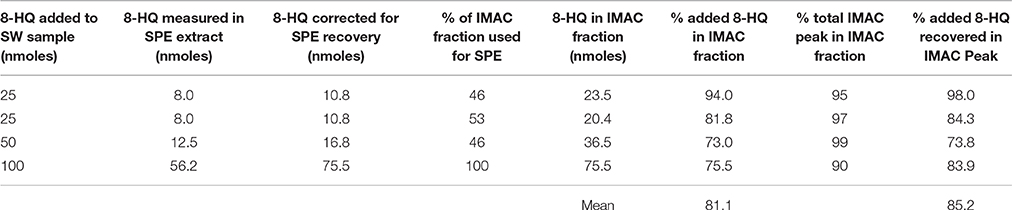
Table 1. Recovery of the model ligand 8-hydroxyquinoline (8-HQ) from seawater by immobilized copper(II)-ion affinity chromatography (IMAC) and solid-phase extraction (SPE).
Electrospray-Ionization Mass Spectrometry
ESI-MS analysis of 8-HQ in methanol detected the protonated molecular ion at m/z 146.2. This was fragmented inside the collision cell of the mass spectrometer to generate a characteristic product-ion MS/MS spectrum for the model ligand (Figure 2). Two discrete and relatively abundant fragments (m/z 128.2 and 91.2) were selected for MRM. The instrument was first calibrated using standards containing 0.5–50 μM 8-HQ in methanol (the SPE eluent). These were analyzed in quadruplicate and the results averaged to generate a linear plot (r2 = 0.9794) of MRM peak area vs. 8-HQ concentration. The lower limit of quantification (LLOQ) was determined by performing replicate analyses (n = 4) of the solvent blank, calculating the standard deviation of these measurements, multiplying by 10, and adding this value to the average blank response. The result was substituted into the linear calibration equation to determine a LLOQ of 0.54 μM.
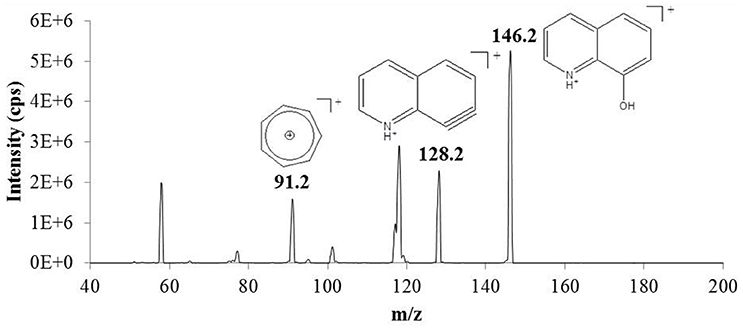
Figure 2. Product-ion mass spectrum of the protonated molecular/precursor ion (m/z 146.2) of 8-hydroxyquinoline (8-HQ) obtained by electrospray ionization-tandem mass spectrometry (ESI-MS/MS). Precursor-to-product ion transitions corresponding to loss of water (m/z 146.2 > 128.2) and formation of the stable tropylium ion (m/z 146.2 > 91.2) were selected for multiple reaction monitoring (MRM) to provide accurate quantification and unambiguous identification of 8-HQ in methanol extracts obtained by solid phase extraction (SPE) (see text).
The calibrated FI-ESI-MS/MS system was used to analyze Cu(II)-IMAC fractions obtained from 1-L seawater samples spiked with up to 100 nmoles 8-HQ (Figure 3). Broadening of the IMAC peak at higher concentrations (Figure 1) meant that the volume of the collected fraction varied from 1 to 10 mL, with a maximum of 4 mL being used for SPE. Variable volumes were accounted for by applying the appropriate corrections during calculation of the 8-HQ concentration in each IMAC fraction. For example, if a 1-L seawater sample spiked with 100 nmoles 8-HQ gave rise to a collected peak fraction of 8 mL, and 4 mL of that fraction were subjected to SPE, the measurement of 25 nmoles 8-HQ in the resulting SPE extract by MRM would indicate that 25% of the spiked ligand had been recovered in the SPE extract and 67% in the original IMAC fraction, following correction for 74.4% recovery during SPE (see Solid-Phase Extraction above). IMAC fractions collected from OSW spiked with 25–100 nmoles 8-HQ were subsequently found to contain an average of 81 ± 8% of the initial spike (Table 1). By applying a further correction based on the total area of the observed IMAC peak and the area corresponding to the fraction actually collected, it was possible to estimate a total recovery of 85 ± 9% for the initial Cu(II)-IMAC step (Table 1).
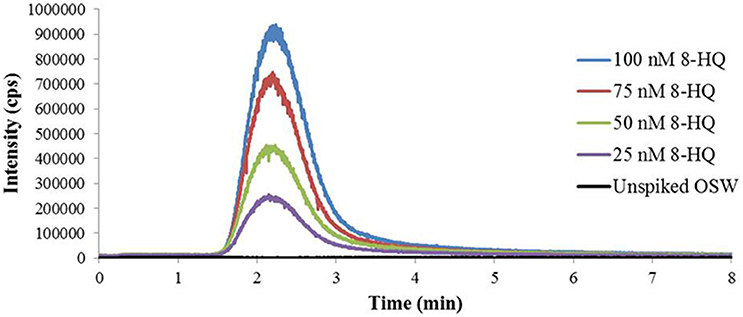
Figure 3. Multiple reaction monitoring (MRM) chromatograms for the m/z 146.2 > 91.2 transition used to quantify the model ligand 8-hydroxyquinoline (8-HQ) in Cu(II)-IMAC fractions desalted by solid-phase extraction (SPE). The fractions correspond to the model ligand peaks observed in the Cu(II)-IMAC chromatogram obtained by fractionating 1-L surface seawater samples spiked with 0–100 nmoles 8-HQ. Ten microliter of each SPE extract were analyzed by flow-injection electrospray ionization tandem mass spectrometry (FI-ESI-MS/MS) using 1 mM ammonium acetate in 70% acetonitrile at 100 μL/min as the carrier solvent.
Characterization of Natural Ligands
Cu(II)-IMAC was used to investigate the properties of natural copper ligands from coastal (CSW) and OSW by fractionating unspiked seawater samples and monitoring the IMAC eluent at different wavelengths (214, 254, or 280 nm) using the AKTA system. A representative IMAC chromatogram for CSW (Figure 4A) shows that the natural ligands absorbing most strongly at each wavelength had different retention times, and that peak area was greater for ligands absorbing primarily at 214 nm than for those absorbing at 254 or 280 nm (although differences in molar absorptivity for individual chromophores preclude any inference as to relative ligand concentrations). Similar results were obtained for OSW samples; however, peak area was greater for CSW than for OSW samples (Figure 4B). Retention times for extracted ligands that absorbed most strongly at a particular wavelength were consistent between samples, but those absorbing primarily at 280 nm had longer retention times (Figure 4C). To investigate the thermal and photochemical stabilities of natural ligands, OSW samples were exposed to heat or artificial sunlight prior to Cu(II)-IMAC. Irradiation of the samples for 24 h decreased the area of the predominant IMAC peak by 40% whereas incubation at 60°C for 24 h without irradiation reduced peak area by 15%. In contrast, changing the salinity of seawater samples or spiking them with Cu2+ ions to a concentration of 100 nM had no significant effect on peak area.
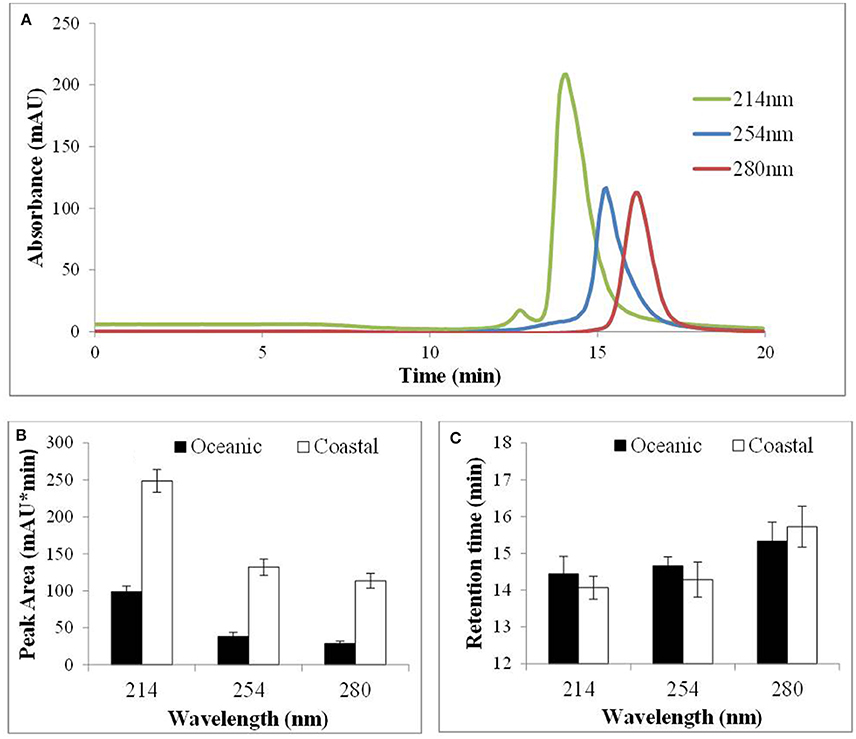
Figure 4. Immobilized copper(II)-ion affinity chromatography of coastal (CSW) and oceanic surface seawater (OSW) samples using the AKTA system (see text) to monitor the elution of replicate samples at 214, 254, and 280 nm. (A) Extracted compounds that absorb most strongly at each wavelength were found to elute at different times. (B) Peak areas were reproducible (n = 10; error bars represent 1 SD) and were consistently greater for coastal than for oceanic surface seawater. (C) Retention times were significantly greater for compounds that absorbed most strongly at 280 nm but were no different for CSW than for OSW samples.
Discussion
Cu(II)-IMAC fractionation of surface seawater samples spiked with 8-HQ gave rise to a UV chromatographic peak with a retention time greater than that observed for natural Cu ligands (Figure 1). The area of this peak was proportional to the mass of 8-HQ originally added to the seawater sample. Analysis of collected IMAC fractions by SPE and FI-ESI-MS/MS confirmed that IMAC fractions corresponding to the model ligand peak contained 8-HQ at a concentration proportional to that in the original sample, with an average recovery of 81%. To our knowledge this is the first time that a model Cu-binding ligand has been recovered, positively identified, and accurately quantified in seawater by MS/MS. Our results validate the previous assignment of measured molecular weights to marine Cu-complexing ligands using an earlier version of the same method (Ross et al., 2003). The product-ion spectrum for 8-HQ (Figure 2) also serves to illustrate the kind of structural information that MS/MS can provide for ligands isolated using this method. The order in which model and natural ligands elute from the Cu(II)-IMAC column is consistent with a higher stability constant for the Cu(II) complex of 8-HQ (see Section Introduction). Indeed, the greater binding strength of 8-HQ forms the basis for its use in determining total Cu concentrations in seawater by cathodic stripping voltammetry (CSV) and other techniques (Watanabe et al., 1981; Van Den Berg, 1986; Coale et al., 1992). Results obtained using 8-HQ suggest that natural Cu ligands of similar binding strength (log K′ ~18) may be recovered from seawater using Cu(II)-IMAC, assuming that they are able to form stable ternary complexes with IDA-Cu2+ (Paunovic et al., 2005)
The LLOQ of 0.54 μM achieved using MRM is equivalent to 1.08 nmoles of 8-HQ in a 2-mL SPE extract. Assuming that 100% of the collected IMAC fraction is used for SPE this corresponds to an original concentration of about 1.8 nM in a 1-L seawater sample, based upon 74.4 % recovery during SPE and 81.1 % during IMAC. Although this is on the order of concentrations measured voltametrically for the stronger L1 class of marine Cu ligands, detection and analysis of a specific ligand by ESI-MS/MS will depend on its individual concentration in seawater. Should greater sensitivity be required the concentration of methanol extracts obtained by SPE can be increased by partial evaporation of the solvent prior to analysis. Pooling Cu(II)-IMAC fractions from replicate seawater samples could also be used to increase the quantity of isolated ligands.
Adding 100 nM copper(II) sulfate to spiked and unspiked OSW did not affect the area of the model or natural ligand peaks observed during IMAC, suggesting that prior complexation with Cu in seawater has no significant effect on ligand recovery (see also Paunovic et al., 2005). Similarly, reducing the sample flow to 0.5 mL/min produced only a slight increase in the areas of model and natural ligand peaks. This suggests that the rates of exchange within the IMAC column are sufficient to achieve good recovery of Cu ligands at 1.0 mL/min despite the relatively short contact time with the sample (~5 min), as indicated by results for 8-HQ (Table 1). Repeated fractionation of seawater samples has been shown to provide incremental increases in the recovery of natural ligands (Donat et al., 1997). However, we found that running seawater samples through the column a second time produced a peak no more than 0.15 times as large as that obtained during the initial extraction, which nevertheless accounts for the incomplete recovery of 8-HQ observed during IMAC (Table 1).
Having evaluated Cu(II)-IMAC for recovery of Cu ligands we proceeded to investigate the fractionation of natural Cu ligands in unspiked CSW and OSW, using the AKTA system to monitor different UV wavelengths. The peak area at a given wavelength and retention time was always greater for coastal that for oceanic samples (Figure 4B). Results obtained previously for northeast Pacific coastal waters, using the more sensitive LKB system (Ross et al., 2003), showed that the IMAC peak corresponding to stronger Cu ligands reduced in size with increasing depth and distance from the mouth of the Fraser River. This is consistent with the production of strong biogenic Cu ligands in surface waters and with riverine input of organic ligands from terrestrial sources, both of which suggest that ligand concentrations are likely to be higher in CSW than in OSW samples. Chromatograms observed for CSW and OSW were consistent for a given wavelength and location, each chromatogram containing a single predominant peak. If this peak represented a single compound, or class of compounds, we would expect peak area to change according to the UV absorbance characteristics of the ligand(s) but the retention time to remain unaltered. This is exactly what we observed for the model ligand 8-HQ in spiked seawater (data not shown). For unspiked surface seawater, however, the retention time for the most abundant ligand peak changed with the detection wavelength (Figures 4A,C).
Gordon et al. (1996) found that the main difference between the two major peaks observed during Cu(II)-IMAC of estuarine samples was that the later-eluting peak absorbed strongly at 280 nm, which they attributed to the presence of proteins or peptides containing aromatic amino acids. They also found that the size of the earlier-eluting peak was reduced by prior treatment with the hydrophobic resin XAD-16. This was attributed to the presence of humic substances, which are known to absorb strongly at 254 nm (Rodrigues et al., 2009). The relative retention times of the peaks detected at 254 and 280 nm in our CSW and OSW samples appear to be consistent with these findings. However, we also observed an earlier-eluting peak using a detection wavelength of 214 nm (Figure 4A), which is characteristic of the peptide bonds found in all proteins. Furthermore, of the two retained fractions observed by Paunovic et al. (2005) in terrestrial organic matter, the earliest-eluting fraction was attributed to peptides and proteins and the later-eluting fraction to aromatic substances, based on comparisons with model ligands. Recovery and analysis of these fractions using a specific detection method like ESI-MS/MS would help to resolve any uncertainties as to the nature of the ligands present. Our results for OSW and CSW suggest that using multiple detection wavelengths can assist in identifying such fractions, and that 2 mL is an appropriate fraction size for capturing ligands with similar UV absorption and Cu-binding characteristics (Figures 4A,C).
Paunovic et al. (2005) suggest that IMAC is an effective tool for fractionating Cu ligands capable of forming ternary complexes with Cu2+ and IDA. Although our results for the bi-dentate ligand 8-HQ support this conclusion, we also performed Cu(II)-IMAC on OSW before and after spiking with the hexa-dentate model ligand EDTA, which absorbs at 214 nm in the IMAC eluant. The resulting (214 nm) UV chromatograms were identical, suggested that EDTA was unable to form stable ternary complex with IDA-Cu2+. Previous studies have shown that both stronger (L1) and weaker (L2) natural ligands are recovered from seawater by IDA-Cu2+ (Donat et al., 1997). However, results for EDTA suggest that recovery of natural multi-dentate ligands including, potentially, chalkophores like methanobactin (Kim et al., 2004) may be limited by steric factors. Nevertheless, model natural ligands tryptophan, histidine, and glutathione were recovered from seawater by Cu(II)-IMAC during preliminary experiments, as indicated by the appearance of additional chromatographic peaks which increased in area with increasing ligand concentration (not shown). The recovery and analysis of natural ligands by SPE and FI-ESI-MS/MS is currently being investigated, along with the use of phytoplankton cultures to produce Cu ligands for further method development and analysis.
Conclusion
A method for isolating, identifying, and quantifying Cu(II)-complexing ligands in seawater has been developed. The method, which employs Cu(II)-IMAC fractionation, SPE recovery, and FI-ESI-MS/MS analysis, was evaluated using the strong model ligands 8-HQ and EDTA. An average recovery of 81% was obtained for the bi-dentate ligand 8-HQ, which was positively identified and quantified in seawater by MRM. The hexa-dentate ligand EDTA was not recovered by Cu(II)-IMAC, suggesting that natural multi-dentate ligands may be under-represented by this method. Cu(II)-IMAC of surface seawater samples using multiple detection wavelengths showed that natural Cu ligands can be resolved into fractions suitable for SPE based on UV absorption and retention time. Identification of these natural ligands by FI-ESI-MS/MS is currently being investigated.
Author Contributions
The Corresponding Author secured funding for the work and is primarily responsible for its design and conception. The First Author is responsible for acquiring and analyzing the data. Both authors interpreted the data, revised the work for intellectual content, approved the final version, and agree to be accountable for all aspects of the work, including its accuracy and integrity.
Funding
Student funding was provided by the NSERC Climate Change and Adaptation Research (CCAR) program in the form of a grant for the Canadian Arctic GEOTRACES program (Application No. 433848-2012) and by the University of Victoria Department of Biochemistry and Microbiology. Additional operational funding was provided by Fisheries and Oceans Canada.
Conflict of Interest Statement
The authors declare that the research was conducted in the absence of any commercial or financial relationships that could be construed as a potential conflict of interest.
Acknowledgments
The key contributions to this research of two University of Victoria undergraduate co-op students, Crystal Sommer and Jose Mendez Campos, are gratefully acknowledged. The authors also wish to thank Alex Hodge for the design and construction of a computer interface for the LKB system, Kyle Simpson for his technical expertise and advice, and Prof. Jay Cullen at the University of Victoria for his invaluable assistance with equilibrium modeling. Finally the authors wish to thank the two reviewers, whose detailed and insightful comments resulted in major improvements to the original manuscript.
References
Bandow, N., Gilles, V. S., Freesmeier, B., Semrau, J. D., Krentz, B., Gallagher, W., et al. (2012). Spectral and copper binding properties of methanobactin from the facultative methanotroph Methylocystis strain SB2. J. Inorg. Biochem. 110, 72–82. doi: 10.1016/j.jinorgbio.2012.02.002
Coale, K. H., Johnson, K. S., Stout, P. M., and Sakamoto, C. M. (1992). Determination of copper in sea water using a flow-injection method with chemiluminescence detection. Anal. Chim. Acta 266, 345–351. doi: 10.1016/0003-2670(92)85062-B
Croot, P. L., Moffett, J. W., and Brand, L. E. (2000). Production of extracellular Cu complexing ligands by eucaryotic phytoplankton in response to Cu stress. Limnol. Oceanogr. 45, 619–627. doi: 10.4319/lo.2000.45.3.0619
Donat, J. R., and Bruland, K. W. (1995). Trace Elements in the Oceans, in Trace Elements in Natural Waters, 247–280. Boca Raton, FL: CRC Press.
Donat, J. R., Kango, R. A., and Gordon, A. S. (1997). Evaluation of immobilized metal affinity chromatography for isolation and recovery of strong copper-complexing ligands from marine waters. Mar. Chem. 5, 1–10. doi: 10.1016/S0304-4203(97)00008-X
Dryden, C. L., Gordon, A. S., and Donat, J. R. (2004). Interactive regulation of dissolved copper toxicity by an estuarine microbial community. Limnol. Oceanogr. 49, 1115–1122. doi: 10.4319/lo.2004.49.4.1115
Dupont, C. L., and Ahner, B. A. (2005). Effects of copper, cadmium, and zinc on the production and exudation of thiols by Emiliania huxleyi. Limnol. Oceanogr. 50, 508–515. doi: 10.4319/lo.2005.50.2.0508
Dupont, C. L., Nelson, R. K., Bashir, S., Moffett, J. W., and Ahner, B. A. (2004). Novel copper-binding and nitrogen-rich thiols produced and exuded by Emiliania huxleyi. Limnol. Oceanogr. 49, 1754–1762. doi: 10.4319/lo.2004.49.5.1754
El Ghazouani, A. I., Basle, A., Firbank, S. J., Knapp, C. W., Gray, J., Graham, D. W., et al. (2011). Copper-binding properties and structures of methanobactins from Methylosin trichosporium OB3b. Inorg. Chem. 50, 1378–1391. doi: 10.1021/ic101965j
Florence, T. M. (1982). The speciation of trace elements in water. Talanta 29, 345–364 doi: 10.1016/0039-9140(82)80169-0
Gârban, Z., Silaghi-Dumitrescu, R., Gârban, G., Avacovici, A., Hadaruga, N., Balta, C., et al. (2014). Metallomics related to gallium compounds: biochemical and xenobiochemical aspects. Macedonian J. Chem. Chem. Eng. 1, 39–52. doi: 10.20450/mjcce.2014.131
Gordon, A. S. (1992). Isolation of compounds with affinity for copper from seawater using immobilized copper ion affinity chromatography. Mar. Chem. 38, 1–12. doi: 10.1016/0304-4203(92)90063-G
Gordon, A. S., Donat, J. R., Kango, R. A., Dyer, B. J., and Stuart, L. M. (2000). Dissolved copper-complexing ligands in cultures of marine bacteria and estuarine water. Mar. Chem. 70, 149–160. doi: 10.1016/S0304-4203(00)00019-0
Gordon, A. S., Dyer, B. J., Kango, R. A., and Donat, J. R. (1996). Copper ligands isolated from estuarine water by immobilized metal affinity chromatography: temporal variability and partial characterization. Mar. Chem. 53, 163–172. doi: 10.1016/0304-4203(96)00022-9
Hutchins, D. A., Witter, A. E., Butler, A., and Luther, G. W. III. (1999). Competition among marine phytoplankton for different chelated iron species. Nature 400, 858–861. doi: 10.1038/23680
Kim, H. J., Graham, D. W., DiSpirito, A. A., Alterman, M. A., Galeva, N., Larive, C. K., et al. (2004). Methanobactin, a copper-acquisition compound from methane-oxidizing bacteria. Science 305, 1612–1615. doi: 10.1126/science.1098322
Kraemer, S. M. (2004). Iron oxide dissolution and solubility in the presence of siderophores. Aquat. Sci. 66, 3–18. doi: 10.1007/s00027-003-0690-5
Leal, M. F. C., Vasconcelos, M. T. S. D., and van den Berg, C. M. G. (1999). Copper-induced release of complexing ligands similar to thiols by Emiliania huxleyi in seawater cultures. Limnol. Oceanogr. 44, 1750–1762. doi: 10.4319/lo.1999.44.7.1750
Leão, P. N., Vasconcelos, M. T., and Vasconcelos, V. M. (2007). Role of marine cyanobacteria in trace metal bioavailability in seawater. Microb. Ecol. 53, 104–109. doi: 10.1007/s00248-006-9153-6
Lohan, M. C., Aguilar-Islas, A. M., Franks, R. P., and Bruland, K. W. (2005). Determination of iron and copper in seawater at pH 1.7 with a new commercially available chelating resin, NTA Superflow. Anal. Chim. Acta 530, 121–129. doi: 10.1016/j.aca.2004.09.005
Maldonado, M. T., Allen, A. E., Chong, J. S., Lin, K., Leus, D., Karpenko, N., et al. (2006). Copper-dependent iron transport in coastal and oceanic diatoms. Limnol. Oceanogr. 51, 1729–1743. doi: 10.4319/lo.2006.51.4.1729
McCormack, P., Worsfold, P. J., and Gledhill, M. (2003). Separation and detection of siderophores produced by marine bacterioplankton using high-performance liquid chromatography with electrospray ionization mass spectrometry. Anal. Chem. 75, 2647–2652. doi: 10.1021/ac0340105
McKnight, D. M., and Morel, F. M. M. (1979). Release of weak and strong copper-complexing agents by algae. Limnol. Oceanogr. 24, 823–837. doi: 10.4319/lo.1979.24.5.0823
McKnight, D. M., and Morel, F. M. M. (1980). Copper complexation by siderophores from filamentous blue-green algae. Limnol. Oceanogr. 25, 62–71. doi: 10.4319/lo.1980.25.1.0062
Midorikawa, T., and Tanoue, E. (1996). Extraction and characterization of organic ligands from oceanic water columns by immobilized metal ion affinity chromatography. Mar. Chem. 52, 157–171. doi: 10.1016/0304-4203(95)00075-5
Midorikawa, T., and Tanoue, E. (1998). Molecular masses and chromophoric properties of dissolved organic ligands for copper(II) in oceanic water. Mar. Chem. 62, 219–239. doi: 10.1016/S0304-4203(98)00040-1
Milne, A., Landing, W., Bizimis, M., and Morton, P. (2010). Determination of Mn, Fe, Co, Ni, Cu, Zn, Cd and Pb in seawater using high resolution magnetic sector inductively coupled mass spectrometry (HR-ICP-MS). Anal. Chim. Acta 665, 200–207. doi: 10.1016/j.aca.2010.03.027
Moffett, J. W., and Brand, L. E. (1996). Production of strong, extracellular Cu chelators by marine cyanobacteria in response to Cu stress. Limnol. Oceanogr. 41, 388–395. doi: 10.4319/lo.1996.41.3.0388
Moffett, J. W., Zika, R. G., and Brand, L. E. (1990). Distribution and potential sources and sinks of copper chelators in the Sargasso Sea. Deep Sea Res. 37, 27–36. doi: 10.1016/0198-0149(90)90027-S
Paunovic, I., Schulin, R., and Nowack, B. (2005). Evaluation of immobilized metal-ion affinity chromatography for the fractionation of natural Cu complexing ligands. J. Chromatogr. A. 1100, 176–184. doi: 10.1016/j.chroma.2005.09.055
Paunovic, I., Schulin, R., and Nowack, B. (2008). Fractionation of dissolved carbon from soil solution with immobilized metal ion affinity chromatography. Eur. J. Soil Sci. 59, 198–207. doi: 10.1111/j.1365-2389.2007.00975.x
Perrin, D. D. (1979). Stability Constants of Metal-Ion Complexes. Part B, Organic Ligands. Oxford: Pergamon Press.
Porath, J., Carlsson, J., Olsson, I., and Belfrage, G. (1975). Metal chelate affinity chromatography, a new approach to protein fractionation. Nature 258, 598–599. doi: 10.1038/258598a0
Rodrigues, A., Brito, A., Janknecht, P., Proença, M. F., and Nogueira, R. (2009). Quantification of humic acids in surface waters: effects of divalent cations, pH and filtration. J. Environ. Monit. 11, 377–382. doi: 10.1039/B811942B
Ross, A. R. S., Ikonomou, M. G., and Orians, K. J. (2000). Characterization of dissolved tannins and their metal-ion complexes by electrospray ionization mass spectrometry. Anal. Chim. Acta 411, 91–102. doi: 10.1016/S0003-2670(00)00746-7
Ross, A. R. S., Ikonomou, M. G., and Orians, K. J. (2003). Characterization of copper-complexing ligands in seawater using immobilized copper(II)-ion affinity chromatography and electrospray ionization mass spectrometry. Mar. Chem. 83, 47–58. doi: 10.1016/S0304-4203(03)00095-1
Ross, A. R. S., Ikonomou, M. G., Thompson, J. A. J., and Orians, K. J. (1998). Determination of dissolved metal species by electrospray ionization mass spectrometry. Anal. Chem. 70, 2225–2235. doi: 10.1021/ac9711908
Semeniuk, D. M., Bundy, R. M., Payne, C. D., Barbeau, K. A., and Maldonado, M. T. (2015). Acquisition of organically complexed copper by marine phytoplankton and bacteria in the Northeast subarctic Pacific Ocean. Mar. Chem. 173, 222–233. doi: 10.1016/j.marchem.2015.01.005
Smith, C. L., Stauber, J. L., Wilson, M. R., and Jolley, D. F. (2014). The use of immobilised metal affinity chromatography (IMAC) to compare expression of copper-binding proteins in control and copper-exposed marine microalgae. Anal. Bioanal. Chem. 406, 305–315. doi: 10.1007/s00216-013-7452-6
Sunda, W. G. (1991). Trace metal interactions with marine phytoplankton. Biol. Oceanogr. 6, 411–442.
Taguchi, G., and Yokoyama, Y. (1994). Taguchi Methods: Design of Experiments. Dearborn, MI: American Supplier Institute.
Vachet, R. W., and Callaway, M. B. (2003). Characterization of Cu(II)-binding ligands from the Chesapeake Bay using high-performance size-exclusion chromatography and mass spectrometry. Mar. Chem. 82, 31–45. doi: 10.1016/S0304-4203(03)00047-1
Van Den Berg, C. M. G. (1986). Determination of copper,cadmium and lead in seawater by cathodic stripping voltammetry of complexes with 8-hydroxyquinoline. J. Electroanal. Chem. 215, 111–121. doi: 10.1016/0022-0728(86)87009-7
Vraspir, J. M., and Butler, A. (2009). Chemistry of marine ligands and siderophores. Ann. Rev. Mar. Sci. 1, 43–58. doi: 10.1146/annurev.marine.010908.163712
Waska, H., Koschinsky, A., Chancho, M. J. R., and Dittmar, T. (2015). Investigating the potential of solid-phase extraction and Fourier transform-ion cyclotron resonance mass spectrometry (FT-ICR-MS) for the isolation and identification of metal-organic complexes from natural waters. Mar. Chem. 173, 78–92. doi: 10.1016/j.marchem.2014.10.001
Watanabe, H., Goto, K., Taguchi, S., McLaren, J. W., Berman, S. S., and Russell, D. S. (1981). Preconcentration of trace elements in sea water by complexation with 8-hydroxyquinoline and adsorption on C18 bonded silica gel. Anal. Chem. 53, 738–739. doi: 10.1021/ac00227a043
Waters Corporation (2014). Care and Use Manual for Oasis HLB Cartridges and 96-well Plates. Available online at: https://www.waters.com/webassets/cms/support/docs/715000109.pdf
Wilhelm, S. W. (1995). Ecology of iron-limited cyanobacteria: a review of physiological responses and implications for aquatic systems. Aquat. Microb. Ecol. 9, 295–303. doi: 10.3354/ame009295
Wiramanaden, C. I. E., Cullen, J. T., Ross, A. R. S., and Orians, K. J. (2008). Cyanobacterial copper-binding ligands isolated from artificial seawater cultures. Mar. Chem. 110, 28–41. doi: 10.1016/j.marchem.2008.02.003
Wu, F., and Tanoue, E. (2001a). Isolation and partial characterization of dissolved copper-complexing ligands in streamwaters. Environ. Sci. Technol. 35, 3646–3652. doi: 10.1021/es0019023
Wu, F., and Tanoue, E. (2001b). Geochemical characterization of organic ligands for copper(II) in different molecular size fractions in Lake Biwa, Japan. Org. Geochem. 32, 1311–1318. doi: 10.1016/S0146-6380(01)00094-8
Keywords: marine copper ligands, organic complexes, immobilized metal-ion affinity chromatography, electrospray ionization tandem mass spectrometry, solid-phase extraction
Citation: Nixon RL and Ross ARS (2016) Evaluation of Immobilized Metal-Ion Affinity Chromatography and Electrospray Ionization Tandem Mass Spectrometry for Recovery and Identification of Copper(II)-Binding Ligands in Seawater Using the Model Ligand 8-Hydroxyquinoline. Front. Mar. Sci. 3:246. doi: 10.3389/fmars.2016.00246
Received: 31 May 2016; Accepted: 11 November 2016;
Published: 25 November 2016.
Edited by:
Kristen Nicolle Buck, University of South Florida, USAReviewed by:
Peter Croot, National University of Ireland, Galway, IrelandDaniel James Repeta, Woods Hole Oceanographic Institution, USA
Copyright © 2016 Nixon and Ross. This is an open-access article distributed under the terms of the Creative Commons Attribution License (CC BY). The use, distribution or reproduction in other forums is permitted, provided the original author(s) or licensor are credited and that the original publication in this journal is cited, in accordance with accepted academic practice. No use, distribution or reproduction is permitted which does not comply with these terms.
*Correspondence: Andrew R. S. Ross, YW5kcmV3LnJvc3NAZGZvLW1wby5nYy5jYQ==