- 1Department of Biology, California State University, Northridge, Northridge, CA, United States
- 2Sesoko Station, Tropical Biosphere Research Center, University of the Ryukyus, Motobu, Japan
- 3Faculty of Science, University of the Ryukyus, Nishihara, Japan
Elevated pCO2 threatens coral reefs through impaired calcification. However, the extent to which elevated pCO2 affects the distribution of the pelagic larvae of scleractinian corals, and how this may be interpreted in the context of ocean acidification (OA), remains unknown. We tested the hypothesis that elevated pCO2 affects one aspect of the behavior (i.e., motility) of brooded larvae from Pocillopora damicornis in Okinawa (Japan), and used UV-transparent tubes that were 68-cm long (45 mm ID) to incubate larvae on a shallow fringing reef. Replicate tubes were filled with seawater at ~400 μatm or ~1,000 μatm pCO2, stocked with 50 larvae each, and incubated vertically for 12 h with their midpoints at 0.3-m (shallow) or 3.3-m (deep) depth over a reef at 4-m depth. Larval behavior was assayed through their position in the tubes, which was scored in situ every 4 h beginning at 08:00 h. Lipid content was measured at the end of the experiment as a potential driver of behavior through its effects on larval buoyancy. Larval position in the tubes varied between depths and time of day at ~400 μatm pCO2 and ~1,000 μatm pCO2. At ~400 μatm, larvae moved toward the top (0.1-m) of shallow tubes throughout the day, but in the deep tubes they aggregated at the bottom of the tubes from 08:00 to 20:00 h. In contrast, larvae incubated at ~1,000 μatm pCO2 aggregated at the bottom of shallow tubes at 08:00 and 20:00 h, however in the deep tubes they were found in the bottom throughout the day. As lipid content of the larvae declined 23–25% at ~1,000 vs. ~400 μatm pCO2, loss of lipid may be a cause of modified larval behavior at high pCO2. If the pCO2-mediated changes in behavior and lipid content during this short experiment occur during longer exposures to high pCO2, our results suggest OA could alter the dispersal capacity of brooded coral larvae.
Introduction
Understanding the processes contributing to the distribution of organisms is a cornerstone of ecology (Hastings and Harrison, 1994), and in the marine environment, behaviorally-modulated dispersal of pelagic larvae plays a critical role in determining the spatial distribution of benthic populations (Levin, 2006). Larval behavior contributes to the capacity for pelagic larvae to disperse, which influences gene flow and connectivity among populations (Cowen and Sponaugle, 2009). To quantify the movement of pelagic larvae in the marine environment, their vertical position in the water column must be measured with a high degree of temporal resolution (i.e., hourly), because their vertical position can change within hours (Harii et al., 2002), and vertical changes as small as 50–100 cm can provide exposure to seawater with differing velocities (Szmant and Meadows, 2006) that are likely to vary throughout the day (e.g., with the tidal cycle). High-resolution descriptions of the vertical position of larvae in the water column requires analyses of their ability to swim, their intrinsic buoyancy, and environmental conditions (e.g., seawater density, temperature, and vertical advection) that together translate into the capacity for dispersal (Metaxas, 2001; Levin, 2006; Gleason and Hofmann, 2011).
The aspects of larval behavior modulating their vertical migration through swimming and buoyancy as a function of environmental conditions and ontogeny (sensu Paris and Cowen, 2004) have been extensively studied in marine organisms (Chia et al., 1984). For coral reef organisms, behaviorally-mediated dispersal of pelagic larvae plays a strong role in determining benthic and pelagic community structure (reviewed in Jones et al., 2009), with these effects evident over areas as small as ≤ 300 m2 (Jones et al., 2005; Almany et al., 2007) and as big as tens-to-hundreds of kilometers through connectivity mechanisms (Cowen et al., 2006). The framework-builders of coral reefs (i.e., scleractinians) are sessile as adults, but like reef fishes (Doherty et al., 1985) and many other marine benthic invertebrates (Thorson, 1950), are dependent on pelagic larvae for dispersal (Harrison and Wallace, 1990). The dispersal of pelagic larvae from undisturbed to disturbed coral reefs is a key factor mediating the recovery of coral populations that have been severely depleted in abundance (van Oppen et al., 2008). One main finding in studies on connectivity among benthic communities on coral reefs is strong spatio-temporal heterogeneity (Cowen and Sponaugle, 2009), which is an enduring feature of community ecology (Cornell and Lawton, 1992) that also emerges in long-term analyses of community structure (Edmunds, 2000; Bruno and Selig, 2007; Pratchett et al., 2011) and studies of the settlement choices of sessile invertebrate larvae (Whalan et al., 2015).
Environmentally-induced changes in the motility of pelagic larvae are likely to affect their vertical distribution in the seawater column, thereby exposing them to differing abiotic factors (e.g., vertically stratified seawater flow) that can modify the direction, distance, or speed of their dispersal. Changes in larval settlement behavior (e.g., delayed timing of peak settlement, extended settlement window) may also increase the dispersal potential of larvae based on their vertical distribution (Platygyra sinensis, Tay et al., 2011). In shallow coastal seawater, even a change in vertical distribution of a few decimeters can expose larvae to different seawater velocities, since seawater motion in such habitats typically is vertically stratified on a commensurate scale (Cowen and Sponaugle, 2009). For example, wind driven surface currents can cause the 5-cm thick surface layer of seawater over a shallow coral reef to move faster, and in the opposite direction, than seawater at 50–100 cm depth (Harii and Kayanne, 2003). Vertical migration and subsequent advection can subject coral larvae to different environmental conditions compared to the benthic adults (Hughes et al., 2000).
Although the pelagic larvae of many marine organisms are provisioned with maternally-derived defenses against environmental stress (Hamdoun and Epel, 2007), and therefore may be more resistant to stress than their parents (Haryanti et al., 2015), there is evidence that the larvae of at least some tropical scleractinians are more vulnerable than adults to changing environmental conditions (Putnam et al., 2010; Byrne, 2011). For pelagic coral larvae, one aspect of their biology that has not been tested for a response to high pCO2 is their behavior in the swimming phase. While various physiological responses to high pCO2, such as increased size (Putnam et al., 2013) or depressed metabolic rates (Rivest and Hofmann, 2014), have been observed in coral larvae, analysis of their behavior in the swimming phase has yet to be conducted to evaluate the implications of elevated pCO2 for their dispersal.
In this study, the behavior of brooded scleractinian larvae under contrasting pCO2 regimes (ambient and elevated) was investigated at two depths to gain insight into the possibility that their dispersal will change in future oceans characterized by higher seawater pCO2 than present-day. Using experiments conducted on the shallow reefs of Okinawa (Japan), we tested the hypothesis that vertical movement of larvae from Pocillopora damicornis is affected by high pCO2 (~1,000 μatm). P. damicornis was chosen since it is a brooding scleractinian coral that releases planula larvae on a monthly lunar cycle. Larvae were incubated in acrylic tubes filled with seawater at either ambient or high pCO2, and suspended vertically in the seawater above a fringing reef. First, the vertical position of the larvae in the tubes was scored over 12 h to test their response to a contrast of ambient vs. elevated pCO2 in shallow water. The experiment was repeated with the tubes incubated in deeper water, and combination of the two experiments provided an orthogonal contrast of the effects of pCO2 and depth on larval behavior. Second, the hypothesis that the total lipid content of the larvae would change under elevated pCO2 vs. ambient pCO2 was tested to explore the possibility that lipid metabolism mediated the vertical position of the larvae in seawater. Lipids play a role in the buoyancy regulation of a wide variety of planktonic marine organisms (Campbell and Dower, 2003), so we reasoned that modulation of lipid content in larvae from P. damicornis could modify their vertical position in the water column by buoyancy effects (Harii et al., 2002).
Materials and Methods
Twelve colonies of Pocillopora damicornis (Linnaeus 1758) were collected in July and August 2016 from ~1-m depth on a patch reef on the northwest shore of Okinawa (26°40′18.24′′ N, 127°53′4.78′′ E). Colonies were collected prior to expected larval release in Okinawa in both months (S. Harii, unpublished data on the study site), in which peak release occurred ~7 days after the new moon. At the times of collection, the ambient seawater temperature at the study site was ~29°C.
Following collection, colonies were transferred to Sesoko Station, part of the Tropical Biosphere Research Center University of the Ryukyus, where they were incubated outdoors in individual containers exposed to natural irradiance in flow-through seawater. Ambient seawater was pumped at 3.0 L min−1 (AC Flowmeter, Tokyo Keiso Co., Japan) from 4 to 5-m depth and stored in two 10-L reservoirs. Air was bubbled constantly in to the reservoirs at 3.0 L min−1 to maintain ambient seawater pCO2 (i.e., the control conditions). Ambient seawater temperature was measured hourly at 1–2-m depth near the collection site prior to, and during, the experiment (HOBO Pro v2, Onset Computer Corporation, USA), and was 29.9 ± 0.2°C (mean ± SE, n = 45 days), with a daily minimum of 28.3°C and daily maximum of 31.8°C (R. Prasetia and S. Harii, unpublished data) that reflects summertime diurnal warming in this location (Takahashi and Kurihara, 2013). Temperature in the containers holding the corals was maintained within this range during the experiment (29.8 ± < 0.2°C, mean ± SE, n = 31) using a chiller (ZR-130E, Zensui, Japan).
Planulae released from P. damicornis during the first quarter moon of July and August were collected at ~05:00 h following their release at ~03:00 h (Isomura and Nishihira, 2001), using containers lined with 110 μm plankton mesh. Larval color and approximate size were evaluated by eye. As larvae from P. damicornis are physiologically dissimilar among days of release (Fan et al., 2002; Cumbo et al., 2013), larvae were collected from the inferred day of peak release and pooled among colonies releasing larvae on this day. Larvae from July and August were used to test the effects of pCO2 (two levels) and depths (two levels) on larval behavior, and the experiment was conducted in two parts. The first part (July 2016) tested the effects of two pCO2 regimes on larval behavior with the tubes positioned with their upper opening adjacent to the air-water interface of the seawater (hereafter “shallow” tubes), and the second part (August 2016) tested the effects of the same two pCO2 regimes on larval behavior with the tubes positioned with their upper opening ~3–4 m below the surface (hereafter “deep” tubes).
Response of Larval Behavior to High pCO2
To determine the position of P. damicornis larvae in the seawater in response to high pCO2, larvae were transferred from collection containers to UV-transparent acrylic tubes (UV-T, ACRYLITE Colorless 0070 GT, Evonik Industries, New Jersey, USA) and incubated in situ at two depths, which centered the tubes vertically at ~0.3 m (July 2016) or ~3.3 m (August 2016). The tubes had a wall thickness of 6 mm, were 68-cm long with an inner diameter of 4.5 cm, and were sealed at either end with UV-T acrylic caps. A 6-mm hole was drilled in the top cap so the tubes could be filled with seawater; the hole was later covered with vinyl electrical tape. While the objective was to completely fill the tubes with seawater, in most cases small bubbles were introduced during the filling process and combined when the tubes were positioned vertically to create an air gap of ≤ 1-cm height at the top of the tube. The acrylic in these tubes transmitted ~92% of ultraviolet radiation (UV-R) (280–400 nm) (J. Bergman, unpublished data), ensuring larvae were exposed to ecologically relevant light conditions for shallow seawater (Gleason and Hofmann, 2011). Previous studies of the vertical movement of P. damicornis larvae in acrylic tubes (Harii et al., 2002), as well as preliminary observations of larval behavior used in the present study, show that larvae of P. damicornis exposed to ambient seawater generally aggregate either in the top or bottom 0–2 cm of tubes regardless of light conditions (i.e., light vs. dark). Therefore, the vertically-oriented tubes were marked into two sections for the purpose of scoring the larvae by position: an upper section (21-cm length) and lower section (47-cm length), since we reasoned it would be unlikely that the larvae would accumulate in the center of the tubes. Of the larvae found in the lower section, 83–88% were located in the bottom 23 cm of the tube, which suggested that the greater size of the lower scoring section in the tubes did not upwardly bias estimates of larvae scored as moving downward.
Eight tubes were used for each experiment, during which four were filled with ambient (~400 μatm pCO2) seawater, and four with treatment (~1,000 μatm pCO2) seawater that simulated the elevated pCO2 conditions predicted to occur by 2100 under a pessimistic scenario of human activity [RCP6.0 (Moss et al., 2010; Intergovernmental Panel on Climate Change, 2014)]. Once tubes were filled with seawater, 50 larvae were haphazardly selected from the larval stock obtained by pooling larvae released that morning from maternal colonies, and added using a Pasteur pipette through the 6-mm hole in the cap of the tube. The hole was sealed when the tubes were stocked with larvae. Each tube was carefully inverted to gently mix the 50 larvae, so each trial began with the larvae scattered along the length of the tube. Although the pelagic larval duration (PLD) of P. damicornis can be >100 d (Richmond, 1987), their larvae commonly settle ≤ 24 h following release (Richmond, 1987; Isomura and Nishihira, 2001; Harii et al., 2002; but see Cumbo et al., 2012), and therefore, the present incubations were designed to last 12 h starting at 08:00 h.
After the tubes were stocked with larvae on the shore, they were transported by a snorkeler to an adjacent fringing reef and suspended vertically, with the midpoint of the tubes at a median depth (MD) of either ~0.3 m (July) or 3.3 m (August) (hereafter referred to as 0.3 and 3.3-m MD) (Figure 1). The median depth was recorded at the middle of the vertically oriented tubes, thus the depth range for the 0.3 m MD tubes extended from the surface to 0.7 m, and from 3 to 3.7 m for the 3.3 m MD tubes. To maintain this configuration, the tubes were attached to a weight at 5-m depth, and a float was used to keep them suspended vertically. While it is not possible to exclude an effect of time (July vs. August) in confounding the contrast of depth, experimental conditions were kept virtually identical between months to reduce temporal bias. Tubes were stocked with larvae shortly after sunrise, and were installed on the reef within 30 min of filling at ~07:30 h. Thereafter, the vertical position of the larvae was scored every 4 h starting at 08:00 h (initial) and finishing at ~19:30–20:00 h (sunset); the tubes were removed from the water at 08:00 h the following day and the larvae processed for lipid content (described below). At each census, the position of the larvae in the tubes was scored as the number of larvae in either the top or bottom sections of the tube. Tubes were not evaluated at night because of logistical constraints associated with nighttime snorkeling. The percentage of the total number of larvae present in the tubes at the time of each sampling was calculated for the top section of the tubes and used as the response variable to evaluate the effects of the treatments (depth and pCO2).
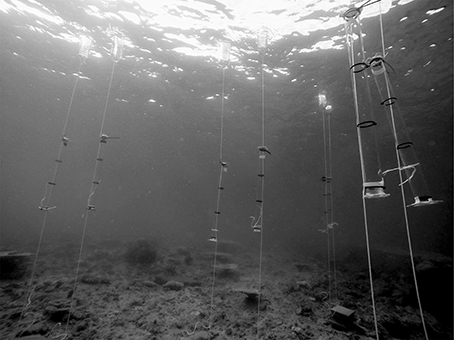
Figure 1. Photograph of 68-cm long tubes deployed in situ at ~3.3-m MD on the shallow fringing reef near Sesoko Station in August 2016. Ambient and high pCO2 treatments were haphazardly distributed among 8 tubes.
Carbonate Chemistry
High pCO2 seawater was prepared in a single 10 L plastic reservoir by bubbling air or pure CO2 gas into seawater using a mass-flow controller (HORIBASTEC, SEC-E40, Japan) that supplied gas at 16 mL min−1. Adjustments of ± 0.1 mL min−1 in the flow rate of CO2 gas were made manually as necessary each morning leading up to the experiment to maintain the desired pCO2, as evaluated from daily measurements of seawater pH and total alkalinity (AT) used to calculate seawater pCO2 using CO2SYS software (Lewis and Wallace, 1998). Seawater pH and temperature in the reservoir were measured daily between 09:00 and 11:00 h using a handheld meter (Multi 3410, WTW, Germany) fitted with a combination probe that recorded pH (±0.001 pH unit) and temperature (±0.1°C) (SenTix 940, WTW, Germany). The probe was calibrated daily prior to use with three NBS buffers (Nacalai Tesque, Japan). The salinity of the seawater used to fill the larval incubation tubes was measured using a conductivity meter (TetraCon 325, WTW, Germany), and AT was determined using open-cell titrations conducted with an autoburette titrator (Kimoto, ATT-05, Japan). Titrations of certified reference materials (batch 155) provided by A.G. Dickson (Scripps Institute of Oceanography) yielded AT values that differed on average ≤ 1.3 μmol kg−1 from the certified value (SE = 9.2 μmol kg−1, n = 6). Seawater pCO2, , , and Ωarag were calculated from pH, temperature, AT, and salinity using CO2SYS (Lewis and Wallace, 1998) with dissociation constants K1 and K2 from Mehrbach et al. (1973).
Lipid Content
To test for changes in total lipid content in the larvae following incubations under ambient and high pCO2, the initial lipid content of larvae was determined in three samples of 50 larvae, freshly released from the maternal colonies at 06:00 h and pooled among them. Each batch of larvae was placed in 1.5-mL vials for processing. The final lipid content of the larvae (i.e., 24 h after the start of incubations) was determined by processing batches of larvae in the same way following 24 h in ambient or high pCO2 in either shallow (July) or deep (August) locations. Tubes incubated on the reef were returned to the lab 24 h after the start of each experiment (i.e., 08:00 h the following day), and all living larvae were collected by rinsing the contents of each tube into a collection container. Retrieved larvae were counted, and a single batch of 50 larvae from each tube at both depths was placed in a 1.5 mL vial for measurement of lipid content.
After transferring the batches of larvae to 1.5-mL vials, excess seawater was removed from the vials using a Pasteur pipette, and the larvae were frozen at −80°C for lipid analysis within 2–4 weeks. Following the protocol of Harii et al. (2010), modified by lengthening the extraction time in an ultrasonic bath to 15 min, lipids were extracted with 5 mL dichloromethane-methanol (6:4) at room temperature. Each batch of 50 larvae was extracted three times, with the solvent and eluted lipid pooled among extracts. Extracted lipids were concentrated in a rotary evaporator for 15–20 min, the residue was dissolved in dichloromethane 6 times, and then centrifuged to separate lipids from any remaining water. The solvent containing dissolved lipids was transferred to a new 50 mL flask after each separation. After the replicate dissolutions in dichloromethane, the samples were partially evaporated once again for ~5 min, and passed through a ~1 cm column of Na2SO4 crystals and plastic wool to remove any remaining water. Samples drained by gravity from the column into 4-mL pre-combusted glass vials (400°C for 4 h), in which they were then dried under nitrogen gas and weighed using a microbalance (±1 μg, MT5, Mettler-Toledo, USA). All solvents used were American Chemical Society (ACS) Guaranteed Reagent (GR) grade. Larval lipid content was expressed as μg larva−1. The extraction efficiency of lipids was evaluated by comparing the lipid content of control larvae with the known lipid content of P. damicornis (Rivest and Hofmann, 2015).
Statistical Analysis
Statistical analyses were conducted using SYSTAT Version 11 software (Systat Software, San Jose, CA). A two-factor Repeated Measures (RM) ANOVA was used to compare the effects of depth and pCO2 on larval position in the tubes, with time of day as the RM factor, and arcsine-transformed values of the percentage of larvae found in the top of each tube as the dependent variable. Post-hoc analyses were conducted as paired t-tests for tubes at each depth and treatment combination, testing for differences between sequential time points using an α = 0.05/3 for three contrasts within each group. Differences in lipid content of larvae were evaluated using a two-sample t-test to compare the effect of pCO2 on total lipid between depths, and a Kruskal-Wallis non-parametric test (due to violations of normality in the data) to compare lipid content after pCO2 incubations regardless of month. Assumptions of normality and homogeneity of variance for the RM-ANOVA were assessed through graphical analyses of the residuals.
Results
Four hundred larvae were used in July and August for incubations in the eight tubes. Larvae were pale brown and prolate spheroid in shape, actively swam throughout incubations, and behaved similarly to each other following release from maternal colonies in July and August (i.e., before each test of the effect of depth). Larvae used at both depths were collected on lunar day 7, which corresponded to July 10th for 0.3-m MD, and August 8th for 3.3-m MD. Seawater temperature in the 14 days prior to larval release did not statistically differ (2-sample t-test, t = 0.617, df = 26, p = 0.118) with a grand mean (pooled among those 14 days in each month) of 29.1 ± 0.1°C (±SE, n = 28 days) (S. Harii, unpublished data).
Behavior of Larvae in High pCO2
The pCO2 in the tubes did not differ between the start and end of the 24 h incubations at both 0.3-m MD (t = 2.550, df = 5, p = 0.238) and 3.3-m MD (t = 0.007, df = 6, p = 0.995) (Table 1). Pooling pCO2 treatment levels between depths, and including both initial and final (24 h later) measurements of seawater chemistry, mean treatment levels were 1,060 ± 31 μatm pCO2 and 356 ± 13 μatm pCO2 (n = 15, ± SE). Larvae incubated in high pCO2 retained their shape and pale brown color at both depths, and visually were indistinguishable from larvae incubated under control conditions. One treatment incubation tube at 0.3-m MD broke prior to the larval census at 16:00 h, and exclusion of this tube from the statistical analysis provided too few observations to test all interactions in the model. To address this limitation, observations on larval distribution at 16:00 and 20:00 h for the damaged tube were replaced with an imputed mean value calculated from the remaining 3 tubes in the same treatment group, and the degrees of freedom were reduced by one for each replacement (Quinn and Keough, 2002; Zar, 2010).
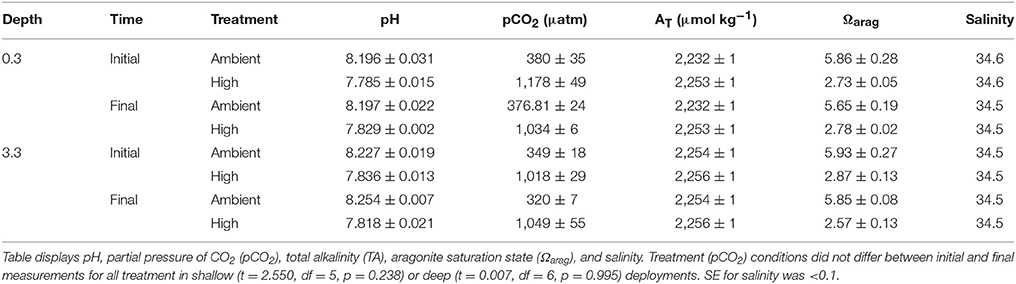
Table 1. Mean carbonate chemistry parameters (mean ± SE, n = 3–4) in sealed vertical tubes in Okinawa, initially and after a 24 h incubation in ambient or high pCO2, for trials at 0.3-m MD and 3.3-m MD.
The percentage of larvae found in the top section of each tube differed between pCO2 treatments in a pattern that varied among times and depths [i.e., there was a significant interaction among depth, time, and treatment, F(3, 34) = 3.762, p = 0.019, Table 2] (Figure 2). Post-hoc analyses were equivocal because of the small sample sizes, but sequential contrasts of times (three comparisons) suggest the interactive effect was caused by differences between 16:00 vs. 20:00 h in the high pCO2 tubes at 0.3-m MD and ambient pCO2 tubes at 3.3-m MD, between 08:00 vs. 12:00 h in the ambient pCO2 tubes at 0.3-m MD, and between 08:00 vs. 12:00 h and 12:00 vs. 16:00 h in the treatment tubes at 3.3-m MD (all p < 0.05). At 0.3-m MD, the percentage of larvae in the top of the tubes in high pCO2 described an inverse parabola as a function of time, increasing from ~42 to 64% between 8:00 and 16:00 h, but decreasing to 49% at 20:00 h (n = 43–50 larvae tube−1). Larval behavior in high pCO2 contrasted with that observed at ambient pCO2, where at 0.3-m MD the percentage of larvae in the upper section of the tubes increased throughout the day, starting at 28% at 08:00 h and ending at 84% at 20:00 h. At 3.3-m MD in high pCO2, 25–50% of the larvae were in the upper section of the tubes regardless of time. Larval behavior was similar at high and ambient pCO2 at 3.3-m MD, where in ambient pCO2 the percentage of larvae in the upper section of the tubes was 28 ± 4% between 8:00 and 20:00 h (mean ± SE, n = 16 based on 200 larvae at each time), although it decreased to 18% (n = 200 larvae) at 16:00 h. Overall, 66 ± 6% and 37 ± 4% of the larvae were found in the upper section of the tubes at 0.3-m MD and 3.3-m MD in high pCO2, respectively (mean ± SE, n = 16), and in all cases the remaining larvae were swimming and behaving normally in the bottom sections of the tubes after 12 h.
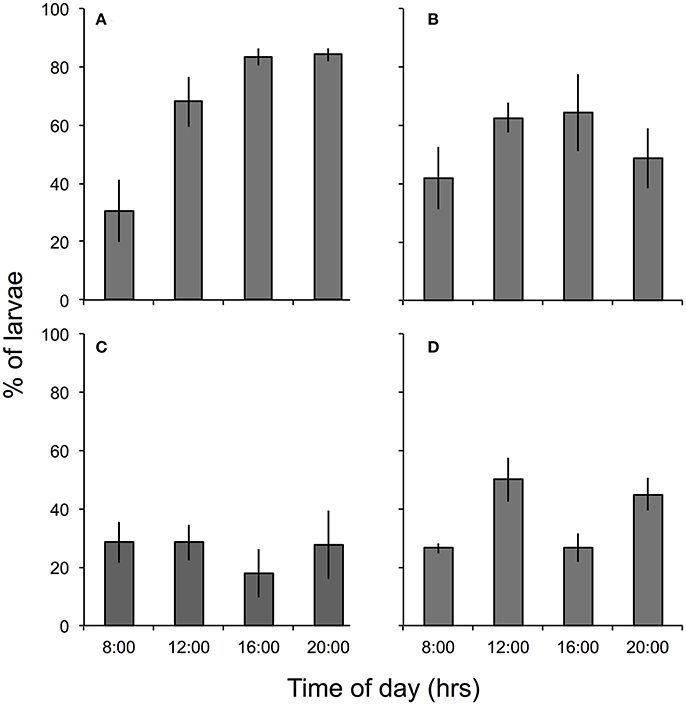
Figure 2. Percent (mean ± SE, n = 4) of P. damicornis larvae in the top (0–21 cm) of tubes filled with seawater at: (A) ambient (~400 μatm) pCO2, 0.3-m MD, (B) high (~1,000 μatm) pCO2, 0.3-m MD, (C) ambient pCO2, 3.3-m MD, and (D) high pCO2, 3.3-m MD. Larval position was scored every 4 h in the first 12 h following initiation of treatment conditions at 08:00 h.
Lipid Content
The larvae used for lipid analysis were similar to one another in color and size (~1-mm long), and were visible in situ when freshly released from the maternal colonies, and following 24 h in the acrylic tubes. The analytical procedure for the lipid analyses required a minimum of 50 larvae for each determination, and this restriction excluded one tube from each pCO2 treatment (high and ambient) in both July and August, when 10–15% of the larvae settled on the walls of the tube after 24 h. Mean dry lipid content at 0.3-m MD was 12.19 ± 0.23 μg lipid larvae−1 after 24 h in ambient pCO2, but this declined ~23% to 9.38 ± 0.79 μg lipid larvae−1 after 24 h in elevated pCO2 (both ± SE, n = 3). Similarly, at 3.3-m MD, mean dry lipid content was 11.96 ± 0.69 μg lipid larvae−1 after 24 h in ambient pCO2, declining ~25% to 8.99 ± 1.24 μg lipid larvae−1 after 24 h in elevated pCO2 (both ± SE, n = 3). Lipid content of freshly released larvae did not differ between July and August (t = 0.092, df = 4, p = 0.928), and averaged 11.98 ± 0.27 μg larvae−1 (± SE, n = 6). As lipid content did not differ between months for larvae incubated in ambient pCO2 (t = 0.248, df = 3, p = 0.820) or high pCO2 (t = 0.267, df = 3, p = 0.803), the results of the lipid analyses following incubations were pooled between months and depths to test for an overall effect of high pCO2. Lipid content of the larvae differed in high pCO2 from ambient pCO2 and initial release (Kruskal Wallis test, H = 9.710, n1 = n2 = n3 = 6, p = 0.008) (Figure 3).
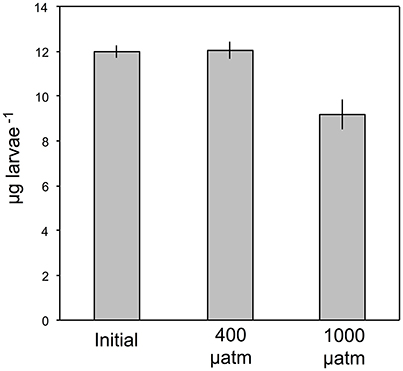
Figure 3. Lipid content of P. damicornis larvae (μg larvae−1) following release from the maternal colony and after 24-h exposures to ambient and high pCO2, pooled between 0.3-m MD and 3.3-m MD (mean ± SE, n = 6).
Discussion
In this study, the vertical position in the water column of Pocillopora damicornis larvae was measured as a function of pCO2, depth, and time of day to test the hypothesis that their vertical positioning was affected by high pCO2. Under current ambient pCO2 conditions directly below the surface, freshly released larvae moved upward in vertically-oriented tubes over 12 h. Upward movement was attenuated by a 3-m increase in median depth, where ~38% fewer larvae remained in the upper section of the tubes all day. Larval behavior in tubes close to the surface changed with exposure to high pCO2, with accentuation of daytime movement that enhanced the occurrence of larvae in the upper portion of the tubes around noon, but this trend reversed in the morning and evening to favor occurrence in the lower portion of the tubes. The pattern of upwards movement at midday disappeared in tubes containing high pCO2 seawater at 3.3-m MD, in which 50–75% of the larvae were found throughout the day in the bottom portion of the tubes (i.e., there was no vertical migration over the 12 h observation period). Lipid content of the larvae declined 23–25% after 24 h at high pCO2 (but not ambient pCO2), and changes in buoyancy due to reduction in lipid content may, therefore, have played a role in the shift in larval behavior at high pCO2. Shifts in vertical position of the larvae on a scale of decimeters as a function of pCO2 can cause differential advection through exposure to dissimilar current velocities (Bingham and Young, 1991) that occur on vertical scales as small as 50–100 cm in shallow seawater over coral reefs (Harii and Kayanne, 2003; Hench et al., 2008). Exposure to different flow speeds, directions, and water types can cause local retention or decreasing pelagic larval duration (PLD) (Largier, 2003; Cowen and Sponaugle, 2009), and therefore affect dispersal (Savina et al., 2010).
The vertical position of pelagic larvae in the water column allows stratified water masses moving at different speeds or varying directions to be “sampled” as larvae move up and down in the water (Largier, 2003). In Okinawa, for example, pelagic larvae are exposed to hydrodynamic features differing in flow direction and speed between wind-driven surface currents (8 cm s−1 north) and net seawater flow 0.5–1 m below the surface (1.5 cm s−1 southeast) (Harii and Kayanne, 2003), thus modifying their dispersal depending on the vertical position. For larvae in the present study, stratification of currents could lead to increased dispersal, notably for those larvae inferred to spend time in the upper 21 cm of the water column, vs. those positioned deeper in the water column (i.e., the lower 47 cm of the present tubes). Larval dispersal mediated by vertical migration through stratified seawater motion is likely to be common for coral larvae, because seawater is commonly stratified by contrasting horizontal flows speeds in reef habitats (e.g., shallow coastal waters, or on a reef crest). For instance, using a vertical resolution of 1 m, Salas-Monreal et al. (2009) demonstrated differences in east-west and north-south velocity components of seawater in the Gulf of Mexico, with these differences establishing a depth-dependent flow gradient extending from 4 to 16 cm s−1 between the surface and 2-m depth. Likewise, measurements of seawater velocity just behind the reef crest in Moorea, French Polynesia, revealed differences of ~5–10 cm s−1 between the surface of the reef at 2-m depth, and the surface of the seawater flowing over the reef (Hench et al., 2008).
Additionally, based on the data presented here, the present study suggests that elevated pCO2 will lead to a higher proportion of larvae at or near the surface of the seawater. In the few coral reef habitats where seawater pCO2 is naturally elevated and localized, such as the Galápagos (Manzello, 2010), the response reported here of coral larvae to elevated pCO2 may have beneficial effects. Such effects could result in dispersal away from areas of higher pCO2, via surface currents that are typically of a greater magnitude than subsurface currents (Harii and Kayanne, 2003). However, the dispersal of coral larvae away from areas of high pCO2 may not be advantageous in the context of OA, which is predicted to result in elevated seawater pCO2 across large spatial scales so that enhanced advection of larvae would not move them to areas of reduced pCO2.
The large number of P. damicornis larvae (an average of 55% pooled across all treatments and times of day) found in the bottom of the present tubes therefore is also likely to have ecological relevance in determining the “sampling” by larvae of multiple strata of seawater differing in seawater velocities. Larvae aggregating near the bottom of the present tubes probably would have reached the benthos ~5 m below if they were not contained in capped tubes, and this would have accentuated the sampling of benthic substrata and possibly hastened settlement, effectively reducing PLD (Miller and Mundy, 2003). Testing of this possibility would require two changes to the present experiment: first, to provide larvae with access to a longer vertical section of seawater, and second, to census their vertical position over more than 12 h to evaluate diel periodicity in vertical movement (Hodgson, 1985). The potential implications of reduced PLD in coral larvae by accentuated downward migration at ≥1,000 μatm pCO2 has ecological relevance, because shorter PLDs are associated with limited dispersal potential and enhanced possibilities of settlement at the natal site (Levin, 2006; Treml et al., 2012).
Lipid Content in High pCO2
The second goal of the present study was to test the hypothesis that high pCO2 affected the lipid content of P. damicornis larvae after 24 h exposure to high pCO2 in situ. Exploration of this goal was motivated by the inference that a change in lipid content in response to high pCO2 would affect larval buoyancy (sensu Harii et al., 2007). A leading determinant of buoyancy in invertebrate larvae is lipid content (Chia et al., 1984), and variation in lipid content can modulate their buoyancy, as in larvae of the abalone Haliotis spp. (Moran and Manahan, 2003) and a diversity of zooplankton (Lee et al., 2006). It is commonly accepted that brooded coral larvae are positively buoyant immediately following release (Oliver and Willis, 1987; Wood et al., 2016), and therefore quickly ascend to the surface of the seawater (Harii et al., 2002), where they are subject to rapid advection in wind-driven surface currents (Wolanski and Kingsford, 2014).
In the present study, the lipid content of P. damicornis larvae was measured at the start of the incubations and 24 h later. We infer that changes in lipid content occurred linearly over this period, which is consistent with trends observed in the larvae of other corals (Figueiredo et al., 2012) and invertebrates [e.g., abalone (Moran and Manahan, 2003) and sea urchins Sewell, 2005]. A linear decline in the lipid content of coral larvae could be a result of several non-exclusive mechanisms, including the catabolism of fatty acids through aerobic respiration (Harii et al., 2010), the incorporation of lipids into membrane phospholipids (Lee et al., 2006), or the loss of lipids in fat-rich mucus secretions (Gaither and Rowan, 2010; Ricardo et al., 2016). Distinguishing among these possibilities will require physiological analyses of different mechanisms by which lipid reserves are depleted by catabolism, and resolution of the lipids to their component classes (e.g., triacylglycerols and wax esters). The decline in lipid content of P. damicornis larvae that we recorded was detected based on initial and final sampling after 24 h in the treatment. Intermediate sampling that might have more completely resolved the rate of lipid decline was precluded by the challenges of working offshore at night. Nevertheless, the results show that larval lipid content declined after exposure to high pCO2, but not after exposure to ambient pCO2.
To our knowledge, the buoyancy of brooded coral larvae has been measured in only one study (Szmant and Meadows, 2006), likely because of the technical difficulty of separating buoyancy from swimming as mechanisms mediating larval motility in this taxon. These difficulties arise from the small size of most coral larvae, the difficulties of quantitatively sampling them in the plankton, and the challenges of quantifying the effects of ciliary swimming. However, larvae freshly released from P. damicornis in Mo'orea, French Polynesia, sank when they were anesthetized with eugenol, thus suggesting they were negatively buoyant (L. Bramanti, personal communication to JB), as has been described for the aposymbiotic larvae of the gorgonian Corallium rubrum (Martínez-Quintana et al., 2014). When interpreting larval movement in the present experiment, we therefore assume that larvae of P. damicornis are negatively buoyant.
Most brooded larvae of tropical scleractinians contain endosymbiotic Symbiodinium, which typically are vertically transmitted from the parent colony (Harrison and Wallace, 1990), and can translocate as much as 27% of their fixed carbon to the cnidarian tissue of the planula (Richmond, 1982). However, in addition to obtaining energy resources from their Symbiodinium, it is likely that brooded coral larvae also metabolize maternally-derived lipids to meet their energy needs (Harii et al., 2010). The use of lipid reserves for this purpose could be particularly important when the photosynthetic capacity of larval Symbiodinium is impaired by light or thermal stress (Haryanti et al., 2015), or in the context of our findings, by increased pCO2, as has been seen in adult corals (Anthony et al., 2008). Understanding of the mechanisms of lipid catabolism is likely to be enhanced by more information on the lipid classes involved in the inferred catabolism. The lipids in P. damicornis larvae are composed of wax esters, triglycerides, and phospholipids, and as wax esters are typically the most abundant class (Harii et al., 2007; Figueiredo et al., 2012), it is reasonable to infer that lipids in the present larvae were also composed of mainly wax esters. The concentration of wax esters in the larvae of P. damicornis is particularly important to understanding their behavior, because wax esters have a lower density than triglycerides and phospholipids (Gurr et al., 2002; Lee et al., 2006). Wax esters are also positively buoyant in seawater (Arai et al., 1993), so they could modulate the buoyancy of larvae in which they occur.
Evidence for lipid catabolism as a mechanism to reduce the wax ester content of coral larvae comes from P. damicornis at Heron Island, Australia, where ≥85% of larval metabolic demand was derived from lipids under low light (~100 μmol m−2 s−1) conditions after 31 days in culture (Harii et al., 2010). Calculations corroborate this finding, because a larval respiration rate in P. damicornis of 0.15 nmol O2 larvae−1 min−1 (Cumbo et al., 2013) would consume 2.42 μg lipid larvae−1 day−1, assuming lipid was the respiratory substrate and that it required 2 mL O2 mg−1 to be consumed in aerobic respiration (Schmidt-Nielsen, 1997). Over 24 h in the present experiment, larvae consumed ~2.8 μg lipid larvae−1 in high pCO2, so while the composition of lipids in P. damicornis larvae was not measured in the present analysis, it is feasible that the decline in lipid content included effects caused by catabolism of wax esters. A reduction of wax esters might be sufficient to reduce the buoyancy of the present larvae incubated at high pCO2 and 0.3-m MD, therefore favoring downward movement in the tubes.
Elucidating the causes of the vertical movement recorded in the present analysis is challenging due to the likelihood that movement was caused by a combination of buoyancy and ciliary swimming. The inability to distinguish larval movement attributed to ciliary swimming from that attributed to passive buoyancy suggests that at least a portion of the movement recorded in the present study was a result of swimming. Utilization of ciliary swimming might explain the interactive effect of pCO2 and depth on the position of larvae within the tubes, because the constancy of lipid content between depths at high pCO2 suggests that changes in buoyancy were independent of depth in high pCO2. According to this hypothesis, larvae might have swum more actively in high pCO2. The downwards shift in vertical position of P. damicornis larvae in 3.3-m MD tubes (vs. 0.3-m MD) at high pCO2 may reflect active swimming, as has been recorded for the larvae of many coral species (Vermeij et al., 2010; Martínez-Quintana et al., 2014), including P. damicornis (Te, 1992; Kuffner and Paul, 2004).
Conclusions
While the present study shows an effect of high pCO2 on vertical position of the pelagic larvae of a brooding coral, this outcome should be interpreted within the constraints of the experimental design employed. First, larval behavior in acrylic tubes is likely to be constrained by the physical conditions in the tubes that differ from the open reef, notably in terms of flow regimes and wall effects. While the implications of these effects should not be ignored, the behavior of brooded coral larvae is similar in vertically-oriented tubes varying in size from 30 cm long × 1 cm ID (Raimondi and Morse, 2000) to 2.2 m long × 5 cm ID (Tay et al., 2011). This observation suggests that wall effects and tube dimensions do not alter larval behavior. Second, our interpretation of depth effects was restricted to an experimental design that comingled an effect of time with depth (i.e., the two depths were tested in separate months), necessitated by the logistical challenges of simultaneously establishing an in situ experiment at two depths. While we cannot exclude the possibility that time confounded the test of depth, the potential influences of this effect were reduced by stringently standardizing protocols between months, sampling larvae from colonies on the same lunar day of each month [as larval phenotype differs among release days in brooding corals (Cumbo et al., 2013)], using new colonies each month (i.e., they were statistically independent), and testing for differences in ambient seawater temperature between months. With an understanding of the limitations of the study, the present results have relevance in evaluating the likelihood of ocean acidification to modify the dispersal and connectivity of reef corals among populations that are part of a larger metapopulation (Levin, 2006). For larvae of brooded corals, the impacts of high pCO2 on the physiology, behavior, and response to physical conditions suggests it will be productive to consider these effects in order to evaluate the potential for larval dispersal in a more acidic ocean.
Animal Rights Statement
All known animal care and ethics issues for invertebrates were followed.
Author Contributions
JB and PE planned the experiments. JB conducted the experiments. JB, SH, and HK contributed to the experiments. JB analyzed the data with advice from PE. JB and PE wrote the manuscript with contributions, review, and editing from SH and HK.
Funding
This research was supported by a grant to JB from the US National Science Foundation - East Asia and Pacific Summer Institutes program (#16-13798), and the Japanese Society for the Promotion of Science Summer Institutes program. Additional funding was provided by Core Research for Evolutional Science and Technology (CREST), Japan Science and Technology Agency, to SH and HK.
Conflict of Interest Statement
The authors declare that the research was conducted in the absence of any commercial or financial relationships that could be construed as a potential conflict of interest.
Acknowledgments
This research was conducted at Sesoko Station, Tropical Biosphere Research Center, in collaboration with the University of the Ryukyus. We thank R. Prasetia and F. Sinniger for guidance and assistance with fieldwork. This is contribution number 263 of the Marine Biology Program of California State University, Northridge.
References
Almany, G. R., Berumen, M. L., Thorrold, S. R., Planes, S., and Jones, G. P. (2007). Local replenishment of coral reef fish populations in a marine reserve. Science 316, 742–744. doi: 10.1126/science.1140597
Anthony, K. R. N., Kline, D. I., Diaz-Pulido, G., Dove, S., and Hoegh-Guldber, O. (2008). Ocean acidification causes bleaching and productivity loss in coral reef builders. Proc. Nat. Acad. Sci. U.S.A. 105, 17442–17446. doi: 10.1073/pnas.0804478105
Arai, I., Kato, M., Heyward, A., Ikeda, Y., Iizuka, T., and Maruyama, T. (1993). Lipid composition of positively buoyant eggs of reef building corals. Coral Reefs 12, 71–75. doi: 10.1007/BF00302104
Bingham, B. L., and Young, C. M. (1991). Larval behavior of the ascidian Ecteinascidia turbinata Herdman; an in situ experimental study of the effects of swimming on dispersal. J. Exp. Mar. Biol. Ecol. 145, 189–204. doi: 10.1016/0022-0981(91)90175-V
Bruno, J. F., and Selig, E. R. (2007). Regional decline of coral cover in the Indo-Pacific: timing, extent, and subregional comparisons. PLoS ONE 2:e711. doi: 10.1371/journal.pone.0000711
Byrne, M. (2011). “Impact of ocean warming and ocean acidification on marine invertebrate life history stages: vulnerabilities and potential for persistence in a changing ocean,” in Oceanography and Marine Biology: An Annual Review, eds R. N. Gibson, R. J. A. Atkinson, and J. D. M. Gordon (Boca Raton, FL: CRC Press), 1–42.
Campbell, R. W., and Dower, J. F. (2003). Role of lipids in the maintenance of neutral buoyancy by zooplankton. Mar. Ecol. Prog. Ser. 263, 93–99. doi: 10.3354/meps263093
Chia, F.-S., Buckland-Nicks, J., and Young, C. M. (1984). Locomotion of marine invertebrate larvae: a review. Can. J. Zool. 62, 1205–1222. doi: 10.1139/z84-176
Cornell, H. V., and Lawton, J. H. (1992). Species interactions, local and regional processes, and limits to the richness of ecological communities: a theoretical perspective. J. Anim. Ecol. 61, 1–12. doi: 10.2307/5503
Cowen, R. K., Paris, C. B., and Srinivasan, A. (2006). Scaling of connectivity in marine populations. Science 311, 522–527. doi: 10.1126/science.1122039
Cowen, R. K., and Sponaugle, S. (2009). Larval dispersal and marine population connectivity. Ann. Rev. Mar. Sci. 1, 443–466. doi: 10.1146/annurev.marine.010908.163757
Cumbo, V. R., Fan, T. Y., and Edmunds, P. J. (2012). Effects of exposure duration on the response of Pocillopora damicornis larvae to elevated temperature and high pCO2. J. Exp. Mar. Biol. Ecol. 439, 100–107. doi: 10.1016/j.jembe.2012.10.019
Cumbo, V. R., Edmunds, P. J., Wall, C. B., and Fan, T. Y. (2013). Brooded coral larvae differ in their response to high temperature and elevated pCO2 depending on the day of release. Mar. Biol. 160, 2903–2917. doi: 10.1007/s00227-013-2280-y
Doherty, P. J., Williams, D. M., and Sale, P. F. (1985). The adaptive significance of larval dispersal in coral reef fishes. Environ. Biol. Fishes 12, 81–90. doi: 10.1007/BF00002761
Edmunds, P. J. (2000). Patterns in the distribution of juvenile corals and coral reef community structure in St. John, US Virgin Islands. Mar. Ecol. Ser. 202, 113–124. doi: 10.3354/meps202113
Fan, T. Y., Li, J. J., Ie, S. X., and Fang, L. S. (2002). Lunar periodicity of larval release by pocilloporid corals in Southern Taiwan. Zool. Stud. 41, 288–293
Figueiredo, J., Baird, A. H., Cohen, M. F., Flot, J.-F., Kamiki, T., Meziane, T., et al. (2012). Ontogenetic change in the lipid and fatty acid composition of scleractinian coral larvae. Coral Reefs 31, 613–619. doi: 10.1007/s00338-012-0874-3
Gaither, M. R., and Rowan, R. (2010). Zooxanthellar symbiosis in planula larvae of the coral Pocillopora damicornis. J. Exp. Mar. Biol. Ecol. 386, 45–53. doi: 10.1016/j.jembe.2010.02.003
Gleason, D. F., and Hofmann, D. K. (2011). Coral larvae: from gametes to recruits. J. Exp. Mar. Biol. Ecol. 408, 42–57. doi: 10.1016/j.jembe.2011.07.025
Gurr, M. I., Harwood, J. L., and Frayn, K. N. (2002). Lipid Biochemistry. London: Chapman & Hall. doi: 10.1002/9780470774366
Hamdoun, A., and Epel, D. (2007). Embryo stability and vulnerability in an always changing world. Proc. Natl. Acad. Sci. U.S.A. 104, 1745–1750. doi: 10.1073/pnas.0610108104
Harii, S., and Kayanne, H. (2003). Larval dispersal, recruitment, and adult distribution of the brooding stony octocoral Heliopora coerulea on Ishigaki Island, southwest Japan. Coral Reefs 22, 188–196. doi: 10.1007/s00338-003-0302-9
Harii, S., Kayanne, H., Takigawa, H., Hayashibara, T., and Yamamoto, M. (2002). Larval survivorship, competency periods and settlement of two brooding corals, Heliopora coerulea and Pocillopora damicornis. Mar. Biol. 141, 39–46. doi: 10.1007/s00227-002-0812-y
Harii, S., Nadaoka, K., Yamamoto, M., and Iwao, K. (2007). Temporal changes in settlement, lipid content and lipid composition of larvae of the spawning hermatypic coral Acropora tenuis. Mar. Ecol. Prog. Ser. 346, 89–96. doi: 10.3354/meps07114
Harii, S., Yamamoto, M., and Hoegh-Guldberg, O. (2010). The relative contribution of dinoflagellate photosynthesis and stored lipids to the survivorship of symbiotic larvae of the reef-building corals. Mar. Biol. 157, 1215–1224. doi: 10.1007/s00227-010-1401-0
Harrison, P. L., and Wallace, C. C. (1990). “Reproduction, dispersal, and recruitment of scleractinian corals,” in Coral Reefs, Ecosystems of the World, Vol. 25, ed Z. Dubinsky (Amsterdam: Elsevier), 133–207.
Haryanti, D., Yasuda, N., Harii, S., and Hidaka, M. (2015). High tolerance of symbiotic larvae of Pocillopora damicornis to thermal stress. Zool. Stud. 54, 52. doi: 10.1186/s40555-015-0134-7
Hastings, A., and Harrison, S. (1994). Metapopulation dynamics and genetics. Annu. Rev. Ecol. Syst. 25, 167–188. doi: 10.1146/annurev.es.25.110194.001123
Hench, J. L., Leichter, J. J., and Monismith, S. G. (2008). Episodic circulation and exchange in a wave-driven coral reef and lagoon system. Limnol. Oceanogr. 53, 2681–2694. doi: 10.4319/lo.2008.53.6.2681
Hodgson, G. (1985). Abundance and distribution of planktonic coral larvae in Kaneohe Bay, Oahu, Hawaii. Mar. Ecol. Prog. Ser. 26, 61–71. doi: 10.3354/meps026061
Hughes, T., Baird, A., and Dinsdale, E. (2000). Supply-side ecology works both ways: the link between benthic adults, fecundity, and larval recruits. Ecology 81, 2241–2249. doi: 10.1890/0012-9658(2000)081[2241:SSEWBW]2.0.CO;2
Intergovernmental Panel on Climate Change (IPCC) (2014). Climate Change 2014: Mitigation of Climate Change, Contribution of Working Group III to the Fifth Assessment Report of the Intergovernmental Panel on Climate Change, ed T. F. Stocker et al. (Cambridge, UK: Cambridge University Press).
Isomura, N., and Nishihira, M. (2001). Size variation of planulae and its effect on the lifetime of planulae in three pocilloporid corals. Coral Reefs 20, 309–315. doi: 10.1007/s003380100180
Jones, G. P., Almany, G. R., Russ, G. R., Sale, P. F., Steneck, R. S., Van Oppen, M. J. H., et al. (2009). Larval retention and connectivity among populations of corals and reef fishes: history, advances and challenges. Coral Reefs 28, 307–325. doi: 10.1007/s00338-009-0469-9
Jones, G. P., Planes, S., and Thorrold, S. R. (2005). Coral reef fish larvae settle close to home. Curr. Biol. 15, 1314–1318. doi: 10.1016/j.cub.2005.06.061
Kuffner, I. B., and Paul, V. J. (2004). Effects of the benthic cyanobacterium Lyngbya majuscula on larval recruitment of the reef corals Acropora surculosa and Pocillopora damicornis. Coral Reefs 23, 455–458. doi: 10.1007/s00338-004-0416-8
Largier, J. (2003). Considerations in estimating larval dispersal distances from oceanographic data. Ecol. Appl. 13, 71–89. doi: 10.1890/1051-0761(2003)013[0071:CIELDD]2.0.CO;2
Lee, R. F., Hagen, W., and Kattner, G. (2006). Lipid storage in marine zooplankton. Mar. Ecol. Prog. Ser. 307, 273–306. doi: 10.3354/meps307273
Levin, L. A. (2006). Recent progress in understanding larval dispersal: new directions and digressions. Integr. Comp. Biol. 46, 282–297. doi: 10.1093/icb/icj024
Lewis, E., and Wallace, D. W. R. (1998). Program Developed for CO2 System Calculations. Oak Ridge, TN: Carbon Dioxide Information Analysis Center, Oak Ridge National Laboratory, U.S. Dept of Energy. ORNL/CDIAC-105.
Manzello, D. P. (2010). Ocean acidification hotspots: Spatiotemporal dynamics of the seawater CO2 system of eastern Pacific coral reefs. Limnol. Oceanogr. 55, 239–248. doi: 10.4319/lo.2010.55.1.0239
Martínez-Quintana, A., Bramanti, L., Viladrich, N., Rossi, S., and Guizien, K. (2014). Quantification of larval traits driving connectivity: the case of Corallium rubrum (L. 1758). Mar. Biol. 162, 309–318. doi: 10.1007/s00227-014-2599-z
Mehrbach, C., Culberson, C. H., Hawley, J. E., and Pytkowicx, R. M. (1973). Measurement of the apparent dissociation constants of carbonic acid in seawater at atmospheric pressure. Limnol. Oceanogr. 18, 897–907. doi: 10.4319/lo.1973.18.6.0897
Metaxas, A. (2001). Behaviour in flow: perspectives on the distribution and dispersion of meroplanktonic larvae in the water column. Can. J. Fish. Aquat. Sci. 58, 86–98. doi: 10.1139/f00-159
Miller, K., and Mundy, C. (2003). Rapid settlement in broadcast spawning corals: implications for larval dispersal. Coral Reefs 22, 99–106. doi: 10.1007/s00338-003-0290-9
Moran, A. L., and Manahan, D. T. (2003). Energy metabolism during larval development of green and white abalone, Haliotis fulgens and H. sorenseni. Biol. Bull. 204, 270–277. doi: 10.2307/1543598
Moss, R. H., Edmonds, J. A., Hibbard, K. A., Manning, M. R., Rose, S. K., van Vuuren, D. P., et al. (2010). The next generation of scenarios for climate change research and assessment. Nature 463, 747–756. doi: 10.1038/nature08823
Oliver, J. K., and Willis, B. L. (1987). Coral-spawn slicks in the Great Barrier Reef: preliminary observations. Mar Biol. 94, 521–530. doi: 10.1007/BF00431398
Paris, C. B., and Cowen, R. K. (2004). Direct evidence of a biophysical retention mechanism for coral reef fish larvae. Limnol. Oceanogr. 49, 1964–1979. doi: 10.4319/lo.2004.49.6.1964
Pratchett, M. S., Trapon, M., Berumen, M. L., and Chong-Seng, K. (2011). Recent disturbances augment community shifts in coral assemblages in Moorea, French Polynesia. Coral Reefs 30, 183–193. doi: 10.1007/s00338-010-0678-2
Putnam, H. M., Edmunds, P. J., and Fan, T. Y. (2010). Effect of a fluctuating thermal regime on adult and larval reef corals. Invertebr. Biol. 129, 199–209. doi: 10.1111/j.1744-7410.2010.00199.x
Putnam, H. M., Mayfield, A. B., Fan, T. Y., Chen, C. S., and Gates, R. D. (2013). The physiological and molecular responses of larvae from the reef-building coral Pocillopora damicornis exposed to near-future increases in temperature and pCO2. Mar. Biol. 160, 2157–2173. doi: 10.1007/s00227-012-2129-9
Quinn, G., and Keough, M. (2002). Experimental Design and Data Analysis for Biologists. Cambridge: Cambridge University Press.
Raimondi, P. T., and Morse, A. N. C. (2000). The consequences of complex larval behavior in a coral. Ecology 81, 3193–3211. doi: 10.1890/0012-9658(2000)081[3193:TCOCLB]2.0.CO;2
Ricardo, G. F., Jones, R. J., Clode, P. L., and Negri, A. P. (2016). Mucous secretion and cilia beating defend developing coral larvae from suspended sediments. PLoS ONE 11:e0162743. doi: 10.1371/journal.pone.0162743
Richmond, R. H. (1982). “Energetic consideration in the dispersal of Pocillopora damicornis (Linnaeus) planulae,” in Proceedings of 4th International Coral Reef Symposium, vol. 2 (Manila), 153–156.
Richmond, R. H. (1987). Energetics, competence, and long-distance dispersal of planula larvae of the coral Pocillopora damicornis. Mar. Biol. 93, 527–533. doi: 10.1007/BF00392790
Rivest, E. B., and Hofmann, G. E. (2014). Responses of the metabolism of the larvae of Pocillopora damicornis to ocean acidification and warming. PLoS ONE 9:e96172. doi: 10.1371/journal.pone.0096172
Rivest, E. B., and Hofmann, G. E. (2015). Effects of temperature and pCO2 on lipid use and biological parameters of planulae of Pocillopora damicornis. J. Exp. Mar. Biol. Ecol. 473, 43–52. doi: 10.1016/J.JEMBE.2015.07.015
Salas-Monreal, D., Salas-de-León, D. A., Monreal-Gómez, M. A., and Riverón-Enzástiga, M. L. (2009). Current rectification in a tropical coral reef system. Coral Reefs 28, 871–879. doi: 10.1007/s00338-009-0521-9
Savina, M., Lacroix, G., and Ruddick, K. (2010). Modelling the transport of common sole larvae in the southern North Sea: influence of hydrodynamics and larval vertical movements. J. Mar. Syst. 81, 86–98. doi: 10.1016/j.jmarsys.2009.12.008
Sewell, M. A. (2005). Utilization of lipids during early development of the sea urchin Evechinus chloroticus. Mar. Ecol. Prog. Ser. 304, 133–142. doi: 10.3354/meps304133
Szmant, A. M., and Meadows, M. G. (2006). Developmental changes in coral larval buoyancy and vertical swimming behavior: implications for dispersal and connectivity. Proceedings of 10th International Coral Reef Symposium, Vol. 1 (Okinawa), 431–437.
Takahashi, A., and Kurihara, H. (2013). Ocean acidification does not affect the physiology of the tropical coral Acropora digitifera during a 5-week experiment. Coral Reefs 32, 305–314. doi: 10.1007/s00338-012-0979-8
Tay, Y. C., Guest, J. R., Chou, L. M., and Todd, P. A. (2011). Vertical distribution and settlement competencies in broadcast spawning coral larvae: implications for dispersal models. J. Exp. Mar. Biol. Ecol. 409, 324–330. doi: 10.1016/j.jembe.2011.09.013
Te, F. T. (1992). Response to higher sediment loads by Pocillopora damicornis planulae. Coral Reefs 11, 131–134. doi: 10.1007/BF00255466
Thorson, G. (1950). Reproductive and larval ecology of marine bottom invertebrates. Biol. Rev. 25, 1–45. doi: 10.1111/j.1469-185X.1950.tb00585.x
Treml, E. A., Roberts, J. J., Chao, Y., Halpin, P. N., Possingham, H. P., and Riginos, C. (2012). Reproductive output and duration of the pelagic larval stage determine seascape-wide connectivity of marine populations. Integr. Comp. Biol. 52, 525–537. doi: 10.1093/icb/ics101
van Oppen, M. J., Lutz, A., De'ath, G., Peplow, L., and Kininmonth, S. (2008). Genetic traces of recent long-distance dispersal in a predominantly self-recruiting coral. PLoS ONE 3:e3401. doi: 10.1371/journal.pone.0003401
Vermeij, M. J. A., Marhaver, K. L., Huijbers, C. M., Nagelkerken, I., and Simpson, S. D. (2010). Coral larvae move toward reef sounds. PLoS ONE 5:e10660. doi: 10.1371/journal.pone.0010660
Whalan, S., Abdul Wahab, M. A., Sprungala, S., Poole, A. J., and De Nys, R. (2015). Larval settlement: the role of surface topography for sessile coral reef invertebrates. PLoS ONE 10:e0117675. doi: 10.1371/journal.pone.0117675
Wolanski, E., and Kingsford, M. J. (2014). Oceanographic and behavioural assumptions in models of the fate of coral and coral reef fish larvae. J. R. Soc. Interface 11:20140209. doi: 10.1098/rsif.2014.0209
Wood, S., Baums, I. B., Paris, C. B., Ridgwell, A., Kessler, W. S., and Hendy, E. J. (2016). El Niño and coral larval dispersal across the eastern Pacific marine barrier. Nat. Commun. 7:12571. doi: 10.1038/ncomms12571
Keywords: ocean acidification, corals, Pocillopora damicornis, brooded larvae, behavior
Citation: Bergman JL, Harii S, Kurihara H and Edmunds PJ (2018) Behavior of Brooded Coral Larvae in Response to Elevated pCO2 Front. Mar. Sci. 5:51. doi: 10.3389/fmars.2018.00051
Received: 28 October 2017; Accepted: 02 February 2018;
Published: 21 February 2018.
Edited by:
Claire Beatrix Paris, University of Miami, United StatesReviewed by:
Rémi M. Daigle, Laval University, CanadaPunyasloke Bhadury, Indian Institute of Science Education and Research Kolkata, India
Copyright © 2018 Bergman, Harii, Kurihara and Edmunds. This is an open-access article distributed under the terms of the Creative Commons Attribution License (CC BY). The use, distribution or reproduction in other forums is permitted, provided the original author(s) and the copyright owner are credited and that the original publication in this journal is cited, in accordance with accepted academic practice. No use, distribution or reproduction is permitted which does not comply with these terms.
*Correspondence: Jessica L. Bergman, amVzc2VsYmVyZ21hbkBnbWFpbC5jb20=