- 1Department of Chemistry and Biochemistry, San Diego State University, San Diego, CA, United States
- 2Department of Biological Oceanography, Centro de Investigación Científica y de Educación Superior de Ensenada, Baja California, Mexico
Phytoplankton blooms can cause acute effects on marine ecosystems either due to their production of endogenous toxins or due to their enormous biomass leading to major impacts on local economies and public health. Despite years of effort, the causes of harmful algal blooms (HAB) are still not fully understood. Our hypothesis is that bacteria that produce photoactive siderophores may provide a bioavailable form of iron to commensally associated phytoplankton, which could in turn affect algal growth and bloom dynamics. Here we report a laboratory-based study of binary cultures of the dinoflagellate Lingulodinium polyedrum, a major HAB species, with Marinobacter algicola DG893, a phytoplankton-associated bacterium that produces the photoactive siderophore vibrioferrin. Comparing binary cultures of L. polyedrum with both the wild type and the vibrioferrin minus mutant of M. algicola shows that bacteria are necessary to promote dinoflagellate growth and that this growth promotion effect is at least partially related to the ability of the bacterium to supply bioavailable iron via the siderophore vibrioferrin. These results support the notion of a carbon for iron mutualism in some bacterial-algal interactions.
Introduction
Phytoplankton blooms are a frequent phenomenon in the coastal regions of every continent in the world. Certain phytoplankton algae occurring in mass proliferations produce toxins. Such harmful algal blooms (HABs) can, directly or indirectly, impact marine ecosystems with repercussions on local economies and public health (Lewitus et al., 2012). Direct effects on human health are often related to the consumption of shellfish that have ingested toxic phytoplankton, resulting in the accumulation of toxins and, consequently upon human consumption, paralytic, diarrheic, and neurotoxic poisoning which can sometimes be fatal (Anderson, 1994; Honner et al., 2012). HABs also have indirect impacts such as impairment of water quality leading to losses in the tourism and recreational sectors. Even phytoplankton blooms that do not produce toxins can be detrimental and lead to ecological impacts such as the displacement of indigenous species, habitat alteration, or oxygen depletion (Glibert et al., 2005). The economic effects caused by HABs in the U.S. alone were estimated at $82 million per year in 2006 (Hoagland and Scatasta, 2006). Current strategies to reduce health-related impacts due to HABs are based on frequent coastal monitoring and early detection of HAB species and toxin levels. Nevertheless, the factors leading to the origins of HABs are still not well understood. Consequently, there is no strategy for their ultimate prevention.
There is an ongoing debate whether the factors leading to HABs are natural or anthropogenic. Oceanographic factors and global climate change including changes in upwelling and or relaxation and reversal of winds may be the major drivers initiating phytoplankton blooms (Roegner et al., 2002; Tweddle et al., 2010). HABs have also been proposed to be the consequence of human activities including eutrophication, changes in land use and agriculture, overfishing, and ballast water discharge (Glibert et al., 2005). Others consider that better analytics result in increased detection of HAB species (Burkholder, 1998). Nevertheless, HABs are a global threat to human health, fisheries, and aquaculture resources. While numerous studies concentrate on the causes of HABs, few have considered that bacterial species coexisting with microalgae could contribute to their development. However since Bell and Mitchell reported that specific bacterial communities occur and microbial activity was altered in the so-called phycosphere (Bell and Mitchell, 1972; Bell and Lang, 1974), more and more studies suggest that such algal-bacterial interactions are very specific and important (Azam and Malfatti, 2007; Amin et al., 2015; Bertrand et al., 2015; Ramanan et al., 2016; Seymour et al., 2017). A plausible hypothesis suggests that the mutualistic association of some phytoplankton and bacteria is based on nutrient exchange. Nitrogen and phosphorus are the best studied in this regard, although the molecular nature of interactions involving these nutrients is not well understood (Hallegraeff and Gollasch, 2006). Alternatively specific bacteria may affect algal growth and bloom dynamics by their control of iron, a trace element which is often growth limiting to phytoplankton in the marine environment (Maldonado et al., 2005; Miethke and Marahiel, 2007; Croot and Heller, 2012).
Iron is an essential element for all living organisms including phytoplankton and bacteria due to its involvement in photosynthesis and respiration. Despite iron being the fourth most abundant element on the Earth, its bioavailability in the marine environment is extremely low due to its poor solubility under the mildly alkaline aerobic conditions present in the ocean (Martin and Fitzwater, 1988; Wu and Luther, 1994). To overcome this low bioavailability, bacteria and fungi have evolved sophisticated systems to produce high-affinity iron-chelating compounds called siderophores to acquire, transport, and process this essential metal ion (Sandy and Butler, 2009). The major role of siderophores is to bind mineral phases of iron and to deliver the iron siderophore complex to specific outer membrane receptors on microbial cells. Several hundred siderophores have been isolated and extensively studied with respect to their synthesis, structures and transport mechanisms over the last three decades (Yamamoto et al., 1994; Challis, 2005; Sandy and Butler, 2009; Raymond et al., 2015). While much research has been done on terrestrial siderophores, the study of marine siderophores is less extensive and only a relatively few have been fully elucidated (Vraspir and Butler, 2009). Nevertheless one of the two major attributes that seem to distinguish marine siderophores from those of terrestrial origin is the tendency of the former to contain an α- and β-hydroxy acid group in their iron-binding domain (Barbeau et al., 2001, 2002; Küpper et al., 2006). The significance of the presence of these functional groups in siderophores is in their ability to make the iron-siderophore complex photoreactive. The chelated Fe(III) in such siderophores is reduced in the presence of sunlight via an internal redox process to release Fe(II), a more soluble form of iron (Amin et al., 2009b). It has been proposed that sunlight-driven reduction of the Fe(III) would transiently produce Fe(II) which might be utilized not only by the siderophore-producing bacteria themselves but also by non-siderophore producing bacteria and other organisms such as phytoplankton (Maldonado et al., 2005; Naito et al., 2008; Amin et al., 2009a, 2012a).
While iron acquisition by bacteria is well understood, that of phytoplankton remains less so. There are data to support the possibility of a variety of iron uptake mechanisms being operative simultaneously depending on the species involved. These include an iron-reductive route via a cell surface reductase, a direct xenosiderophore-mediated mechanism, and cell surface-enhanced processes (Sutak et al., 2012; McQuaid et al., 2018). However as of yet there are no well documented examples, with some exceptions among the cyanobacteria, where phytoplankton have been shown to produce their own siderophores for iron acquisition (Raven, 2013). Thus how phytoplankton effectively acquire this metal from the low concentration iron environment is still unclear.
Our hypothesis is that algal growth and bloom dynamics may be affected by the increased bioavailability of iron engendered by the presence of photoreactive siderophores produced by mutualistic bacteria. Previously, we studied a bloom of the dinoflagellate, Lingulodinium polyedrum, at the Scripps Pier (San Diego, CA, USA) in 2011. L. polyedrum is one of the HAB species known to produce Yessotoxins, a group of polyether toxins which can accumulate in shellfish and show high toxicity to mice via intraperitoneal injection (Tubaroa et al., 2004). As the first step to search for an association of phytoplankton with specific bacterial photoreactive siderophore producers (producing petrobactin, aerobactin, and vibrioferrin siderophores), we monitored both the population of L. polyedrum and the bacterial siderophore producers before, during, and after the 2011 bloom. The results showed that both L. polyedrum and the bacterial siderophore producers simultaneously increased and decreased during the bloom period (Yarimizu et al., 2014). At the L. polyedrum bloom maximum, the total number of bacterial producers of photoreactive siderophore reached their maximum accounting for roughly 9% of the total bacterial population suggesting that such high abundance of photoreactive siderophore producers could potentially provide bioavailable iron to the phytoplankton in this environment. Furthermore, when the PCR-derived amplicons were sequenced, a phylogenetic tree constructed from the sequencing results showed that the community of siderophore producers in pre-bloom was statistically significantly different than those found during and after the bloom by UniFrac analysis suggesting that this particular bacterial community could be involved in bloom initiation.
As a proof of concept, the present study was performed using laboratory culture data to correlate the association of the vibrioferrin siderophore producing bacterium, Marinobacter algicola DG893 and the dinoflagellate L. polyedrum with iron as a nutrient. It is hoped that finding an algal-bacterial mutualism based on iron availability may be useful for a more thorough understanding of the mechanisms of HAB formation.
Materials and Methods
Trace Iron Cleaning Procedures
The container cleaning technique was adopted from Bruland and Franks (1979). All plastic and glass containers were washed with pure water and soaked in 3 N hydrochloric acid for at least 2 weeks at ambient temperature. The acid-washed containers were rinsed with Milli-Q water and dried in a laminar-flow air bench. For those experiments requiring sterile conditions, acid-washed containers were placed in pouches and autoclaved at 121°C for 30 min followed by a 45 min dry cycle. Alternatively, purchased sterile containers Nunc™ Cell Culture Treated Flasks with Filter Caps were used.
Growth Media
Of eight sources of natural waters screened, Pacific Ocean oligotrophic water was initially selected for our use due to its extremely low dissolved iron concentration (pM). This was replaced later by Scripps Pier seawater because of its easier accessibility and comparable L. polyedrum growth patterns observed in the two sources of seawater. Seawater from Scripps Pier (32.153°N, 117.115°W, San Diego, CA) was collected (pH 8.2–8.4, 980 mOsm/Kg H2O, total dissolved iron 3–4 nM) and filtered immediately through 0.22 mm pore size membrane. The filtered seawater was mixed with approximately 0.005% metal free hydrochloric acid, autoclaved for 30 min, cooled at ambient temperature for a day, and stored in 5°C until use. The pH of the autoclaved seawater was ensured to be between 8.0 and 8.2 at ambient temperature. L1 nutrient was added to sterile seawater per manufacturer's instruction (L1 medium). For those cultures requiring controlled iron concentrations in media, L1 trace element solution without FeCl3 and Na2EDTA was prepared in house (Guillard and Hargraves, 1993) and added along with other L1 nutrients (nitrate, phosphate, silicate, vitamins) to sterile seawater (L1-Fe medium). Serial dilutions were made on L1 medium with L1-Fe medium to prepare growth medium with defined total iron concentrations, [Fe]T.
Artificial Sea Water (ASW)
The component solutions were prepared in three separate containers, one with 15 g NaCl, 0.75 g KCl, 1 g NH4Cl in approximately 500 mL water, one with 12.4 g MgSO4.7H2O in small volume of water, and one with 3.0 g of CaCl2.2H2O in a small volume of water. The three solutions were mixed and 0.1 g disodium ß-glycerol phosphate was added. The pH was adjusted to 8.0–8.2 and volume was adjusted to 1 L. The solution was autoclaved at 121°C for 30 min.
Algal Maintenance and Growth Monitoring
The L. polyedrum strain used in this study was isolated from Venice Beach, California, and kindly provided to us by Avery Tatters (University of Southern California). L. polyedrum cells were maintained in sterile 1L Erlenmeyer flasks containing L1 medium and capped with a ventilation sponge. Cultures were exposed to 100 μmol photons m−2 s−1 on a 12-h alternating light and dark cycle at a temperature of 20 ± 2°C (standard growth condition). The upper portion of a culture containing healthy cells was diluted 1/5 with L1 medium every 3 weeks. Algal growth was monitored by direct cell count using an inverted microscope (Nikon Eclipse TE2000-U, 4× objective). To prepare cells in controlled iron medium, cultures containing 104-105 cells per mL was placed in a 15-mL Falcon tubes and centrifuged for a few seconds to pellet cells which were gently washed three times and re-suspended to an appropriate volume with controlled iron medium.
“Axenic” L. polyedrum Preparation
Non-axenic L. polyedrum cells were grown to 105 cells/mL in L1 medium. Antibiotics 0.1% (v/v) (Penicillin 5 units/mL, Streptomycin 5 μg/mL, Neomycin 10 μg/mL) were added to a non-axenic culture and incubated for 24 h under the standard growth condition. Antibiotic-treated cultures were placed in 15 mL Falcon tubes and gently centrifuged for a few seconds. The pelleted cells were washed three times and re-suspended with L1 medium containing 0.05% (v/v) antibiotics in a sterile culture flask to appropriate volume. Antibiotics 0.05% (v/v) was added every 3 days to maintain the culture “axenic.” The absence of cultureable bacteria in the antibiotic treated L. polyedrum was tested by spreading a 10 μL sample of the culture on a marine broth plate (5 g/L peptone, 1 g/L yeast extract, 15 g/L agar in 75% seawater) followed by incubation at 25°C for 2–3 days. The absence of any visible bacterial colonies was considered indicative of an “axenic” culture of L. polyedrum.
Bacteria Strains and Growth Monitoring
The model bacterium used was Marinobacter algicola DG893 (GenBank code NZ_ABCP00000000.1) since it has been isolated by Green et al. (2004) and well characterized as a producer of the photoactive siderophore vibrioferrin (Amin et al., 2007). The mutant ΔpvsAB-DG893 was made as described by Amin et al. (2012a) by 872 base pair deletion from the wild type to knock out two siderophore biosynthesis genes, pvsA and pvsB. To monitor bacterial growth, two methods were used: Optical density at 600 nm was applied to apparently turbid samples which generally contained ≥107/mL of bacterial cells. Enumeration of bacteria by serial dilution was applied for samples containing lower numbers of bacteria cells (<107/mL). For enumeration techniques, serial dilution was made on those samples with sterile seawater, each diluted sample was spread on marine broth plates, and the number of colony forming units (CFU) recorded from the lowest diluted sample plate. CFU in original sample per mL was calculated by (CFU in diluted sample)/(volume plated in mL) × dilution factor.
Stock Bacteria Solution
One liter of marine broth was prepared (5 g/L peptone, 1 g/L yeast extract, 15 g/L agar in 75% seawater, pH 8.2, sterile) and dispensed into sterile tubes with a 5 mL volume. A few colonies of bacteria picked from stock plates were transferred into the marine broth tubes and shaken at 25°C for 1–2 days until they reached log phase. The cells were collected by centrifugation, washed three times and re-suspended in sterile medium to an optical density (OD600) of 0.3 (stock bacteria solution).
CAS-Dye Assay
The method was adopted from Alexander and Zuberer (1991) to detect siderophore production by DG893. Using acid washed glassware, the following stock solutions were prepared: 10 mM HDTMA in water, 2 mM CAS in water, 1 mM FeCl3.6H2O in water, 50 mM piperazine anhydrous buffer (pH 5.6), and 0.2 M 5-sulfosalicylic acid in water. CAS solution was prepared by pouring a mixture of 15 mL of CAS and 3 mL of FeCl3.6H2O slowly into 12 mL of HDTMA followed by addition of 50 mL of piperazine. The final volume was adjusted to 200 mL with water. CAS shuttle solution was prepared by adding 20 μL of 5-sulfosalicylic acid to every mL of CAS solution (used within 1 day of preparation). Samples to be tested were centrifuged to remove particles and 0.5 mL supernatant was mixed with 0.5 mL of CAS shuttle solution followed by incubation in the dark at ambient temperature for 10 min. A color change from dark purple to clear red indicated siderophore production.
Materials and Reagents
The following materials were purchased from Sigma-Aldrich: Chrome azurol S (CAS, C-1018, MW605.28), antibiotics (Penicillin/Streptomycin/Neomycin, P4083-100 mL), peptone (P-1265), iron(III) chloride hexahydrate (FeCl3.6H2O, 10025-77-1, MW270.30), manganese(II) chloride tetrahydrate (MnCl2.4H2O, 13446-34-9, MW197.91), hexadecyltrimethylammonium bromide (HDTMA, H6269-100G, MW364.45), 1,4-diazacyclohexane, diethylenediamine (Piperazine, Sigma-Aldrich, P45907-100G, MW 86.14), 5-sulfosalicylic acid (390275, MW218. 18), zinc sulfate heptahydrate (ZnSO4.7H2O, 1088830500, MW287.54), cobalt(II) chloride hexahydrate (CoCl2.6H2O, 8025400010, MW129.83), copper(II) sulfate pentahydrate (CuSO4.5H2O, 209198-5G, MW249.69), sodium molybdate dehydrate (Na2MoO4.2H2O, 331058-5G, MW241.95), selenous acid (H2SeO3, 211176-10G, MW128.97), nickel(II) sulfate hexahydrate (NiSO4.6H2O, 227676-100G, MW262.85), sodium orthovanadate (Na3VO4, 450243-10G, MW183.91), potassium chromate (K2CrO4, 216615-100G, MW194.19), salicylaldoxime (SA, 84172-100G). The following materials were purchased from Fisher Scientific: trace metal-free hydrochloric acid (Optima, A466-250), yeast extract (BP9727-500), agar (BP1423-500), Casamino Acids (BP1424-500), NuncTM Cell Culture Treated Flasks with Filter Caps (12-565-57), ethylenediaminetetraaceticacid, tetrasodium salt dihydrate (Na4EDTA.2H2O, BP121-500, MW416.2), boric acid (AAJ67202A1). Other materials were purchased as follows: 0.22 μm filter membrane (Millipore, MillexGV, SLGV033RS), L1 medium kit (Bigelow, MKL150L), pure water (Barnstead water system, 18.2 mΩ). Vibrioferrin (VF, MW434.35) was isolated and purified in house according to Amin et al. (2007).
Results
Bacterial Growth
The growth of DG893 and mutant ΔpvsAB-DG893 in L1 medium containing different total iron concentrations [Fe]T (1,000, 100, 10, and 0 nM) was monitored. All media were divided in two parts, one maintaining [EDTA] at 10,000 nM (L1 nutrient level) with variable [Fe]T, and the other reducing [EDTA] along with [Fe]T to maintain molar ratio of Fe: EDTA of 1:1. All media were prepared with and without sterile Casamino Acids. In each medium, ca. 106 cells/mL of DG893 or mutant ΔpvsAB-DG893 were added and shaken in the dark at 30°C. The bacterial growth in each medium was monitored for 5 days by optical density at 600 nm. Neither WT DG893 nor the mutant grew in simple seawater or L1-Fe medium without a carbon source (Figure 1A). Addition of iron to seawater or L1-Fe medium also did not maintain growth (Figure 1B). However upon addition of Casamino Acids, both DG893 and the mutant grew and their growth increased with increasing [Fe]T in media (Figure 1C). There was no remarkable difference in growth pattern between DG893 and the mutant, consistent with the report by Amin et al. (2012a). In summary, both DG893 and the mutant require a carbon source to grow to a detectable level in seawater medium.
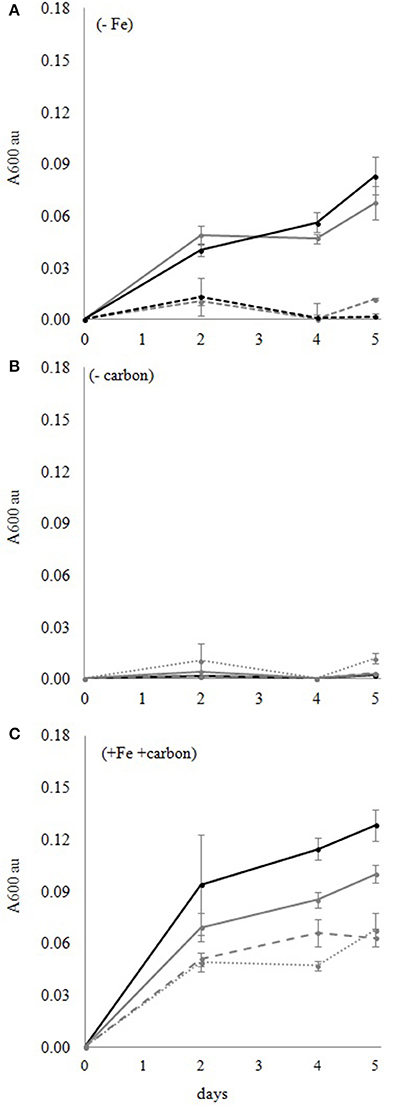
Figure 1. DG893 growth in media with and without Fe and carbon. (A) DG893 growth in media without added Fe. The bold lines represent media containing 10 mg/mL Casamino Acids. The dotted lines represent media without Casamino Acids. The gray lines represent L1-Fe media. The black lines represent seawater. The VF null mutant of DG893 showed a similar pattern. (B) DG893 incubated in L1 media containing different [Fe]T but without an added carbon source. The black bold line, gray bold line, gray wide dotted line, and gray narrow dotted line represent L1 media with 1,000, 100, 10, 0 nM [Fe]T, respectively. The mutant DG893 showed a similar pattern. (C) DG893 incubated in L1 media containing 10 mg/mL Casamino Acids and different [Fe]T. The black bold line, gray bold line, gray wide dotted line, and gray narrow dotted line represent L1 media with 1,000, 100, 10, 0 nM [Fe]T, respectively.
Siderophore Production and Total Iron Concentration [Fe]T
The ability to produce the siderophore vibrioferrin (VF) by DG893 but not by the mutant was confirmed by the CAS-dye assay. Since siderophore biosynthesis is generally turned on under low iron concentrations and turned off under high concentrations (Braun, 1995; Braun et al., 1998; Miethke and Marahiel, 2007), WT was grown in L1 medium containing various [Fe]T with 0.3% Casamino Acids at 30°C in dark for 24 h to find the approximate [Fe]T under which DG893 produces VF. The WT did not produce VF in the media with [Fe]T ≥10 μM but did so with a [Fe]T of 1 μM or less. The mutant ΔpvsAB-DG893 did not show signs of siderophore production under any of the conditions tested. The results suggest that under normal seawater conditions, where [Fe]T is estimated to be nM to pM, that siderophore biosynthesis in DG893 is likely turned on.
Axenic Culture Preparation
The difficulty in preparing and maintaining axenic cultures of many marine algae has been reported in the past (Green et al., 2004; Jauzein et al., 2015; Liu et al., 2017). In fact, Lupette et al. (2016) noted that completely axenic cultures of a model green algal species could not be maintained despite the use of antibiotic treatment protocols. We were partially successful in our efforts to prepare axenic L. polyedrum. Initially, non-axenic L. polyedrum cultures containing 105 cells/mL were treated with antibiotics (1% v/v Penicillin 50 units/mL, Streptomycin 50 μg/mL, Neomycin 100 μg/mL) for 24 h under the normal growth conditions. The treated cells were washed and re-suspended with sterile L1 media and while the “axenicity” of the culture at this point was confirmed, bacterial colonies reappeared after only a few days. An attempt was made to keep 1% antibiotics in the culture longer than 24 h, however, dinoflagellate cells began to disintegrate after 2 days suggesting that 1% antibiotics must be removed from the culture within 24 h. The fact that the negative control and sterile L1 medium without phytoplankton cells remained bacteria-free for weeks ruled out a possibility of culture contamination. We believe that the antibiotics are most likely eliminating only free-living bacteria leaving attached bacteria unaffected. The next effort of preparing axenic cultures was made by immediate dilution of antibiotic-treated cultures. The rationale of this attempt was to minimize attached bacteria on L. polyedrum cells by dilution. The antibiotic treated L. polyedrum culture was diluted 200-fold with sterile L1 medium and incubated under standard growth conditions. The diluted culture remained bacteria-free for the first 10 days, however microbial growth was observed on the 12th day as the number of L. polyedrum cells also increased. Although this dilution method suggested that the culture stayed bacteria free for up to 10 days, the L. polyedrum cell count was too dilute to be useful for further study. The next attempt at axenic culture preparation was made with three sequential antibiotic treatments. The non-axenic culture was treated with 1% antibiotics for 24 h, washed three times, and re-suspended with sterile L1 medium. The cells were allowed to recover under normal growth conditions for a day and then again treated with 1% antibiotics. This procedure was repeated three times. This procedure also failed as the dinoflagellate cells began to disintegrate. Finally, using a similar procedure but reducing the concentration of antibiotics to 0.1% for the first treatment and adding 0.05% subsequently every 2–3 days resulted in L. polyedrum cultures without visible cell damage that appeared to be free of free-living bacteria for more than a month.
L. polyedrum Growth Screening
A matrix containing thirty cultures of L. polyedrum in L1 medium containing different numbers of DG893 cells in the initial inoculum (107, 106, 105, 104, 103, 0 CFU/mL) and different [Fe]T (104, 103, 102, 101, and 0 nM) was set up. The same matrix was set up with the mutant ΔpvsAB-DG893. Bacterial growth was monitored by enumeration of bacteria by serial dilution and phytoplankton growth was monitored by direct cell counts under the microscope. Regardless of the starting bacterial cell number, both DG893 wild type and mutant grew in all the binary culture matrices over 28 days to eventually reach a bacterial population of ca. 105 CFU/mL. The group of media containing 1,000 nM [Fe]T showed the greatest growth of L. polyedrum regardless of the starting bacterial inoculum (Figure 2A). The group of media containing ≤10 nM [Fe]T showed very slow L. polyedrum growth regardless of starting bacterial inoculum. No significant differences in L. polyedrum growth co-cultured with DG893 or the mutant were observed, which left a question as to whether VF secreted by DG893 would have a significant effect on L. polyedrum growth. It was speculated that under these growth conditions, the amount of VF being produced by DG893 was insufficient to see detectable changes in L. polyedrum growth. This was later confirmed (vide infra) when excess VF artificially added to the culture produced pronounced L. polyedrum growth. Finally, the growth pattern of axenic L. polyedrum was similar to that of a non-axenic culture. This observation left open another question as to whether DG893 and L. polyedrum were commensally associated. However this also was confirmed by experiments described below where after subsequent culturing the “axenic” L. polyedrum ceased to grow while binary L. polyedrum DG893 kept growing exponentially (Figure 3).
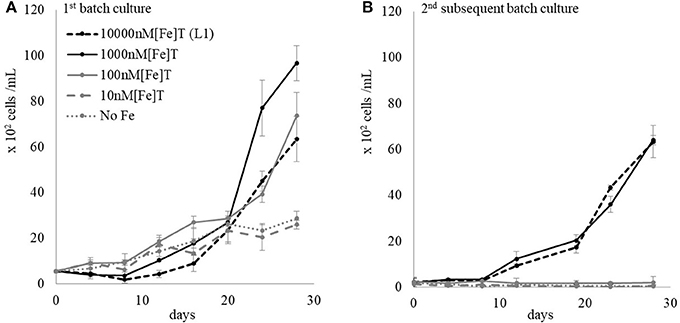
Figure 2. L. polydrum co-cultured with a starting inoculum of DG893 at 103 cells/mL in media with various [Fe]T. (A) First generation growth of L. polyedrum in the presence of DG893. The starting inoculum of DG893 was 103 cells/mL which reached 105 cells/mL after 28 days incubation under the standard growth conditions. A similar pattern was observed in all other groups: i.e., media containing a starting inoculum of DG893 of 107, 106, 105, 104, 103, 0 cells/mL and [Fe]T of 104, 103, 102, 101, or 0 nM. (B) Second generation growth of L. polyedrum in the presence of DG893. After 28 days the cultures in (A) were diluted to a L. polyedrum concentration of 102 cells/mL with appropriate media and incubated under the standard growth condition for an additional 28 days.
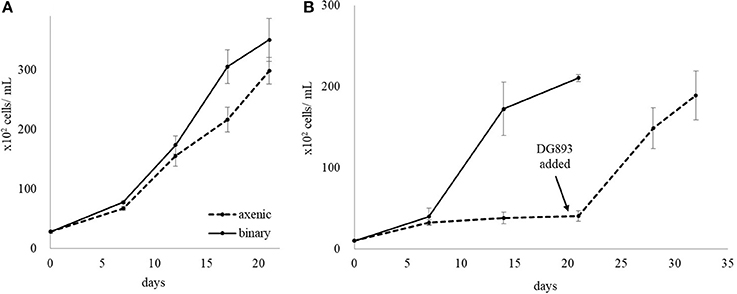
Figure 3. Rescue of L. polyedrum by addition of DG893. (A) First generation of L. polyedrum growth maintained in L1 media either “axenic” or co-cultured with DG893. (B) Growth of L. polyedrum in the second subculture either “axenic” or co-cultured with DG893. After the second subculture of “axenic” L. polyedrum was incubated under the standard growth conditions for 21 days, an inoculum containing approximately 102 cells/mL of DG893 was added and incubation continued for an additional 14 days.
Subsequent Batch Culture Growth of L. polyedrum in DG893 Binary Culture
L. polyedrum grown in L1 media containing various [Fe]T and DG893 (Figure 2A) for 28 days were diluted with appropriate media to adjust L. polyedrum to 102 cells/mL in the 2nd subsequent batch culture. The L. polyedrum growth in this 2nd subsequent culture was monitored for another 28 days under each growth condition. The result showed that the 2nd subsequent batch culture L. polyedrum in the higher two [Fe]T (10,000 and 1,000 nM) maintained their exponential growth while those in the lower three [Fe]T did not grow (Figure 2B). Although the cells under the latter conditions did not completely die out, the few cells that survived had their swimming activity under the microscope clearly reduced.
Axenic L. polyedrum Culture and Rescue
The growth of “axenic” and binary cultures of L. polyedrum with DG893 in L1 was compared over several subsequent batch cultures. No significant difference in growth was observed in the 1st culture, however, a clear difference was observed in the 2nd subsequent batch culture of L. polyedrum with and without DG893. While L. polyedrum with DG893 grew fully in both the 1st and 2nd subsequent batch cultures, “axenic” L. polyedrum ceased to grow (Figure 3). When DG893 was added to the 2nd subsequent “axenic” batch culture, L. polyedrum cells began to regrow exponentially demonstrating that DG893 could rescue “axenic” L. polyedrum.
Non-axenic L. polyedrum Growth and Rescue
The non-axenic L. polyedrum was maintained in L1 medium and assumed to contain not only siderophore-producing bacteria but also other unknown bacteria. The non-axenic culture was divided in two parts, one diluted to 1/5 with L1 medium and other diluted to 1/5 with L1-Fe medium (batch subculture 1). When the cell count reached approximately 104 cells/mL, both cultures were further diluted with appropriate medium. Therefore, one culture maintained [Fe]T at L1 level throughout the subsequent batch cultures while the other contained successively reduced [Fe]T. The L. polyedrum growth was monitored for 28 days for each subculture. After the 6th subculture where [Fe]T in the medium was estimated to be 2 nM, L. polyedrum stopped growing completely (Figure 4). On 29th day of the 6th subculture, an iron supplement to give a [Fe]T of 5,850 nM was added to the culture and growth was continued to be monitored for additional 28 days. The results indicate that the L. polyedrum culture which stopped growing under 2 nM [Fe]T, could be rescued by addition of iron after 28 days (Figure 5).
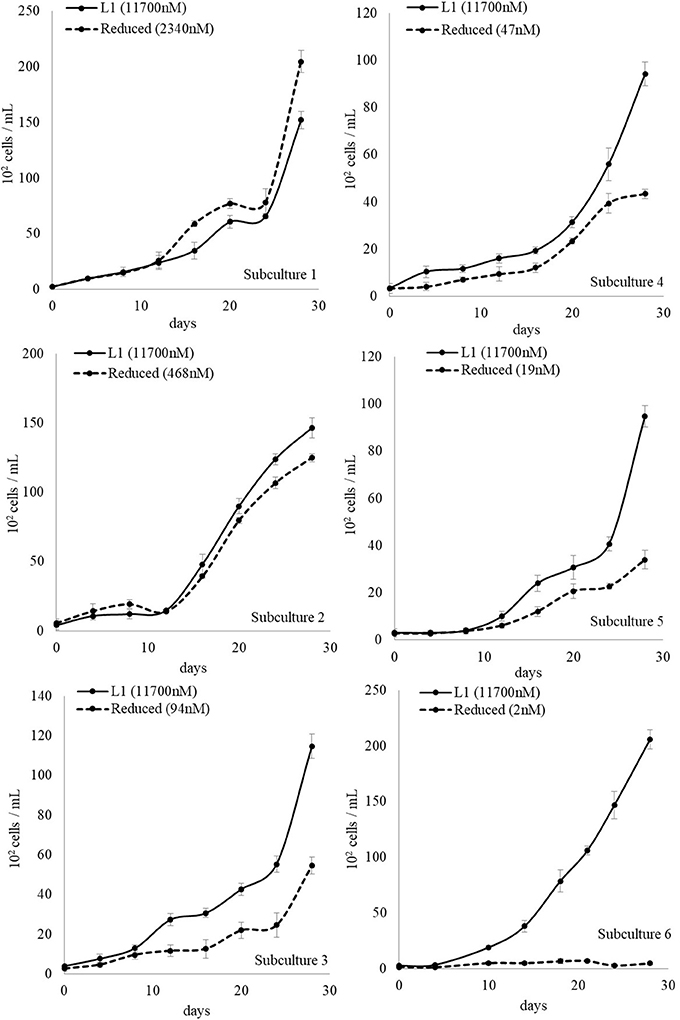
Figure 4. Non-Axenic L. polyedrum growth in L1 media and reduced [Fe]T over 6 subculturings. Solid line: cultures maintained with a [Fe]T at the L1 level throughout the subculturing. Dotted line: cultures where the [Fe]T continually decreased over the subcultures due to dilution.
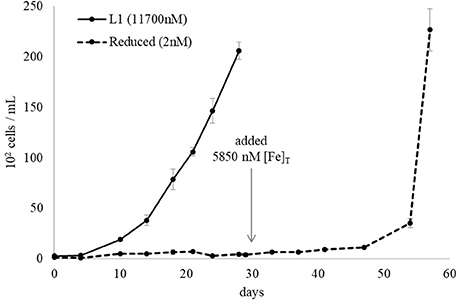
Figure 5. Iron starved non-axenic L. polyedrum growth rescued by iron addition. The iron sufficient culture (solid black line) contained 11,700 nM [Fe]T while the iron deficient culture contained 2 nM (dotted black line). After 28 days 5,850 nM [Fe]T was added to the iron deficient culture which rescued L. polyedrum growth.
L. polyedrum Growth in DG893 Supernatant
Earlier it was stated that when L. polyedrum was co-cultured with either DG893 or the mutant ΔpvsAB-DG893, little difference was observed in its growth pattern. We speculated that this may have been due to the small amount of VF produced by the equilibrium population of DG893 in the binary culture. To test this idea, samples of the supernatant of the media from the WT DG893 grown under ideal conditions and containing detectable amounts of VF by CAS-dye assay, as well as the mutant ΔpvsAB-DG893 which did not, respectively, were added to L. polyedrum cultures and growth under these different conditions was compared. It should be noted that while we traditionally grow DG893 and the mutant in marine broth containing Casamino Acids, these were found to be toxic to L. polyedrum cells. In this experiment, we instead used ASW with succinic acid (at from 0.001 to 0.1%) as a carbon source (pH 8.2) to grow DG893 and the mutants prior to their supernatant being added to L. polyedrum cultures. L. polyedrum growth was enhanced the most by the addition of the DG893 supernatant. The mutant supernatant also showed a slightly positive effect on L. polyedrum growth compared to media without bacterial supernatant, however the degree of growth was not as large as that induced by the DG893 supernatant (Figure 6). These results suggest that VF has potential influence on L. polyedrum growth, but that other extracts from bacteria (vitamin B12?) may also have a potential effect on their growth. Further experiments were performed using purified VF itself. When purified VF at 57 μM was directly added to a L. polyedrum culture in L1 media containing 100 nM [Fe]T, L. polyedrum reached cell counts approximately 1.5 times as large as those in media without VF.
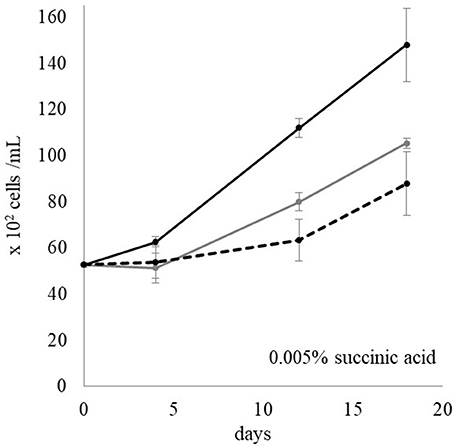
Figure 6. L. polyedrum growth in media with DG893 supernatant, mutant supernatant, and without bacterial supernatant. The black lines, gray lines, and dotted lines represent L. polyedrum growth in media with added DG893 supernatant, with added VF null mutant supernatant, and without any bacterial supernatant, respectively.
DG893 Growth in L. polyedrum Supernatant
“Axenic” L. polyedrum cells were resuspended in L1 media containing 100, 1,000, 10,000 nM [Fe]T and incubated under the standard growth conditions for 2 days to collect their organic extracts. The L. polyedrum cultures were divided in two parts, one of which was filtered through a 0.22 μm membrane to collect only organics and the other was kept without filtration to keep phytoplankton cell debris. The L. polyedrum supernatant with and without cells was added to the stock DG893 containing 0.3% Casamino Acids, and the mixture shaken at 30°C for several days. The control was prepared in the same manner without L. polyedrum supernatant and cells. In the media containing 1,000 and 10,000 nM [Fe]T, DG893 growth was enhanced by the presence of L. polyedrum supernatant and was even more pronounced with the supernatant containing cell debris (Figure 7). In media with 100 nM [Fe]T, only a subtle difference was observed in DG893 growth with and without L. polyedrum supernatant.
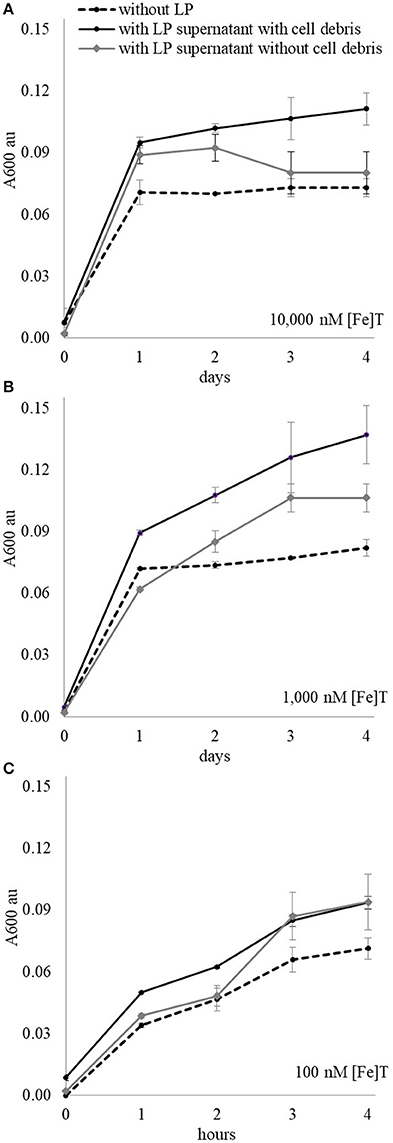
Figure 7. DG893 growth in media containing different [Fe]T with and without presence of L. polyedrum supernatant and cell debris. The solid black lines represent growth of DG893 in the presence of L. polyedrum supernatant with their cell debris, the gray lines are DG893 growth in L. polyedrum supernatant without their cell debris, and the dotted lines are DG893 without any L. polyedrum supernantant. DG893 was grown in media containing (A) 10,000 nM [Fe]T, (B) 1,000 nM [Fe]T, and (C) 100 nM [Fe]T.
Discussion
Before proceeding to a discussion of the results presented here it is important to operationally define what we mean by [Fe]T and “axenic” cultures. Here we used the term total iron concentrations [Fe]T to mean the total analytical amount of iron added to culture and includes all phases of iron present in the solution. This is different from the total dissolved iron concentration which we routinely measure for environmental seawater samples (and which is approximately 2–6 nM for Scripps Pier water for example) as determined by cathodic stripping voltammetry (CSV). Total dissolved iron in such environmental samples are processed by filtration through a 0.22 μm membrane followed by acidification. As a result, total dissolved iron in a sample is much lower than the total analytical iron concentration. We use this operational definition as it is not possible to measure total dissolved iron in culture media using the CSV method because equilibrium between the various iron oxo/hydroxo species potentially present in seawater at pH 8.2 is reached only extremely slowly and thus concentrations of total dissolved iron are constantly changing over the time course utilized in these experiments. Using a straight forward expression of total analytical iron concentration is thus a potentially more reproducible approach with which to compare the effects of iron on bacterial and algal growth in a laboratory setting.
Secondly we use the term “axenic” in quotation marks for describing cultures of L. polyedrum, to mean the absence of any observable cultureable bacteria based on the absence of visual bacterial colonies when cultures were placed on solid media and incubated for several days. We were only partially successful making the culture “axenic” by treating the culture with low concentration of antibiotics and sequential addition of the antibiotics to the culture over extended times. Using this method the culture appeared to remain bacteria free for at least a month. However when the sequential addition of antibiotics to the culture was stopped, bacteria growth was observed to return within a few days. Thus clearly the cultures were not truly axenic. Nevertheless our main concern was that the dominant species of bacteria growing in our binary culture should be DG893 and that “axenic” cultures should contain insignificant numbers of unknown bacteria. We confirmed the former by qPCR and the latter was further supported by presence of few if any bacteria seen via DAPI staining. To our knowledge, there is at present no general protocol for preparing a completely axenic culture of L. polyedrum hence our use of the operational definition of “axenic” described here.
We initially investigated conditions under which the bacterium Marinobacter DG893 and the dinoflagellate L. polyedrum could grow independently. As expected, in the absence of an added carbon source DG893 could not grow in natural unamended seawater or in L1 supplemented ASW irrespective of the iron concentration. In the presence of an adequate artificial source of carbon however bacterial growth was controlled by the available concentration of iron. Under idealized conditions, both DG893 and the VF null mutant reached a final concentration of around 105 CFU/mL independent of the initial inoculum. However even without any externally added carbon source DG893 grew well in the presence of L. polyedrum indicating that the dinoflagellate leaked enough dissolved organic carbon (DOC) to fully support the growth of the bacterium. The notion that DG893 could utilize L. polyedrum as a carbon source was further supported by the observation that growth of DG893 was stimulated when it was incubated in the media containing the supernatant from a filtered L. polyedrum culture which contains non-specific organics secreted from L. polyedrum. These may possibly include dimethylsulfoniopropionate (DMSP) a labile carbon and sulfur source known to “leaked” from dinoflagellates (Caruana and Malin, 2014).
In the case of both “axenic” and nonaxenic cultures of the photosynthetic dinoflagellate L. polyedrum, iron proved to be a growth limiting nutrient. Thus maximum growth occurred at total iron concentrations of 100–1,000 nM while higher concentrations proved toxic and lower concentrations (0–10 nM) were growth limiting. In the first culture, both “axenic” and non-axenic cultures grew equally well. However, their growth showed a remarkable difference in the second batch subculture. Here the non-axenic L. polyedrum culture continued to grow exponentially although now only at the higher [Fe]T. When the iron concentrations were kept high, L. polyedrum cultures continued to thrive up to at least 6 subcultures. However when the iron concentrations were allowed to be reduced by dilution in subsequent subculturing, the growth gradually ceased. Poorly growing subcultures in low iron media could however be rescued by the addition of additional iron to the culture. Remarkably “axenic” L. polyedrum subcultures ceased to grow regardless of [Fe]T but could be rescued by the addition of DG893. These results emphasize the importance of both bacteria and iron to L. polyedrum growth.
Our results further suggest that the L. polyedrum growth promoting effects of the bacterium Marinobacter DG893 were in fact not unspecific but at least partially related to its production of the photoactive siderophore vibrioferrin. Thus only the supernatant of DG893 that contained sufficient amounts of vibrioferrin facilitated L. polyedrum growth. While the addition of the supernatant from a culture of the VF null mutant of DG893 also improved growth of L. polyedrum the effect was much less than that from the WT. This observation was further confirmed by direct addition of purified VF into the L. polyedrum culture which growth increased 1.5 times more than those without VF. These results imply that the presence of the siderophore vibrioferrin clearly influences L. polyedrum growth, but that other factors such as vitamin B12 (Cruz-Lopez and Maske, 2015) provided by bacteria may also be important in promoting this apparent bacterial-algal mutualism.
Here we have focused on a bacteria that produces the photoreactive siderophore, vibrioferrin. Vibrioferrin was chosen for more detailed study for a number of reasons. First vibrioferrin has been isolated from several different bacteria which are known to be closely associated with HAB species (Amin et al., 2007) and we found that bacteria that had the ability to synthesize vibrioferrin were by far the most numerous as compared to the producers of other photoreactive siderophores which we followed during the bloom of L. polyedrum at Scripps Pier in 2011 (Yarimizu et al., 2014). We initially supposed that vibrioferrin was a good candidate for a siderophore that could provide bioavailable iron for both the bacterial producers and their algal partners as it is the most rapidly photolyzed of the photoactive siderophores tested and that photolyzed vibrioferrin has no further affinity for iron. This feature is unusual in that most other photoactive siderophores retain the ability to strongly bind Fe(III) even after photolysis (Amin et al., 2009b). However we have recently shown that while vibrioferrin was the best source of iron for L. polyedrum of the ones we tested (Yarimizu et al., 2017), it was not due to its photolysis since iron uptake from VF was the same in the dark as in the light. We therefore attribute its ability to provide bioavailable iron to the dinoflagellate to its relatively weak iron binding properties (with respect to other more traditional siderophores) as it lacks the sixth donor group required to complete the octahedral coordination geometry preferred by Fe(III) (Amin et al., 2009b). This in turn leads to a less negative reduction potential (that falls within the biological range) so that the iron can readily be released from it by the cell surface reductases known to be present in L. polyedrum.
As a final note, while this laboratory study strongly supports a carbon for iron mutualism between the dinoflagellate L. polyedrum and Marinobacter DG893 as previously proposed (Amin et al., 2009a), it is unclear at present how, or indeed if, such a mutualism will operate in the field. To this end we have collected data from several field studies involving both HAB and non-HAB bloom events and are currently searching for relationships between phytoplankton, vibrioferrin producing bacteria, and available iron. We hope that both these laboratory and field studies will complement each other and advance our knowledge of the potential role of bacterial-algal interactions in understanding the mechanisms of HAB formation.
Author Contributions
KY, RC-L, and CC designing the study, executing the experiments, analyzing the data, interpreting the data, reviewing the data for accuracy and integrity, drafting the work, and revising this report.
Conflict of Interest Statement
The authors declare that the research was conducted in the absence of any commercial or financial relationships that could be construed as a potential conflict of interest.
Acknowledgments
We thank Avery Tatters (University of Southern California) for providing the L. polyedrum strain and helping us to set up an algal culture laboratory. We thank Professor Michael Latz (Scripps Institute of Oceanography) for providing us L. polyedrum. We also thank Professor Katharine Barbeau (Scripps Institute of Oceanography) for providing us Pacific Ocean oligotrophic water and advice on iron measurement techniques as well as Professor Frithjof C. Küpper (University of Aberdeen) for a critical reading of the manuscript. A National Science Foundation grant to CJC (CHE-1664657) is gratefully acknowledged for partial support of this work.
References
Alexander, D. B., and Zuberer, D. A. (1991). Use of chrome azurol S reagents to evaluate siderophore production by rhizosphere bacteria. Biol. Fertil. Soils 12, 39–45. doi: 10.1007/BF00369386
Amin, S. A., Green, D. H., Al Waheeb, D., Gärdes, A., and Carrano, C. J. (2012a). Iron transport in the genus Marinobacter. Biometals 25, 135–147. doi: 10.1007/s10534-011-9491-9
Amin, S. A., Green, D. H., Gärdes, A., Romano, A., Trimble, L., and Carrano, C. J. (2012b). Siderophore-mediated iron uptake in two clades of Marinobacter spp. associated with phytoplankton: the role of light. Biometals 25, 181–192. doi: 10.1007/s10534-011-9495-5
Amin, S. A., Green, D. H., Hart, M. C., Küpper, F. C., Sunda, W. G., and Carrano, C. J. (2009a). Photolysis of iron–siderophore chelates promotes bacterial–algal mutualism. Proc. Natl. Acad. Sci. U.S.A. 106, 17071–17076. doi: 10.1073/pnas.0905512106
Amin, S. A., Green, D. H., Küpper, F. C., and Carrano, C. J. (2009b). Vibrioferrin, an unusual marine siderophore: iron binding, photochemistry, and biological implications. Inorg. Chem. 48, 11451–11458. doi: 10.1021/ic9016883
Amin, S. A., Hmelo, L. R., van Tol, H. M., Durham, B. P., Carlson, L. T., Heal, K. R., et al. (2015). Interaction and signalling between a cosmopolitan phytoplankton and associated bacteria. Nature 522, 98–101. doi: 10.1038/nature14488
Amin, S. A., Küpper, F. C., Green, D. H., Harris, W. R., and Carrano, C. J. (2007). Boron binding by a siderophore isolated from marine bacteria associated with the toxic dinoflagellate Gymnodinium catenatum. J. Am. Chem. Soc. 129, 478–479. doi: 10.1021/ja067369u
Azam, F., and Malfatti, F. (2007). Microbial structuring of marine ecosystems. Nat. Rev. Microbiol. 5, 782–791. doi: 10.1038/nrmicro1747
Barbeau, K., Rue, E. L., Bruland, K. W., and Butler, A. (2001). Photochemical cycling of iron in the surface ocean mediated by microbial iron(iii)-binding ligands. Nature 413, 409–413. doi: 10.1038/35096545
Barbeau, K., Zhang, G., Live, D. H., and Butler, A. (2002). Petrobactin, a photoreactive siderophore produced by the oil-degrading marine bacterium Marinobacter hydrocarbonoclasticus. J. Am. Chem. Soc. 124, 378–379. doi: 10.1021/ja0119088
Bell, W. H., and Lang, J. M. (1974). Selective stimulation of marine bacteria by algal extracellular products. Limnol. Oceanogr. 19, 833–839. doi: 10.4319/lo.1974.19.5.0833
Bell, W., and Mitchell, R. (1972). Chemotactic and growth responses of marine bacteria to algal extracellular products. Biol. Bull. 143, 265–277. doi: 10.2307/1540052
Bertrand, E. M., McCrow, J. P., Moustafa, A., Zheng, H., McQuaid, J. B., Delmont, T. O., et al. (2015). Phytoplankton-bacterial interactions mediate micronutrient colimitation at the coastal Antarctic sea ice edge. Proc. Natl. Acad. Sci. U.S.A. 112, 9938–9943. doi: 10.1073/pnas.1501615112
Braun, V. (1995). Energy-coupled transport and signal transduction through the gram-negative outer membrane via TonB-ExbB-ExbD-dependent receptor proteins. FEMS Microbiol. Rev. 16, 295–307. doi: 10.1111/j.1574-6976.1995.tb00177.x
Braun, V., Hantke, K., and Köster, W. (1998). Bacterial iron transport: mechanisms, genetics, and regulation. Met. Ions Biol. Syst. 35, 67–145.
Bruland, K. W., and Franks, R. P. (1979). Sampling and analytical methods for the determination of copper, cadmium, zinc, and nickel at the nanogram per liter level in sea water. Anal. Chim. Acta 105, 233–245. doi: 10.1016/S0003-2670(01)83754-5
Burkholder, J. M. (1998). Implications of harmful microalgae and heterotrophic dinoflagellates in management of sustainable marine fisheries. Ecol. Appl. 8, S37–S62. doi: 10.1890/1051-0761(1998)8[S37:IOHMAH]2.0.CO;2
Caruana, A. M. N., and Malin, G. (2014). The variability in DMSP content and DMSP lyase activity in marine dinoflagellates. Prog. Oceanogr. 120, 410–424. doi: 10.1016/j.pocean.2013.10.014
Challis, G. L. (2005). A widely distributed bacterial pathway for siderophore biosynthesis independent of nonribosomal peptide synthetases. Chembiochem 6, 601–611. doi: 10.1002/cbic.200400283
Croot, P. L., and Heller, M. I. (2012). The importance of kinetics and redox in the biogeochemical cycling of iron in the surface ocean. Front. Microbiol. 3:219. doi: 10.3389/fmicb.2012.00219
Cruz-Lopez, R., and Maske, H. (2015). A non-amplified FISH protocol to identify simultaneously different bacterial groups attached to eukaryotic phytoplankton. J. Appl. Phycol. 27, 797–804. doi: 10.1007/s10811-014-0379-2
Glibert, P. M., Anderson, D. M., Gentien, P., Granéli, E., and Sellner, K. G. (2005). The global complex phenomena of harmful algae blooms. Oceanography 18, 130–141. doi: 10.5670/oceanog.2005.49
Green, D. H., Llewellyn, L. E., Negri, A. P., Blackburn, S. I., and Bolch, C. J. (2004). Phylogenetic and functional diversity of the cultivable bacterial community associated with the paralytic shellfish poisoning dinoflagellate Gymnodinium catenatum. FEMS Microbiol. Ecol. 47, 345–357. doi: 10.1016/S0168-6496(03)00298-8
Guillard, R. R. L., and Hargraves, P. E. (1993). Stichochrysis immobilis is a diatom, not a chrysophyte. Phycologia 32, 234–236. doi: 10.2216/i0031-8884-32-3-234.1
Hallegraeff, G., and Gollasch, S. (2006). “Ecological studies: ecology of harmful algae,” in Anthropogenic Introductions of Microalgae, eds M. M. Caldwell, G. Heldmaier, R. B. Jackson, O. L. Lange, H. A. Mooney, E.-D. Schulze, and U. Sommer (Berlin; Heidelberg: Springer), 379–402.
Hoagland, P., and Scatasta, S. (2006). “Ecological studies: ecology of harmful algae,” in The Economic Effects of Harmful Algal Blooms, eds M. M. Caldwell, G. Heldmaier, R. B. Jackson, O. L. Lange, H. A. Mooney, E.-D. Schulze, and U. Sommer (Berlin; Heidelberg: Springer), 391–402.
Honner, S., Kudela, R. M., and Handler, E. (2012). Bilateral mastoiditis from red tide exposure. J. Emerg. Med. 43, 663–666. doi: 10.1016/j.jemermed.2010.06.007
Jauzein, C., Evans, A. N., and Erdner, D. L. (2015). The impact of associated bacteria on morphology and physiology of the dinoflagellate Alexandrium tamarense. Harmful Algae 50, 65–75. doi: 10.1016/j.hal.2015.10.006
Küpper, F. C., Carrano, C. J., Kuhn, J.-U., and Butler, A. (2006). Photoreactivity of iron(III)-aerobactin: photoproduct structure and iron(III) coordination. Inorgan. Chem. 45, 6028–6033. doi: 10.1021/ic0604967
Lewitus, A. J., Horner, R. A., Caron, D. A., Garcia-Mendoza, E., Hickey, B. M., Hunter, M., et al. (2012). Harmful algal blooms along the North American west coast region: history, trends, causes, and impacts. Harmful Algae 19, 133–159. doi: 10.1016/j.hal.2012.06.009
Liu, C. L., Place, A. R., and Jagus, R. (2017). Use of antibiotics for maintenance of axenic cultures of amphidinium carterae for the analysis of translation. Marin Drugs 15:242. doi: 10.3390/md15080242
Lupette, J., Lami, R., Krasovec, M., Grimsley, N., Moreau, H., Piganeau, G., et al. (2016). Marinobacter dominates the bacterial community of the Ostreococcus tauri phycosphere in culture. Front. Microbiol. 7:1414. doi: 10.3389/fmicb.2016.01414
Maldonado, M. T., Strzepek, R. F., Sander, S., and Boyd, P. W. (2005). Acquisition of iron bound to strong organic complexes, with different Fe binding groups and photochemical reactivities, by plankton communities in Fe-limited subantarctic waters. Glob. Biogeochem. Cycles 19:GB4S23. doi: 10.1029/2005GB002481
Martin, J. H., and Fitzwater, S. E. (1988). Iron deficiency limits phytoplankton growth in the north-east Pacific subarctic. Nature 331, 341–343. doi: 10.1038/331341a0
McQuaid, J. B., Kustka, A. B., Oborník, M., Horák, A., McCrow, J. P., Karas, B. J., et al. (2018). Carbonate-sensitive phytotransferrin controls high-affinity iron uptake in diatoms. Nature 555, 534–537. doi: 10.1038/nature25982
Miethke, M., and Marahiel, M. A. (2007). Siderophore-based iron acquisition and pathogen control. Microbiol. Mol. Biol. Rev. 71, 413–451. doi: 10.1128/MMBR.00012-07
Naito, K., Imai, I., and Nakahara, H. (2008). Complexation of iron by microbial siderophores and effects of iron chelates on the growth of marine microalgae causing red tides. Phycol. Res. 56, 58–67. doi: 10.1111/j.1440-1835.2008.00485.x
Ramanan, R., Kim, B. H., Cho, D. H., Oh, H. M., and Kim, H. S. (2016). Algae-bacteria interactions: evolution, ecology and emerging applications. Biotechnol. Adv. 34, 14–29. doi: 10.1016/j.biotechadv.2015.12.003
Raven, J. A. (2013). Iron acquisition and allocation in stramenopile algae. J. Exp. Bot. 64, 2119–2127. doi: 10.1093/jxb/ert121
Raymond, K. N., Allred, B. E., and Sia, A. K. (2015). Coordination chemistry of microbial iron transport. Acc. Chem. Res. 48, 2496–2505. doi: 10.1021/acs.accounts.5b00301
Roegner, G. C., Hickey, B. M., Newton, J. A., Shanks, A. L., and Armstrong, D. A. (2002). Wind-induced plume and bloom intrusions into Willapa Bay, Washington. Limnol. Oceanogr. 47, 1033–1042. doi: 10.4319/lo.2002.47.4.1033
Sandy, M., and Butler, A. (2009). Microbial iron acquisition: marine and terrestrial siderophores. Chem. Rev. 109, 4580–4595. doi: 10.1021/cr9002787
Seymour, J. R., Amin, S. A., Raina, J. B., and Stocker, R. (2017). Zooming in on the phycosphere: the ecological interface for phytoplankton-bacteria relationships. Nat. Microbiol. 2:17065. doi: 10.1038/nmicrobiol.2017.65
Sutak, R., Botebol, H., Blaiseau, P. L., Léger, T., Bouget, F. Y., Camadro, J. M., et al. (2012). A comparative study of iron uptake mechanisms in marine microalgae: iron binding at the cell surface is a critical step. Plant Physiol. 160, 2271–2284. doi: 10.1104/pp.112.204156
Tubaroa, A., Sosaa, S., Altiniera, G., Soranzob, M. R., Satakec, M., Loggiaa, R. D., et al. (2004). Short-term oral toxicity of homoyessotoxins, yessotoxin and okadaic acid in mice. Toxicon 43, 439–445. doi: 10.1016/j.toxicon.2004.02.015
Tweddle, J. F., Strutton, P. G., Foley, D. G., O'Higgins, L., Wood, A. M., Scott, B., et al. (2010). Relationships among upwelling, phytoplankton blooms, and phycotoxins in coastal Oregon shellfish. Mar. Ecol. Prog. Ser. 405, 131–145. doi: 10.3354/meps08497
Vraspir, J. M., and Butler, A. (2009). Chemistry of marine ligands and siderophores. Ann. Rev. Mar. Sci. 1, 43–63. doi: 10.1146/annurev.marine.010908.163712
Wu, J., and Luther, G. W. III. (1994). Size-fractionated iron concentrations in the water column of the western North Atlantic Ocean. Limnol. Oceanogr. 39, 1119–1129. doi: 10.4319/lo.1994.39.5.1119
Yamamoto, S., Okujo, N., Yoshida, T., Matsuura, S., and Shinoda, S. (1994). Structure and iron transport activity of vibrioferrin, a new siderophore of Vibrio parahaemolyticus. J. Biochem. 115, 868–874. doi: 10.1093/oxfordjournals.jbchem.a124432
Yarimizu, K., Cruz-López, R., Auerbach, H., Heimann, L., Schünemann, V., and Carrano, C. J. (2017). Iron uptake and storage in the HAB dinoflagellate Lingulodinium polyedrum. Biometals, 30, 945–953. doi: 10.1007/s10534-017-0061-7
Keywords: Lingulodinium polyedrum, Marinobacter, iron, algal-bacterial interactions, binary culture
Citation: Yarimizu K, Cruz-López R and Carrano CJ (2018) Iron and Harmful Algae Blooms: Potential Algal-Bacterial Mutualism Between Lingulodinium polyedrum and Marinobacter algicola. Front. Mar. Sci. 5:180. doi: 10.3389/fmars.2018.00180
Received: 27 February 2018; Accepted: 07 May 2018;
Published: 05 June 2018.
Edited by:
Stanley Chun Kwan Lau, Hong Kong University of Science and Technology, Hong KongReviewed by:
Marius Nils Müller, Universidade Federal de Pernambuco, BrazilSimon M. Dittami, UMR8227 Laboratoire de Biologie Intégrative des Modèles Marins, France
Copyright © 2018 Yarimizu, Cruz-López and Carrano. This is an open-access article distributed under the terms of the Creative Commons Attribution License (CC BY). The use, distribution or reproduction in other forums is permitted, provided the original author(s) and the copyright owner are credited and that the original publication in this journal is cited, in accordance with accepted academic practice. No use, distribution or reproduction is permitted which does not comply with these terms.
*Correspondence: Carl J. Carrano, Y2NhcnJhbm9AbWFpbC5zZHN1LmVkdQ==