- 1Department of Biology and Chemistry, University of Bremen, Bremen, Germany
- 2Centro de Estudios Avanzados de Blanes, Consejo Superior de Investigaciones Cientificas, Blanes, Spain
- 3Center for Marine Ecosystems Research, Edith Cowan University, Perth, WA, Australia
- 4Leibniz Centre for Tropical Marine Research, Bremen, Germany
The recent surge in research on organic carbon sequestration by seagrass ecosystems has begun to reveal the complexity of the carbon cycle within these ecosystems. In this prospective we discuss two areas of investigation that require further scrutiny: (1) why organic carbon is stabilized in seagrass sediments, and (2) how long organic carbon resides within these sediments. By delving into these topics, pointing out current pitfalls, and highlighting methodological advances, our motive is to focus future efforts and provide a frame work to manage the complexity found within the diverse seagrass bioregions. The high rate of seagrass degradation and loss, coupled with increasing atmospheric CO2 concentrations gives precedence to these lines of research, which require rigorous reevaluation if we are to substantially advance our understanding of OC dynamics in seagrass ecosystems.
Introduction
Seagrasses provide key ecological functions and services to coastal ecosystems, including sediment stabilization, coastline protection, nutrient cycling, support of fisheries, and enhancement of biodiversity (de la Torre-Castro and Rönnbäck, 2004; Duffy, 2006; Orth et al., 2006) due to the role they play as ecosystem engineers trapping sediment, altering hydrodynamics, and modifying biogeochemical processes in the water column and sediment (Marbá et al., 2006). From when seagrass meadows were recognized as potentially important “blue” carbon (organic carbon sequestered by vegetated coastal ecosystems) sinks, there has been a surge in efforts to determine the magnitude and variability of organic carbon (OC) storage within their sediments (Nellemann et al., 2009; Duarte et al., 2010, 2013; Fourqurean et al., 2012; Lavery et al., 2013; Campbell et al., 2014; Macreadie et al., 2014; Serrano et al., 2014, 2015, 2016b; Miyajima et al., 2015; Phang et al., 2015; Armitage and Fourqurean, 2016; Dahl et al., 2016; Jankowska et al., 2016; Röhr et al., 2016; Samper-Villarreal et al., 2016; Schile et al., 2016). Here, we point out two notable knowledge gaps, along with current methodological pitfalls, that are obscuring our ability to understand seagrass OC dynamics. First, we explore why OC is stabilized in coastal marine sediments, with emphasis on the need to look beyond a unifying mechanism governing OC storage across all seagrass ecosystems. Second, we address the question of how long OC is stored in seagrass sediments, with focus on how current methodologies may give misleading estimates of OC accumulation rates and residence times. Both of these topics have a rich a history of exploration and by pointing out common stumbling blocks our quest is to clarify the obstacles we face and propose a frame work for a direction forward. We hope to open dialogue and refocus efforts, so we do not repeat the past two decades of research, but instead utilize and build upon it to understand the unique dynamics of OC cycling in coastal marine sediments. Accelerating seagrass degradation and loss (Waycott et al., 2009) and rising atmospheric CO2 concentrations (IPCC, 2013) emphasize the relevance and timeliness of this discussion.
Why is OC Stabilized in Seagrass Sediments?
Although it is important to identify where high carbon storage occurs in coastal marine landscapes, it is equally important to correctly identify why. In the past 5 years there has been a concerted effort to determine which environmental or biological variables predict carbon stock location and size across the broad range of seagrass bioregions (Lavery et al., 2013; Serrano et al., 2014, 2015, 2016a; Miyajima et al., 2015; Dahl et al., 2016; Röhr et al., 2016; Samper-Villarreal et al., 2016). An unintended consequence of searching for these broad unifying predictors is the potential to narrow our understanding of OC dynamics, which creates the risk of misidentifying why OC is stabilized within seagrass sediments. Within the process of OC stabilization, there is often a mechanism (crucial step or physiochemical condition) that disproportionally affects the stabilization or destabilization of organic matter (Torn et al., 2009). In the past two decades a paradigm shift in carbon research has broadened focus to include four primary mechanisms of OC stabilization (Sollins et al., 1996; Six et al., 2004; Burdige, 2007; Marschner et al., 2008; Torn et al., 2009; Trumbore, 2009; Schmidt et al., 2011):
• Inherent molecular characteristics of organic matter (recalcitrance).
• Physical stabilization of OC on mineral surfaces.
• Inaccessibility of OC to microbes due to barriers of interaction among microbes and substrates, such as occlusion of OC within aggregates.
• Biotic suppression of microbial abundance and/or activity due to factors such as freezing temperatures, extreme pH, or low O2 content.
However, there is still a tendency within seagrass OC research to focus on correlative attributes of the seagrass, landscape, or sediment without direct investigation into the mechanism(s) of stabilization. Many propose carbon source mechanisms (recalcitrance) when explaining OC stabilization based largely on correlative isotopic (13C) evidence and seagrass tissue stoichiometry (C:N ratios, (Kennedy et al., 2010; Duarte et al., 2013; Serrano et al., 2015; Röhr et al., 2016). To definitively determine when, where, and to what extent recalcitrance controls OC stability in seagrass sediments there needs to be further research into the molecular composition of the OC (Vichkovitten and Holmer, 2005; Kaal et al., 2016), in tandem with rigorous investigation into its decomposability under the physical conditions experienced within the sediment. Several studies have shown sediment characteristics, such as grain size and type, to be important predictors for seagrass OC stocks (Dahl et al., 2016; Röhr et al., 2016; Serrano et al., 2016a), implying that biotic suppression (due to low O2 content within fine grain sediment) or physical stabilization on certain sediment types are potentially important mechanisms. The next steps must be taken to investigate molecular composition and decomposability, along with sediment mineralogy and geochemistry, and microbial diversity and activity (Mikutta et al., 2006; Chabbi et al., 2009; Schmidt et al., 2011; Simpson and Simpson, 2012; Macreadie et al., 2015; Bracho et al., 2016), if we are to draw definitive conclusions about why OC is stabilized in seagrass sediments.
Here, we outline some of the existing methodologies that can aid in our understanding of OC stabilization (Table 1). We have grouped methods under four broad lines of investigation: (1) determining the molecular composition of OC; (2) mapping the physical and chemical structure of the sediment environment; (3) tracing pathways and timelines of OC; and (4) revealing the identity, function, and activities of the sediment community. To substantially advance our understanding of OC dynamics in coastal marine ecosystems it will require a collaborative effort across disciplines, utilizing this broad spectrum of methodologies. However, this does not negate the importance of the continued efforts and expert knowledge of seagrass ecologists, who provide valuable insights and data required to understand the complex spatial and temporal variation of OC stabilization across seagrass ecosystems.
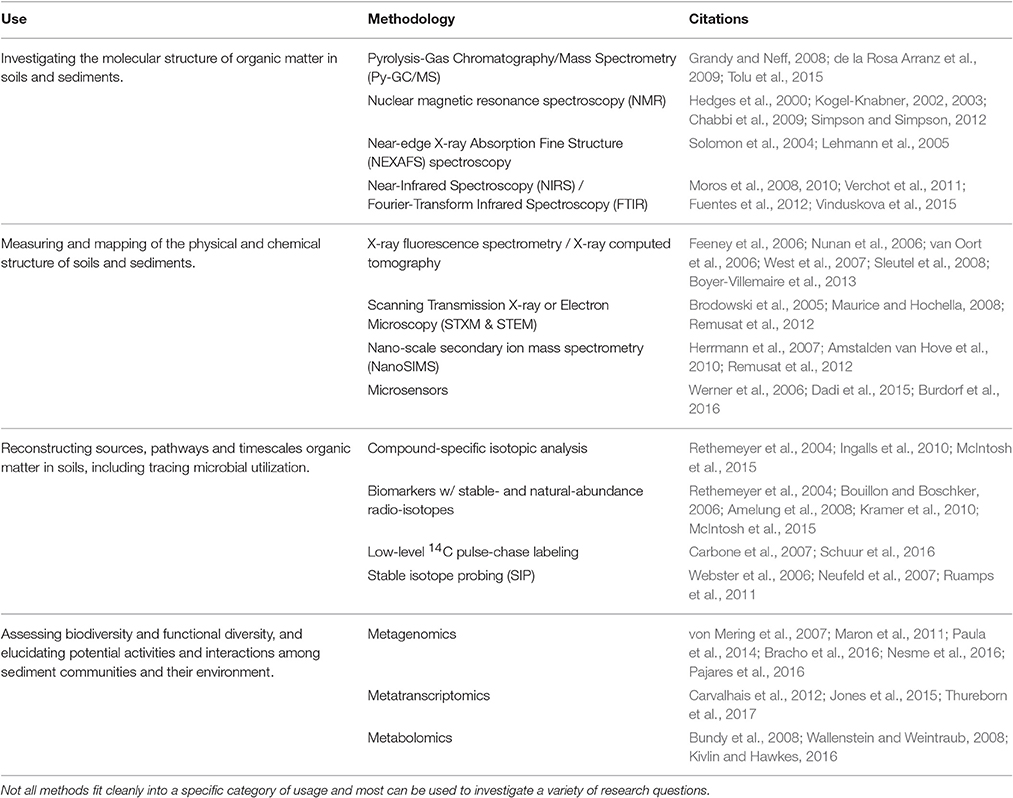
Table 1. Some of the existing methodologies utilized to aid in the understanding of organic carbon stabilization in soils and sediments.
How Long is OC Stabilized in Seagrass Sediments?
The second major knowledge gap we face is in our understanding of the timelines of OC stabilization within coastal marine sediments. This information, in addition to pool size, is critical for understanding the influence of seagrass carbon reservoirs on atmospheric CO2 concentrations. The ability of a reservoir to act as a net sink depends on both the fluxes of carbon into the reservoir and the timescale it is stabilized within it (Trumbore, 2000). Only systems with long carbon residence times (decades or longer) act as important mitigators for climate change.
The need to quantify timelines of carbon storage within seagrass sediments was pointed out two decade ago (Mateo et al., 1997, 2006); however, carbon accumulation rates and residence times have been reported based on estimates from age-depth relationships acquired by dating material within sediment layers (Mateo et al., 1997, 2010; Serrano et al., 2012, 2014, 2015, 2016b,c; Miyajima et al., 2015; Jankowska et al., 2016; Rozaimi et al., 2016) or by simply assuming sedimentation rates equal OC accumulation rates (Duarte et al., 2004; Kennedy et al., 2010; McLeod et al., 2011; Lavery et al., 2013; Macreadie et al., 2014; Röhr et al., 2016). These methodologies lead to an overestimation of OC ages and accumulation rates because they do not take into account decomposition losses (however small) from deeper sediment layers (Clymo, 1984; Korhola et al., 1995; Mateo et al., 1997). Furthermore, they ignore the addition of younger carbon (via root turnover and exudates) deeper in the sediment profile (Duarte et al., 1998, 2005). Even though many marine sediments vertically accrete, we must consider that OC does not accumulate in a static fashion, after deposition it continues to be cycled by microbes where it can be transformed and/or lost from the reservoir at rates that depend on the efficacy of the stabilization mechanisms (Torn et al., 2009). Additionally, seagrasses are vascular plants that transfer OC throughout the plant and exudate an estimated 6–17% of production via roots (Moriarty et al., 1986), which equates to various ages of carbon continuously entering the sediment pool.
Models can improve estimates of OC accumulation rates and residence times but have limitations. In an effort to account for OC decomposition, Clymo (1984) adapted a simple one-pool “box” model fit to cumulative carbon vs. age to achieve a more reasonable accumulation estimate that decreases as the stock grows. In this model decomposition is represented as a first-order process: dC/dt = − kCt, where C is the amount of carbon at a given time and k is the decomposition rate, and the residence time (also known as transit time) of the carbon within the pool is 1/k. To better represent sediment OC dynamics, which have continual OC inputs, this model was adapted as a multi-input system (vs. the decay of a single input; Trumbore and Harden, 1997; Manzoni et al., 2009, 2012; Hicks Pries et al., 2012), where both the decay constant and inputs can be estimated. Although these types of models have a rich history of usage for modeling OC dynamics, they are typically fit to data of mass loss through time (i.e., litter decomposition) or fluxes of CO2 (incubations; Eriksson, 1971; Bosatta and Agren, 1985; Ågren and Bosatta, 1996; Manzoni et al., 2009, 2012; Cornwell and Weedon, 2014). To fit them to data of cumulative OC vs. time (dated depths) from a sediment core the following assumptions must be made: (a) the system is in steady state; (b) initial OC in the sediment is zero; (c) carbon is accreted vertically (no inputs of new OC below dated depths); (d) the dated material is equal to the age of the bulk OC at the same depth in the sediment profile (see below); (e) there are no changes in OC dynamics through time, so recent OC dynamics (top of core) are representative of initial OC dynamics of the system. According to the above critique, none of these assumption holds true for the actual situation and OC dynamics in marine sediments. On top of this, it is assumed the entire core is one pool of OC, all residing for the same time period, and although multi-pool box models exist, the data from sediment cores often do not support the increased model complexity. Furthermore, the use of cumulative data, which are not independent, leads to unrealistic confidence in model estimates (due to underestimation of model uncertainty; King et al., 2015). In spite of these limitations, these models have proven to be useful for providing more realistic estimates of OC accumulation and residence time of OC within non-vascular dominated, vertically-accreting ecosystems (Clymo, 1984; Trumbore and Harden, 1997; Hicks Pries et al., 2012). However, extreme caution must be used when applying them to sediment-core data from vascular plant dominated ecosystems such as seagrass meadows.
Another factor that influences estimates of OC ages and residence times is the type of material used for dating. Previous works in seagrass ecosystems have utilized closed-system materials (assumed to be no longer exchanging carbon with the environment), such as shells or sheaths, for radiocarbon (14C) dating (Mateo et al., 1997, 2010; Serrano et al., 2012, 2014, 2015, 2016b,c; Rozaimi et al., 2016). Because sediment OC consist of a spectrum of pools cycling on different time scales, choosing the oldest, most stable carbon within the sediment and ignoring all other OC (of a variety of ages) residing at the same depth in the sediment profile, leads to an overestimation of OC ages and residence times (Trumbore and Zheng, 1996; Torn et al., 2009; Trumbore, 2009; Trumbore et al., 2016). To illustrate the potential for overestimation, OC ages have been shown to vary by three orders of magnitude, from 3 years (Δ14C = +170‰) to ~3,000 (Δ14C = −310‰) years, within a 5-cm sediment layer in tropical forest soils (Trumbore and Zheng, 1996; Trumbore, 2009). Therefore, when the goal is to understand OC dynamics, and estimates of residence times of the various carbon pools are needed (as opposed to exact calendar ages), a different methodological approach is required.
Because of the limitations outlined above we suggest an alternative methodology utilized in terrestrial systems over the past two decades (Trumbore, 1993, 2000, 2009; Schuur et al., 2016). Organic matter is more appropriately thought of as a heterogeneous open-system (continuously exchanging carbon with its environment), so directly measuring 14C of the bulk sediment OC pool along with manually separating and dating pools (that are relatively homogenous in terms of decomposition rates) provide more realistic estimates of the suite OC ages occurring within the sediment (Trumbore, 2009; Schuur et al., 2016; Trumbore et al., 2016). Since OC is a complex mixture of compounds that cycle along a continuum of time scales from minutes to millennia, no separation method will be perfect. However, methods exist to partition OC into various pools, which allows for the determination of both pool size and residence time (Trumbore and Zheng, 1996; von Lützow et al., 2007; Trumbore, 2009; Trumbore et al., 2016). The same linear-system box models can be adapted to estimate the residence time of 14C of each pool:
where λ is the radioactive decay constant (1.21 × 10−4 yr−1), I*14C(t) are the inputs of 14C based off a time series of 14C from of the reservoir the plant uptakes CO2 from, and k*14C(t) are the estimated outputs. These models can also be extended to multi-pool models and only requires a measurement from your desired pool of either the F (fraction modern prior to 1950) or F' (fraction modern that includes bomb 14C) and a bomb carbon time series of F' of the reservoir (Manzoni et al., 2009, 2012; Trumbore et al., 2016). For terrestrial systems, the time series of the reservoir (atmosphere) F' is well known (Hua et al., 2013), but becomes more complicated in marine systems because of the reservoir effect (Mangerud, 1972; Stuiver et al., 1986). However, in many coastal systems, post-bomb 14C time series exist (Weidman and Jones, 1993; Kalish et al., 2001; Mahadevan, 2001; Kilada et al., 2007; Scourse et al., 2012; Tisnérat-Laborde et al., 2013). Another potential hurdle in marine systems is the presence of terrestrial carbon (fixed from the atmospheric pool), so care must be taken to disentangle these signals (Marshall et al., 2007; Yu et al., 2007). Although there are some hurdles, this methodology avoids complications created by simplistic assumptions of carbon ages and depths, and provides direct measurements of residence times directly linked to pools of known sizes and locations. These techniques could be used in tandem with attributes of the OC pool and sediment (such as mineralogy, redox, and molecular composition), to advance our understanding of the timescales over which different stabilization mechanisms operate (Mikutta et al., 2006; Chabbi et al., 2009).
A Way Forward
Here, we propose a conceptual framework to focus research efforts across the diverse environments where seagrasses occur. This framework is adapted from the Jenny (1941) state-factor system utilized in terrestrial ecosystems, to incorporate factors identified for subaqueous soils (Demas and Rabenhorst, 2001; Torn et al., 2009; Trumbore, 2009). In this model a soil property such as OC storage is described by the function:
where C = climate, O = organism (vegetation), B = bathymetry, F = flow regime, P = parent material, T = time). These factors set the boundary conditions and modulate the mechanisms that determine the magnitude and timescales of carbon stabilization (Figure 1). By selecting study sites where one state-factor varies, while the other factors are held relatively constant, we can begin to identify the role each factor plays and what mechanism(s) operate within the unique combination of state-factors that occur throughout the seagrass bioregions (Short et al., 2007). For example, within Posidonia spp. meadows OC storage varies predictably with bathymetry in sheltered bays (flow regime) in both the Mediterranean and Western Australia (Serrano et al., 2014), with the difference in magnitude of OC storage between the locations potentially explained by variations between seagrass species (organism-vegetation). The usefulness of this framework can be further illustrated by the finding that across the European geographic distribution (climate) of Zostera marina (organism-vegetation), OC can be predicted by sediment grain size (parent material and/or flow regime; (Dahl et al., 2016). Furthermore, in two sites with contrasting sediment characteristics they found that OC did not decrease with sediment depth (indicating minimal decomposition over time), which lead to the hypothesis that different stabilization mechanisms were at play: a) biotic suppression due to low oxygen availability in the fine-grain site, and b) recalcitrance of allochthonous carbon inputs in the course-grain site. This framework can also potentially explain why sediment grain size does not correlate to OC storage when looking across a mixture of different parent materials, climates, seagrass species, flow regimens, and bathymetry (Serrano et al., 2016a). We believe that embracing the complexity found within seagrass ecosystems and utilizing the state-factor system to systematically identify which mechanism(s) control the spatial and temporal variations of OC is a way forward to understand carbon dynamics in coastal marine systems.
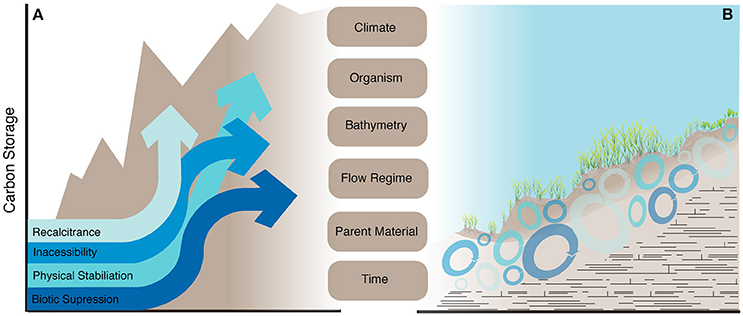
Figure 1. Conceptual diagram of (A) the four OC stabilization mechanisms operating over time (x-axis) that lead to an increase of OC storage, represented by the increasing brown shaded area behind the arrows. Each mechanism can work alone or in tandem, and the strength of each can potentially change over time, which is illustrated by the changes in slope and direction of the arrow heads. The relative importance and strength of the four mechanisms are modulated by the six state-factors (Jenny, 1941; Demas and Rabenhorst, 2001). To illustrate (B) is a hypothetical seagrass meadow with OC cycling over a spectrum of timescales (illustrated by different sized circles) with the four stabilization mechanisms (represented by the various blue shades) dominating in different locations. The exact location and prevalence of each mechanism(s), with their inherent effects on OC residence times, will ultimately depend on the unique combination of state factors, which set the boundary conditions for the process of OC stabilization.
Conclusion
As policy makers and managers identify pathways to conserve seagrass systems embedded under a blue carbon framework (Herr et al., 2012), the potential for OC sequestration opens the door for both positive and negative consequences. On the positive side, this creates an avenue for conservation of these valuable ecosystems, along with their well-established ecosystem services. On the negative side, CO2 will be emitted in exchange for carbon credits, resulting in an increase in atmospheric CO2 unless we are able to correctly estimate the magnitude and timelines of OC sequestration. The future of blue carbon research is not trivial. There are both spatial and temporal scales on which OC stabilization/destabilization mechanisms operate, with complex processes and controls working in combination. As we come to terms with this complexity, and embrace technological advances in genetic, molecular, spectrometric, and isotope-tracing techniques in tandem with gathering more data from a diversity of seagrass ecosystems, we can move forward with this important line of research.
Author Contributions
EB produced the conceptual framework, wrote the first draft of the manuscript, and synthesized the inputs and revisions of the coauthors. MM contributed to the conceptual knowledge and critically revised the manuscript. LG contributed to the conceptual knowledge and critically revised the manuscript. MZ contributed to the conceptual knowledge and critically revised the manuscript. MT contributed to the conceptual knowledge and critically revised the manuscript.
Funding
The work leading to this publication was supported by the German Academic Exchange Service (DAAD) with funds from the German Federal Ministry of Education and Research (BMBF) and the People Programme (Marie Curie Actions) of the European Union's Seventh Framework Programme (FP7/2007-2013) under REA grant agreement n° 605728 (PRIME—Postdoctoral Researchers International Mobility Experience), and the projects SUMILEN (CTM2013-47728-R) and PALEOPARK (1104/2014), funded by the Spanish National Parks and State Research Schemes.
Conflict of Interest Statement
The authors declare that the research was conducted in the absence of any commercial or financial relationships that could be construed as a potential conflict of interest.
Acknowledgments
We would like to acknowledge Caitlin Hicks-Pries for conceptual help on alternative ways to subsample and radiocarbon date sediment cores, and Jose Sanjuan Munoz for helping us to understand and model using input-output box models.
References
Ågren, G. I., and Bosatta, E. (1996). A bridge between theory and experiment in soil organic matter studies. Oikos 76, 522–528. doi: 10.2307/3546345
Amelung, W., Brodowski, S., Sandhage-Hofmann, A., and Bol, R. (2008). Combining biomarker with stable isotope analyses for assessing the transformation and turnover of soil organic matter. Adv. Agron. 100, 155–250. doi: 10.1016/S0065-2113(08)00606-8
Amstalden van Hove, E. R., Smith, D. F., and Heeren, R. M. A. (2010). A concise review of mass spectrometry imaging. J. Chromatogr. A 1217, 3946–3954. doi: 10.1016/j.chroma.2010.01.033
Armitage, A. R., and Fourqurean, J. W. (2016). Carbon storage in seagrass soils: long-term nutrient history exceeds the effects of near-term nutrient enrichment. Biogeosciences 13, 313–321. doi: 10.5194/bg-13-313-2016
Bosatta, E., and Agren, G. I. (1985). Theoretical analysis of decomposition of heterogeneous substrates. Soil Biol. Biochem. 17, 601–610. doi: 10.1016/0038-0717(85)90035-5
Bouillon, S., and Boschker, H. T. S. (2006). Bacterial carbon sources in coastal sediments: a cross-system analysis based on stable isotope data of biomarkers. Biogeosciences 3, 175–185. doi: 10.5194/bg-3-175-2006
Boyer-Villemaire, U., St-Onge, G., Bernatchez, P., Lajeunesse, P., and Labrie, J. (2013). High-resolution multiproxy records of sedimentological changes induced by dams in the Sept-Îles area (Gulf of St. Lawrence, Canada). Mar. Geol. 338, 17–29. doi: 10.1016/j.margeo.2012.11.012
Bracho, R., Natali, S., Pegoraro, E., Crummer, K. G., Schadel, C., Celis, G., et al. (2016). Temperature sensitivity of organic matter decomposition of permafrost-region soils during laboratory incubations. Soil Biol. Biochem. 97, 1–14. doi: 10.1016/j.soilbio.2016.02.008
Brodowski, S., Amelung, W., Haumaier, L., Abetz, C., and Zech, W. (2005). Morphological and chemical properties of black carbon in physical soil fractions as revealed by scanning electron microscopy and energy-dispersive X-ray spectroscopy. Geoderma 128, 116–129. doi: 10.1016/j.geoderma.2004.12.019
Bundy, J. G., Davey, M. P., and Viant, M. R. (2008). Environmental metabolomics: a critical review and future perspectives. Metabolomics 5, 3–21. doi: 10.1007/s11306-008-0152-0
Burdige, D. J. (2007). Preservation of organic matter in marine sediments: controls, mechanisms, and an imbalance in sediment organic carbon budgets? Chem. Rev. 107, 467–485. doi: 10.1021/cr050347q
Burdorf, L., Hidalgo-Martinez, S., Cook, P., and Meysman, F. (2016). Long-distance electron transport by cable bacteria in mangrove sediments. Mar. Ecol. Prog. Series 545, 1–8. doi: 10.3354/meps11635
Campbell, J. E., Lacey, E. A., Decker, R. A., Crooks, S., and Fourqurean, J. W. (2014). Carbon Storage in Seagrass Beds of Abu Dhabi, United Arab Emirates. Estuaries Coasts 38, 243–251. doi: 10.1007/s12237-014-9802-9
Carbone, M. S., Czimczik, C. I., McDuffee, K. E., and Trumbore, S. E. (2007). Allocation and residence time of photosynthetic products in a boreal forest using a low-level 14C pulse-chase labeling technique. Global Change Biol. 13, 466–477. doi: 10.1111/j.1365-2486.2006.01300.x
Carvalhais, L. C., Dennis, P. G., Tyson, G. W., and Schenk, P. M. (2012). Application of metatranscriptomics to soil environments. J. Microbiol. Methods 91, 246–251. doi: 10.1016/j.mimet.2012.08.011
Chabbi, A., Kogel-Knabner, I., and Rumpel, C. (2009). Stabilised carbon in subsoil horizons is located in spatially distinct parts of the soil profile. Soil Biol. Biochem. 41, 256–261. doi: 10.1016/j.soilbio.2008.10.033
Clymo, R. S. (1984). The limits to Peat Bog growth. Philosophical Trans. R. Soc. London B Biol. Sci. 303, 605–654. doi: 10.1098/rstb.1984.0002
Cornwell, W. K., and Weedon, J. T. (2014). Decomposition trajectories of diverse litter types: a model selection analysis. Methods Ecol. Evol. 5, 173–182. doi: 10.1111/2041-210X.12138
Dadi, T., Völkner, C., and Koschorreck, M. (2015). A sediment core incubation method to measure the flux of dissolved organic carbon between sediment and water. J. Soils Sediments 15, 2350–2358. doi: 10.1007/s11368-015-1213-4
Dahl, M., Deyanova, D., Gütschow, S., Asplund, M. E., Lyimo, L. D., Karamfilov, V., et al. (2016). Sediment properties as important predictors of carbon storage in Zostera marina meadows: a comparison of four European areas. PLoS ONE 11:e0167493. doi: 10.1371/journal.pone.0167493
Demas, G. P., and Rabenhorst, M. C. (2001). Factors of subaqueous soil formation: a system of quantitative pedology for submersed environments. Geoderma 102, 189–204. doi: 10.1016/S0016-7061(00)00111-7
de la Rosa Arranz, J. M., González-Vila, F. J., López-Capel, E., Manning, D. A. C., Knicker, H., and González-Pérez, J. A. (2009). Structural properties of non-combustion-derived refractory organic matter which interfere with BC quantification. J. Anal. Appl. Pyrol. 85, 399–407. doi: 10.1016/j.jaap.2008.11.019
de la Torre-Castro, M., and Rönnbäck, P. (2004). Links between humans and seagrasses—an example from tropical East Africa. Ocean Coast. Manage. 47, 361–387. doi: 10.1016/j.ocecoaman.2004.07.005
Duarte, C. M., Holmer, M., and Marba, N. (2005). Plant-Microbe Interactions in Seagrass Meadows in Macroand Microorganisms. Mar. Sediments Coast. Estuarine Stud. 60, 1–30. doi: 10.1029/ce060p0031
Duarte, C. M., Kennedy, H., Marba, N., and Hendriks, I. (2013). Assessing the capacity of seagrass meadows for carbon burial: current limitations and future strategies. Ocean Coast. Manage. 83, 32–38. doi: 10.1016/j.ocecoaman.2011.09.001
Duarte, C. M., Marba, N., Gacia, E., Fourqurean, J. W., Beggins, J., Barron, C., et al. (2010). Seagrass community metabolism: assessing the carbon sink capacity of seagrass meadows. Global Biogeochem. Cycles 24:GB4032. doi: 10.1029/2010GB003793
Duarte, C. M., Merino, M., Agawin, N. S. R., Uri, J., Fortes, M. D., Gallegos, M. E., et al. (1998). Root production and belowground seagrass biomass. Mar. Ecol. Prog. Ser. 171, 97–108. doi: 10.3354/meps171097
Duarte, C. M., Middelburg, J. J., and Caraco, N. (2004). Major role of marine vegetation on the oceanic carbon cycle. Biogeosci. Discuss. 1, 659–679. doi: 10.5194/bgd-1-659-2004
Duffy, J. E. (2006). Biodiversity and the functioning of seagrass ecosystems. Mar. Ecol. Prog. Ser. 311, 233–250. doi: 10.3354/meps311233
Eriksson, E. (1971). Compartment models and reservoir theory. Ann. Rev. Ecol. Syst. 2, 67–84. doi: 10.1146/annurev.es.02.110171.000435
Feeney, D. S., Crawford, J. W., Daniell, T., Hallett, P. D., Nunan, N., Ritz, K., et al. (2006). Three-dimensional microorganization of the soil–root–microbe system. Microb Ecol. 52, 151–158. doi: 10.1007/s00248-006-9062-8
Fourqurean, J. W., Duarte, C. M., Kennedy, H., Marba, N., Holmer, M., Mateo, M. A., et al. (2012). Seagrass ecosystems as a globally significant carbon stock. Nat. Geosci. 5, 505–509. doi: 10.1038/ngeo1477
Fuentes, M., Hidalgo, C., González-Martín, I., Hernández-Hierro, J. M., Govaerts, B., Sayre, K. D., et al. (2012). NIR Spectroscopy: an alternative for soil analysis. Commun. Soil Sci. Plant Anal. 43, 346–356. doi: 10.1080/00103624.2012.641471
Grandy, A. S., and Neff, J. C. (2008). Molecular C dynamics downstream: The biochemical decomposition sequence and its impact on soil organic matter structure and function. Sci. Total Environ. 404, 297–307. doi: 10.1016/j.scitotenv.2007.11.013
Hedges, J. I., Eglinton, G., Hatcher, P. G., Kirchman, D. L., Arnosti, C., Derenne, S., et al. (2000). The molecularly-uncharacterized component of nonliving organic matter in natural environments. Org. Geochem. 31, 945–958. doi: 10.1016/s0146-6380(00)00096-6
Herr, D., Pidgeon, E., and Laffoley, D. eds. (2012). Blue Carbon Policy Framework: Based on the Discussion of the International Blue Carbon Policy Working Group Gland. Switzerland: IUCN and Arlington.
Herrmann, A. M., Ritz, K., Nunan, N., Clode, P. L., Pett-Ridge, J., Kilburn, M. R., et al. (2007). Nano-scale secondary ion mass spectrometry — A new analytical tool in biogeochemistry and soil ecology: a review article. Soil Biol. Biochem. 39, 1835–1850. doi: 10.1016/j.soilbio.2007.03.011
Hicks Pries, C. E., Schuur, E., and Crummer, K. (2012). Holocene carbon stocks and carbon accumulation rates altered in soils undergoing permafrost thaw. Ecosystems 15, 162–173. doi: 10.1007/s10021-011-9500-4
Hua, Q., Barbett, M., and Rakowski, A. Z. (2013). Atmospheric radiocarbon for the period 1950-2010. Radiocarbon 55, 2059–2072. doi: 10.2458/azu_js_rc.v55i2.16177
Ingalls, A. E., Ellis, E. E., Santos, G. M., McDuffee, K. E., Truxal, L., Keil, R. G., et al. (2010). HPLC purification of higher plant-dervied lignin phenols for compound specific radiocarbon analysis. Anal. Chem. 82, 8931–8938. doi: 10.1021/ac1016584
IPCC (2013). “Summary for Policymakers,” in Climate Change The Physical Science Basis. Contribution of Working Group I to the Fifth Assessment Report of the Intergovernmental Panel on Climate Change, eds. T. F. Stocker, D. Qin, G. K. Plattner, M. Tignor, S. K. Allen, J. Boschung (Cambridge; New York, NY: Cambridge University Press), 1–28.
Jankowska, E., Michel, L. N., Zaborska, A., and Włodarska Kowalczuk, M. (2016). Sediment carbon sink in low-density temperate eelgrass meadows (Baltic Sea). J. Geophys. Res. Biogeo 121, 2918–2934. doi: 10.1002/2016jg003424
Jones, D. S., Flood, B. E., and Bailey, J. V. (2015). Metatranscriptomic insights into polyphosphate metabolism in marine sediments. ISME J.10, 1015–1019. doi: 10.1038/ismej.2015.169
Kaal, J., Serrano, O., Nierop, K. G. J., Schellekens, J., Martínez Cortizas, A., and Mateo, M. A. (2016). Molecular composition of plant parts and sediment organic matter in a Mediterranean seagrass (Posidonia oceanica) mat. Aquatic Bot. 133, 50–61. doi: 10.1016/j.aquabot.2016.05.009
Kalish, J. M., Nydal, R., Nedreaas, K. H., Burr, G. S., and Eine, G. L. (2001). A time history of pre- and post-bomb radiocarbon in the barents sea derived from arcto-norwegian cod otoliths. Radiocarbon 43, 843–855. doi: 10.1017/S0033822200041515
Kennedy, H., Beggins, J., Duarte, C. M., Fourqurean, J. W., Holmer, M., Marba, N., et al. (2010). Seagrass sediments as a global carbon sink: Isotopic constraints. Global Biogeochem. Cycles 24:GB4026. doi: 10.1029/2010GB003848
Kilada, R. W., Campana, S. E., and Roddick, D. (2007). Validated age, growth, and mortality estimates of the ocean quahog (Arctica islandica) in the western Atlantic. ICES J. Mar. Sci. 64, 31–38. doi: 10.1093/icesjms/fsl001
King, A. A., Domenech de Celles, M., Magpantay, F., and Rohani, P. (2015). Avoidable errors in the modeling of outbreaks of emerging pathogens, with special reference to Ebola. Proc. R. Soc. B 282, 20150347. doi: 10.1098/rspb.2015.0347
Kivlin, S. N., and Hawkes, C. V. (2016). Temporal and spatial variation of soil bacteria richness, composition, and function in a neotropical rainforest. PLoS ONE 11:e0159131. doi: 10.1371/journal.pone.0159131
Kogel-Knabner, I. (2002). The macromolecular organic composition of plant and microbial residues as inputs to soil organic matter. Soil Biol. Biochem. 34, 139–162. doi: 10.1002/chin.200223287
Kogel-Knabner, I. (2003). 13C and 15N NMR spectroscopy as a tool in soil organic matter studies. Gerderma 80, 243–270. doi: 10.1016/S0016-7061(97)00055-4
Korhola, A., Tolonen, K., Turunen, J., and Jungner, H. (1995). Estimating long-term carbon accumulation rates in boreal peatlands by radiocarbon dating. Radiocarbon 37, 575–584. doi: 10.1017/S0033822200031064
Kramer, C., Trumbore, S., FrOberg, M., Dozal, L. M. C., Zhang, D., Xu, X., et al. (2010). Recent (<4 year old) leaf litter is not a major source of microbial carbon in a temperate forest mineral soil. Soil Biol. Biochem. 42, 1028–1037. doi: 10.1016/j.soilbio.2010.02.021
Lavery, P. S., Mateo, M. A., Serrano, O., and Rozaimi, M. (2013). Variability in the carbon storage of seagrass habitats and its implications for global estimates of blue carbon ecosystem service. PLoS ONE 8:e73748. doi: 10.1371/journal.pone.0073748
Lehmann, J., Liang, B., Solomon, D., Lerotic, M., Luizão, F., Kinyangi, J., et al. (2005). Near-edge X-ray absorption fine structure (NEXAFS) spectroscopy for mapping nano-scale distribution of organic carbon forms in soil: Application to black carbon particles. Global Biogeochem. Cycles 19:GB1013. doi: 10.1029/2004GB002435
Macreadie, P. I., Baird, M. E., Trevathan-Tackett, S. M., Larkum, A. W. D., and Ralph, P. J. (2014). Quantifying and modelling the carbon sequestration capacity of seagrass meadows – A critical assessment. Mar. Pollut. Bull. 83, 30–439. doi: 10.1016/j.marpolbul.2013.07.038
Macreadie, P. I., Trevathan-Tackett, S. M., Skilbeck, C. G., Sanderman, J., Curlevski, N., Jacobsen, G., et al. (2015). Losses and recovery of organic carbon from a seagrass ecosystem following disturbance. Proc. R. Soc. B 282:20151537. doi: 10.1098/rspb.2015.1537
Mahadevan, A. (2001). An analysis of bomb radiocarbon trends in the Pacific. Mar. Chem. 73, 273–290. doi: 10.1016/S0304-4203(00)00113-4
Mangerud, J. (1972). Radiocarbon dating of marine shells, including a discussion of apparent age of recent shells from Norway. Boreas 1, 143–172. doi: 10.1111/j.1502-3885.1972.tb00147.x
Manzoni, S., Katul, G. G., and Porporato, A. (2009). Analysis of soil carbon transit times and age distributions using network theories. J. Geophys. Res 114:G04025. doi: 10.1029/2009jg001070
Manzoni, S., Piñeiro, G., Jackson, R. B., Jobbágy, E. G., Kim, J. H., and Porporato, A. (2012). Analytical models of soil and litter decomposition: solutions for mass loss and time-dependent decay rates. Soil Biol. Biochem. 50, 66–76.
Marbá, N., Holmer, M., Gacia, E., and Barron, C. (2006). “Seagrass beds and coastal biogeochemistry,” in Seagrasses: Biology, Ecology and Conservation, eds A. W. D. Larkum, R. J. Orth, and C. M. Duarte (Dordrecht: Springer), 135–157.
Maron, P.-A., Mougel, C., and Ranjard, L. (2011). Soil microbial diversity: Methodological strategy, spatial overview and functional interest. C. R. Biol. 334, 403–411. doi: 10.1016/j.crvi.2010.12.003
Marschner, B., Brodowski, S., Dreves, A., Gleixner, G., Gude, A., Grootes, P. M., et al. (2008). How relevant is recalcitrance for the stabilization of organic matter in soils? J. Plant Nutr. Soil Sci. 171, 91–110. doi: 10.1002/jpln.200700049
Marshall, W. A., Gehrels, W. R., Garnett, M. H., Freeman, S. P. H. T., Maden, C., and Xu, S. (2007). The use of “bomb spike” calibration and high-precision AMS 14C analyses to date salt-marsh sediments deposited during the past three centuries. Quaternary Res. 68, 325–337. doi: 10.1016/j.yqres.2007.07.005
Mateo, M. A., Cebrian, J., Dunton, K. H., and Mutchler, T. (2006). “Carbon Flux in Seagrass Ecosystems,” in Seagrasses: Biology, Ecology and Conservation, eds A. W. D. Larkum, R. J. Orth, and C. M. Duarte (Dordrecht: Springer), 159–192.
Mateo, M. Á., Renom, P., and Michener, R. H. (2010). Long-term stability in the production of a NW Mediterranean Posidonia oceanica (L.) Delile meadow. Palaeogeogr. Palaeoclimatol. Palaeoecol. 291, 286–296. doi: 10.1016/j.palaeo.2010.03.001
Mateo, M. A., Romero, J., Pereza, M., Littler, M. M., and Littler, D. S. (1997). Dynamics of millenary organic deposits resulting from the growth of the mediterranean seagrass Posidonia oceanica. Estuarine Coast. Shelf Sci. 44, 103–110. doi: 10.1006/ecss.1996.0116
Maurice, P. A., and Hochella, M. F. (2008). Nanoscale Particles and Processes: A New Dimension in Soil Science, 1st Edn. Cambridge, MA: Elsevier Inc.
McIntosh, H. A., McNichol, A. P., Xu, L., and Canuel, E. A. (2015). Source-age dynamics of estuarine particulate organic matter using fatty acid δ13C and Δ 14C composition. Limnol. Oceanogr. 60, 611–628. doi: 10.1002/lno.10053
McLeod, E., Chmura, G. L., Bouillon, S., Salm, R., Björk, M., Duarte, C. M., et al. (2011). A blueprint for blue carbon: toward an improved understanding of the role of vegetated coastal habitats in sequestering CO2. Front. Ecol. Environ. 9, 552–560. doi: 10.1890/110004
Mikutta, R., Kleber, M., Torn, M. S., and Jahn, R. (2006). Stabilization of soil organic matter: association with minerals or chemical recalcitrance? Biogeochemistry 77, 25–56. doi: 10.1007/s10533-005-0712-6
Miyajima, T., Hori, M., Hamaguchi, M., Shimabukuro, H., Adachi, H., Yamano, H., et al. (2015). Geographic variability in organic carbon stock and accumulation rate in sediments of East and Southeast Asian seagrass meadows. Global Biogeochem. Cycles 29, 1–19. doi: 10.1002/2014GB004979
Moriarty, D. J. W., Iverson, R. L., and Pollard, P. C. (1986). Exudation of organic carbon by the seagrass Halodule wrightii Aschers. And its effect on bacterial growth in the sediment. J. Exp. Mar. Biol. Ecol. 96, 115–126. doi: 10.1016/0022-0981(86)90237-6
Moros, J., Barciela-Alonso, M. C., Pazos-Capeáns, P., Bermejo-Barrera, P., Peña-Vázquez, E., Garrigues, S., et al. (2008). Characterization of estuarine sediments by near infrared diffuse reflectance spectroscopy. Anal. Chim. Acta 624, 113–127. doi: 10.1016/j.aca.2008.06.030
Moros, J., Cassella, R. J., Barciela-Alonso, M. C., Moreda-Piñeiroc, A., Herbello-Hermelo, P., Bermejo-Barrera, P., et al. (2010). Estuarine sediment quality assessment by Fourier-transform infrared spectroscopy. Vibrational Spectrosc. 53, 204–213. doi: 10.1016/j.vibspec.2010.03.001
Nellemann, C., Corcoran, E., Duarte, C. M., Valdes, L., De Young, C., Fonseca, L., et al. (eds). (2009). Blue Carbon. Norway Available online at: www.grida.no
Nesme, J., Achouak, W., Agathos, S. N., Bailey, M., Baldrian, P., Brunel, D., et al. (2016). Back to the future of soil metagenomics. Front. Microbiol. 7:73. doi: 10.3389/fmicb.2016.00073
Neufeld, J. D., Wagner, M., and Murrell, J. C. (2007). Who eats what, where and when? Isotope-labelling experiments are coming of age. ISME J. 1, 103–110. doi: 10.1038/ismej.2007.30
Nunan, N., Ritz, K., Rivers, M., Feeney, D. S., and Young, I. M. (2006). Investigating microbial micro-habitat structure using X-ray computed tomography. Geoderma 133, 398–407. doi: 10.1016/j.geoderma.2005.08.004
Orth, R. J., Carruthers, T. J. B., Dennison, W. C., Duarte, C. M., Fourqurean, J. W., Heck, K. L., et al. (2006). A global crisis for seagrass ecosystems. Bioscience 56, 987–996. doi: 10.1641/0006-3568(2006)56[987:AGCFSE]2.0.CO;2
Pajares, S., Escalante, A. E., Noguez, A. M., García-Oliva, F., Martínez-Piedragil, C., Cram, S. S., et al. (2016). Spatial heterogeneity of physicochemical properties explains differences in microbial composition in arid soils from Cuatro Cienegas, Mexico. PeerJ. 4, e2459–e2420. doi: 10.7717/peerj.2459
Paula, F. S., Rodrigues, J. L. M., Zhou, J., Wu, L., Mueller, R. C., Mirza, B. S., et al. (2014). Land use change alters functional gene diversity, composition and abundance in Amazon forest soil microbial communities. Mol. Ecol. 23, 2988–2999. doi: 10.1111/mec.12786
Phang, V., Chou, L. M., and Friess, D. A. (2015). Ecosystem carbon stocks across a tropical intertidal habitat mosaic of mangrove forest, seagrass meadow, mudflat and sandbar. Earth Surf. Process. Landforms 40, 1387–1400. doi: 10.1002/esp.3745
Remusat, L., Hatton, P.-J., Nico, P. S., Zeller, B., Kleber, M., and Derrien, D. (2012). NanoSIMS study of organic matter associated with soil aggregates: advantages, limitations, and combination with STXM. Environ. Sci. Technol. 46, 3943–3949. doi: 10.1021/es203745k
Rethemeyer, J., Kramer, C., Gleixner, G., Wiesenberg, G. L. B., Schwark, L., Andersen, N., et al. (2004). Complexity of soil organic matter: AMS 14C analysis of soil lipid fractions and individual compounds. Radiocarbon 46, 465–473. doi: 10.1017/S0033822200039771
Röhr, M. E., Boström, C., Canal-Vergés, P., and Holmer, M. (2016). Blue carbon stocks in Baltic Sea eelgrass (Zostera marina) meadows. Biogeosciences 13, 6139–6153. doi: 10.5194/bg-13-6139-2016
Rozaimi, M., Lavery, P. S., and Serrano, O. (2016). Long-term carbon storage and its recent loss in an estuarine Posidonia australis meadow (Albany, Western Australia). Estuar. Coast. Shelf Sci. 171, 58–65. doi: 10.1016/j.ecss.2016.01.001
Ruamps, L. S., Nunan, N., and Chenu, C. (2011). Microbial biogeography at the soil pore scale. Soil Biol. Biochem. 43, 280–286. doi: 10.1016/j.soilbio.2010.10.010
Samper-Villarreal, J., Lovelock, C. E., Saunders, M. I., Roelfsema, C., and Mumby, P. J. (2016). Organic carbon in seagrass sediments is influenced by seagrass canopy complexity, turbidity, wave height, and water depth. Limnol. Oceanogr. 61, 938–952. doi: 10.1002/lno.10262
Schile, L. M., Kauffman, J. B., Crooks, S., Fourqurean, J. W., Glavan, J., and Megonigal, J. P. (2016). Limits on carbon sequestration in arid blue carbon ecosystems. Ecol. Appl. 27, 859–874. doi: 10.1002/eap.1489
Schmidt, M. W. I., Torn, M. S., Abiven, S., Dittmar, T., Guggenberger, G., Janssens, I. A., et al. (2011). Persistence of soil organic matter as an ecosystem property. Nature 478, 49–56. doi: 10.1038/nature10386
Schuur, E. A. G., Carbone, M. S., Hicks Pries, C. E., Hopkins, F. M., and Natali, S. M. (2016). “Radiocarbon in terrestrial systems,” in Radiocarbon and Climate Change, eds E. A. G. Schuur, E. R. M. Druffel, and S. E. Trumbore (Springer International Publishing), 167–220.
Scourse, J. D., Wanamaker, A. D., Weidman, C., Heinemeier, J., Reimer, P. J., Butler, P. G., et al. (2012). The marine radiocarbon bomb pulse across the temperate North Atlantic: a compliation of Δ14C time histories from Arctica Islandica growth increments. Radiocarbon 54, 165–186. doi: 10.2458/azu_js_rc.v54i2.16026
Serrano, O., Lavery, P. S., Duarte, C. M., Kendrick, G. A., Calafat, A., York, P. H., et al. (2016a). Can mud (silt and clay) concentration be used to predict soil organic carbon content within seagrass ecosystems? Biogeosciences 13, 4915–4926. doi: 10.5194/bg-13-4915-2016
Serrano, O., Lavery, P. S., López-Merino, L., Ballesteros, E., and Mateo, M. Á. (2016b). Location and associated carbon storage of erosional escarpments of seagrass posidonia mats. Front. Mar. Sci. 3:42. doi: 10.3389/fmars.2016.00042
Serrano, O., Lavery, P. S., Rozaimi, M., and Mateo, M. A. (2014). Influence of water depth on the carbon sequestration capacity of seagrasses. Global Biogeochem. Cycles 28, 950–961. doi: 10.1002/2014GB004872
Serrano, O., Mateo, M. A., Renom, P., and Julià, R. (2012). Characterization of soils beneath a Posidonia oceanica meadow. Geoderma 185–186, 26–36. doi: 10.1016/j.geoderma.2012.03.020
Serrano, O., Ricart, A. M., Lavery, P. S., Mateo, M. A., Arias-Ortiz, A., Masqué, P., et al. (2015). Key biogeochemical factors affecting soil carbon storage in Posidoniameadows. Biogeosci. Discussions 12, 18913–18944. doi: 10.5194/bgd-12-18913-2015
Serrano, O., Ruhon, R., Lavery, P. S., Kendrick, G. A., Hickey, S., Masqué, P., et al. (2016c). Impact of mooring activities on carbon stocks in seagrass meadows. Sci. Rep. 6:23193. doi: 10.1038/srep23193
Short, F., Carruthers, T., Dennison, W., and Waycott, M. (2007). Global seagrass distribution and diversity: a bioregional model. J. Exp. Mar. Biol. Ecol. 350, 3–20. doi: 10.1016/j.jembe.2007.06.012
Simpson, M. J., and Simpson, A. J. (2012). The chemical ecology of soil organic matter molecular constituents. J. Chem. Ecol. 38, 768–784. doi: 10.1007/s10886-012-0122-x
Six, J., Bossuyt, H., Degryze, S., and Denef, K. (2004). A history of research on the link between (micro) aggregates, soil biota, and soil organic matter dynamics. Soil Tillage Res. 79, 7–31. doi: 10.1016/j.still.2004.03.008
Sleutel, S., Cnudde, V., Masschaele, B., Vlassenbroek, J., Dierick, M., Van Hoorebeke, L., et al. (2008). Comparison of different nano- and micro-focus X-ray computed tomography set-ups for the visualization of the soil microstructure and soil organic matter. Comput. Geosci. 34, 931–938. doi: 10.1016/j.cageo.2007.10.006
Sollins, P., Homann, P., and Caldwell, B. A. (1996). Stabilization and destabilization of soil organic matter: mechanisms and controls. Geoderma 74, 1–41. doi: 10.1016/S0016-7061(96)00036-5
Solomon, D., Lehmann, J., Kinyangi, J., Liang, B., and Schafer, T. (2004). Carbon K-Edge NEXAFS and FTIR-ATR spectroscopic investigation of organic carbon speciation in soils. Soil Sci. Soc. Am. J. 69, 107–119. doi: 10.2136/sssaj2005.0107dup
Stuiver, M., Pearson, G. W., and Braziunas, T. F. (1986). Radiocarbon age calibration of marine samples back to 9000 cal yr BP. Radiocarbon 28, 980–1021. doi: 10.1017/S0033822200060264
Thureborn, P., Hu, Y. O. O., Franzetti, A., Sjöling, S., and Lundin, D. (2017). Accumulation of DNA in an anoxic sediment – rDNA and rRNA presence of members of the microbial community from Landsort Deep, the Baltic Sea. PeerJ 5:e2051v2. doi: 10.7287/peerj.preprints.2051v2
Tisnérat-Laborde, N., Montagna, P., McCulloch, M., Siani, G., Silenzi, S., and Frank, N. (2013). A high-resolution coral-based Δ14C record of surface water processes in the Western Mediterranean Sea. Radiocarbon 55, 1617–1630. doi: 10.1017/S0033822200048530
Tolu, J., Gerber, L., Boily, J.-F., and Bindler, R. (2015). High-throughput characterization of sediment organic matter by pyrolysis–gas chromatography/mass spectrometry and multivariate curve resolution: a promising analytical tool in (paleo)limnology. Anal. Chim. Acta 880, 93–102. doi: 10.1016/j.aca.2015.03.043
Torn, M. S., Swanston, C. W., Castanha, C., and Trumbore, S. E. (2009). “Storage and turnover of organic matter in soil,” in Biophysico-Chemical Processes Involving Natural Nonliving Organic Matter in Environmental Systems, eds N. Senesi, B. Xing, and P. M. Huang (Hoboken, NJ: John Wiley & Sons, Inc.), 219–272. doi: 10.1002/9780470494950.ch6
Trumbore, S. E. (1993). Comparison of carbon dynamics in tropical and temperate soils using radiocarbon measurements. Global Biogeochem. Cycles 7, 275–290. doi: 10.1029/93GB00468
Trumbore, S. E. (2000). Age of soil organic matter and soil respiration: radiocarbon constraints on belowground C dynamics. Ecol. Appl. 10, 399–411. doi: 10.1890/1051-0761(2000)010[0399:AOSOMA]2.0.CO;2
Trumbore, S. E. (2009). Radiocarbon and soil carbon dynamics. Annu. Rev. Earth Planet Sci. 37, 47–66. doi: 10.1146/annurev.earth.36.031207.124300
Trumbore, S. E., and Harden, J. W. (1997). Accumulation and turnover of carbon in organic and mineral soils of the BOREAS northern study area. J. Geophys. Res. 102, 28817–28830. doi: 10.1029/97JD02231
Trumbore, S. E., Sierra, C. A., and Hicks Pries, C. E. (2016). “Radiocarbon nomenclature, theory, models, and interpretation: measuring age, determining cycling rates, and tracing source pools,” in Radiocarbon and Climate Change, eds E. A. G. Schuur, E. R. M. Druffel, and S. E. Trumbore (Springer International Publishing), 45–82.
Trumbore, S. E., and Zheng, S. (1996). Comparison of fractionation methods for soil organic matter 14C analysis. Radiocarbon 38, 219–229. doi: 10.1017/S0033822200017598
von Lützow, M., Kögel-Knabner, I., Ekschmitt, K., Flessa, H., Guggenberger, G., Matzner, E., et al. (2007). SOM fractionation methods: relevance to functional pools and to stabilization mechanisms. Soil Biol. Biochem. 39, 2183–2207. doi: 10.1016/j.soilbio.2007.03.007
von Mering, C., Hugenholtz, P., Raes, J., Tringe, S. G., Doerks, T., Jensen, L. J., et al. (2007). Quantitative phylogenetic assessment of microbial communities in diverse environments. Science 315, 1126–1130. doi: 10.1126/science.1133420
van Oort, F., Jongmans, A. G., Citeau, L., Lamy, I., and Chevallier, P. (2006). Microscale Zn and Pb distribution patterns in subsurface soil horizons: an indication for metal transport dynamics. Eur. J. Soil Sci. 57, 154–166. doi: 10.1111/j.1365-2389.2005.00725.x
Verchot, L. V., Dutaur, L., Shepherd, K. D., and Albrecht, A. (2011). Organic matter stabilization in soil aggregates: understanding the biogeochemical mechanisms that determine the fate of carbon inputs in soils. Geoderma 161, 182–193. doi: 10.1016/j.geoderma.2010.12.017
Vichkovitten, T., and Holmer, M. (2005). Dissolved and particulate organic matter in contrasting Zostera marina (eelgrass) sediments. J. Exp. Mar. Biol. Ecol. 6, e18311. doi: 10.1016/j.jembe.2004.11.002
Vinduskova, O., Sebag, D., Cailleau, G., Brus, J., and Frouz, J. (2015). Methodological comparison for quantitative analysis of fossil and recently derived carbon in mine soils with high content of aliphatic kerogen. Org. Geochem. 89–90, 14–22. doi: 10.1016/j.orggeochem.2015.10.001
Wallenstein, M. D., and Weintraub, M. N. (2008). Emerging tools for measuring and modeling the in situ activity of soil extracellular enzymes. Soil Biol. Biochem. 40, 2098–2106. doi: 10.1016/j.soilbio.2008.01.024
Waycott, M., Duarte, C. M., Carruthers, T. J. B., Orth, R. J., Dennison, W. C., Olyarnik, S., et al. (2009). Accelerating loss of seagrasses across the globe threatens coastal ecosystems. Proc. Natl. Acad. Sci.U.S.A. 106, 12377–12381. doi: 10.1073/pnas.0905620106
Webster, G., Watt, L. C., Rinna, J., Fry, J. C., Evershed, R. P., Parkes, R. J., et al. (2006). A comparison of stable-isotope probing of DNA and phospholipid fatty acids to study prokaryotic functional diversity in sulfate-reducing marine sediment enrichment slurries. Environ. Microbiol. 8, 1575–1589. doi: 10.1111/j.1462-2920.2006.01048.x
Weidman, C. R., and Jones, G. A. (1993). A shell-derived time history of bomb 14C on Georges Bank and its Labrador Sea implications. J. Geophys. Res. Oceans 98, 14577–14588. doi: 10.1029/93JC00785
Werner, U., Bird, P., Wild, C., Ferdelman, T., Polerechy, L., Eickert, G., et al. (2006). Spatial patterns of aerobic and anaerobic mineralization rates and oxygen penetration dynamics in coral reef sediments. Mar. Ecol. Prog. Ser. 309, 93–105. doi: 10.3354/meps309093
West, M., Ellis, A. T., Kregsamer, P., Potts, P. J., Streli, C., Vanhoof, C., et al. (2007). Atomic spectrometry update. X-ray fluorescence spectrometry. J. Anal. Atmos. Spectrom. 22, 1304–1329. doi: 10.1039/b712079f
Keywords: organic carbon, seagrass, residence times, state-factors, stabilization mechanisms
Citation: Belshe EF, Mateo MA, Gillis L, Zimmer M and Teichberg M (2017) Muddy Waters: Unintentional Consequences of Blue Carbon Research Obscure Our Understanding of Organic Carbon Dynamics in Seagrass Ecosystems. Front. Mar. Sci. 4:125. doi: 10.3389/fmars.2017.00125
Received: 24 January 2017; Accepted: 18 April 2017;
Published: 04 May 2017.
Edited by:
Christian Grenz, Mediterranean Institute of Oceanography, FranceReviewed by:
Jan Marcin Weslawski, Institute of Oceanology (PAN), PolandGerard Pergent, University of Corsica Pascal Paoli, France
Copyright © 2017 Belshe, Mateo, Gillis, Zimmer and Teichberg. This is an open-access article distributed under the terms of the Creative Commons Attribution License (CC BY). The use, distribution or reproduction in other forums is permitted, provided the original author(s) or licensor are credited and that the original publication in this journal is cited, in accordance with accepted academic practice. No use, distribution or reproduction is permitted which does not comply with these terms.
*Correspondence: E. Fay Belshe, ZmJlbHNoZUBnbWFpbC5jb20=