- 1Reef and Ocean Ecology Laboratory, College of Science and Engineering, James Cook University, Townsville, QLD, Australia
- 2Centre of Excellence for Coral Reef Studies, Australian Research Council, Townsville, QLD, Australia
- 3Tropical Water & Aquatic Ecosystem Research (TropWATER), James Cook University, Townsville, QLD, Australia
- 4Institute of Mechanics, Materials and Civil Engineering, Université catholique de Louvain, Louvain-la-Neuve, Belgium
Most coral reef fishes have a pelagic larval stage before recruiting to reefs. The survival of larvae and their subsequent recruitment can drive the dynamics of reef populations. Here we show that the recruitment of the snapper Lutjanus carponotatus to One Tree Island in the Capricorn Bunker Group, in the southern Great Barrier Reef, was highly variable over 23 years. We predicted that the currents in the Capricorn Bunker Group, including their wind driven components and the Capricorn Eddy (a nearby transient oceanic eddy), would affect patterns of recruitment. A biophysical model was used to investigate this prediction. L. carponotatus were collected from One Tree Island and the dates when they were in the plankton as larvae were determined from their otoliths. The winds present during the pelagic phases of the fish were examined; they were found to have survived either longshore (SSE) winds that induced little cross shelf movement in the larval plume or cross shelf (ENE) winds that induced little longshore movement. The unidirectional transportation of the larval plume in these conditions was favorable for recruitment as it kept the plume concentrated in the Capricorn Bunker Group. These winds were more prevalent in the periods of peak L. carponotatus production that preceded high recruitment. Dispersal under average winds (6.2 m s−1 from the prevailing ESE) and strong winds (velocity 1.5 times average), with and without the Capricorn Eddy, was also modeled. Each of these combinations were less favorable for recruitment than the longshore and cross shelf winds larval L. carponotatus survived before reaching OTI. The larval plume was comparatively less concentrated in the Capricorn Bunker Group under average winds. Strong winds transported the larval plume far longshore, to the NW, away from the Capricorn Bunker Group, while the Capricorn Eddy transported larvae seaward into oceanic waters. Larval swimming could counteract these dispersive forces; however, significant dispersion had occurred before larvae developed strong swimming and orientation abilities. This study provides a physical proxy for the recruitment of snapper. Further, we have demonstrated that great insights into recruitment variability can be gained through determining the specific conditions experienced by survivors.
Introduction
Most coral reef fishes have bipartite lifecycles, where adults produce large numbers of larvae that spend some time developing in the plankton before metamorphosing, settling and recruiting to reefs. Recruitment is the raw material for post-settlement processes. It can be a fundamental driver of the population dynamics of coral reef fishes, especially for relatively short-lived species (e.g., Victor, 1983; Kingsford, 2009). Only a small fraction of the larvae produced by coral reef fishes will survive the pelagic phase (Houde, 2008). As large numbers of larvae are produced, small changes in the pre-settlement mortality rate can greatly affect the strength of recruitment events (Cowen et al., 2000; Houde, 2008). The pre-settlement mortality rate of fish larvae can depend on multiple factors including the availability of food (e.g., Cushing, 1975, 1990), the abundance of predators (e.g., Moller, 1984; McGurk, 1986) and favorable or unfavorable oceanography (e.g., Sponaugle and Pinkard, 2004; Sponaugle et al., 2005), and this complexity can make it difficult to identify causes of variability in recruitment (Leggett and Deblois, 1994; Houde, 2008).
Flow fields can influence the magnitude of recruitment over multiple spatial scales. For example, at small spatial scales, tidal eddies regularly form as tidal currents flow past reefs and these eddies can retain larvae (Burgess et al., 2007). Furthermore, water circulation can be reduced in dense reef matrices (the “sticky water effect”) and larvae can be retained in this slow moving water (Andutta et al., 2012). Alternatively, currents can expatriate fish larvae away from suitable habitat, leading to poor recruitment (Sinclair, 1988; Lenanton et al., 1991; Fletcher, 1995).
In addition to physical oceanographic features, the biological characteristics of fish larvae can greatly affect their dispersion (Kingsford et al., 2002; Paris et al., 2013; Wolanski and Kingsford, 2014; Bottesch et al., 2016). The larvae of many fish species can maintain swim speeds that exceed local mean current speeds for extended periods of time, they can, therefore, influence their dispersal trajectories (Fisher, 2005; Leis, 2006). They can also orient to reefs using a variety of senses including a sun compass (e.g., Leis and Carson-Ewart, 2003; Mouritsen et al., 2013) and a magnetic compass (e.g., Bottesch et al., 2016) for long distance orientation, and hearing (e.g., Mann et al., 2007; Wright et al., 2010) and chemotaxis (e.g., Gerlach et al., 2007; Dixson et al., 2008; Paris et al., 2013) when they are closer to reefs. The exceptional swimming and orientation abilities of reef fish larvae, in combination with hydrodynamic retention mechanisms near reefs, may prevent the expatriation of some larvae away from natal reefs (Almany et al., 2007; Andutta et al., 2012). Indeed, high rates of self-seeding have been reported in local populations of coral reef fishes (Jones et al., 1999; Almany et al., 2007). However, many larvae will not return to their natal reefs and dispersal kernels (the potential for successful dispersal connections) can extend tens to hundreds of kilometers from the spawning of adults (Jones, 2015; Williamson et al., 2016).
Given the complex oceanographic regimes of coral reef ecosystems and the considerable swimming and orientation abilities of fish larvae, biophysical modeling is an ideal technique for investigating variability in recruitment (Simpson et al., 2013; Wolanski, 2017). Biophysical models combine oceanographic and behavioral models. They have been utilized to model connectivity and recruitment variability in coral reef systems to great effect (e.g., Paris and Cowen, 2004). They have also been used extensively to investigate the recruitment of commercially important species to fisheries (e.g., Caputi et al., 1996; Hare et al., 1999). Biophysical models are useful tools for making predictions on self-seeding and connectivity between populations at biologically relevant spatial and temporal scales, which can aid in the management of coral reef fish populations (Cowen et al., 2006; Melbourne-Thomas et al., 2011).
The inherent stochasticity and complexity of coral reef systems is not always well captured in biophysical models (Siegel et al., 2003, 2008). The models are typically forced with general physical conditions. While this approach is useful for reaching broadly applicable conclusions, great insights can be gained from using tailored forcings to answer specific questions (e.g., Mitarai et al., 2008). High variability in recruitment, both within and among seasons, appears to be typical of many coral reef systems (e.g., Doherty, 1987; Tolimieri et al., 1998). A comprehensive understanding of recruitment variability can be attained through investigating the conditions present when successful recruits were known to be in the plankton (Sponaugle and Pinkard, 2004; Valles et al., 2009). Accordingly, the causal factors of recruitment variability could be investigated by forcing a biophysical model with the conditions present during the pelagic larval phases of successful recruits.
Stripey snapper, Lutjanus carponotatus, is a predatory coral reef fish that is also targeted by fisheries. The recruitment of L. carponotatus to One Tree Island (OTI) in the Capricorn Bunker Group (CBG) in the southern Great Barrier Reef (GBR) has been monitored since 1995, and has been found to vary greatly interannually (Kingsford, 2009). The complex oceanography of the region could potentially affect the strength of recruitment events. The Capricorn Eddy (CE), a large, transient mesoscale oceanic eddy, forms offshore of the CBG (Weeks et al., 2010; Mao and Luick, 2014). It is presumably ecologically important as it upwells cool nutrient rich water to the surface (Weeks et al., 2010) and mesoscale eddies can greatly influence the dispersal trajectories of fish larvae (Sponaugle et al., 2005). Additionally, the CBG lies on a shallow section of the Australian continental shelf, and the surface currents in this region are largely wind driven (Andutta et al., 2013; Mao and Luick, 2014). A biophysical model has previously been used to simulate the dispersion of larvae seeded from OTI, and the subsequent recruitment of the larvae to OTI and to nearby downstream reefs (Wolanski and Kingsford, 2014). The dispersal trajectories of larvae were found to be greatly influenced by swimming behavior.
The broad objective of this study was to determine the conditions experienced by L. carponotatus larvae before they successfully recruited to OTI. Identifying the conditions that facilitated recruitment could improve our current understanding of the inter-annual variability in recruitment that is common among coral reef fishes. The specific aims were to: (1) examine the otoliths of successful L. carponotatus recruits caught from OTI to determine when they were in the plankton as larvae; (2) identify the wind conditions that fish experienced on these dates; (3) establish if the prevalence of these conditions could be used as a proxy to predict recruitment; (4) use biophysical modeling to determine the influence of wind, the CE and the swimming behavior of larvae on patterns of recruitment. Further, we hypothesized that the wind conditions survived by successful recruits during their larval phase (as identified in specific aim 2) would generate currents that reduced larval morality via expatriation, facilitating recruitment. We evaluated this hypothesis in specific aim 4 by modeling the identified winds.
Materials and Methods
The Long-Term Abundance of Lutjanus carpontatus at One Tree Island
One Tree Island (OTI) is a small coral cay, located on the eastern side of a ~5.5 km by 3.5 km coral reef (23°29′48.38″S, 152°4′8.32″E; Figure 1). OTI is in the Capricorn Bunker Group (CBG), within the southern Great Barrier Reef (GBR). The CBG lies near the edge of the Australian continental shelf, in waters approximately 50 m deep.
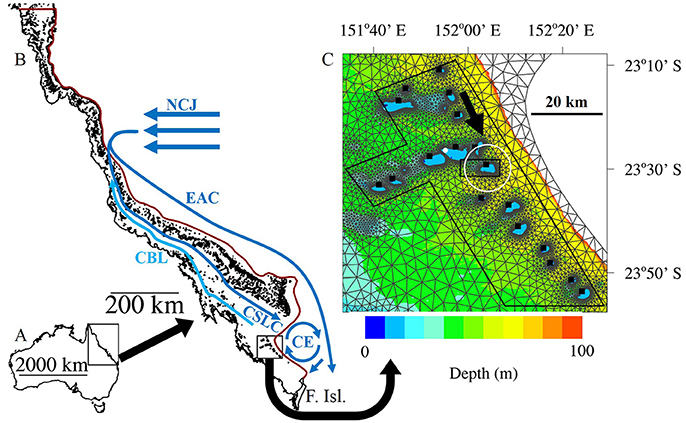
Figure 1. The study region. (A) Australia. (B) The Great Barrier Reef (GBR). The broad scale oceanographic features of the southern GBR are shown including: The North Caledonian Jet (NCJ), the East Australian Current (EAC), the Coastal Boundary Layer (CBL), the Coral See Lagoonal Current (CSLC) and the Capricorn Eddy (CE). Adapted from Wolanski et al. (2013), and Mao and Luick (2014). The red line denotes the 200m isobath, which is also the open boundary of the model. The open boundary joins with the Queensland coast (closed boundary) at the tip of Australia to the north and at Fraser Island (F. Isl.) to the south. (C) A zoomed view of the Capricorn Bunker Group (CBG) overlayed with the SLIM mesh. Lutjanus carponotatus larvae were seeded from the locations marked by the black squares. When the larvae were active (B scenarios) they were programmed to swim to the SSE at a bearing of 155° (black arrow) unless they were within 5 km of One Tree Island (OTI; denoted by the white circle), where they were made to swim toward the center of OTI instead. The number of larvae remaining in the black irregular octagon were tracked through time to calculate the residence time of the CBG region. The number of potential settlers present around OTI were counted within the black rectangle at the end of the Pelagic Larval Duration. The white dot shows the location of Heron Island weather station. The bathymetry is only shown for depths less than 100 m.
Lutjanus carponotatus have been counted in visual surveys performed in reef slope, lagoon edge and lagoon center habitats at OTI in January or February of each year since 1995. Counts were made at six or seven sites per habitat in five 5 × 25 m transects per site (for complete methods see Kingsford, 2009). Fish were not counted in the lagoon center habitat in 1998 and 1999, and they were not counted in the reef slope habitat in 2014, but L. carponotatus are rare in this habitat. The Standard Lengths (SL's) of the fish were estimated to the nearest 50 mm and these measurements were used to divide the fish into size categories. Length estimates were cross-checked by measuring the lengths of speared fish (n = 114) and, for consistency, Kingsford conducted all of the fish counts. Kingsford (1998) found that experienced divers could place large fish into the correct 50 mm size category 80% of the time. Further, in studies on smaller species (less than 300 mm), experienced divers may be able to consistently estimate lengths to within 10 mm of the true value (Kingsford, 1998). It is therefore acceptable to collect size-frequency data in 50 mm size categories (Kingsford, 1998). Fish with SL's greater than 200 mm were classified as adults. Most L. carponotatus of this size (200 mm SL ~ 237 mm FL) are mature (Kritzer, 2004) and have three or more annulus bands on their otoliths (Kingsford, 2009), suggesting they have lived through at least three winters (Newman et al., 1996). Fish with SL's less than 150 mm were classified as recruits as most L. carponotatus of this size (150 mm SL ~ 177 mm FL) are immature (Kritzer, 2004) and have no or only one annulus band on their otoliths (Kingsford, 2009), suggesting they are yet to live through a winter or have only lived through one (Newman et al., 1996).
Although there are no data available on the sources of L. carponotatus recruits to OTI, it was possible that the abundance of recruits would vary with the abundance of adult L. carponotatus at OTI as dispersal kernels tend to decrease rapidly with distance from the natal reef (e.g., Buston et al., 2012; Williamson et al., 2016). Accordingly, the relationship between recruit abundance (1996–2017) and adult abundance (1995–2016) was tested using a Spearman's Correlation. A Spearman's Correlation was performed because the data did not meet the Pearson's Correlation assumption of a bivariate normal distribution. A one-year time lag was applied as the recruits observed in any given year would have been produced by the adult population present in the previous year. Adult and recruit abundance data from 1998 and 1999 were not included as the lagoon center habitat was not sampled in these years. Recruit abundance data from the reef slope habitat were not included as no recruits were observed in this habitat. The adult abundance data from 2014 were not included as the reef slope habitat was not sampled in this year.
Otolith Analysis
Thirty-Five L. carponotatus were caught at OTI, either with spear-guns and hand spears (February 2004/2005, n = 12 recruits) or in light traps over the reef slope (January 2015, n = 23 potential settlers). These fish were caught opportunistically so samples were only available in some years. The fish had survived the hydrodynamic conditions present during their planktonic phases. It was, therefore, hypothesized that the currents, including their wind driven components, had facilitated their successful recruitment to OTI from their unknown sources. The dates when the fish were in the plankton were determined from the pre-settlement phases recorded on their otoliths. Sagittal otoliths were removed from the 35 L. carponotatus and sectioned following Kingsford (2009). Photographs of the otoliths were taken at 400 times magnification using a Leica microscope camera and the daily rings were counted from the photographs using Leica IM50 software. Counts were made from the hatch marks at the cores of the otoliths to the edges. The otoliths were examined in random order twice, with each session separated by a few weeks. If the counts differed by more than 10%, the otoliths were re-examined a third time. The third count was used if it was within 10% of either of the previous counts. The average of the closest counts was taken as the age of the recruit. Only one otolith was rejected via this method.
It was assumed that the fish hatched approximately 1 day after they were spawned, which is well within the range of hatching times recorded for other lutjanid species (15–30 h; Leu and Liou, 2013), and so a day was added to the counts to determine the date of spawning. The recruits from 2004/2005 ranged in age from 197 days (approximately 5 months) to 264 days old (approximately 9 months). They were spawned in May, June and July, and typically at around the same time. The earliest spawning date recorded was the 10 May. The potential settlers from 2015 ranged in age from 18 to 25 days, and were all spawned between the end of December 2014 and the beginning of January 2015. Accordingly, the spawning season for L. carponotatus could be long, continuous or made up of multiple discreet spawning periods within a year.
The locations of the settlement marks on the otoliths of the recruits from 2004/2005 were noted. The pelagic larval duration (PLD) of each recruit was calculated as the number of days between spawning and settlement. The average PLD was 25 days ± 0.6 SE (range 22–28 days) and the ages of 35% of the potential settlers from 2015 fell within the observed range of PLD's. The dates between spawning and settlement (2004/2005 recruits) or between spawning and capture (2015 potential settlers) were taken as the dates the fish were in the plankton as larvae.
Survivor Specific Wind Analysis
We determined the wind conditions present during the periods when the surviving L. carponotatus caught from OTI were in the plankton. The wind conditions have been measured hourly at a weather station on Heron Island (HI; 23°26′24.00″S, 151°55′12.00″E; Figure 1C), which is less than 10 km from OTI, from February 1999. These data were provided by the Australian Government Bureau of Meteorology. The longshore and cross shelf wind velocities were respectively calculated as the velocity components parallel and perpendicular to the 200 m isobath east of the CBG, which was measured to be offset 30° counter clockwise from the north/south axis. The ratios between the sums of the positive and negative vector components in the cross shelf (from the WSW and from the ENE) and longshore (from the SSE and from the NNW) directions were calculated for the dates when the fish were in the plankton. Longshore winds from the SSE were present when the 2004/2005 cohort of recruits were in the plankton as larvae, and these winds had a balanced (near 50:50) cross shelf vector ratio. Cross shelf winds from the ENE, with a balanced longshore wind ratio, were present during the pelagic larval phases of the potential settlers from the 2015 cohort. The cross shelf and longshore vector wind ratios were also calculated over 25 day intervals starting on successive days, from 1 January 2000 to 31 December 2016, in the same manner as a running average. A 25 day interval was used because it was the average PLD of the L. carponotatus recruits in this study. The wind ratios over the intervals, therefore, represent the winds that would have been present during the pelagic phases of L. carponotatus larvae spawned on each successive day over the 18 year period. The rarity of the identified conditions was determined by counting the number of historic 25 day intervals with winds as or more balanced (ratios closer to 50:50) than the winds the fish in this study survived during their pelagic phases.
We hypothesized that the identified longshore winds with a balanced cross shelf component and the identified cross shelf winds with a balance longshore component would be favorable for recruitment as they were survived by successful recruits. Defining these conditions as favorable, we predicted that the recruitment of L. carponotatus to OTI could have been related to the prevalence favorable winds during the time of peak larval production. Kritzer (2004) found that the gonadosomatic indices of female L. carponotatus from the Palm and Lizard Island groups peaked in October, November and December. Additionally, Russell et al. (1977) found that the recruitment of juvenile reef fish from multiple taxa to OTI peaked in January and February, suggesting larval production peaked in December and January. Therefore, the production of L. carponotatus larvae probably peaks at OTI from October through to January. Of the identified winds with balanced cross shelf and longshore components, only the winds with a balanced longshore component occurred during the period of peak production. The number of historic 25 day intervals with a balanced longshore component occurring in the peak production period (2000/2001–2015/2016) were correlated with the measured abundance of L. carponotatus recruits at OTI (2002–2017) to determine if the magnitude of recruitment was related to the prevalence of the winds. A Spearman's Correlation was performed because the data did not meet the Pearson's Correlation assumption of a bivariate normal distribution. A one-year time lag was applied as the recruits observed in any given year would have been produced in the preceding period of peak production. Further, biophysical modeling was carried out to determine the capacity for the identified winds to generate favorable currents when compared to seasonally dominant conditions, and to investigate the influence of the Capricorn Eddy (CE) and larval swimming behavior on recruitment.
Biophysical Modeling
The Second-generation Louvain-la-Neuve Ice-ocean Model (SLIM) was used to model the dispersion of L. carponotatus larvae from reefs in the CBG. SLIM has an unstructured grid which can be made finer in areas where a high resolution is required and coarser in open areas, prioritizing the allocation of computational power. Unstructured grids are particularly useful in coral reef systems (Lambrechts et al., 2008) as a high spatial resolution is required to avoid erasing small reefs and to accurately simulate the current shear distribution near reef edges (Wolanski et al., 1996). SLIM has been used to effectively model the hydrodynamics of the GBR system (e.g., Lambrechts et al., 2008; Delandmeter et al., 2017; Wildermann et al., 2017). The two-dimensional depth averaged version of SLIM was used in this study; this was valid because two-dimensional depth averaged models can realistically simulate observed currents in shallow, vertically well mixed water (Lambrechts et al., 2008). GBR shelf waters are generally shallow and vertically well mixed (Luick et al., 2007). Additionally, small scale three-dimensional modeling of the area around OTI showed that passive larvae released from OTI had similar dispersal patterns whether they were set to remain near the surface, at mid-depth or near the bottom (Critchell et al., 2015).
SLIM solves the shallow-water equations discretized in space with a second order discontinuous Galerkin finite element method and in time with a second order implicit Runge-Kutta method (Lambrechts et al., 2008). The hydrodynamic model has a time step of 10 min. The model domain encompassed the entire GBR with the open boundary following the 200 m isobath and the closed boundary following the Queensland coastline. The model mesh was made up of nearly 26,000 triangles, which ranged in size from less than 300 m near reefs to more than 20 km in open water (Figure 1B). The bathymetry data used in the model for areas outside of the CBG were derived from Geoscience Australia's Australian Bathymetry and Topography 2005 data set which has an ~250 m resolution (Webster and Petkovic, 2005). The data for the CBG area were derived from Project 3DGBR data which has a 100 m resolution (Beaman, 2010). The model was forced at the open boundary (Figure 1B) with tides, the inflow of the North Caledonian Jet (NCJ) from the Coral Sea and the CE (some scenarios; Table 1). It was also forced with wind via a spatially uniform vector field applied over the entire domain.
The tidal conditions at OTI were predicted using AusTide 2015 version 1.10.1 and approximated with a synthetic semidiurnal tide with an amplitude of 1 m and a period of 12 h for use in the model. The magnitude and latitude of the NCJ inflow into the GBR system from the Coral Sea are largely unknown (Ganachaud et al., 2014). The NCJ was simulated as a 1.5 × 106 m3 s−1 inflow between 15°54′36″ S and 17°43′12″ S following Andutta et al. (2011). The CE periodically forms off the continental shelf, to the east of the CBG. While the conditions preceding the formation of the eddy have been described (Mao and Luick, 2014), the frequency of formation and the magnitude of the resulting cyclonic currents are largely unknown. In scenarios 2 and 4, the CE was modeled as a 0.3 × 106 m3 s−1 inflow from the open boundary north of Fraser Island and an outflow of equal magnitude added to the Coral See Lagoonal Current (Figure 1B).
The yearly average wind measured at Heron Island weather station from 1999 to 2013 was 6.2 m s−1 ± 2.7 SD and the prevailing wind direction was 119.9°. These average conditions were imposed as a constant wind in the average wind model runs (all 1 and 2 scenarios). In the strong wind runs (all 3 and 4 scenarios), a constant wind was still forced from the prevailing direction, but the velocity imposed was 1.5 times average (9.35 m s−1). All combinations of constant wind (average or strong wind from the prevailing direction) and CE activity were modeled in scenarios 1–4 to predict the influence of physical conditions on L. carponotatus recruitment to OTI.
The longshore SSE and cross shelf ENE wind conditions present during the pelagic phases of the fish caught at OTI were also modeled to determine if they would generate currents conducive to the recruitment of L. carponotatus to OTI. These conditions were imposed as spatially constant, but temporally variable. For the 2004/2005 cohort, winds from the pelagic phases of fish that survived the SSE winds with the most balanced (cross shelf vector wind ratio closest to 50:50; scenario 5, SSE 1) and least balanced (ratio farthest from 50:50; scenario 6, SSE 2) cross shelf components were used. The fish in the 2015 cohort were all spawned within 8 days of each other so they experienced very similar conditions. Accordingly, a fish from this cohort was randomly chosen, and the ENE winds present during its pelagic phase were modeled (scenario 7, ENE). The output of the hydrodynamic model was saved every 30 min and coupled with the advection-dispersion model.
L. carponotatus larvae were seeded as particles in the advection-dispersion model. Although there are no data on the sources of L. carponotatus recruits to OTI, reefs in the CBG are a likely source. The potential for successful larval dispersal (dispersal kernels) tends to decrease with distance from the natal reef (Jones, 2015; Williamson et al., 2016). Further, Williamson et al. (2016) conducted a genetic parentage analysis to investigate patterns of connectivity in populations of two coral trout species, Plectropomus maculatus and Plectropomus leopardus, in the southern GBR. The PLD of both species was 26 days ± 2 SD, comparable to the 25 days ± 2 SD reported for L. carponotatus in this study. Samples were taken from reefs in the CBG, the Keppel Islands (~100 km from the CBG) and the Percy Island Group (~250 km from the CBG). P. leopardus were substantially more abundant in the CBG than P. maculatus, comprising 81% of all coral trout sightings in the region. However, P. maculatus were more abundant than P. leopardus in the other study regions, comprising 99 and 84% of coral trout sightings in the Keppel Islands and the Percy Island Group respectively. A large majority (74%) of the parentage assigned P. leopardus recruits to the CBG were spawned from reefs in the CBG. Despite the comparable rarity of P. maculatus in the CBG, a large proportion (44%) of the parentage assigned P. maculatus recruits to the CBG also came from within the region. Further, late stage P. leopardus larvae did not swim as strongly as L. carponotatus larvae when tested in a flume tank (Fisher et al., 2005), suggesting L. carponotatus have a greater capacity to limit their dispersal. Due to a lack of available data, we made the simplifying assumptions that recruits to OTI would come from OTI and other reefs in the CBG, and that the adults from these reefs would have the same reproductive capacity. Accordingly, 1,000 larvae per reef were seeded from 18 reefs in the CBG, including OTI (18,000 larvae in total; Figure 1C). We made the further simplifying assumption that larvae advected outside of the model domain would not be able to return, even though mesoscale eddy activity has been associated with the delivery of fish larvae to reefal waters (e.g., Sponaugle et al., 2005) and the CE lies just outside of the model domain (see Discussion section The Capricorn Eddy). The effect of sub-grid scale processes on diffusion were simulated with a horizontal diffusion coefficient. While the exact value of the coefficient is unknown, 5 m2 s−1 is realistic (Spagnol et al., 2002; Wolanski and Kingsford, 2014) and was therefore used in this study. The use of a two-dimensional model necessitated the assumption that larvae were vertically well mixed in the water column.
Larvae were either passive or active, depending on the model scenario. The swimming speed of the active larvae was set to change through time as they developed, following Wolanski and Kingsford (2014). Larvae were set to begin swimming when they reached post-flexion. The swimming speed then increased linearly to the maximum sustainable swimming speed, which was maintained up until the end of the PLD. Thereafter, the swimming speed was reduced to zero because the larvae were assumed to be exhausted. The swimming speed was changed through time as follows:
where t was the time and Uswim was the swimming speed of the larvae. The maximum sustainable swimming speed, Umax, was set to 26 cm s−1, which is half of the critical swimming speed for late stage L. carponotatus larvae (52 cm s−1; Fisher et al., 2005). Half of the critical swimming speed is a good approximation of Umax (Fisher and Wilson, 2004) and a Umax of 26 cm s−1 is within the range of values calculated for lutjanids (18–29 cm s−1) by Fisher and Bellwood (2002). Tpost−flexion was the time taken for the larvae to develop to post-flexion. Eggs were assumed to hatch 1 day after spawning (Leu and Liou, 2013). Notochord flexion, which marks the beginning of the post-flexion phase, was assumed to be complete 16 days after hatching, which is within the range of values recorded for lutjanid species (16 to 18 days; Clarke et al., 1997). Tpost−flexion was therefore set to 17 days. Tmax was the time taken for the larvae to be able to swim at Umax. As mentioned the average PLD calculated for the L. carponotatus recruits caught at OTI was 25 days ± 0.6 SE. Tmax was set to 21 days, 4 days before the end of the PLD (Wolanski and Kingsford, 2014).
The swimming direction of the active larvae was determined from the prescribed behaviors. Larvae were made to swim to the SSE at a bearing of 155°. A strong SSE directional swimming response was reported for pre-settlement larvae and early settlers of the cardinal fish species Ostorhinchus doederleini caught and tested near OTI (Mouritsen et al., 2013). Mouritsen et al. (2013) suggested that the response evolved to counteract the prevailing NNW current. It was demonstrated that the O. doederleini larvae were navigating using a sun compass mechanism (Mouritsen et al., 2013) and sun compass orientation has also been documented in other coral reef fish species (e.g., Leis and Carson-Ewart, 2003; Berenshtein et al., 2014). Bottesch et al. (2016) demonstrated that newly settled O. doederleini at OTI can also orient their swimming to the SE at night via a magnetic compass mechanism. In this study, it was assumed that L. carponotatus spawned from reefs in the CBG (which are affected by the same prevailing NNW current as OTI) would have a strong SSE directional swimming response like O. doederleini.
When larvae got to within 5 km of OTI, they were made to swim toward the center of OTI, rather than to the SSE. This represents the chemotaxis driven orientation documented in the larvae of many coral reef fish species, where they can swim to reefs by discriminating between water with a reef odor and oceanic water (e.g., Paris et al., 2013). Wolanski and Kingsford (2014) simulated the odor plume from OTI and found it extended several kilometers from the reef.
For each combination of physical forcings, larvae were modeled as passive in the “A” scenarios and as actively swimming in the “B” scenarios to determine the influence of swimming behavior on recruitment. All scenarios were run for 27 days, 2 days longer than the average PLD, and are summarized in Table 1. Three replicate runs were conducted per scenario to account for the inherent variability in the model.
Quantifying the Modeling Results
The residence time (the average time a “tracer” will remain in a system; Black et al., 1991) of the CBG was calculated to determine the capacity for retention within the CBG for each of the modeled scenarios (Figure 1C). It was calculated as the time taken for the concentration of larvae within the CBG to fall to 1/e (~0.37) of its original value, following Prandle (1984) and Choi and Lee (2004).
The number of larvae near OTI at the end of the PLD (25 days) were counted for each of the modeled scenarios to estimate the number of potential settlers (Figure 1C). It was assumed that the potential settlers would be able to hear the ambient noise from OTI if they were within 1 km of the reef (Mann et al., 2007). The number of potential settlers were therefore counted within a rectangular capture box, with bounds located 1 km to the north, south and east of OTI. Larvae were counted at high tide, so the box was extended out to the west by 5 km to account for the larvae that would have been transported into the capture area with the tidal excursion over 6 h as the tide went out. The capture distances used are conservative given Radford et al. (2011) detected reef noise 5 km from a reef and larvae can likely smell OTI from several kilometers away (Wolanski and Kingsford, 2014). The Self-Seeding rate (% SS) was subsequently calculated as the percentage of the total number of settlers counted around OTI that had originally been seeded from OTI. The return rate (% return) was calculated at the percentage of the 1,000 larvae seeded from OTI that returned to OTI. Expressed mathematically:
where nTTL was the total number of potential settlers counted around OTI and nOTI was the subset of counted potential settlers that had originally been seeded from OTI.
Model Validation
The currents simulated by SLIM were an accurate reproduction of the currents in the CBG region. The hydrodynamic model was validated by comparing the outputs to drifter data from Mantovanelli et al. (2012) and depth averaged Acoustic Doppler Current Profiler (ADCP) data (averaged from below the depth affected by side lobe interference) from the Integrated Marine Observing System (IMOS) as follows. Mantovanelli et al. (2012) released 4 drifters to the south of Heron Island on 25 April 2010 and left them to drift for 20 days, until 15 May 2010. One of the drifters ran aground on the 03 May 2010 and was redeployed for a further 27 days, from 15 May 2010 to 11 June 2010. The winds present over the entire 47 day period (average velocity = 5.5 m s−1 ± 2.4 SD, prevailing direction = 160.8°) most closely matched the winds modeled in scenario 6 (SSE 2; average velocity = 6.3 m s−1 ± 3.0 SD, prevailing direction = 160.0°). Of all the seed locations used in the modeling, the location on the lee side of Lamont Reef was the closest to the initial release point of the drifters. One thousand passive larvae were seeded from Lamont Reef in scenario 6 A; the dispersal of the larval plume at the end of the 27 day run approximately matched the dispersal of the drifters (Figure 2A). Nearly half of the larvae remaining in the model domain at the end of the run were within the area bound by the paths the drifters traveled (448 of 939 remaining larvae, 47.7%). The northernmost distance a larva traveled from Lamont Reef approached the northernmost distance reached by a drifter. Further, the median longshore and cross shelf current velocities simulated in scenario 7, which was forced with wind from the 01 January 2015 to 25 January 2015, closely matched the median depth averaged current velocities measured at the Heron Island South and One Tree East moorings in the same period (Figure 2B). The simulated current ranges also approximately matched the measured ranges.
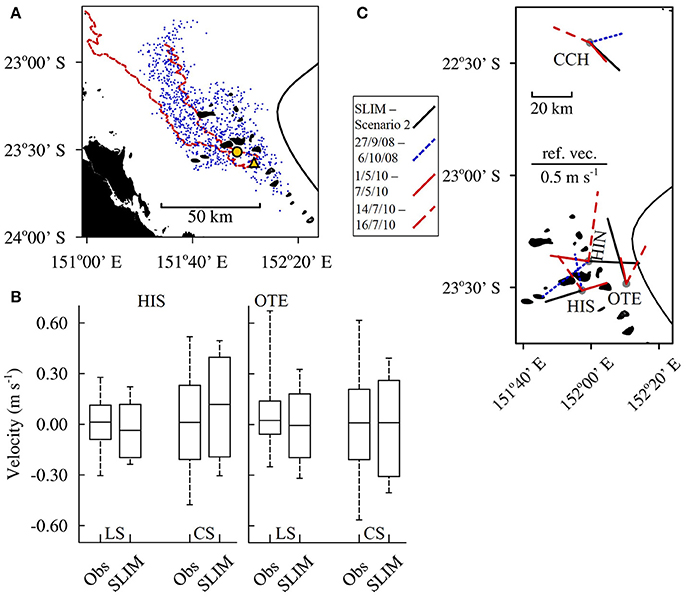
Figure 2. SLIM validation. (A) The maximum extents (red dashed line) traveled by the 4 drifters Mantovanelli et al. (2012) released south of Heron Island (yellow circle) over the 20–27 days they were left adrift. The paths are superimposed on top of a plume of passive larvae (blue dots) 27 days after they were seeded from Lamont reef (yellow triangle) in model scenario 6. (B) The LongShore (LS) and Cross Shelf (CS) currents that were simulated (SLIM) at mooring locations Heron Island South (HIS) and One Tree East (OTE) in scenario 7, which was forced with wind from 01 January 2015 to 25 January 2015. These data are compared to the depth averaged currents that were measured (obs) at the moorings over the same period. The locations of the moorings are shown in (C). (C) The currents simulated in scenario 2 (solid black line), where the Capricorn Eddy was modeled as present, in combination with wind of average strength (6.2 m s−1) from the prevailing direction (119.9°), compared to the depth averaged currents measured at moorings during identified eddy events (blue and red lines). The lengths of the lines indicate the mean current velocities and the line orientations indicate the prevailing directions the currents traveled away from the mooring locations (gray dots). Data from the Capricorn CHannel (CCH), Heron Island North (HIN), HIS and OTE moorings are shown.
Finally, to validate the simulation of the CE, we compared the currents simulated in scenario 2, which was forced with the CE and average wind, with ADCP measurements taken during identified eddy events. Weeks et al. (2010) identified an eddy event which lasted for a least 9 days, from 27 September 2008 to 6 October 2008. Mao and Luick (2014) identified additional eddy events; one lasted for at least 6 days, from 1 May 2010 to 7 May 2010, and a second lasted for at least 2 days, from 14 July 2010 to 16 July 2010. The average velocities simulated in SLIM at the mooring locations (0.25 m–0.45 m s−1; Figure 2C) were within the range of velocities measured during the identified eddy events (0.17–0.46 m s−1). The current directions predicted in SLIM aligned well with the directions measured at the Capricorn Channel and One Tree East moorings during the May 2010 eddy event. The current directions predicted at the Heron Island North and Heron Island South moorings did not align with any identified event; however, the current directions measured at these mooring varied greatly between events.
Results
The Long-Term Abundance of Lutjanus carpontatus at One Tree Island
The number of recruits observed within the lagoon at One Tree Island (OTI) varied greatly among years over the 23 year period, from 1995 to 2017 (Figure 3A). Either zero or very few Lutjanus carponotatus recruits were observed in edge or center habitats from 1995 to 2002 and from 2013 to 2015. After 2002, four peaks (sensu average > 0.3 recruits per 125 m2) in recruitment were recorded in the lagoon habitats. The greatest numbers of recruits were observed in 2011. The average abundance recorded in the lagoon habitats in this year was 1.7 times greater than the averages recorded for the next highest peaks in recruitment, which occurred in 2003 and 2007. Greater numbers of recruits were generally observed at the lagoon center habitat than the lagoon edge.
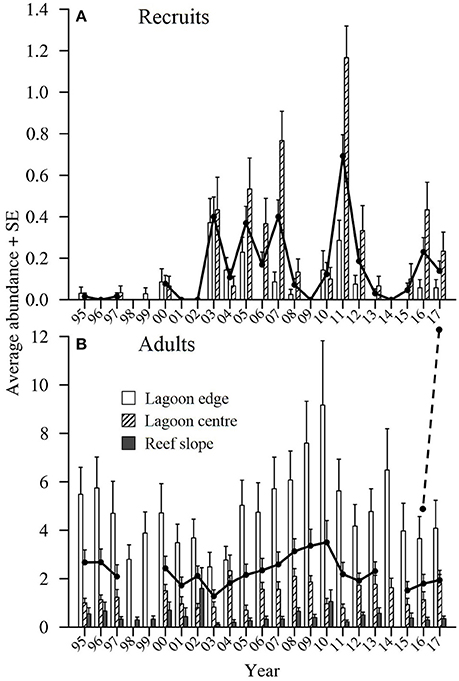
Figure 3. The average abundance of (A) Lutjanus carponotatus recruits (<150 mm standard length; SL) and (B) L. carponotatus adults (>200 mm SL) per 125 m2 ± SE recorded in lagoon center, lagoon edge and reef slope habitats at OTI in January/February of each year (six or seven sites per habitat and n = 5 transects). The black lines show the overall average abundance. The overall average recruit abundance does not include data from the reef slope habitat as no recruits were observed in this habitat. The overall averages have not been shown for years when all relevant habitats were not sampled. A one-year time lag was applied when the abundance of adults was correlated with the abundance of recruits, as indicated by the dashed line.
The average abundance of L. carponotatus adults recorded in the lagoon was relatively high in all years, although the numbers observed fluctuated among years (Figure 3B). The average abundance among all habitats peaked at 3.50 adults per 125 m2 ± 0.9 SE (used as the measure of variance thereafter unless otherwise specified) in 2010. Adult abundance increased from 2004 to 2010, after sustained recruitment from 2003 to 2007. It also increased from 2012 to 2014 following the largest recruitment peak in 2011. The abundance of adults was relatively stable from 2015 to 2017, following a recruitment failure in 2014 and moderate to low recruitment thereafter. The average abundance of recruits did not correlate with the average abundance of adults (used as a proxy for spawner biomass) recorded in the previous year [Spearman's rank correlation, rs = −0.13, z(2) = −0.55, p > 0.05, n = 18].
Wind Conditions Present During the Larval Period
Wind in the CBG region typically blew from the ESE (117.8° from true north) with an average velocity of 6.1 m s−1 ± 2.6 SD (Table 2). When the recruits from the 2004/2005 cohort were in the plankton as larvae, the wind predominantly blew longshore, from the SSE (155.0°) at a near average velocity (6.9 m s−1 ± 0.7 SD; Figures 4A,B). At the end of the recruits Pelagic Larval Duration's (PLD's), the cumulative sum of the wind's cross shelf component neared zero as the component alternated in direction, blowing from the ENE (42.4% ± 7.7 SD) and the WSW (57.6% ± 7.7 SD). These sums were much more balanced (cross shelf cumulative sum ratio closer to 50:50 ENE:WSW) than in an average 25 day period (78.6% ± 21.5 SD from the ENE and 21.4% ± 21.5 SD from the WSW). Winds with cross shelf components as or more balanced than the observed SSE winds occurred on 72 PLD length intervals ±7 in a year on average. Winds with balanced cross shelf components were most common in May, June, and July (Figure 5), when the recruits from the 2004/2005 cohort were spawned.
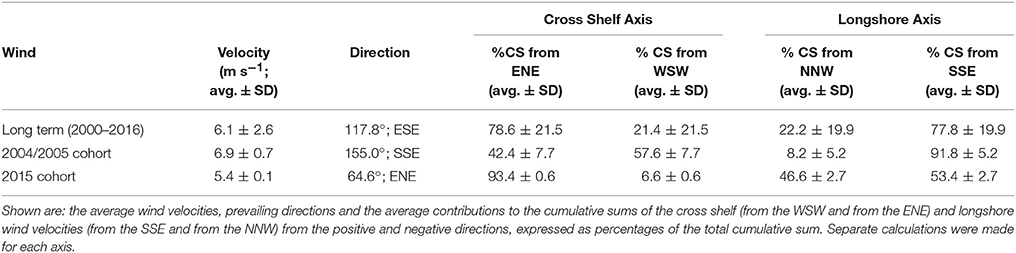
Table 2. Wind metrics for: Row (1) historic, successive 25 day (average Pelagic Larval Duration length) intervals from 1 January 2000 to 31 December 2016 (long term), Row (2) the winds present during the planktonic phases of the recruits from the 2004/2005 cohort, Row (3) the winds present during the planktonic phases of the potential settlers from the 2015 cohort.
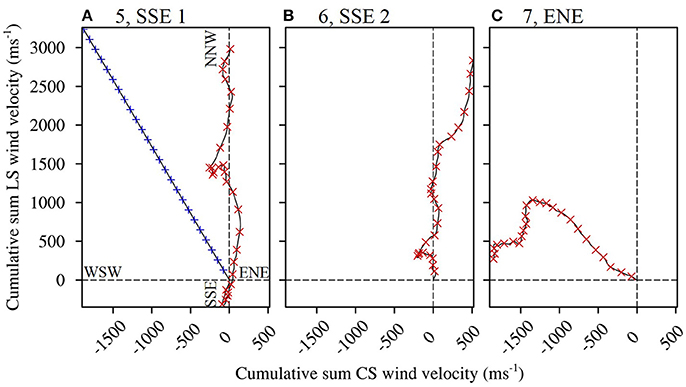
Figure 4. Progressive vector diagrams of the winds present during the planktonic larval phases of select fish caught from One Tree Island. Trajectories to the right of the Y axis indicate a net Cross Shelf (CS) velocity component from the WSW, and trajectories to the left of the axis indicate a net ENE component. Trajectories above the X axis indicate a net LongShore (LS) velocity component from the SSE, and trajectories below the axis indicate a net NNW component. The winds were forced in the modeling scenarios as follows. (A) Scenario 5, LS winds predominantly from the SSE with the most balanced cross shelf (CS) component (red cross symbols). Scenarios 1 and 2, with wind of average speed (6.2 m s−1) from the prevailing direction (from 119.9°; blue plus symbols). (B) Scenario 6, LS winds predominantly from the SSE with the least balanced CS component (a greater WSW component). (C) Scenario 7, CS winds predominantly from the ENE with a balanced LS component.
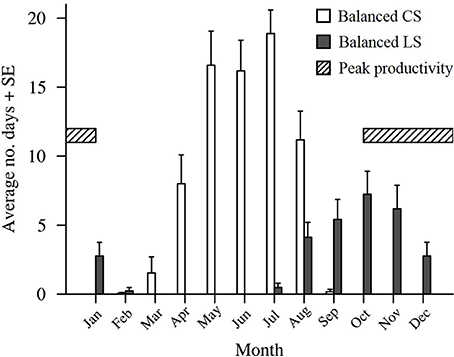
Figure 5. The average number of historic 25 day (average Pelagic Larval Duration length) intervals starting in each month (2000–2016) that had winds with LongShore (LS) and Cross Shelf (CS) components as or more balanced than the winds present during the planktonic larval phases of the fish caught from One Tree Island. The striped section indicates the period of peak Lutjanus carponotatus larval production.
The wind predominantly blew across the shelf, from the ENE (64.6°), at below average velocity (5.4 m s−1 ± 0.1 SD), when the pre-settlement fish from the 2015 cohort were in the plankton as larvae (Figure 4C). The cumulative sum of the wind's longshore component neared zero over the period when the fish were in the plankton, as the component alternated in direction, blowing from the NNW (46.6% ± 2.7 SD) and the SSE (53.4% ± 2.7). These sums were much more balanced (longshore cumulative sum ratio closer to 50:50 NNW:SSE transport) than in an average 25 day period (22.2% ± 19.9 from the NNW and 77.8% ± 19.9 from the SSE). Winds with longshore components as or more balanced than the observed ENE winds were rarer than balanced cross shelf winds, occurring on only 29 PLD length intervals ± 4 in a year on average. They were most common from August to January, when winds with balanced cross shelf components were largely absent (Figure 5). This period includes the months when the production of L. carponotatus larvae is likely greatest, from October to January. The prevalence of winds with a balanced longshore component during this period of peak production was positively correlated with the abundance of recruits observed in the following year [Spearman's rank correlation, rs = 0.51, z(2) = 1.97, p < 0.05, n = 15; Figure 6].
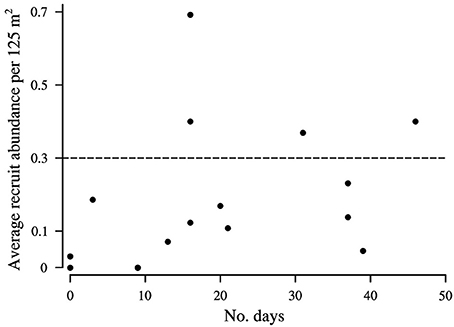
Figure 6. The average number of Lutjanus Carponotatus recruits observed at OTI in the lagoon habitats per 125 m2 (2002–2017) in relation to the number of historic 25 day (average Pelagic Larval Duration length) intervals with a balanced longshore component present in the period of peak L. carponotatus larval production (2000/2001 to 2015/2016). Recruitment peaks occurred where the average abundance exceeded 0.3 recruits per 125 m2, as marked by the dashed line. The SE's of the average abundances are shown in Figure 3.
Retention Under Modeled Conditions
The wind conditions present during the pelagic phases of the L. carponotatus caught at OTI in both the 2004/2005 and the 2015 cohorts drove currents that minimized the expatriation of larvae from the Capricorn Bunker Group (CBG). The SSE winds present during the pelagic phases of the recruits from the 2004/2005 cohort partly advected the plume of passive larvae longshore, to the NNW, but induced little net cross shelf transport (scenarios 5A and 6A; Figures 7E,F). The plume, therefore, remained concentrated in the CBG for the duration of the 27 day run; the residence time of the CBG in these scenarios (22.9 days ± < 0.1 to 24.8 days ± 0.1; Figure 8C, Table 1) approached the average PLD of the L. carponotatus recruits from this study of 25 days ± 0.6. Analogously, the plume of passive larvae was partly advected across the shelf, to the WSW, by the ENE winds present during the pelagic phases of the potential settlers from the 2015 cohort (scenario 7A; Figure 7G). These winds induced little longshore transport and so the plume remained concentrated in the CBG; the residence time of the CBG in this scenario (>27 days; Figure 8C) exceeded the average L. carponotatus PLD. The plume of passive larvae also remained relatively concentrated in the CBG under winds of average strength from the prevailing ESE direction and no Capricorn Eddy (CE; scenario 1A; Figure 7A). The plume was partly advected to the WNW, and the residence time in this scenario (18.3 days ± 0.2; Figure 8A) was at least 4 days shorter than the times recorded under the SSE and ENE winds real larvae had survived. Strong wind conditions in the prevailing direction quickly advected passive larvae to the NW, far away from the CBG (scenarios 3A and 4A; Figures 7C,D). The CE also reduced retention in the CBG by expatriating large numbers of passive larvae eastward into oceanic waters over the shelf slope. This was consistent when the CE was modeled in combination with average winds (scenario 2A; Figure 7B) and with strong winds (scenario 4A; Figure 7D). Accordingly, the residence times of the CBG recorded in these scenarios (5.7 days ± < 0.1 to 12.0 days ± 0.1; Figures 8A,B) were days to weeks shorter than the residence times recorded under the survivor specific wind conditions, or under average winds and no CE.
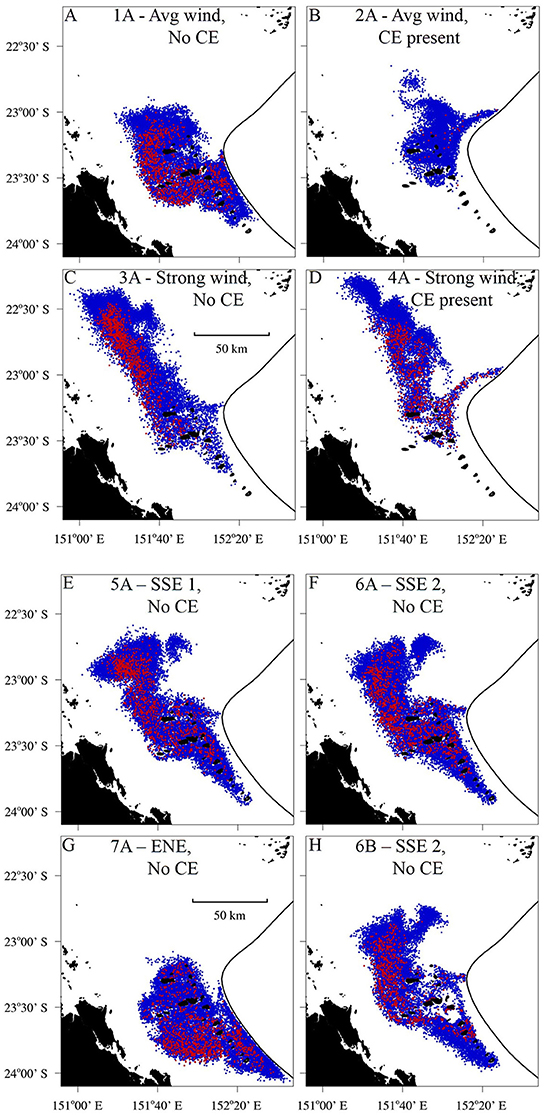
Figure 7. The dispersion of the larval plume at the end of the Pelagic Larval Duration (PLD), 25 days after the larvae were seeded. The black line denotes the open boundary of the model which corresponds to the 200 m isobath. The red particles were seeded from One Tree Island (OTI) and the blue particles were seeded from other reefs in the Capricorn Bunker Group (Figure 1C). (A–D) Constant wind scenarios. (A) Wind of average strength (6.2 m s−1) from the prevailing direction (119.9°), no Capricorn Eddy (CE) and passive larvae. (B) Average wind from the prevailing direction, the CE present and passive larvae. (C) Strong wind (1.5 times average) from the prevailing direction, no CE and passive larvae. (D) Strong wind from the prevailing direction, the CE present and passive larvae. (E–G) Alternating wind scenarios. (E) The LongShore SSE winds with the most balanced cross shelf (CS) component, no CE and passive larvae. (F) The LS SSE winds with the least balanced CS component (i.e., a stronger WSW component), no CE and passive larvae. (G) The CS winds with a balanced LS component, no CE and passive larvae. (H) The LS SSE winds with the least balanced CS component, no CE and swimming larvae.
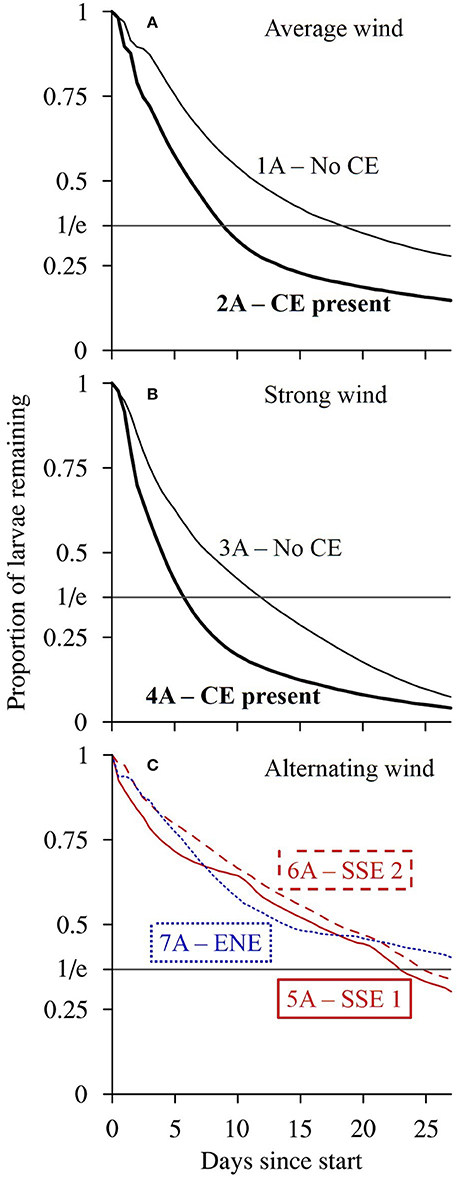
Figure 8. The modeled proportion of passive larvae (A scenarios) seeded from reefs in the Capricorn Bunker Group (CBG, Figure 1C) remaining in the CBG through time up to the end of the 27-day model runs. The scenario numbers are shown. (A) Winds of average strength (6.2 m s−1) from the prevailing direction (119.9°), with (bold) and without the Capricorn Eddy (CE). (B) Strong winds (1.5 times average) from the prevailing direction with (bold) and without the CE. (C) The LongShore (LS) SSE winds with balanced Cross Shelf (CS) components (red; most balanced = solid, least balanced = dashed) and the CS ENE winds with a balanced LS component (blue dotted) present during the planktonic larval phases of fish caught from OTI. The residence time is calculated as the time taken for the proportion remaining to fall to 1/e ~ 0.37.
The impact of larval swimming behavior on the dispersal of the larval plume, and the corresponding residence time of the CBG, depended on the model scenario. In the scenarios where the plume of passive larvae remained relatively concentrated in the CBG (scenarios 1A, 5A, 6A, and 7A), the inclusion of swimming behavior increased the residence time by several days (Table 1). For example, when the model was forced with the longshore SSE winds that had the least balanced cross shelf component, and no CE (scenarios 6A and 6B), the inclusion of swimming behavior reduced the NNW movement of the larval plume (Figures 7F,H) and increased the residence time of larvae in the CBG from 24.8 days ± 0.1 to >27 days. Swimming behavior had little effect on the residence time when the model was forced with strong winds or the CE, which both advected the larval plume away from the CBG. For example, when the CE was modeled in combination with average winds (scenarios 2A and 2B), the residence time did not measurably increase from 8.9 days ± < 0.1 when swimming behavior was included.
At a smaller spatial scale, when the larvae were set to be passive (A scenarios), the highest numbers of potential settlers were recorded around OTI in the scenarios forced with the real winds survived by L. carponotatus during their pelagic phase's (scenarios 5A, 6A and 7A; # settlers rank 1P to 3P; 157.3 settlers ± 4.6–215.7 settlers ± 11.3; Figure 9A, Table 1). Slightly lesser numbers were recorded under average winds and no CE (scenario 1A; # settlers rank 4P; 136.7 settlers ± 8.4). Only around one third of the number of potential settlers recorded in the survivor specific conditions were counted in the model scenarios that included strong winds and/or the CE (scenarios 2A, 3A, and 4A; # settlers rank 5P to 7P; 36.3 settlers ± 0.9 to 56.3 settlers ± 3.3).
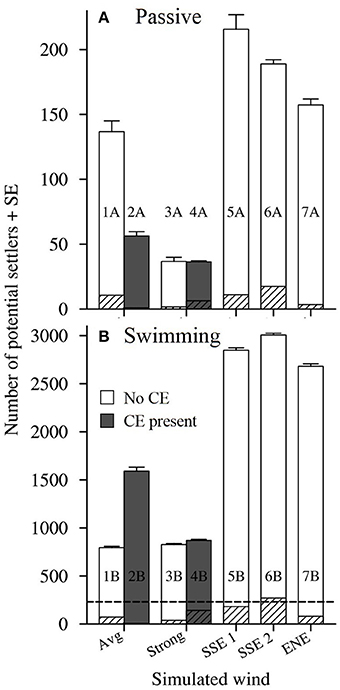
Figure 9. The number of potential settlers seeded from reefs in the Capricorn Bunker Group (Figure 1C) that were present around One Tree Island at the end of the average Pelagic Larval Duration (PLD; 25 days ± 0.6 SE). (A) Passive larvae, A scenarios. (B) Swimming larvae, B scenarios. Runs with the Capricorn Eddy (CE) are shown in gray and runs without the CE are shown in white. The striped sections indicate the number of settlers counted that originated from OTI. The panes have different Y-axes, with the maximum extent of the Y-axis in A shown by the dotted line in (B).
When swimming behavior was included (B scenarios), order of magnitude increases in the number of potential settlers were predicted for all the modeled physical conditions (Figure 9B). For example, in scenario 5, which was forced with longshore winds from the SSE, the number of potential settlers increased by 13 times, from 215.7 settlers ± 11.3–2848.0 settlers ± 25.5 when larval swimming was included. The greatest numbers of settlers were again counted in the scenarios forced with the real winds survived by L. carponotatus from OTI during their pelagic phase (scenarios 5B, 6B, and 7B; # settlers rank 1S to 3S; 2681.0 settlers ± 25.4 to 3006.0 settlers ± 19.2). In contrast to the results obtained for passive larvae, the next highest numbers of settlers were counted in the scenarios that included strong winds and/or the CE (scenarios 2B, 3B and 4B; # settlers rank 4S to 6S; 826.3 settlers ± 9.8–1589.0 settlers ± 42.6), which had one third to one half of the numbers of settlers recorded under the survivor specific wind conditions. Among the scenarios that included swimming, the lowest number of potential settlers were recorded in scenario 1B, which was forced with average winds and no CE (# settlers rank 7S; 793.3 settlers ± 15.2).
Additionally, including swimming behavior reduced the larval mortality from expatriation. The percentage of the original 1,000 larvae seeded from OTI that were present around OTI at the end of the PLD (% returned) increased when swimming behavior was included, and was substantial in some scenarios (Table 1). For example, the % return increased from 1.7% ± 0.2 under the longshore SSE winds with the least balanced cross shelf component, no CE and passive larvae (scenario 6A) to 26.9% ± 0.6 when the same oceanographic forcings were used, but the larvae were made to swim (scenario 6B).
Modeled Self-Seeding of One Tree Island
There was no clear pattern relating the Self-Seeding rate (% SS) at OTI to the modeled oceanographic conditions. For example, when the model was forced with winds of average strength from the prevailing direction and no CE, and larvae were made to swim (scenario 1B), the % SS was 9.0 % ± 0.7 (Table 1). When the CE was modeled as present, with the same wind and larval behavior (scenario 2B), the % SS decreased by an order of magnitude to 0.4 % ± 0.1, the lowest modeled % SS. Contrastingly, the inclusion of the CE increased the % SS that was projected with swimming larvae under strong winds, from 4.4% ± 0.4 (scenario 3B) to 16.2 % ± 0.6 (scenario 4B). The highest modeled % SS was 17.3% ± 2.0, and it was recorded in scenario 4A, with strong winds from the prevailing direction, the CE present and passive larvae. The inclusion of swimming behavior had little effect on the % SS in all scenarios. For example, the % SS only differed by 1.2% in the scenarios with and without swimming, when the model was forced with the longshore SSE winds with the most balanced cross shelf component, and no CE (scenarios 5A and 5B).
Discussion
Lutjanus carponotatus Population Dynamics
The abundance of adult Lutjanus carponotatus at One Tree Island (OTI) remained relatively high throughout the 23 years of sampling and did not vary as much as the abundance of recruits. Our observations of increases in adult abundance following recruitment events suggested that the size of the population of L. carponotatus at OTI was determined by the cumulation of a few “stored” strong pulses of recruitment. The recruitment limitation of populations can occur, where the size of an adult population varies with the level of recruitment, assuming post settlement mortality is density independent (Doherty, 1983; Victor, 1983, 1986). Our findings align with those of Kingsford (2009) who investigated the age structure of the L. carponotatus population at OTI in the years after the 2003 recruitment pulse, which broke an 8-year lull in recruitment. The cohort that recruited in 2003 made up a large proportion (40–50%) of the total population in the 2 years following the pulse (Kingsford, 2009).
The lack of a correlation between the abundance of adult L. carponotatus at OTI and the abundance of the recruits that they could have spawned suggests that, at the scale of OTI, there was a decoupling between spawner biomass and recruitment. Numerous factors can act between spawning and recruitment, obscuring the relationship between the biomass of spawners and the magnitude of recruitment (Jennings et al., 2001). An overview of some of the factors that can affect the abundance of recruits is provided in the next section of the discussion. The population of adult L. carponotatus at OTI may only make up a fraction of the total population of adults contributing offspring to the island, hindering our ability to identify a spawner-recruit relationship (Jennings et al., 2001). This idea is supported by the low levels of self-seeding projected for OTI as, if the potential for self-seeding is limited, most of the fish recruiting to OTI would have been spawned from other reefs. The self-seeding rate was calculated to be less than 17% among all the modeled scenarios. Comparatively, the percentage of self-seeding has been estimated to be as high as 60% in other reef fish populations (Jones et al., 1999; Almany et al., 2007). The projected low levels of self-seeding at OTI are probably related to the geography of the Capricorn Bunker Group (CBG). The potential for self-seeding in reef matrices has been linked to the “sticky water” effect, whereby the net currents within a matrix are reduced through the deflection of currents around reefs, facilitating the retention of larvae (Andutta et al., 2012). The strength of the effect is dependent on the density of the reef matrix (Andutta et al., 2012). The capacity for self-seeding is therefore limited at more isolated reefs like those in the CBG, including OTI (Wolanski and Kingsford, 2014).
The rate of self-seeding of L. carponotatus at OTI presented here was estimated using biophysical modeling. We used a two-dimensional, depth average model and restricted the geographic extent of the seed locations to the CBG. All models are subject to potential error and, while these choices were necessary and justified (see methods), they could have affected the modeled patterns of connectivity. Two dimensional models can only incorporate two-dimensional behaviors so our model could not include larval vertical migration, which can enhance retention near natal reefs (Paris and Cowen, 2004). Although Great Barrier Reef (GBR) shelf waters are generally well mixed (Luick et al., 2007), L. carponotatus larvae may perform vertical migrations. Accordingly, the self-seeding percentage we projected would probably have been higher if our behavioral model had included vertical migration. Further, our assumption that recruits to OTI could only come from reefs in the CBG would have also affected the projected self-seeding rate. In justifying this assumption, we argued that a majority of L. carponotatus recruits to OTI probably come from within the CBG based on: (1) the well documented reduction in dispersal kernels (the potential for successful dispersal connections) with distance from natal reefs (Jones, 2015; Williamson et al., 2016) and (2) the results of a genetic parentage analysis of two coral trout species, Plectropomus maculatus and Plectropomus leopardus, in the southern GBR (Williamson et al., 2016). Multidirectional larval dispersal was recorded in the genetic parentage analysis and some coral trout recruits originating from the Keppel Islands and the Percy Island Group, ~100 and ~250 km from the CBG respectively, successfully dispersed to reefs in the CBG. It is, therefore, possible that some L. carponotatus larvae make the same journey. An additional source of larvae to the CBG could be the Swain Reefs ~150 km away. With the method applied in this study, considering sources of recruits from outside of the CBG would have reduced the self-seeding rate projected for OTI. As equal numbers of larvae were released from all seed locations, the proportion of the total pool of larvae originating from OTI would have been reduced. Ideally, the numbers of larvae released would have been scaled to reflect the different reproductive capacities of the local adult populations inhabiting the different reefs, but the data required to make this adjustment were not available. The sources of L. carponotatus recruits to OTI could be identified more definitively through genetic parentage analyses (e.g., Williamson et al., 2016) or mark, recapture experiments, where the otoliths of larvae are tagged with maternally transmitted stable isotopes (e.g., Almany et al., 2007).
The Recruitment of Lutjanus carponotatus to One Tree Island
There was considerable inter-annual variability in the abundance of L. carponotatus recruits recorded at OTI. In some years there was total recruitment failure, where no recruits were observed. While variability in recruitment is common in coral reef ecosystems, the virtual absence of recruits seems unusually extreme (Doherty, 2002; DeMartini et al., 2013), especially since lutjanid larvae are typically robust and strong swimming (Leis, 2006) and some self-recruitment would be expected (Jones, 2015). We found that the abundance of recruits varied with the prevalence of survivor specific favorable winds during the period of peak production, when the recruits were most likely to be in their larval stage. Biophysical modeling suggested that the identified winds drove currents that kept the larval plume concentrated in the CBG, reducing larval mortality through expatriation. The larval period is the only stage in the lifecycle of L. carponotatus where the wind-driven current could feasibly have a direct effect on mortality because L. carponotatus are strong swimming and are reef-associated after settlement. The significance of the correlation between recruitment and favorable winds suggests that variability in recruitment was influenced by physical forcing during the larval stage. Physical forcings that affect larval mortality have previously been linked to the recruitment of commercially important species, where recruitment into the fishery occurs years after the larvae are spawned (e.g., Caputi et al., 1996; Wilderbuer et al., 2013). However, robust empirical models where physical conditions are good predictors of recruitment are rare and scarcely used in fisheries management (Skern-Mauritzen et al., 2016). There are exceptions, such as the use of an upwelling index in the models predicting the recruitment of Anchovy, Engraulis encrasicholus, to the Bay of Biscay in the northeast Atlantic Ocean (Skern-Mauritzen et al., 2016). Here we have provided a model to predict when the recruitment of L. carponotatus to OTI will be good or bad; similar empirical models may have broader applicability in the CBG, and in other reef complexes in the GBR. As ocean monitoring technologies improve, enhancing our ability to forecast biological indices, scientists and end-users must communicate effectively to utilize such empirical models to their full potential (Payne et al., 2017).
The correlation we present here only considers the effects of larval mortality on recruit abundance, specifically by expatriation from wind driven currents. The significance of the relationship suggests that larval mortality by expatriation is an important determinant of the recruitment of L. carponotatus to OTI. However, there was considerable unexplained variability in the relationship which was probably attributable to other reproductive and pre and post settlement processes known to influence recruitment. Further, the implications of the assumptions made in the biophysical modeling were discussed previously; assumptions were similarly made in the empirical modeling which would have contributed to the unexplained variability in the correlation.
The influence of variability in reproduction was not accounted for in our correlation. Firstly, variability in the reproductive capacity of adults can affect the magnitude of recruitment. The larval supply can be a good predictor of recruitment (Milicich et al., 1992), and it can be affected by fluctuations in spawner biomass (Jennings et al., 2001). We presented data showing that consistently high numbers of L. carponotatus adults were present at OTI over the 23-year sampling period; however, there were no data available for the numbers of L. carponotatus adults at other reefs in the CBG or the surrounding region. The magnitude of fluctuations in the biomass of the stock of L. carponotatus contributing offspring to OTI is therefore unknown. Changes in fecundity can also affect the reproductive capacity of a population. The fecundity of an individual can depend on the availability of resources, where the abundance/scarcity of resources determines their allocation toward reproduction (Duponchelle et al., 2000). Upwelling can boost productivity in coral reefs which are generally located in oligotrophic surface waters (Wolanski and Delesalle, 1995). Increased productivity could have a trickle up affect whereby an increase in primary production boosts secondary production, increasing the abundance of the prey species targeted by the predatory L. carponotatus (Jennings et al., 2001). Multiple sources of upwelling have been identified in the southern GBR, including strong northerly winds and the Capricorn Eddy (CE; Weeks et al., 2010; Mao and Luick, 2014). Finally, when we correlated the prevalence of favorable winds with recruitment, we only considered winds, and therefore spawning, in the period of peak L. carponotatus production (October to January; Russell et al., 1977; Kritzer, 2004). Some L. carponotatus clearly spawn outside of these months. Kritzer (2004) found that at least 50% of the ovaries from L. carponotatus females in the Palm and Lizard Island groups were ripe for 7 months of the year from September to February and recruits from this study were spawned in May. Variability in the magnitude and timing of spawning can cause large fluctuations in the supply of larvae within and among spawning seasons (Milicich et al., 1992). Such fluctuations can be more pronounced at the southern extremes of the distributions of species (e.g., Fowler, 1991), and the CBG is at the southern end of the range of L. carponotatus. Given the expected irregularity in larval production, misalignments could have occurred where favorable winds were present when larval production was low and vice versa. Such misalignments would weaken the relationship between recruitment and the prevalence of favorable winds.
While retention under favorable winds was considered in the presented correlation, numerous other pre-settlement processes can affect the magnitude of recruitment. Larval mortality can affect larval supply and, subsequently, recruitment (Milicich et al., 1992). There are many sources of larval mortality including starvation (e.g., Cushing, 1975, 1990), predation (e.g., Moller, 1984; McGurk, 1986) and expatriation (e.g., Sponaugle and Pinkard, 2004). Starvation can be a major source of mortality in fish larvae (Leggett and Deblois, 1994). As with the adults, the upwelling of nutrient rich water can increase the availability of species that larvae prey on, and improve the nutritional content of prey items (Jennings et al., 2001). The larval mortality rate of L. carponotatus larvae in the southern GBR could be reduced if their spawning coincides with an upwelling event, as proposed in the match mismatch hypothesis (Cushing, 1990). In this study, we defined “favorable winds” as balanced; if the northerly winds linked to upwelling in the southern GBR formed part of a balanced wind cycle, they could have been included in the correlation through being categorized as “favourable.” In this way, differential mortality from starvation could have indirectly influenced the presented correlation. Further, predation pressure on the eggs and larvae of marine species can be intense (Purcell, 1985; Bailey and Houde, 1989), and can vary with physical conditions (e.g., Frank and Leggett, 1982). It is possible that inter-annual variability in the rate of predation on L. carponotatus eggs and larvae influenced recruitment to OTI but there are no data available to examine this possibility. Finally, while the impact of larval retention under favorable winds was examined in the presented correlation, the impact of retention/expatriation in other scenarios was not considered. Larvae can be retained in the CBG under non-favorable wind conditions, as demonstrated with the biophysical model. Settlers arriving under these alternate wind conditions would degrade the presented relationship between the prevalence of survivor specific winds and recruitment. Additionally, the correlation we presented does not incorporate data on the influences of other oceanographic forces, such as the CE and the North Caledonian Jet (NCJ). The CE can greatly affect the currents in the CBG (present study; Mao and Luick, 2014) and the NCJ is a principal driver of the currents in the GBR system (Andrews and Clegg, 1989; Wolanski, 1994). Inter-annual variability in the prevalence and strength of the CE, and the strength of the NCJ could therefore greatly influence larval mortality through expatriation, independent of the influence of favorable winds. Further, we did not examine the potential for these and other dispersive forces to bring recruits from reefs outside of the CBG to OTI. Post settlement mortality (Hixon, 2015) can also affect recruitment patterns. A 13–16 month gap between peak larval production and recruitment was incorporated into the model, meaning recruits could have been settled for up to 15 months before recruitment was measured. Given this lag, and the substantial mortality newly settled fish can experience (Sale and Ferrell, 1988), the decoupling of a settlement signal from the later measure of recruitment is plausible. Variation in habitat quality (Jones et al., 2004), predation (Hixon, 2015), and density dependant mortality (Schmitt and Holbrook, 1999) can all affect the survivorship of recruits.
The Wind and Recruitment
Wind patterns had a great influence on the modeled larval dispersal trajectories and, therefore, probably greatly affected the recruitment of L. carponotatus to OTI. The impact of the wind was unsurprising given the CBG lies on a shallow section of the Australian continental shelf, and winds can be the principal driver of currents in shallow waters (e.g., Pattrick et al., 2013). Indeed, Mao and Luick (2014) found strong correlations between the winds and the surface currents in the CBG region. In this study we found that fish that had successfully arrived at OTI survived either longshore SSE winds with a balanced cross shelf component, or cross shelf ENE winds with a balanced longshore component. Both conditions induced insubstantial, unidirectional movement in the larval plume, keeping it concentrated in the CBG. Consequently, the scenarios with these winds had the greatest recruitment potential. Other studies have similarly found that wind induced oscillatory flow patterns can concentrate plumes of fish larvae, facilitating recruitment (Basterretxea et al., 2013). Further, the winds identified to be favorable for recruitment in this study were highly seasonal. The seasonality of favorable recruitment conditions has been documented in multiple environments, from coral reefs in the Philippines (Abesamis and Russ, 2010; Abesamis et al., 2017) to coastal fisheries in the NW Mediterranean (Basterretxea et al., 2012).
The larval supply of lutjanids to OTI over the course of 1 month has previously been linked to periods (8/9 days) of offshore winds advecting larvae to the island from the NW (Kingsford and Finn, 1997). These winds could have been part of an alternating longshore wind cycle, like the cycle that occurred when the L. carponotatus from the 2015 cohort were in the plankton. The conclusions of Kingsford and Finn (1997) reinforce the importance of wind to recruitment in the CBG region, but suggest that there may be a greater variety of favorable wind scenarios than were identified in this study. Logically, the transportation of larvae to OTI from the NW would be favorable for recruitment if larval production was high at reefs NW of OTI. The direction of larval transport would change over an extended spawning season, such as that of L. carponotatus, due to the seasonality of the winds in the region. Further research into the spatial and temporal variability in L. carponotatus reproductive output, in relation to the seasonality of the wind, may refine our understanding of recruitment variability.
Relatedly, “balanced winds,” as defined in this paper, could arise from numerous scenarios where there are alternations in the strength and duration of the longshore and cross shelf winds. Each distinct scenario would have a unique influence on larval transportation and survival. For example, the dispersive forces acting on a larval plume could be reduced during a wind relaxation (Abesamis and Russ, 2010; Cuif et al., 2014). Conversely, the waves generated by strong winds could increase mixing and induce a current shear (Simpson and Dickey, 1981). A more detailed knowledge of the composition of “balanced winds” could be gained through analyzing the variability in the wind. Time series analysis techniques, such as spectral analysis (e.g., Taggart and Leggett, 1987), could be used to decompose the variability in the wind. Alternatively, future research could look at the frequency of calm periods or extreme events, analogous to the approach of Peterman and Bradford (1987) who found that the frequency of low wind speed periods (Lasker events) during the northern anchovy (Engraulis mordax) spawning season was correlated with the larval mortality rate.
The Capricorn Eddy
The CE was detrimental for recruitment in most scenarios as it advected large numbers of larvae eastward from the CBG into oceanic waters. Sponaugle et al. (2005) found that the arrival of multi-taxa larval pulses to reefs in the Florida Keys coincided with the passage of sub mesoscale eddies with diameters of 10 to 40 km, produced from the decay of larger mesoscale eddies 100–200 km in diameter. The little researched CE appears to have a diameter of approximately 100 km when present (Weeks et al., 2010; Mao and Luick, 2014). The CE may retain the larvae that it advects from reefs in the CBG, and from the Swain Reefs to the north of the CBG. However, the timely transport of these larvae back to reefal waters within the 22 to 28 day PLD of L. carponotatus is unlikely given the size of the CE in relation to the sub-mesoscale eddies linked to favorable recruitment in the Florida Keys. As mentioned previously, the CE can be a source of upwelling in the southern GBR (Weeks et al., 2010; Mao and Luick, 2014). Consequently, larval mortality from starvation may be reduced when the CE is present, and the settlers that arrive at OTI may be in better condition than those that were in the plankton when the CE was absent. In this way, the positive ecological effects of the CE could counteract its negative dispersive effects. Research into the characteristics of the CE is required to better understand its ecological impact on the southern GBR.
The Importance of Larval Swimming Behavior
The inclusion of swimming behavior caused order of magnitude increases in the number of potential settlers present around OTI at the end of the PLD. Larval mortality from expatriation was reduced when swimming was included, as indicated by the modeled increase in the return rate. Wolanski and Kingsford (2014) also calculated the percentage of modeled passive and swimming larvae seeded from OTI that had returned to OTI by the end of a PLD. They sequentially varied the physical and biological parameterization of a biophysical model and similarly found that the inclusion of behavior improved larval survival. The projected rate of return ranged from 0.6 to 5.9% when the larvae were modeled as passive. When they were programmed to swim the upper limit of the modeled rate of return increased significantly, with the projected rates ranging from 0.4 to 28.8%. Many studies have similarly found that the inclusion of behavior in models vastly improved the predicted larval retention and subsequent recruitment (e.g., Paris et al., 2007; Butler et al., 2011; Basterretxea et al., 2013). In addition to improving the overall projected recruitment, when larvae were made to swim, they counteracted the adverse dispersive forces of strong winds and the CE. Consequently, the relative favorability of the modeled oceanographic conditions changed so equivalent or greater numbers of potential settlers were recorded in the scenarios with strong winds and/or the CE, compared to the scenario with average winds and no CE. Such fundamental changes in results have been recorded in other biophysical modeling studies following the inclusion of behavior. For example, Paris et al. (2007) found that the inclusion of diel vertical migration behavior and larval mortality into a biophysical model of the western Caribbean changed the simulated connectivity in the region. The present study supports the consensus that the inclusion of behavior in biophysical models is critical if the organism being modeled can influence its dispersion (Wolanski, 2017). This has been shown true for a range of species, from fishes and corals with short PLD's (e.g., Wolanski and Kingsford, 2014) to crustaceans with long PLD's (e.g., Butler et al., 2011) and jellyfishes with adult pelagic phases (e.g., Fossette et al., 2015; Schlaefer et al., 2018).
Conclusions
The recruitment of L. carponotatus to OTI was highly variable over the 23-year study period. The gains in adult abundance that followed sustained recruitment suggested that the size of the adult population was largely determined through variation in recruitment. The potential for L. carponotatus larvae from the CBG to recruit to OTI was greatest in the currents driven by the winds that were present during the pelagic phases of the fish we collected at OTI. There was a significant positive relationship between the prevalence of these wind conditions during the period of peak L. carponotatus larval production and the measured recruitment in the following year. The prevalence of the identified winds could therefore be used as a proxy to predict recruitment. Larvae arrived at OTI in comparably smaller numbers in the modeling scenarios forced with either average or strong winds, and with or without the CE. This study highlighted the substantial impact that the inclusion of larval swimming behavior can have on the predicted recruitment, in accordance with other biophysical modeling studies. We worked at a fine temporal resolution, identifying and modeling the specific pelagic conditions survived by fish that had successfully arrived at OTI. The favorable winds may not have been identified if the investigation was conducted at a coarser temporal resolution, demonstrating the efficacy of studying the conditions experienced by survivors.
Ethics Statement
This study was carried out in accordance with the recommendations of James Cook Universities policies, procedures and guidelines, Animal Ethics Committee at James Cook University. The protocol was approved by the Animal Ethics Committee at James Cook University.
Author Contributions
JS, EW, and MK: Study conception and design; JS, EW, and MK: Data collection and analysis; JS, EW, and JL: Biophysical modeling; JS: Drafting the manuscript; JS, EW, JL, and MK: Revising the manuscript.
Funding
This research was supported by multiple grants awarded to MK from the Australian Research Council (ARC), including funding from the ARC Centre of Excellence for Coral Reef Studies.
Conflict of Interest Statement
The authors declare that the research was conducted in the absence of any commercial or financial relationships that could be construed as a potential conflict of interest.
Acknowledgments
We thank the numerous people who assisted with the fish counts over the 23 years of data collection. We particularly thank Mark O'Callaghan, who prepared the otoliths for analysis in addition to assisting with the fish counts, and Kynan Hartog-Burnett who collected the potential settlers. The Acoustic Doppler Current Profiler (ADCP) data used to validate the SLIM simulations were sourced from the Integrated Marine Observing System (IMOS; http://www.imos.org.au) - IMOS is supported by the Australian Government through the National Collaborative Research Infrastructure Strategy and the Super Science Initiative.
References
Abesamis, R. A., and Russ, G. R. (2010). Patterns of recruitment of coral reef fishes in a monsoonal environment. Coral Reefs 29, 911–921. doi: 10.1007/s00338-010-0653-y
Abesamis, R. A., Saenz-Agudelo, P., Berumen, M. L., Bode, M., Jadloc, C. R. L., Solera, L. A., et al. (2017). Reef-fish larval dispersal patterns validate no-take marine reserve network connectivity that links human communities. Coral Reefs 36, 791–801. doi: 10.1007/s00338-017-1570-0
Almany, G. R., Berumen, M. L., Thorrold, S. R., Planes, S., and Jones, G. P. (2007). Local replenishment of coral reef fish populations in a marine reserve. Science 316, 742–744. doi: 10.1126/science.1140597
Andrews, J. C., and Clegg, S. (1989). Coral Sea circulation deduced from modal information models. Deep Sea Res. A Oceanogr. Res. Papers 36, 957–974. doi: 10.1016/0198-0149(89)90037-X
Andutta, F. P., Kingsford, M. J., and Wolanski, E. (2012). ‘Sticky water’ enables the retention of larvae in a reef mosaic. Estuarine Coast. Shelf Sci. 101, 54–63. doi: 10.1016/j.ecss.2012.02.013
Andutta, F. P., Ridd, P. V., and Wolanski, E. (2011). Dynamics of hypersaline coastal waters in the Great Barrier Reef. Estuarine Coast. Shelf Sci. 94, 299–305. doi: 10.1016/j.ecss.2011.06.009
Andutta, F. P., Ridd, P. V., and Wolanski, E. (2013). The age and the flushing time of the Great Barrier Reef waters. Cont. Shelf Res. 53, 11–19. doi: 10.1016/j.csr.2012.11.016
Bailey, K. M., and Houde, E. D. (1989). Predation on eggs and larvae of marine fishes and the recruitment problem. Adv. Mar. Biol. 25, 1–83. doi: 10.1016/S0065-2881(08)60187-X
Basterretxea, G., Catalan, I. A., Jordi, A., Alvarez, I., Palmer, M., and Sabates, A. (2013). Dynamic regulation of larval fish self-recruitment in a marine protected area. Fish. Oceanogr. 22, 477–495. doi: 10.1111/fog.12035
Basterretxea, G., Jordi, A., Catalan, I. A., and Sabates, A. (2012). Model-based assessment of local-scale fish larval connectivity in a network of marine protected areas. Fish. Oceanogr. 21, 291–306. doi: 10.1111/j.1365-2419.2012.00625.x
Beaman, R. J. (2010). “Project 3DGBR: A High-Resolution Depth Model for the Great Barrier Reef and Coral Sea. Marine and Tropical Sciences Research Facility (MTSRF) Project 2.5i.1a Final Report” (Cairns, Australia: MTSRF).
Berenshtein, I., Kiflawi, M., Shashar, N., Wieler, U., Agiv, H., and Paris, C. B. (2014). Polarized light sensitivity and orientation in coral reef fish post-larvae. PLoS ONE 9:e88468. doi: 10.1371/journal.pone.0088468
Black, K. P., Moran, P. J., and Hammond, L. S. (1991). Numerical-models show coral reefs can be self-seeding. Mar. Ecol. Prog. Ser. 74, 1–11. doi: 10.3354/meps074001
Bottesch, M., Gerlach, G., Halbach, M., Bally, A., Kingsford, M. J., and Mouritsen, H. (2016). A magnetic compass that might help coral reef fish larvae return to their natal reef. Curr. Biol. 26, R1266–R1267. doi: 10.1016/j.cub.2016.10.051
Burgess, S. C., Kingsford, M. J., and Black, K. P. (2007). Influence of tidal eddies and wind on the distribution of presettlement fishes around One Tree Island, Great Barrier Reef. Mar. Ecol. Prog. Ser. 341, 233–242. doi: 10.3354/meps341233
Buston, P. M., Jones, G. P., Planes, S., and Thorrold, S. R. (2012). Probability of successful larval dispersal declines fivefold over 1 km in a coral reef fish. Proc. R. Soc. B Biol. Sci. 279, 1883–1888. doi: 10.1098/rspb.2011.2041
Butler, M. J., Paris, C. B., Goldstein, J. S., Matsuda, H., and Cowen, R. K. (2011). Behavior constrains the dispersal of long-lived spiny lobster larvae. Mar. Ecol. Prog. Ser. 422, 223–237. doi: 10.3354/meps08878
Caputi, N., Fletcher, W. J., Pearce, A., and Chubb, C. F. (1996). Effect of the leeuwin current on the recruitment of fish and invertebrates along the Western Australian coast. Mar. Freshwater Res. 47, 147–155. doi: 10.1071/MF9960147
Choi, K. W., and Lee, J. H. W. (2004). Numerical determination of flushing time for stratified water bodies. J. Mar. Syst. 50, 263–281. doi: 10.1016/j.jmarsys.2004.04.005
Clarke, M. E., Domeier, M. L., and Laroche, W. A. (1997). Development of larvae and juveniles of the mutton snapper (Lutjanus analis), lane snapper (Lutjanus synagris) and yellowtail snapper (Lutjanus chrysurus). Bull. Mar. Sci. 61, 511–537.
Cowen, R. K., Lwiza, K. M. M., Sponaugle, S., Paris, C. B., and Olson, D. B. (2000). Connectivity of marine populations: open or closed? Science 287, 857–859. doi: 10.1126/science.287.5454.857
Cowen, R. K., Paris, C. B., and Srinivasan, A. (2006). Scaling of connectivity in marine populations. Science 311, 522–527. doi: 10.1126/science.1122039
Critchell, K., Grech, A., Schlaefer, J., Andutta, F. P., Lambrechts, J., Wolanski, E., et al. (2015). Modelling the fate of marine debris along a complex shoreline: lessons from the Great Barrier Reef. Estuarine Coast. Shelf Sci. 167, 414–426. doi: 10.1016/j.ecss.2015.10.018
Cuif, M., Kaplan, D. M., Lefevre, J., Faure, V. M., Caillaud, M., Verley, P., et al. (2014). Wind-induced variability in larval retention in a coral reef system: a biophysical modelling study in the South-West lagoon of New Caledonia. Prog. Oceanogr. 122, 105–115. doi: 10.1016/j.pocean.2013.12.006
Cushing, D. H. (1990). Plankton production and year-class strength in fish populations - an update of the match mismatch hypothesis. Adv. Mar. Biol. 26, 249–293. doi: 10.1016/S0065-2881(08)60202-3
Delandmeter, P., Lambrechts, J., Marmorino, G. O., Legat, V., Wolanski, E., Remacle, J. F., et al. (2017). Submesoscale tidal eddies in the wake of coral islands and reefs: satellite data and numerical modelling. Ocean Dyn. 67, 897–913. doi: 10.1007/s10236-017-1066-z
DeMartini, E. E., Wren, J. L. K., and Kobayashi, D. R. (2013). Persistent spatial patterns of recruitment in a guild of Hawaiian coral reef fishes. Mar. Ecol. Prog. Ser. 485, 165–179. doi: 10.3354/meps10306
Dixson, D. L., Jones, G. P., Munday, P. L., Planes, S., Pratchett, M. S., Srinivasan, M., et al. (2008). Coral reef fish smell leaves to find island homes. Proc. R. Soc. B Biol. Sci. 275, 2831–2839. doi: 10.1098/rspb.2008.0876
Doherty, P. J. (1983). Tropical territorial damselfishes - is density limited by aggression or recruitment. Ecology 64, 176–190. doi: 10.2307/1937339
Doherty, P. J. (1987). The replenishment of populations of coral-reef fishes, recruitment surveys, and the problems of variability manifest on multiple scales. Bull. Mar. Sci. 41, 411–422.
Doherty, P. J. (2002). “Variable replenishment and the dynamics of reef fish populations,” in Coral Reef Fishes: Dynamics and Diversity in a Complex Ecosystem, ed P. F. Sale (San Diego, CA: Elsevier Science).
Duponchelle, F., Cecchi, P., Corbin, D., Nunez, J., and Legendre, M. (2000). Variations in fecundity and egg size of female Nile tilapia, Oreochromis niloticus, from man-made lakes of Cote d'Ivoire. Environ. Biol. Fishes 57, 155–170. doi: 10.1023/A:1007575624937
Fisher, R. (2005). Swimming speeds of larval coral reef fishes: impacts on self-recruitment and dispersal. Mar. Ecol. Prog. Ser. 285, 223–232. doi: 10.3354/meps285223
Fisher, R., and Bellwood, D. R. (2002). The influence of swimming speed on sustained swimming performance of late-stage reef fish larvae. Mar. Biol. 140, 801–807. doi: 10.1007/s00227-001-0758-5
Fisher, R., Leis, J. M., Clark, D. L., and Wilson, S. K. (2005). Critical swimming speeds of late-stage coral reef fish larvae: variation within species, among species and between locations. Mar. Biol. 147, 1201–1212. doi: 10.1007/s00227-005-0001-x
Fisher, R., and Wilson, S. K. (2004). Maximum sustainable swimming speeds of late-stage larvae of nine species of reef fishes. J. Exp. Mar. Biol. Ecol. 312, 171–186. doi: 10.1016/j.jembe.2004.06.009
Fletcher, W. J. (1995). Application of the otolith weight - age relationship for the pilchard, sardinops-sagax neopilchardus. Can. J. Fish. Aquat. Sci. 52, 657–664. doi: 10.1139/f95-066
Fossette, S., Gleiss, A. C., Chalumeau, J., Bastian, T., Armstrong, C. D., Vandenabeele, S., et al. (2015). Current-oriented swimming by jellyfish and its role in bloom maintenance. Curr. Biol. 25, 342–347. doi: 10.1016/j.cub.2014.11.050
Fowler, A. J. (1991). Reproductive-biology of bisexual and all-female populations of Chaetodontid fishes from the southern Great-Barrier-Reef. Environ. Biol. Fishes 31, 261–274. doi: 10.1007/BF00000691
Frank, K. T., and Leggett, W. C. (1982). Coastal water mass replacement - its effect on zooplankton dynamics and the predator prey complex associated with larval capelin (mallotus-villosus). Can. J. Fisheries Aquat. Sci. 39, 991–1003. doi: 10.1139/f82-134
Ganachaud, A., Cravatte, S., Melet, A., Schiller, A., Holbrook, N. J., Sloyan, B. M., et al. (2014). The Southwest Pacific Ocean circulation and climate experiment (SPICE). J. Geophys. Res.Oceans 119, 7660–7686. doi: 10.1002/2013JC009678
Gerlach, G., Atema, J., Kingsford, M. J., Black, K. P., and Miller-Sims, V. (2007). Smelling home can prevent dispersal of reef fish larvae. Proc. Natl. Acad. Sci. U.S.A. 104, 858–863. doi: 10.1073/pnas.0606777104
Hare, J. A., Quinlan, J. A., Werner, F. E., Blanton, B. O., Govoni, J. J., Forward, R. B., et al. (1999). Larval transport during winter in the SABRE study area: results of a coupled vertical larval behaviour-three dimensional circulation model. Fish. Oceanogr. 8, 57–76. doi: 10.1046/j.1365-2419.1999.00017.x
Hixon, M. A. (2015). “Predation: Piscivory and the Ecology of Coral Reef Fishes,” in Ecology of Fishes on Coral Reefs, ed C. Mora (Cambridge: Cambridge University Press), 41–52.
Houde, E. D. (2008). Emerging from Hjort's Shadow. J. Northwest Atlantic Fish. Sci. 41, 53–70. doi: 10.2960/J.v41.m634
Jennings, S., Kaiser, M. J., and Reynolds, J. D. (2001). Marine Fisheries Ecology. Oxford and Northampton, Great Britain: Blackwell Science Ltd.
Jones, G. P. (2015). “Mission Impossible: Unlocking the Secrets of Coral Reef Fish Dispersal,” in Ecology of Fishes on Coral Reefs, ed C. Mora (Cambridge: Cambridge University Press), 16–27.
Jones, G. P., Mccormick, M. I., Srinivasan, M., and Eagle, J. V. (2004). Coral decline threatens fish biodiversity in marine reserves. Proc. Natl. Acad. Sci. U.S.A. 101, 8251–8253. doi: 10.1073/pnas.0401277101
Jones, G. P., Milicich, M. J., Emslie, M. J., and Lunow, C. (1999). Self-recruitment in a coral reef fish population. Nature 402, 802–804. doi: 10.1038/45538
Kingsford, M. J. (1998). “Reef fishes,” in Studying Temperate Marine Environments, eds M.J. Kingsford and C. Battershill (Christchurch, New Zealand: Canterbury University Press), 162–166.
Kingsford, M. J. (2009). Contrasting patterns of reef utilization and recruitment of coral trout (Plectropomus leopardus) and snapper (Lutjanus carponotatus) at One Tree Island, southern Great Barrier Reef. Coral Reefs 28, 251–264. doi: 10.1007/s00338-008-0421-4
Kingsford, M. J., and Finn, M. (1997). The influence of phase of the moon and physical processes on the input of presettlement fishes to coral reefs. J. Fish Biol. 51, 176–205. doi: 10.1111/j.1095-8649.1997.tb06099.x
Kingsford, M. J., Leis, J. M., Shanks, A., Lindeman, K. C., Morgan, S. G., and Pineda, J. (2002). Sensory environments, larval abilities and local self-recruitment. Bull. Mar. Sci. 70, 309–340.
Kritzer, J. P. (2004). Sex-specific growth and mortality, spawning season, and female maturation of the stripey bass (Lutjanus carponotatus) on the Great Barrier Reef. Fish. Bull. 102, 94–107.
Lambrechts, J., Hanert, E., Deleersnijder, E., Bernard, P.-E., Legat, V., Remacle, J.-F., et al. (2008). A multi-scale model of the hydrodynamics of the whole Great Barrier Reef. Estuarine Coast. Shelf Sci. 79, 143–151. doi: 10.1016/j.ecss.2008.03.016
Leggett, W. C., and Deblois, E. (1994). Recruitment in marine fishes: is it regulated by staravtion and predation in the egg and larval stage? Netherlands J. Sea Res. 32, 119–134. doi: 10.1016/0077-7579(94)90036-1
Leis, J. M. (2006). “Are Larvae of Demersal Fishes Plankton or Nekton?” in Advances in Marine Biology, eds A. J. Southward and D. W. Sims (London: Academic Press Ltd-Elsevier Science Ltd.), 57–141.
Leis, J. M., and Carson-Ewart, B. M. (2003). Orientation of pelagic larvae of coral-reef fishes in the ocean. Mar. Ecol. Prog. Ser. 252, 239–253. doi: 10.3354/meps252239
Lenanton, R. C., Joll, L., Penn, J., and Jones, K. (1991). The influence of the leeuwin current on coastal fisheries of Western Australia. J. R. Soc. West. Aust. 74, 101–114.
Leu, M. Y., and Liou, C. H. (2013). The larval development of the Russell's snapper, Lutjanus russellii (Teleostei: Lutjanidae) reared under laboratory conditions. J. Mar. Biol. Assoc. UK. 93, 1695–1701. doi: 10.1017/S0025315413000131
Luick, J. L., Mason, L., Hardy, T., and Furnas, M. J. (2007). Circulation in the Great Barrier Reef Lagoon using numerical tracers and in situ data. Cont. Shelf Res. 27, 757–778. doi: 10.1016/j.csr.2006.11.020
Mann, D. A., Casper, B. M., Boyle, K. S., and Tricas, T. C. (2007). On the attraction of larval fishes to reef sounds. Mar. Ecol. Prog. Ser. 338, 307–310. doi: 10.3354/meps338307
Mantovanelli, A., Heron, M. L., Heron, S. F., and Steinberg, C. R. (2012). Relative dispersion of surface drifters in a barrier reef region. J. Geophys. Res. Oceans 117, 15. doi: 10.1029/2012JC008106
Mao, Y., and Luick, J. L. (2014). Circulation in the southern Great Barrier Reef studied through an integration of multiple remote sensing and in situ measurements. J. Geophys. Res. Oceans 119, 1621–1643. doi: 10.1002/2013JC009397
McGurk, M. D. (1986). Natural mortality of marine pelagic fish eggs and larvae: role of spatial patchiness. Mar. Ecol. Prog. Ser. 34, 227–242. doi: 10.3354/meps034227
Melbourne-Thomas, J., Johnson, C. R., Alino, P. M., Geronimo, R. C., Villanoy, C. L., and Gurney, G. G. (2011). A multi-scale biophysical model to inform regional management of coral reefs in the western Philippines and South China Sea. Environ. Model. Softw. 26, 66–82. doi: 10.1016/j.envsoft.2010.03.033
Milicich, M. J., Meekan, M. G., and Doherty, P. (1992). Larval supply: a good predictor of recuitment of three species of reef fish (Pomacentridae). Mar. Ecol. Prog. Ser. 86, 153–166. doi: 10.3354/meps086153
Mitarai, S., Siegel, D. A., and Winters, K. B. (2008). A numerical study of stochastic larval settlement in the California current system. J. Mar. Syst. 69, 295–309. doi: 10.1016/j.jmarsys.2006.02.017
Moller, H. (1984). Reduction of a larval herring population by jellyfish predator. Science 224, 621–622. doi: 10.1126/science.224.4649.621
Mouritsen, H., Atema, J., Kingsford, M. J., and Gerlach, G. (2013). Sun compass orientation helps coral reef fish larvae return to their natal reef. PLoS ONE 8:e66039. doi: 10.1371/journal.pone.0066039
Newman, S. J., Williams, D. M., and Russ, G. R. (1996). Age validation, growth and mortality rates of the tropical snappers (Pisces: Lutjanidae) Lutjanus adetii (Castelnau, 1873) and L. quinquelineatus (Bloch, 1790) from the central Great Barrier Reef, Australia. Mar. Freshwater Res. 47, 575–584. doi: 10.1071/MF9960575
Paris, C. B., Atema, J., Irisson, J. O., Kingsford, M., Gerlach, G., and Guigand, C. M. (2013). Reef odor: a wake up call for navigation in Reef Fish Larvae. PLoS ONE 8:e72808. doi: 10.1371/journal.pone.0072808
Paris, C. B., Cherubin, L. M., and Cowen, R. K. (2007). Surfing, spinning, or diving from reef to reef: effects on population connectivity. Mar. Ecol. Prog. Ser. 347, 285–300. doi: 10.3354/meps06985
Paris, C. B., and Cowen, R. K. (2004). Direct evidence of a biophysical retention mechanism for coral reef fish larvae. Limnol. Oceanogr. 49, 1964–1979. doi: 10.4319/lo.2004.49.6.1964
Pattrick, P., Strydom, N. A., and Goschen, W. S. (2013). Shallow-water, nearshore current dynamics in Algoa Bay, South Africa, with notes on the implications for larval fish dispersal. Afr. J. Mar. Sci. 35, 269–282. doi: 10.2989/1814232X.2013.798593
Payne, M. R., Hobday, A. J., Mackenzie, B. R., Tommasi, D., Dempsey, D. P., Fässler, S. M. M., et al. (2017). Lessons from the First Generation of Marine Ecological Forecast Products. Front. Mar. Sci. 4:289. doi: 10.3389/fmars.2017.00289
Peterman, R. M., and Bradford, M. J. (1987). Wind-speed and mortality-rate of a marine fish, the northern anchovy (Engraulis mordax). Science 235, 354–356. doi: 10.1126/science.235.4786.354
Prandle, D. (1984). A modeling study of the mixing of 137Cs in the seas of the European continental shelf. Philos. Trans. R. Soc. Lond. Series A 310, 407–436. doi: 10.1098/rsta.1984.0002
Purcell, J. E. (1985). Predation on fish eggs and larvae by pelagic cnidarians and ctenophores. Bull. Mar. Sci. 37, 739–755.
Radford, C. A., Tindle, C. T., Montgomery, J. C., and Jeffs, A. G. (2011). Modelling a reef as an extended sound source increases the predicted range at which reef noise may be heard by fish larvae. Mar. Ecol. Prog. Ser. 438, 167–174. doi: 10.3354/meps09312
Russell, B. C., Anderson, G. R. V., and Talbot, F. H. (1977). Seasonality and recruitment of coral-reef fishes. Austr. J. Mar. Freshwater Res. 28, 521–528. doi: 10.1071/MF9770521
Sale, P. F., and Ferrell, D. J. (1988). Early survivorship of juvenile coral reef fishes. Coral Reefs 7, 117–124. doi: 10.1007/BF00300971
Schlaefer, J. A., Wolanski, E., and Kingsford, M. J. (2018). Swimming behavior can maintain localised jellyfish (Chironex fleckeri, Cubozoa) populations. Mar. Ecol. Progr. Ser. 591, 287–302. doi: 10.3354/meps12305
Schmitt, R. J., and Holbrook, S. J. (1999). Mortality of juvenile damselfish: implications for assessing processes that determine abundance. Ecology 80, 35–50. doi: 10.1890/0012-9658(1999)080[0035:MOJDIF]2.0.CO;2
Siegel, D. A., Kinlan, B. P., Gaylord, B., and Gaines, S. D. (2003). Lagrangian descriptions of marine larval dispersion. Mar. Ecol. Prog. Ser. 260, 83–96. doi: 10.3354/meps260083
Siegel, D. A., Mitarai, S., Costello, C. J., Gaines, S. D., Kendall, B. E., Warner, R. R., et al. (2008). The stochastic nature of larval connectivity among nearshore marine populations. Proc. Natl. Acad. Sci. U.S.A. 105, 8974–8979. doi: 10.1073/pnas.0802544105
Simpson, J. J., and Dickey, T. D. (1981). The relationship between downward irradiance and upper ocean structure. J. Phys. Oceanogr. 11, 309–323. doi: 10.1175/1520-0485(1981)011<0309:TRBDIA>2.0.CO;2
Simpson, S. D., Piercy, J. J. B., King, J., and Codling, E. A. (2013). Modelling larval dispersal and behaviour of coral reef fishes. Ecol. Complex. 16, 68–76. doi: 10.1016/j.ecocom.2013.08.001
Sinclair, M. (1988). Marine Populations: An Essay on Population Regulation and Speciation. Seattle, WA: University of Washington Press.
Skern-Mauritzen, M., Ottersen, G., Handegard, N. O., Huse, G., Dingsor, G. E., Stenseth, N. C., et al. (2016). Ecosystem processes are rarely included in tactical fisheries management. Fish Fish. 17, 165–175. doi: 10.1111/faf.12111
Spagnol, S., Wolanski, E., Deleersnijder, E., Brinkman, R., Mcallister, F., Cushman-Roisin, B., et al. (2002). An error frequently made in the evaluation of advective transport in two-dimensional Lagrangian models of advection-diffusion in coral reef waters. Mar. Ecol. Prog. Ser. 235, 299–302. doi: 10.3354/meps235299
Sponaugle, S., Lee, T., Kourafalou, V., and Pinkard, D. (2005). Florida current frontal eddies and the settlement of coral reef fishes. Limnol. Oceanogr. 50, 1033–1048. doi: 10.4319/lo.2005.50.4.1033
Sponaugle, S., and Pinkard, D. (2004). Lunar cyclic population replenishment of a coral reef fish: shifting patterns following oceanic events. Mar. Ecol. Prog. Ser. 267, 267–280. doi: 10.3354/meps267267
Taggart, C. T., and Leggett, W. C. (1987). Wind-forced hydrodynamics and their interaction with larval fish and plankton abundance - a time-series analysis of physical biological data. Can. J. Fish. Aquat. Sci. 44, 438–451. doi: 10.1139/f87-052
Tolimieri, N., Sale, P. F., Nemeth, R. S., and Gestring, K. B. (1998). Replenishment of populations of Caribbean reef fishes: are spatial patterns of recruitment consistent through time? J. Exp. Mar. Biol. Ecol. 230, 55–71. doi: 10.1016/S0022-0981(98)00083-5
Valles, H., Hunte, W., and Kramer, D. L. (2009). Variable temporal relationships between environment and recruitment in coral reef fishes. Mar. Ecol. Prog. Ser. 379, 225–240. doi: 10.3354/meps07886
Victor, B. C. (1983). Recruitment and population-dynamics of a coral-reef fish. Science 219, 419–420. doi: 10.1126/science.219.4583.419
Victor, B. C. (1986). Larval settlement and juvenile mortality in a recruitment-limited coral-reef fish population. Ecol. Monogr. 56, 145–160. doi: 10.2307/1942506
Webster, M. A., and Petkovic, P. (2005). Australian Bathymetry and Topography Grid June 2005. Record 2005/12. Geoscience Australia.
Weeks, S. J., Bakun, A., Steinberg, C. R., Brinkman, R., and Hoegh-Guldberg, O. (2010). The Capricorn Eddy: a prominent driver of the ecology and future of the southern Great Barrier Reef. Coral Reefs 29, 975–985. doi: 10.1007/s00338-010-0644-z
Wilderbuer, T., Stockhausen, W., and Bond, N. (2013). Updated analysis of flatfish recruitment response to climate variability and ocean conditions in the Eastern Bering Sea. Deep Sea Res. Part II Topical Stud. Oceanogr. 94, 157–164. doi: 10.1016/j.dsr2.2013.03.021
Wildermann, N., Critchell, K., Fuentes, M., Limpus, C. J., Wolanski, E., and Hamann, M. (2017). Does behaviour affect the dispersal of flatback post-hatchlings in the Great Barrier Reef? R. Soc. Open Sci. 4, 15. doi: 10.1098/rsos.170164
Williamson, D. H., Harrison, H. B., Almany, G. R., Berumen, M. L., Bode, M., Bonin, M. C., et al. (2016). Large-scale, multidirectional larval connectivity among coral reef fish populations in the Great Barrier Reef Marine Park. Mol. Ecol. 25, 6039–6054. doi: 10.1111/mec.13908
Wolanski, E. (1994). Physical Oceanographic Processes of the Great Barrier Reef . (Boca Raton, FL: CRC Press).
Wolanski, E. (2017). Bounded and unbounded boundaries – Untangling mechanisms for estuarine-marine ecological connectivity: Scales of m to 10,000 km – A review. Estuarine, Coast. Shelf Sci. 198, 378–392. doi: 10.1016/j.ecss.2016.06.022
Wolanski, E., Asaeda, T., Tanaka, A., and Deleersnijder, E. (1996). Three-dimensional island wakes in the field, laboratory and numerical models. Cont. Shelf Res. 16, 1437–1452. doi: 10.1016/0278-4343(95)00087-9
Wolanski, E., and Delesalle, B. (1995). Upwelling by internal waves, Tahiti, French-Polynesia. Cont. Shelf Res. 15, 357–368. doi: 10.1016/0278-4343(93)E0004-R
Wolanski, E., and Kingsford, M. J. (2014). Oceanographic and behavioural assumptions in models of the fate of coral and coral reef fish larvae. J. R. Soc. Interface 11:20140209. doi: 10.1098/rsif.2014.0209
Wolanski, E., Lambrechts, J., Thomas, C., and Deleersnijder, E. (2013). The net water circulation through Torres strait. Cont. Shelf. Res. 64, 66–74. doi: 10.1016/j.csr.2013.05.013
Keywords: biophysical model, recruitment, wind, SLIM, stripey snapper (Lutjanus carponotatus)
Citation: Schlaefer JA, Wolanski E, Lambrechts J and Kingsford MJ (2018) Wind Conditions on the Great Barrier Reef Influenced the Recruitment of Snapper (Lutjanus carponotatus). Front. Mar. Sci. 5:193. doi: 10.3389/fmars.2018.00193
Received: 29 November 2017; Accepted: 14 May 2018;
Published: 31 May 2018.
Edited by:
Michael Arthur St. John, National Institute of Aquatic Resources, Technical University of Denmark, DenmarkReviewed by:
Brian R. MacKenzie, Technical University of Denmark, DenmarkAngel Pérez-Ruzafa, Universidad de Murcia, Spain
Copyright © 2018 Schlaefer, Wolanski, Lambrechts and Kingsford. This is an open-access article distributed under the terms of the Creative Commons Attribution License (CC BY). The use, distribution or reproduction in other forums is permitted, provided the original author(s) and the copyright owner are credited and that the original publication in this journal is cited, in accordance with accepted academic practice. No use, distribution or reproduction is permitted which does not comply with these terms.
*Correspondence: Jodie A. Schlaefer, am9kaWUuc2NobGFlZmVyQG15LmpjdS5lZHUuYXU=