- School of Marine and Atmospheric Sciences at Stony Brook University, Southampton, NY, United States
Climate change processes are warming, acidifying, and promoting a reduction of plankton biomass within World oceans. While the effects of these stressors on marine fish have been studied individually, their combined and interactive impacts remain unclear. Here we present experiments investigating the interactive effects of increased pCO2, temperature, and food-limitation on the early life history traits of two species of marine schooling fish native to Northeast US estuaries, Menidia beryllina (inland silverside) and Cyprinodon variegatus (sheepshead minnow). While each stressor significantly altered hatching times, growth rates, and/or survival of fish, significant interactions between stressors resulted in impacts that could not have been predicted based upon exposures to individual stressors. Fish that were unaffected by high pCO2 when reared at ideal temperatures experienced significant declines in survivorship when exposed to elevated pCO2 at temperatures above or below their thermal optimum. Similarly, fish provided with less food were more vulnerable to elevated pCO2 than fish provided with adequate nutrition. These findings highlight the significance of incorporating multiple stressors in studies investigating the impacts of climate change stressors on marine life. Collectively, these results suggest that climate change stressors may interact to synergistically suppress the productivity of fisheries in coastal ecosystems and that these effects may intensify as climate changes continue.
Introduction
Climate change is altering multiple aspects of the World's oceans (Doney et al., 2012; Poloczanska et al., 2013). Since the industrial revolution, atmospheric carbon dioxide (CO2) levels have progressively risen from ~280 to over 400 ppm (as of December 2017; http://scrippsCO2.ucsd.edu). World oceans have absorbed nearly a third of all anthropogenically-derived CO2 (Sabine et al., 2004). As CO2 enters the ocean it reacts with water forming carbonic acid (H2CO3), which quickly disassociates into bicarbonate () releasing a hydrogen ion (H+) that reduces ocean pH and subsequently sequesters carbonate ions (), a process commonly known as ocean acidification (Sabine et al., 2004). As a result, the pH within the sea surface has decreased ~0.1 units and is predicted to decrease an additional 0.2–0.3 units by the end of this century if CO2 emissions continue (Heogh-Guldberg et al., 2014). Concurrently, mean surface-ocean temperatures have risen ~1°C and are predicted to increase and additional 2–4°C depending on the rate of future CO2 emissions (Solomon, 2007; IPCC, 2014).
In addition to the acidification of open-ocean systems, many coastal systems experience seasonal and local acidification caused by increased microbial respiration stimulated by nutrient loading (Cai et al., 2011; Wallace et al., 2014; Baumann et al., 2015). For example, some hypereutrophic estuaries can experience extreme acidification (pHT < 7.2) coinciding with high levels of pCO2 (> 2,000 μatm) during spring and summer months (Melzner et al., 2013; Wallace et al., 2014; Baumann et al., 2015), periods during which many fish and invertebrates are spawned in temperate ecosystems (Kennedy and Krantz, 1982; Sherman et al., 1984; Helluy and Beltz, 1991). The onset and persistence of these conditions may have negative implications for fisheries whose early life-stages are sensitive to acidification (Baumann et al., 2012; Murray et al., 2014; DePasquale et al., 2015).
While a large number of studies have demonstrated the negative effects of acidification on externally calcifying organisms (Doney et al., 2009; Kroeker et al., 2010, 2013; Talmage and Gobler, 2010), careful study of acidification effects on internally calcifying organisms such as marine fish are comparatively less common. The majority of those doing so report adverse effects on the growth, development, and size of larval marine fish exposed to high pCO2 (e.g., Baumann et al., 2012; Miller et al., 2012; Chambers et al., 2014; Murray et al., 2014; DePasquale et al., 2015), while fewer report no ill effects (see Munday et al., 2011; Frommel et al., 2013). For species adversely affected by low pH, experimental evidence suggests that the earliest stages (e.g., embryonic thru larval phases) are the most sensitive to acidification (Ishimatsu et al., 2008; Baumann et al., 2012; Chambers et al., 2014). When combined with other stressors such as hypoxia and thermal stress, acidification can both additively and synergistically reduce the survival rate of larval fish (DePasquale et al., 2015; Gobler and Baumann, 2016).
Beyond acidification, other climate change stressors are predicted to have strong influences on the early life-stages of marine fish. Temperature in particular has profound influences on the physiology, behavior, and phenology of temperate marine fish (Houde, 1989; Pepin, 1991; Nye et al., 2009). Current observations suggest that some fish populations may be moving poleward and/or into deeper locales where temperatures are closer to their thermal optimums, changes that may alter the productivity of fisheries in these regions (Perry et al., 2005; Nye et al., 2009; Pinsky et al., 2013). Warming of the oceans has also enhanced thermal stratification of the water column decreasing vertical mixing and, in turn, has reduced primary productivity and lowered planktonic food availability (Roemmich and McGowan, 1995; Behrenfeld et al., 2006; Boyce et al., 2010), a scenario which may render organisms feeding at or near the base of marine food webs more vulnerable to climate change stressors (Melzner et al., 2011; Pansch et al., 2014; Ramajo et al., 2016). Additionally, changes in the carbonate chemistry (e.g., pCO2 and pH) of seawater and/or temperature may result in match-mismatch scenarios that separate predators and prey over time during important feeding periods (Durant et al., 2007; Parry et al., 2007; Siddon et al., 2013).
The objective of this study was to assess the individual and combined effects of varying levels of pCO2, temperature, and food supply on the early development and survival of larval Menidia beryllina (inland silverside) and Cyprinodon variegatus (sheepshead minnow). Both species are small schooling fish, native to Northeast US estuaries, feed at or near the base of marine food webs, and are critical trophic intermediaries transferring energy to higher trophic levels (i.e., commercially harvested species). Both species exist along a broad thermal range (e.g., ~0–30°C, Middaugh and Hemmer, 1992) and become reproductively active in temperate latitudes as water temperatures warm (e.g., ~13°C) in early-spring and continue to lay eggs into the summer (Middaugh and Hemmer, 1992; Able and Fahay, 1998), periods when warm and acidified conditions can become established within temperate, net heterotrophic estuaries (Melzner et al., 2013; Wallace et al., 2014; Baumann et al., 2015). Despite their abundance in Northeast US coastal systems, as well as the persistence of coastal acidification and other stressors (see above) within their geographical range, the current and future impacts of concurrent exposure to multiple marine stressors are unknown. In addition, these two fish species are useful for comparative purposes, as they lay pelagic and demersal eggs, respectively (Able and Fahay, 1998), and have been previously shown to be vulnerable and resistant to ocean acidification, respectively (DePasquale et al., 2015).
Methods
Care and Maintenance of Organisms
Experiments were performed at the Stony Brook Southampton Marine Science Center (Southampton, NY, USA). Embryos of M. beryllina and C. variegatus, were obtained ≤ 36 h post-fertilization from Aquatic Research Organisms (ARO; Hampton, NH) and originated from hundreds of wild-collected adult broodstock maintained at 21–22°C at a salinity of ~30. Ethical approval was provided by Stony Brook University's Institutional Animal Care and Use Committee (IACUC #; 2010 - 1842 - R2 - 12.16.16 – FI). Care was taken to limit the number of animals sacrificed during the course of experimentation and to prevent unnecessary pain and/or suffering. Upon the completion of experiments, animals were euthanized and preserved in a 3% (v/v) phosphate buffered formalin solution.
Temperature and Carbonate Chemistry Manipulations
Treatment levels for all trials were identified based upon ideal conditions for model organisms (Able and Fahay, 1998), present-day conditions within the geographical ranges for these species (Murray et al., 2014; Wallace et al., 2014; Baumann et al., 2015), and future climate change projections for coastal oceans along the Northeast US (Heogh-Guldberg et al., 2014; IPCC, 2014). To achieve desired exposure temperatures and to maintain identical conditions among experimental replicates within treatments, aquaria were held in temperature-controlled water baths maintained with electronically controlled heat exchangers (Aquatic Eco-systems, Inc., Florida, USA) that remained within ≤ 1% of target temperatures for the duration of the experiment. Temperatures within control treatments (19–22°C) were maintained at ambient temperatures and due to fluctuations between separately conducted trials, slight differences in control conditions were observed. However, fluctuations were minor and temperatures between control conditions and experimentally-manipulated conditions remained statistically distinct (see Tables 1–5). Carbonate chemistry within elevated pCO2 (2,042 ± 328 μatm) treatment replicates (n = 4) was maintained via the inclusion of concentrated (5%) CO2 gas mixed with ambient air (see Tables 1–5) added directly to each experimental vessel. Multichannel gas proportioners (Cole Parmer®) were used to control the mixing rates of gases (CO2 and air) to achieve targeted low pH levels (pH on the total scale = pHT ~7.2) within exposure aquaria. Ambient air (~400 μatm CO2) was bubbled into ambient-treatment aquaria (n = 4) at similar rates (~500 mL min−1) to those within elevated CO2 treatments. Target pH levels within ambient CO2 treatments were ~7.9 (pHT, 397 ± 58 μatm), levels representative of ideal conditions along the Northeast US coastline (Wallace et al., 2014; Baumann et al., 2015). The rate of gas delivery turned over the full volume of experimental chambers several times daily and sporadic dissolved oxygen measurements using a YSI 5100 probe indicated this kept dissolved oxygen levels at or near saturated levels during experiments.
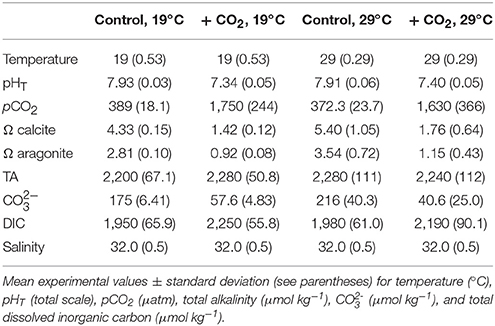
Table 1. Seawater chemistry for the experiment investigating temperature and CO2 effects on Menidia beryllina.
Temperature and pHT values were recorded daily using a Honeywell Durafet Ion-Sensitive Field-Effect Transistor (ISFET)-based pH sensor calibrated with a seawater pH standard (Dickson, 1993). In addition to daily measurements, temperatures were continuously monitored with in situ data loggers (HOBO Onset©). Discrete samples were taken prior to and after the conclusion of each experiment and analyzed for total dissolved inorganic carbon (DIC) using an infrared-based Environmental Gas Analyzer (EGM-4, PP Systems) calibrated with sodium bicarbonate standards. Certified reference material for DIC (University of California San Diego, Scripps Institution of Oceanography certified reference material for DIC, Batches 132-147) was analyzed before and after each analytical run and yielded recoveries of 103 ± 5%. Levels of pCO2 were calculated based on measured levels of DIC, pH, temperature, salinity, phosphate, silicate, and known first and second dissociation constants of carbonic acid in seawater (Millero, 2010) using CO2SYS software (http://cdiac.ornl.gov/ftp/co2sys/).
Interactive Effects of Temperature and High CO2—Menidia beryllina
To investigate interactive effects of pCO2 and temperature on the early life history of M. beryllina, fertilized eggs were exposed to simulated high pCO2 (low pH) environments at varying temperatures. Eighty eggs were included in each 8-L exposure aquaria (n = 4 replicates treatment−1) at start of each of two separately conducted trials. The first experiment (Trial 1) involved exposures of larval fish (embryonic—late-larval phases) to ambient (~400 μatm CO2) and elevated pCO2 (~2,000 μatm) concurrently exposed to temperatures near (19–21°C) or above (29–30°C) their current thermal optimum (Environmental Protection Agency, 1976; Table 1). Fish during the second experiment (Trial 2) were exposed to similar levels of pCO2 but, in addition to temperatures near their thermal optimum (e.g., 19–20°C), were subjected to an expanded thermal gradient (23, 27, and 30°C; see Table 2). The number of eggs and/or larvae present per experimental vessel was recorded daily. Upon hatching, fish were fed live-cultured rotifers (Brachionus plicatilis; 400 rotifers individual−1 day−1), after day 5 (post-hatch) larvae were fed brine shrimp (Artemia salina; 100 shrimp individual−1 day−1). The addition of food sources to experimental chambers had minimal impact on carbonate chemistry and the delivery rates of gases (e.g., ambient air and 5% CO2), if needed, were adjusted to ensure experimental parameters remained at or near to targeted levels. Feeding rates for temperature and pCO2 exposures, as defined by the US Environmental Protection Agency (EPA), were considered to be ad libitum (Environmental Protection Agency, 1976). Approximately 50% of water was exchanged within exposure aquaria every other day to limit the buildup of waste products and/or leftover food. Experiments concluded as initial mortality rates declined, 12 and 16 days post-fertilization for the first and second trial, respectively. Sub-samples of fish larvae were preserved in a 3% (v/v) phosphate buffered formalin solution and total length determined using a dissecting microscope and ImageJ® image analysis software.
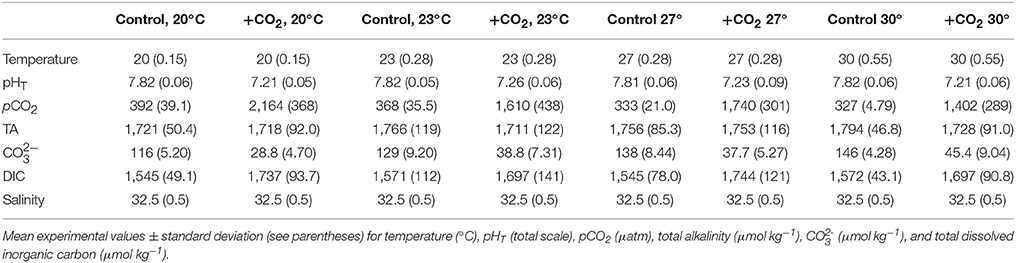
Table 2. Seawater chemistry for the experiment investigating expanded temperature and CO2 effects on Menidia beryllina.
Interactive Effects of High CO2 and Food-Limitation—Menidia beryllina
An additional experiment was conducted to examine the effect of high pCO2 and food availability on M. beryllina (Trial 3). Carbonate chemistry (elevated and ambient pCO2), fish embryos (n = 80 embryos aquaria−1, n = 4 replicate aquaria treatment−1), and experimental aquaria were maintained and monitored as described above (see Table 3). Upon hatching individuals in fed treatments were provided an optimal diet throughout the experiment as described above. Individuals in food-limited treatments were fed 20% of their optimal diet (see Environmental Protection Agency, 1976). The number of eggs and/or larvae present per experimental vessel was recorded daily. Sub-samples of fish larvae were preserved at the conclusion of experimentation and total lengths of fish from each experimental treatment quantified (detailed above). Experiment concluded ~10 days post-hatch as initial rates of mortality declined.
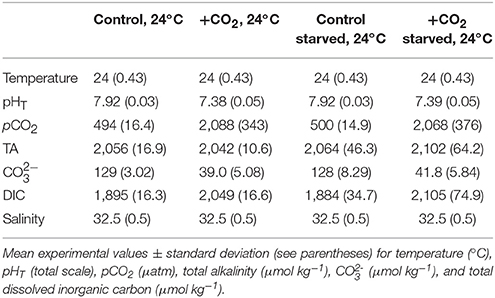
Table 3. Seawater chemistry for experiments investigating food-limitation and effects of high pCO2 on Menidia beryllina.
Interactive Effects of High CO2, Elevated Temperature, and Food-Limitation— Menidia beryllina and Cyprinodon variegatus
The combined effects of elevated pCO2, increased temperature, and food-limitation on the early-life stages of both M. beryllina and C. variegatus were characterized (Trials 4 and 5, respectively). Embryos (n = 80 embryos aquaria−1, n = 4 replicates treatment−1) collected from M. beryllina broodstock (detailed above) were added to 8-L polyethylene aquaria at temperatures near (e.g., 20–22°C) and above (30°C) their thermal optimum. Within each temperature, high, and ambient pCO2 (see above) conditions were paired with optimal and sub-optimal (e.g., 20% of optimal; Environmental Protection Agency, 1976) food conditions. The maintenance and monitoring of experiments was conducted as described above (see Table 4). Upon hatching, the percentage of eggs hatching and/or number of surviving larvae was quantified within each aquaria daily. Sub-samples of larvae from each experimental replicate were preserved at the conclusion of the experiment and total lengths quantified (as described above).
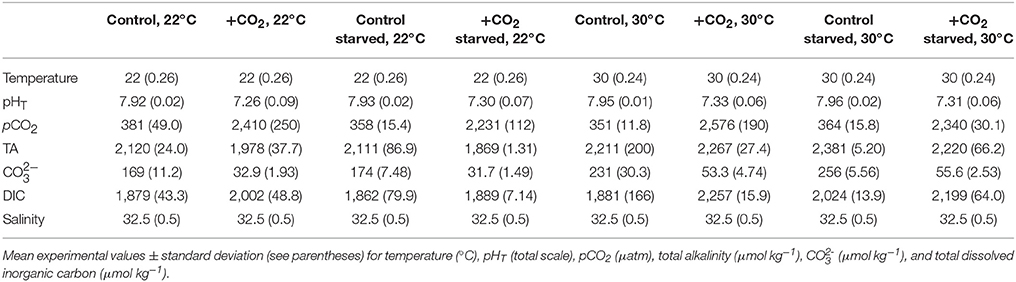
Table 4. Seawater chemistry for experiments investigating temperature, food-limitation, and high pCO2 effects on Menidia beryllina.
For C. vareigatus, embryos (n = 80 embryos aquaria−1) were added to each experimental vessel (n = 4 replicate treatment−1) and exposed to temperatures below (16°C), near (23°C), and above 30°C their thermal optimum at high and ambient pCO2 (see above). Within the 23°C treatment only, high and ambient pCO2 conditions were coupled with fed and food-limited conditions (e.g., 20% of optimal). The maintenance of organisms and monitoring of all experimental parameters were conducted as described for all preceding experimental trials (Table 5). Sub-sets of larvae were preserved and total lengths of surviving individuals from each exposure vessel were quantified as detailed above.
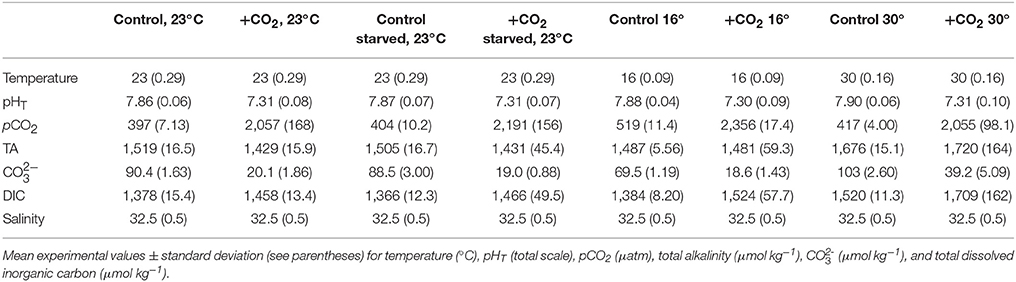
Table 5. Seawater chemistry for experiments investigating temperature, food-limitation, and high pCO2 effects on Cyprinodon variegatus.
Statistical Analysis
Differences in the time to hatch, hatch success, survival, and size (e.g., mean total length of survivors) of M. beryllina exposed to varying temperature and pCO2 were analyzed using a two-way analysis of variance (ANOVA, Trials 1 and 2). To interpret the effects of food limitation in combination with elevated pCO2 conditions on the final survival and size of M. beryllina larvae, a two-way ANOVA was conducted (see Trial 3). For Trial 3, as fish embryos do not feed exogenously until post-hatch, dietary effects on time to hatch and/or hatch percentage were not assessed. As such, differences in the hatching success and time to hatch among M. beryllina embryos exposed to varying carbonate chemistry regimes were analyzed using a Welch's t-test by pooling fed and food-limited treatment means within respective pCO2 treatments. The effects of temperature, food-limitation, and pCO2 on the final size and survival of M. beryllina larvae were assessed using a three-way ANOVA (Trial 4). Similarly, to interpret effects of pCO2 and temperature on the time to hatch and overall hatch success on M. beryllina embryos, a two-way ANOVA was conducted by pooling mean responses among fed and food-limited treatments within each exposure temperature and pCO2 level. The effects of temperature, pCO2, and food-limitation on the final size and survival of C. variegatus larvae were compared using a three-way ANOVA (Trial 5). Differences in the time to hatch and overall hatch success among C. variegatus embryos subjected to elevated and ambient pCO2 levels were compared using a Welch's t-test. Again, within 23°C exposure treatments, fed and food-limited treatment means were pooled within each respective pCO2 treatment prior to analysis. When significant differences were detected amongst main effects (e.g., temperature, pCO2, nutrition), a Holm-Sidak procedure for multiple comparisons was used to identify the source of variance. Assumptions of normality and homoscedasticity were assessed and confirmed using Lilliefors (Kolmogorov–Smirnov) and Levene's tests, respectively. To correct violations of a normal distribution and/or homogeneity of variance an arcsine-square-root transformation was applied as needed. All results were deemed significant at α ≤ 0.05. All analyses were performed using SigmaPlot™ 11.0 and R© (www.cran.r-project.org, Version 3.2.5) statistical software. Experimental conditions within experimental aquaria were monitored multiple times daily assuring that conditions among replicate aquaria within each treatment were identical and that variables influencing the normal growth and development of model organisms other than those experimentally controlled were entirely random among treatments and among replicates within each treatment.
Results
Interactive Effects of Temperature and High CO2—Menidia beryllina
Within the first trial (see Trial 1) temperature had a significant impact on the hatching time of M. beryllina, with lower temperature (19°C) extending the hatch time of eggs (p < 0.001, two-way ANOVA, Figure 1A) by five days relative to the warmer treatment (29°C). Within the cooler treatment overall hatch success was 80 ± 12%, a significant (p < 0.01, two-way ANOVA) reduction relative to the warm treatment (96 ± 3.1%; p < 0.01, two-way ANOVA, Figure 1B). Elevated pCO2, at warmer temperature, had significant (p < 0.01, two-way ANOVA; Figure 1C) impacts on larval survival 10 days post-hatch, with the elevated pCO2 treatment yielding lower survival (6.0 ± 2.0%; p < 0.05; Holm-Sidak) rates than the ambient treatment (35 ± 7.9%). In addition, there were significant (p < 0.001, two-way ANOVA) effects of temperature on the final size of larvae (Figure 1D). Individuals within the cooler (19°C) temperature treatment, regardless of pCO2 level, were significantly (p < 0.05, Holm-Sidak) smaller (mean length = 4.3 ± 0.14 mm) than larvae within the warmer (29°C) treatment (mean length = 5.9 ± 0.68 mm) at 10 days post-hatch. There was also a significant (p < 0.05, two-way ANOVA) effect of pCO2 on final size of fish within the warm temperature treatment only (e.g., 30°C), with fish exposed to elevated pCO2 displaying a 15 ± 0.1% reduction in total length relative to ambient pCO2-treatment fish although there was no interaction with temperature (p < 0.001, Holm-Sidak, Figure 1D).
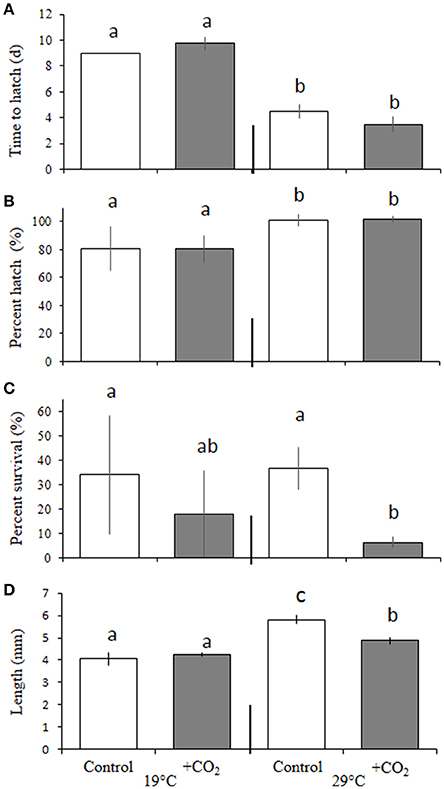
Figure 1. Temperature and pCO2 effect Menidia beryllina. (A) Days to hatch. (B) Percent hatched. (C) Percent survival. (D) Length. Bars represent the mean (n = 4) and error bars represent the standard deviation (letters denote significant groupings).
In the experiment with an expanded temperature range (e.g., Trial 2; 20, 23, 27, and 30°C) considered in tandem with ambient and elevated levels of pCO2 (~400 and 2,000 μatm, respectively), temperature again, significantly (p < 0.05, two-way ANOVA) altered the time to hatch of embryos. Larvae within the cooler (20°C) temperature treatment took significantly longer to hatch (11.0 ± 0.0 days) than eggs incubated at 23 (8.4 ± 1.2 days), 27 (6.1 ± 0.4 days) and 30°C (7.3 ± 0.65 days; p < 0.001, Holm-Sidak, Figure 2). Similar to Trial 1, temperature (p < 0.05, two-way ANOVA), but not CO2, controlled hatching success across broader temperature regimes (Figure 2B). Hatch success rates within 27 and 30°C treatments were 94 ± 0.9 and 85 ± 4.3%, respectively, with the percentage of individuals hatching at 20°C (61 ± 5.6%) being significantly (p < 0.05, two-way ANOVA) depressed relative to individuals reared at 23 (67 ± 1.0%) and 27°C, and successes at 23°C being significantly (p < 0.05, two-way ANOVA) depressed relative to those at 27°C. Temperature also had a significant (p < 0.01, two-way ANOVA) impact on larval survival (10 days post-hatch, Figure 2C). Survival was greatest at 27°C (80 ± 11%) and significantly (p < 0.01: Holm-Sidak) greater than survival at 20 (60 ± 8.6%) and 23°C (71 ± 6.0%), respectively. Temperature, again, had significant (p < 0.05, two-way ANOVA) effects on the growth of larvae with larvae reared at 20°C exhibiting 16 ± 0.07, 21 ± 0.05, and 18 ± 0.08% reductions in length relative to fish raised at 23, 27, and 30°C, respectively, 10 days post-hatch (all p < 0.01, Holm-Sidak, Figure 2D).
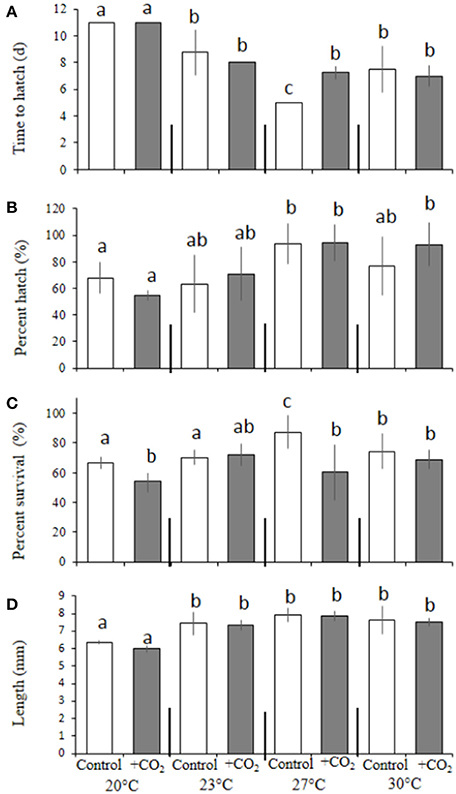
Figure 2. Expanded effects of temperature and pCO2 on Menidia beryllina. (A) Days to hatch. (B) Percent hatched. (C) Percent survival. (D) Length. Bars represent the mean (n = 4) and error bars represent the standard deviation (letters denote significant groupings).
There was no independent effect of pCO2 on larval survival, but a significant (p < 0.05, two-way ANOVA) interactive effect between temperature and pCO2 was observed (Figure 2C). Specifically, elevated pCO2 (~2,000 μatm) reduced larval survival within 20 and 27°C treatments only, where 54 ± 6.0 and 60 ± 18% of individuals survived, a significant (p < 0.05, Holm-Sidak) reduction relative to the survival of rates of 67 ± 4.1 and 87 ± 11% observed in fish maintained at the same temperatures but exposed to lower pCO2 (~400 μatm) levels.
Interactive Effects of High CO2 and Food-Limitation—Menidia beryllina
Levels of pCO2 had no significant bearing on time to hatching or the overall hatching success among M. beryllina embryos (Figures 3A,B; p > 0.05, Welch's t-test), but did significantly (p < 0.05, two-way ANOVA) lower larval survival (Figure 3C). In addition, a significant interactive (p < 0.05, two-way ANOVA) effect between diet and pCO2 was observed (Figure 3C) whereby, survivorship of 10 day post-hatch larvae was greater in the fed treatment (14 ± 13%) compared with the low food treatment (4 ± 5%) in the high pCO2 groups, but there was no difference in the survivorship between food treatment in the controls (71 ± 4.57%; Figure 3C). With regards to final size, levels of pCO2 and diet both had significant independent effects on total fish length (p < 0.001, two-way ANOVA, Figure 3D). Fish within control treatments, were 11 ± 0.5% larger than the elevated pCO2 treatment-fish and were 14 ± 0.6% larger than fish provided with less food at ambient pCO2 (both p < 0.01, Holm-Sidak, Figure 3D).
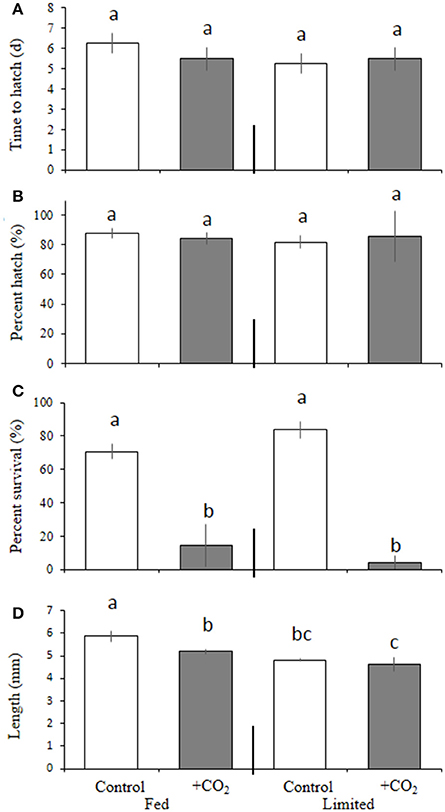
Figure 3. Diet and pCO2 effect on Menidia beryllina. (A) Days to hatch. (B) Percent hatched. (C) Percent survival. (D) Length. Bars represent the mean (n = 4) and error bars represent the standard deviation (letters denote significant groupings).
Interactive Effects of High CO2, Temperature, and Food-Limitation— Menidia beryllina
Warmer temperature significantly (p < 0.001, two-way ANOVA) decreased egg incubation time for M. beryllina (Figure 4A), with embryos incubated at 22°C hatching within 9.6 ± 0.8 days, whereas the mean time to hatch of embryos incubated at 30°C was 6.3 ± 1.2 days (p < 0.05, Holm-Sidak, Figure 4A). Temperature and pCO2 did not significantly (p > 0.05, two-way ANOVA) alter hatch success (Figure 4B). However, significant (p < 0.001, three-way ANOVA) dietary effects were observed whereby survival, regardless of pCO2 and temperature, was decreased (p < 0.05, three-way ANOVA) within food-limited treatments (7.4 ± 14%) relative to fish fed ad libitum (52 ± 17%; Figure 4C). Significant (p < 0.05, three-way ANOVA), temperature-driven reductions in survival were observed within the 30°C treatment only (Figure 4C). Elevated pCO2 also had significant (p < 0.001, three-way ANOVA) effects on larval survival (10 days post-hatch). The mean survival of fish exposed to elevated pCO2, regardless of temperature and nutrition, was 44 ± 3% and significantly reduced relative to the survival of fish (59 ± 5%) exposed to ambient pCO2 (Figure 4C). In addition, final lengths were significantly (p < 0.001, three-way ANOVA) impacted by dietary conditions (Figure 4D) with larvae provided with less food, regardless of pCO2 and temperature levels, displaying a 25 ± 0.4% reduction in total length relative to adequately-nourished counterparts (Figure 4D).
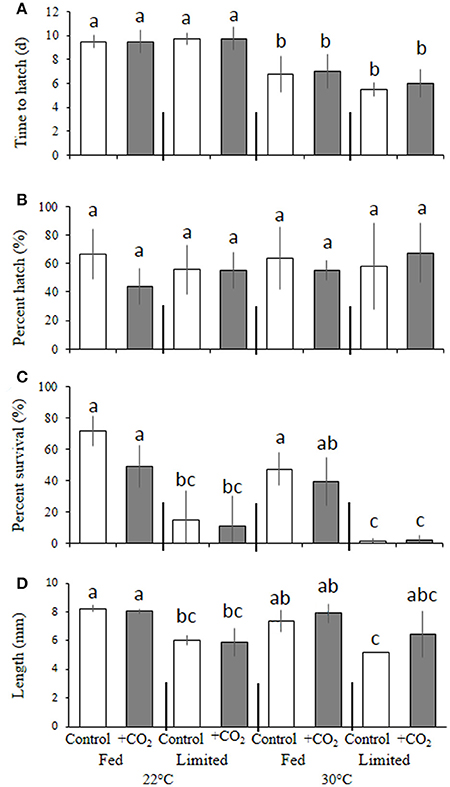
Figure 4. Temperature, food, and pCO2 effect on Menidia beryllina. (A) Days to hatch. (B) Percent hatched. (C) Percent survival. (D) Length. Bars represent the mean (n = 4) and error bars represent the standard deviation (letters denote significant groupings).
Interactive Effects of High CO2, Temperature, and Food-Limitation— Cyprinodon variegatus
In a manner similar to M. beryllina, temperature significantly affected the time to hatch (e.g., 100% hatch) of C. variegatus (p < 0.001, two-way ANOVA), where the hatching times of fish exposed to 30°C (5.8 ± 0.9 days), regardless of pCO2 level, were significantly (p < 0.001, Holm-Sidak) more rapid than fish reared at 16 and 23°C (12 ± 4.1 and 34 ± 5.9 days, respectively; Figure 5A). Hatching success was also significantly (p < 0.05, two-way ANOVA) affected by temperature. The mean percentage of eggs hatching within the warmer (30°C) temperature treatment was 87 ± 7.3% and significantly (p < 0.001, Holm-Sidak) reduced to 77 ± 7.5 and 59 ± 3.5% at 23 and 16°C, respectively (Figure 5B). Survival (10 days post-hatch) was also significantly (p < 0.001, two-way ANOVA) affected by temperature, with the lower temperature treatment (16°C) yielding greater (p < 0.01, Holm-Sidak) rates of survival (94 ± 0.2%) than those maintained in the 23 (87 ± 0.1%) and 30°C treatments (38 ± 0.1%; Figure 5C). Again, significant interactive effects (p < 0.05, two-way ANOVA) were observed, with temperature and elevated pCO2 causing reductions in survival among larvae at high and low temperature treatments only (16 and 30°C; both p < 0.05, Holm-Sidak, Figure 5C).
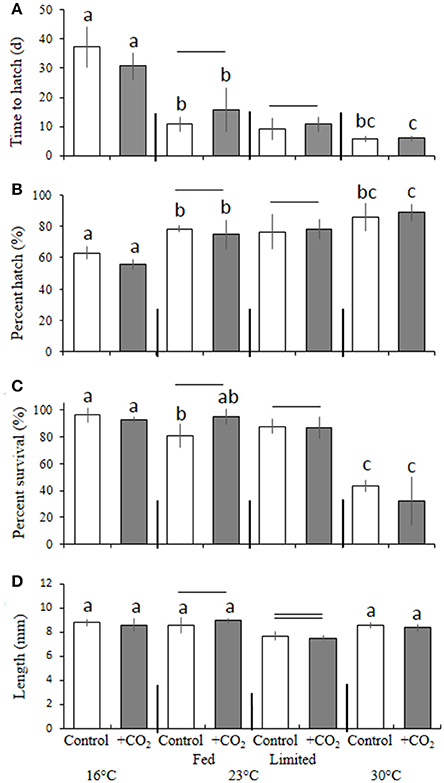
Figure 5. Temperature, food, and CO2 effect on Cyprinodon variegatus. (A) Days to hatch. (B) Percent hatched. (C) Percent survival. (D) Length. Bars represent the mean (n = 4) and error bars represent the standard deviation (letters denote significant groupings among temperature and CO2 temperature and CO2 treatment, — Denote significant groupings among food treatments within 23°C only; all p < 0.05).
With regards to final size, among fish reared at 23°C, dietary condition had significant (p < 0.05, two-way ANOVA) impacts on final size, whereby fed larvae were 9 ± 0.4% larger than counterparts provided with 80% less food (Figure 5D). In addition, larvae within adequately-fed treatments and exposed to elevated pCO2 were significantly (12 ± 0.1%; p < 0.001, Holm-Sidak) larger than fish exposed to similar pCO2 conditions, but provided with less food (Figure 5D).
Discussion
Temperate estuaries are important nursery and breeding grounds for forage fish (Pikitch et al., 2014) but are prone to fluctuations in pCO2, temperature, and phytoplankton levels during the spawning season (Nixon et al., 2004; Baumann and Doherty, 2013; Wallace et al., 2014; Baumann et al., 2015). Climate changes are predicted to intensify these variations in the near future (Doney et al., 2012). Here, we demonstrate that changing temperatures, rising pCO2 levels, and reduced food supplies can individually and synergistically act to reduce survival and development of small schooling fish native to Northwest Atlantic estuaries. These findings provide novel insight regarding the presence of multiple climate change-associated stressors within temperate estuaries and their potential impacts on associated fisheries.
Temperature plays a central role in early-embryonic and larval fish development (Houde, 1989; Pepin, 1991). Many marine fish lack the ability to regulate their internal body temperature and generally inhabit waters at or near the limits of their thermal niche (Sunday et al., 2012), a common trend among ectothermic organisms. Therefore, temperature has direct control over basal metabolic demands and can alter nutritional requirements of fish in temperature-altered environments (Sherman et al., 1984; Kucharczyk et al., 1997; Bobe and Labbé, 2010). Results presented here indicate that rising temperature may accelerate hatching times and improve hatching rates, outcomes that could be of potential benefit to population levels for these species existing in warmed estuaries (Lasker, 1981; Sissenwine, 1984). However, these benefits were short-lived as larval survival (10 days post-hatch) within the highest temperature treatments was, for M. beryllina, similar or worse than larvae reared at near-optimal temperatures and was significantly lower for C. variegatus, perhaps due to temperature-accelerated hatching occurring before complete embryonic development (Kucharczyk et al., 1997). The rapid shift in outcomes for fish reared at elevated temperature from positive to neutral or negative for embryos and larval stages, respectively, suggests warm temperatures that accelerate development result in metabolic rates that are unsustainable for early life-stage fish. These outcomes may be may become progressively worsened throughout later-larval and early-juvenile development.
As ocean waters warm, fish are migrating into deeper and/or higher latitude environments (Sunday et al., 2012) and in some cases, these new, cooler habitats may be slightly outside of their optimal thermal range (Nye et al., 2009). Results presented here suggest such movements may affect productivity within impacted populations. During experiments with M. beryllina, fish developing at cooler temperatures exhibited lower survival and slower growth, likely a result of reduced metabolism and decreased ability to convert energy into growth (Kucharczyk et al., 1997). In contrast to M. beryllina, C. variegatus seemed to thrive in cooler temperatures with survival and length being maximal at 16°C despite an extended incubation time for eggs. This outcome may be related to differences in early-life histories of the two organisms as unlike M. beryllina eggs that are deposited off-bottom on vegetation, C. variegatus deposit eggs on the seabed (Able and Fahay, 1998) where temperatures are often cooler, perhaps making them better adapted to lower temperatures.
Another consequence of rising temperatures is accelerated rates of nutrient acquisition by some phytoplankton. Decreases in nutrients coupled with temperature-enhanced rates of herbivory may reduce phytoplankton biomass (Rose and Carron, 2007). In addition, temperature-induced stability of the water column may inhibit vertical mixing of nutrients that further limit plankton inventories within surface waters (Roemmich and McGowan, 1995; Behrenfeld et al., 2006; Boyce et al., 2010). Such conditions (i.e., food-limitation) have been shown to render some planktivores more vulnerable to climate change stressors such as acidification (Melzner et al., 2011; Pansch et al., 2014; Ramajo et al., 2016). During this study, restricted food supplies significantly reduced survival and resulted in M. beryllina larvae that were smaller. There were also complex interactions between food supply and pCO2 levels for larval M. beryllina in a manner somewhat consistent with prior studies involving early-life stage bivalves (see Melzner et al., 2011). Specifically, the survival of M. beryllina larvae was depressed when food was limited and pCO2 was increased, more so than would be predicted based upon singular exposures with either stressor. Similar patterns have been observed among tropical marine fish exposed to high pCO2 environments, whereby fish within high pCO2 treatments were more sensitive to starvation than fish exposed to ambient pCO2 regimes (Bignami et al., 2016). Physiologically, this is intuitive as the stress of acidification likely increased basal energy demands rendering fish more vulnerable to food-limited conditions (Pörtner and Farrell, 2008). In contrast to the patterns in fish survival that were synergistically suppressed by low food and high pCO2, fish lengths that were depressed by food restriction or pCO2 were not further depressed by both stressors, perhaps due to the newly-hatched fish already being at a minimal size.
Larval stage fish, in general (Chambers et al., 2014; Stiasny et al., 2016), and M. beryllina (Baumann et al., 2012; DePasquale et al., 2015) in particular have been shown to be sensitive to acidification, more so than their later-life-stage counterparts. During this study, increased pCO2 reduced the number of M. beryllina larvae that survived to 10 days in all four experiments performed. This 10-day mark is a critical bottle-neck in the early-life cycle for M. beryllina larvae, as it is the time point at which larvae become less reliant upon maternally-derived energy stores (i.e., yolk sac), more dependent upon external sources, and are more physiologically adept to cope with environmental stressors (Mangor-Jensen, 1987; Perry and Gilmour, 2006; Ishimatsu et al., 2008; Baumann et al., 2012). M. beryllina also exhibited shorter lengths when exposed to increased pCO2 levels, a consequence that could lead to enhanced mortality and a prolonged period of predation within an ecosystem setting (Ware, 1975; Peterson and Wroblewski, 1984; Sogard, 1997).
In contrast to M. beryllina, elevated pCO2 alone had no effect on C. variegatus, a finding consistent with prior studies involving this species (see DePasquale et al., 2015) and perhaps with its preferred habitat as this species lays eggs on bottom sediments (Chitty and Able, 2004) that can regularly exhibit elevated pCO2 levels in temperate estuaries (Wallace et al., 2014). However, larval C. variegatus became vulnerable to high pCO2 when it occurred in tandem with temperatures above or below its thermal optimum (e.g., 16 or 30°C), conditions that yielded significantly higher rates of mortality for this species. This outcome demonstrates a key mechanism by which fisheries may be impacted by climate change. Embryos and larval fish exposed to temperatures outside of their thermal optimum may expend more energy maintaining homeostasis and thus may be less capable of coping with additional stressors (Pörtner and Farrell, 2008). Similarly, acidification can cause the narrowing of an organism's thermal tolerance (Pörtner and Knust, 2007; Pörtner and Farrell, 2008). Results presented here demonstrate that even acidification-tolerant fish may be negatively impacted by high pCO2 if they are concurrently exposed to elevated temperature.
Synergistic effects of temperature and pCO2 were also observed within trials involving M. beryllina. For example, within treatments at optimal and elevated temperatures, high pCO2 resulted in smaller larvae at warmer, and not optimal, temperatures. When thermal gradients were expanded, elevated pCO2 caused significant reductions in survival only at low and high exposure temperatures and not at optimal temperatures. In the same experiment, hatching success was inhibited by high pCO2 at low but not high temperature. This result, as well as depressed mortality at lower temperature and elevated pCO2, are not surprising given that prior research has found that the negative effects of high pCO2 on M. beryllina are largely the result of embryonic rather than larval exposure to elevated pCO2 (Baumann et al., 2012). Hence, cooler temperatures that extend egg-hatching times and thus lengthen the time during which embryos experience the negative effects of acidification ultimately cause greater rates of embryonic and larval mortality. The synergistically-negative effects of elevated pCO2 on larval fitness at higher temperatures reported here are in agreement with other theoretical frameworks of organismal physiology that suggest acidification depresses thermal tolerances (e.g., Pörtner, 2008, 2010). These outcomes demonstrate the importance of considering the combined effects of multiple climate change stressors on marine life, as the effects of multiple stressors can be unexpected compared to the individual effects of each stressor (Gobler et al., 2014; Gobler and Baumann, 2016).
Recent investigations suggest adaptive potential among marine forage fish exposed to climate change stressors. Larval fish originating from adults acclimated to elevated temperature produced larvae that were resistant to elevated temperatures themselves (Salinas and Munch, 2012). Additionally, the sensitivity of larval fish challenged with high pCO2 was observed to vary seasonally and with conditions present during gametogenesis (Murray et al., 2014). Specifically, larvae spawned earlier in the season, prior to the onset of seasonal acidification (i.e., coastal acidification see, Wallace et al., 2014; Baumann et al., 2015), displayed greater sensitivity to low pH than larvae spawned later in the season originating from adults acclimated to seasonal decreases in pH (Murray et al., 2014), potentially due to maternal provisioning (Snyder et al., 2018). Altogether, these recent findings suggest adaptive potential for fish exposed transgenerationally to climate change stressors in natural environments. Findings presented here, however, suggest that the presence of multiple co-stressors may render adaptation/acclimation to adverse conditions more challenging. Future transgenerational investigations should seek to incorporate multiple, additional stressors as results presented here and elsewhere (e.g., Clark and Gobler, 2016; Gobler et al., 2017) suggest that the combined impacts of stressors cannot be entirely predicted based upon singular exposures to individual stressors.
Conclusion
Acidification, thermal extremes, and sub-optimal food supplies occur within temperate-latitude estuaries and are expected to become more common as climate change progresses. However, little is known regarding the interactive effects of these stressors on most marine organisms. While pCO2 levels within the sea surface have increased by more than 40% since the Industrial Revolution, the consequences of these changes within marine ecosystems are not fully understood (Kroeker et al., 2013). This study has demonstrated that elevated pCO2 coupled with warming temperatures and limited food supplies can lead to significant reductions in growth and survival of small schooling fish that incubate, hatch, and remain in estuaries where these conditions are common and likely to worsen with climate change. Given that these fish species are integral components of coastal marine food webs and can influence the productivity of commercially significant fisheries, this study has important implications regarding the management of estuaries, future oceans, and coastal fisheries in climate-impacted regions. In addition, this work may have relevance to a broader range of fish, those with similar larval life histories that are outside the classification of small schooling fish (Houde, 1997). Population levels are also likely to be affected by these individual and combined climate change stressors, since year-classes are predominately controlled by larval mortality and growth and it has been shown that even small changes in these factors cause disproportionate changes in recruitment that can be orders of magnitude in effect (Houde, 1997).
Ethics Statement
Ethical approval was provided by Stony Brook University's Institutional Animal Care and Use Committee (IACUC #; 2010 - 1842 - R2 - 12.16.16 – FI). Care was taken to limit the number of animals sacrificed during the course of experimentation and to prevent unnecessary pain and/or suffering. Upon the completion of experiments, animals were euthanized and preserved in a 3% (v/v) phosphate buffered formalin solution.
Author Contributions
CG obtained funding for and designed experiments, analyzed data, and wrote the manuscript; LM designed experiments, conducted experiments, analyzed data, and wrote the manuscript; AG designed experiments, analyzed data, and wrote the manuscript; BM conducted experiments, analyzed data, and wrote the manuscript.
Conflict of Interest Statement
The authors declare that the research was conducted in the absence of any commercial or financial relationships that could be construed as a potential conflict of interest.
Acknowledgments
This research was supported by the New York Sea Grant (Award # R-FBM- 38), the Laurie Landeau Foundation, the Simons Foundation, and the Chicago Community Trust.
References
Able, K. W., and Fahay, M. P. (1998). First Year in the Life of Estuarine Fishes in the Middle Atlantic Bight. Rutgers University Press.
Baumann, H., and Doherty, O. (2013). Decadal changes in the world's coastal latitudinal temperature gradients. PLoS ONE 8:e67596. doi: 10.1371/journal.pone.0067596
Baumann, H., Talmage, S. C., and Gobler, C. J. (2012). Reduced early life growth and survival in a fish in direct response to increased carbon dioxide. Nat. Clim. Change 2, 38–41. doi: 10.1038/nclimate1291
Baumann, H., Wallace, R. B., Tagliaferri, T., and Gobler, C. J. (2015). Large natural pH, CO2 and O2 fluctuations in a temperate tidal salt marsh on diel, seasonal, and interannual time scales. Estuar. Coasts 38, 220–231. doi: 10.1007/s12237-014-9800-y
Behrenfeld, M. J., O'Malley, R. T., Siegel, D. A., McClain, C. R., Sarmiento, J. L., and Feldman, G. C. (2006). Climate-driven trends in contemporary ocean productivity. Nature 444, 752–755. doi: 10.1038/nature05317
Bignami, S., Sponaugle, S., Hauff, M., and Cowen, R. K. (2016). Combined effects of elevated pCO2, temperature, and starvation stress on larvae of a large tropical marine fish. ICES J. Mar. Sci. 74, 1220–1229. doi: 10.1093/icesjms/fsw216
Bobe, J., and Labbé, C. (2010). Egg and sperm quality in fish. Gen. Comp. Endocrinol. 165, 535–548. doi: 10.1016/j.ygcen.2009.02.011
Boyce, D. G., Lewis, M. R., and Worm, B. (2010). Global phytoplankton decline over the past century. Nature 466, 591–596. doi: 10.1038/nature09268
Cai, W. J., Hu, X. P., Huang, W. J., Murrell, M. C., Lehrter, J. C., Lohrenz, S. E., Chou, W. C., et al. (2011). Acidification of subsurface coastal waters enhanced by eutrophication. Nat. Geosci. 4, 766–770. doi: 10.1038/ngeo1297
Chambers, R. C., Candelmo, A. C., Habeck, E. A., Poach, M. E., Wieczorek, D., Cooper, K. R., Greenfield, C. E., et al. (2014). Effects of elevated CO2 in the early life stages of summer flounder, Paralichthys dentatus, and potential consequences of ocean acidification. Biogeosciences 11, 1613–1626. doi: 10.5194/bg-11-1613-2014
Chitty, J. D., and Able, K. W. (2004). Habitati Use, Movements and Growth of the Sheepshead Minnow, Cyprinodon variegatus, in a restored salt marsh in Deleware Bay. The Bulletin, New Jersey Academy of Science.
Clark, H. R., and Gobler, C. J. (2016). Diurnal fluctuations in CO2 and dissolved oxygen concentrations do not provide a refuge from hypoxia and acidification for early-life-stage bivalves. Mar. Ecol. Prog. Ser. 558, 1–14. doi: 10.3354/meps11852
DePasquale, E., Baumann, H., and Gobler, C. J. (2015). Vulnerability of early life stage Northwest Atlantic forage fish to ocean acidification and low oxygen. Mar. Ecol. Prog. Ser. 523, 145–156. doi: 10.3354/meps11142
Dickson, A. G. (1993). The measurement of sea water pH. Mar. Chem. 44, 131–142. doi: 10.1016/0304-4203(93)90198-W
Doney, S. C., Fabry, V. J., Feely, R. A., and Kleypas, J. A. (2009). Ocean acidification: the other CO2 problem. Ann. Rev. Mar. Sci. 1, 169–192. doi: 10.1146/annurev.marine.010908.163834
Doney, S. C., Ruckelshaus, M., Duffy, J. E., Barry, J. P., Chan, F., English, C. A., et al. (2012). Climate change impacts on marine ecosystems. Ann. Rev. Mar. Sci. 4, 11–37. doi: 10.1146/annurev-marine-041911-111611
Durant, J. M., Hjermann, D., Ottersen, G., and Stenseth, N. C. (2007). Climate and the match or mismatch between predator requirements and resource availability. Clim. Res. 33, 271–283. doi: 10.3354/cr033271
Environmental Protection Agency (1976). Bioassay Procedures for the Ocean Disposal Permit Program. Gulf Breeze, FL: Environmental Research Laboratory Office of Research and Development U.S. Environmental Protection Agency.
Frommel, A. Y., Schubert, A., Piatkowski, U., and Clemmesen, C. (2013). Egg and early larval stages of Baltic cod, Gadus morhua, are robust to high levels of ocean acidification. Mar. Biol. 160, 1825–1834. doi: 10.1007/s00227-011-1876-3
Gobler, C. J., and Baumann, H. (2016). Hypoxia and acidification in ocean ecosystems: coupled dynamics and effects on marine life. Biol. Lett. 12:20150976. doi: 10.1098/rsbl.2015.0976
Gobler, C. J., Clark, H. R., Griffith, A. W., and Lusty, M. V. (2017). Dirunal flucutation in acidification and hypoxia reduce growth and survival of larval and juvenile bay scallops (Argopecten irradians) and hard clams (Mercenaria mercenaria). Front. Mar. Sci. 3:282. doi: 10.3389/fmars.2016.00282
Gobler, C. J., DePasquale, E. L., Griffith, A. W., and Baumann, H. (2014). Hypoxia and acidification have additive and synergistic negative effects on the growth, survival, and metamorphosis of early life stage bivalves. PLoS ONE 9:e83648. doi: 10.1371/journal.pone.0083648
Helluy, S. M., and Beltz, B. S. (1991). Embryonic development of the American lobster (Homarus americanus): quantitative staging and characterization of an embryonic molt cycle. Biol. Bull. 180, 355–371. doi: 10.2307/1542337
Heogh-Guldberg, O., Cai, R., Poloczanska, E. S., Brewer, P. G., Sundby, S., Hilmi, K., Fabry, V. J., et al. (2014). “The ocean,” in Climate Change 2014: Impacts, Adaptation, and Vulnerability. Part B: Regional Aspects. Contribution of Working Group II to the Fifth Assessment Report of the Intergovernmental Panel on Climate Change, eds V. R. Barros, C. B. Field, D. J. Dokken, M. D. Mastrandrea, K. J. Mach, T. E. Bilir, M. Chatterjee, K. L. Ebi, Y. O. Estrada, R. C. Genova, B. Girma, E. S. Kissel, A. N. Levy, S. MacCracken, P. R. Mastrandrea, and L. L. White (Cambridge, UK; New York, NY: Cambridge University Press), 1655–1731.
Houde, E. D. (1989). Comparative growth, mortality, and energetics of marine fish larvae: temperature and implied latitudinal effects. Fish. Bull. 87, 471–495.
Houde, E. D. (1997). Patterns and trends in larval-stage growth and mortality of teleost fish. J. Fish Biol. 51, 52–83. doi: 10.1111/j.1095-8649.1997.tb06093.x
IPCC (2014). Climate Change 2014: Synthesis Report. Contribution of working groups I, II and III to the Fifth Assessment Report of the Intergovernmental Panel on Climate Change, eds R. K. Pachauri and L. A. Meyer (Geneva: IPCC), 151.
Ishimatsu, A., Hayashi, M., and Kikkawa, T. (2008). Fishes in high-CO2, acidified oceans. Mar. Ecol. Prog. Ser. 373, 295–302. doi: 10.3354/meps07823
Kennedy, V. S., and Krantz, L. B. (1982). Comparative gametogenic and spawning patterns of the oyster Crassostrea virginica in central Chesapeake Bay, USA. J. Shellfish Res. 2, 133–140.
Kroeker, K. J., Kordas, R. L., Crim, R., Hendriks, I. E., Ramajo, L., Singh, G. S., et al. (2013). Impacts of ocean acidification on marine organisms: quantifying sensitivities and interaction with warming. Glob. Chang. Biol. 19, 1884–1896. doi: 10.1111/gcb.12179
Kroeker, K. J., Kordas, R. L., Crim, R. N., and Singh, G. G. (2010). Meta-analysis reveals negative yet variable effects of ocean acidification on marine organisms. Ecol. Lett. 13, 1419–1434. doi: 10.1111/j.1461-0248.2010.01518.x
Kucharczyk, D., Luczynski, M., Kujawa, R., and Czerkies, P. (1997). Effect of temperature on embryonic and larval development of bream (Abramis brama L.). Aquat. Sci. 59, 214–224. doi: 10.1007/BF02523274
Lasker, R. (1981). “The role of a stable ocean in larval fish survival and subsequent recruitment,” in Marine Fish Larvae: Morphology, Ecology and Relation to Fisheries, ed R. Lasker (Washington, DC: University of Washington), 81–87.
Mangor-Jensen, A. (1987). Water balance in developing eggs of the cod Gadus morhua L. Fish Physiol. Biochem. 3, 17–24. doi: 10.1007/BF02183990
Melzner, F., Stange, P., Trübenbach, K., Thomsen, J., Casties, I., Panknin, U., et al. (2011). Food supply and seawater pCO2 impact calcification and internal shell dissolution in the blue mussel Mytilus edulis. PLoS ONE 6:e24223. doi: 10.1371/journal.pone.0024223
Melzner, F., Thomsen, J., Koeve, W., Oschlies, A., Gutowska, M. A., Bange, H. W., et al. (2013). Future ocean acidification will be amplified by hypoxia in coastal habitats. Mar. Biol. 160, 1875–1888. doi: 10.1007/s00227-012-1954-1
Middaugh, D. P., and Hemmer, M. J. (1992). Reproductive ecology of the inland silverside, Menidia beryllina, (Pisces: Atherinidae) from Blackwater Bay, Florida. Copeia 1992, 53–61. doi: 10.2307/1446535
Miller, G. M., Watson, S.-A., Donelson, J. M., McCormick, M. I., and Munday, P. L. (2012). Parental environment mediates impacts of increased carbon dioxide on a coral reef fish. Nat. Clim. Chang. 2, 858–861. doi: 10.1038/nclimate1599
Millero, F. J. (2010). Carbonate constants for estuarine waters. Mar. Freshw. Res. 61, 139–142. doi: 10.1071/MF09254
Munday, P., Gagliano, M., Donelson, J., Dixson, D., and Thorrold, S. (2011). Ocean acidification does not affect the early life history development of a tropical marine fish. Mar. Ecol. Prog. Ser. 423, 211–221. doi: 10.3354/meps08990
Murray, C. S., Malvezzi, A., Gobler, C. J., and Baumann, H. (2014). Offspring sensitivity to ocean acidification changes seasonally in a coastal marine fish. Mar. Ecol. Prog. Ser. 504, 1–11. doi: 10.3354/meps10791
Nixon, S. W., Granger, S., Buckley, B. A., Lamont, M., and Rowell, B. (2004). A one hundred and seventeen year coastal water temperature record from woods hole, Massachusetts. Estuaries 27, 397–404. doi: 10.1007/BF02803532
Nye, J. A., Link, J. S., Hare, J. A., and Overholtz, W. J. (2009). Changing spatial distribution of fish stocks in relation to climate and population size on the Northeast United States continental shelf. Mar. Ecol. Prog. Ser. 393, 111–129. doi: 10.3354/meps08220
Pansch, C., Schaub, I., Havenhand, J., and Wahl, M. (2014). Habitat traits and food availability determine the response of marine invertebrates to ocean acidification. Glob. Chang. Biol. 20, 765–777. doi: 10.1111/gcb.12478
Parry, M. L., Canziani, O. F., Palutikof, J. P., van Linden, P. J., and Hanson, C. E. (2007). Contribution of Working Group II to the Fourth Assessment Report of the Intergovernmental Panel on Climate Change. The intergovernmental Panel on Climate Change.
Pepin, P. (1991). Effect of temperature and size on development, mortality, and survival rates of the pelagic early life history stages of marine fish. Can. J. Fish. Aquat. Sci. 48, 503–518. doi: 10.1139/f91-065
Perry, A. L., Low, P. J., Ellis, J. R., and Reynolds, J. D. (2005). Climate change and distribution shifts in marine fishes. Science 308, 1912–1915. doi: 10.1126/science.1111322
Perry, S. F., and Gilmour, K. M. (2006). Acid-base balance and CO2 excretion in fish: unanswered questions and emerging models. Respir. Physiol. Neurobiol. 154, 199–215. doi: 10.1016/j.resp.2006.04.010
Peterson, I., and Wroblewski, J. S. (1984). Mortality rate of fishes in the pelagic ecosystem. Can. J. Fish. Aquat. Sci. 41, 1117–1120. doi: 10.1139/f84-131
Pikitch, E. K., Rountos, K. J., Essington, T. E., Santora, C., Pauly, D., Watson, R., et al. (2014). The global contribution of forage fish to marine fisheries and ecosystems. Fish Fish. 15, 43–64. doi: 10.1111/faf.12004
Pinsky, M. L., Worm, B., Fogarty, M. J., Sarmiento, J. L., and Levin, S. A. (2013). Marine taxa track local climate velocities. Science 341, 1239–1242. doi: 10.1126/science.1239352
Poloczanska, E. S., Brown, C. J., Sydeman, W. J., Kiessling, W., Schoeman, D. S., Moore, P. J., et al. (2013). Global imprint of climate change on marine life. Nat. Clim. Chang. 3, 919–925. doi: 10.1038/nclimate1958
Pörtner, H. O. (2008). Ecosystem effects of ocean acidification in times of ocean warming: a physiologist's view. Mar. Ecol. Prog. Ser. 373, 203–217. doi: 10.3354/meps07768
Pörtner, H. O. (2010). Oxygen- and capacity-limitation of thermal tolerance: a matrix for integrating climate-related stressor effects in marine ecosystems. J. Exp. Biol. 213, 881–893. doi: 10.1242/jeb.037523
Pörtner, H. O., and Farrell, A. P. (2008). Physiology and climate change. Science 322, 690–692. doi: 10.1126/science.1163156
Pörtner, H. O., and Knust, R. (2007). Climate change affects marine fishes through the oxygen limitation of thermal tolerance. Science 315, 95–97. doi: 10.1126/science.1135471
Ramajo, L., Pérez-León, E., Hendriks, I. E., Marbà, N., Krause-Jensen, D., and Sejr, M. K. (2016). Food supply confers calcifiers resistance to ocean acidification. Sci. Rep. 6:19374. doi: 10.1038/srep19374
Roemmich, D., and McGowan, J. (1995). Climatic warming and the decline of zooplankton in the California current. Science 267, 1324–1326.
Rose, J. M., and Carron, D. A. (2007). Does low temperature constrain the growth rates of heterotrophic protists? Evidence and implications for algal blooms in cold waters. Limnol. Oceanogr. 52, 886–895. doi: 10.4319/lo.2007.52.2.0886
Sabine, C. L., Feely, R. A., Gruber, N., Key, R. M., Lee, K., Bullister, J. L., et al. (2004). The oceanic sink for anthropogenic CO2. Science 305, 367–371. doi: 10.1126/science.1097403
Salinas, S., and Munch, S. B. (2012). Thermal legacies: transgenerational effects of temperature on growth in a vertebrate. Ecol. Lett. 15, 159–163. doi: 10.1111/j.1461-0248.2011.01721.x
Sherman, K., Smith, W., Morse, W., Berman, M., Green, J., and Ejsymont, L. (1984). Spawning strategies of fishes in relation to circulation, phytoplankton production, and pulses in zooplankton off the northeastern United States. Mar. Ecol. Prog. Ser. 18, 1–19. doi: 10.3354/meps018001
Siddon, E. C., Kristiansen, T., Mueter, F. J., Holsman, K. K., Heintz, R. A., and Farley, E. V. (2013). Spatial match-mismatch between juvenile fish and prey provides a mechanism for recruitment variability across contrasting climate conditions in the eastern Bering Sea. PLoS ONE 8:e84526. doi: 10.1371/journal.pone.0084526
Sissenwine, M. P. (1984). “Why do fish populations vary?” in Exploitation of Marine Communities, ed R. M. May (Berlin: Springer-Verlag), 59–94.
Snyder, J. T., Murray, C. S., and Baumann, H. (2018). Potential for maternal effects on offspring CO2 sensitivities in the Atlantic silverside (Menidia menidia). J. Exp. Mar. Biol. Ecol. 499, 1–8. doi: 10.1016/j.jembe.2017.11.002
Sogard, S. M. (1997). Size-selective mortality in the juvenile stage of teleost fishes: a review. Bull. Mar. Sci. 60, 1129–1157.
Solomon, S. (2007). The Physical science Basis: Contribution of Working Group I to the Fourth Assessment Report of the Intergovernmental Panel on Climate Change. Cambridge University Press.
Stiasny, M. H., Mittermayer, F. H., Sswat, M., Voss, R., Jutfelt, F., Chierici, M., et al. (2016). Ocean acidification effects on Atlantic cod larval survival and recruitment to the fished population (CJ Gobler, Ed.). PLoS ONE 11:e0155448. doi: 10.1371/journal.pone.0155448.
Sunday, J. M., Bates, A. E., and Dulvy, N. K. (2012). Thermal tolerance and the global redistribution of animals. Nat. Clim. Chang. 2, 686–690. doi: 10.1038/nclimate1539
Talmage, S. C., and Gobler, C. J. (2010). Effects of past, present, and future ocean carbon dioxide concentrations on the growth and survival of larval shellfish. Proc. Natl. Acad. Sci. U.S.A. 107, 17246–17251. doi: 10.1073/pnas.0913804107
Wallace, R. B., Baumann, H., Grear, J. S., Aller, R. C., and Gobler, C. J. (2014). Coastal ocean acidification: the other eutrophication problem. Estuar. Coast. Shelf Sci. 148, 1–13. doi: 10.1016/j.ecss.2014.05.027
Keywords: climate change, ocean acidification, Menidia beryllina, Cyprinodon variegatus, multiple stressors
Citation: Gobler CJ, Merlo LR, Morrell BK and Griffith AW (2018) Temperature, Acidification, and Food Supply Interact to Negatively Affect the Growth and Survival of the Forage Fish, Menidia beryllina (Inland Silverside), and Cyprinodon variegatus (Sheepshead Minnow). Front. Mar. Sci. 5:86. doi: 10.3389/fmars.2018.00086
Received: 20 September 2017; Accepted: 01 March 2018;
Published: 16 March 2018.
Edited by:
Philip Boyd, University of Tasmania, AustraliaReviewed by:
Jorge M. Navarro, Universidad Austral de Chile, ChilePhilip Munday, Arc Centre of Excellence For Coral Reef Studies, Australia
Copyright © 2018 Gobler, Merlo, Morrell and Griffith. This is an open-access article distributed under the terms of the Creative Commons Attribution License (CC BY). The use, distribution or reproduction in other forums is permitted, provided the original author(s) and the copyright owner are credited and that the original publication in this journal is cited, in accordance with accepted academic practice. No use, distribution or reproduction is permitted which does not comply with these terms.
*Correspondence: Christopher J. Gobler, Y2hyaXN0b3BoZXIuZ29ibGVyQHN0b255YnJvb2suZWR1