Niche Differentiation and Prey Selectivity among Common Bottlenose Dolphins (Tursiops truncatus) Sighted in St. George Sound, Gulf of Mexico
- 1Earth Ocean and Atmospheric Sciences, Florida State University, Tallahassee, FL, United States
- 2Sarasota Dolphin Research Program, Chicago Zoological Society, Sarasota, FL, United States
- 3University of Louisiana, Lafayette, LA, United States
- 4JHT, Inc., National Oceanic and Atmospheric Administration, Charleston, SC, United States
- 5National Marine Mammal Foundation, San Diego, CA, United States
- 6Duke University Marine Laboratory, Nicholas School of the Environment and Pratt School of Engineering, Duke University, Beaufort, NC, United States
Two groups of common bottlenose dolphins (Tursiops truncatus) have been identified within St. George Sound, Florida, USA: high site-fidelity individuals (HSF) which are individuals sighted multiple times in the region (i.e., ≥2 months, ≥2 seasons, and ≥2 years), and low site-fidelity individuals (LSF), which are individuals sighted fewer than 2 months, in 2 different seasons among 2 different years. Our goal was to determine whether differences in foraging behaviors were correlated with differences in sighting frequency and overall usage of St. George Sound by the two groups. We used carbon, nitrogen, and sulfur stable isotopes and niche hypervolume metrics to model the foodweb of St. George Sound. Mixing model results indicated that croaker, mojarra, pigfish, pinfish, and silverperch were the most important prey items for dolphins. The hypervolume metrics demonstrate niche partitioning between HSFs and LSFs, with the HSFs relying more heavily on pinfish, pigfish, and mojarra, while the LSFs relied more on silverperch. Plankton, benthic diatoms, seagrass, and epiphytes all contributed to secondary production within St. George Sound. This diversity of source utilization by seagrass-associated consumers supported by a high rate of total production likely sustains high secondary productivity despite the potential for competition in this system. Zooplankton was the most important basal source to the system, followed by seagrass and benthic primary production (as indicated by a sanddollar proxy). The reliance of dolphins on seagrass-dependent prey indicates that alteration of seagrass habitat would significantly impact the dolphin community foraging in St. George Sound and suggests that preservation of seagrass habitat is an important component of an effective management strategy for dolphin populations in the region.
Introduction
Seasonal variation in bottlenose dolphin (Tursiops truncatus) abundance has been observed in multiple study regions along the northern Gulf of Mexico (GoM), including St. Joseph Bay, FL (Balmer et al., 2008), Charlotte Harbor, FL (Bassos-Hull et al., 2013), Mississippi Sound, MS (Hubard et al., 2004), Sarasota Bay, FL (Irvine et al., 1981), and St. George Sound, FL (Tyson et al., 2011). These variations have been attributed to prey movements, temperature, and/or potential responses to stressors such as harmful algal blooms (Irvine et al., 1981; Hubard et al., 2004; Balmer et al., 2008). While relatively stable cohorts of dolphins with long-term site-fidelity, termed resident populations (sighted across multiple seasons and years), have been identified in GoM regions such as Sarasota Bay (Scott et al., 1990; Wells, 2009, 2014) and St. Joseph Bay (Balmer et al., 2008), variations in dolphin abundances have been attributed to the influx and efflux of individuals that utilize these areas for only a short period of time and may or may not return to a region on a seasonal basis. Recently, similar variations in dolphin abundances have been inferred in St. George Sound, FL from differences in site fidelity observed among dolphins identified using photographic-identification (photo-ID) techniques (described in Tyson et al., 2011). However, in contrast to regions such as Sarasota Bay and St. Joseph Bay that have high rates of site fidelity within enclosed embayments, St. George Sound appears to support a highly transient population of dolphins, with 46% of dolphins sighted by Tyson et al. (2011) being seen only once during a photo-ID study conducted in the region from 2004 to 2008. Tyson et al. (2011) suggested this may be the result of St. George Sound offering relatively greater accessibility to the GoM than other bays and estuaries, such as Sarasota Bay and St. Joseph Bay, which are enclosed to a greater degree by barrier islands. These results are comparable to those observed by Hubard et al. (2004) in Mississippi Sound, which has a similar geography to St. George Sound (large open water embayment with barrier islands) and an extremely low dolphin resighting rate (catalog size, N = 515; mean sightings/dolphin = 1.57). Indeed, Tyson et al. (2011) found that only 28% of dolphins sighted in Apalachicola Bay, a protected waterway located just west of St. George Sound, were seen only once although the mean (± SD) sighting rate was similar to that in St. George Sound (2.93 ± 1.86 and 2.47 ± 1.96, respectively).
Stable isotopes can be a useful indicator of bottlenose dolphin foraging behavior (Rossman et al., 2013) and have proven useful in differentiating populations of bottlenose dolphins based on habitat types (e.g., Barros et al., 2010). They have also been successfully used to identify fine-scale spatial and seasonal variations in forage base among sympatric dolphin groups (e.g., Olin et al., 2012). Sympatric populations of bottlenose dolphins have been shown to demonstrate distinct foraging tactics and resource partitioning in regions such as the estuarine and coastal waters of North Carolina and South Carolina (Gannon and Waples, 2004; Olin et al., 2012), Florida Bay, Florida (Torres and Read, 2009), and Galicia, Spain (Fernández et al., 2011). We hypothesized that HSFs and LSFs would have different isotope values reflecting their different habitat utilization patterns, either from differences in prey species selection or because of differences in geographic home range. We have shown that the dolphins sampled within St. George Sound most closely reflect the isotope values of fish sampled within St. George Sound suggesting that these dolphins were foraging primarily within St. George Sound, in the immediate weeks before sampling (Wilson et al., 2013a). Thus, isotopic differences between HSF and LSF dolphins likely reflect differences in prey selection, rather than geographic variation in prey values.
The goal of this study was to examine whether differences in habitat use and foraging behavior exist between dolphins with varying levels of site fidelity in St. George Sound. Based on the relatively low resighting rates of individual dolphins observed by Tyson et al. (2011) compared with resident populations in other Florida estuaries (e.g., Sarasota Bay, St. Joseph Bay), and the definition of residency provided by Rosel et al. (2011) (i.e., individuals spending greater than 50% of their time in an estuary in a given year) we adopted the designation of high site fidelity individual (HSF) rather than “resident” in this paper to describe dolphins observed multiple times in the region (i.e., ≥2 months, ≥2 seasons, and ≥2 years). Individuals seen less frequently will be referred to as low site fidelity individuals (LSFs). We compared the isotope values of dolphin skin with those in fish muscle sampled within St. George Sound coupled with photo-ID sighting histories of the dolphins, to evaluate potential differences in the dietary compositions of HSF and LSF dolphins in St. George Sound. In addition to determining the dietary contributions of prey fish species to dolphin diets, we were also interested in determining the ultimate organic matter source(s) underlying the St. George Sound foodweb. Applying a Bayesian isotope mixing model (MixSIAR, Parnell et al., 2010) in a nested approach, we constructed a three-level foodweb describing the differences in foraging behaviors for the two sympatric dolphin groups and quantified the underlying organic matter sources supporting the St. George Sound foodweb. Using newly developed niche metrics we constructed niche hypervolumes to identify how resource use differs between dolphin types. This approach allowed us to identify the potential bottom-up influences of dolphin foraging in this system that will be useful for more effectively managing dolphin populations in the region.
Materials and Methods
Organic Matter Sources
We collected primary organic matter sources, including plankton, seagrass, seagrass epiphytes, and benthic microalgae, from St. George Sound (Figure 1), from April 2007 through July 2009. We adhere to the definition for epiphytes suggested by Zieman and Zieman (1989) as “any sessile organism growing on a plant,” although epiphytes are likely largely algal in nature. Seagrass samples were collected from May to September of 2007 and 2008, and were dominated by Syringodium sp. and Thalassia testudinum, but Halodule wrightii was also present in small quantities. We separated seagrass species and made composites of the samples from each day such that only one sample per species per collection date (spaced ~1 month apart during the growing season) was obtained. We include only live seagrass blades in our analyses (no sub-sediment material; i.e., rhizomes). We removed attached epiphytes by gently scraping the grass blades, then rinsing the seagrass blades with distilled water. We then acid-washed half of each seagrass sample with 10% HCl followed by a distilled water rinse to remove seawater carbonate. We rinsed the remaining half of each sample with distilled water to remove seawater sulfate contamination before δ34S analysis.
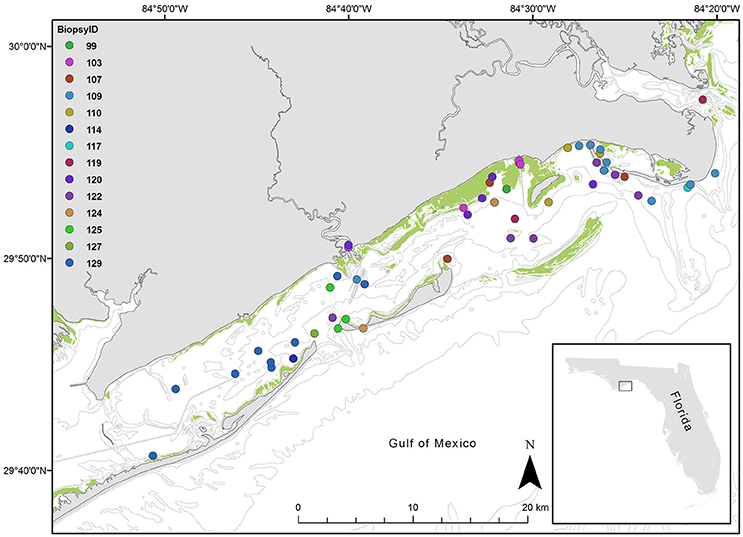
Figure 1. Map of study area, St. George Sound, Gulf of Mexico. Points represent each sighting location for dolphins sighted over the study period. Colors indicate dolphin ID as indicated in the legend. The inset map shows St. George Sound in the context of the state of Florida, USA. The green shading indicates known seagrass coverage.
We collected plankton samples bi-monthly from June through September 2008 and in June-July 2009 except when prevented by inclement weather. We used 64-μm plankton tows at the surface over visible seagrass beds for 5–15 min. We sampled only on days when wind speed was low (e.g., <10 knots) and in Beaufort Sea States of 2 or less to minimize sampling of resuspended bottom material. On average, we collected 7 tows on each sampling date. We combined all tows from a single day to make one composite plankton sample for that day. Upon returning to the lab, we placed tow contents under lights for up to 1 h to draw zooplankton to the top layer and to allow non-planktonic material to sink. We carefully extracted samples from the top and bottom layers using clean transfer pipets and filtered through 10-μm nylon mesh. We removed a small aliquot of each and examined it microscopically after fixation with Lugol's (iodine) solution. Top layer samples consisted primarily of zooplanktonic species, in most cases copepods, although we also observed amphipods in top layer samples. Bottom layer samples contained a mixture of copepods, phytoplankton (including Rhizosolenia sp.), as well as other unidentified materials (possibly detrital in nature). To reduce complications from sampling mixed components (i.e., zooplankton, phytoplankton, and detritus), we used only the top layer, predominantly zooplankton samples, in the analyses. We divided composite samples for each day into two subsamples, one for the carbon and nitrogen analysis and the remainder for the sulfur analysis. We acid-washed samples destined for the carbon and nitrogen analysis with a 10% HCl solution and sonicated them for 30 min. After sonication, we centrifuged the samples and decanted the acidic supernatant. We repeated this washing procedure for a total of three times for each sample. Although some researchers have suggested that acidification could alter δ15N values (Bunn et al., 1995; Logan et al., 2008), Chanton and Lewis (1999) found no differences in δ15N values of plankton samples subjected to acidification and those washed with a distilled water rinse. Thus, we were confident that acidification of the plankton samples did not alter δ15N results. We then washed all samples, including those destined for sulfur analysis, with distilled water and sonicated for 30 min, then centrifuged and removed the resulting supernatant to remove remaining acid or sulfate contamination, respectively. We repeated the distilled water washes three times.
We combined epiphytes to make a single epiphyte composite for each sampling date. We then divided each composite epiphyte sample into two aliquots, one destined for δ34S and one for δ13C/δ15N analysis. We acid-rinsed the aliquots destined for δ13C/δ15N analysis and then rinsed them with distilled water. We washed the aliquots destined for δ34S analysis with distilled water, sonicated, and then centrifuged them to remove seawater sulfate contamination. We used forceps to carefully remove seagrass and non-epiphytic material from each sample under a dissecting microscope following the distilled water rinses and drying.
Although benthic microalgae have been suggested as an important source driving secondary production in nearby habitats (Wilson et al., 2009a), attempts to collect benthic diatoms directly proved unsuccessful. This region is characterized by dynamic wave and tidal action which prevents the formation of benthic algal mats (Chanton and Lewis, 2002). Further, sediments at our site consist of small grain size mud which inhibits the migration of benthic diatoms (Markus Huettel pers comm. 2009). Because we were unable to obtain pure benthic microflora samples, we used sand dollars (Mellita quinquiesperforata) as an isotopic proxy for benthic microalgae following the suggestion of Moncreiff and Sullivan (2001). We collected sand dollars from sandy areas at the seagrass bed margin in less than 3 m water depth. We rinsed each sand dollar thoroughly upon returning to the lab with distilled water. We only analyzed sand dollars larger than 10 cm in diameter to ensure enough material was available for all isotopic analyses. We used forceps and a scalpel to carefully pry each sand dollar apart exposing the interior surfaces. We then rinsed the inner soft tissues with distilled water and carefully peeled the soft tissue from the carbonate structure. We analyzed each sand dollar independently. However, we divided each sample into two aliquots and placed them in separate 1.5 mL microcentrifuge tubes. We acid-washed the first aliquot, which was destined for δ13C/δ15N analysis, and rinsed to remove carbonate contamination. We rinsed the second aliquot, which was destined for sulfur isotope analysis, repeatedly with distilled water.
Consumers
We collected fish samples by otter trawl (2.5 cm mesh, 5 m long net) during April through November 2007–2009 directly over visible seagrass habitat where water depths were 1–3 m. We pulled tows at approximately 2 km h−1 for 5–15 min each. These tow collection times were dictated by drift algae abundance. Periods of high drift algae abundance (June-August) cause accumulation of significant mass in the tows and therefore tow periods had to be shortened to prevent equipment damage and permit researchers to safely retrieve trawls. Fortunately, this period also corresponds with high fish abundance in the seagrass (Nelson et al., 2011) such that we typically caught large volumes of fish despite the shorter tow times.
The fish species we collected included: pinfish (Lagodon rhomboides), spot (Leiostomus xanthurus), silver perch (Bairdiella chrysoura), flounder (Paralichthys sp.), mojarra (Eucinostomus gula), black seabass (Centropristis striata), lane snapper (Lutjanus synagris), Atlantic croaker (Micropogonias undulatus), lizardfish (Synodus foetens), pigfish (Orthopristis chrysoptera), and spotted seatrout (Cynoscion arenarius). We recorded fork lengths for each fish collected before placing on ice for transport back to shore (Table 1). Upon return to the lab, we removed fish muscle tissue from bones and skin and then dried the muscle at 60°C for up to 48 h. We then ground the dried tissue to a fine powder using a SPEX Sampleprep mill and froze it at −20°C before isotopic analysis. Because of the large number of fish collected, we could not analyze the isotopes for all fish caught. If less than 8 individuals of any species were collected, then we analyzed all individuals. By far the dominant species in our tows were pinfish, pigfish, silver perch, and flounder. Of these species, we chose samples haphazardly for isotopic analysis.
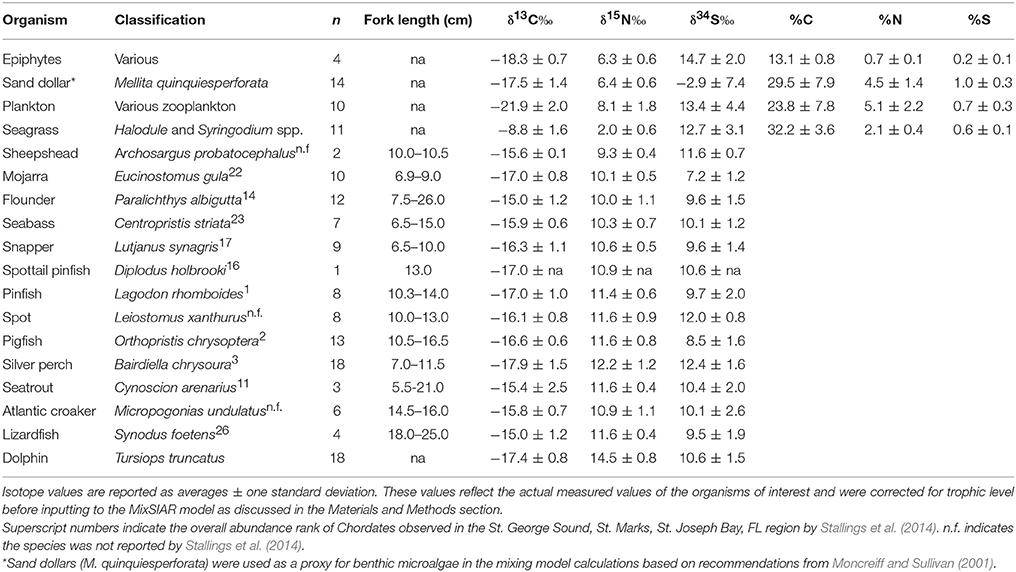
Table 1. Stable isotope abundances in St. George Sound producers and consumers examined in this study.
We obtained dolphin skin tissues in May 2004 via remote dart biopsy as reviewed in Sinclair et al. (2015) and prepared them for isotopic analysis as described by Wilson et al. (2012) (Figure 1). We sent an aliquot of the skin to the Southeast Fisheries Science Center (Lafayette, LA) for determination of sex by molecular techniques (Rosel and Block, 1996; Rosel, 2003). We also photographed dolphins at the time of biopsy and, when possible, matched them to the catalog of distinctive individuals according to the photo-ID methods described in Balmer et al. (2008) and Tyson et al. (2011). We collected dolphin samples during a single month (May 2004), thereby minimizing seasonal variations (e.g., Olin et al., 2012). Although fish and organic matter source samples were not collected concurrently with dolphin samples, annual and seasonal variation at the site is likely low given that investigations at the highly seasonal nearby estuary Apalachicola Bay have revealed little temporal variation in consumer isotope values (Chanton and Lewis, 2002). Thus we feel that any temporal variability will have a minimal impact on our isotopic interpretations. Although this assumption merits further investigation.
Isotopic Analysis
We obtained carbon (δ13C) and nitrogen (δ15N) isotope values using a Finnigan MAT Delta PLUS XP stable isotope ratio mass spectrometer (IRMS) at the National High Magnetic Field Laboratory in Tallahassee, Florida. We determined elemental concentrations concurrently using a Finnigan MAT TC/EA high-temperature conversion elemental analyzer interfaced to the IRMS. We sent samples to the Stable Isotope Core Laboratory, Washington State University for determination of sulfur stable isotope (δ34S) and sulfur elemental analyses. In addition to homogenous laboratory isotope standards, we analyzed (or sent for analysis in the case of sulfur samples) duplicate samples of select consumers, sand dollars, algae, seagrass, and plankton samples to determine homogeneity of the samples and consistency of results across runs (Jardine and Cunjak, 2005). Analytical error, measured as the standard deviation of replicate samples measured across all runs, was 0.2 and 3.0 for δ13C and elemental carbon percentage (respectively); 0.5 and 0.6 for δ15N and elemental nitrogen percentage; 0.8 and 0.2 for δ34S and elemental sulfur percentage.
Modeling the St. George sound foodweb
We analyzed all stable isotope data in R (v 3.3.2, R Development Core Team) using the packages MASS (v 7.3-47), MixSIAR (v 3.1.7, Stock et al., 2014) and hypervolume (v 1.4.1, Blonder et al., 2014). We first used a discriminant function analysis (DFA) to determine whether differences in sighting frequencies resulted in isotopic differences (MASS package in R). In this analysis, the characterization of individual dolphins as either LSF or HSF was used as the predictor for δ13C, δ15N, and δ34S withholding the four dolphins with unknown sighting histories. The jacknifed classification results on the training set found that the model correctly classified 79% of the dolphins with known sighting status to the “correct” sighting group based on the three isotope indicators. Therefore, we used the predict function to assign the four individuals without corresponding sighting frequencies to the LSF or HSF group. Given the DFA results, we chose to model these two dolphin groups separately in the subsequent foodweb modeling.
We ran MixSIAR models on the “very long” parameter setting to allow for adequate model convergence. Concentration-dependent percent contributions of each source were calculated for each individual. The sources in the first MixSIAR model run were the teleost fish species as these were the most likely to be directly consumed by the dolphins. We obtained trophic fractionation values incorporated in the model from literature consensus values. Carbon isotopes were corrected to reflect a +1.3‰ per trophic level shift (McCutchan et al., 2003), sulfur isotopes were corrected assuming a +0.5‰ per trophic level shift (McCutchan et al., 2003), and nitrogen isotopes were corrected by +3.4‰ per trophic level shift (Post, 2002). To correct for lipid content, we incorporated uncertainties of ±1.7‰ into the carbon isotope fractionation factors used to assess dolphin source contributions (Wilson et al., 2013b). Fish C/N ratios were less than 4 for all samples and, therefore, did not require lipid correction (following Post et al., 2007). Based on the results of this MixSIAR analysis, we excluded prey items that made up less than 10% of the diet contributions.
We used the hypervolume package (v 1.4.1, Blonder et al., 2014) to construct multi-dimensional hypervolumes, with fish prey contributions to the dolphins as axes, to understand the way each group's trophic niche changed based on their behavior pattern. All data were z-transformed before analysis to allow for standardized, comparable axes in n-dimensional space (Lamanna et al., 2014). Z-score values were calculated based on the following equation:
where xij is the individual value for a given axis, is the global mean of that axis, across both species and all complexity categories, and sj is the standard deviation of that axis. Hypervolumes were created by simulating 5,000,000 points for each behavior type/density bin subset based on the data of that subset. The bandwidth of each constructed hypervolume was estimated for relevant data using the Silverman estimator, as other bandwidth estimations are sensitive to high (≥4) dimensionality. The quantile threshold used was 0.05, so that each hypervolume included 95% of the total probability density. For this model run, we divided the dolphins (N = 14) into two sighting-based groups (i.e., HSFs and LSFs) based on observations made by Tyson et al. (2011) and the results of the DFA.
We used a second run of the MixSIAR model to elucidate the underlying sources of organic matter to the resultant important prey species. In this second model run, the fish now represented the mixtures and we used the MixSIAR model to estimate the contribution of various organic matter sources to the fish. The end-members we used in this model run were plankton, seagrass, epiphytes, and benthic microalgae (represented by sand dollars, Mellita quinquiesperforata as per Moncreiff and Sullivan, 2001). Carbon, nitrogen, and sulfur isotopes were used simultaneously to resolve source contributions and we elected to implement a concentration-correction. Isotope correction factors were taken from McCutchan et al. (2003), Post (2002), and Wilson et al. (2013b) and the isotope values of the zooplankton and sand dollar proxies were pre-corrected by one trophic level to account for differences in trophic level among the sources.
Results
Organic Matter Sources
The base of the food web (i.e., primary production) in St. George Sound (Figure 1) was represented by four groups: plankton (represented primarily by Acartia tonsa), benthic microalgae (represented by sand dollars, Mellita quinquiesperforata), seagrass (Thalassia testudinum, Syringodium spp., and Halodule wrightii), and epiphytic algae (Table 1). Zooplankton had the most 13C-depleted isotope abundances of any of the sources measured (δ13C = −21.9‰ ± 2.0, reported as mean ± one standard deviation here and elsewhere) (Figure 2). Nitrogen isotopes of zooplankton averaged 8.1‰ ± 1.8 and sulfur isotopes of zooplankton averaged 13.4‰ ± 4.4. Isotope abundances of T. testudinum and Syringodium seagrass species were similar (δ13C = −9.1‰ ± 0.3, −7.0‰ ± 0.7; δ15N = 1.8‰ ± 0.7, 2.1‰ ± 0.7; and δ34S = 13.5‰ ± 1.7, 14.2‰ ± 2.9 for the two species, respectively); however, while Halodule wrightii nitrogen isotope values were similar to other seagrass species measured, the carbon and sulfur isotope abundances were depleted (δ13C = −12.1‰, δ15N = 2.1‰, δ34S = 5.7‰) relative to T. testudinum and Syringodium spp. Because of the similarity in isotope abundances between T. testudinum and Syringodium and their greater abundance during collection relative to Halodule wrightii, we averaged isotope values for all seagrass samples to get overall composite seagrass isotope values. The generated seagrass composite had the most 13C-enriched values of any of the primary producers sampled (δ13C = −8.8‰ ± 1.6, δ15N = 2.0‰ ± 0.6, δ34S = 12.7‰ ± 3.1) (Figure 2). Sand dollars (our benthic microflora proxy) had moderate δ13C values (−17.5‰ ± 1.4) relative to other sources, but the lowest δ34S values observed (−2.9‰ ± 7.4). Isotope values for epiphytic algae were intermediate relative to the other sources sampled (δ13C = −18.3‰ ± 0.7, δ15N = 6.3‰ ± 0.6, δ34S = 14.7‰ ± 2.0) (Figure 2).
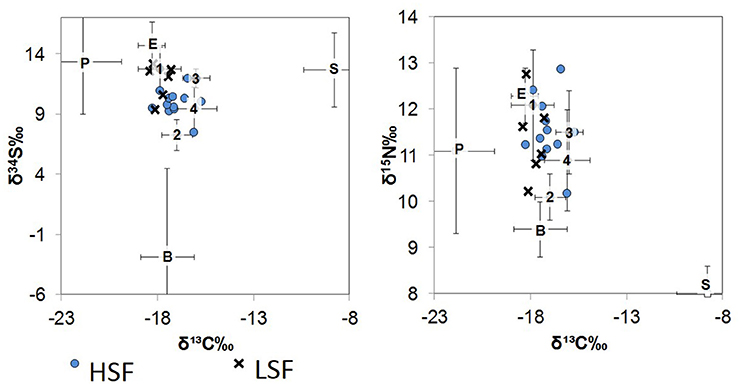
Figure 2. δ34S vs. δ13C (left) and δ15N vs. δ13C (right) biplots of the St. George Sound ecosystem. Numbers represent fish values plotted according to groups (average ± one standard deviation): Group 1 (1): silver perch, Group 2 (2): mojarra, Group 3 (3): spot, and Group 4 (4): pinfish, pigfish, seabass, flounder, lizardfish, croaker, and snapper. Organic matter source value means ± one standard deviation are also plotted as P, plankton; B, benthic; S, seagrass; E, epiphytes. Whiskers representing the standard deviation for source values are symmetrical about the mean, edges were cut-off to improve graph readability. Blue circles represent the HSF dolphin isotope values, while × 's represent the LSF dolphin isotope values.
Consumers
St. George Sound fish stable isotope values were well-constrained by the carbon and sulfur isotope values of plankton, benthic microalgae, seagrass, and associated epiphytes (Figure 2). Species-level isotope data for the fish and basal sources are presented in Table 1. Isotope results for the dolphin skin are presented in Table 2. The MixSIAR model for dolphin consumers quantified the four primary prey types consumed by dolphins. We then used the MixSIAR model to determine the primary production sources that supported these prey items and therefore the dolphin community. The four prey items were: silver perch, mojarra, croaker, and pinfish/pigfish. These species accounted for more than 80% of dolphin biomass based on the MixSIAR results with each group contributing >10% to both HFS and LSF groups. Dolphin isotope values were generally well-constrained by the four fish groups (Figure 2).
St. George sound foodweb
The hypervolume analysis revealed strong differences in the niche partitioning between HSF and LSF dolphins (Figure 3). Where HSF individuals were more reliant on mojarra, pigfish and pinfish. While LSF dolphins were more reliant on silver perch.
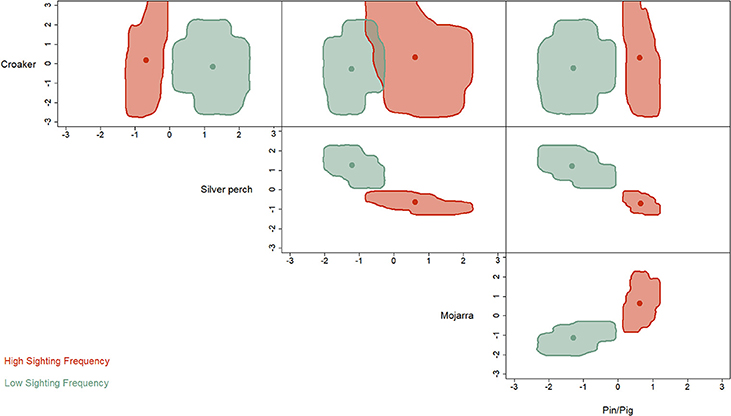
Figure 3. Niche hypervolume metrics indicate that HSF individuals (blue) feed more heavily on seagrass associated prey items (mojarra and pinfish/pigfish) than LSF dolphins who prefer silverperch.
MixSIAR model results were used to inform trophic linkages from primary producers through dolphins (Figure 4). Graphs of the proportional contribution of each source to each examined mixture output from MixSIAR are included in the schematics for the foodwebs leading up to each of the two dolphin groups. These graphs show the MixSIAR estimates of the contributions of each source to the corresponding consumer which were in agreement with the results of the hypervolume analysis.
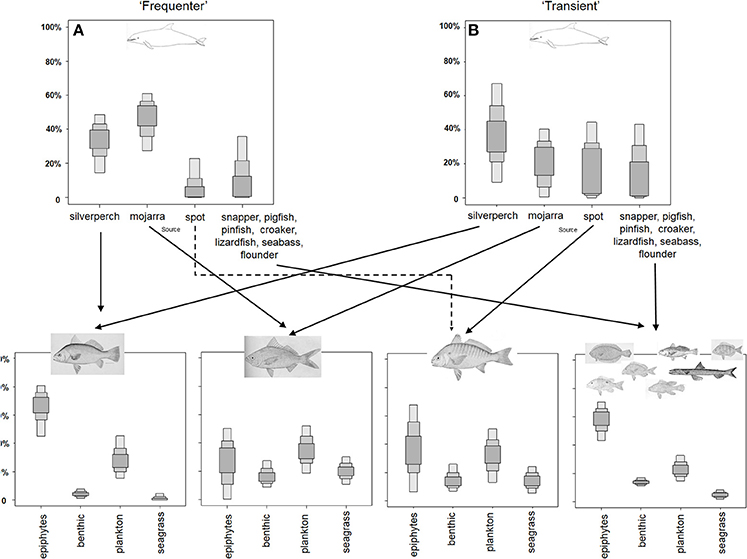
Figure 4. Diagram of the St. George Sound foodweb leading to HSF (A) and LSF (B) bottlenose dolphins based on the nested MixSIAR modeling results. MixSIAR modeling results of relative diet and source contributions were used to inform linkages between dolphins, their prey, and the ultimate primary producers that supported those prey species. Boxes outline the 25, 75, and 95% credibility intervals of relative contributions. Fish images incorporated into this figure were obtained from WikiCommons and the Freshwater and Marine Image Bank and are in the public domain.
Dolphins designated as HSFs of St. George Sound received the highest contributions from mojarra and silver perch in the MixSIAR model. Similarly, the contributions of spot to HSF dolphin diets was low or zero (Figure 4A). The LSF dolphins, had higher contributions of silver perch than of other species (Figure 4B). The remaining three groups made low, fairly uniform contributions to LSF dolphin diets. Silver perch were heavily dependent on planktonic production, while seagrass and benthic production did not contribute to silver perch diets (Figure 4). Similarly, group 4 fishes were heavily dependent on seagrass and plankton; however, benthic sources made a small, but non-zero contribution. Seagrass contributions to this group were small or absent. Both mojarra and spot had higher contributions of seagrass and benthic production (Figure 4), each contributing about 15% of their diets. Fish contributions to dolphins likely reflect direct linkages as dolphins directly consume fish prey. However, linkages between fish and primary production sources are of uncertain length. Fish could be directly consuming sources (particularly epiphytes and zooplankton), but could also be one or more trophic steps removed from primary production sources (e.g., consuming benthic invertebrates or smaller fish that are themselves consuming benthic diatoms).
Discussion
Sighting Differences
Dolphins are typically considered generalist predators whose diets are influenced primarily by locally abundant prey species (Shane et al., 1986; Vollmer and Rosel, 2013). However, differences in the niche hypervolumes between HSFs and LSFs (Figure 3) do not support this conclusion for dolphins in St. George Sound. We divided the St. George Sound dolphins a priori into two groups: HSFs and LSFs based on photo-ID sighting histories from Tyson et al. (2011) for all remote biopsy sampled dolphins. Despite the small number of biopsies (n = 18), discriminate function analysis correctly assigned 14 of these individuals to these corresponding sighting groups based on differences in their stable isotope content (Table 2). The hypervolume metrics based on the stable isotope analysis further demonstrates niche partitioning between the HSF and LSF groups. Because stable isotope ratios reflect dietary inputs, this result suggests that, in addition to differences in site fidelity, these two dolphin groups also differ in foraging habits. Isotopic differences among groups could result from foraging in different areas with differing source isotopes (e.g., Olin et al., 2012) from different prey species selection (e.g., Barros et al., 2010), or some combination of these two factors. Previous isotopic analyses have demonstrated that the isotope values of these dolphins were significantly different from fish in nearby bays and reefs outside of St. George Sound (Wilson et al., 2013b). These results were consistent with the hypothesis that both HSFs and LSFs were foraging primarily within the sound during the immediate weeks before sampling (Wilson et al., 2013a) as dolphin skin turns over on the order of about 70 days (Hicks et al., 1985) and some studies suggest that isotopic turnover in dolphin skin may be on the order of 10–20 days (Browning et al., 2014). Thus, the isotopic differences between these two groups were not the result of foraging in different areas, but more likely arose from differences in prey species selection. Such instances of resource partitioning among sympatric groups of cetaceans (Parra, 2006; Friedlaender et al., 2009; Gross et al., 2009; Kiszka et al., 2011) and even between groups of dolphins (Torres and Read, 2009; Fernández et al., 2011; Olin et al., 2012) are relatively common and may provide a mechanism to limit competition in habitats or periods of low prey abundance (Torres and Read, 2009).
Pinfish are the numerically dominant species of fish in St. George Sound, more abundant by an order of magnitude than the second and third most common species, pigfish and silver perch, respectively (Stallings and Koenig, 2011). However, pinfish and pigfish were not the greatest contributor to the diets of either HSF or LSF dolphins (Figure 4). Instead, silver perch, the third most abundant species in St. George Sound (Stallings and Koenig, 2011), were a large contributor in both of these groups, and HSFs actually received the greatest contributions from mojarra which make up less than 1% of the teleost population in the St. George Sound seagrass (Stallings and Koenig, 2011). Recently, it has been shown that Sarasota Bay dolphins may actively select soniferous prey and avoid non-soniferous species (Barros and Wells, 1998; Berens McCabe et al., 2010). Dolphins may passively listen for fish vocalizations to initially detect prey species and employ active echolocation only after potential prey has already been detected through passive means (Gannon et al., 2005). This strategy may reduce the energetic expenditure associated with echolocation or lower the possibility of echolocation signals alerting prey to the dolphins' presence (Gannon et al., 2005). Silver perch, along with toadfish (Opsanus beta), were the two most frequently identified soniferous fish species during acoustic surveys conducted concurrent with the collection of these dolphin samples (Rycyk, 2007). Thus it is possible that silver perch are more easily detected by passive listening strategies than more abundant species such as pinfish, therefore accounting for their disproportionate contribution (relative to their numerical abundance) to dolphin diets in St. George Sound.
Sonifery does not fully account for the differences however, because pigfish are also soniferous and thus should be better represented in dolphin diets if passive listening is the only control on prey selection in HSF diets. Although, besides being soniferous, silver perch in the Florida Big Bend region seem to prefer shallow waters with low seagrass cover, while pigfish were more abundant in shallow sites with higher seagrass cover (Stallings and Koenig, 2011). Thus, if seagrass is providing an acoustic refuge (sensu Wilson et al., 2013) this could explain the higher abundance of silver perch relative to pigfish in dolphin diets in the region. However, mojarra, the largest contributor to HSF diets, are not generally considered to be spontaneously soniferous at all (Fish and Mowbray, 1970; Berens McCabe et al., 2010). In fact, Gerreids, the family of mojarra, were actively avoided by dolphins in Sarasota Bay, potentially because they are not easily detected by passive listening (Berens McCabe et al., 2010). Thus, the high contribution of mojarra to HSF diets in St. George Sound is surprising. It is possible that mojarra have similar isotope values to some species that were not caught in our tows.
On the other hand, some studies have suggested mojarra may contribute to bottlenose dolphin diets (Barros and Odell, 1990; Shane, 1990), and mojarra may be an energetically attractive prey item because they have about 50% more lipid by weight than either pinfish or pigfish (Juanes et al., 2013); they would provide an incentive for individuals to specialize in foraging strategies that successfully capitalize on energy dense mojarra (e.g., Spitz et al., 2010). It is often assumed that dolphins feed primarily in seagrass beds where fish are most abundant. However, recent studies suggest that seagrass may actually attenuate echolocation and fish vocalizations by scattering sound energy, creating an acoustic refuge (Wilson et al., 2013) which inhibits both active and passive means of acoustically detecting prey. In areas where seagrass is abundant, dolphins may prefer to forage either outside or on the edge of seagrass beds (Allen et al., 2001; Nowacek, 2005) where acoustic detection is more efficient. Mojarra occur more frequently over areas with sparser seagrass cover (Randall, 1967) possibly making them easier to detect (either visually or via active echolocation) than more abundant species acoustically “hidden” within the seagrass. In Florida Bay, sympatric subpopulations of dolphins frequent different habitat types where they can most effectively utilize the different foraging tactics they have developed (Torres and Read, 2009). Thus, HSFs that spend a large proportion of their time in St. George Sound may know where mojarra like to congregate or may otherwise be able to capture mojarra more efficiently than LSFs. While LSF dolphins, who are less familiar with St. George Sound and may not have adapted strategies to feed in acoustically efficient areas, may find the spontaneously acoustically-active silver perch easier to detect and capture.
Up until now we have focused on foraging theory explanations for the observed dietary differences; however, the differences could instead stem from behavioral or social differences. Bottlenose dolphins employ many different foraging strategies of which many are learned behaviors. Because of the remote nature of the biopsy sampling, age could not be determined exactly, however, groups with calves were actively avoided during biopsy collection so that animals included in this study were likely receiving the bulk of their diet from foraging. Dolphins may feed alone or in groups, during day or night (Barros and Wells, 1998). Some appear to have very small preferred foraging areas near structure (e.g., a particular bridge) while others have been observed to forage over wide home ranges (Barros and Wells, 1998). Such specialized foraging techniques may be based on individual learning and experience (Berens McCabe et al., 2010) and therefore diets within a population may vary substantially. Some learned foraging behaviors appear to be passed from mother to offspring and allow dolphins to specialize on a particular prey item that might otherwise appear to be undesirable. For example, while most fish prey are swallowed whole, dolphins have been known to prey on catfish by severing the head, swallowing just the body, and discarding the noxious barb along with the head (Gunter, 1942; Ronje et al., 2017). In this study we cannot evaluate to what extent such learned behavior might have contributed to dolphin prey choice, but it is conceivable that dolphins from two different populations may have developed different feeding strategies and preferences based on previous experience and group knowledge.
More research is warranted to determine if HSFs and LSFs employ acoustically different foraging strategies, forage more frequently at the edge of seagrass areas, or exhibit different foraging strategies and whether such behavioral differences are correlated with prey choice.
Organic Matter Sources
The MixSIAR model was also used to evaluate potential organic matter sources (plankton, benthic production, epiphytes, and seagrass) to the fish occupying St. George Sound. The relative contributions of these sources varied among the four fish groups, but plankton was the major contributor to many of the St. George Sound consumers, including silver perch, which, in turn, was an important prey species for both groups of dolphins (Figure 3). Epiphytes are themselves only transiently available, most abundant during the warmer months of the year when seagrass coverage is greatest and nearly absent during the winter months at this latitude (Virnstein and Carbonara, 1985; Iverson and Bittaker, 1986; Fourqurean et al., 2001). Similarly, many of the teleost species that inhabit the seagrass during the warm summer months undergo an annual egress (Nelson et al., 2011, 2013). Larval stages of many species settle in the seagrass bed early in the year (Nelson, 1998), grow to maturity during the highly productive summer months, and then move offshore to spawn, most never returning, instead being consumed by offshore species (Nelson et al., 2011, 2013). In this way, the seagrass community experiences a nearly complete turnover every year, with more than 90% of individual fish found in the seagrass being young-of-the-year (Nelson, 2002; Stallings and Koenig, 2011). While HSF dolphins appear to be taking advantage of the high productivity in this system throughout most of the year, LSFs may be capitalizing on the boom-and-bust cycle of seagrass and epiphyte production by moving into the bay when productivity is high. We speculate that the LSF dolphins may follow the egress of fish offshore in the late fall/early winter or may travel to distant sites that we have yet to identify.
Although the relative contributions vary among species and perhaps even individuals, the distribution of fish isotope values and modeling results suggest that seagrass (both directly through blades and indirectly as a substrate for epiphyte growth), is a necessary contributing source to at least some of the St. George Sound consumers (Figures 2, 3), highlighting the importance of seagrass as an organic matter source itself and not just as physical shelter or a substrate for epiphyte growth. Earlier studies have observed no significant change in carbon or sulfur stable isotope composition during seagrass blade decomposition (Thayer et al., 1978; Schwinghamer et al., 1983; Chanton, 1997), thus it was not possible to distinguish between grazing on live seagrass material and detrital seagrass contributions in this study. However, our isotope results suggest that seagrasses are an important component either directly or indirectly to many of the abundant fish species occupying the St. George Sound seagrass bed.
Many seagrass species experience ontogenetic shifts in diet that could complicate our isotopic analysis (e.g., Stoner, 1980), however, we did not find significant correlations between isotope values and length in the fish we sampled. This is similar to results reported at other sites (Wilson et al., 2009b; Olin et al., 2011) and may be due to the small sample sizes (Galvan et al., 2010) or the range of fish lengths that are sampled during trawling efforts which do not capture the smallest size classes where ontogenetic dietary changes may be most dramatic (Wilson et al., 2009b). Since dolphins likely prefer prey >7 cm long (Barros and Odell, 1990; Barros and Wells, 1998), any such ontogenetic diet changes associated with post-larval settling in prey species are likely to have a minimal impact to our findings.
Adams (1976) suggested that diversity of source utilization and specialization into feeding niches (i.e., trophic guilds) explained the high productivity (in terms of fish biomass) of eelgrass communities. Likewise, isotopic comparisons suggest that a diversity of sources is utilized by consumers in the St. George Sound seagrass community (Figure 2). St. George Sound consumer isotope values were consistent with contributions from all four sources (surface plankton, epiphytes, seagrass, and benthic microalgae; Figure 2). Diversity of source utilization could explain how so many highly abundant species are able to coexist within St. George Sound. We suggest that the plurality of available sources result in a high rate of total production to sustain the diverse and abundant foodweb in St. George Sound despite the potential for competition.
St. George Sound is located in the Big Bed region of Florida which is home to the second largest contiguous seagrass meadow in Florida and subsequently one of the largest seagrass meadows in the world (Zieman and Zieman, 1989; Hale et al., 2004; Stallings and Koenig, 2011). Though it also remains one of the least disturbed seagrass meadows in the world (Stallings et al., 2015), the seagrass meadow in this region is considered “poor and declining” by Florida's Wildlife Legacy Initiative's Comprehensive Wildlife Conservation Strategy in part because increased nutrient loading has led to declining light levels and subsequent seagrass retreat from deeper waters (Hale et al., 2004). The dependence of the St. George Sound community on seagrass and associated epiphytes suggests that alteration of that seagrass habitat could have catastrophic consequences for this ecosystem (Zieman and Zieman, 1989). Such effects are likely far-reaching as it has been demonstrated that these fish also subsidize an important offshore grouper fishery via the annual egress (Nelson et al., 2011). It is estimated that this region supports an annual cohort of approximately 1.5 billion pinfish (Stallings and Koenig, 2011), which move each fall to offshore reefs where nearly all of them are consumed, supplying an estimated 18-25% of the nitrogen needs of offshore grouper species (Nelson et al., 2013). In addition to subsidizing offshore reef fish, fish species associated with seagrass habitats likely make up the bulk of nearshore dolphin diets (Berens McCabe et al., 2010). While dolphins may preferentially forage at the edge of seagrass habitats (Allen et al., 2001; Wilson et al., 2013), our results demonstrate that the diet of all dolphins occupying St. George Sound is ultimately tied to seagrass and associated epiphyte production (see also Nelson et al., 2013; Wilson et al., 2013). The identification of this link is important for assessing the potential impacts of ecosystem change in this region. The reliance of dolphins on seagrass-dependent prey suggests that alteration of seagrass habitat would significantly impact the dolphin community foraging in St. George Sound.
Ethics Statement
This study was carried out in accordance with the recommendations of Marc Freeman and Kathleen Harper, D.V.M. of the Florida State University Animal Care and Use Committee. The protocol was approved by the Florida State University Animal Care and Use Committee. Dolphin dart biopsies and sightings were collected under NMFS General Authorization No. 1055-1732.
Author Contributions
DN, RT, BB conceived, designed, and carried out the sighting surveys, analyzed, and interpreted the sighting data. JC, RW, and JN collected fish, conceived, and carried out the isotopic analyses. RW and JN interpreted isotopic data and conducted the modeling and statistical analyses. All authors contributed to the drafting of the current manuscript.
Funding
Funding for this project was provided by the Florida State University Department of Oceanography and Harbor Branch Protect Wild Dolphins Project (Grants 2004-06 and 2005-12), by the National Oceanic and Atmospheric Administration via the Northern Gulf Institute, by the BP/Gulf of Mexico Research Initiative Deep-C administered by Florida State University, and by the Florida State University Coastal and Marine Laboratory. Data were collected under NMFS General Authorization No. 1055-1732.
Conflict of Interest Statement
The authors declare that the research was conducted in the absence of any commercial or financial relationships that could be construed as a potential conflict of interest.
Acknowledgments
Thank you to E. J. Berens McCabe for providing thoughtful insights on an earlier draft of this manuscript and to 3 reviewers who provided insightful comments. Special thanks to Y. Xu, National High Magnetic Field Laboratory, Tallahassee, Florida and B. Harlow, Washington State University for analytical assistance. Fish samples were collected via a grant from the Florida State University Coastal and Marine Laboratory.
References
Adams, S. M. (1976). Feeding ecology of eelgrass fish communities. Trans. Am. Fish. Soc. 105, 514–519. doi: 10.1577/1548-8659(1976)105<514:FEOEFC>2.0.CO;2
Allen, M., Read, A., Gaudet, J., and Sayigh, L. (2001). Fine-scale habitat selection of foraging bottlenose dolphins Tursiops truncatus near Clearwater, Florida. Mar. Ecol. Prog. Ser. 222, 253–264. doi: 10.3354/meps222253
Balmer, B. C., Wells, R. S., Nowacek, S., Nowacek, D. P., Schwacke, L. H., McLellan, W. A., et al. (2008). Seasonal abundance and distribution patterns of common bottlenose dolphins (Tursiops truncatus) near St. Joseph Bay, Florida, USA. J. Cetacean Res. Manag. 10, 157–167.
Barros, N. B., and Odell, D. K. (1990). “Food habits of bottlenose dolphins in the southeastern United States,” in The Bottlenose Dolphin, eds S. Leatherwood and R. Reeves (San Diego, CA: Academic Press), 309–328.
Barros, N. B., and Wells, R. S. (1998). Prey and feeding patterns of resident bottlenose dolphins (Tursiops truncatus) in Sarasota Bay, Florida. J. Mammal. 79, 1045–1059. doi: 10.2307/1383114
Barros, N. B., Ostrom, P. H., Stricker, C. A., and Wells, R. S. (2010). Stable isotopes differentiate bottlenose dolphins off west-central Florida. Mar. Mammal. Sci. 26, 324–336. doi: 10.1111/j.1748-7692.2009.00315.x
Bassos-Hull, K., Perrtree, R. M., Shepard, C. C., Schilling, S., Barleycorn, A. A., Allen, J. B., et al. (2013). Long-term site fidelity and seasonal abundance estimates of common bottlenose dolphins (Tursiops truncatus) along the southwest coast of Florida and responses to natural pertubations. J. Cetacean Res. Manage 13, 19–30.
Berens McCabe, E. J., Gannon, D. P., Barros, N. B., and Wells, R. S. (2010). Prey selection by resident common bottlenose dolphins (Tursiops truncatus) in Sarasota Bay, Florida. Mar. Biol. 157, 931–942. doi: 10.1007/s00227-009-1371-2
Blonder, B., Lamanna, C., Violle, C., and Enquist, B. J. (2014). The n-dimensional hypervolume. Global Ecol. Biogeogr. 23, 595–609. doi: 10.1111/geb.12146
Browning, N. E., Dold, C., I-Fan, J., and Worthy, G. A. J. (2014). Isotope turnover rates and diet-tissue discrimination in skin of ex situ bottlenose dolphins (Tursiops truncatus). J. Exp. Biol. 217, 214–221. doi: 10.1242/jeb.093963
Bunn, S., Loneragan, N., and Kempster, M. (1995). Effects of acid washing on stable isotope ratios of C and N in penaeid shrimp and seagrass: implications for food-web studies using multiple stable isotopes. Limnol. Oceanogr. 40, 622–625. doi: 10.4319/lo.1995.40.3.0622
Chanton, J. (1997). Examination of the Coupling between Primary and Secondary Production in the Apalachicola River and Bay. Draft report to the ACF/ACT Comprehensive Study. Northwest Florida Water Management District, Tallahassee, FL.
Chanton, J. P., and Lewis, F. G. (1999). Plankton and dissolved inorganic carbon isotopic composition in a river-dominated estuary: Apalachicola Bay, Florida. Estuaries 22:575. doi: 10.2307/1353045
Chanton, J., and Lewis, F. G. (2002). Examination of coupling between primary and secondary production in a river-dominate estuary: Apalachicola Bay, Florida, U. S. A. Limnol. Oceanogr. 47, 683–697. doi: 10.4319/lo.2002.47.3.0683
Fernández, R., García-Tiscar, S., Begoña Santos, M., López, A., Martínez-Cedeira, J. A., Newton, J., et al. (2011). Stable isotope analysis in two sympatric populations of bottlenose dolphins, Tursiops truncatus: evidence of resource partitioning? Mar. Biol. 158, 1043–1055. doi: 10.1007/s00227-011-1629-3
Fish, M. P., and Mowbray, W. H. (1970). Sounds of Western North Atlantic Fishes: a Reference File of Biological Underwater Sounds. Baltimore, MD: Johns Hopkins Press.
Fourqurean, J. W., Willsie, A., Rose, C. D., and Rutten, L. M. (2001). Spatial and temporal pattern in seagrass community composition and productivity in south Florida. Mar. Biol. 138, 341–354. doi: 10.1007/s002270000448
Friedlaender, A. S., Lawson, G. L., and Halpin, P. N. (2009). Evidence of resource partitioning between humpback and minke whales around the western Antarctic Peninsula. Mar. Mammal Sci. 25, 402–415. doi: 10.1111/j.1748-7692.2008.00263.x
Galvan, D., Sweeting, C., and Reid, W. (2010). Power of stable isotope techniques to detect size-based feeding marine fishes. Mar. Ecol. Prog. Ser. 407, 271–278. doi: 10.3354/meps08528
Gannon, D. P., Barros, N. B., Nowacek, D. P., Read, A. J., Waples, D. M., and Wells, R. S. (2005). Prey detection by bottlenose dolphins, Tursiops truncatus: an experimental test of the passive listening hypothesis. Anim. Behav. 69, 709–720. doi: 10.1016/j.anbehav.2004.06.020
Gannon, D. P., and Waples, D. M. (2004). Diets of coastal bottlenose dolphins from the mid-Atlantic coast differ by habitat. Mar. Mammal Sci. 20, 527–545. doi: 10.1111/j.1748-7692.2004.tb01177.x
Gross, A., Kiszka, J., Van Canneyt, O., Richard, P., and Ridoux, V. (2009). A preliminary study of habitat and resource partitioning among co-occurring tropical dolphins around Mayotte, southwest Indian Ocean. Estuar. Coast. Shelf Sci. 84, 367–374. doi: 10.1016/j.ecss.2009.05.017
Gunter, G. (1942). Contributions to the natural history of the bottle-nose dolphin, Tursiops truncatus (Montague), on the Texas coast, with particular reference to food habits. J. Mammal. 23, 267–276. doi: 10.2307/1374993
Hale, J., Fraser, T., Tomasko, D., and Hall, M. (2004). Changes in the distribution of seagrass species along Florida's central gulf coast: iverson and Bittaker revisited. Estuaries Coasts 27, 36–43. doi: 10.1007/BF02803558
Hicks, B. D., Aubin, D. J., Geraci, J. R., and Brown, W. R. (1985). Epidermal growth in the bottlenose dolphin, Tursiops truncatus. J. Invest. Dermatol. 85, 60–63. doi: 10.1111/1523-1747.ep12275348
Hubard, C. W., Maze-Foley, K., Mullin, K. D., and Schroeder, W. W. (2004). Seasonal abundance and site fidelity of bottlnose dolphins (Tursiops truncatus) in Mississippi sound. Aquat. Mamm. 30, 299–310. doi: 10.1578/AM.30.2.2004.299
Irvine, A. B., Scott, M. D., Wells, R. S., and Kaufmann, J. H. (1981). Movements and activities of the Atlantic bottlenose dolphin, Tursiops truncatus, near Sarasota, Florida. Fish. Bull. 79, 671–688.
Iverson, R. L., and Bittaker, H. F. (1986). Seagrass distribution and abundance in Eastern Gulf of Mexico coastal waters. Estuar. Coast. Shelf Sci. 22, 577–602. doi: 10.1016/0272-7714(86)90015-6
Jardine, T. D., and Cunjak, R. A. (2005). Analytical error in stable isotope ecology. Oecologia 144, 528–533. doi: 10.1007/s00442-005-0013-8
Juanes, F., Clarke, P., and Murt, J. (2013). Fall and winter estuarine recruitment of bluefish Pomatomus saltatrix: selectivity for large lipid-rich prey increases depleted energy levels. Mar. Ecol. Prog. Ser. 492, 235–252. doi: 10.3354/meps10471
Kiszka, J., Simon-Bouhet, B., Martinez, L., Pusineri, C., Richard, P., and Ridoux, V. (2011). Ecological niche segregation within a community of sympatric dolphins around a tropical island. Mar. Ecol. Prog. Ser. 433, 273–288. doi: 10.3354/meps09165
Lamanna, C., Blonder, B., Violle, C., Kraft, N. J. B., Sandel, B., Šímová, I., et al. (2014). Functional trait space and the latitudinal diversity gradient. Proc. Natl. Acad. Sci. U.S.A. 111, 13745–13750. doi: 10.1073/pnas.1317722111
Logan, J. M., Jardine, T. D., Miller, T. J., Bunn, S. E., Cunjak, R. A., and Lutcavage, M. E. (2008). Lipid corrections in carbon and nitrogen stable isotope analyses: comparison of chemical extraction and modelling methods. J. Anim. Ecol. 77, 838–846. doi: 10.1111/j.1365-2656.2008.01394.x
McCutchan, J. H., Lewis, W. M., Kendall, C., and Mcgrath, C. C. (2003). Variation in trophic shift for stable isotope ratios of carbon, nitrogen, and sulfur. Syst. Ecol. 2, 378–390. doi: 10.1034/j.1600-0706.2003.12098.x
Moncreiff, C., and Sullivan, M. (2001). Trophic importance of epiphytic algae in subtropical seagrass beds: evidence from multiple stable isotope analyses. Mar. Ecol. Prog. Ser. 215, 93–106. doi: 10.3354/meps215093
Nelson, G. A. (2002). Age, growth, mortality and distribution of pinfish (Lagodon rhomboides) in Tampa Bay and adjacent Gulf of Mexico waters. Mar. Biol. 100, 582–592
Nelson, G. (1998). Abundance, growth, and mortalitiy of young-of-the-year pinfish, Lagodon rhomboides, in three estuaries along the gulf coast of Florida. Fish. Bull. 96, 315–328.
Nelson, J. A., Stallings, C. D., Landing, W. M., and Chanton, J. (2013). biomass transfer subsidizes nitrogen to offshore food webs. Ecosystems 16, 1130–1138. doi: 10.1007/s10021-013-9672-1
Nelson, J., Wilson, R., Coleman, F., Koenig, C., DeVries, D., Gardner, C., et al. (2011). Flux by fin: fish-mediated carbon and nutrient flux in the northeastern Gulf of Mexico. Mar. Biol. 159, 365–372. doi: 10.1007/s00227-011-1814-4
Nowacek, D. (2005). Acoustic ecology of foraging (Tursiops truncatus) habitat-specific use of three sound types. Mar. Mammal Sci. 21, 587–602. doi: 10.1111/j.1748-7692.2005.tb01253.x
Olin, J. A., Fair, P. A., Recks, M. A., Zolman, E., Adams, J., and Fisk, A. T. (2012). Unique seasonal forage bases within a local population of bottlenose dolphin (Tursiops truncatus). Mar. Mammal Sci. 28, E28–E40. doi: 10.1111/j.1748-7692.2011.00470.x
Olin, J. A., Rush, S. A., MacNeil, M. A., and Fisk, A. T. (2011). Isotopic ratios reveal mixed seasonal variation among fishes from two subtropical estuarine systems. Estuaries Coasts 35, 811–820. doi: 10.1007/s12237-011-9467-6
Parnell, A. C., Inger, R., Bearhop, S., and Jackson, A. L. (2010). Source partitioning using stable isotopes: coping with too much variation. PLoS ONE 5:e9672. doi: 10.1371/journal.pone.0009672
Parra, G. J. (2006). Resource partitioning in sympatric delphinids: space use and habitat preferences of Australian snubfin and Indo-Pacific humpback dolphins. J. Anim. Ecol. 75, 862–874. doi: 10.1111/j.1365-2656.2006.01104.x
Post, D. M. (2002). Using stable isotopes to estimate trophic position: models, methods, and assumptions. Ecology 83:703. doi: 10.1890/0012-9658(2002)083[0703:USITET]2.0.CO;2
Post, D. M., Layman, C. A., Arrington, D. A., Takimoto, G., Quattrochi, J., and Montaña, C. G. (2007). Getting to the fat of the matter: models, methods and assumptions for dealing with lipids in stable isotope analyses. Oecologia 152, 179–189. doi: 10.1007/s00442-006-0630-x
Randall, J. E. (1967). Food habits of reef fishes of the West Indies. Stud. Trop. Ocean. 5, 665–847.
Ronje, E. I., Barry, K. P., Sinclair, C., Grace, M. A., Barros, N., Allen, J., et al. (2017). A common bottlenose dolphin (Tursiops truncatus) prey handling technique for marine catfish (Ariidae) in the northern Gulf of Mexico. PLoS ONE 12:e0181179. doi: 10.1371/journal.pone.0181179
Rosel, P. E., Mullin, K. D., Garrison, L., Schwacke, L., Adams, J., Balmer, B., et al. (2011). Photo-Identification Capture-Mark-Recapture Techniques for Estimating Abundance of Bay, Sound and Estuary Populations of Bottlenose Dolphins along the US East Coast and Gulf of Mexico: A Workshop Report. NOAA Technical Memorandum NMFS-SEFSC 621, 30.
Rosel, P. (2003). PCR based sex determination in Odontocete cetaceans. Conserv. Genet. 4, 647–9. doi: 10.1023/A:1025666212967
Rosel, P., and Block, B. (1996). Mitochondrial control region variability and global population structure in the swordfish Xiphias gladius. Mar. Biol. 125, 11–22. doi: 10.1023/A:1025666212967
Rossman, S., Barros, N. B., Ostrom, P. H., Stricker, C. A., Hohn, A. A., Gandhi, H., et al. (2013). Retrospective analysis of bottlenose dolphin foraging: a legacy of anthropogenic ecosystem disturbance. Mar. Mammal Sci. 29, 705–718. doi: 10.1111/j.1748-7692.2012.00618.x
Rycyk, A. (2007). Acoustic Ecology of the Bottlenose Dolphin (Tursiops truncatus) in the Big Bend Region of Florida. Doctoral. dissertation, Florida State University.
Schwinghamer, P., Tan, F. C., and Gordon, D. C. Jr. (1983). Stable carbon isotope studies on the pecks cove mudflat ecosystem in the Cumberland Basin, Bay of Fundy. Can. J. Fish. Aquat. Sci. 40, s262–s272. doi: 10.1139/f83-288
Scott, M. D., Wells, R. S., and Irvine, B. (1990). “A long-term study of bottlenose dolphins on the west coast of Florida,” in The Bottlnose Dolphin, eds S. Leatherwood and R. R. Reeves (San Diego, CA: Academic Press), 235–244.
Shane, S. H. (1990). “Behavior and ecology of the bottlenose dolphin at Sanibel Island, Florida,” in The Bottlenose Dolphin, eds S. Leatherwood and R. R. Reeves (San Diego, CA: Academic Press), 245–226.
Shane, S. H., Wells, R. S., and Wursig, B. (1986). Ecology, behavior and social organization of the bottlenose dolphin: a review. Mar. Mammal Sci. 2, 34–63. doi: 10.1111/j.1748-7692.1986.tb00026.x
Sinclair, C., Sinclair, J., Zolman, E., Martinez, A., Balmer, B., and Barry, K. (2015). Remote Biopsy Sampling Field Procedures for Cetaceans Used during the Natural Resource Damage Assessment of the MSC252 Deepwater Horizon oil spill. NOAA Technical Memorandum NMFS-SEFSC-670, 36.
Spitz, J., Mourocq, E., Leauté, J.-P., Quéro, J.-C., and Ridoux, V. (2010). Prey selection by the common dolphin: fulfilling high energy requirements with high quality food. J. Exp. Mar. Biol. Ecol. 390, 73–77. doi: 10.1016/j.jembe.2010.05.010
Stallings, C. D., Brower, J. P., Loch, J. M. H., and Mickle, A. (2014). Commercial trawling in seagrass beds: bycatch and long-term trends in effort of a major shrimp fishery. Mar. Ecol. Prog. Ser. 513, 143–153. doi: 10.3354/meps10960
Stallings, C. D., Mickle, A., Nelson, J. A., McManus, M. G., and Koenig, C. C. (2015). Faunal communities and habitat characteristics of the Big Bend seagrass meadows, 2009–2010. Ecology 96:304. doi: 10.1890/14-1345.1
Stallings, C., and Koenig, C. (2011). Faunal communities of the Big Bend seagrass meadows. FWC State Wildl. Grants Initiative Final Report, 8007.
Stock, B., Semmens, B., Ward, E., Moore, J., Parnell, A., Jackson, A., et al. (2014). “MixSIAR: advanced stable isotope mixing models in R,” in Presented at Ecological Society of Amerca (Sacramento, CA).
Stoner, A. W. (1980). Feeding ecology of Lagodon rhomboides (Pisces: Sparidae): variation and functional responses. Fish. Bull. 78, 337–352.
Thayer, G. W., Parker, P. L., LaCroix, M. W., and Fry, B. (1978). The stable carbon isotope ratio of some components of an eelgrass, Zostera marina, bed. Oecologia 35, 1–12. doi: 10.1007/BF00345537
Torres, L. G., and Read, A. J. (2009). Where to catch a fish? The influence of foraging tactics on the ecology of bottlenose dolphins (Tursiops truncatus) in Florida Bay, Florida. Mar. Mammal Sci. 25, 797–815. doi: 10.1111/j.1748-7692.2009.00297.x
Tyson, R., Nowacek, S., and Nowacek, D. (2011). Community structure and abundance of bottlenose dolphins Tursiops truncatus in coastal waters of the northeast Gulf of Mexico. Mar. Ecol. Prog. Ser. 438, 253–265. doi: 10.3354/meps09292
Virnstein, R. W., and Carbonara, P. A. (1985). Seasonal abundance and distribution of drift algae and seagrasses in the mid-Indian river lagoon, Florida. Aquat. Bot. 23, 67–82. doi: 10.1016/0304-3770(85)90021-X
Vollmer, N. L., and Rosel, P. E. (2013). A Review of common bottlenose dolphins (Tursiops truncatus truncatus) in the Northern Gulf of Mexico: population biology, potential threats, and management. Southeast Nat. 13, 1–43. doi: 10.1656/058.012.m601
Wells, R. S. (2009). Learning from nature: Bottlenose dolphins care and husbandry. Zoo Biol. 28, 1–17. doi: 10.1002/zoo.20252
Wells, R. S. (2014). “Social structure and life history of common bottlenose dolphins near Sarasota Bay, Florida: insights from four decades and five generations,” in Primates and Cetaceans: Field Research and Conservation of Complex Mammalian Societies Primatology Monographs, eds J. Yamagiwa and L. Karczmarski (Toyko: Springer), 149–172.
Wilson, C., Wilson, P., Greene, C., and Dunton, K. (2013). Seagrass meadows provide an acoustic refuge for estuarine fish. Mar. Ecol. Prog. Ser. 472, 117–127. doi: 10.3354/meps10045
Wilson, R. M., Chanton, J. P., Balmer, B. C., and Nowacek, D. P. (2013b). An evaluation of lipid extraction techniques for interpretation of carbon and nitrogen isotope values in bottlenose dolphin (Tursiops truncatus) skin tissue. Mar. Mammal Sci. 30, 85–103. doi: 10.1111/mms.12018
Wilson, R. M., Chanton, J., Lewis, G., and Nowacek, D. (2009a). Combining organic matter source and relative trophic position determinations to explore trophic structure. Estuaries Coasts 32, 999–1010. doi: 10.1007/s12237-009-9183-7
Wilson, R. M., Chanton, J., Lewis, G., and Nowacek, D. (2009b). Isotopic variation (δ15N, δ13C, and δ34S) with body size in post-larval estuarine consumers. Estuar. Coast. Shelf Sci. 83, 307–312. doi: 10.1016/j.ecss.2009.04.006
Wilson, R. M., Kucklick, J. R., Balmer, B. C., Wells, R. S., Chanton, J. P., and Nowacek, D. P. (2012). Spatial distribution of bottlenose dolphins (Tursiops truncatus) inferred from stable isotopes and priority organic pollutants. Sci. Total Environ. 425, 223–230. doi: 10.1016/j.scitotenv.2012.02.030
Wilson, R. M., Nelson, J. A., Balmer, B. C., Nowacek, D. P., and Chanton, J. P. (2013a). Stable isotope variation in the northern Gulf of Mexico constrains bottlenose dolphin (Tursiops truncatus) foraging ranges. Mar. Biol. 160, 2967–2980. doi: 10.1007/s00227-013-2287-4
Keywords: stable isotope analysis, seagrass community, food web, bottlenose dolphin, mixing model
Citation: Wilson RM, Tyson RB, Nelson JA, Balmer BC, Chanton JP and Nowacek DP (2017) Niche Differentiation and Prey Selectivity among Common Bottlenose Dolphins (Tursiops truncatus) Sighted in St. George Sound, Gulf of Mexico. Front. Mar. Sci. 4:235. doi: 10.3389/fmars.2017.00235
Received: 15 May 2017; Accepted: 12 July 2017;
Published: 26 July 2017.
Edited by:
Luis Cardona, University of Barcelona, SpainReviewed by:
Leslie Cornick, Eastern Washington University, United StatesAmy C. Hirons, Nova Southeastern University, United States
Joan Gonzalvo, Tethys Research Institute, Italy
Copyright © 2017 Wilson, Tyson, Nelson, Balmer, Chanton and Nowacek. This is an open-access article distributed under the terms of the Creative Commons Attribution License (CC BY). The use, distribution or reproduction in other forums is permitted, provided the original author(s) or licensor are credited and that the original publication in this journal is cited, in accordance with accepted academic practice. No use, distribution or reproduction is permitted which does not comply with these terms.
*Correspondence: Rachel M. Wilson, rmwilson@fsu.edu