- 1Division of Biological and Environmental Sciences and Engineering, Red Sea Research Center, King Abdullah University of Science and Technology, Thuwal, Saudi Arabia
- 2Biology Department, University of Dammam, Dammam, Saudi Arabia
As coastal plants that can survive in salt water, mangroves play an essential role in large marine ecosystems (LMEs). The Red Sea, where the growth of mangroves is stunted, is one of the least studied LMEs in the world. Mangroves along the Central Red Sea have characteristic heights of ~2 m, suggesting nutrient limitation. We assessed the nutrient status of mangrove stands in the Central Red Sea and conducted a fertilization experiment (N, P and Fe and various combinations thereof) on 4-week-old seedlings of Avicennia marina to identify limiting nutrients and stoichiometric effects. We measured height, number of leaves, number of nodes and root development at different time periods as well as the leaf content of C, N, P, Fe, and Chl a in the experimental seedlings. Height, number of nodes and number of leaves differed significantly among treatments. Iron treatment resulted in significantly taller plants compared with other nutrients, demonstrating that iron is the primary limiting nutrient in the tested mangrove population and confirming Liebig's law of the minimum: iron addition alone yielded results comparable to those using complete fertilizer. This result is consistent with the biogenic nature of the sediments in the Red Sea, which are dominated by carbonates, and the lack of riverine sources of iron.
Introduction
Mangroves play a key role in coastal ecosystems as a source of food, nursery grounds, and a carbon sink as well as for coastal protection (Polidoro et al., 2010). Mangroves often grow in river mouths and deltaic areas, where they receive abundant nutrient supply from riverine discharge, but they also grow in areas devoid of riverine inputs, such as carbonate shores in the Caribbean and islands in South East Asia and the Red Sea, where they are often nutrient limited and, as a result, have a dwarf stature (Feller, 1995; Duarte et al., 1998; Lovelock et al., 2004). In particular, nitrogen, phosphorus, or iron is often reported to limit mangrove growth, while other essential nutrients are abundant in seawater, making them available to mangroves (Alongi, 2011). Indeed, low availability of nitrogen, phosphorus, and iron has been suggested as the cause for the absence of mangroves along shorelines that are otherwise suitable for mangrove growth (Sato et al., 2011). Mangrove fertilization experiments have demonstrated site-specific nutrient limitation of nitrogen and phosphorous (Feller, 1995; Koch and Snedaker, 1997; Feller et al., 2003a,b; Lovelock et al., 2004, 2006; Naidoo, 2009; Alongi, 2011), whereas an experimental study conducted on mangroves in north Queensland, Australia, demonstrated iron deficiency (Alongi, 2010). Previous work has not considered these three nutrients together, which is the focus of this work.
Mangroves cover ~135 km2 of the Red Sea coastline (Almahasheer et al., 2016a), providing natural coastal vegetation along an otherwise arid topography that is devoid of rivers and significant rainfall. A recent assessment (Almahasheer et al., 2016a) established that the area of the Red Sea covered by mangroves has increased by 12% since 1972. High concentrations of nutrients are mainly found in the southern (Churchill et al., 2014) and northern (Triantafyllou et al., 2014) reaches of the Red Sea and generally taper in the central area (Eshel and Naik, 1997). The Central Red Sea is the most oligotrophic part of the basin, which is clearly evident in the Chl a concentrations there, which are the lowest in the Red Sea (Raitsos et al., 2013). The monsoons and prevailing regional winds play a key role in driving the mechanisms that supply nutrients and consequently regulate the seasonal and interannual variability of Chl a concentrations in the Red Sea (Raitsos et al., 2015). The lack of riverine input and negligible precipitation mean that the main nutrient source is the monsoonal-driven horizontal intrusion of nutrient-rich waters from the Indian Ocean (Murray and Johns, 1997; Johns and Sofianos, 2012; Churchill et al., 2014; Raitsos et al., 2015). The intruding water flows northward along the African and Arabian coasts of the Red Sea, hardly reaching the Central Red Sea (Churchill et al., 2014). Additional nutrients are supplied by sub-surface mixing below the nutricline in the Northern Red Sea (Triantafyllou et al., 2014; Yao et al., 2014) and dust deposition (Brindley et al., 2015).
The low nutrient concentrations in the Red Sea resulting from the absence of riverine inputs and limited hydrographic exchange with the Indian ocean suggest that any mangroves growing there will be nutrient limited (Thompson et al., 2013). Indeed, the dwarf status of the trees, with an approximate height of 2 to 3 m in the Central Red Sea area (Almahasheer et al., 2016b) is characteristic of nutrient-limited mangroves (Krauss et al., 2008). Low nutrient inputs from the land in this area may also result in nutrient limitation. Alternatively, it has been suggested that the reduced size of mangroves could result from other stresses, such as the extremely high temperature and salinity (Yao et al., 2014) characteristic of the Red Sea region (Douabul and Haddad, 1970). However, mangroves are comparatively taller in the southern area of the Red Sea where salinity is higher than the ocean average, sea surface temperature is higher than in the Central Red Sea and nutrient concentrations are much higher than in the central area, (Mandura et al., 1987; Mandura, 1997), indicating that nutrient availability is the primary factor limiting growth.
Although, mangroves support a very tight nutrient economy to avoid nutrient loss (Feller, 1995), their reproduction is a major vector for nutrient loss as the seedlings are dispersed away from the mother tree. Effective reproduction relies on developing propagules that contain enough nutrients to support the survival and dispersal of seedlings (McKee, 1995). The nutrient status of the seedling is particularly critical and affects the tree's resistance to light, high salinity and floods (Koch, 1997). Acute nutrient limitation, likely experienced by mangrove trees in the Central Red Sea, may reduce the mother tree's capacity to supply sufficient nutrients to the propagule, thus hindering seedling growth and performance. The assesment of nutrient limitation during seedling growth in mangroves is therefore particularly important.
Here, we assess the nutrient status monospecific stands of Avicennia marina trees in the ultra-oligotrophic Central Red Sea and experimentally examine the response of seedlings to the addition of nutrients. We ascertain if nutrient deficiency in the mother trees affects the capacity of propagules to fully support early growth in seedlings. Specifically, we evaluate the nutrient status of mangrove trees by assessing nutrient concentrations in leaves across a wide range of stands in the Central Red Sea area, from pristine stands to stands receiving nutrient inputs from industrial activity. We also experimentally test the responses of A. marina seedlings to the addition of nutrients (N, P and Fe and various combinations) to assess which nutrients limit their growth and development.
Materials and Methods
Study Area
The Red Sea has about 135 Km2 of mangroves distributed up to 28.207302°N, the northern biogeographical boundary of mangroves. Unlike the coverage of mangroves in other regions, the area covered by mangroves in the Red Sea remains relatively stable (Almahasheer et al., 2016a). Nutrient inputs to the Red Sea are dominated by inputs from the Indian Ocean (Murray and Johns, 1997; Johns and Sofianos, 2012; Churchill et al., 2014; Raitsos et al., 2015), leading to a gradient of oligotrophication toward the north (Ismael, 2015), concurrent with an increase in salinity due to high evaporation losses (Talley, 2002). On the other hand, the sea surface temperature declines from south to north (Rushdi, 2015). The Central Red Sea is an arid environment characterized by high temperatures (Table S1 presents the weather conditions during the experiment), sparse rainfall (Edwards, 1987); the mean annual (sporadic) rainfall in Jeddah is 55 mm), high salinity (Bruckner, 2011) and low nutrient inputs and concentrations (Mandura, 1997). The tidal range on the Central Red Sea is only 20 to 30 cm (Sultan et al., 1996), resulting in mangrove habitats that develop as a narrow (typically one to three trees wide) fringe along the shore, adjacent to sand flats that may occasionally be flooded, with desert further inland. The mangrove fringe supports small halophytes. Recent urban and industrial development of the Saudi shore in the Central Red Sea area has resulted in locally elevated nutrient inputs, providing an opportunity to assess how such inputs may affect the nutrient status of mangroves.
The sampled sites in the study area (Figure 1) were selected to capture the diversity of environments in the region. In particular, we sampled mangrove leaves from four locations in the Central Red Sea, including Thuwal Island, Economic City lagoon, Petro Rabigh and Khor Alkharar (Table 1, Figure 1). Away from anthropogenic sources of nutrients, the mangrove stands at Khor Alkharar, Economic City lagoon and Thuwal Island are pristine: King Abdullah Economic City, about 40 km south of Rabigh, is a new and fast-growing urban development; Khor Alkharar lagoon lies on the coastal plain northwest of Rabigh and is connected to the adjacent Red Sea by a narrow inlet on its northwestern side (Gheith and Abou-ouf, 1996; Al-Farawati, 2011); on Thuwal Island, A. marina grows on shallow soils of weathered coral (Balk et al., 2015). In contrast, the city of Rabigh hosts a large petrochemical complex and the mangrove stand are likely influenced by the industrial environment.
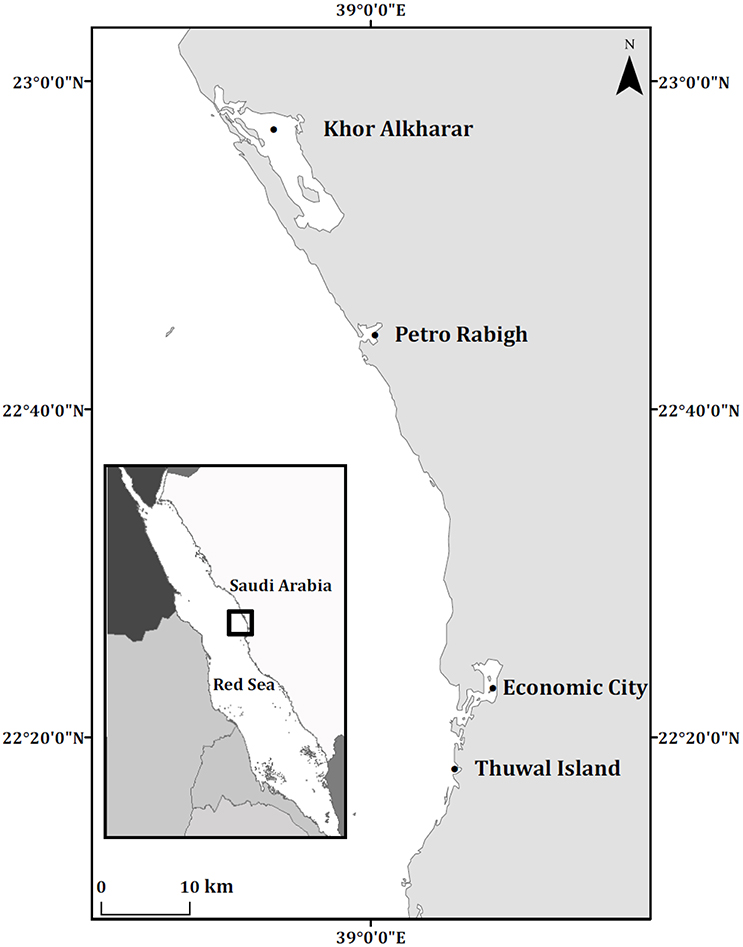
Figure 1. Location of the sampled Central Red Sea mangrove stands. The map was produced with ArcMap Version 10.2. Background map credits: the World Administrative Divisions layer provided by Esri Data and Maps and DeLorme Publishing Company.
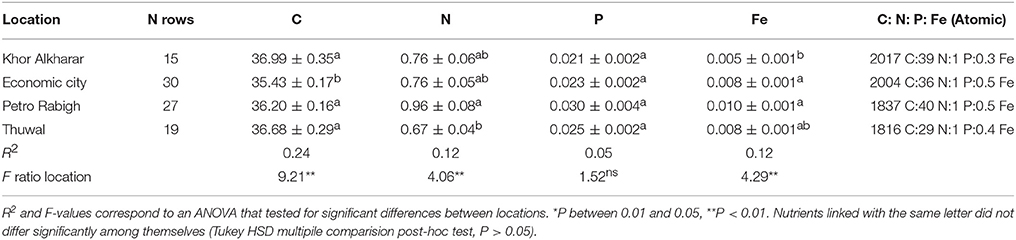
Table 1. Mean (± SE) for nutrient concentrations (mmol g DW−1) in Avicennia marina leaves from four different locations in the Central Red Sea.
Nutrient Status in Central Red Sea Mangrove Stands
We assessed the nutrient status of mangrove stands in the Red Sea by examining the concentrations of nitrogen, phosphorous and iron, the nutrients most often reported to limit mangrove growth (Feller, 1995; Koch and Snedaker, 1997; Feller et al., 2003a,b; Lovelock et al., 2004, 2006; Naidoo, 2009; Alongi, 2011). We analyzed nutrient concentrations in mature leaves as well as the stoichiometric ratios of the nutrients to carbon. Whereas examination of leaf nutrient concentration alone does not suffice to establish nutrient budgets (Lü et al., 2012), leaf nutrient concentration robustly indicates the nutrient status of plants (Duarte, 1990, 1992), including mangroves (Duarte et al., 1998; Feller et al., 2003b). Moreover, leaf nutrient concentration is an important ecosystem property because it affects the decomposition rates of leaf litter (Enriquez et al., 1993). Deeming that quadrant or transect sampling would not achieve the representativeness of the leaf nutrient concentration required by this study, we used a nested sampling strategy to characterize leaf nutrient concentration at each location. First, we selected two to three disjoint stands at each location. From each of these stands, we randomly selected one tree and sampled 6 to 10 leaves from the main stem of the tree. In total, this yielded 15 to 30 leaves from each location (Table 1). We collected the mangrove leaves in March 2015, following propagule release, which causes significant nutrient loss and may render the plants vulnerable to nutrient limitation.
Seedling Fertilization Experiments
We conducted two experiments to examine the response of seedlings to the addition of nutrients. The experiments were designed to test whether propagules collected from nutrient-deficient stands on Thuwal Island contained enough nutrients to support seedling growth or if their growth and development could be increased by nutrient input. In addition, we identified the limiting nutrient by using various combinations of treatments of the three nutrients. Two varied treatment experiments (see below) were conducted, the first in 2014 and the second in 2015. The experiments were conducted in a nursery covered with a green mesh to reduce incoming solar radiation by around 90% (Quantum Meter MQ-100, μmol PAR m−2 s−1). The average radiation in the nursery (mean ± SE = 8.3 ± 2.5% of the incoming radiation) was slightly greater than that received under natural growing conditions in Thuwal (5.5 ± 1.7%, Table 2). The plants were exposed to solar radiation and temperature regimes comparable to those in the Thuwal Island mangrove stand where the propagules were produced. We carefully controlled nutrient conditions in both the sediment and water. In particular, we washed the sediments and diluted the seawater to reduce external nutrient inputs in the control treatment, thereby measuring seedling growth as a response to added nutrients in the different treatments. The control treatment thus captures the growth and development capacity of A. marina seedlings as determined by the nutrients allocated by the mother plant to the propagules. Accordingly, the response of the seedling to various nutrient treatments indicates which nutrients are deficient in the propagules relative to those required to support nutrient-sufficient growth of the resulting seedlings because propagule production is likely to be a major vector for nutrient loss in the mangrove stands studied.
The nursery was fitted with raceways periodically flooded with brackish water using pumps to simulate the tidal cycle (see below). The experiments were initiated in February 2014 and March 2015, using A. marina seedlings germinated from propagules collected from healthy mature trees on Thuwal Island. The collected propagules were fully formed, with a hard pericarp and an average dry weight of 2.98 ± 0.12 g DW (n = 33). Five propagules were dried for nutrient analysis. The raceways were washed with 1% acid followed by nutrient-free water to avoid contamination before the propagules were germinated. The propagules were soaked in brackish water for 3 days until their covers shed naturally. All the propagules used in the experiment germinated on the same day. The sediment used to accommodate the seedlings in the raceway consisted of sand collected from Thuwal that was washed with nutrient-free water to reduce the concentrations of available nutrients. The control treatment was thereby rendered nutrient depleted. The brackish water was a mixture of equal volumes of seawater collected near Thuwal and nutrient-depleted fresh water collected from King Abdullah University of Science and Technology (KAUST), which was found to provide suitable growth conditions (Connor, 1969; Clough, 1984). Previously, between September and November 2013, we conducted a preliminary test of the growth response of seedlings to various salinity regimes (0, 25, 50, 75, 100% seawater) and confirmed that a 50:50 mixure of Red Sea seawater and fresh water provided the best growth conditions. Indeed, this finding is consistent with the occurrence of Red Sea mangroves near groundwater inputs in the Red Sea; many mangrove stands are located near Wadis (dry river beds with associated groundwater discharge). The raceways were flooded with brackish water by pumps for 6 h daily, following (Clarke and Johns, 2002). The excess water then slowly flowed back into the tanks located under the raceways (Figure 2). This flooding simulated the intertidal cycle while also avoiding excess algal growth in the raceways. Furthermore, to avoid water loss due to evaporation and evapotranspiration, we replaced the water in the tanks every month with freshly prepared brackish water as described above. Tanks, valves, and raceways were washed monthly during the experiment.
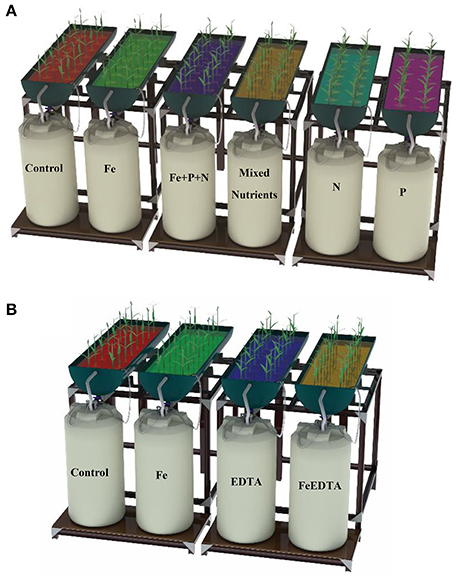
Figure 2. Experimental setup for (A) the experiment testing for N, P, and Fe limitation, alone and in combination conducted in 2014, and (B) the experiment testing for the role of iron vs. the chelant used in the first experiment (EDTA) conducted in 2015. The colors representing the treatments are used for the same treatment in all figures.
Propagules were planted at half height in the sediment (Bovell, 2011) in individual pots. The experimental treatments were initiated 4 weeks after planting. By this time, the seedlings were equal in height and had developed 4 leaves each (Clough, 1984; Ball et al., 1987). We used 30 replicated seedlings per treatment in the first experiment and 21 replicated seedlings per treatment in the second experiment, with the treatments applied independently to each replicated seedling.
The first experiment aimed at assessing the roles of nitrogen, phosphorus and iron, alone and in combination, in promoting seedling growth beyond the growth capacity corresponding to the nutrients received from the mother plant, whereas the second experiment aimed at separating the possible effects of iron and the chelant used in combination in the first experiment, which was found to significantly enhance seedling growth (see Results). A chelant is an organic ligand incorporating metal ions in a structure that facilitates metal uptake by plants (Salt et al., 1998; Hart, 2000). Specifically, Ethylenediaminetetraacetic acid (EDTA) has been found to facilitate Fe uptake. We used the nutrient concentrations of (Ball et al., 1987) for nitrogen and phosphorus and (Steiner and van Winden, 1970) for Fe-EDTA. The first experiment, initiated in March 2014, included the following final nutrient concentrations in six treatments applied to the brackish water used to flood the raceways: (1) nitrogen (as 2 mmol NH4NO3L−1); (2) phosphorus (0. 2 mmol NaH2PO4L−1); (3) iron (0. 2 mmol Fe-EDTA L−1); (4) nitrogen, phosphorous, and iron, each supplied at the preceding concentrations; and (5) a complete fertilizer, including 4 mmol Ca (NO3)2L−1, 0. 2 mmol L−1 NaH2PO4, 1 mmol L−1 MgSO4, 0. 2 mmol Fe-EDTA L−1, 2 mmol NH4NO3L−1, 0.025 mmol H3BO3L−1, 0.002 mmol MnSO4L−1, 0.002 mmol ZnSO4L−1, 0.0005 mmol CuSO4L−1, 0.0005 mmol H2MoO4L−1, and 0.025 mmol MgCl2L−1; and (6) a control without nutrient addition. In the second experiment, the following four treatments were tested: (1) iron (0. 2 mmol FeCl2, L−1); (2) EDTA (0. 2 mmol EDTA L−1); (3) Fe + EDTA (0.2 mmol Fe-EDTA L−1); and (4) the control without iron or EDTA addition. Nutrient concentrations in the brackish water were adjusted monthly. The nutrient concentrations used in the treatments were higher than in the water in a lagoon near Thuwal, where the (mean ± SE) concentrations of NO2 were 0.062 ± 0.006 μmol L−1 and NO2+NO3 were 0.181 ± 0.017 μmol L−1 (Banguera-Hinestroza et al., 2016). They were also higher than values found in coastal water from Duba (north) and Jazan (south) along the Saudi coast of the Red Sea (NO3 = 2.351 ± 0.386 μmol L−1 and PO4 = 0.239 ± 0.039μmol L−1; (Pearman et al., 2016).
Measurements and Chemical Analysis
The following parameters were measured once per month in the first experiment and every month and a half in the second experiment: (a) C, N, P, Fe. and Chl a concentrations in the first pair of fully developed apical leaves of three seedlings per treatment; and (b) growth rate, as determined by the elongation rate along the main meristem, number of nodes, number of leaves and root development for each seedling in the experiment. Chl a was extracted from 1-cm diameter discs cut from fresh leaves using a QIAGEN TissueLyser II and extracted overnight in 10 ml 80% acetone. The Chl a concentration in the extract was measured spectrophotometrically based on absorbance at 663 nm following (Wellburn, 1994). The other apical leaf was washed with nutrient-free water, dried at 60°C in a drying oven and ground for carbon and nutrient analyses. Carbon and nitrogen concentrations were determined on 2 mg samples of powderized leaf material wrapped in a pre-combusted aluminum capsule using a FLASH 2000 CHNS Analyzer (Zimmermann et al., 1997). Iron and phosphorous concentrations were determined after digesting 0.50 mg of the same powderized leaf with 5 ml concentrated HCL and a few drops of H2O2 in a Digi PREP digestion system, following three temperature steps: 30°C for 30 min, 50°C for 30 min. and 75°C for 45 min. The samples were allowed to cool, diluted to 25 ml with Mili-Q water and analyzed by Inductively Coupled Plasma-Optical Emission Spectrometry (Varian Inc. model 720-ES).
Iron and phosphorous analyses were run in duplicate. Two different standards (Inorganic Ventures and PerkinElmer's Pure Plus) were used to assess the accuracy of the measurements, with recovery rates of 100.1% for iron and 99.1% for phosphorous (n = 10) in the first experiment (2014) and 98.8% for iron (n = 10) in the second (2015). The recovery was, on average, 98.7% for phosphorous and 104.9% for iron (n = 20) in the analyses of leaves collected in the survey (n = 30). We also used a standard reference material from The National Institute of Standards and Technology (NIST) to verify the accuracy of the phosphorous and iron concentrations, resulting in 94.6% accuracy in apple leaves and 81.9% accuracy in peach leaves for the second experiment and 73.3% accuracy in apple leaves and 79.2% accuracy in peach leaves in the analyses run for the leaves collected in the survey. To analyze carbon and nitrogen concentrations, we used a standard reference material (BBOT) and a calibration standard (Sulfanilamide) to obtain an average recovery of 99% for nitrogen and 102% for carbon (n = 14) for the first experiment and an average of 101.1% for nitrogen and 100.7% for carbon for the leaves collected in the survey (n = 30).
Statistical Analysis
Seedling growth rates were calculated from the slopes of the fitted linear regressions on the relationship between the development metrics and time. Statistical analysis, including descriptive statistics, linear regression analyses, general linear models to test for the effects of the different experimental treatments or differences among stands, and Tukey's HSD (honest significant difference) post-hoc test to assess pairwise differences were carried out using JMP, a computer program for statistical analyses developed by SAS Institute.
Results
Nutrient concentrations in A. marina leaves were low (N < 1.5%, P < 0.09%, Fe < 0.06) and differed significantly between locations (ANOVA, P < 0.05, Table 1). The plants at Petro Rabigh exhibited increased concentrations of N, P, and Fe in the leaves compared with the other populations sampled. Those in Khor Alkharar Lagoon exhibited the lowest leaf nutrient concentrations (except for nitrogen in the leaves collected from Thuwal Island; Table 1). The C: N: P: Fe ratios were extremely high, indicative of acute nutrient depletion relative to carbon (Table 1).
The propagules collected in Thuwal were characterized by a low nutrient concentration compared to the seedlings (C = 32.61 ± 0.74, N = 0.86 ± 0.10, P = 0.035 ± 0.001, Fe = 0.0006 ± 0.0001 mmol g DW−1), indicating extreme iron depletion relative to the leaves (930 C: 25 N: 1 P: 0.02 Fe, n = 5).
All seedlings survived the first experiment, but three seedlings died in the EDTA treatment and one in the Fe + EDTA treatment in the second experiment. There were significant differences between treatments in both experiments in terms of seedling height, number of leaves and nodes after 3 months. Seedling growth differed significantly with treatments in the first experiment (R2 = 0.62, F = 49.75, P < 0.0001), with the treatment receiving iron reaching the largest size, even exceeding that in the treatment receiving complete nutrients (Figure 3A). We also found significant differences in seedling growth in the second experiment (R2 = 0.68, F = 47.21, P < 0.0001), with the seedlings fertilized with iron alone reaching significantly higher heights than those receiving EDTA or Fe+EDTA and those under control conditions (Figure 3B). Hence, iron addition consistently led to a fast growth rate in both experiments (Tukey HSD post-hoc test, P < 0.05., Table 3).
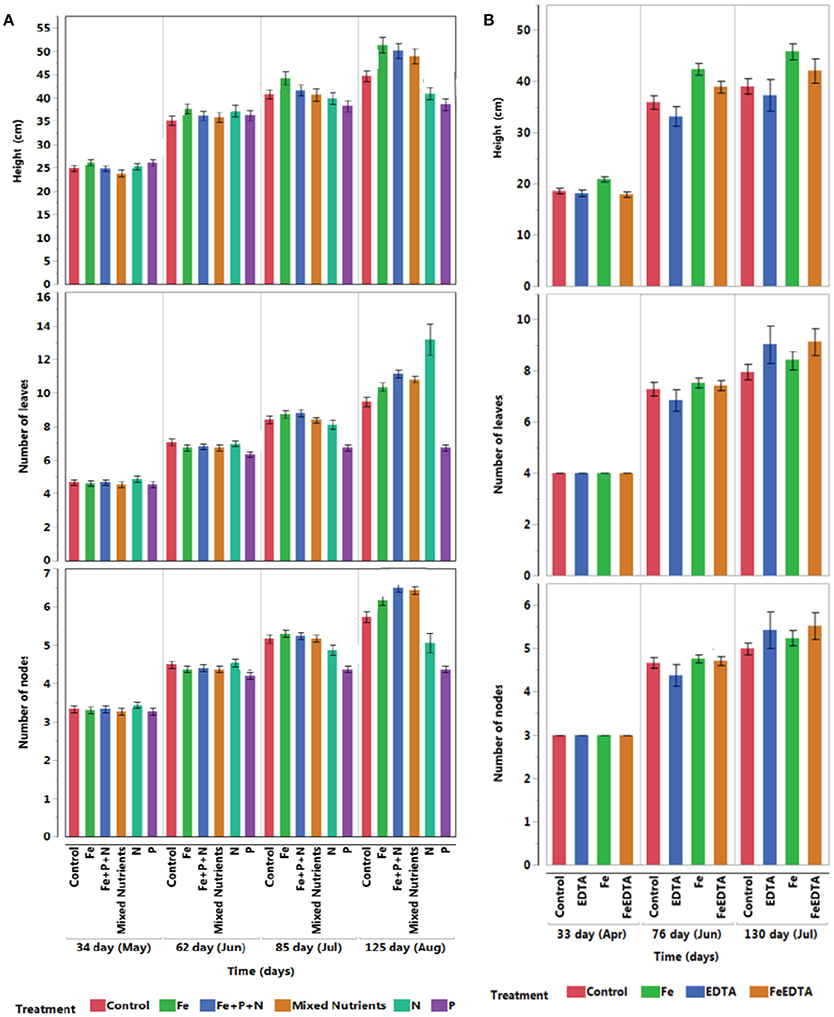
Figure 3. Mean (± SE) for height, number of nodes, and leaves of mangrove seedlings over time under control and different nutrient addition treatments across experiments testing for (A) N, P, and Fe addition and (B) components of response to iron addition (Fe vs. EDTA).
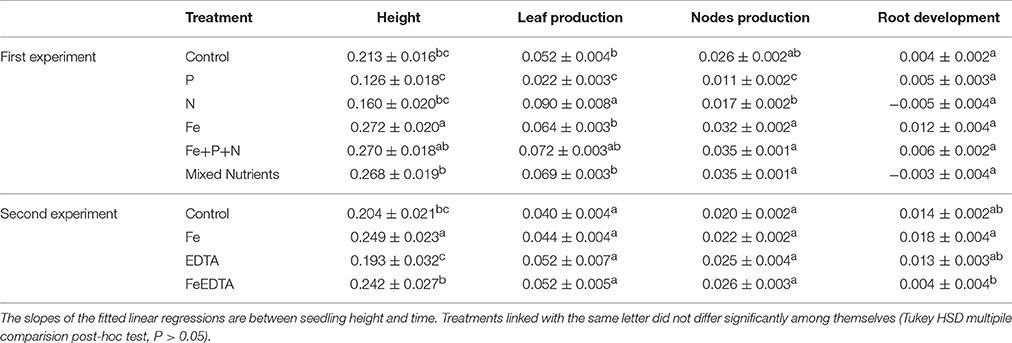
Table 3. Mean (± SE) growth rate (cm seedling−1 day−1), leaf and node production rate (number of leaves and nodes seedling−1 day−1), and root development (g DW seedling−1 day−1) of Red Sea mangrove seedlings under different nutrient addition treatments.
Comparable significant results were found in the number of nodes produced during the first experiment (R2 = 0.73, F = 82.1, P < 0.0001), where all treatments involving iron addition produced significantly more nodes than the control. Node production in the second experiment was slightly higher in the EDTA and Fe+EDTA treatments (R2 = 0.57, F = 29.10, P < 0.0001) than those receiving iron alone and the control (Figures 3A,B and Table 3, Tukey HSD post-hoc test, P < 0.05).
The number of leaves produced also differed significantly among treatments but was significantly higher in the treatment receiving nitrogen in the first experiment (R2 = 0.71, F = 73.92, P < 0.0001) and the treatment receiving Fe+EDTA in the second experiment (R2 = 0.62, F = 36.98, P < 0.0001) (Figures 3A,B and Table 3, Tukey HSD post-hoc test, P < 0.05).
Root development (as g DW seedling−1) increased under iron and phosphorous addition at the end of the first experiment (R2 = 0.62, F = 3.50, P = 0.0008, Tukey HSD post-hoc test, P < 0.05 Figure 4A and Table 3). Root development was significantly higher in the iron treatment in both experiments (R2 = 0.59, F = 9.38, P < 0.0001, Tukey HSD post-hoc test, P < 0.05 Figure 4B and Table 3).
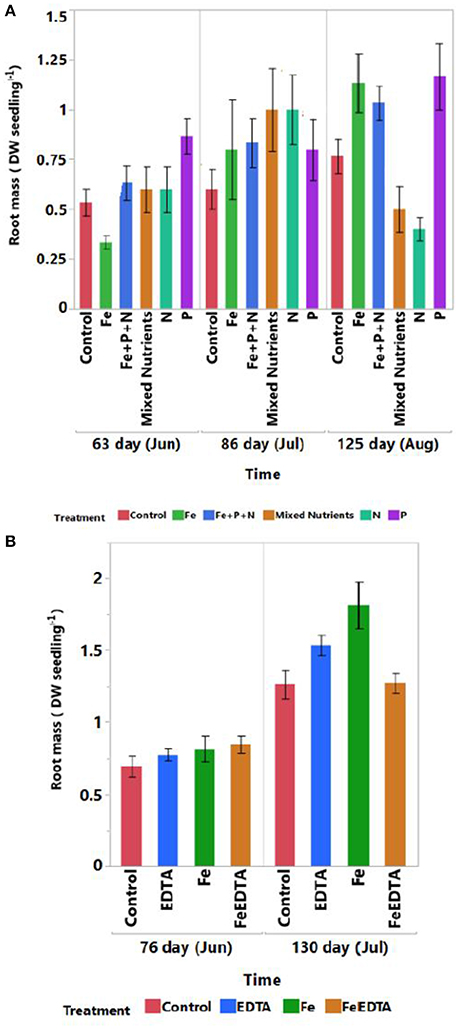
Figure 4. Mean (± SE) for root development of mangrove seedlings over time under control and different nutrient addition treatments across experiments testing for (A) N, P, and Fe addition and (B) components of response to iron addition (Fe vs. EDTA).
Iron addition led to higher leaf Chl a concentration in the first experiment (R2 = 0.76, F = 7.68, P < 0.0001, Tukey HSD post-hoc test, P < 0.05, Figure 5A), whereas leaf Chl a concentration in the second experiment was higher in both EDTA and FeEDTA treatments than in the iron treatment alone and in the control (R2 = 0.85, F = 30.24, P < 0.0001, Tukey HSD post-hoc test, P < 0.05, Figure 5B).
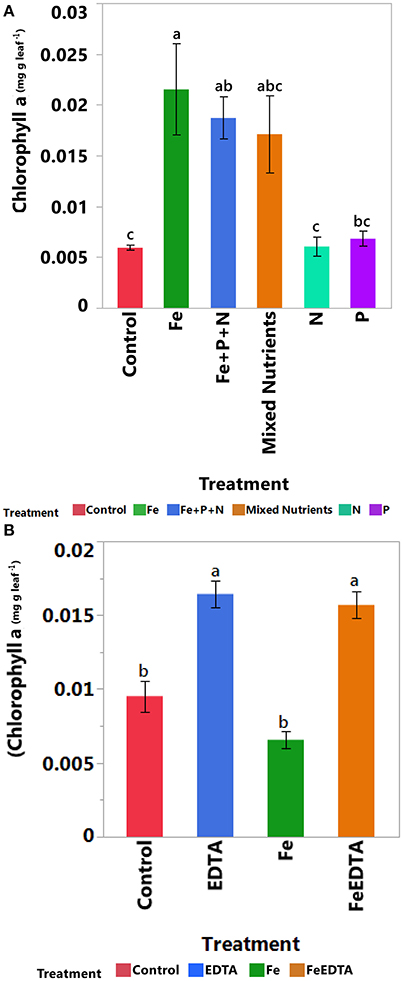
Figure 5. Mean (± SE) for leaf Chl a concentration over time of fully developed mangrove apical leaf under control and different nutrient addition treatments across experiments testing for (A) N, P, and Fe addition and (B) components of response to iron addition (Fe vs. EDTA). Bars showing different letters within a sampling event identify significantly different treatments (P < 0.05), as indicated by post Tukey's HSD multiple comparison test.
Nutrient addition resulted in significant differences in carbon, nitrogen and phosphorus concentrations in the leaves (R2 = 0.89 and F = 18.70; R2 = 0.93 and F = 30.95; and R2 = 0.78 and F = 7.84, respectively, P < 0.0001), with treatments receiving mixed nutrients exhibiting higher leaf nitrogen and phosphorous concentrations. The iron concentration differed significantly between treatments and time (R2 = 0.53, F = 2.39 and P = 0.0137, Table S2) in the first experiment, but it was significantly higher in the plants receiving only iron compared with the EDTA, Fe + EDTA and control treatments in the second experiment (R2 = 0.38, F = 4.18 and P = 0.0188, Table S3). The leaf carbon concentration declined sharply when leaf nitrogen, phosphorous and iron concentrations reached levels below limiting thresholds (N < 2 mmol N g DW−1, P < 0.04 mmol P g DW−1, and Fe < 0.001 mmol Fe g DW−1 (Figure 6).
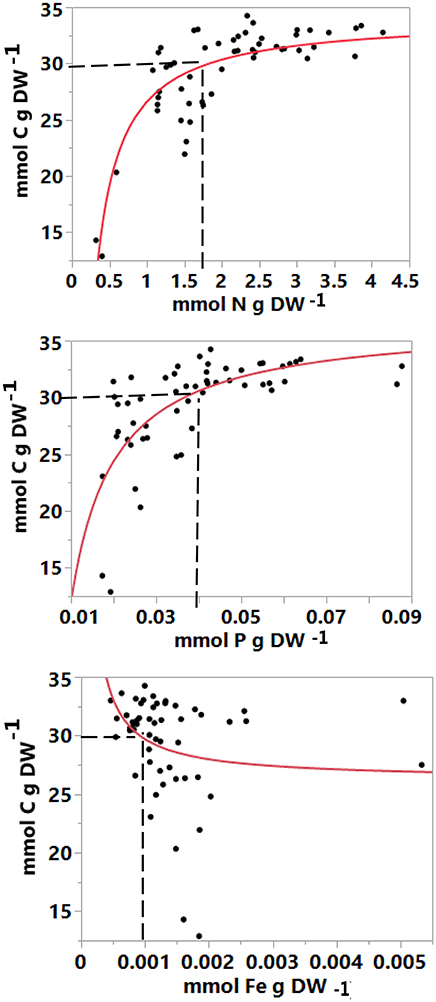
Figure 6. The relationship between carbon and nutrient concentration (mmol nutrient g−1 DW) in fully developed apical leaves of Avicennia marina seedlings. The solid line represents the reciprocal regression equation fitted across data derived from all treatments combined (mmol C = 34.071879 − 7.4611856*Recip mmol N), (mmol C = 36.763021 - 0.2475941*Recip mmol P), and (mmol C = 26.25834 + 0.0034299*Recip mmol Fe).
The C:N ratio increased over time in plants receiving P, followed by the control and Fe treatments (Figure 7). The C:P ratio was relatively high, indicative of phosphorous limitation in the control, iron, nitrogen and mixed nutrient treatments. The C:Fe ratio was high and decreased in the control, mixed nutrient and nitrogen treatments, whereas treatment with Fe, P and Fe+P+N exhibited an increase followed by a subsequent decrease (Figure 7). The N:P ratio showed no significant changes in the control and iron treatments, although the N:P ratio increased over time in the treatments receiving nitrogen and mixed nutrients. Both N:Fe and P:Fe ratios decreased over time in the control, mixed nutrient, nitrogen and phosphorous treatments and increased and then decreased subsequently in the Fe+P+N and Fe treatments (Figure 7).
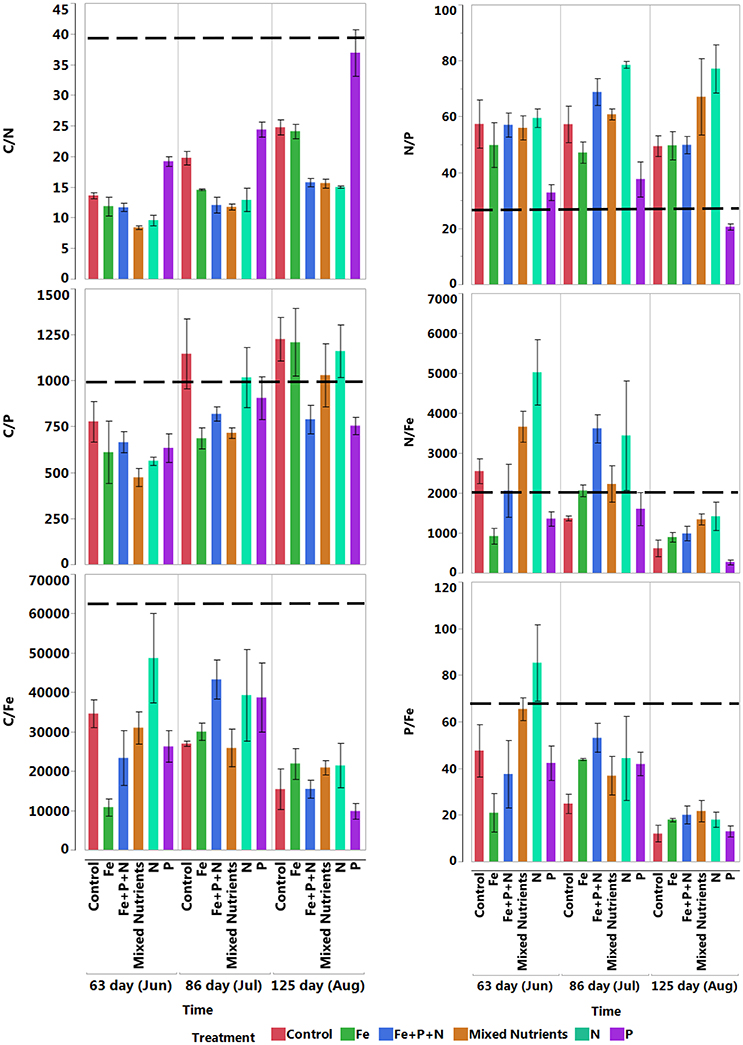
Figure 7. Mean (± SE) nutrients stoichiometric ratios over time of fully developed apical leaves receiving different nutrient additions (n = 3). The dashed line represents the value for propagules.
Discussion
Nutrient Concentration and Stoichiometric Ratios
Mangrove stands in the Central Red Sea are characterized by low leaf nutrient concentrations and low carbon-to-nutrient stoichiometric ratios, with an overall mean of 1918 C: 36 N:1 P: 0.5 Fe, indicative of severe nutrient depletion, particularly P and Fe, across stands and pointing to the likelihood of nutrient limitation of Central Red Sea mangroves. However, there were important differences among stands, with mangroves receiving nutrient inputs from industrial and urban sources at Petro Rabigh showing the highest concentrations of nitrogen, phosphorus and iron across stands. Fe deficiency was particularly acute in the propagules collected to initiate the seedling experiments, which had P:Fe ratios that were 10 to 20-fold higher than those of leaves, indicative of extreme Fe deficiency in these propagules. Unfortunately, C: N: P: Fe ratios for balanced mangrove growth have not yet been defined. Nevertheless, C: N: P ratios for nutrient sufficient A. marina growth can be inferred from those in the fertilized treatments reported by Alongi (Alongi, 2011), which correspond to 346 C: 14 N: 1 P, similar to the treatments receiving P in our experiments, which had the lowest C:N:P ratio. Even the control plants, which did not receive any nutrient additions, in the stand examined by Alongi (Alongi, 2011) had a C:N:P ratio of 711:30:1, well below the ratios found in leaves of Central Red Sea mangroves. The stoichiometric ratios in A. marina seedlings receiving Fe+N+P in our experiments can be considered to be nutrient-sufficient plants. These ratios are quite similar to those for nutrient-sufficient macroalgae and N-fertilized A. marina plants in Northern Queensland, Australia, but are higher than those for nutrient-sufficient seagrass and phytoplankton (Table 4).
Nutrient Requirements and Critical Concentrations
The experimental results allow a first approximation of the critical level of nutrient concentrations required to support nutrient-sufficient growth of A. marina seedlings. These were defined as the nutrient concentrations below which carbon concentrations declined steeply (cf. Figure 6). The inferred thresholds are 2 mmol N g DW−1, 0.04 mmol P g DW−1, and 0.001 mmol Fe g DW−1. These critical thresholds are higher than the thresholds of nitrogen and phosphorus reported for seagrass (N < 1.2 mmol N g−1, P < 0.06 mmol P g−1, recalculated from Duarte, 1990).
Our results provide compelling evidence that the growth of A. marina seedlings in the oligotrophic Central Red Sea requires environmental Fe inputs, as iron addition alone led to a growth response and an increase in Chl a concentrations comparable with those in the treatments also receiving nitrogen and phosphorous. Although, our experiment showed iron to be the main limiting factor for seedling growth, nitrogen addition significantly increased the number of leaves while addition of phosphorous and particularly iron led to increased root development. Yet, the experimental treatment that combined N, P, and Fe addition did not increase growth beyond that achieved by Fe fertilization alone, substantiating Liebig's law of the minimum, with iron as the limiting nutrient. The results from the second experiment confirmed that it was indeed iron, and not the ligand added in the first experiment (EDTA), which elicited the observed growth responses.
The finding of iron-limited growth of Central Red Sea seedlings is consistent with (Alongi, 2010), who reported high iron requirements in the transition stage from seedling to sapling in five mangrove species growing in north Queensland, Australia. Iron limitation in mangrove growth in the Central Red Sea is expected, as iron limitation is characteristic of marine coastal habitats dominated by biogenic carbonate sediments, which are typically iron depleted (Duarte et al., 1995). Moreover, the lack of riverine inputs, which are the main vector of iron to coastal marine sediments (Poulton and Raiswell, 2002; Raiswell, 2006), also renders the Red Sea prone to iron limitation. Iron-limited seagrass growth has also been reported in the Mexican Caribbean (Duarte et al., 1995), where the karstic landscape lacks riverine input and where mangroves are also stunted. Similar to our findings in the Red Sea, iron addition stimulated leaf Chl a concentration in Caribbean seagrasses receiving iron (Duarte et al., 1995).
Nutrient Inputs to the Red Sea
In the absence of rivers and negligible precipitation, such as in the Red Sea, nutrient inputs from the Indian Ocean (Murray and Johns, 1997; Johns and Sofianos, 2012; Churchill et al., 2014; Raitsos et al., 2015), sub-surface mixing (Triantafyllou et al., 2014; Yao et al., 2014), and atmospheric sources are likely to be the most significant path for new nutrient input (Brindley et al., 2015), including nitrogen fixation and inputs of phosphorus and iron with dust deposition (Aerts and Chapin, 1999; Schroth et al., 2009). The Red Sea receives one of the highest dust inputs of all the oceans (Jish Prakash et al., 2015), and mangrove canopies may intercept dust during periods of dust storms, thereby increasing iron supply above that corresponding to passive dust deposition. The solubility of atmospheric iron varies between 0.01 and 80% depending on acid deposition, with 95% of atmospheric iron derived from desert dust and the rest from combustion processes (Mahowald et al., 2009). Although, data from the study area are lacking, seabird guano e.g., brown booby birds, Red-billed tropic-birds and Bridled terns may also be an important source of nutrients to Red Sea mangroves, which support abundant bird communities (Evans, 1987). The results presented also suggest anthropogenic inputs to be significant in mangrove stands near developed areas, as exemplified by the mangroves near Petro Rabigh, which exhibited elevated concentrations of iron as well as nitrogen and phosphorous.
A range of mangrove fertilization experiments were previously conducted, mostly focused on nitrogen and phosphorous addition, but those exploring iron limitation have been scarce. The outcome of these experiments depend on location, with some mangrove stands shown to be limited by phosphorous (Feller, 1995; Koch and Snedaker, 1997; Feller et al., 2003a; Lovelock et al., 2004, 2006) and others to be limited by nitrogen (Feller et al., 2003b; Naidoo, 2009). Prior to the present study, iron limitation in mangrove growth had been reported, on the basis of experimental addition, only in mangroves in north Queensland, Australia (Alongi, 2010). The finding that mangroves in the Central Red Sea are nutrient-limited, as evidenced by low nutrient concentrations in their leaves and very low iron levels in the propagules they form, allows us to begin to understand the functioning and drivers of these important components of the Red Sea ecosystem. Nutrient-limited plants are likely to re-absorb significant amounts of nutrients before shedding their leaves (Reef et al., 2010), suggesting that nutrient-limited mangroves may act as a sink rather than a source of nutrients to the ecosystem. Moreover, the decomposition rate of plant litter decreases with increasing C:N ratios (Enriquez et al., 1993). Nutrient-limited Central Red Sea mangroves are thus likely to produce relatively recalcitrant litter, which may act as a carbon sink in mangrove sediments. Lastly, our results show that nutrient-deficient mangroves are likely to produce nutrient-poor propagules. The resulting seedlings require additional nutrient inputs to support high growth and survival. The absence of significant nutrient inputs may, thus, act as a bottle-neck in the stability of the population (Selvam, 2007). Nutrient-deficient propagules may also occur in other nutrient-limited mangrove stands. This suggestion requires further testing. Increased anthropogenic nutrient emissions in the Central Red Sea are likely to lead to increased mangrove growth and improved nutrient status with consequences for the ecosystem. Nutrient limitation may also affect the capacity of mangroves to respond to other stresses affecting the Red Sea, such as the high temperature and lack of fresh water supply, as nutrient limitation would impose limits to the capacity of the plants to synthesize proteins and other molecules involved in building resilience to these stresses. Hence, nutrient limitation likely renders the plants more vulnerable to the extreme environmental conditions in the Central Red Sea. However, responses to multiple stresses have not yet been investigated as we lack information on the thermal thresholds of A. marina in the Central Red Sea as well as on the consequences of a low fresh water supply for the performance of the plants. These responses, along with the implications of nutrient limitation in modulating the resistance to stress, should be targeted by future research.
Conclusion
In conclusion, nutrient concentrations in A. marina leaves point to the presence of nutrient-deficient mangrove stands in the Central Red Sea, with particularly low concentrations of phosphorus and iron. Moreover, experimental nutrient addition confirms iron to be the primary limiting nutrient for A. marina seedlings in the Central Red Sea. The stunted nature of Red Sea mangroves, with heights typically below 2 m, is, therefore, likely the result of acute iron limitation, although only long-term fertilization experiments can confirm this suggestion. The iron-limited nature of Red Sea A. marina stands is consistent with the biogenic nature of the sediments in the Red Sea, dominated by carbonates, and the lack of riverine sources of iron. We submit that dust deposition may be the most important source of iron to undisturbed mangrove stands, although anthropogenic nutrient inputs, which are still highly localized along the Red Sea, can also locally elevate iron and phosphorus concentrations in the receiving stands.
Author Contributions
HA, CD, and XI designed the study, HA carried out the experimental and analytical measurements, HA, CD, and XI did the statistical analysis, and HA, CD, and XI wrote the manuscript.
Conflict of Interest Statement
The authors declare that the research was conducted in the absence of any commercial or financial relationships that could be construed as a potential conflict of interest.
Acknowledgments
We thank KAUST workshops, Coastal and Marine Resources Core Labs, and Analytical core lab for help with sampling and analyses and the Presidency of Meteorology and Environment (PME) for providing weather data. We also thank Joao Curdia for help with the nutrient addition experiment and Vincent Saderne for providing data on light levels under mangrove canopies in the region. We also thank Virginia Unkefer for reviewing the manuscript and Heno Hwang for his illustration of the nursery experiment. The research reported in this paper was supported by King Abdullah University of Science and Technology.
Supplementary Material
The Supplementary Material for this article can be found online at: http://journal.frontiersin.org/article/10.3389/fmars.2016.00271/full#supplementary-material
Table S1. Data for the weather conditions during the experiment. Data were provided by the Presidency of Meteorology and Environment (PME).
Table S2. Mean (± SE) nutrient concentration (mmol g DW-1) in fully developed apical leaves (n = 3) of Avicennia marina seedlings grown under different experimental nutrient addition treatments. R2- and F-value correspond to an ANOVA testing for significant differences between treatments over time. *P between 0.01 and 0.05, **P < 0.01. Treatments linked with the same letter did not differ significantly among themselves (Tuckey HSD multiple comparison post-hoc test, P > 0.05).
Table S3. Mean (± SE) for nutrients concentrations (mmol g DW-1) in the experimental fully developed apical leaves of Avicennia marina seedlings comparing Iron with EDTA (n = 3). R2- and F-value correspond to an ANOVA testing for significant differences between treatments. *P between 0.01 and 0.05, **P < 0.01. Treatments linked with the same letter did not differ significantly among themselves (Tuckey HSD multiple comparison post-hoc test, P > 0.05).
References
Aerts, R., and Chapin, F. S. (1999). The mineral nutrition of wild plants revisited: a re-evaluation of processes and patterns. Adv. Ecol. Res. 30, 1–67. doi: 10.1016/S0065-2504(08)60016-1
Al-Farawati, R. (2011). Spatial and seasonal distribution of total dissolved copper and nickel in the surface coastal waters of Rabigh, Eastern Red Sea, Saudi Arabia. J. King Abdulaziz Univ. Earth Sci. 22, 29–44. doi: 10.4197/ear.22-1.2
Almahasheer, H., Aljowair, A., Duarte, C. M., and Irigoien, X. (2016a). Decadal stability of Red Sea mangroves. Estuar. Coast. Shelf Sci. 169, 164–172. doi: 10.1016/j.ecss.2015.11.027
Almahasheer, H., Duarte, C. M., and Irigoien, X. (2016b). Phenology and Growth dynamics of Avicennia marina in the Central Red Sea. Sci. Rep. 6:37785. doi: 10.1038/srep37785
Alongi, D. (2011). Early growth responses of mangroves to different rates of nitrogen and phosphorus supply. J. Exp. Mar. Biol. Ecol. 397, 85–93. doi: 10.1016/j.jembe.2010.11.021
Alongi, D. M. (2010). Dissolved iron supply limits early growth of estuarine mangroves. Ecology 91, 3229–3241. doi: 10.1890/09-2142.1
Atkinson, M., and Smith, S. (1983). C:N:P ratios of benthic marine plants. Limnol. Oceanogr. 28, 568–574. doi: 10.4319/lo.1983.28.3.0568
Balk, M., Keuskamp, J. A., and Laanbroek, H. J. (2015). Potential activity, size, and structure of sulfate-reducing microbial communities in an exposed, grazed and a sheltered, non-grazed mangrove stand at the Red Sea Coast. Front. Microbiol. 6:1478. doi: 10.3389/fmicb.2015.01478
Ball, M., Chow, W., and Anderson, J. (1987). Salinity-induced potassium deficiency causes loss of functional photosystem II in leaves of the grey mangrove, Avicennia marina, through depletion of the atrazine-binding polypeptide. Funct. Plant Biol. 14, 351–361. doi: 10.1071/pp9870351
Banguera-Hinestroza, E., Eikrem, W., Mansour, H., Solberg, I., Cúrdia, J., Holtermann, K., et al. (2016). Seasonality and toxin production of Pyrodinium bahamense in a Red Sea lagoon. Harmful Algae 55, 163–171. doi: 10.1016/j.hal.2016.03.002
Brindley, H., Osipov, S., Bantges, R., Smirnov, A., Banks, J., Levy, R., et al. (2015). An assessment of the quality of aerosol retrievals over the Red Sea and evaluation of the climatological cloud-free dust direct radiative effect in the region. J. Geophys. Res. 120, 10862–10878. doi: 10.1002/2015jd023282
Bruckner, A. (2011). Khaled bin Sultan Living Oceans Foundation Habitat Mapping and Characterization of Coral Reefs of the Saudi Arabian Red Sea: 2006–2009. Final Report Part I. Phoenix: Panoramic Press.
Churchill, J. H., Bower, A. S., McCorkle, D. C., and Abualnaja, Y. (2014). The transport of nutrient-rich Indian Ocean water through the Red Sea and into coastal reef systems. J. Mar. Res. 72, 165–181. doi: 10.1357/002224014814901994
Clarke, A., and Johns, L. (2002). Mangrove Nurseries: Construction, Propagation and Planting: Fisheries Guidelines. Queensland: Department of Primary Industries, Queensland. Fish Habitat Guideline FHG 4.
Clough, B. (1984). Growth and salt balance of the mangroves Avicennia marina (Forsk.) Vierh. and Rhizophora stylosa Griff. in relation to salinity. Funct. Plant Biol. 11, 419–430.
Connor, D. (1969). Growth of grey mangrove (Avicennia marina) in nutrient culture. Biotropica 1, 36–40. doi: 10.2307/2989759
Douabul, A., and Haddad, A. M. (1970). The Red Sea and Yemen's Red Sea Environments. Yemen: Hassell and Assoc., AMSAT and UNOPS, 1–16.
Duarte, C. M. (1990). Seagrass nutrient content. Mar. Ecol. Prog. Ser. 6, 201–207. doi: 10.3354/meps067201
Duarte, C. M. (1992). Nutrient concentration of aquatic plants: patterns across species. Limnol. Oceanogr. 37, 882–889. doi: 10.4319/lo.1992.37.4.0882
Duarte, C. M., Geertz-Hansen, O., Thampanya, U., Terrados, J., Fortes, M. D., Kamp-Nielsen, L., et al. (1998). Relationship between sediment conditions and mangrove Rhizophora apiculata seedling growth and nutrient status. Mar. Ecol. Prog. Ser. 175, 277–283. doi: 10.3354/meps175277
Duarte, C. M., Martín, M., and Margarita, G. (1995). Evidence of iron deficiency in seagrasses growing above carbonate sediments. Limnol. Oceanogr. 40, 1153–1158. doi: 10.4319/lo.1995.40.6.1153
Edwards, F. J. (1987). Climate and oceanography. Red Sea 1, 45–68. doi: 10.1016/B978-0-08-028873-4.50008-6
Enriquez, S., Duarte, C. M., and Sand-Jensen, K. (1993). Patterns in decomposition rates among photosynthetic organisms: the importance of detritus C:N:P content. Oecologia 94, 457–471. doi: 10.1007/BF00566960
Eshel, G., and Naik, N. H. (1997). Climatological coastal jet collision, intermediate water formation, and the general circulation of the Red Sea*. J. Phys. Oceanogr. 27, 1233–1257. doi: 10.1175/1520-0485(1997)027<1233:CCJCIW>2.0.CO;2
Evans, P. G. (1987). “Sea birds of the Red Sea,” in Red Sea: Key Environments, eds A. J. Edwards and S. M. Head (Oxford: Pergamon Books Ltd.), 315–338.
Feller, I. C. (1995). Effects of nutrient enrichment on growth and herbivory of dwarf red mangrove (Rhizophora mangle). Ecol. Monogr. 65, 477–505. doi: 10.2307/2963499
Feller, I. C., McKee, K. L., Whigham, D. F., and O'Neill, J. P. (2003a). Nitrogen vs. phosphorus limitation across an ecotonal gradient in a mangrove forest. Biogeochemistry 62, 145–175. doi: 10.1023/A:1021166010892
Feller, I. C., Whigham, D. F., McKee, K. L., and Lovelock, C. E. (2003b). Nitrogen limitation of growth and nutrient dynamics in a disturbed mangrove forest, Indian River Lagoon, Florida. Oecologia 134, 405–414. doi: 10.1007/s00442-002-1117-z
Gheith, A. M., and Abou-ouf, M. A. (1996). Textural characteristics, mineralogy and fauna in the shore zone sediments at Rabigh and Sharm Al-Kharrar, Eastern Red Sea, Saudi Arabia. Mar. Sci. 17, 1–2. doi: 10.4197/mar.7-1.10
Hart, J. R. (2000). “Ethylenediaminetetraacetic acid and related chelating agents,” in Ullmann's Encyclopedia of Industrial Chemistry. (Weinheim: Verlag GmbH & Co. KGaA), 1–7. doi: 10.1002/14356007.a10_095
Ismael, A. A. (2015). “Phytoplankton of the Red Sea,” in The Red Sea, eds N. M. A. Rasul and I. C. F. Stewart (Berlin; Heidelberg: Springer-Verlag), 567–583. doi: 10.1007/978-3-662-45201-1_32
Jish Prakash, P., Stenchikov, G., Kalenderski, S., Osipov, S., and Bangalath, H. (2015). The impact of dust storms on the Arabian Peninsula and the Red Sea. Atmos. Chem. Phys. 15, 199–222. doi: 10.5194/acp-15-199-2015
Johns, W. E., and Sofianos, S. S. (2012). Atmospherically forced exchange through the Bab el Mandeb Strait. J. Phys. Oceanogr. 42, 1143–1157. doi: 10.1175/JPO-D-11-0157.1
Koch, M. S. (1997). Rhizophora mangle L. seedling development into the sapling stage across resource and stress gradients in subtropical Florida. Biotropica 29, 427–439. doi: 10.1111/j.1744-7429.1997.tb00037.x
Koch, M. S., and Snedaker, S. C. (1997). Factors influencing Rhizophora mangle L. seedling development in Everglades carbonate soils. Aquat. Bot. 59, 87–98. doi: 10.1016/S0304-3770(97)00027-2
Krauss, K. W., Lovelock, C. E., McKee, K. L., López-Hoffman, L., Ewe, S. M., and Sousa, W. P. (2008). Environmental drivers in mangrove establishment and early development: a review. Aquat. Bot. 89, 105–127. doi: 10.1016/j.aquabot.2007.12.014
Lovelock, C. E., Feller, I. C., Ball, M. C., Engelbrecht, B. M., and Ewe, M. L. (2006). Differences in plant function in phosphorus- and nitrogen-limited mangrove ecosystems. New Phytol. 172, 514–522. doi: 10.1111/j.1469-8137.2006.01851.x,
Lovelock, C., Feller, I. C., McKee, K., Engelbrecht, B., and Ball, M. (2004). The effect of nutrient enrichment on growth, photosynthesis and hydraulic conductance of dwarf mangroves in Panama. Funct. Ecol. 18, 25–33. doi: 10.1046/j.0269-8463.2004.00805.x
Lü, X. T., Freschet, G. T., Flynn, D. F., and Han, X. G. (2012). Plasticity in leaf and stem nutrient resorption proficiency potentially reinforces plant–soil feedbacks and microscale heterogeneity in a semi-arid grassland. J. Ecol. 100, 144–150. doi: 10.1111/j.1365-2745.2011.01881.x
Mahowald, N. M., Engelstaedter, S., Luo, C., Sealy, A., Artaxo, P., Benitez-Nelson, C., et al. (2009). Atmospheric iron deposition: global distribution, variability, and human perturbations*. Ann. Rev. Mar. Sci. 1, 245–278. doi: 10.1146/annurev.marine.010908.163727
Mandura, A. (1997). A mangrove stand under sewage pollution stress: Red Sea. Mangroves Salt Marshes 1, 255–262. doi: 10.1023/A:1009927605517
Mandura, A., Saifullah, S., and Khafaji, A. (1987). Mangrove ecosystem of Southern Red Sea Coast of Saudi Arabia. Proc. Saudi Biol. Soc 10, 165–193.
McGroddy, M. E., Daufresne, T., and Hedin, L. O. (2004). Scaling of C:N:P stoichiometry in forests worldwide: implications of terrestrial Redfield-type ratios. Ecology 85, 2390–2401. doi: 10.1890/03-0351
McKee, K. L. (1995). Interspecific variation in growth, biomass partitioning, and defensive characteristics of neotropical mangrove seedlings: response to light and nutrient availability. Am. J. Bot. 82, 299–307. doi: 10.2307/2445575
Murray, S. P., and Johns, W. (1997). Direct observations of seasonal exchange through the Bab el Mandab Strait. Geophys. Res. Lett. 24, 2557–2560. doi: 10.1029/97GL02741
Naidoo, G. (2009). Differential effects of nitrogen and phosphorus enrichment on growth of dwarf Avicennia marina mangroves. Aquat. Bot. 90, 184–190. doi: 10.1016/j.aquabot.2008.10.001
Ochieng, C. A., and Erftemeijer, P. L. (2002). Phenology, litterfall and nutrient resorption in Avicennia marina (Forssk.) Vierh in Gazi Bay, Kenya. Trees 16, 167–171. doi: 10.1007/s00468-001-0146-2
Pearman, J., Kürten, S., Sarma, Y., Jones, B., and Carvalho, S. (2016). Biodiversity patterns of plankton assemblages at the extremes of the Red Sea. FEMS Microbiol. Ecol. 92, 1–13. doi: 10.1093/femsec/fiw002
Polidoro, B. A., Carpenter, K. E., Collins, L., Duke, N. C., Ellison, A. M., Ellison, J. C., et al. (2010). The loss of species: mangrove extinction risk and geographic areas of global concern. PLoS ONE 5:e10095. doi: 10.1371/journal.pone.0010095
Poulton, S., and Raiswell, R. (2002). The low-temperature geochemical cycle of iron: from continental fluxes to marine sediment deposition. Am. J. Sci. 302, 774–805. doi: 10.2475/ajs.302.9.774
Raiswell, R. (2006). Towards a global highly reactive iron cycle. J. Geochem. Explor. 88, 436–439. doi: 10.1016/j.gexplo.2005.08.098
Raitsos, D. E., Pradhan, Y., Brewin, R. J., Stenchikov, G., and Hoteit, I. (2013). Remote sensing the phytoplankton seasonal succession of the Red Sea. PLoS ONE 8:e64909. doi: 10.1371/journal.pone.0064909
Raitsos, D. E., Yi, X., Platt, T., Racault, M. F., Brewin, R. J., Pradhan, Y., et al. (2015). Monsoon oscillations regulate fertility of the Red Sea. Geophys. Res. Lett. 42, 855–862. doi: 10.1002/2014GL062882
Reef, R., Feller, I. C., and Lovelock, C. E. (2010). Nutrition of mangroves. Tree Physiol. 30, 1148–1160. doi: 10.1093/treephys/tpq048
Rushdi, A. I. (2015). “Calcite and aragonite saturation states of the Red Sea and biogeochemical impacts of excess carbon dioxide,” in The Red Sea, eds N. M. A. Rasul and I. C. F. Stewart (Berlin; Heidelberg: Springer-Verlag), 267–279. doi: 10.1007/978-3-662-45201-1_16
Salt, D. E., Smith, R., and Raskin, I. (1998). Phytoremediation. Annu. Rev. Plant Biol. 49, 643–668. doi: 10.1146/annurev.arplant.49.1.643
Sato, G., Negassi, S., and Tahiri, A. Z. (2011). The only elements required by plants that are deficient in seawater are nitrogen, phosphorous and iron. Cytotechnology 63, 201–204. doi: 10.1007/s10616-011-9342-0
Schroth, A. W., Crusius, J., Sholkovitz, E. R., and Bostick, B. C. (2009). Iron solubility driven by speciation in dust sources to the ocean. Nat. Geosci. 2, 337–340. doi: 10.1038/ngeo501
Steiner, A. A., and van Winden, H. (1970). Recipe for ferric salts of ethylenediaminetetraacetic acid. Plant Physiol. 46:862. doi: 10.1104/pp.46.6.862
Sultan, S., Ahmad, F., and Elghribi, N. (1996). Sea level variability in the Central Red Sea. Oceanol. Acta 19, 607–615.
Talley, L. D. (2002). “Salinity patterns in the ocean,” in Encyclopedia of Global Change, Vol. 1, The Earth System: Physical and Chemical Dimensions of Global Environmental Change, eds M. C. MacCracken and J. S. Perry (Chichester: John Wiley & Sons, Ltd.), 629–640.
Thompson, L. R., Field, C., Romanuk, T., Ngugi, D., Siam, R., Dorry, H., et al. (2013). Patterns of ecological specialization among microbial populations in the Red Sea and diverse oligotrophic marine environments. Ecol. Evol. 3, 1780–1797. doi: 10.1002/ece3.593
Triantafyllou, G., Yao, F., Petihakis, G., Tsiaras, K., Raitsos, D., and Hoteit, I. (2014). Exploring the Red Sea seasonal ecosystem functioning using a three-dimensional biophysical model. J. Geophys. Res. Oceans 119, 1791–1811. doi: 10.1002/2013jc009641
Wellburn, A. R. (1994). The spectral determination of chlorophylls a and b, as well as total carotenoids, using various solvents with spectrophotometers of different resolution. J. Plant Physiol. 144, 307–313. doi: 10.1016/S0176-1617(11)81192-2
Yao, F., Hoteit, I., Pratt, L. J., Bower, A. S., Zhai, P., Köhl, A., et al. (2014). Seasonal overturning circulation in the Red Sea: 1. Model validation and summer circulation. J. Geophys. Res. Oceans 119, 2238–2262. doi: 10.1002/2013jc009004
Zimmermann, C. F., Keefe, C. W., and Bashe, J. (1997). Method 440.0: Determination of Carbon and Nitrogen in Sediments and Particulates of Estuarine/Coastal Waters Using Elemental Analysis. United States Environmental Protection Agency, Office of Research and Development, National Exposure Research Laboratory.
Keywords: Avicennia marina, ratios, Chl a content, carbon, nitrogen, phosphorous, iron
Citation: Almahasheer H, Duarte CM and Irigoien X (2016) Nutrient Limitation in Central Red Sea Mangroves. Front. Mar. Sci. 3:271. doi: 10.3389/fmars.2016.00271
Received: 13 May 2016; Accepted: 07 December 2016;
Published: 22 December 2016.
Edited by:
Christos Dimitrios Arvanitidis, Hellenic Centre for Marine Research, GreeceReviewed by:
Dionysios E. Raitsos, Plymouth Marine Laboratory, UKEmily Dangremond, Roosevelt University, USA
Copyright © 2016 Almahasheer, Duarte and Irigoien. This is an open-access article distributed under the terms of the Creative Commons Attribution License (CC BY). The use, distribution or reproduction in other forums is permitted, provided the original author(s) or licensor are credited and that the original publication in this journal is cited, in accordance with accepted academic practice. No use, distribution or reproduction is permitted which does not comply with these terms.
*Correspondence: Hanan Almahasheer, aGFuYW4uYWxtYWhhc2hlZXJAa2F1c3QuZWR1LnNh
†Present Address: Xabier Irigoien, AZTI - Marine Research, Pasaia, Spain; IKERBASQUE, Basque Foundation for Science, Bilbao, Spain