- 1Centre for Sustainable Tropical Fisheries and Aquaculture and College of Science and Engineering, James Cook University, Townsville, QLD, Australia
- 2AIMS@JCU, James Cook University, Townsville, QLD, Australia
- 3Australian Institute of Marine Science, Townsville, QLD, Australia
The increased frequency of publications concerning trophic ecology of coral reefs suggests a degree of interest in the role species and functional groups play in energy flow within these systems. Coral reef ecosystems are particularly complex, however, and assignment of trophic positions requires precise knowledge of mechanisms driving food webs and population dynamics. Competent analytical tools and empirical analysis are integral to defining ecosystem processes and avoiding misinterpretation of results. Here we examine the contribution of trophodynamics to informing ecological roles and understanding of coral reef ecology. Applied trophic studies of coral reefs were used to identify recent trends in methodology and analysis. Although research is increasing, clear definitions and scaling of studies is lacking. Trophodynamic studies will require more precise spatial and temporal data collection and analysis using multiple methods to fully explore the complex interactions within coral reef ecosystems.
Introduction
Understanding the biological organization and physical nature of an environment has enabled ecological research to play a central role in providing management and conservation advice for important ecosystems. While ecology comprises a wide array of components, food web structure, and trophic links are fundamental aspects of biological organization (Odum and Barrett, 1971; McIntosh, 1986) and encompass a large body of literature. Trophodynamics, “the dynamics of nutrition or metabolism,” was first proposed by Lindeman (1942) and is fundamental in understanding the flow of energy through food webs. Relationships within a community, energy flow, and linkages between biota and the environment are all encompassed in Lindeman's approach. The idea of energy flow in an ecosystem strengthened earlier studies such as biomass pyramids (Elton, 1927; Turney and Buddle, 2016), opening the way for incorporation of food webs into ecology to understand ecosystem processes (McIntosh, 1986; Sale, 2002). Definition of ecosystem processes is crucial to trophodynamic studies because they encompass biological, physical, and chemical mechanisms that link species and facilitate energy flow. These processes explain the contribution of decomposition, production, and nutrient cycling to ecosystem function (i.e., the way an ecosystem distributes energy) (Libralato et al., 2014). Although trophodynamics was not originally defined for marine ecosystems, researchers have applied this concept to marine food webs including coral reef ecosystems (Paine, 1966; Ryther, 1969). Contemporary trophodynamic analyses integrate ecosystem processes and food webs within a spatially and temporally explicit context to understand energy flow and trophic relationships in coral reefs. In a conservation and management context, trophodynamics can be used to predict the ecological effects of disturbances or fishing, and trophodynamic patterns are used as indicators for the state of coral reef systems.
Incorporating trophodynamics in marine studies is difficult due to the degree of uncertainty in describing interactions within highly complex marine food webs. Traditionally, marine food webs were thought to be resource-driven systems based on phytoplankton availability to lower-level consumers (Verity and Smetacek, 1996; Frank et al., 2007). For example, Odum and Kuenzler (1955) used trophodynamics to study coral reefs and identified energy pathways through both turf algae and fish to consumers, demonstrating that energy can be derived from both benthic and pelagic sources in a single system. Complex interactions among species also increase challenges in characterizing ecological functions within coral reefs (Huston, 1983; Pinnegar et al., 2000; Hubert et al., 2011). This complexity increases the difficulty in defining the trophic position of an individual or species within a food web (Choat and Bellwood, 1991; Choat et al., 2002; Frisch et al., 2014).
An additional complicating factor is the high level of spatial and temporal variation in coral reef systems, adding to the complexity of mapping ecological functionality on larger scales (Newman et al., 1997). Interpretation of these interactions can be difficult without long-term data (McIntosh, 1986; Sale, 2002) and are challenging to apply in conservation and management (Alva-Basurto and Arias-González, 2014). Physical and biological factors change over space and time in reef ecosystems (Sale, 2002). Therefore, even if trophic interactions have been well-described for a species and reef system in one location, the key processes that regulate ecosystem dynamics can be missed if the system is not observed over appropriate temporal (Scheffer et al., 2008) or spatial scales (Heymans et al., 2016). While quantitative analytical techniques have improved, complexity of trophic variability within populations, tissue turnover rates, and limited understanding of source pools (e.g., benthic vs. pelagic sources) can significantly affect interpretation of results (Layman, 2007; Layman et al., 2012). Furthermore, scaling issues limit the interpretability of trophodynamic data. Due to the complexity and variability of coral reef ecosystems, simplifications are often applied. For example, global databases (e.g., Fishbase; Froese and Pauly, 2000) may be used to source available data in lieu of extensive field collection to obtain site specific data (Bauman et al., 2010; Alva-Basurto and Arias-González, 2014; Ashworth et al., 2014; Ceccarelli et al., 2014; Aguilar-Medrano and Barber, 2016). However, information within these databases is often limited to specific regions. Similarly, reef fish feeding habits and trophic position can differ by population, so modeling over broad areas may not reflect interactions for an entire region unless spatial variation is understood and included (Michener et al., 2007).
Current trends in coral reef management highlight both biodiversity and biomass as indicators of reef health (Huston, 1983; Bellwood et al., 2004; Aguilar-Medrano and Calderon-Aguilera, 2016; Turney and Buddle, 2016). Documented declines in top predators and keystone species from anthropogenic disturbance (Dulvy et al., 2004; Sandin et al., 2008; Estes et al., 2011) have resulted in exploration of trophic cascades and assessment of ecological roles of predators in coral reef reefs (Heupel et al., 2014; Boaden and Kingsford, 2015; Rizzari et al., 2015; Weijerman et al., 2015; Thillainath et al., 2016). However, identifying trophic cascades is difficult in reef ecosystems. Only recently has there been evidence of a predator driven coral reef trophic cascade, but this was linked to tidal effects reducing predator occurrence rather than fishing effects (Rasher et al., 2017). Given the lack of examples of trophic cascades in coral reef habitats, it is necessary to ensure an appropriate framework is used to interpret data for these ecosystems. While applied research is a necessary step for improved conservation, misinterpretation of ecological roles can lead to poor conservation and management outcomes (Grubbs et al., 2016).
This review explores: (1) the variables most considered in trophodynamics studies, (2) critiques the adequacy of methods used, and (3) contemplates whether recent publications applied methods suitable to support prominent theories in coral reef ecology. We discuss how recent research on drivers of coral reef trophodynamics often do not account for spatial and temporal variation and methodological issues, and provide recommendations for future trophodynamic research.
Article Selection
Searches were performed through different bibliographic platforms to ensure access to the widest range of literature. Web of Science, Scopus, and Google Scholar were used to explore and extract available published material (Falagas et al., 2008). Google Books NGram Viewer (Lin et al., 2012), Scopus (Kähler, 2010), and SciVal (Colledge and Verlinde, 2014) metrics were used to analyze trends in keywords and publications to select articles for review (Table S1). Selection of publications was similar to Libralato et al. (2014) who showed increased frequency of key phrases such as “food chains,” “food webs,” and “trophic level” in publications since 1960 from Scopus and NGram searches. They established an historical timeline for the development of trophodynamics in research, but did not link keyword searches to coral reefs. For this review, 347 abstracts were chosen from 1942 to 2016 and filtered for keywords with highest relevance to trophodynamics in coral reef ecology. To evaluate recent trends in publications, additional searches were performed through Scival for “food web” and “trophic” relative to coral reefs to determine the top 50 keywords based on 291 publications from 2011 to 2016. Herbivores and predators were the two most common trophic groups studied (Table S2). Predators outnumbered herbivores in the literature in the past 5 years with up to 24 publications in 2016 (Table S2). For percent scholarly output, habitat, community structure, trophic level, and stable isotopes were among the top ranking keywords in publication growth.
Ecological Concepts, Trophodynamics, and Coral Reefs
The foundation of trophodynamics is the understanding of how food webs contribute to energy flow. In ecology, food web dynamics are typically based on a hierarchical, pyramid structure where organisms requiring more energy are less abundant than lower level consumers and producers (Libralato et al., 2014). This structure is seen across terrestrial and aquatic environments and is attributed to biomass scaling where resource availability limits the number of large-bodied organisms and higher trophic levels (Trebilco et al., 2013; Hatton et al., 2015). Coral reefs typically follow this classical food pyramid structure, however, due to complex food webs (Choat and Bellwood, 1985) and variability in habitat structure (Cox et al., 2000), it has been difficult to define generalized ecological relationships.
Identification of pathways linking sources of nutrition to consumers is essential for understanding ecological relationships in food webs (Table 1). Low-level organisms in a food web, known as producers (such as phytoplankton), provide energy to higher levels through consumption and assimilation. Large-bodied organisms typically hold higher positions in food pyramids as their energetic requirements require consumption of lower level producers and consumers (Lindeman, 1942). In biochemical ocean cycling, production occurs through: (1) fixing inorganic source pools of dissolved gases from nitrogen (i.e., nitrates, ammonia), carbon (CO2), and other essential elements (sulfur and hydrogen), or (2) particulate organic food uptake from nitrogen substrate, detritus (marine snow), and carbon into biological cycles (Michener et al., 2007). These sources of primary production are considered food-web baselines in trophic ecology and their availability is largely dependent on environmental and hydrodynamic variables unique to a region (Paulay, 1997). Producers are the origins of bottom-up forcing which influence resource limitation and carrying capacity of higher trophic levels (Terborgh, 2015). Biogeographic differences in reef resource availability are explained by factors such as: latitudinal and longitudinal gradients (Harmelin-Vivien, 2002), distance from human disturbance, position on the continental shelf, degrees of isolation, and oceanographic variables such as sea surface temperature, upwelling, and currents (Paulay, 1997). How each of these components affect food web production and resource availability should be considered in trophodynamic studies.
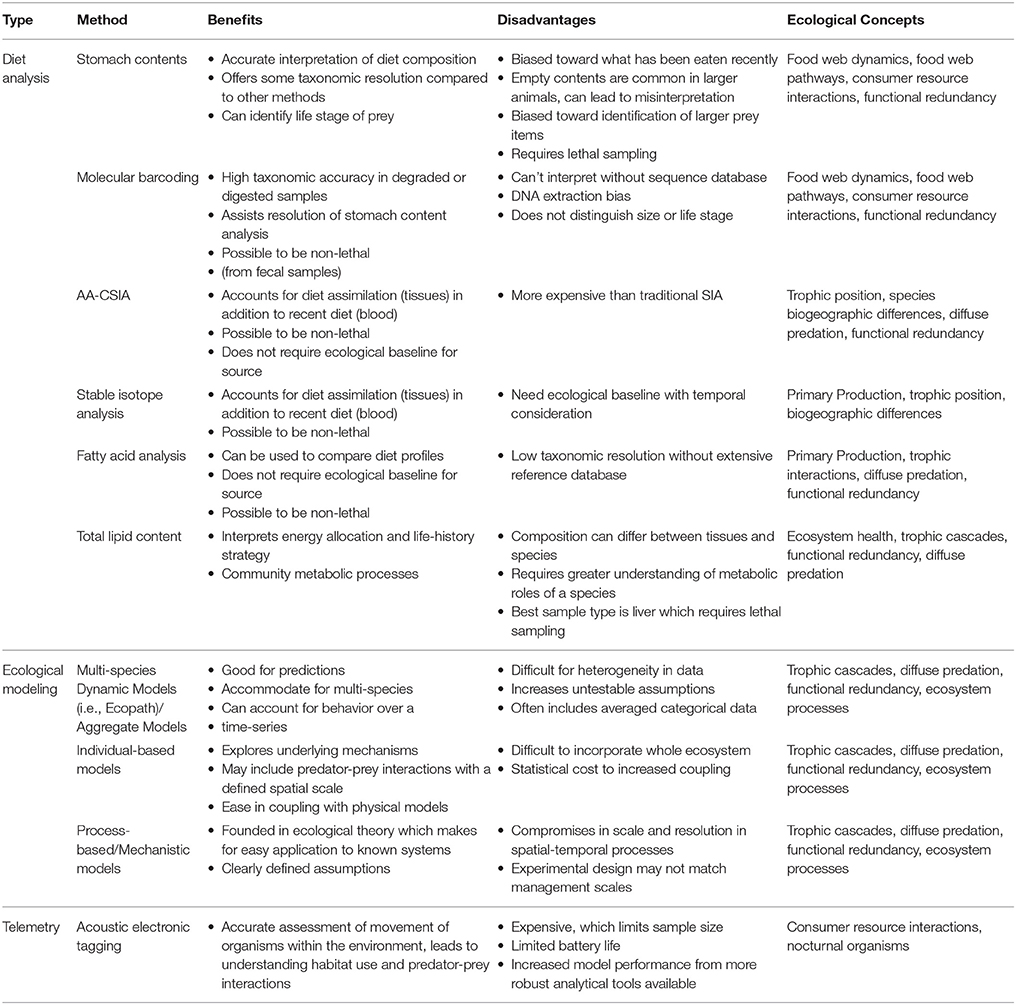
Table 1. Benefits and disadvantages of methods commonly used in trophodynamic study and which ecological concepts they most relate to.
Within a marine food web, an organism's role in energy transfer is assigned a trophic position (Bowen, 1997; Layman et al., 2012). Interactions among organisms and energy flow are typically defined by resource control or “trophic forcing,” where energy flow within a system can be consumer-driven (top-down), resource-driven (bottom-up), or middle-driven (mid-level consumers) (Verity and Smetacek, 1996; Frank et al., 2007; Young et al., 2015). The type of resource control can have major impacts on communities at localized and/or large-scales via cascades or pyramid structures (Sandin et al., 2008; Estes et al., 2011; Sandin and Zgliczynski, 2015). Therefore, it is important to examine how trophodynamics influence a community at a defined scale. Contributions from benthic and pelagic sources limit understanding of bottom-up processes on coral reefs because biogeochemical cycles are not well-described over spatiotemporal scales (Young et al., 2015). Nutrient enrichment and herbivory have been recognized as crucial bottom-up and top-down processes respectively; influencing ecosystem function and community structure and providing competing hypotheses (Smith et al., 2010). Meanwhile some researchers describe the influence of multiple controlling forces on coral reefs (Lapointe, 1999; Terborgh, 2015), and others report higher importance of specific trophic groups on resource availability (Lewis and Wainwright, 1985; Hughes et al., 1987, 2007). Disruption of trophic levels through loss and mortality of organisms in a community alter the stability of a food pyramid which can lead to trophic cascades.
For example, pressure exerted by higher trophic levels can control abundance of lower trophic groups preventing cascading effects across food webs. This assumes that keystone species and apex predators are the strongest controlling forces on food web dynamics (McClanahan and Branch, 2008). A few cases have described inverse pyramids where the biomass of predators is greater than that of lower level consumers (DeMartini et al., 2008; Sandin et al., 2008; Sandin and Zgliczynski, 2015), which is uncharacteristic of a typical marine environment. These examples have only been documented in near-pristine environments (Sandin and Zgliczynski, 2015; Mourier et al., 2016; Simpfendorfer and Heupel, 2016) and to date have not been reported outside of steep-sided, isolated atolls exposed to upwelling. Whether productivity subsidies supporting inverse pyramids in smaller isolated reefs can be possible for larger, continental environments is unknown. Continental reefs are also exposed to human activity, where high predator abundance is less common (Sandin and Zgliczynski, 2015). Without long-term data, it is unclear whether inverse pyramids are more representative of a natural, balanced state than bottom-up pyramids.
Additionally, functional redundancy and diffuse predation may prevent degradation under disturbance, masking the potential controlling effects of trophic groups or individual species (Bascompte et al., 2005). For example, many reef ecosystems have multiple mesopredators feeding at a similar trophic level. Interactions within and among these species and available prey complicate the ability to define trophic pathways. Aside from the concept of “mesopredator release” caused by removal of apex predators (Stallings, 2008; Ruppert et al., 2013, 2016; Roff et al., 2016a,b), mesopredator influence on communities is largely unknown. Middle-driven systems caused by intermediate consumer influence have only been described for oceanic environments where small pelagic fish control the abundance of both the predator and their prey (Cury et al., 2000; Young et al., 2015). To our knowledge, middle driven systems have not been explored for coral reefs. Beyond defining trophic position, the importance of understanding specific interactions between and among trophic groups is necessary to interpret ecological roles (Figures 1, 2).
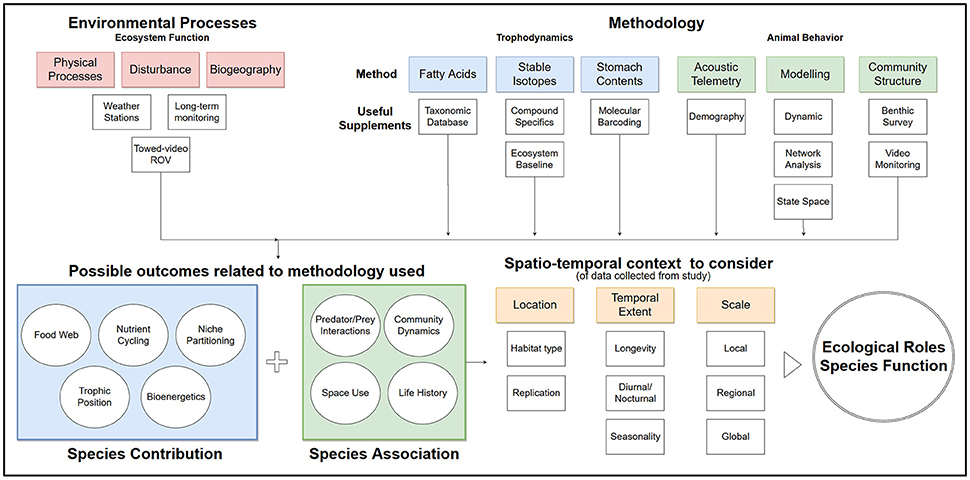
Figure 2. Flow chart of suggested process to inform ecological roles from common methodology used in trophodynamic study for species in coral reefs.
The presence of multiple-food webs within coral reefs decreases our ability to understand mechanisms that regulate stability. Based on present knowledge, it is still unclear whether coral reefs behave similar to other marine ecosystems from a trophodynamic perspective. Defining which ecosystem processes contribute to the abundance and biodiversity of coral reefs is controversial (Karlson and Hurd, 1993). While it is generally accepted that coral reef communities are stable, the interactions among communities and connectivity from larval dispersal and recruitment are less well-known (Vance and Steele, 2002). More research is needed to outline consumer-resource interactions and identify knowledge gaps in food webs. While energy availability is an indicator of reef health evidenced by species richness, habitat is also an influential factor (Bellwood et al., 2005). Even at the reef scale, community food web length can differ with habitat (Kolasinski et al., 2016). The importance of understanding spatial dynamics within the study area, whether local or regional, is crucial to assessing ecological roles and energy contribution of a species to a population, community, or ecosystem.
Additionally, more attention should be directed toward the influence of under-studied groups such as non-target, cryptic, nocturnal, and benthic macrofauna (Young et al., 2015). For instance, parasites are rarely included in trophodynamic study despite being the most common consumer type in ecological food webs (Demopoulos and Sikkel, 2015). Recent reef food web models that include parasitism show insignificant changes to overall flow of models, but can dramatically affect specific trophic pathways (Arias-González and Morand, 2006). Parasites are also thought to affect feeding behavior of herbivorous grazers (Fox et al., 2009). Other cryptic organisms can affect trophic links in consumer species with commonly accepted ecological roles. For example, consumption of copepods (Kramer et al., 2013) and high concentration of autotrophs (Clements et al., 2016) sourced within the epilithic algal matrix (EAM) by parrotfish raises questions about the main components of their nutrition. Exclusion of other trophic links such as connectivity to mangrove or seagrass habitats is also common, where grazers are known to make nocturnal migrations off coral reefs to avoid micropredation (Sikkel et al., 2017), or exploit foraging opportunities (Nagelkerken et al., 2000). Limited time and resources prevent incorporation of every component of a food web into a study, but the composition of components included, or excluded, requires consideration when interpreting results.
Methods Used to Study Trophodynamics in Tropical Coral Reefs
The main focus of trophodynamic research is determining spatially and temporally appropriate consumption and interactions across trophic groups (Figure 2). Paine (1980) demonstrated the effect of interaction strength of food webs on communities in aquatic systems, and new techniques are constantly being developed to better describe these interactions. Most methods for identifying food web relationships are limited by spatiotemporal scales and variable biological and environmental conditions (Sale, 2002; Chabanet et al., 2010; Layman et al., 2012; Young et al., 2015). Fortunately, new techniques in methodology and analysis are available to enhance ecological study (Table 1). Most of the methods in this section have already been well-reviewed or described by other authors (Layman, 2007; Ilves et al., 2011; Layman et al., 2012; McCauley et al., 2012; Gilby and Stevens, 2014; Young et al., 2015), so this text highlighted publications that used these methods to describe common ecological roles and functions of coral reef ecosystems. Much of the current research focuses on specific groups such as higher trophic levels or herbivores. While this focus helps define the roles of certain aspects of the food web, research is still needed to incorporate under-represented components of ecosystems to develop a more comprehensive view of coral reef ecosystems.
Diet Analyses
Diet analysis is one of the most common methods in trophodynamic study along with direct observation of predator prey-relationships (Choat et al., 2004; Fox et al., 2009; Kramer et al., 2013; Young et al., 2015; Wen et al., 2016). Stomach contents can directly inform what a consumer ingests by examining the frequency of occurrence of species in stomach samples (Cortés, 1999). This method can work well to uncover trophic interactions for commonly occurring and abundant species. However, there is often uncertainty in prey identification and metabolic requirements of a consumer (Young et al., 2015). For species of conservation concern, sample sizes for study can be low as there are limitations to lethal sampling. Non-lethal methods such as gastric lavage show what has been recently consumed (Cortés, 1997; Frisch et al., 2016), but may be unreliable for determining the full scope of the diet of an individual. For larger species such as sharks, angling may also induce gastric emptying before landing (Frisch et al., 2016), which can affect results. In fact, many studies using gastric lavage and baited capture report high proportions of empty stomachs in predators. Due to these limitations, biochemical tracers, and immunological testing through molecular identification of prey (Symondson, 2002) have been used to supplement stomach content studies. Taxonomic molecular barcoding can supplement gut content study and assist in characterization of diet (Paula et al., 2016). Molecular barcodes designed to identify diet specialization on invasive macroalgae by coral reef grazers has uncovered trophic links previously unknown in herbivorous fishes in Hawai'i (Stamoulis et al., 2017). Combination of multiple methods in diet analysis can identify trophic position and food web links for trophodynamics, but care is needed when using these methods to identify ecological roles. Habitat is known to be an important consideration in most ecological studies, and it is mentioned most frequently in studies examining stomach contents (98 percent, Table 2). Yet even recent studies have ignored potential effects of habitat by combining samples across regions without understanding community composition and available prey. Spatial scale and habitat variables are crucial elements to defining diet analysis in trophodynamics research. Seasonal (i.e., temporal) variability in prey abundance or availability must also be considered. While these aspects are present in some studies, they must be more widely applied to help define ecological processes and trophodynamics in coral reefs and other marine habitats.
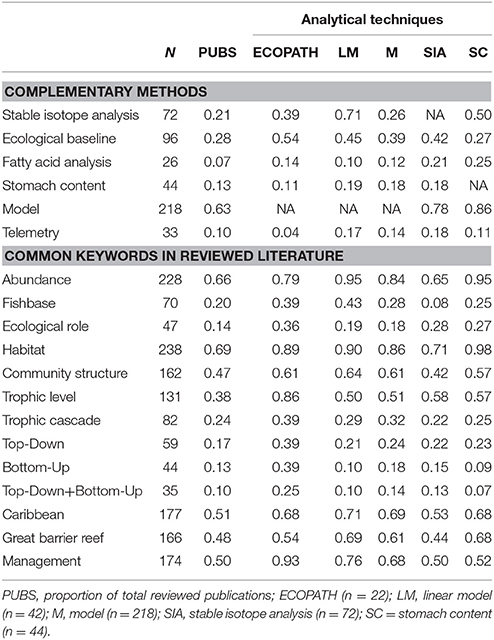
Table 2. Proportion of reviewed publications that use additional methods for trophodynamic study or mention common keywords in related literature (N = 398). EndNote® (Thompson Reuters) was used to annotate documents for searches.
Biochemical Tracers
Biochemical tracers identify dietary sources in a food web and what individuals directly consume (Post, 2002; Young et al., 2015). Tracers quantitatively measure assimilation through tissues to define the diet of an individual without the uncertainty of species identification of stomach content studies (Post, 2002; Layman et al., 2007). Nitrogen and carbon isotopic enrichment in bulk tissue are examined through stable isotope analysis to define the relationship between a predator and its prey (Post, 2002; Layman et al., 2007, 2012; McClanahan et al., 2011). For producers, carbon isotope proportions vary due to different methods of energy production through photosynthesis (Michener et al., 2007). Carbon (δ13C) is used to identify source pools as there is limited variation in values after trophic transfer, while nitrogen (δ15N) is used to identify trophic position as enrichment is progressive across trophic levels (Kolasinski et al., 2016). Spatiotemporal comparison of diet is also possible with fatty acid analysis. When a predator consumes a species, fatty acids are absorbed with little change in the unique signature, meaning that prey can be identified from adipose tissue and blood samples (Budge et al., 2006). Without a known database of fatty acid profiles, these studies can be limiting. For biochemical tracers, food web limitations mainly come from time and resources available to define an appropriate ecological baseline. Without understanding carbon sources, studies may lack context. For example, carbon flow is challenging to elucidate in coral reefs due to multiple end-members feeding from both benthic and pelagic sources. Application of compound specific amino acid analysis (AA-CSIA) and Bayesian mixing-model techniques can assist in defining these baselines (McMahon et al., 2015). AA-CSIA can provide better baselines by looking at specific amino acids that fractionate with each trophic step (Bradley et al., 2016). AA-CSIA can also reduce the number of samples for analysis by eliminating the need for an exhaustive baseline. But tissue turnover rates, functional redundancy, and complications in retention of nitrogen in certain organisms can also limit analysis (Post, 2002; Layman et al., 2007, 2012; McClanahan et al., 2011). Without species-specific study, improved techniques, and time-series baselines, it is difficult to get a true estimate of trophic position (Layman et al., 2012). Recently, Matley et al. (2016) showed that diet tissue determination factors and turnover rates measured for temperate species may not apply to tropical species, as δ15N enrichment was lower than published values for a slow-growing predatory reef fish. In larger predatory species such as sharks, care in sample preparation is also necessary as urea retention without extraction can severely affect values, which is often not a common method employed for stable isotope analysis (Li et al., 2016). The physical and biogeochemical nature of a habitat can also have large effects on trophic community structure even at a fine scale. For example, Kolasinski et al. (2016) studied macro-invertebrate communities of coral reefs and found significant temporal and spatial differences in food web lengths indicating a variety of energy pathways which complicate trophic level definition. Therefore, consideration should be given to understanding the data sources that may limit research to specific habitats or time periods. Recent studies use large-scale spatial and temporal differences in sample collection as caveats in the discussion (Frisch et al., 2016), as opposed to incorporation into the study design. From reviewed publications, only 42 percent of stable isotope analyses included a reference to carbon sources, and 21 percent for fatty acid analyses; 50 percent of studies used a combination of stable isotopes and stomach contents, while 25 percent used a combination of fatty acid and stomach contents (Table 2). Stable isotopes contributed to 58 percent of studies that assessed trophic levels of organisms and have been used in 39 percent of Ecopath studies. Refined analysis of trophic levels through biochemical tracers is likely to strengthen and improve trophodynamics research as applications of these methods expand.
Ecological Modeling
Statistical models are widely used in ecological studies to understand energy flow and provide a whole of system interpretation. Due to the complexities of marine food webs, trophic relationships are often used to categorize interactions between trophic levels and among individuals within models (Bozec et al., 2005). While linear models often show patterns in specific relationships from quantitative study, they are not robust enough to handle complex food webs such as those on coral reefs (Evans et al., 2013). Dynamic models are required to describe ecosystem organization through networks of species that interact (Liu et al., 2009). In trophodynamic studies, species abundance and diversity are typically categorized by functional groups where diet and habitat similarities are shared by multiple species. This allows simplification of models to a tractable level where input parameters are determined by functional group and often include known values of productivity, biomass, and metabolic efficiency.
While there are multiple dynamic food web models available, Ecopath (Polovina, 1984) is the most commonly used for marine and aquatic study (Heymans et al., 2016) and has been applied to coral reefs. Under the assumption of energy equilibrium, Ecopath uses mass balance equations to determine trophic relationships of biomass and productivity (Polovina, 1984). Ecopath can be fitted for time series with Ecosim (EwE) (Gotelli and Entsminger, 2004) and also account for spatial differentiation of habitat with Ecospace (Opschoor, 1995). While Ecopath is a popular method, few studies using this model in marine research include time series and spatial data, and even fewer are applied to coral reef ecosystems (Heymans et al., 2016).
For Ecopath to be effective, thermodynamics and ecological knowledge behind fitting a model are required to prevent misuse (Evans et al., 2013; Young et al., 2015). Thirty-nine percent of reviewed Ecopath studies (Table 2) for coral reefs used Fishbase (Froese and Pauly, 2000) as a source of data for the model. While this resource is a reputable data repository with relevant diet and productivity information, there is considerable uncertainty in empirical data and regional species variation. Without understanding ontogenetic diet change, temporal and biological shifts in behavior and foraging of a species, responses to disturbance may be poorly interpreted (Young et al., 2015). Even if an organism has a highly specialized diet and small home range, external stressors and biogeography vary (Sale, 2002). Ecopath relies upon the strength of interactions between predators and prey, and requires extensive knowledge of each species fitted into the model. While these models take diet into account, predator avoidance is less well-described and parameters such as refuge availability and niche occupation are difficult to fit into a model. For mobile, large predators, effects on lower trophic groups are poorly understood as top-down forces often result in diffuse predation that is difficult to characterize with Ecopath parameters. Predator-prey structure in ecological models was recently examined and researchers found that more complex models do not necessarily invalidate predicted behaviors in simpler models, but care must be used when employing the latter (Walters et al., 2016). The authors showed how assumptions of feeding rates of predators, prey availability and other factors drastically change isocline patterns in predator-prey models. They note that uniform spatial assumptions should be avoided when using trophic models. Of the reviewed studies, habitat was referenced in 89 percent of Ecopath studies focused on coral reefs (Table 2), but many were not specific to a single area and assumed homogenous habitat across regional scales. Testing underlying assumptions is critical when employing predator-prey models (Heymans et al., 2016), and understanding interactions at an appropriate spatial scale will assist in increasing the efficacy of these models.
While some scientists have urged that more process-based ecological models be developed to identify the underlying mechanistic behavior of an ecosystem (Evans et al., 2013; Turney and Buddle, 2016), recent articles still often ignore dynamics of spatial and temporal variability as well as historical baselines (Lamy et al., 2016). Some authors have moved past dynamic models such as Ecopath and explored individual-based predator-prey population models to account for spatiotemporal heterogeneity (Thierry et al., 2015). Others have tested vulnerability of reef ecosystems by measuring overall entropy from bioenergetics (Arreguín-Sánchez and Ruiz-Barreiro, 2014), interaction strength between trophic levels (Bascompte et al., 2005) and applied network analysis (Navia et al., 2016). Integration of unified models may also be beneficial. While unified models often are used to explain theories of biodiversity, recent developments of metacommunity analysis between labrid fishes and corals have identified patterns of species associations (Connolly et al., 2017). Species associations are critical in integration of trophodynamics into management. Critics of food web models for coral reefs highlight that any model will be a simplified version of real-time processes and caution should be taken when using them to inform management. Although management is mentioned in 98 percent of reviewed publications (Table 2), few articles using Ecopath in marine environments have been used for management purposes (Heymans et al., 2016). To better inform management, research is needed on spatiotemporal variability to better fit models. As Ecopath provides an informed snapshot of behavior of a system, including variability over space and time may assist in reducing uncertainty.
Telemetry
Acoustic telemetry is widely used in aquatic ecology, but rarely incorporated into trophodynamic studies. Telemetry can be used to better understand the distribution, residency, and behavioral patterns of species and applied to understanding how predators interact with prey (McCauley et al., 2012; Young et al., 2015; Matley et al., 2016). Matley et al. (2016) determined that although two species of co-occurring reef fish had overlapping diets, space use differed between the two species suggesting niche separation. Studying movement can also show how behavior may be affected by environmental stressors and conditions. Telemetry can also be used to supplement other methods to identify behavioral adaptions to resources such as targeting invasive species (Bierwagen et al., 2017). Improved analytical methods for ecology such as network analysis (Espinoza et al., 2015), Bayesian statistics (Johnson et al., 2010) and state-space models (Jonsen et al., 2005) are assisting predictive capability of movement relative to environmental variability. For example, telemetry has helped define population dynamics through focused mark-recapture models (Dudgeon et al., 2015). Other applications such as identifying feeding patterns from movement and prey associations that may influence food web dynamics may play a role in future trophodynamic analyses. Despite the advantages of telemetry, the time and financial investment required may limit sample sizes and application to trophodynamics (Young et al., 2015). Telemetry was used in less than 10 percent of reviewed studies and less than 20 percent in combination with other empirical methods (Table 2).
Challenges and Discussion
The key challenges in trophodynamic study in coral reefs come from logistical difficulties, methodology limitations, and context of study design. Whether a study intends to identify an energy pathway, or consider management decisions relating to a species, the conclusions should not go beyond the limitations derived from the study. Sufficient information of reef ecology such as spatiotemporal abundance, distribution, habitat associations, environmental inputs, diet, and life history of a species is necessary to inform ecological roles and function (Figure 2). While recent examples of poor management decisions have created a need for standardizing approaches in the field of trophodynamics (Grubbs et al., 2016), studies are still omitting key concepts before implementing applied research. We have highlighted such studies in relation to popular methods, but also identified recent research that is incorporating and combining new methods to account for some of the challenges faced within the field. In addition to using combined methodology, researchers should consider concepts that better explain the organizational structure of coral reefs and how multiple food webs or communities interact.
Accounting for Variation in Studies
Habitat and community structure are well-documented to be important variables in ecosystem processes, particularly when examining trophodynamics. Yet, scientists often take a “one size fits all approach” to food-web studies. Dornelas et al. (2006) demonstrated that coral reefs cannot be explained by widely-accepted theories of biodiversity, which suggests that spatiotemporal stochasticity observed in coral reefs is not well-understood. This validates the need to understand process based interactions. Most trophodynamic studies of coral reefs come from the Caribbean and Great Barrier Reef, which cover large spatial scales and latitudinal gradients. While biogeographic variability is constantly acknowledged, publications link conservation concern to broad topics such as management zones irrespective of reef variability or geographic position (Frisch et al., 2014; Rizzari et al., 2014; Boaden and Kingsford, 2015), and perform large scale analyses based on databases that do not account for fine-scale variation (Campbell and Pardede, 2006; Graham et al., 2008; Campbell et al., 2011; Barneche et al., 2014; Alonso et al., 2015; Aguilar-Medrano and Barber, 2016). While management zones are important to understanding human disturbance, many studies exploring fishing effects lack historical baselines, movement data, and diet relative to changing diversity and community structure (Greenwood et al., 2010; Edgar et al., 2011). Additionally, the influence of natural cycles is rarely considered (Kruse et al., 2016) although they can play an important role in ecosystem function. A review by Bijoux et al. (2013) discussed the effect of natural cycles (diurnal, tidal, lunar, and seasonal variation) on fish movement where the authors found that studies ignoring natural cycles increased unexplained variation in the data thereby reducing their effectiveness in defining ecosystem processes.
Controlling Forces in Trophodynamics
In addition to lacking spatiotemporal context, researchers may be misinterpreting controlling forces in coral reefs. Across all ecosystems, biomass pyramids and the role of trophic subsidies are not well-understood (Trebilco et al., 2013). Top-down vs. bottom-up organization of coral reefs is debated in the field, particularly in regard to the focus of conservation efforts (herbivore focused or predator focused). Pyramids are the primary structure used to argue resource control, but may oversimplify the unique and fine-scale interactions that occur at the reef level. Marine food webs have been described as “ecological road maps” and the varying networks that link different trophic levels in coral reefs could explain their resilience to cascading effects (Turney and Buddle, 2016). In coral reefs, the semi-open nature of communities increases the difficulty in mapping food webs from source to consumer and the influence mesopredators may have on lower trophic levels. Trophic cascades were mentioned in 24 percent of reviewed publications, yet there is little empirical evidence documenting cascading effects in coral reefs. Even in the few cases documenting trophic cascades, the results are inconclusive. Estes et al. (2011) used the Northern Line Islands as an example of trophic cascade, but the original research by Sandin et al. (2008) showed no evidence of trophic cascade through use of principle component analysis. The work by Sandin et al. (2008) reflects conclusions made by Bascompte et al. (2005) who showed strength of trophic interactions on coral reefs buffer cascading effects but become weaker under pressure of external perturbations such as overfishing. This does not mean that cascading effects do not exist, but evidence is limited and little is known outside of correlative associations regarding herbivore biomass increases due to predator removal or other effects of disturbance.
In trophic cascades, if a trophic level is added or removed, coral-algal phase shifts and changes in ecosystem stability can occur (Terborgh, 2015). The strongest evidence of cascades found in coral reef systems come from herbivory, where depletion of grazers such as echinoderms from overfishing or pathogens allowed for explosive population growth of macroalgae in the Caribbean (Hughes et al., 1987; Mumby et al., 2005). Rasher et al. (2017) also described a trophic cascade via reduced exposure of herbivores to predators which resulted in variation in feeding patterns based on fear effects. Though the expected negative effects of cascades in reefs aren't always clear. In cases where conservation efforts have maintained predator populations via reduced fishing, the expected cascading effect of macroalgal increase from a depletion of grazers as prey doesn't always occur (Mumby et al., 2007). Keystone species and functional redundancy are not always present in reefs with high biodiversity (Hoey and Bellwood, 2009), which makes comparison across spatial scales all the more difficult. Even if assumed cascading events are observed such as phase shifts, finding the cause of such patterns at large spatial scales is challenging (Dulvy et al., 2004). Attention should also be paid to temporal gradients over which cascades occur, considering life-history and growth capabilities of populations as well as lagged effects from perturbations that can occur over extended time periods (Dulvy et al., 2004; Grubbs et al., 2016). The difficulty in linking trophic levels to the same event that categorizes a trophic cascade is difficult to support empirically, particularly when most reefs are already in a degraded state from external stressors. The fact that cascades have not yet been effectively documented for coral reefs undermines the assumed organization of coral reef food webs. High biodiversity complicates ecosystem models and trophodynamics, but may be key to the functionality of reef ecosystems in a changing environment.
Although pyramid structure is evident in coral reefs, there are high numbers of mesopredators representing intermediate trophic levels that are thought to exhibit functional redundancy. Mesopredators are considered to have less of an effect on the trophic structure of a system (Paine, 1980; Estes et al., 2011), but there is little empirical evidence to support this due to difficulty in defining predator-prey relationships. A recent meta-analysis of food web studies found that aquatic models produced a strong pyramid pattern, suggesting scale variance in predator-prey ratios according to biomass power laws consistent with Hatton et al. (2015) (Turney and Buddle, 2016). The analysis also showed that on average aquatic communities have a higher diversity of mesopredator species than herbivores, with low abundance of top predators. Intermediate effects of mesopredators on aquatic systems are largely unknown and often grouped within many ecosystem types such as intertidal, pelagic, and reef (Hatton et al., 2015). Elasmobranchs and other fishes are known to feed at different trophic levels based on stage of maturity, where they exhibit high functional redundancy as mesopredators and limited redundancy as apex (Navia et al., 2017). Thus, assigning a single ecological role to a species is limiting and can affect the predictive nature of model capability. The role and effects of mesopredators requires further exploration and definition to refine where greatest predation and productivity sources occur in reef systems. Many researchers believe that coral reefs are influenced by both top-down and bottom-up processes, and it should not be a question of one vs. the other (Terborgh, 2015). Further exploration of middle-driven systems and consideration of the driving interactions between trophic groups should be considered.
The Contributions of Trophodynamics to Coral Reef Ecology
There are many components of trophodynamics that we are beginning to understand which can be used to inform ecosystem function and changes over time. Many of these components are shared among marine systems, but discretion should be used when assuming similar trophodynamics processes that are not fully described for coral systems. Current research validates that coral reefs fundamentally have:
(1) Highly complex, semi-open systems (Sale, 2002).
(2) Resident species and mobile visitors that utilize reef habitats (Dudgeon et al., 2015).
(3) Influence from both benthic and pelagic productivity sources (Michener et al., 2007).
(4) High abundance and diversity which play a large role in ecosystem function (Choat et al., 2004).
(5) Spatiotemporal variability which is essential to assessing trophic position (Heymans et al., 2016).
(6) Small areas with high diversity exhibiting functional redundancy between producers and consumers (Aguilar-Medrano and Calderon-Aguilera, 2016).
(7) Food web omnivory that can weaken chance of trophic cascades, even in the presence of exploitation of predators (Bascompte et al., 2005).
(8) Predators that are known to exhibit diffuse predation, although their effect on lower trophic levels is still poorly understood (Heupel et al., 2014).
(9) Cryptic, invertebrate, and nocturnal organisms which are often ignored in food webs (Marnane and Bellwood, 2002; Kolasinski et al., 2016).
(10) Pyramids of species richness that are not generated by chance. (Turney and Buddle, 2016).
These concepts need to be explicitly considered in study designs of trophodynamic research and uncertainty should be acknowledged before drawing conclusions regarding the ecological role of any species. Additionally, the underlying trophodynamic processes describing these observations should incorporate long-term datasets that accurately reflect the scope of data used. Before describing ecological roles of reef organisms, it is necessary to determine which interactions may have the highest influence on the trophic structure of these complex systems. For researchers, this space is still largely under-studied, and collaborative efforts are likely needed to elucidate the mechanisms that contribute to the stability of these ecosystems.
There are still many ecological unknowns in coral reef systems and there will likely never be a perfect approach to fully describe coral reef trophodynamics, but we can strive for better empirical data collection and analysis of patterns. Standardizing the approach to applied questions may help create a more cohesive space for collaboration in future studies. There is a need to apply multiple methods and clear definitions of spatial and temporal scale to meet the needs of trophodynamic research (Figure 2). How a species contributes energetically and how they interact with other species within a community take different methodological approaches and clear synthesis between the two to identify ecological roles. Arguably, coral reef systems do not appear to energetically behave the same as other marine food webs, such as intertidal or pelagic systems, and trophodynamic study should consider different scenarios and models. Based on concepts in this paper, we suggest recent literature may not adequately acknowledge the unique differences in coral reef food webs against the broader literature in marine trophodynamics, particularly over varying spatiotemporal scales. While theories for coral reefs are constantly being modified, conclusions of many articles still resort to generic descriptions of standard pyramid structure to explain biodiversity. Predation and competition within predator-prey interactions should be further considered in addition to exploring the effects of both bottom-up and top-down approaches. Without a better understanding of essential reef processes that affect ecological roles of species over both space and time, caution should be used in applying results to management and conservation efforts.
Author Contributions
All persons who qualify under authorship criteria to this review are listed as authors and all of which take responsibility for the content of the article. All authors SB, MH, AC, and CS contributed to the concept and design of the article. The corresponding author, SB, is responsible for research, acquisition of data, and drafting of the manuscript. MH, AC, and CS contributed equally to critical revisions of content and subject matter within the body of the manuscript. All authors agree that this version of the manuscript is acceptable for submission.
Funding
Access to resources were provided through James Cook University where no additional funding was needed. We would like to thank the Australian Government and the James Cook University JCUPRS scholarship for support to undertake the research for this manuscript.
Conflict of Interest Statement
The authors declare that the research was conducted in the absence of any commercial or financial relationships that could be construed as a potential conflict of interest.
Supplementary Material
The Supplementary Material for this article can be found online at: https://www.frontiersin.org/articles/10.3389/fmars.2018.00024/full#supplementary-material
References
Aguilar-Medrano, R., and Barber, P. H. (2016). Ecomorphological diversification in reef fish of the genus Abudefduf (Percifomes, Pomacentridae). Zoomorphology 135, 103–114. doi: 10.1007/s00435-015-0291-6
Aguilar-Medrano, R., and Calderon-Aguilera, L. E. (2016). Redundancy and diversity of functional reef fish groups of the Mexican Eastern Pacific. Mar. Ecol. 37, 119–133. doi: 10.1111/maec.12253
Alonso, D., Pinyol-Gallemí, A., Alcoverro, T., and Arthur, R. (2015). Fish community reassembly after a coral mass mortality: higher trophic groups are subject to increased rates of extinction. Ecol. Lett. 18, 451–461. doi: 10.1111/ele.12426
Alva-Basurto, J. C., and Arias-González, J. E. (2014). Modelling the effects of climate change on a Caribbean coral reef food web. Ecol. Modell. 289, 1–14. doi: 10.1016/j.ecolmodel.2014.06.014
Arias-González, J. E., and Morand, S. (2006). Trophic functioning with parasites: a new insight for ecosystem analysis. Mar. Ecol. Prog. Ser. 320, 43–53. doi: 10.3354/meps320043
Arreguín-Sánchez, F., and Ruiz-Barreiro, T. M. (2014). Approaching a functional measure of vulnerability in marine ecosystems. Ecol. Indic. 45, 130–138. doi: 10.1016/j.ecolind.2014.04.009
Ashworth, E. C., Depczynski, M., Holmes, T. H., and Wilson, S. K. (2014). Quantitative diet analysis of four mesopredators from a coral reef. J. Fish Biol. 84, 1031–1045. doi: 10.1111/jfb.12343
Barneche, D. R., Kulbicki, M., Floeter, S. R., Friedlander, A. M., Maina, J., and Allen, A. P. (2014). Scaling metabolism from individuals to reef-fish communities at broad spatial scales. Ecol. Lett. 17, 1067–1076. doi: 10.1111/ele.12309
Bascompte, J., Melian, C. J., and Sala, E. (2005). Interaction strength combinations and the overfishing of a marine food web. Proc. Natl. Acad Sci. U.S.A. 102, 5443–5447. doi: 10.1073/pnas.0501562102
Bauman, A. G., Burt, J. A., Feary, D. A., Marquis, E., and Usseglio, P. (2010). Tropical harmful algal blooms: an emerging threat to coral reef communities? Mar. Pollut. Bull. 60, 2117–2122. doi: 10.1016/j.marpolbul.2010.08.015
Bellwood, D., Hughes, T., Connolly, S., and Tanner, J. (2005). Environmental and geometric constraints on Indo-Pacific coral reef biodiversity. Ecol. Lett. 8, 643–651. doi: 10.1111/j.1461-0248.2005.00763.x
Bellwood, D. R., Hughes, T. P., Folke, C., and Nyström, M. (2004). Confronting the coral reef crisis. Nature 429, 827–833. doi: 10.1038/nature02691
Bierwagen, S. L., Price, D. K., Pack, A. A., and Meyer, C. G. (2017). Bluespine unicornfish (Naso unicornis) are both natural control agents and mobile vectors for invasive algae in a Hawaiian Marine Reserve. Mar. Biol. 164:25. doi: 10.1007/s00227-016-3049-x
Bijoux, J., Dagorn, L., Gaertner, J.-C., Cowley, P., and Robinson, J. (2013). The influence of natural cycles on coral reef fish movement: implications for underwater visual census (UVC) surveys. Coral Reefs 32, 1135–1140. doi: 10.1007/s00338-013-1075-4
Boaden, A. E., and Kingsford, M. J. (2015). Predators drive community structure in coral reef fish assemblages. Ecosphere 6, 1–33. doi: 10.1890/ES14-00292.1
Bowen, W. D. (1997). Role of marine mammals in aquatic ecosystems. Mar. Ecol. Prog. Ser. 158, 267–274. doi: 10.3354/meps158267
Bozec, Y. M., Kulbicki, M., Chassot, E., and Gascuel, D. (2005). Trophic signature of coral reef fish assemblages: towards a potential indicator of ecosystem disturbance. Aquat. Living Resour. 18, 103–109. doi: 10.1051/alr:2005013
Bradley, C. J., Longenecker, K., Pyle, R. L., and Popp, B. N. (2016). Compound-specific isotopic analysis of amino acids reveals dietary changes in mesophotic coral-reef fish. Mar. Ecol. Prog. Ser. 558, 65–79. doi: 10.3354/meps11872
Budge, S. M., Iverson, S. J., and Koopman, H. N. (2006). Studying trophic ecology in marine ecosystems using fatty acids: a primer on analysis and interpretation. Mar. Mamm. Sci. 22, 759–801. doi: 10.1111/j.1748-7692.2006.00079.x
Campbell, S. J., Kartawijaya, T., and Sabarini, E. K. (2011). Connectivity in reef fish assemblages between seagrass and coral reef habitats. Aquat. Biol. 13, 65–77. doi: 10.3354/ab00352
Campbell, S. J., and Pardede, S. T. (2006). Reef fish structure and cascading effects in response to artisanal fishing pressure. Fish. Res. 79, 75–83. doi: 10.1016/j.fishres.2005.12.015
Ceccarelli, D. M., Frisch, A. J., Graham, N. A. J., Ayling, A. M., and Beger, M. (2014). Habitat partitioning and vulnerability of sharks in the Great Barrier Reef Marine Park. Rev. Fish Biol. Fish. 24, 169–197. doi: 10.1007/s11160-013-9324-8
Chabanet, P., Guillemot, N., Kulbicki, M., Vigliola, L., and Sarramegna, S. (2010). Baseline study of the spatio-temporal patterns of reef fish assemblages prior to a major mining project in New Caledonia (South Pacific). Mar. Pollut. Bull. 61, 598–611. doi: 10.1016/j.marpolbul.2010.06.032
Choat, J., and Bellwood, D. (1985). Interactions amongst herbivorous fishes on a coral reef: influence of spatial variation. Mar. Biol. 89, 221–234. doi: 10.1007/BF00393655
Choat, J., and Bellwood, D. (1991). “Reef fishes: their history and evolution,” in The Ecology of Fishes on Coral Reefs, ed P.F Sale (San Diego, CA: Academic Press), 39–66.
Choat, J. H., Clements, K. D., and Robbins, W. D. (2002). The trophic status of herbivorous fishes on coral reefs 1: dietary analyses. Mar. Biol. 140, 613–623. doi: 10.1007/s00227-001-0715-3
Choat, J. H., Robbins, W. D., and Clements, K. D. (2004). The trophic status of herbivorous fishes on coral reefs - II. Food processing modes and trophodynamics. Mar. Biol. 145, 445–454. doi: 10.1007/s00227-004-1341-7
Clements, K. D., German, D. P., Piché, J., Tribollet, A., and Choat, J. H. (2016). Integrating ecological roles and trophic diversification on coral reefs: multiple lines of evidence identify parrotfishes as microphages. Biol. J. Linn. Soc. 120, 729–751. doi: 10.1111/bij.12914
Connolly, S. R., Hughes, T. P., and Bellwood, D. R. (2017). A unified model explains commonness and rarity on coral reefs. Ecol. Lett. 20, 477–486. doi: 10.1111/ele.12751
Cortés, E. (1997). A critical review of methods of studying fish feeding based on analysis of stomach contents: application to elasmobranch fishes. Can. J. Fish. Aquat. Sci. 54, 726–738. doi: 10.1139/f96-316
Cortés, E. (1999). Standardized diet compositions and trophic levels of sharks. ICES J. Mar. Sci. 56, 707–717. doi: 10.1006/jmsc.1999.0489
Cox, C. B., Moore, P. D., Marquardt, W., Demaree, R., and Grieve, R. (2000). Biogeography: An Ecological and Evolutionary Approach, 6th Edn. Oxford: Blackwell Scientific Publications.
Cury, P., Bakun, A., Crawford, R. J., Jarre, A., Quinones, R. A., Shannon, L. J., et al. (2000). Small pelagics in upwelling systems: patterns of interaction and structural changes in “wasp-waist” ecosystems. ICES J. Mar. Sci. 57, 603–618. doi: 10.1006/jmsc.2000.0712
DeMartini, E. E., Friedlander, A. M., Sandin, S. A., and Sala, E. (2008). Differences in fish-assemblage structure between fished and unfished atolls in the northern Line Islands, central Pacific. Mar. Ecol. Prog. Ser. 365, 199–215. doi: 10.3354/meps07501
Demopoulos, A. W., and Sikkel, P. C. (2015). Enhanced understanding of ectoparasite–host trophic linkages on coral reefs through stable isotope analysis. Int. J. Parasitol. Parasites Wildl. 4, 125–134. doi: 10.1016/j.ijppaw.2015.01.002
Dornelas, M., Connolly, S. R., and Hughes, T. P. (2006). Coral reef diversity refutes the neutral theory of biodiversity. Nature 440, 80–82. doi: 10.1038/nature04534
Dudgeon, C. L., Pollock, K. H., Braccini, J. M., Semmens, J. M., and Barnett, A. (2015). Integrating acoustic telemetry into mark–recapture models to improve the precision of apparent survival and abundance estimates. Oecologia 178, 761–772. doi: 10.1007/s00442-015-3280-z
Dulvy, N. K., Freckleton, R. P., and Polunin, N. V. C. (2004). Coral reef cascades and the indirect effects of predator removal by exploitation. Ecol. Lett. 7, 410–416. doi: 10.1111/j.1461-0248.2004.00593.x
Edgar, G. J., Banks, S. A., Bessudo, S., Cortes, J., Guzman, H. M., Henderson, S., et al. (2011). Variation in reef fish and invertebrate communities with level of protection from fishing across the Eastern Tropical Pacific seascape. Glob. Ecol. Biogeogr. 20, 730–743. doi: 10.1111/j.1466-8238.2010.00642.x
Espinoza, M., Heupel, M. R., Tobin, A. J., and Simpfendorfer, C. A. (2015). Residency patterns and movements of grey reef sharks (Carcharhinus amblyrhynchos) in semi-isolated coral reef habitats. Mar. Biol. 162, 343–358. doi: 10.1007/s00227-014-2572-x
Estes, J. A., Terborgh, J., Brashares, J. S., Power, M. E., Berger, J., Bond, W. J., et al. (2011). Trophic downgrading of planet Earth. Science 333, 301–306. doi: 10.1126/science.1205106
Evans, M. R., Bithell, M., Cornell, S. J., Dall, S. R., Díaz, S., Emmott, S., et al. (2013). Predictive systems ecology. Proc. Biol. Sci. 280:20131452. doi: 10.1098/rspb.2013.1452
Falagas, M. E., Pitsouni, E. I., Malietzis, G. A., and Pappas, G. (2008). Comparison of PubMed, Scopus web of science, and Google scholar: strengths and weaknesses. FASEB J. 22, 338-342. doi: 10.1096/fj.07-9492LSF
Fox, R. J., Sunderland, T. L., Hoey, A. S., and Bellwood, D. R. (2009). Estimating ecosystem function: contrasting roles of closely related herbivorous rabbitfishes (Siganidae) on coral reefs. Mar. Ecol. Prog. Ser. 385, 261–269. doi: 10.3354/meps08059
Frank, K. T., Petrie, B., and Shackell, N. L. (2007). The ups and downs of trophic control in continental shelf ecosystems. Trends Ecol. Evol. 22, 236–242. doi: 10.1016/j.tree.2007.03.002
Frisch, A. J., Ireland, M., and Baker, R. (2014). Trophic ecology of large predatory reef fishes: energy pathways, trophic level, and implications for fisheries in a changing climate. Mar. Biol. 161, 61–73. doi: 10.1007/s00227-013-2315-4
Frisch, A. J., Ireland, M., Rizzari, J. R., Lönnstedt, O. M., Magnenat, K. A., Mirbach, C. E., et al. (2016). Reassessing the trophic role of reef sharks as apex predators on coral reefs. Coral Reefs 35, 459–472. doi: 10.1007/s00338-016-1415-2
Froese, R., and Pauly, D. (eds.). (2000). FishBase 2000: Concepts, Design and Data Sources. Los Baños: ICLARM, 344.
Gilby, B. L., and Stevens, T. (2014). Meta-analysis indicates habitat-specific alterations to primary producer and herbivore communities in marine protected areas. Glob. Ecol. Conserv. 2, 289–299. doi: 10.1016/j.gecco.2014.10.005
Gotelli, N. J., and Entsminger, G. L. (2004). EcoSim: Null Models Software for Ecology. Version 7. Acquired Intelligence Inc. & Kesey-Bear. Jericho, VT 05465. Computer software. Available online at: http://garyentsminger.com/ecosim/index.htm (Accessed April 19, 2014).
Graham, N. A., McClanahan, T. R., MacNeil, M. A., Wilson, S. K., Polunin, N. V., Jennings, S., et al. (2008). Climate warming, marine protected areas and the ocean-scale integrity of coral reef ecosystems. PLoS ONE 3:3039. doi: 10.1371/journal.pone.0003039
Greenwood, N. D. W., Sweeting, C. J., and Polunin, N. V. C. (2010). Elucidating the trophodynamics of four coral reef fishes of the Solomon Islands using delta N-15 and delta C-13. Coral Reefs 29, 785–792. doi: 10.1007/s00338-010-0626-1
Grubbs, R. D., Carlson, J. K., Romine, J. G., Curtis, T. H., McElroy, W. D., McCandless, C. T., et al. (2016). Critical assessment and ramifications of a purported marine trophic cascade. Sci. Rep. 6:20970. doi: 10.1038/srep20970
Harmelin-Vivien, M. L. (2002). “Energetics and fish diversity,” in The Ecology of Fishes on Coral Reefs, ed P. F. Sale (San Diego, CA: Academic Press), 265–274.
Hatton, I. A., McCann, K. S., Fryxell, J. M., Davies, T. J., Smerlak, M., Sinclair, A. R., et al. (2015). The predator-prey power law: biomass scaling across terrestrial and aquatic biomes. Science 349:6284. doi: 10.1126/science.aac6284
Heupel, M. R., Knip, D. M., Simpfendorfer, C. A., and Dulvy, N. K. (2014). Sizing up the ecological role of sharks as predators. Mar. Ecol. Prog. Ser. 495, 291–298. doi: 10.3354/meps10597
Heymans, J. J., Coll, M., Link, J. S., Mackinson, S., Steenbeek, J., Walters, C., et al. (2016). Best practice in Ecopath with Ecosim food-web models for ecosystem-based management. Ecol. Modell. 331, 173–184. doi: 10.1016/j.ecolmodel.2015.12.007
Hoey, A. S., and Bellwood, D. R. (2009). Limited functional redundancy in a high diversity system: single species dominates key ecological process on coral reefs. Ecosystems 12, 1316–1328. doi: 10.1007/s10021-009-9291-z
Hubert, N., Paradis, E., Bruggemann, H., and Planes, S. (2011). Community assembly and diversification in Indo-Pacific coral reef fishes. Ecol. Evol. 1, 229–277. doi: 10.1002/ece3.19
Hughes, T. P., Reed, D. C., and Boyle, M.-J. (1987). Herbivory on coral reefs: community structure following mass mortalities of sea urchins. J. Exp. Mar. Biol. Ecol. 113, 39–59. doi: 10.1016/0022-0981(87)90081-5
Hughes, T. P., Rodrigues, M. J., Bellwood, D. R., Ceccarelli, D., Hoegh-Guldberg, O., McCook, L., et al. (2007). Phase shifts, herbivory, and the resilience of coral reefs to climate change. Curr. Biol. 17, 360–365. doi: 10.1016/j.cub.2006.12.049
Huston, M. A. (1983). Biological Diversity: The Coexistence of Species on Changing Landscapes. Cambridge, UK: Cambridge University Press; Keever. C.
Ilves, K. L., Kellogg, L. L., Quattrini, A. M., Chaplin, G. W., Hertler, H., and Lundberg, J. G. (2011). Assessing 50-year change in bahamian reef fish assemblages: evidence for community response to recent disturbance? Bull. Mar. Sci. 87, 567–588. doi: 10.5343/bms.2010.1052
Johnson, H. E., Scott Mills, L., Wehausen, J. D., and Stephenson, T. R. (2010). Combining ground count, telemetry, and mark–resight data to infer population dynamics in an endangered species. J. Appl. Ecol. 47, 1083–1093. doi: 10.1111/j.1365-2664.2010.01846.x
Jonsen, I. D., Flemming, J. M., and Myers, R. A. (2005). Robust state-space modeling of animal movement data. Ecology 86, 2874–2880. doi: 10.1890/04-1852
Kähler, O. (2010). Combining peer review and metrics to assess journals for inclusion in Scopus. Learn. Publ. 23, 336–346. doi: 10.1087/20100411
Karlson, R., and Hurd, L. (1993). Disturbance, coral reef communities, and changing ecological paradigms. Coral Reefs 12, 117–125. doi: 10.1007/BF00334469
Kolasinski, J., Nahon, S., Rogers, K., Chauvin, A., Bigot, L., and Frouin, P. (2016). Stable isotopes reveal spatial variability in the trophic structure of a macro-benthic invertebrate community in a tropical coral reef. Rapid Commun. Mass Spectrom. 30, 433–446. doi: 10.1002/rcm.7443
Kramer, M. J., Bellwood, O., and Bellwood, D. R. (2013). The trophic importance of algal turfs for coral reef fishes: the crustacean link. Coral Reefs 32, 575–583. doi: 10.1007/s00338-013-1009-1
Kruse, M., Taylor, M., Muhando, C. A., and Reuter, H. (2016). Lunar, diel, and tidal changes in fish assemblages in an East African marine reserve. Regul. Stud. Mar. Sci. 3, 49–57. doi: 10.1016/j.rsma.2015.05.001
Lamy, T., Galzin, R., Kulbicki, M., Lison de Loma, T., and Claudet, J. (2016). Three decades of recurrent declines and recoveries in corals belie ongoing change in fish assemblages. Coral Reefs 35, 293–302. doi: 10.1007/s00338-015-1371-2
Lapointe, B. E. (1999). Simultaneous top-down and bottom-up forces control macroalgal blooms on coral reefs (Reply to the comment by Hughes et al.). Limnol. Oceanog. 44, 1586–1592. doi: 10.4319/lo.1999.44.6.1586
Layman, C. A. (2007). What can stable isotope ratios reveal about mangroves as fish habitat? Bull. Mar. Sci. 80, 513–527.
Layman, C. A., Araujo, M. S., Boucek, R., Hammerschlag-Peyer, C. M., Harrison, E., Jud, Z. R., et al. (2012). Applying stable isotopes to examine food-web structure: an overview of analytical tools. Biol. Rev. Camb. Philos. Soc. 87, 545–562. doi: 10.1111/j.1469-185X.2011.00208.x
Layman, C. A., Arrington, D. A., Montaña, C. G., and Post, D. M. (2007). Can stable isotope ratios provide for community-wide measures of trophic structure? Ecology 88, 42–48. doi: 10.1890/0012-9658(2007)88[42:CSIRPF]2.0.CO;2
Lewis, S. M., and Wainwright, P. C. (1985). Herbivore abundance and grazing intensity on a Caribbean coral reef. J. Exp. Mar. Biol. Ecol. 87, 215–228. doi: 10.1016/0022-0981(85)90206-0
Li, Y., Zhang, Y., Hussey, N. E., and Dai, X. (2016). Urea and lipid extraction treatment effects on δ15N and δ13C values in pelagic sharks. Rapid Commun. Mass. Spectrom. 30, 1–8. doi: 10.1002/rcm.7396
Libralato, S., Pranovi, F., Stergiou, K. I., and Link, J. S. (2014). Trophodynamics in marine ecology: 70 years after Lindeman Introduction. Mar. Ecol. Prog. Ser. 512, 1–7. doi: 10.3354/meps11033
Lin, Y., Michel, J.-B., Aiden, E. L., Orwant, J., Brockman, W., and Petrov, S. (2012). Syntactic annotations for the google books ngram corpus,” in Proceedings of the ACL 2012 System Demonstrations. Association for Computational Linguistics (Jeju).
Lindeman, R. L. (1942). The trophic-dynamic aspect of ecology. Ecology 23, 399–417. doi: 10.2307/1930126
Liu, P. J., Shao, K. T., Jan, R. Q., Fan, T. Y., Wong, S. L., Hwang, J. S., et al. (2009). A trophic model of fringing coral reefs in Nanwan Bay, southern Taiwan suggests overfishing. Mar. Environ. Res. 68, 106–117. doi: 10.1016/j.marenvres.2009.04.009
Marnane, M. J., and Bellwood, D. R. (2002). Diet and nocturnal foraging in cardinalfishes (Apogonidae) at One Tree Reef, Great Barrier Reef, Australia. Mar. Ecol. Prog. Ser. 231, 261–268. doi: 10.3354/meps231261
Matley, J., Heupel, M., Fisk, A., Simpfendorfer, C., and Tobin, A. (2016). Measuring niche overlap between co-occurring Plectropomus spp. using acoustic telemetry and stable isotopes. Mar. Freshw. Res. 68, 1468–1478. doi: 10.1071/MF16120
McCauley, D. J., Young, H. S., Dunbar, R. B., Estes, J. A., Semmens, B. X., and Michel, F. (2012). Assessing the effects of large mobile predators on ecosystem connectivity. Ecol. Appl. 22, 1711–1717. doi: 10.1890/11-1653.1
McClanahan, T., and Branch, G. (2008). Food Webs and the Dynamics of Marine Reefs. New York, NY: Oxford University Press, 79–107.
McClanahan, T. R., Muthiga, N. A., and Coleman, R. A. (2011). Testing for top-down control: can post-disturbance fisheries closures reverse algal dominance? Aquat. Conserv. Mar. Freshw. Ecosyst. 21, 658–675. doi: 10.1002/aqc.1225
McIntosh, R. P. (1986). The Background of Ecology: Concept and Theory. New York, NY: Cambridge University Press, 378.
McMahon, K. W., Thorrold, S. R., Houghton, L. A., and Berumen, M. L. (2015). Tracing carbon flow through coral reef food webs using a compound-specific stable isotope approach. Oecologia 180, 809–821. doi: 10.1007/s00442-015-3475-3
Michener, R. H., Kaufman, L., Michener, R., and Lajtha, K. (2007). Stable isotope ratios as tracers in marine food webs: an update. Stab. Isotop. Ecol. Environ. Sci. 2, 238–282. doi: 10.1002/9780470691854.ch9
Mourier, J., Maynard, J., Parravicini, V., Ballesta, L., Clua, E., Domeier, M. L., et al. (2016). Extreme inverted trophic pyramid of reef sharks supported by spawning groupers. Curr. Biol. 26, 2011–2016. doi: 10.1016/j.cub.2016.05.058
Mumby, P. J., Foster, N. L., and Fahy, E. A. G. (2005). Patch dynamics of coral reef macroalgae under chronic and acute disturbance. Coral Reefs 24, 681–692. doi: 10.1007/s00338-005-0058-5
Mumby, P. J., Harborne, A. R., Williams, J., Kappel, C. V., Brumbaugh, D. R., Micheli, F., et al. (2007). Trophic cascade facilitates coral recruitment in a marine reserve. Proc. Natl. Acad. Sci. U.S.A. 104, 8362–8367. doi: 10.1073/pnas.0702602104
Nagelkerken, I., Dorenbosch, M., Verberk, W., De La Morinière, E. C., and Van Der Velde, G. (2000). Day-night shifts of fishes between shallow-water biotopes of a Caribbean bay, with emphasis on the nocturnal feeding of Haemulidae and Lutjanidae. Mar. Ecol. Prog. Ser. 194, 55–64. doi: 10.3354/meps194055
Navia, A. F., Cruz-Escalona, V. H., Giraldo, A., and Barausse, A. (2016). The structure of a marine tropical food web, and its implications for ecosystem-based fisheries management. Ecol. Modell. 328, 23–33. doi: 10.1016/j.ecolmodel.2016.02.009
Navia, A. F., Mejía-Falla, P. A., López-García, J., Giraldo, A., and Cruz-Escalona, V. H. (2017). How many trophic roles can elasmobranchs play in a marine tropical network? Mar. Freshw. Res. 68, 1342–1353. doi: 10.1071/MF16161
Newman, S. J., Williams, D. M., and Russ, G. R. (1997). Patterns of zonation of assemblages of the Lutjanidae, Lethrinidae and Serranidae (Epinephelinae) within and among mid-shelf and outer-shelf reefs in the central Great Barrier Reef. Mar. Freshw. Res. 48, 119–128. doi: 10.1071/MF96047
Odum, E. P., and Barrett, G. W. (1971). Fundamentals of Ecology, 5th Edn. Philadelphia, PA: Thomson Brooks/Cole.
Odum, E. P., and Kuenzler, E. J. (1955). Measurement of territory and home range size in birds. Auk 72, 128–137. doi: 10.2307/4081419
Opschoor, J. H. B. (1995). Ecospace and the fall and rise of throughput intensity. Ecol. Econ. 15, 137–140. doi: 10.1016/0921-8009(95)00070-4
Paine, R. T. (1966). Food web complexity and species diversity. Am. Nat. 100, 65–75. doi: 10.1086/282400
Paine, R. T. (1980). Food webs: linkage, interaction strength and community infrastructure. J. Anim. Ecol. 49, 667–685. doi: 10.2307/4220
Paula, D. P., Linard, B., Crampton-Platt, A., Srivathsan, A., Timmermans, M. J., Sujii, E. R., et al. (2016). Uncovering trophic interactions in arthropod predators through DNA shotgun-sequencing of gut contents. PLoS ONE 11:e0161841. doi: 10.1371/journal.pone.0161841
Paulay, G. (1997). “Diversity and distribution of reef organisms,” in Life and Death of Coral Reefs, ed C. Birekland (New York, NY: Chapman and Hall), 298–353.
Pinnegar, J. K., Polunin, N. V. C., Francour, P., Badalamenti, F., Chemello, R., Harmelin-Vivien, M. L., et al. (2000). Trophic cascades in benthic marine ecosystems: lessons for fisheries and protected-area management. Environ. Conserv. 27, 179–200. doi: 10.1017/S0376892900000205
Polovina, J. J. (1984). Model of a coral-reef ecosystem.1. The ecopath model and its application to french frigate shoals. Coral Reefs 3, 1–11. doi: 10.1007/BF00306135
Post, D. M. (2002). Using stable isotopes to estimate trophic position: models, methods, and assumptions. Ecology 83, 703–718. doi: 10.1890/0012-9658(2002)083[0703:USITET]2.0.CO;2
Rasher, D. B., Hoey, A. S., and Hay, M. E. (2017). Cascading predator effects in a Fijian coral reef ecosystem. Sci. Rep. 7:15684. doi: 10.1038/s41598-017-15679-w
Rizzari, J. R., Bergseth, B. J., and Frisch, A. J. (2015). Impact of conservation areas on trophic interactions between apex predators and herbivores on coral reefs. Conserv. Biol. 29, 418–429. doi: 10.1111/cobi.12385
Rizzari, J. R., Frisch, A. J., and Connolly, S. R. (2014). How robust are estimates of coral reef shark depletion? Biol. Conserv. 176, 39–47. doi: 10.1016/j.biocon.2014.05.003
Roff, G., Doropoulos, C., Rogers, A., Bozec, Y.-M., Krueck, N. C., Aurellado, E., et al. (2016a). The ecological role of sharks on coral reefs. Trends Ecol. Evol. 31, 395–407. doi: 10.1016/j.tree.2016.02.014
Roff, G., Doropoulos, C., Rogers, A., Bozec, Y.-M., Krueck, N. C., Aurellado, E., et al. (2016b). Reassessing shark-driven trophic cascades on coral reefs: a Reply to Ruppert et al. Trends Ecol. Evol. 31, 587–589. doi: 10.1016/j.tree.2016.05.005
Ruppert, J. L., Fortin, M. J., and Meekan, M. G. (2016). The ecological role of sharks on coral reefs: response to Roff et al. Trends Ecol. Evol. 31, 586–587. doi: 10.1016/j.tree.2016.05.003
Ruppert, J. L., Travers, M. J., Smith, L. L., Fortin, M. J., and Meekan, M. G. (2013). Caught in the middle: combined impacts of shark removal and coral loss on the fish communities of coral reefs. PLoS ONE 8:e74648. doi: 10.1371/journal.pone.0074648
Ryther, J. H. (1969). Photosynthesis and fish production in the sea. The production of organic matter and its conversion to higher forms of life vary throughout the world ocean. Science 166, 72–76. doi: 10.1126/science.166.3901.72
Sale, P. F. (2002). “The science we need to develop for more effective management. Coral reef fishes dynamics and diversity in a complex ecosystem,” in The Ecology of Fishes on Coral Reefs, ed P. F. Sale PF (San Diego, CA: Academic Press), 361–376.
Sandin, S. A., Smith, J. E., DeMartini, E. E., Dinsdale, E. A., Donner, S. D., Friedlander, A. M., et al. (2008). Baselines and degradation of coral reefs in the Northern Line Islands. PLoS ONE 3:e1548. doi: 10.1371/journal.pone.0001548
Sandin, S. A., and Zgliczynski, B. J. (2015). “Inverted trophic pyramids,” in The Ecology of Fishes on Coral Reefs, ed C. Mora (New York, NY: Cambridge University Press), 247.
Scheffer, M., Van Nes, E. H., Holmgren, M., and Hughes, T. (2008). Pulse-driven loss of top-down control: the critical-rate hypothesis. Ecosystems 11, 226–237. doi: 10.1007/s10021-007-9118-8
Sikkel, P. C., Welicky, R. L., Artim, J. M., McCammon, A. M., Sellers, J. C., Coile, A. M., et al. (2017). Nocturnal migration reduces exposure to micropredation in a coral reef fish. Bull. Mar. Sci. 93, 475–489. doi: 10.5343/bms.2016.1021
Simpfendorfer, C. A., and Heupel, M. R. (2016). Ecology: the upside-down world of coral reef predators. Curr. Biol. 26, R708–R710. doi: 10.1016/j.cub.2016.05.074
Smith, J. E., Hunter, C. L., and Smith, C. M. (2010). The effects of top–down versus bottom–up control on benthic coral reef community structure. Oecologia 163, 497–507. doi: 10.1007/s00442-009-1546-z
Stallings, C. D. (2008). Indirect effects of an exploited predator on recruitment of coral-reef fishes. Ecology 89, 2090–2095. doi: 10.1890/07-1671.1
Stamoulis, K. A., Friedlander, A. M., Meyer, C. G., Fernandez-Silva, I., and Toonen, R. J. (2017). Coral reef grazer-benthos dynamics complicated by invasive algae in a small marine reserve. Sci. Rep. 7:43819. doi: 10.1038/srep43819
Symondson, W. (2002). Molecular identification of prey in predator diets. Mol. Ecol. 11, 627–641. doi: 10.1046/j.1365-294X.2002.01471.x
Terborgh, J. W. (2015). Toward a trophic theory of species diversity. Proc. Natl. Acad. Sci. U.S.A. 112, 11415–11422. doi: 10.1073/pnas.1501070112
Thierry, H., Sheeren, D., Marilleau, N., Corson, N., Amalric, M., and Monteil, C. (2015). From the Lotka–Volterra model to a spatialised population-driven individual-based model. Ecol. Modell. 306, 287–293. doi: 10.1016/j.ecolmodel.2014.09.022
Thillainath, E. C., McIlwain, J. L., Wilson, S. K., and Depczynski, M. (2016). Estimating the role of three mesopredatory fishes in coral reef food webs at Ningaloo Reef, Western Australia. Coral Reefs 35, 261–269. doi: 10.1007/s00338-015-1367-y
Trebilco, R., Baum, J. K., Salomon, A. K., and Dulvy, N. K. (2013). Ecosystem ecology: size-based constraints on the pyramids of life. Trends Ecol. Evol. 28, 423–431. doi: 10.1016/j.tree.2013.03.008
Turney, S., and Buddle, C. M. (2016). Pyramids of species richness: the determinants and distribution of species diversity across trophic levels. Oikos 125, 1224–1232. doi: 10.1111/oik.03404
Vance, G. E. F. R. R., and Steele, M. A. (2002). “Simulating large-scale population dynamics using small-scale data,” in The Ecology of Fishes on Coral Reefs, ed P. F. Sale (San Diego, CA: Academic Press), 275–301
Verity, P. G., and Smetacek, V. (1996). Organism life cycles, predation, and the structure of marine pelagic ecosystems. Mar. Ecol. Prog. Ser. 130, 277–293. doi: 10.3354/meps130277
Walters, C., Christensen, V., Fulton, B., Smith, A. D., and Hilborn, R. (2016). Predictions from simple predator-prey theory about impacts of harvesting forage fishes. Ecol. Modell. 337, 272–280. doi: 10.1016/j.ecolmodel.2016.07.014
Weijerman, M., Fulton, E. A., Kaplan, I. C., Gorton, R., Leemans, R., Mooij, W. M., et al. (2015). An integrated coral reef ecosystem model to support resource management under a changing climate. PLoS ONE 10:e0144165. doi: 10.1371/journal.pone.0144165
Wen, C. K. C., Bonin, M. C., Harrison, H. B., Williamson, D. H., and Jones, G. P. (2016). Dietary shift in juvenile coral trout (Plectropomus maculatus) following coral reef degradation from a flood plume disturbance. Coral Reefs 35, 451–455. doi: 10.1007/s00338-016-1398-z
Keywords: trophodynamics, coral reefs, ecological roles, trophic ecology, food webs
Citation: Bierwagen SL, Heupel MR, Chin A and Simpfendorfer CA (2018) Trophodynamics as a Tool for Understanding Coral Reef Ecosystems. Front. Mar. Sci. 5:24. doi: 10.3389/fmars.2018.00024
Received: 21 January 2017; Accepted: 18 January 2018;
Published: 06 February 2018.
Edited by:
Hajime Kayanne, The University of Tokyo, JapanReviewed by:
Paul Carl Sikkel, Arkansas State University, United StatesDavid Chagaris, University of Florida, United States
Copyright © 2018 Bierwagen, Heupel, Chin and Simpfendorfer. This is an open-access article distributed under the terms of the Creative Commons Attribution License (CC BY). The use, distribution or reproduction in other forums is permitted, provided the original author(s) and the copyright owner are credited and that the original publication in this journal is cited, in accordance with accepted academic practice. No use, distribution or reproduction is permitted which does not comply with these terms.
*Correspondence: Stacy L. Bierwagen, c3RhY3kuYmllcndhZ2VuQG15LmpjdS5lZHUuYXU=