Iron Availability Influences Silicon Isotope Fractionation in Two Southern Ocean Diatoms (Proboscia inermis and Eucampia antarctica) and a Coastal Diatom (Thalassiosira pseudonana)
- 1Research School of Earth Sciences, Australian National University, Canberra, ACT, Australia
- 2Ecochemistry Laboratory, Institute for Applied Ecology, University of Canberra, Canberra, ACT, Australia
The fractionation of silicon (Si) isotopes was measured in two Southern Ocean diatoms (Proboscia inermis and Eucampia Antarctica) and a coastal diatom (Thalassiosira pseudonana) that were grown under varying iron (Fe) concentrations. Varying Fe concentrations had no effect on the Si isotope enrichment factor (ε) in T. pseudonana, whilst E. Antarctica and P. inermis exhibited significant variations in the value of ε between Fe-replete and Fe-limited conditions. Mean ε values in P. inermis and E. Antarctica decreased from (± 1SD) −1.11 ± 0.15‰ and −1.42 ± 0.41 ‰ (respectively) under Fe-replete conditions, to −1.38 ± 0.27 ‰ and −1.57 ± 0.5 ‰ (respectively) under Fe-limiting conditions. These variations likely arise from adaptations in diatoms arising from the nutrient status of their environment. T. pseudonana is a coastal clone typically accustomed to low Si but high Fe conditions whereas E. Antarctica and P. inermis are typically accustomed to High Si, High nitrate low Fe conditions. Growth induced variations in silicic acid (Si(OH)4) uptake arising from Fe-limitation is the likely mechanism leading to Si-isotope variability in E. Antarctica and P. inermis. The multiplicative effects of species diversity and resource limitation (e.g., Fe) on Si-isotope fractionation in diatoms can potentially alter the Si-isotope composition of diatom opal in diatamaceous sediments and sea surface Si(OH)4. This work highlights the need for further in vitro studies into intracellular mechanisms involved in Si(OH)4 uptake, and the associated pathways for Si-isotope fractionation in diatoms.
Introduction
Diatoms play a vital role in the biogeochemical cycles of carbon (C) and silicon (Si). They dominate the production of biogenic silica (BSi) in the ocean, and hence have a controlling influence on the marine Si cycle through the utilization of dissolved silicon [silicic acid, Si(OH)4], which they use in the formation of their cell wall, or frustule (Nelson et al., 1995; Trèguer et al., 1995; Tréguer and De La Rocha, 2013). Diatoms make up a significant proportion of phytoplankton communities in nutrient rich regions of the ocean such as the Southern Ocean, where they contribute significantly to the deep export of C and Si to ocean sediments (Smetacek, 1999; Kemp et al., 2000; Roca-Martí et al., 2017). BSi preservation rates can reach as high as 30% in some of these areas due to increased sedimentation rates of diatom produced opaline silica (McManus et al., 1995; Nelson et al., 1995; Ragueneau et al., 2000). While the accumulation of sedimentary BSi (or opal) presents a promising tool for reconstructing the degree of coupling between the Si and C cycles, indices based on the accumulation of sedimentary opal are often subject to winnowing and focusing of sediments, and the dissolution of opal at the sediment-water interface (De La Rocha et al., 1998; Ragueneau et al., 2000). Variations in the ratios of 30Si to 28Si (expressed as δ30Si in ‰) in opaline sediments and in the Si(OH)4 pool present in surface waters represents a promising tool in providing information on the marine Si cycle, and as such, has been used as a proxy for marine diatom production and Si(OH)4 utilization in several palaeo-oceanographic studies (De La Rocha et al., 1998; Beucher et al., 2007; Pichevin et al., 2009; Ellwood et al., 2010; Rousseau et al., 2016); and in field studies (Varela et al., 2004; Cardinal et al., 2007; Beucher et al., 2011).
During Si uptake, diatoms discriminate against heavier isotopes, which changes the isotopic composition of both the diatom opal and the residual Si(OH)4 pool (De La Rocha et al., 1997; Sutton et al., 2013). This results in a lighter isotope composition (28Si or 29Si vs. 30Si) in the frustule, relative to its dissolved Si source. Although the exact mechanism of fractionation of silicon isotopes in diatoms is unknown, fractionation has been determined to resemble Rayleigh style fractionation kinetics under closed-system conditions, where the substrate [dissolved Si in the form of Si(OH)4] is precipitated into the product, BSi (Criss and Criss, 1999). The isotope fractionation factor (α) for Rayleigh fractionation determines the rate of fractionation between the Si(OH)4 and the Si-BSi product, and is calculated by the following equation (De La Rocha et al., 1997):
where αBSi—DSi is the isotope fractionation factor between the Si(OH)4 and the BSi product, f is the fractional depletion of Si(OH)4 in the media and δ30Siacc and δ30Sio are the δ30Si values of the accumulated BSi and of Si(OH)4 in the media at f = 1.0 (respectively). Isotope fractionation is often described in terms of a per mil (‰) value such as the enrichment factor (ε). The relationship between α and ε can be described using the following equation:
Estimates for Si isotope fractionation in diatoms under controlled laboratory conditions were initially found to be (expressed as ε in ‰), −1.1 ± 0.4 ‰ (average ± 1SD), with little variation between species (Thalassiosira sp., Thalassiosira weissflogii and Skeletonema costatum) (De La Rocha et al., 1997). Fractionation was also found to be independent of changes in temperature (12–22°C) (De La Rocha et al., 1997); Si quota, Si efflux and pCO2 concentration in T. weisflogii (Milligan et al., 2004). While these studies focused mainly on temperate and sub-polar species of diatoms, they did not adequately represent the isotope composition of individual Polar and Southern Ocean diatoms, and further studies found an inter-species effect between the Southern Ocean diatoms Fragilariopsis kergulensis (−0.54 ± 0.09 ‰) and Chaetoceros brevis (−2.09 ± 0.09 ‰) (Sutton et al., 2013). While the reasons for the difference in isotopic composition of these species is unclear, Sutton et al. (2013) suggested that potential phylogenetic and morphological effects could play an important role in Si isotope fractionation.
Diatoms in the Southern Ocean experience rapid fluctuations in both physical and chemical conditions associated with the variations in sea ice extent, deep winter mixing and alterations in nutrient supply (Sackett et al., 2013). In the short term, these extremes in physico-chemical conditions can potentially influence Si isotope fractionation in diatoms through variations in cell morphology, growth rate and nutrient uptake rate (Hutchins and Bruland, 1998; Takeda, 1998; Brzezinski et al., 2002; Leynaert et al., 2004; Meyerink et al., 2017). Field studies show that Si isotopic fractionation by siliceous phytoplankton varies across the Antarctic Polar Frontal Zone (APFZ), and coincides with the presence of a strong northward gradient in Si(OH)4 concentrations (Cardinal et al., 2007; Fripiat et al., 2011). Despite the large zonal variations in Si isotopic fractionation, there was no change among different size fractions, suggesting that the latitudinal variations in Si isotope fractionation in siliceous phytoplankton could be a direct result of nutrient availability, more than species composition (Cardinal et al., 2007). In order to decipher the effects both factors have on the surface Si isotope composition in the Southern Ocean, a better understanding of the effects nutrient availability has on Si isotope fractionation in diatoms is required.
Iron (Fe) availability affects the growth rate, Si uptake, cell morphology and BSi content in diatoms (Leynaert et al., 2004; Hoffmann et al., 2008; Marchetti and Cassar, 2009; Boutorh et al., 2016; Meyerink et al., 2017). These microscale effects can have far reaching consequences on the physico-chemical environment, particularly in the Southern Ocean. Fe-limited diatoms in Antarctic water take up more Si(OH)4 relative to nitrate (), so that by the time surface waters reach the sub-Antarctic zone (SAZ), it is depleted in Si(OH)4 relative to (Varela et al., 2004; Matsumoto et al., 2014). Alleviation of Fe-limitation in Antarctic waters may have potentially altered the Si(OH)4: uptake ratio in diatoms during glacial times, and resulted in a “leakage” of silicic acid to sub-polar waters (Brzezinski et al., 2002; Matsumoto et al., 2002). Opaline sediments exhibit variations in their Si isotope composition from glacial times, and can potentially be a useful tool in resolving the mechanisms behind the glacial-interglacial transitions of the past (De La Rocha et al., 1998; Beucher et al., 2007; Pichevin et al., 2009; Ellwood et al., 2010; Rousseau et al., 2016). In order to quantitatively use δ30Si to determine diatom production during these periods, a better understanding of how Fe-availability affects diatom δ30Si composition is required; especially since it is thought that the supply of Fe to Southern Ocean waters was higher during glacial periods (Martinez-Garcia et al., 2011). Here, the results of the fractionation of Si-isotopes in these diatoms under varying degrees of Fe-limitation are presented.
Methods
Culture Conditions
The centric diatom T. pseudonana (Strain CS-20) was obtained from the Australian national algae culture collection in Hobart, Australia and maintained in f/2 medium (CSIRO recipe) at 20°C under a continuous photon flux density (PFD) of 120–145 μE m−2 s−1, before being transferred to Fe-replete Aquil medium (Price et al., 1988). Stock cultures of the Southern Ocean diatoms P. inermis and E. Antarctica were isolated from waters south of the Antarctic Polar Frontal Zone in December 2001 (Strzepek et al., 2011). The Southern Ocean diatoms have since been maintained in Fe-replete Aquil medium at a temperature of 3°C under a continuous photon flux density (PFD) of ~35 μE m−2 s−1. Cultures were acclimated to the different Fe concentrations in 28 mL polycarbonate vials for a minimum of three transfers (approximately 10–12 generations), before being transferred to 1 L polycarbonate bottles for experimental work. All cultures were grown in batches rather than under semi-continuous conditions to minimize contamination from trace metals. Average PFD's for cultures of E. Antarctica, P. inermis, and T. pseudonana were 45 μE m−2 s−1, 60 μE m−2 s−1 and 133μE m−2 s−1 respectively (Sunda and Huntsman, 1997; Strzepek et al., 2012). Experimental temperatures were 20°C for T. pseudonana and 3°C for the Southern Ocean species.
Medium Preparation
Aquil medium was prepared using trace-metal ultra-clean techniques and enriched with the following nutrients; 10 μmol L−1 phosphate, 100 μmol L−1Si(OH)4, 300 μmol L−1 nitrate, 0.55 μg L−1 vitamin B12, 0.5 μg L−1 Biotin and 100 μg L−1thiamin. Basal medium and stock solutions were eluted through a column containing Toyopearl AF-chelate-650M (Tosohbioscience) ion-exchange resin to remove metal contaminants and filter sterilized (0.2 μm) (Price et al., 1988). Iron contamination of the basal medium was measured using high-resolution IC-PMS after pre-concentration (Ellwood et al., 2008) and found to be 0.56 ± 0.02 nmol L−1 (n = 3). Trace metal ion concentrations were controlled through the addition of a trace-metal ion buffer system, which used 10 and 100 μmol L−1 ethylene-diamine-tetra-acetic-acid (EDTA), as the chelating agent, for both Southern Ocean species and T. pseudonana (respectively). Trace metal concentrations were 7.91 nmol L−1 ZnSO4, 1.98 nmol L−1 CuSO4, 5 nmol L−1 CoCl2, 22.8 nmol L−1 MnCl2, 9.96 nmol L−1 Na2SeO3 and 100 nmol L−1 Na2MoO4 for media containing P. inermis and E. Antarctica; and 100 nmol L−1 ZnSO4, 40 nmol L−1 CuSO4, 40 nmol L−1 CoCl2, 100 nmol L−1 MnCl2, 10 nmol L−1 Na2SeO3 and 100 nmol L−1 Na2MoO4 for media containing T. pseudonana. Free ion concentrations were calculated using Visual MINTEQ, giving concentrations (expressed as –log free metal ion concentration = pMetal) of, pCu 14.07, pMn 8.18, pZn 10.78 and pCo 11.09 for Aquil medium at a temperature of 3°C and a pH of 8.4 for Southern Ocean media, while pMetal values for T. pseudonana media were pCu 13.65, pMn 8.35, pZn 10.72 and pCo 11.26 for Aquil medium at a temperature of 20°C and a pH of 8.1.
Measurement of Culture pH
Culture pH was measured by the change in absorbance of m-cresol purple in culture medium at specific wavelengths of 434, 578, and 730 nm respectively on a Varian Cary 1E UV-visible spectrophotometer with attached temperature controller (Clayton and Byrne, 1993; Yao et al., 2007). Measurements were made at 25°C and corrected for dye-induced changes in the pH. The pH was then adjusted to the culture temperature of 20°C using CO2SYS (http://cdiac.ornl.gov/oceans/co2rprt.html).
Iron Manipulation
T. pseudonana cultures were treated with a range of Fe concentrations varying from Fe limiting to Fe replete: from 30 through to 50, 80, 250, and 500 nmol L−1 of total dissolved Fe, equating to –log Fe3+ concentration (pFe) values of 20.59, 20.36, 20.16, 19.67, and 19.36 respectively. The Fe concentrations were selected to induce different degrees of limitation as defined by the reduction in growth rate from μmax. Inorganic Fe concentrations (Fe′) were calculated according to Sunda and Huntsman (2003) for a temperature of 20°C, a mean irradiance of 133 μE m−2 s−1 and a mean starting pH of 7.98. Fe′ values based on total dissolved Fe concentrations of 500, 250, 80, 50, and 30 nM equated to 0.42, 0.21, 0.07, 0.04, and 0.02 nM respectively (Table 1) (Sunda and Huntsman, 2003).
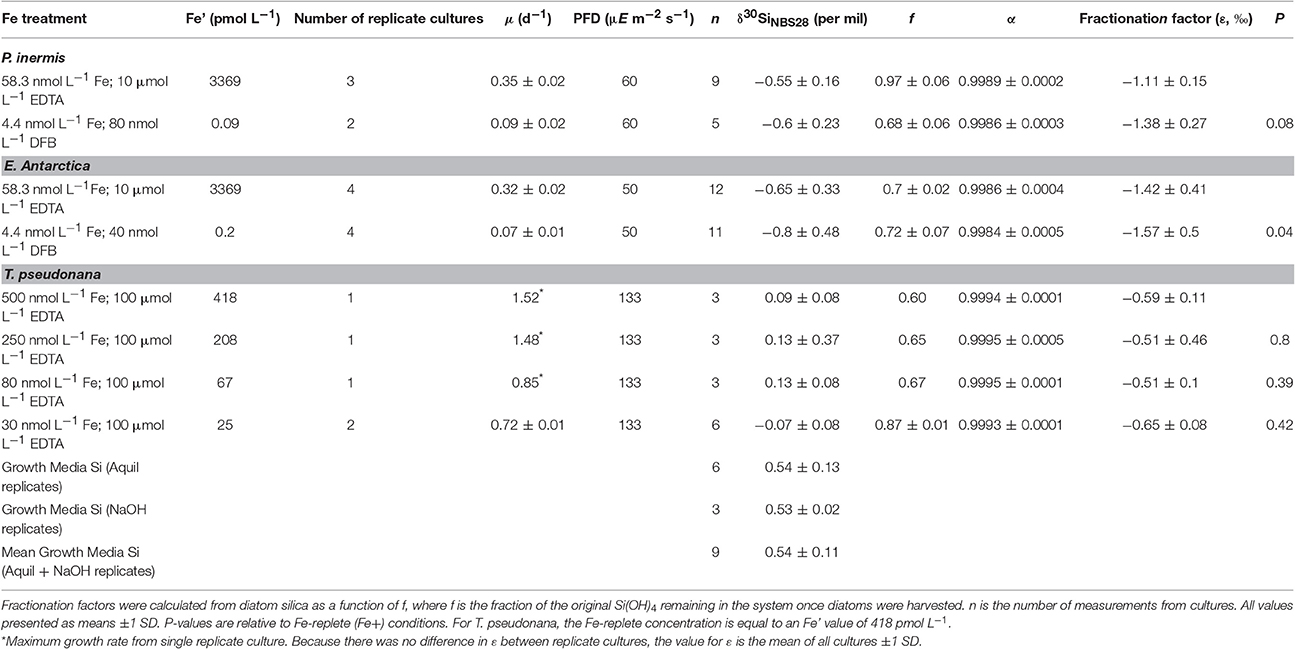
Table 1. Culture conditions, growth rates, Fe concentrations and Results from the Si isotope fractionation experiments for Proboscia inermis, Eucampia antarctica and Thalassiosira pseudonana.
Southern Ocean cultures were treated differently to T. pseudonana cultures, and two competing ligands were used to induce Fe-limitation. This is because Southern Ocean diatoms are well adapted to iron limiting conditions over coastal isolates such as T. pseudonana. Iron replete media was prepared through the addition of a filter-sterilized FeEDTA complex (1:1.05[mol:mol]) to Aquil containing 10 μmol L−1 EDTA for a final concentration (including contamination) of 58.3 nmol L−1 of total dissolved Fe. Iron limited media was prepared by adding Fe pre-complexed with the terrestrial siderophore desferrioxamine B mesylate (DFB; Sigma Aldridge) to Aquil media containing 10 μMol L−1 EDTA for a final concentration of 4.4 nmol L−1. Varying degrees of Fe limitation were induced in culture by increasing the amount of DFB in culture at concentrations of 40 and 80 nmol L−1 for E. Antarctica and P. inermis (respectively). The Fe′ concentration in the iron-replete media was calculated according to Sunda and Huntsman (2003) for a temperature of 3°C, a mean irradiance of 52 μE m−2 s−1 and a pH of 8.4. The overall conditional dissociation constant () was calculated according to methods described by Strzepek et al. (2011) and represents the sum of the conditional stability constant in the dark (3.52 × 10−7) and the conditional photo-dissociation constant (Khv = 2.17 × 10−6) of EDTA at 3°C. The Fe′ concentration for FeDFB treatment was calculated according to the equation [Fe′] = [FeDFB] / [L′] × ; where = 1011.8 (Maldonado et al., 2005). In the instance where total Fe exceeded the concentration of DFB bound Fe (Fetot = 4.4 nmol L−1; DFB = 4 nmol L−1), we assumed a 0.1 nmol L−1 excess of DFB in the media and a 0.5 nmol L−1 excess of total Fe that was available for interaction with EDTA (Table 1).
Specific Growth Rate, Cell Counts and Si(OH)4 Consumption
Growth rates were determined from in vivo chlorophyll a fluorescence using a Turner Designs model 10-AU Fluorometer. Specific growth rates of exponentially growing cultures were determined from linear regressions of ln in vivo fluorescence vs. time. Cell density (cells mL−1) was determined by microscopy (Sedgewick-Rafter or Haemocytometer – brightline, Neubauer improved). Each sample was enumerated a minimum of three times resulting in a S.E between counts <11% for P. inermis and E. Antarctica, and <5% for T. pseudonana. Consumption of Si(OH)4 in culture was obtained by measuring samples of culture media shortly after inoculation and at the exponential stage of growth (respectively), when cultures were harvested.
δ30Si Determination of Diatom Silica
Once cultures were in their exponential phase of growth, 50–100 ml of cell culture was collected onto a 2 μm, 25 mm polycarbonate filter, rinsed with nutrient free Aquil, and washed into 3 mL Teflon bombs. Purification of the samples were done according to similar methods for sponge spicules by Wille et al. (2010). The samples were evaporated to dryness at 50°C in a drying oven overnight. To remove organics, samples were treated with 1 mL of 30% hydrogen-peroxide (H2O2) solution, and allowed to reflux for 24 h at 70°C on a hotplate. Following oxidation, the lids were removed, and the samples were left to evaporate to dryness before adding 2 ml of 0.5 M sodium hydroxide (NaOH) to dissolve the sediment. Samples were then left to reflux overnight again for 24 h at 50°C. The Si(OH)4concentration within the sample digest was then measured colorimetrically (Strickland and Parsons, 1965). Sodium was removed from the samples using cation exchange columns to prevent any subsequent interference during isotope determination. Cation exchange columns consisted on 1 mL Dowex 50W-X8 cation exchange resin (200–400 mesh) with a 2.5 mL reservoir. Columns were cleaned with 0.5 mL 8% (w/w) hydrofluoric acid (HF) followed by 2.25 mL of deionized water. The resin with protonated by passing 2.25 mL of 4 mol L−1 HCl followed by 2.25 mL of deionized water. The columns were then loaded with 0.5 mL of sample, before being rinsed with 4 × 0.5 mL of deionized water. To minimize contamination, samples were collected in vials that were cleaned using 8% (w/w) HF, before being rinsed with deionized water. Samples for δ30Si determination of the growth media were prepared by dissolving the sodium silicate standard used for nutrient enrichment in the growth medium to a concentration of 2 mmol L−1 in separate solutions of nutrient free Aquil and 0.5 M sodium hydroxide. These samples were then treated with the same column procedure as the cell samples prior to analysis.
Determination of δ30Si of Diatom Silica
The δ30Si composition of diatom silica was determined according to methods developed by Wille et al. (2010) using a multi-collector inductively coupled plasma mass spectrometer (MC-ICP-MS) (Finnigan Neptune, Germany) operating in dry plasma mode at medium-resolution (M/ΔM ~2,000). An ESI-Apex nebulizer fitted with a Teflon inlet system and a demountable torch fitted with an alumina injector was used for sample introduction to minimize any background interference. A standard-sample-standard bracketing technique was used for data acquisition and reduction (Wille et al., 2010). The δ30Si signal (based on the relative abundance of 30Si to 28Si (30Si/28Si) was calculated using the following formula:
Where Rsample is the ratio of 30Si/28 Si of the sample and RStd is the 30Si/28Si of the in-house standard developed from dissolution a diatomaceous sediment and purified (Figure 1). Measurements of sample blanks were made prior to each run to ensure that the combined blank and background was <1% of the total sample signal. Inter-laboratory NBS-28 and diatomite standards were prepared with each daily run, and were measured with every three samples (≤ 8 diatomite/NBS -28 standards per daily run).
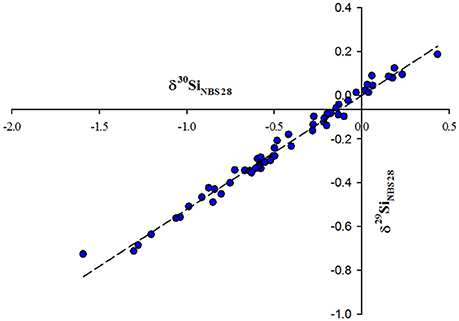
Figure 1. Mass dependent fractionation of δ29Si vs. δ30Si for all diatom samples relative to NBS-28. Regression line represents δ29Si = [0.520 ± 0.009].δ30Si −0.002 ± 0.006, (r2 = 0.99, n = 55 samples).
δ30Si and δ29Si values relative to NBS-28 plotted on an mass-dependent fractionation line with a slope of 0.520 ± 0.009 and is consistent with the consensus slope of 0.511 obtained from inter-laboratory silicon standard measurements (Reynolds et al., 2007). The reproducibility of the δ30Si signal measured for the NBS-28 standard (prepared in full and measured on 5 separate occasions) was 0.25 ± 0.04 ‰. Measurements of the “diatomite standard” produced a mean δ30Si value of 1.29 ± 0.25 ‰ (2 SD, n = 33), and is in good agreement with inter-laboratory comparisons (δ30Si = 1.27 ‰) (Reynolds et al., 2007).
Results
Growth rates between replicate cultures varied by <6 % (coefficient of variation, CV) and f was kept above a value of 0.6 in all cultures (Table 1). All values for ε are presented as means, ± 1 standard deviation (S.D.). P. inermis, E. Antarctica and T. pseudonana exhibited mean ε values of −1.11 ± 0.15 ‰, −1.42 ± 0.41 ‰ and −0.59 ± 0.11 ‰, respectively when grown under Fe-replete conditions (Figure 2). The mean ε values for P. inermis and E. Antarctica grown under Fe-replete conditions were not statistically different (p > 0.05) from the mean value of −1.1 ‰ obtained by De La Rocha et al. (1997); however, they were statistically different compared to the mean ε value for T. pseudonana (p < 0.05). Whilst a relatively low f compared to alternate studies (e.g., Sutton et al., 2013) may introduce an underestimation of the results (De La Rocha et al., 1997); the observation that T. pseudonana exhibited little variation in its isotope composition despite the range in f values suggests that this bias was insignificant (Table 2).
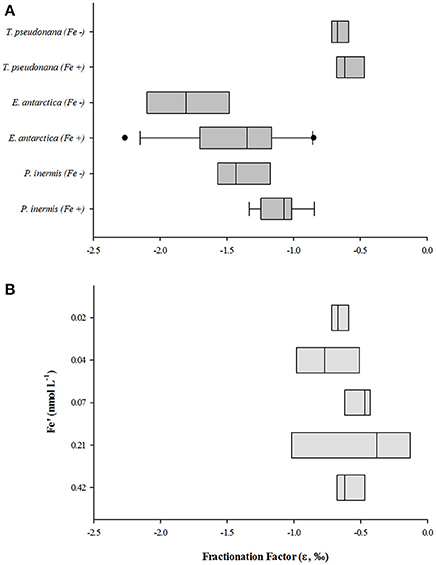
Figure 2. Box and whisker plot displaying the effects of Fe-availability on T. pseduonana, P. inermis and E. Antarctica. (A) Represents comparisons between all species, (B) represents the variation in Si-isotope fractionation factor across multiple Fe-concentrations for T. pseudonana. Lines are median values, Boxes represent 1st and 3rd quartiles and dots represent outliers (n ≥ 3).
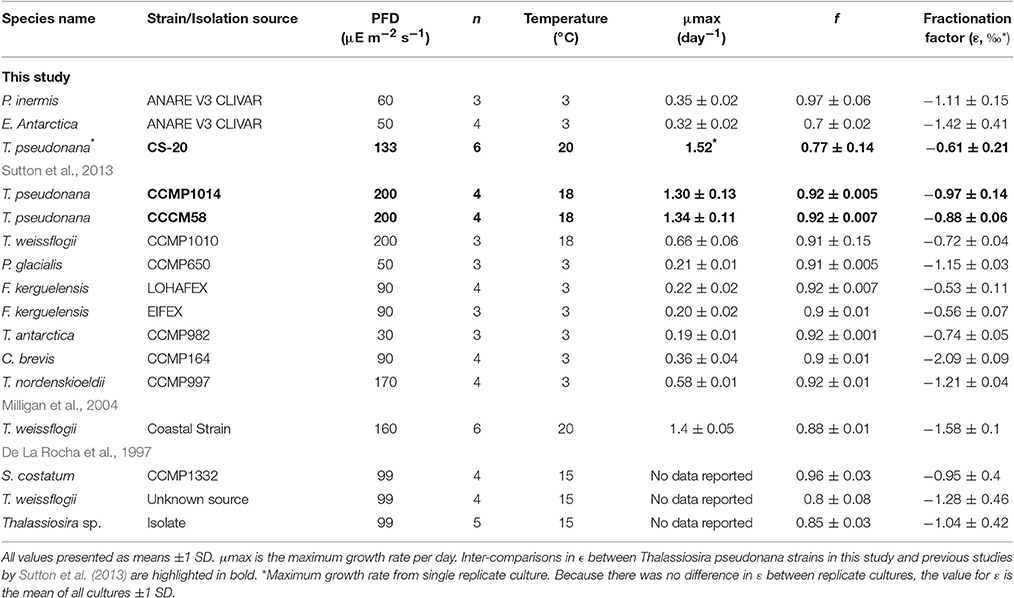
Table 2. Comparison of Fe-replete, Si-isotope fractionation factors (ε, ‰) between this study and previous studies. n is the number of replicate cultures.
Effects of Fe-Availability on Si Isotope Fractionation
T. pseudonana displayed little variation in ε with decreasing Fe concentration (Figure 2). No clear trends were observed across the five Fe-conditions, with ε values ranging between 0.51 ± 0.46 ‰ and 0.75 ± 0.24 ‰. Comparisons of ε values between Fe-limited (Fe′ = 208, 67, 42, and 25 pmol L−1) and Fe-replete conditions (Fe′ = 418 pmol L−1) were not statistically significant (ANOVA, p > 0.1). Because of the statistical insignificance between these ε values, the mean was taken of all values (−0.61 ± 0.21 ‰) and compared against ε values obtained from two strains of T. pseudonana (CCMP1014 and CCCM58, −0.97 ± 0.14 and −0.88 ± 0.06 ‰, respectively) cultured under Fe-replete conditions by Sutton et al. (2013). We observed a difference (p < 0.05) between the ε in our strain (CS-20) and the two strains grown by Sutton et al. (2013) (Table 2).
Mean values for ε varied between the Fe-replete and Fe-limited conditions for the two Southern Ocean diatoms (Table 2). While there was some variability in the values of ε for E. Antarctica, there was a significant difference in ε (p = 0.04) between Fe-replete and Fe-limited conditions, with mean ε values being more negative for Fe-limited cultures (Fe-limited, ε = −1.57 ± 0.50 ‰; Fe-replete, ε = −1.40 ± 0.41 ‰) (Table 1). Mean ε values were also more negative for P. inermis cultured under Fe-limited conditions (Fe-limited, ε = −1.38 ± 0.27 ‰; Fe-replete, ε = −1.11 ± 0.15 ‰). Both Fe-replete and Fe-limited datasets for P. inermis exhibited a significant difference at the 90% confidence interval (p = 0.08), and removing outliers from both Fe-replete and Fe-limited data sets for P. inermis makes the difference more significant (p = 0.04).
Discussion
This is the first study to specifically investigate the effects of Fe-limitation on Si-isotope fractionation in diatoms. Previous work has demonstrated that Fe-limitation can alter Si(OH)4uptake kinetics, cell morphology and nutrient stoichiometry in diatoms (Hutchins and Bruland, 1998; Takeda, 1998; Brzezinski et al., 2002; Leynaert et al., 2004; Meyerink et al., 2017). Here, the processes by which Si isotopes are fractionated during diatom Si uptake and cell wall synthesis are explored and related back to mechanisms involving Si(OH)4 uptake.
Fractionation of Si-Isotopes in Diatoms
Whilst little effect in the variability of the Si-isotope fractionation factor was observed in T. pseudonana in response to Fe-stress (Figure 2B), both Southern Ocean species exhibited a fractionation factor that was more negative under Fe-limitation (Figure 2A). Values for ε have been observed to vary under optimal growth conditions between diatom species (Sutton et al., 2013), so it is possible that there may be an inter-species effect when it comes to environmental controls on Si-isotope fractionation in diatoms. Sutton et al. (2013) demonstrated that Si-isotope fractionation can vary by as much as 1.5 ‰ between diatom species, enough to potentially confound palaeoceanographic interpretations utilizing δ30Si from diatom opal to estimate the nutrient status of the surface ocean. In addition to interspecies effects, variations in the value for ε between individual diatom strains have been observed (Sutton et al., 2013). Silicon isotope fractionation independently measured in T. weissflogii across three seperate studies yielded ε values ranging between −0.72 ± 0.04 ‰ and −1.5 ± 0.1 ‰ (De La Rocha et al., 1997; Milligan et al., 2004; Sutton et al., 2013). Similar variations have also been observed for three T. pseudonana clones; −0.61 ± 0.21 ‰ measured in this study and −0.94 ± 0.14 ‰ and −0.88 ± 0.06 ‰ measured for two clones cultured by Sutton et al. (2013). Sutton et al. (2013) suggest that the differences in ε values for T. weissflogii can be attributable to the diatom being a coastal isolate rather than an open ocean isolate. There were remarkable similarities, however, in ε values between the two T. pseudonana strains (CCMP1014 and CCCM58) cultured by Sutton et al. (2013), even though CCMP1014 is an open ocean isolate from the (North Pacific Gyre; https://ncma.bigelow.org/) and CCCM58 is a coastal isolate (Moriches Bay, Long island, NY; http://www3.botany.ubc.ca/cccm/index.html). While it is possible that inherent differences between strains as a result of the growth conditions in their endemic environment, variations culturing conditions need to be ruled out first before this hypothesis can be invoked.
Potential Mechanisms Controlling Si Isotope Fractionation in Diatoms
Silicon-processing in diatoms is affected by Fe bioavailability (Meyerink et al., 2017) and hence can manifest to variations in the δ30Si composition of diatom silica. Understanding how diatoms fractionate Si isotopes during uptake and bio-mineralization requires a thorough investigation into how diatoms take up Si(OH)4 from the surrounding water column and use it in the formation of their cell frustules (Sutton et al., 2013; Meyerink et al., 2017). There are numerous intracellular pathways during the synthesis of biogenic silica in diatoms where fractionation of Si isotopes can potentially occur.
In diatoms, Si(OH)4 uptake from the surrounding water column is typically characterized either by diffusion or Michaelis-Menten saturation kinetics (Hildebrand, 2008; Javaheri et al., 2014). At low ambient Si(OH)4 concentrations, uptake is mainly a saturatable process (≤ 10 μmol L−1), and is facilitated by Si transporters (SITs) that are localized in cell membranes (Shrestha and Hildebrand, 2015). SITs are membrane bound proteins that actively take up Si(OH)4and transport it across the outer cell membrane to the cell cytoplasm against a concentration gradient (Hildebrand, 2008). At higher ambient Si(OH)4 concentrations (≥30 μmol L−1), diffusional uptake dominates Si acquisition with SITs playing more of a regulatory role (Thamatrakoln and Hildebrand, 2008; Shrestha and Hildebrand, 2015). Passive diffusion of Si(OH)4 appears to be proportional to the permeability of the cell membrane and the concentration gradient across the cell membrane (Hildebrand, 2008; Javaheri et al., 2014). Once past the cell membrane, Si(OH)4 is then transported intracellularly to the Si deposition vesicle (SDV) by as yet unknown transporters/compounds classified simply as “Si binding components” (Thamatrakoln and Hildebrand, 2008). Si binding components play an important role in setting the Si(OH)4 concentration gradient across the cell membrane under scenarios where diffusional uptake dominates (Thamatrakoln and Hildebrand, 2008). Active transport involving SITs likely plays an important role in facilitating the transport of Si from the cell cytoplasm into the SDV, as concentration gradients across the intracellular membrane separating the SDV from the cell cytoplasm are extremely high (Hildebrand, 2008). A new cell wall is created when the SDV is exocytosed at the M (mitosis) stage of the cell cycle. Cell wall formation during this stage is facilitated by silafins and long chain polyamines, which act as a “template” on to which the new cell wall is precipitated (Mock et al., 2008).
Fractionation of Si isotopes can potentially occur along any of these pathways. Milligan et al. (2004) explored where isotopic discrimination takes place within the cell by conducting a number of experiments where they varied the efflux:influx ratio of Si(OH)4 into and out of the cell. They suggested that fractionation of Si isotopes occurs during the membrane transport step and not during Si polymerization, as Si isotope fractionation should scale linearly with the efflux:influx ratio. In addition, the relatively small Si isotope effect may also be evidence of discrimination at the transport level, as this does not necessitate the breaking or forming of chemical bonds (Milligan et al., 2004). If this is the case, fractionation of Si isotopes occurs either at the membrane/seawater interface, as Si(OH)4 is taken up from the water column and transported to the cell cytoplasm, or, as Si is transported from the cell cytoplasm into the Silicon deposition vesicle.
Effects of Fe-Limitation on Si-Isotope Fractionation in Diatoms
Sutton et al. (2013) observed an inter-specific variation in mean ε values in Southern Ocean diatom species based on mono-culture in-vitro incubations. They argue that variability in diatom community composition could partly explain the observed variability in Southern Ocean Si-isotope fractionation factors. It is possible that variations in the Fe-status of certain regions in the Southern Ocean may contribute to variations in the apparent Si isotope fractionation factor. For example, Si isotope fractionation factors of diatom communities can potentially vary as a result of certain diatoms adapted to low Fe-conditions out competing other diatoms under Fe-limiting conditions. This may alter the δ30SiDSi composition of surface waters. While this scenario is hypothetical, it may contribute to some of the variability in the observed relationship between the mixed layer mean apparent fractionation factor (Δ30Si = δ30SiDSi – δ30SiBSi) and Si(OH)4 concentration in the Southern Ocean and the seasonal variations in Si uptake:dissolution (Varela et al., 2004; Cardinal et al., 2007; Fripiat et al., 2012).
Silicon metabolism in diatoms is particularly sensitive to variations in Fe-supply and as such, variations in ε can potentially be dependent on Fe-induced physiological variations within the cell. A notable result from our study was the relative response in the Si isotope fractionation factor (ε) of T. pseudonana under Fe-limitation compared to P. inermis and E. Antarctica. T. pseudonana exhibited no variation in ε in response to Fe-limitation, and maintained a mean ε value of –0.61 ± 0.21 ‰ across all Fe′ concentrations. In contrast, we observed a negative response in Si isotope fractionation as a result of increased Fe-limitation in both Southern Ocean species (Figure 2). The reason behind this difference could lie in how Si is metabolized by Southern Ocean diatoms under Fe-stress.
Kinetic uptake experiments show diatoms decrease their Si(OH)4 uptake rate under Fe-limitation (Franck et al., 2000; Leynaert et al., 2004; Meyerink et al., 2017). This is generally manifested by a reduction in the maximal Si(OH)4 uptake rate (VSi−max) and half saturation constant (KSi). In addition, diatoms grown under Fe-limiting conditions generally exhibit reductions in their growth rate, and variations in their cell morphology. A recent study by Meyerink et al. (2017) investigated the effects of Fe availability on the Si(OH)4 uptake kinetics of two Southern Ocean diatoms (E. Antarctica and P. inermis) and a coastal isolate (T. pseudonana). An interesting result from the study shows that when VSi−max is normalized to cell surface area, it exhibits a linear relationship with cell growth rate. This relationship is independent of any variations in cell morphology or species. It is likely that this relationship can be explained by the fact that silicon uptake in the diatoms was under diffusion control, as they were acclimatized under high Si conditions. Under diffusion controlled uptake, the VSi−max values reflect the realized Si(OH)4 uptake rates in culture which at steady state cannot exceed cellular demands for Si(OH)4 (Thamatrakoln and Hildebrand, 2008). In contrast, variations in KSi in response to Fe availability exhibited no relationship with other cell parameters, however it was noticeably higher in value in the Southern Ocean species than the KSi value for T. pseudonana. This is likely because the Southern Ocean diatoms are well adapted to growing under high Si conditions.
Diatoms with a high KSi for Si(OH)4 such as E. Antarctica and P. inermis (≥10 μmol L−1) (Meyerink et al., 2017) could possibly fractionate Si-isotopes differently to diatoms with a relatively low KSi such as T. pseudonana because of their likely dependence on diffusional uptake of Si(OH)4 at concentrations greater than 30 μmol L−1 (Thamatrakoln and Hildebrand, 2008). Under Fe-limitation, diatoms decrease their cellular growth rate, which results in a subsequent decrease in their maximal Si(OH)4 uptake rate (VSi−max) (Franck et al., 2000; Leynaert et al., 2004; Meyerink et al., 2017). This may result in a decrease in a diatoms specific affinity for Si(OH)4 that can be maintained by a decrease in the KSi (Franck et al., 2000; Leynaert et al., 2004; Meyerink et al., 2017). Previous studies suggest that diatoms maintain their affinity by altering their cell size (Leynaert et al., 2004); however, E. Antarctica exhibits a 2-fold decrease in KSi when Fe-limited, which is concomitant with an increase in cell-size (Meyerink et al., 2017). It is therefore more likely that diatoms maintain their affinity for Si(OH)4 by adjusting the point where diffusive transport takes over from active transport. Active transport persists at Si(OH)4 concentrations greater than 30 μmol L−1 under Fe-limitation, and as a result, could possibly induce a decrease in the diatoms Si-isotope fractionation factor (Shrestha and Hildebrand, 2015). If this is correct, why do E. Antarctica and P. inermis exhibit a more negative response in ε when compared to T. pseudonana? It is possible that different genera of diatoms switch from active to diffusive transport at different Si(OH)4 concentrations. While T. pseudonana does this at ~30 μmol L−1 under Fe-replete conditions (Shrestha and Hildebrand, 2015), this value could be higher for Southern Ocean diatoms. When diatoms approached Fe-limiting conditions, such as in this study, T. pseudonana possibly remained under the threshold where active transport takes place, while E. Antarctica and P. inermis did not. If this is the case, then Si-isotope signatures may potentially be subject to the synergistic effects of species diversity and resource limitation.
Conclusions
This is the first culture study to investigate the effects of Fe-limitation on Si-isotope fractionation in diatoms. Whilst any effects arising from Fe-limitation on the value of ε were not evident in T. pseudonana, we observed that both Southern Ocean diatoms under Fe-limitation exhibit mean ε values that are 0.19–0.27‰ (within error) more negative compared to Fe-replete values. While this variation is likely not enough to have any significant effect on interpretations involving δ30Si in diatomaceous opal or sea surface Si(OH)4/BSi, the finding that the value for ε in the Southern Ocean species was prone to changes in the physico-chemical environment compared to that of the coastal species highlights the need to improve our understanding of how diatoms fractionate Si-isotopes during Si-uptake and frustule formation, and thus, warrants further investigation into the biochemical pathways in diatoms where Si-isotope fractionation can potentially occur.
Author Contributions
SM and ME conceived of the research. SM undertook the experiments and SM and ME interpreted the results with contributions from WM and RS. SM wrote the manuscript with contributions from ME, WM, and RS.
Conflict of Interest Statement
The authors declare that the research was conducted in the absence of any commercial or financial relationships that could be construed as a potential conflict of interest.
Acknowledgments
The Australian Research Council (DP130100679) and the Australian Antarctic Division (AAD Project 3120) are acknowledged for financial support of this work. The Authors would also like to thank two reviewers for their helpful comments.
References
Beucher, C. P., Brzezinski, M. A., and Crosta, X. (2007). Silicic acid dynamics in the glacial sub-Antarctic: implications for the silicic acid leakage hypothesis. Global Biogeochem. Cycles 21:GB3015. doi: 10.1029/2006GB002746
Beucher, C. P., Brzezinski, M. A., and Jones, J. L. (2011). Mechanisms controlling silicon isotope distribution in the Eastern Equatorial Pacific. Geochim. Cosmochim. Acta 75, 4286–4294. doi: 10.1016/j.gca.2011.05.024
Boutorh, J., Moriceau, B., Gallinari, M., Ragueneau, O., and Bucciarelli, E. (2016). Effect of trace metal-limited growth on the postmortem dissolution of the marine diatom Pseudo-nitzschia delicatissima. Global Biogeochem. Cycles 30, 57–69. doi: 10.1002/2015GB005088
Brzezinski, M. A., Pride, C. J., Franck, V. M., Sigman, D. M., Sarmiento, J. L., Matsumoto, K., et al. (2002). A switch from Si(OH)4 to depletion in the glacial Southern Ocean. Geophys. Res. Lett. 29, 5-1–5-4. doi: 10.1029/2001gl014349
Cardinal, D., Savoye, N., Trull, T. W., Dehairs, F., Kopczynska, E. E., Fripiat, F., et al. (2007). Silicon isotopes in spring Southern Ocean diatoms: large zonal changes despite homogeneity among size fractions. Mar. Chem. 106, 46–62. doi: 10.1016/j.marchem.2006.04.006
Clayton, T. D., and Byrne, R. H. (1993). Spectrophotometric seawater pH measurements: total hydrogen ion concentration scale calibration of m-cresol purple and at-sea results. Deep Sea Res. Pt I 40, 2115–2129. doi: 10.1016/0967-0637(93)90048-8
Criss, R. E., and Criss, R. (1999). Principles of Stable Isotope Distribution. New York, NY: Oxford University Press.
De La Rocha, C., Brzezinski, M., DeNiro, M., and Shemeshk, A. (1998). Silicon-isotope composition of diatoms as an indicator of past oceanic change. Phys. Rev. Lett. 77, 2041–2044.
De La Rocha, C. L., Brzezinski, M. A., and DeNiro, M. J. (1997). Fractionation of silicon isotopes by marine diatoms during biogenic silica formation. Geochim. Cosmochim. Acta 61, 5051–5056. doi: 10.1016/S0016-7037(97)00300-1
Ellwood, M. J., Boyd, P. W., and Sutton, P. (2008). Winter-time dissolved iron and nutrient distributions in the Subantarctic zone from 40-52S; 155-160E. Geophys. Res. Lett. 35:L11604. doi: 10.1029/2008gl033699
Ellwood, M. J., Wille, M., and Maher, W. (2010). Glacial silicic acid concentrations in the Southern Ocean. Science 330, 1088–1091. doi: 10.1126/science.1194614
Franck, V. M., Brzezinski, M. A., Coale, K. H., and Nelson, D. M. (2000). Iron and silicic acid concentrations regulate Si uptake north and south of the polar frontal zone in the pacific sector of the southern ocean. Deep Sea Res. Pt II 47, 3315–3338. doi: 10.1016/S0967-0645(00)00070-9
Fripiat, F., Cavagna, A.-J., Dehairs, F., Brauwere, A. D., André, L., and Cardinal, D. (2012). Processes controlling the Si-isotopic composition in the Southern Ocean and application for paleoceanography. Biogeosciences 9, 2443–2457. doi: 10.5194/bg-9-2443-2012
Fripiat, F., Cavagna, A.-J., Dehairs, F., Speich, S., Andre, L., and Cardinal, D. (2011). Silicon pool dynamics and biogenic silica export in the Southern Ocean inferred from Si-isotopes. Ocean Sci. 7, 533–547. doi: 10.5194/os-7-533-2011
Hildebrand, M. (2008). Diatoms, biomineralization processes, and genomics. Chem. Rev. 108, 4855–4874. doi: 10.1021/cr078253z
Hoffmann, L., Peeken, I., and Lochte, K. (2008). Iron, silicate, and light co-limitation of three Southern Ocean diatom species. Polar Biol. 31, 1067–1080. doi: 10.1007/s00300-008-0448-6
Hutchins, D. A., and Bruland, K. W. (1998). Iron-limited diatom growth and Si:N uptake ratios in a coastal upwelling regime. Nature 393, 561–564. doi: 10.1038/31203
Javaheri, N., Dries, R., and Kaandorp, J. (2014). Understanding the sub-cellular dynamics of silicon transportation and synthesis in diatoms using population-level data and computational optimization. PLoS Comput. Biol. 10:e1003687. doi: 10.1371/journal.pcbi.1003687
Kemp, A. E. S., Pike, J., Pearce, R. B., and Lange, C. B. (2000). The “Fall dump” – a new perspective on the role of a “shade flora” in the annual cycle of diatom production and export flux. Deep Sea Res. Pt II 47, 2129–2154. doi: 10.1016/S0967-0645(00)00019-9
Leynaert, A., Bucciarelli, E., Claquin, P., Dugdale, R. C., Martin-Jezequel, V., Pondaven, P., et al. (2004). Effect of iron deficiency on diatom cell size and silicic acid uptake kinetics. Limnol. Oceanogr. 49, 1134–1143. doi: 10.4319/lo.2004.49.4.1134
Maldonado, M. T., Strzepek, R. F., Sander, S., and Boyd, P. W. (2005). Acquisition of iron bound to strong organic complexes, with different Fe binding groups and photochemical reactivities, by plankton communities in Fe-limited subantarctic waters. Global Biogeochem. Cycles 19:GB4S23. doi: 10.1029/2005GB002481
Marchetti, A., and Cassar, N. (2009). Diatom elemental and morphological changes in response to iron limitation: a brief review with potential paleoceanographic applications. Geobiology 7, 419–431. doi: 10.1111/j.1472-4669.2009.00207.x
Martinez-Garcia, A., Rosell-Mele, A., Jaccard, S. L., Geibert, W., Sigman, D. M., and Haug, G. H. (2011). Southern Ocean dust-climate coupling over the past four million years. Nature 476, 312–315. doi: 10.1038/nature10310
Matsumoto, K., Chase, Z., and Kohfeld, K. (2014). Different mechanisms of silicic acid leakage and their biogeochemical consequences. Paleoceanography 29, 238–254. doi: 10.1002/2013PA002588
Matsumoto, K., Sarmiento, J. L., and Brzezinski, M. A. (2002). Silicic acid leakage from the southern ocean: a possible explanation for glacial atmospheric pCO2. Global Biogeochem. Cycles 16, 5–1. doi: 10.1029/2001GB001442
McManus, J., Hammond, D. E., Berelson, W. M., Kilgore, T. E., Demaster, D. J., Ragueneau, O. G., et al. (1995). Early diagenesis of biogenic opal: dissolution rates, kinetics, and paleoceanographic implications. Deep Sea Res. Pt II 42, 871–903. doi: 10.1016/0967-0645(95)00035-O
Meyerink, S. W., Ellwood, M. J., Maher, W. A., Price, G. D., and Strzepek, R. F. (2017). Effects of iron limitation on silicon uptake kinetics and elemental stoichiometry in two Southern Ocean diatoms, Eucampia antarctica and Proboscia inermis, and the temperate diatom Thalassiosira pseudonana. Limnol. Oceanogr. doi: 10.1002/lno.10578. [Epub ahead of print].
Milligan, A. J., Varela, D. E., Brzezinski, M. A., and Morel, F. M. M. (2004). Dynamics of silicon metabolism and silicon isotopic discrimination in a marine diatom as a function of pCO2. Limnol. Oceanogr. 49, 322–329. doi: 10.4319/lo.2004.49.2.0322
Mock, T., Samanta, M. P., Iverson, V., Berthiaume, C., Robison, M., Holtermann, K., et al. (2008). Whole-genome expression profiling of the marine diatom Thalassiosira pseudonana identifies genes involved in silicon bioprocesses. Proc. Nat. Acad. Sci. U.S.A. 105, 1579–1584. doi: 10.1073/pnas.0707946105
Nelson, D. M., Treguer, P., Brzezinski, M. A., Leynaert, A., and QuÈguiner, B. (1995). Production and dissolution of biogenic silica in the ocean: revised global estimates, comparison with regional data and relationship to biogenic sedimentation. Global Biogeochem. Cycles 9, 359–372. doi: 10.1029/95GB01070
Pichevin, L. E., Reynolds, B. C., Ganeshram, R. S., Cacho, I., Pena, L., Keefe, K., et al. (2009). Enhanced carbon pump inferred from relaxation of nutrient limitation in the glacial ocean. Nature 459, 1114–1117. doi: 10.1038/nature08101
Price, N. M., Harrison, G. I., Hering, J. G., Hudson, R. J., Nirel, P. M., Palenik, B., et al. (1988). Preparation and chemistry of the artificial algal culture medium Aquil. Biol. Oceanogr. 6, 443–461.
Ragueneau, O., Treguer, P., Leynaert, A., Anderson, R. F., Brzezinski, M. A., DeMaster, D. J., et al. (2000). A review of the Si cycle in the modern ocean: recent progress and missing gaps in the application of biogenic opal as a paleoproductivity proxy. Glob. Planet. Change 26, 317–365. doi: 10.1016/S0921-8181(00)00052-7
Reynolds, B. C., Aggarwal, J., André, L., Baxter, D., Beucher, C., Brzezinski, M. A., et al. (2007). An inter-laboratory comparison of Si isotope reference materials. J. Anal. Atom. Spectrom. 22, 561–568. doi: 10.1039/B616755A
Roca-Martí, M., Puigcorbé, V., Iversen, M. H., van der Loeff, M. R., Klaas, C., Cheah, W., et al. (2017). High Particulate organic carbon export during the decline of a vast diatom bloom in the Atlantic sector of the Southern Ocean. Deep-Sea Res. Pt. II 138, 102–115. doi: 10.1016/j.dsr2.2015.12.007
Rousseau, J., Ellwood, M. J., Bostock, H., and Neil, H. (2016). Estimates of late Quaternary mode and intermediate water silicic acid concentration in the Pacific Southern Ocean. Earth Planet. Sci. Lett. 439, 101–108. doi: 10.1016/j.epsl.2016.01.023
Sackett, O., Petrou, K., Reedy, B., De Grazia, A., Hill, R., Doblin, M., et al. (2013). Phenotypic plasticity of southern ocean diatoms: key to success in the sea ice habitat? PLoS ONE 8:e81185. doi: 10.1371/journal.pone.0081185
Shrestha, R. P., and Hildebrand, M. (2015). Evidence for a regulatory role of diatom silicon transporters in cellular silicon responses. Eukaryot. Cell 14, 29–40. doi: 10.1128/EC.00209-14
Smetacek, V. (1999). Diatoms and the ocean carbon cycle. Protist 150, 25–32. doi: 10.1016/S1434-4610(99)70006-4
Strickland, J. D. H., and Parsons, T. R. (1965). A Manual of Sea Water Analysis. Ottawa, ON: Fisheries Research Board of Canada.
Strzepek, R. F., Hunter, K. A., Frew, R. D., Harrison, P. J., and Boyd, P. W. (2012). Iron-light interactions differ in Southern Ocean phytoplankton. Limnol. Oceanogr. 57, 1182–1200. doi: 10.4319/lo.2012.57.4.1182
Strzepek, R. F., Maldonado, M. T., Hunter, K. A., Frew, R. D., and Boyd, P. W. (2011). Adaptive strategies by Southern Ocean phytoplankton to lessen iron limitation: Uptake of organically complexed iron and reduced cellular iron requirements. Limnol. Oceanogr. 56, 1983–2002. doi: 10.4319/lo.2011.56.6.1983
Sunda, W., and Huntsman, S. (2003). Effect of pH, light, and temperature on Fe-EDTA chelation and Fe hydrolysis in seawater. Mar. Chem. 84, 35–47. doi: 10.1016/S0304-4203(03)00101-4
Sunda, W. G., and Huntsman, S. A. (1997). Interrelated influence of iron, light and cell size on marine phytoplankton growth. Nature 390, 389–392. doi: 10.1038/37093
Sutton, J. N., Varela, D. E., Brzezinski, M. A., and Beucher, C. P. (2013). Species-dependent silicon isotope fractionation by marine diatoms. Geochim. Cosmochim. Acta 104, 300–309. doi: 10.1016/j.gca.2012.10.057
Takeda, S. (1998). Influence of iron availability on nutrient consumption ratio of diatoms in oceanic waters. Nature 393, 774–777. doi: 10.1038/31674
Thamatrakoln, K., and Hildebrand, M. (2008). Silicon uptake in diatoms revisited: a model for saturable and nonsaturable uptake kinetics and the role of silicon transporters. Plant Physiol. 146, 1397–1407. doi: 10.1104/pp.107.107094
Trèguer, P., Nelson, D. M., Van Bennekom, A. J., DeMaster, D. J., Leynaert, A., and Queguiner, B. (1995). The silica balance in the world ocean: a reestimate. Science 268:375. doi: 10.1126/science.268.5209.375
Tréguer, P. J., and De La Rocha, C. L. (2013). The world ocean silica cycle. Ann. Rev. Mar. Sci. 5, 477–501. doi: 10.1146/annurev-marine-121211-172346
Varela, D. E., Pride, C. J., and Brzezinski, M. A. (2004). Biological fractionation of silicon isotopes in Southern Ocean surface waters. Global Biogeochem. Cycles 18. doi: 10.1029/2003GB002140
Wille, M., Sutton, J., Ellwood, M. J., Sambridge, M., Maher, W., Eggins, S., et al. (2010). Silicon isotopic fractionation in marine sponges: A new model for understanding silicon isotopic variations in sponges. Earth Planet. Sci. Lett. 292, 281–289. doi: 10.1016/j.epsl.2010.01.036
Keywords: silicon isotopes, Southern Ocean, diatom, isotope fractionation, iron
Citation: Meyerink S, Ellwood MJ, Maher WA and Strzepek R (2017) Iron Availability Influences Silicon Isotope Fractionation in Two Southern Ocean Diatoms (Proboscia inermis and Eucampia antarctica) and a Coastal Diatom (Thalassiosira pseudonana). Front. Mar. Sci. 4:217. doi: 10.3389/fmars.2017.00217
Received: 28 February 2017; Accepted: 22 June 2017;
Published: 07 July 2017.
Edited by:
Brivaela Moriceau, Centre National de la Recherche Scientifique, FranceReviewed by:
Damien Cardinal, Université Pierre et Marie Curie, FranceFrank Dehairs, Vrije Universiteit Brussel, Belgium
Copyright © 2017 Meyerink, Ellwood, Maher and Strzepek. This is an open-access article distributed under the terms of the Creative Commons Attribution License (CC BY). The use, distribution or reproduction in other forums is permitted, provided the original author(s) or licensor are credited and that the original publication in this journal is cited, in accordance with accepted academic practice. No use, distribution or reproduction is permitted which does not comply with these terms.
*Correspondence: Scott Meyerink, scott.meyerink@anu.edu.au
Michael J. Ellwood, michael.ellwood@anu.edu.au