- 1Institute for Chemistry and Biology of the Marine Environment, University of Oldenburg, Wilhelmshaven, Germany
- 2Department for Marine Research, Senckenberg am Meer, Wilhelmshaven, Germany
- 3MARUM, University of Bremen, Bremen, Germany
The aim of the study is to compare spatial variation of macrofauna communities in the near- and offshore zone of the beach system of the island of Spiekeroog (German North Sea) in order to environmental parameters such as hydrodynamics and sediment type. The analysis of hydroacoustic backscatter signals have been used to classify the sea bottom characteristics in terms of surface roughness. Sampling was carried out in May 2014. Samples were taken along a 3.4 km transect in north-south direction. The analyses of the spatial distribution structure of the environmental parameters and the macrofauna communities revealed a clear zonation of the transect line into an inner, outer nearshore, and offshore zone. The inner nearshore was exposed to high hydrodynamic energy with a high variability in sediment composition, a lack of biogenic structures, lowest taxa numbers, but a considerably high diversity (Shannon Wiener index). The hydrodynamic conditions in the nearshore zone were more stable. Sediment distribution was homogenous. Taxa number and abundances increased and polychaete species such as Magelona johnstonii, Spiophanes bombyx, and Lanice conchilega characterized the community. In the offshore zone, taxa number and abundances increased even further. Lanice conchilega dominated the community. While current velocities of the bottom layers decreased, mud contents slightly increased.
Introduction
Sandy beach ecosystems constitute an important transition zone between continental and marine zones (Bergamino et al., 2011). They dominate most temperate and tropical coastlines (McLachlan, 1983; Dugan et al., 2007; Rodil et al., 2007), where they provide a wide range of key ecosystem services (Schlacher et al., 2008; Nel et al., 2014; Beck et al., 2017). Although studies on beach ecosystems have significantly increased over the last decades, biodiversity studies of their benthic macrofauna have received attention only quite recently. There is still a need for further information on the function of interactions between abiotic and biotic factors within these systems (Defeo and McLachlan, 2011; Nel et al., 2014).
Biotic communities are known to be structured by their physical environment, which is therefore defined as the “driving element” of these ecosystems (Defeo and McLachlan, 2005). Largely lacking any biogenic structures, sandy beach systems were often described as a function of variability in grain size composition, wave and tidal regime characteristics. Waves are transformed by shoaling, breaking, and swash. The influence of wave action reaches down to the sea bottom. Current direction and current velocities impact in different ways on the sea bottom profile (Nam et al., 2009; Tang et al., 2009; Short, 2012; Carter, 2013). Hydrodynamic parameters in combination with topographic features force beach systems into a range of different morphodynamic types (Brown and McLachlan, 1990; Short, 1996; Defeo and McLachlan, 2005; Defeo et al., 2009; Nel et al., 2014). These types range from microtidal reflective (narrow and steep) to macrotidal ultradissipative (wide and flat) extremes (Short, 1996; Schlacher et al., 2008; Nel et al., 2014). Most beaches are, however, in between those extremes and are defined as intermediate state (Defeo and McLachlan, 2005).
On large spatial scales, the relationship between the physical environment and the biodiversity of intertidal macrofauna communities reveals increasing species richness, abundance, and biomass from microtidal reflective beaches to macrotidal dissipative beaches as well as from temperate areas to the tropics (McLachlan, 1990; Brazeiro, 2001; Defeo and McLachlan, 2005, 2011; McLachlan and Dorvlo, 2005; Bergamino et al., 2011).
On a mesoscale the along and across-shore distribution of species differs, depending on short- (hourly, daily), or medium- (seasonal) term reactions to environmental conditions. Short- and medium-term reactions result in different zonation of the beach systems, which are highly dynamic and cannot be sharply defined (Defeo and McLachlan, 2005).
The morphology of the beaches can be determined into the backshore, foreshore, nearshore, and offshore zone following the nomenclature of Hallermeier (1980), Mangor (1998), Defeo and McLachlan (2011), and Short (2012). The most energy dominated zone is the nearshore, including the shoreface, and the breaker and surf zone, where waves dissipate most of their energy. Models imply high impact of hydrodynamic systems on the sediment transport. Sediments of the nearshore zone can be transported onshore, alongshore and offshore, and sand bars can also build up (Short, 2012; Carter, 2013).
There are several hypotheses trying to explain the distribution of macrofauna species within the different zones of sandy beach systems (reviewed by Defeo and McLachlan, 2005). One well supported is the Autecological Hypothesis adapted by McLachlan (1990) to the context of beach ecology. It states that in physically controlled environments, communities are structured by the independent responses of individual species to the physical environment with biological interactions being minimal (McLachlan and Dorvlo, 2005). Biological interactions become more important by decreasing “harshness of the environment” (Defeo and McLachlan, 2005; McLachlan and Dorvlo, 2005). Macrofauna species show some key adaptions, which provide the ability to survive in such environments. Primarily mobility, burrowing ability, rhythmic (e.g., tidal, circadian, lunar, seasonal) behavior, orientation mechanisms, and flexibility to cope with rapidly changing conditions account for the prevalence of species within these systems (Schlacher et al., 2008).
Being squeezed between rising sea levels from the marine side and expanding human populations on the landward side, beaches are under threat worldwide (Dugan et al., 2007; Schlacher et al., 2007, 2008; Defeo et al., 2009). Therefore, it is of major importance to gain widespread knowledge on the functional role of these ecosystems.
Between Den Helder and the Jade Bay in the southern part of the North Sea, the West, and East Frisian Wadden Seas forms a classical barrier island chain (Backhaus, 1943), which protects the mainland coastline from the direct influence of erosion by waves and storm events of the open sea (Hild et al., 1999).
Our study focused on the near- and offshore extension of a cross-shore transect starting in the dunes of the back barrier island of Spiekeroog (Beck et al., 2017) studying key processes in high-energy beach systems in an interdisciplinary approach. We aimed to (1) analyse spatial patterns in the abiotic and biotic environment, and to (2) test whether spatial patterns of environmental parameters and macrofauna communities relate to the near- and offshore zonation of a common sandy beach system.
Methods
Study Site
The beach system is located at the northern side of the back barrier island of Spiekeroog. The nearshore and offshore zone of Spiekeroog is exposed to the hydrodynamics of the open sea and strongly affected by tides, winds and wave driven processes (Badewien et al., 2009; Lettmann et al., 2009; Grashorn et al., 2015; Holinde et al., 2015; Schulz et al., 2015). Recent modeling studies suggest that density gradients are among the major drivers of long-term coastal dynamics (Burchard et al., 2008; Burchard and Badewien, 2015). With an average tidal range of 2.7 m at its western end and 2.9 m in the front of the mainland, Spiekeroog represents a standard mesotidal system (Hild et al., 1999). Characteristic are shoreface connected ridges in front of the island coastline (Markert et al., 2015). Those ridges running from southwest to northeast in water depths from 4 to 8 m and from southeast to northwest in water depths of 9 to 20 m. Inflowing high tide waters (flood), as well as outflowing low tide waters (ebb) are guessed to pass these reefs alongshore and offshore. The effects of current and wave driven processes result in different seasonal influences on the system (Dobrynin et al., 2010; Grashorn et al., 2015). During sampling time in May 2014, the beach system was in a seasonal transitional stage and can, thus, be classified as intermediate stage (McLachlan et al., 1996; Short, 1996; Defeo and McLachlan, 2005). The study site at the near and offshore zone of Spiekeroog island included 17 sampling stations, which are located along a 3.4 km long transect line perpendicular to the shore line (Figure 1). The distance between the sampling stations was 0.2 km. The topography of the sampled transect shows a smooth increase in depth with no remarkable structures along the transect line. The beach slope is 0.3°.
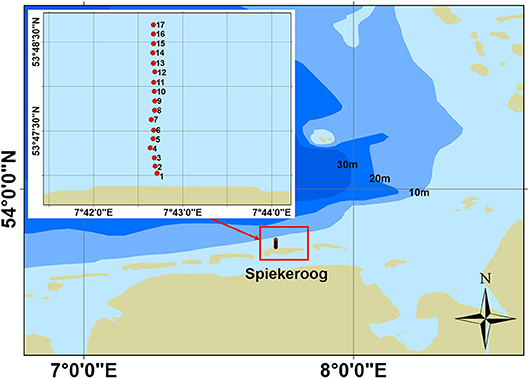
Figure 1. Location of the study site and the sampling stations on the 3.4 km long transect at the northern side of the island of Spiekeroog. The distance between the sampling stations was 0.2 km. Depth constantly increased with distance to the shore line.
All measurements were conducted at calm weather conditions during the 21st to the 23rd May 2014 and obtained with the RB “Otzum” and the RV “SENCKENBERG.” Continuous hydroacoustic and current measurements were conducted on 13 north-south and 7 west-east orientated transects. These transects included the transect, where samples for macrofauna and sediment analyses were taken (Station 1–17). At the starting and end point of each transects, a profiling CTD was used for determining water temperature, salinity and depth.
Macrofauna Sampling and Sample Procedure
At each of the 17 sampling stations (Figure 1), three replicate samples were taken with a 0.1 m2 van Veen grab. Samples were sieved through a 0.5 mm mesh. The fauna was fixed with 4 % buffered formaldehyde. Organisms were identified to the lowest possible taxonomic level.
Hydrodynamic Measurements
CTD–Conductivity, Temperature, Depth–Probe
Vertical profile measurements of water temperature, conductivity and pressure were conducted with a CTD48M (Sea&Sun Technology, Germany) nearshore (water depths between 1 and 5 m) and further offshore (water depths between 9 and 10 m) within the studied transect. Salinity was calculated based on the CTD measurements using the practical salinity scale (1978). Only profiles with more than 25 data points and of depths > 0.7 m were used.
ADCP–Acoustic Doppler Current Profiler
Current velocities in three dimensions were measured with an Acoustic Doppler Current Profiler (ADCP, Workhorse Sentinel, 1,200 kHz, Teledyne RD Instruments, USA) with a high temporal (~0.7 s) and vertical (0.25 m) resolution. The ADCP was mounted into the moon pool of the research vessel Otzum at a depth of 0.8 m below sea level. Measurements were done on 13 north-south orientated transects covering the transect sampled for marcofauna.
Vertical profiles of magnitude velocity at the 17 sampling stations were smoothed by a margin of two over depth and averaged over the measurement period. Therefore, the resulting profiles are averaged flow profiles at each position at the time of the measurement. Trajectories of the current velocity at the 17 sampling stations of the transect line were generated chronologically for the upper and lower part of the water column (each averaged with 3 depth cells). To generate the vectors the north- and east-components of the current velocities were used. The values used for further statistical analyses were directly generated from the current profiles and the velocity trajectories.
Sediment Sampling and Sample Procedure
At each of the 17 stations, sediment samples were sampled from the 0.1 m2 van Veen grabs taken for macrofauna identification. After a photographic documentation of the sediment surface and a macroscopic description, subsamples of the sediment surface (the upper 2 cm) were taken from the grabs for grain size analyses.
In the laboratory, the sediment samples were desalted by means of fresh water drainage over 24 h at semipermeable hoses. To separate the mud fraction (< 0.063 mm), the desalted sample was separated using a 0.063 mm mesh screen. The coarse fraction was then dried and dry-sieved over a 2 mm mesh screen to separate gravel and shell debris (coarser 2 mm). To determine the content of shell debris in the sand fraction (>0.063 to < 2 mm), differences of the weight content were registered before and after the elimination of carbonats using hydrochloric acid. The weight content of the shell debris of both grain size fractions (gravel and sand) were simply summed up to get the total amount of shell debris content of the entire samples. The sand fraction was analyzed in a settling tube by means of hydraulic grain size calculation on the base settling velocities measurements by a Macro-GranometerTM (Flemming and Thum, 1978; Brezina, 1979). Following the Wentworth (1922) grain size scale the higher resolved hydraulic grainsize distribution were normalized to 0.25 phi intervals in order to the mechanical sieved coarse fractions.
Acoustic Seafloor Classification and Subbottom Profiling
A parametric echosounder coupled with a dual-frequency SideScanSonar system (SES2000 standard plus INNOMARTMRostock) was used to survey the 13 north-south orientated and 7 west-east orientated transects covering the transect sampled for macrofauna (Figure 2). It was surveyed by means of the parametric echosounder and dual-frequency SideScanSonar system SES2000 standard plus (INNOMARTM Rostock). SES2000 sub bottom profiler was running at a primary frequency of ~100 kHz, secondary frequency was 8 to 10 kHz. The SideScanSonar system recorded data on the base of 250 kHz. A swath range of 75 m was selected. Chirp was modulated by linear frequency mode (LFM).
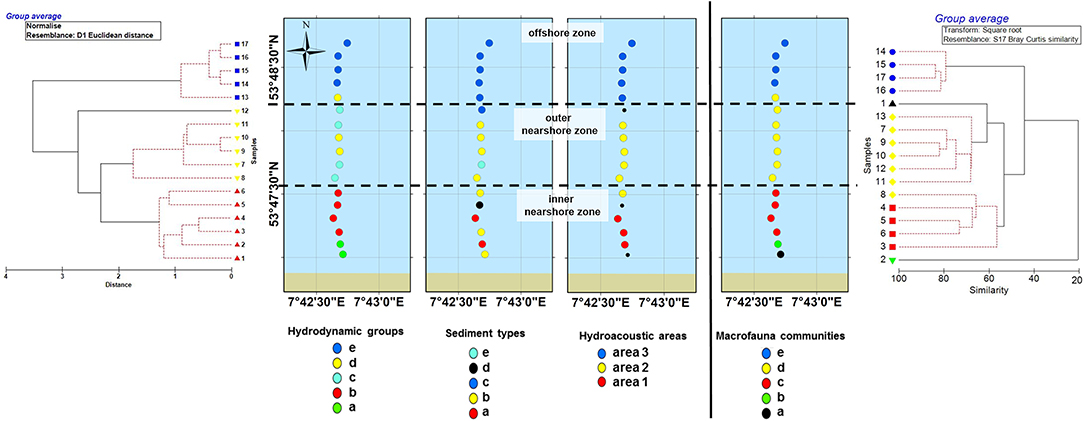
Figure 2. Hierarchical cluster analysis of the environmental parameters (Left), including distance and macrofauna communities (Right). Lines show the subdivision into three different zones. The first zone included stations 1 to 6, the second stations 7 to 12, and the third stations 13 to 17, see also Table 1.
Bathymetry was extracted of the bottom track of the generated SES vertical profiles. High resolution positioning was done with Trimble852 Real Time Kinematic system, with an accuracy of < 5 cm in all 3 directions. The data was further processed and georeferenced by ArcGIS10.22013 Esri.
Statistical Analyses
In a first step, a hierarchical cluster analysis followed by a similarity profile test (SIMPROF; Clarke and Gorley, 2006) was conducted based on square root transformed species abundance data using the statistical PRIMER package, v6 (Clarke and Gorley, 2006) to identify macrofauna communities. Similarities were calculated using the Bray-Curtis coefficient. ANOSIM randomization test (Clarke and Gorley, 2006) was used to test significant spatial differences in the community structure. Discriminating species, that significantly (p < 0.05) separated the different communities, were identified using the similarity percentage routine (SIMPER). The evenness (J') and the Shannon Wiener Index (H') were calculated using the DIVERSE tool.
Also spatial patterns for hydrodynamic, sediment and hydroacoustic parameters of the beach system were analyzed separately by a hierarchical cluster analysis followed by a similarity profile test (SIMPROF; Clarke and Gorley, 2006) based on Euclidean-distance-similarity of normalized environmental data sets of the sediment and the hydrodynamic parameters. For the spatial seafloor characterization, classified acoustic backscatter data and ground truth information of visual and grab data were compiled.
In a second step, the relationships between the macrofauna communities and all environmental parameters combined were analyzed by a joint hierarchical cluster analysis followed by a similarity profile test (SIMPROF; Clarke and Gorley, 2006) based on Euclidean-distance-similarity and performed on the environmental groups. Distance from the shore line was included as categorized variable. Data were further processed and visualized by ArcGIS10.22013 Esri.
Based on this analysis, a spatial zonation of the beach system could be defined. This previous zonation was improved by a principal component ordination analysis (PCO) performed on Euclidean-distance-similarity of environmental parameters using the PERMANOVA+ add on PRIMER v6 (Anderson et al., 2008). The most important environmental parameters were chosen according to Pearson's correlation on the PCO.
Results
Macrofauna Communities
A total of 85 taxa were identified. Thirty six taxa were polychaetes, 29 crustaceans, 14 bivalves, 3 echinoderms, and 3 represented other taxonomic groups. Polychaetes were by far the most abundant taxonomic group constituting 90.3% of the total abundance of recorded species, followed by bivalves (5.2%), echinoderms (2.8%) and crustaceans (1.7%).
The multivariate cluster analysis with square root transformed abundance data, based on SIMPROF (p < 0.05), revealed three clusters significantly separated (ANOSIM 0.85; p < 0.0001) and two stations, which were not allocated to a cluster (Figure 3, Table 1). The two non-allocated stations were stations 1 and 2 on the transect line located near to the shoreline. The three clusters and two stations represented five statistically significant different macrofauna communities according to different abundances of the characterizing species Lanice conchilega, Magelona johnstoni, and Spiophanes bombyx.
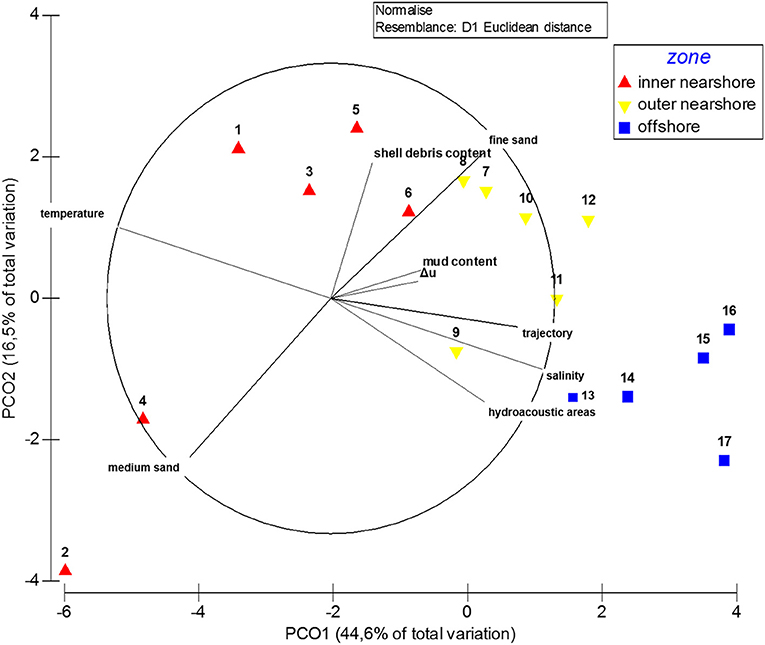
Figure 3. PCO revealing relationship of the environmental parameters to the sampling stations and the defined zones. Stations represent the positions of the zones within the ordination space. Vector lines represent the relationship of significant environmental parameters to the ordination axes; their length is proportional to their relative significance.
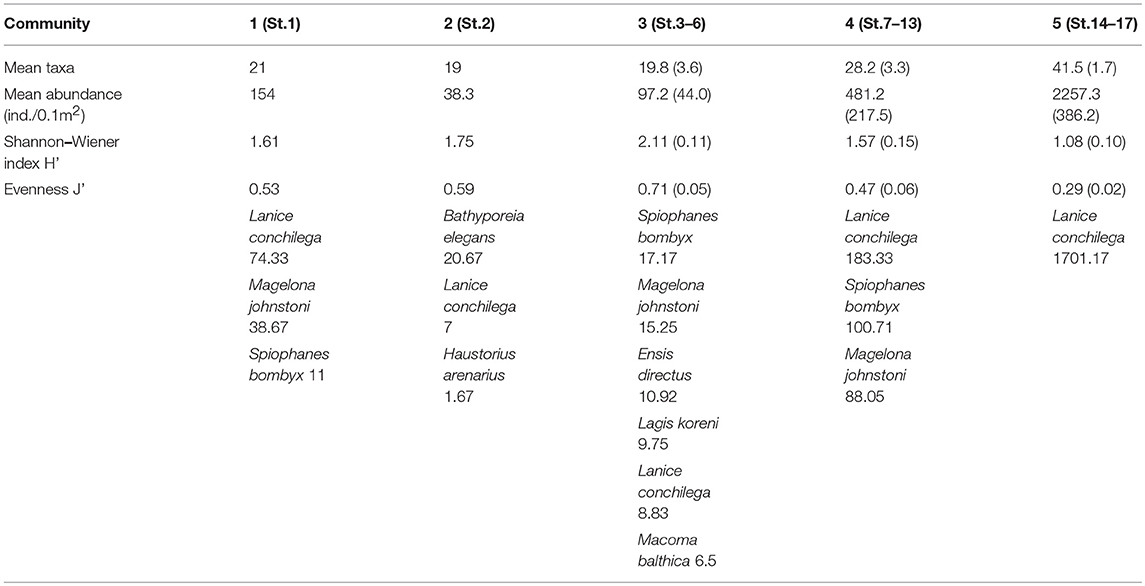
Table 1. Mean taxa number/0.1 m2, mean abundance/0.1 m2, mean Shannon-Index./0.1 m2, and mean evenness./0.1 m2, and characteristic species (ind./0.1 m2) of the macrofauna communities based on the SIMPER analysis.
Communities 1 to 3 (Table 1) revealed mean taxa numbers of 19–21/0.1 m2, mean abundances of 38–154/0.1 m2, Shannon-Wiener diversities of 1.61–2.11 and evenness of 0.53–0.71. The characterizing species Lanice conchilega, Magelona johnstoni and Spiophanes bombyx occurred in different abundances in the communities, while Bathyporeia elegans was the dominant species in Community 2.
Community 4 (Table 1) revealed higher mean taxa number and mean abundance than Communities 1–3, but Shannon-Wiener diversity and evenness were a bit lower. Lanice conchilega was the dominant species, followed by Spiophanes bombyx and Magelona johnstoni.
Community 5 (Table 1) was characterized by a species-rich benthic fauna and highest mean abundances, while lowest Shannon-Wiener evenness were found. Abundances of Lanice conchilega reached 1702 ind./ 0.1 m2.
Hydrodynamic Groups
The normalized hydrodynamic data obtained from the sampling stations for depths of the bottom boundary layer (BBL) and the upper bottom boundary layer (UBBL) were clustered hierarchically based on similarity. Looking at current velocities within the two layers, differences in depth (Δz) and in current velocity (Δu), as well as differences in temperature (Δt), salinity (Δs), and direction of outflowing low tide waters, we identified five hydrodynamic groups (A-E), which were significantly separated by SIMPROF (p < 0.05). The underlying environmental data of the five groups is shown in Table 2.
Current velocity in the UBBL increased continuously along the transect from group A to E from 0.25 to 0.40 m/s, while in the BBL it was highest in group D resulting in low difference in velocity of both layers (Δu), similar to group A (Table 2). Temperature was 1.73°C lower in group E than in group A. Salinity increased from group A to E. In groups A and B, low tide waters flowed in northwest direction, in groups C to E in southwestern direction.
Sediment Types
The hierarchical clustering based on similarity of the normalized environmental data of depth, bulk contents of sand, mud and shell debris, and mean sand grain sizes revealed five sediment types (a-e). Within this types only type a was significantly separated by SIMPROF (p < 0.05). The underlying environmental data of the five sediment types is shown in Table 2.
Sandy sediments dominated. Sand contents decreased generally along the transect from 96 (type a) to 90% (type e), while lowest content was found in type c with 84%. Content of shell debris ranged between 1.78 (type a) and 3.53% (type d) (Table 2). Contents of medium and fine sand were highest in type a, but content of medium sand decreased significantly from type b onwards, while content of fine sand increased (Table 2).
Acoustic Seafloor Classification
Based on backscatter data of the SideScanSonar and the ground truth data of the grab samples, the study site could be subdivided into three different areas along the transect (Table 2).
Area 1 was found within water depths of ~2 to ~3 m at low tide level. It included sampling stations 2 to 4, which backscatter signals were classified as areas of coarser sand with virtually no shell fragments.
Area 2 was found within water depths of ~5 to ~6 m at low tide level. It included sampling stations 7 to 11, which were classified as mixed areas, since there were some muddy areas, as well as fine sand areas with shell fragments and smaller worm tubes of the species Lanice conchilega. Some fecal pellets covered smaller shell fragments and the sand material.
Area 3 was found within water depths of ~8 to ~11 m at low tide level. It included sampling stations 13 to 17. Photographs showed an increase in shells of the bivalve Ensis directus and dense aggregations of Lanice conchilega tubes.
Beach Zonation of the Study Site
The cluster analysis of all environmental parameters and the macrofauna communities subdivided the sandy beach system of the near- and offshore zone of Spiekeroog island into three zones (Figure 2, Table 3): The first zone included stations 1 to 6 in the inner nearshore zone, the second zone included stations 7 to 12 in the outer nearshore zone, and the third habitat included stations 13 to 17 in the offshore zone.
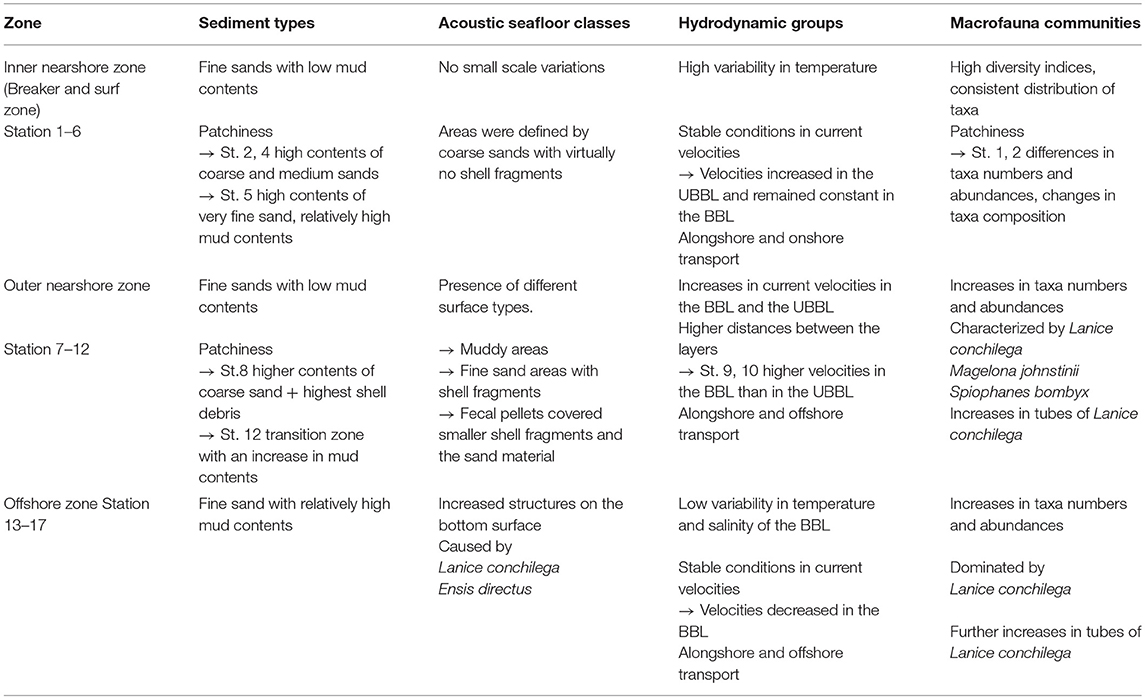
Table 3. Summary of the characteristics of the environmental parameters and macrofauna communities for the defined zones within the sandy beach systems of the island of Spiekeroog.
The principal component ordination analysis (PCO) of the environmental parameters confirmed the separation of the stations within three zones depending on their distance from the shoreline (Figure 3). The outcome states that 61.1% of the spatial structure shown by PCO can be explained by the environmental parameters. The first environmental axis was explained by the hydroacoustic seafloor classification, and by the hydrodynamic parameters (temperature, salinity, Δu, trajectories of outflowing low tide waters). The second environmental axis was associated with the sediment parameters (shell debris content, mud content, fine sand, medium sand).
Discussion
The analysis of the spatial distribution structure of the environmental parameters and the macrofauna communities revealed a classical zonation of a sandy beach system in near- and offshore zone along the transect. The spatial structure of the environmental parameters hereby explained >60 % of the zonation structure. Our findings confirm a strong influence of environment parameters on the macrofauna communities as it is postulated by several studies on sandy beach systems on regional or global scale (e.g., Dörjes, 1976; McLachlan, 1983; Brown and McLachlan, 1990; Armonies and Reise, 2000; Defeo and McLachlan, 2005, 2013; McLachlan and Dorvlo, 2005; Short, 2012; Nel et al., 2014). The separation of the beach into different zones is caused by the relationships between environmental parameters and macrofauna communities. Although the cluster analysis for each of the parameters (macrofauna communities, hydrodynamic groups, sediment types) resulted in five clusters (Tables 1, 2), the joint cluster analysis of macrofauna communities and all environmental parameters combined (Figure 2) resulted in a separation into three clusters/zones only, which correspond with the hydroacoustic seafloor classification (Table 2). This difference in numbers of clusters was probably caused by the high spatial variability in the abiotic parameters in the exposed inner nearshore zone, where especially hydrodynamic forces, resulting from wave action and tides, form a highly dynamic environment in the nearshore zone of sandy beaches, the breaker and surf zone (Short, 2012; Carter, 2013).
The main difference, which we found in the hydrodynamic groups between the inner and outer nearshore zone, was an increase of the current velocity in the UBBL toward the offshore zone and a change of the direction of outflowing low tide waters from SW to NW from the inner to the outer nearshore zone. Carter (2013) found that the lack of wave action on the sea bottom in hydrodynamic systems of sandy beaches is marked by a transition point in the direction of current flow. He also stated that the interaction of wave approaches and existing hydrodynamic systems, including tides, result in high variability of the sediment distribution (Carter, 2013) which was also present in our study. Similar to our results, Short (2012) described a high variability in sediment distribution as a consequence of high hydrodynamic energy. Sediments, containing higher contents of medium sand, should be exposed to higher hydrodynamic energy. Even though the weather conditions during the measurements of our survey were quite calm, the inner nearshore zone still was clearly separated from the other parts of the transect. This confirms the spatial sustainability of hydrodynamic forces on beach systems.
Inner Nearshore Zone
Within the inner nearshore zone, we found three different sediment types: sediments, which contained high contents of sand; sediments, which contained high contents of fine sand; and sediments with increased mud contents (< 3% to ~ 6%). Several studies already showed the relationship between hydrodynamic mediated sediment composition and spatial distribution of macrofauna communities (e.g., Rachor et al., 2007; Kröncke et al., 2011; Schückel et al., 2013; Armonies et al., 2014; Markert et al., 2015). The physical environment can significantly influence food availability and effect sediment stabilization (Brown and McLachlan, 1990; Short, 1996; Defeo and McLachlan, 2005; Defeo et al., 2009). Small changes in any environmental parameter can form small microhabitats for macrofauna organisms (McLachlan et al., 1993; Defeo and McLachlan, 2005). Since in the highly dynamic environment of sandy beaches habitats are under permanent pressure and can change within short-time scale, macrofauna has to adapt quickly. Interesting in our study was for example the decrease in abundances and the change in species composition in Community B and C, where the sediments were characterized by an increase in fine sand contents probably due to decreasing hydrodynamic energy.
We found three different macrofauna communities in the inner nearshore zone, representing a comparably small distance (~1 km). Communities were characterized by higher diversity indices, while taxa numbers and abundances were lower indicating an intermediate level of disturbance (Clarke and Gorley, 2006). McLachlan et al. (1993) postulates by the swash exclusion hypothesis (SEH) that swash climate, mainly influenced by wave height and beach slope, is the main driver controlling the intertidal sandy beach macrofauna. Defeo et al. (2001) formulated the Habitat Harshness Hypothesis (HHH), which states that in harsh reflective beaches, organisms need to divert more energy toward maintenance, and, therefore, have lower fecundity and higher mortality. However, the decrease in abundances found in the Community B might have resulted from a punctual increase in the “harshness of the environment” reflected also by greater depth. The occurrence of characterizing species such as Bathyporeia elegans and Haustorius arenarius in Community B, which are common in medium sands and high exposition rates to hydrodynamics such as the inner nearshore zone of the nearby island of Norderney (Dörjes, 1976), support a strong influence of hydrodynamics in the exposed inner nearshore areas of sandy beach systems. Higher mud contents and lower depth in Community C revealed the high spatial variability and reflect higher food supply for species such as Lagis koreni, Ensis directus, and Macoma balthica probably caused by nutrient discharge mediated growth of phytoplankton and microphytobenthos (Beck et al., 2017).
Outer Nearshore Zone and Offshore Zone
In the outer nearshore zone the environmental parameters generally revealed more stable conditions ongoing with changes in the community composition and distribution. We found a slight increase in the current velocities of the BBL and the UBBL. The trajectories of the current velocities changed and implied alongshore and offshore transports. Based on the assumption that the transition point, characterized by the change in the direction of outflowing low tide water, marks the end of the breaker and surf zone (Short, 2012; Carter, 2013), influences of wave action on the sea bottom in the outer nearshore zone should be minimal. This fits to our findings of the sediment distribution in the outer nearshore zone. Sediments were quite homogenous and mainly consisted of fine sand material with low mud contents. With regard to the adapted Autecological Hypotheses of McLachlan (1990), the abundances and taxa numbers of macrofauna communities should now increase due to the decreased “harshness of the environment” in the outer nearshore zone; biological interactions should become more important (Defeo and McLachlan, 2005; McLachlan and Dorvlo, 2005). Indeed, the community of the outer nearshore zone showed an increase in abundances and taxa numbers, and a decrease in diversity indices. Similar to an earlier study in the offshore zone of the nearby island of Norderney (Dörjes, 1976), the community was dominated by three characterizing species: Lanice conchilega, Spiophanes bombyx, and Magelona jonstonii. Information on the ecology of Magelona johnstonii and Spiophanes bombyx is limited. Studies showed that Magelona johnstonii and Spiophanes bombyx mainly occur in areas of fine sand. They are interface feeding polychaetes present in several subtidal areas of the North Sea (Fiege et al., 2000; Kröncke et al., 2011; Markert et al., 2015). All species probably benefit from food supply via nutrient discharge mediated growth of phytoplankton and microphytobenthos in the nearshore zone (Beck et al., 2017).
Lanice conchilega is a tube building polychaete colonizing both intertidal and subtidal sediments down to about 100 m depth (Dittmann, 1999; Ropert and Dauvin, 2000; Callaway, 2003). It occurs in high densities of several thousand individuals per m2 on all coasts of Europe and in both the Atlantic and the Pacific (Holthe, 1977). Maximum abundances of up to 20,000 individuals per m2 were reported from shallow subtidal areas of the German coast (Callaway, 2003). Tubes of Lanice conchilega protrudes 1 to 4 cm above the sediment surface and consist of smaller particles attached to an inner thin organic layer (Ziegelmeier, 1952; Dittmann, 1999). Studies on the ecology of Lanice conchilega showed that in both inter- and subtidal areas aggregations of tubes provide niches for a generally more species rich and abundant benthic faunal community (Rabaut et al., 2007; van Hoey et al., 2008). Lanice conchilega is, thus, often referred to as an important ecosystem engineer (Callaway, 2003; Rabaut et al., 2007; van Hoey et al., 2008; Callaway et al., 2010; de Smet et al., 2013). High abundances of Lanice conchilega increase the habitat quality in otherwise uniform habitats, and can increase sediment stability in exposed areas, resulting in a higher survival of the surrounding benthic species (Callaway, 2003; Rabaut et al., 2007; van Hoey et al., 2008; Callaway et al., 2010; de Smet et al., 2013). Indeed, the finding of a total number of 85 taxa, observed for the whole system, is surprisingly high compared to those known from literature. Here a total maximum of 25 taxa in a dissipative beach system were reported (McLachlan and Dorvlo, 2005).
The tubes of Lanice conchilega can also be detected by hydroacoustic measurements (Galloway and Collins, 1998; Ellingsen et al., 2002; Freitas et al., 2003; Wienberg and Bartholomä, 2005; Markert et al., 2013, 2015). Bartholomä (2006) stated that the occurrence of benthic faunal patterns covering a few m2 immediately produces changes in backscatter signals. These changes correspond to shifts in the acoustic class assignment. For the outer nearshore zone of Spiekeroog we detected variation within the hydroacoustic signals by the backscatter measurements, becoming more obvious toward the offshore zone. In the offshore zone also current velocities of the BBL were lower compared to the nearshore zone. The community structure revealed again an increase in taxa number and in the total mean abundances. Eckman et al. (1981) showed that a decrease of the current velocities can be caused by an increase in habitat structure by tubes of Lanice conchilega; they cause deflections of the earlier laminar flow. The high abundances found in the offshore zone, thus, might have caused the decrease in the current velocities of the bottom layers.
Conclusions
Our approach on the ecology of the near- and offshore areas of the island of Spiekeroog revealed interesting insights, especially in the relationship between environmental parameters of hydrodynamics and sediments, and the macrofauna communities. Our results show that these relationships are representable for the spatial zonation of the beach system. The beach system of Spiekeroog is an exception to the assumptions of several studies, which state that sandy beach systems generally show a lack of biogenic structures (Brown and McLachlan, 1990; Short, 1996; Defeo and McLachlan, 2005; Defeo et al., 2009; Nel et al., 2014).
Author Contributions
IK, TB, AB, and OZ designed the study. IK, TB, AB, A-CS, and OZ participated in sampling. LB carried out taxonomic identification of macrofauna species and wrote first version of the manuscript, IK revised manuscript. TB, AB, A-CS, and OZ gave original input to the manuscript, revision and proof-read final manuscript. IK supervised LB, TB, and OZ supervised A-CS.
Conflict of Interest Statement
The authors declare that the research was conducted in the absence of any commercial or financial relationships that could be construed as a potential conflict of interest.
Acknowledgments
We thank the captains and crews of RV “Senckenberg” and RB “Otzum” for help with sampling. We are grateful to Daniela Meier and Astrid Raschke for technical support in the field and in the lab.
References
Anderson, M. J., Gorley, R. N., and Clarke, K. R. (2008). PERMANOVA+ for PRIMER Guide to Software and Statistical Methods. Plymouth. 1–217.
Armonies, W., Buschbaum, C., and Hellwig-Armonies, M. (2014). The seaward limit of wave effects on coastal macrobenthos. Helgol. Mar. Res. 68, 1–16. doi: 10.1007/s10152-013-0364-1
Armonies, W., and Reise, K. (2000). Faunal diversity across a sandy shore. Mar. Ecol. Prog. Ser. 196, 49–57. doi: 10.3354/meps196049
Backhaus, H. (1943). Die ostfriesischen Inseln und ihre Entwicklung. Oldenburg: Gerhard Stalling Verlag.
Badewien, T. H., Zimmer, E., Bartholomä, A., and Reuter, R. (2009). Towards continuous long-term measurements of suspended particulate matter (SPM) in turbid coastal waters. Ocean. Dyn. 59, 227–238. doi: 10.1007/s10236-009-0183-8
Bartholomä, A. (2006). Acoustic bottom detection and seabed classification in the German Bight, southern North Sea. Geo-Marine Lett. 26, 177–184. doi: 10.1007/s00367-006-0030-6
Beck, M., Reckhardt, A., Amelsberg, J., Bartholom,ä, A., Brumsack, H.-J., Cypionka, H., et al. (2017). The drivers of biogeochemistry in beach ecosystems: A cross-shore transect from the dunes to the low-water line. Mar. Chem. 190, 35–50. doi: 10.1016/j.marchem.2017.01.001
Bergamino, L., Lercari, D., and Defeo, O. (2011). Food web structure of sandy beaches: temporal and spatial variation using stable isotope analysis. Estuar. Coast. Shelf. Sci. 91, 536–543. doi: 10.1016/j.ecss.2010.12.007
Brazeiro, A. (2001). Relationship between species richness and morphodynamics in sandy beaches - what are the underlying factors? Mar. Ecol. Prog. Ser. 224, 35–44. doi: 10.3354/meps224035
Brezina, J. (1979). “Particle size and settling rate distributions of sand-sized materials,” in PARTEC - 2nd European Symposium on Particle Characterization (Nürnberg), 1–47.
Burchard, H., and Badewien, T. H. (2015). Thermohaline residual circulation of the Wadden Sea. Ocean. Dyn. 65, 1717–1730. doi: 10.1007/s10236-015-0895-x
Burchard, H., Flöser, G., Staneva, J. V., Badewien, T. H., and Riethmüller, R. (2008). Impact of density gradients on net sediment transport into the Wadden Sea. J. Phys. Oceanogr. 38, 566–587. doi: 10.1175/2007JPO3796.1
Callaway, R. (2003). Juveniles stick to adults: Recruitment of the tube-dwelling polychaete Lanice conchilega (Pallas, 1766). Hydrobiologia 503, 121–130. doi: 10.1023/B:HYDR.0000008494.20908.87
Callaway, R., Desroy, N., Dubois, S. F., Fournier, J., Frost, M., Godet, L., et al. (2010). Ephemeral bio-engineers or reef-building polychaetes: How stable are aggregations of the tube worm Lanice conchilega (Pallas, 1766)? Integr. Comp. Biol. 50, 237–250. doi: 10.1093/icb/icq060
Carter, R. W. G. (2013). Coastal Environments: An Introduction to the Physical, Ecological, and Cultural Systems of Coastlines. London: Academic Press. 1–617.
Clarke, R. K., and Gorley, R. N. (2006). PRIMER V6: User Manual - Tutorial. Plymouth: Prim. Plymouth Mar Lab, 1–190.
de Smet, B., Godet, L., Fournier, J., Desroy, N., Jaffr,é, M., Vincx, M., et al. (2013). Feeding grounds for waders in the Bay of the Mont Saint-Michel (France): The Lanice conchilega reef serves as an oasis in the tidal flats. Mar. Biol. 160, 751–761. doi: 10.1007/s00227-012-2130-3
Defeo, O., Gomez, J., and Lercari, D. (2001). Testing the swash exclusion hypothesis in sandy beach populations: The mole crab Emerita brasiliensis in Uruguay. Mar. Ecol. Prog. Ser. 212, 159–170. doi: 10.3354/meps212159
Defeo, O., and McLachlan, A. (2005). Patterns, processes and regulatory mechanisms in sandy beach macrofauna: a multi-scale analysis. Mar. Ecol. Prog. Ser. 295, 1–20. doi: 10.3354/meps295001
Defeo, O., and McLachlan, A. (2011). Coupling between macrofauna community structure and beach type: a deconstructive meta-analysis. Mar. Ecol. Prog. Ser. 433, 29–41. doi: 10.3354/meps09206
Defeo, O., and McLachlan, A. (2013). Global patterns in sandy beach macrofauna: species richness, abundance, biomass and body size. Geomorphology 199, 106–114. doi: 10.1016/j.geomorph.2013.04.013
Defeo, O., McLachlan, A., Schoeman, D. S., Schlacher, T. A., Dugan, J., Jones, A., et al. (2009). Threats to sandy beach ecosystems: a review. Estuar. Coast. Shelf Sci. 81, 1–12. doi: 10.1016/j.ecss.2008.09.022
Dittmann, S. (1999). The Wadden Sea ecosystem. Heidelberg: Springer-Verlag. doi: 10.1007/978-3-642-60097-5
Dobrynin, M., Gayer, G., Pleskachevsky, A., and Günther, H. (2010). Effect of waves and currents on the dynamics and seasonal variations of suspended particulate matter in the North Sea. J. Mar. Syst. 82, 1–20. doi: 10.1016/j.jmarsys.2010.02.012
Dörjes, J. (1976). Primärgefüge, Bioturbation Und Makrofauna Als Indikatoren Des Sandversatzes Im Seegebiet Vor Norderney (Nordsee). II. Zonierung und Verteilung der Makrofauna. Senckenbergiana marit. 8, 171–188.
Dugan, J. E., Defeo, O., Jaramillo, E., Jones, A. R., Lastra, M., Nel, R., et al. (2007). Give beach ecosystems their day in the sun. Science 80, 4–6. doi: 10.1126/science.329.5996.1146-a
Eckman, J. E., Nowell, A. R. M., and Jumars, P. A. (1981).Sediment destabilization by animal tubes. J. Mar. Res. 39, 361–374.
Ellingsen, K. E., Gray, J. S., and Bjørnbom, E. (2002). Acoustic classification of seabed habitats using the QTC VIEWTM system. ICES J. Mar. Sci. 59, 825–835. doi: 10.1006/jmsc.2002.1198
Fiege, D., Licher, F., and Mackie, A. S. Y. (2000). A partial review of the European Magelonidae (Annelida: Polychaeta): Magelona mirabilis redefined and M. johnstoni sp. nov. distinguished. J. Mar. Biol. Assoc. 80, 215–234. doi: 10.1017/S0025315499001800
Flemming, B. W., and Thum, A. B. (1978). The settling tube - a hydraulic method for grain size analysis of sands. Kieler Meeresforsch. 4, 82–95.
Freitas, R., Rodrigues, A. M., and Quintino, V. (2003). Benthic biotopes remote sensing using acoustics. J. Exp. Mar. Bio. Ecol. 285–286, 339–353. doi: 10.1016/S0022-0981(02)00536-1
Galloway, J. L., and Collins, W. T. (1998). “Dual frequency acoustic classification of seafloor habitat using the QTC VIEW,” in IEEE Oceanic Engineering Society. OCEANS'98. Conference Proceedings (Nice). doi: 10.1109/OCEANS.1998.726277
Grashorn, S., Lettmann, K. A., Wolff, J. O., Badewien, T. H., and Stanev, E. V. (2015). East Frisian Wadden Sea hydrodynamics and wave effects in an unstructured-grid model. Ocean Dyn. 65, 419–434. doi: 10.1007/s10236-014-0807-5
Hallermeier, R. J. (1980). A profile zonation for seasonal sand beaches from wave climate. Coast Eng. 4, 253–277. doi: 10.1016/0378-3839(80)90022-8
Hild, A., Niesel, V., and Günther, C.-P. (1999). “The backbarrier tidal flats of spiekeroog description of the spiekeroog backbarrier system,” in The Wadden Sea ecosystem, ed S. Dittmann (Heidelberg: Springer-Verlag), 15–49.
Holinde, L., Badewien, T. H., Freund, J. A., Stanev, E. V., and Zielinski, O. (2015). Processing of water level derived from water pressure data at the Time Series Station Spiekeroog. Earth Syst. Sci. Data 7, 289–297. doi: 10.5194/essd-7-289-2015
Holthe, T. (1977). The zoogeography of the Terebellomorpha (Polychaeta) of the northern European waters. Sarsia 63, 191–198. doi: 10.1080/00364827.1978.10411339
Kröncke, I., Reiss, H., Eggleton, J. D., Aldridge, J., Bergman, M. J. N., Cochrane, S., et al. (2011). Changes in North Sea macrofauna communities and species distribution between 1986 and 2000. Estuar. Coast. Shelf Sci. 94, 1–15. doi: 10.1016/j.ecss.2011.04.008
Lettmann, K. A., Wolff, J.-O., and Badewien, T. H. (2009). Modeling the impact of wind and waves on suspended particulate matter fluxes in the East Frisian Wadden Sea (southern North Sea). Ocean. Dyn. 59, 239–262 doi: 10.1007/s10236-009-0194-5
Mangor, K. (1998). “Coastal restoration considerations,” in Coastal Engineering Proceedings (Copenhhagen), 3425–3438. doi: 10.9753/icce.v26.%25p
Markert, E., Holler, P., Kröncke, I., and Bartholomä, A. (2013). Benthic habitat mapping of sorted bedforms using hydroacoustic and ground-truthing methods in a coastal area of the German Bight/ North Sea. Estuar. Coast. Shelf Sci. 129, 94–104. doi: 10.1016/j.ecss.2013.05.027
Markert, E., Kröncke, I., and Kubicki, A. (2015). Small scale morphodynamics of shoreface-connected ridges and their impact on benthic macrofauna. J. Sea Res. 99, 47–55. doi: 10.1016/j.seares.2015.02.001
McLachlan, A. (1983). “Sandy beach ecology - a review,” in Sandy Beaches as Ecosystems. Developments in Hydrobiology Vol. 19, eds A. McLachlan and T. Erasmus (Dordrecht: Springer), 321–80. doi: 10.1007/978-94-017-2938-3_25
McLachlan, A. (1990). Dissipative beaches and macrofauna communities on exposed intertidal sands. J. Coast. Res. 6, 57–71.
McLachlan, A., De Ruyck, A., and Hacking, N. (1996). Community structure on sandy beaches: patterns of richness and zonation in relation to tide range and latitude. Rev. Chil. Hist. Nat. 69, 451–467.
McLachlan, A., and Dorvlo, A. (2005). Global patterns in sandy beach macrobenthic communities. J. Coast. Res. 21, 674–687. doi: 10.2112/03-0114.1
McLachlan, A., Jaramillo, E., Donn, T. E., and Wessels, F. (1993). Sandy beach macrofauna communities and their control by the physical environment: a geographical comparison. J. Coast. Res. Special Issue 15, 27–38.
Nam, P. T., Larson, M., Hanson, H., and Hoan, L. X. (2009). A numerical model of nearshore waves, currents, and sediment transport. Coast. Eng. 56, 1084–1096. doi: 10.1016/j.coastaleng.2009.06.007
Nel, R., Campbell, E. E., Harris, L., Hauser, L., Schoeman, D. S., McLachlan, A., et al. (2014). The status of sandy beach science: past trends, progress, and possible futures. Estuar. Coast. Shelf Sci. 150, 1–10. doi: 10.1016/j.ecss.2014.07.016
Rabaut, M., Guilini, K., van Hoey, G., Vincx, M., and Degraer, S. (2007). A bio-engineered soft-bottom environment: the impact of Lanice conchilega on the benthic species-specific densities and community structure. Estuar. Coast. Shelf Sci. 75, 525–536. doi: 10.1016/j.ecss.2007.05.041
Rachor, E., Reiss, H., Degraer, S., Duineveld, G. C. A., van Hoey, G., Lavaleye, M., et al. (2007). “Structure, distribution, and characterizing species of North Sea macro-zoobenthos communities in 2000,” in Structure and Dynamics of the North Sea Benthos eds H. L. Rees, J. D. Eggleton, E. Rachor, and E. Vanden Berghe (ICES Coop. Res. Rep) 288, 46–59.
Rodil, I. F., Lastra, M., and López, J. (2007). Macroinfauna community structure and biochemical composition of sedimentary organic matter along a gradient of wave exposure in sandy beaches (NW Spain). Hydrobiol. 579, 301–316. doi: 10.1007/s10750-006-0443-2
Ropert, M., and Dauvin, J. C. (2000). Renewal and accumulation of a Lanice conchilega (Pallas) population in the baie des Veys, western Bay of Seine. Oceanol. Acta 23, 529–546. doi: 10.1016/S0399-1784(00)00143-2
Schlacher, T. A., Dugan, J., Schoeman, D. S., Lastra, M., Jones, A., Scapini, F., et al. (2007). Sandy beaches at the brink. Divers. Distrib. 13, 556–560. doi: 10.1111/j.1472-4642.2007.00363.x
Schlacher, T. A., Schoeman, D. S., Dugan, J., Lastra, M., Jones, A., Scapini, F., et al. (2008). Sandy beach ecosystems: Key features, sampling issues, management challenges and climate change impacts. Mar. Ecol. 29, 70–90. doi: 10.1111/j.1439-0485.2007.00204.x
Schückel, U., Beck, M., and Kröncke, I. (2013). Spatial variability in structural and functional aspects of macrofauna communities and their environmental parameters in the Jade Bay (Wadden Sea Lower Saxony, southern North Sea). Helgol. Mar. Res. 67, 121–136. doi: 10.1007/s10152-012-0309-0
Schulz, A. C., Badewien, T. H., and Zielinski, O. (2015). “Impact of currents and turbulence on turbidity dynamics at the time series station Spiekeroog (Wadden sea, Southern North sea),” in 2015 IEEE/OES Eleveth Current, Waves and Turbulence Measurement (CWTM) (St. Petersburg, FL), 1–6.
Short, A. D. (1996). The role of wave height, period, slope, tide range and embaymentisation in beach classifications: a review. Revista Chilena de Historia Nat. 69, 589–604.
Tang, H. S., Keen, T. R., and Khanbilvardi, R. (2009). A model-coupling framework for nearshore waves, currents, sediment transport, and seabed morphology. Commun. Nonlinear Sci. Numer. Simulor. 14, 2935–2947. doi: 10.1016/j.cnsns.2008.10.012
van Hoey, G., Guilini, K., Rabaut, M., Vincx, M., and Degraer, S. (2008). Ecological implications of the presence of the tube-building polychaete Lanice conchilega on soft-bottom benthic ecosystems. Mar. Biol. 154, 1009–1019. doi: 10.1007/s00227-008-0992-1
Wentworth, C. K. (1922). A scale of grade and class terms for clastic sediments. J. Geol. 30, 377–392. doi: 10.1086/622910
Wienberg, C., and Bartholomä, A. (2005). Acoustic seabed classification in a coastal environment (outer Weser Estuary, German Bight) - a new approach to monitor dredging and dredge spoil disposal. Cont. Shelf Res. 25, 1143–1156. doi: 10.1016/j.csr.2004.12.015
Keywords: beach dynamics, hydrodynamics, sediments, acoustic seafloor classification, Lanice conchilega
Citation: Kröncke I, Becker LR, Badewien TH, Bartholomä A, Schulz A-C and Zielinski O (2018) Near- and Offshore Macrofauna Communities and Their Physical Environment in a South-Eastern North Sea Sandy Beach System. Front. Mar. Sci. 5:497. doi: 10.3389/fmars.2018.00497
Received: 24 August 2018; Accepted: 11 December 2018;
Published: 21 December 2018.
Edited by:
Lene Buhl-Mortensen, Norwegian Institute of Marine Research (IMR), NorwayReviewed by:
Juan Moreira Da Rocha, Universidad Autónoma de Madrid, SpainJan Marcin Weslawski, Institute of Oceanology (PAN), Poland
Copyright © 2018 Kröncke, Becker, Badewien, Bartholomä, Schulz and Zielinski. This is an open-access article distributed under the terms of the Creative Commons Attribution License (CC BY). The use, distribution or reproduction in other forums is permitted, provided the original author(s) and the copyright owner(s) are credited and that the original publication in this journal is cited, in accordance with accepted academic practice. No use, distribution or reproduction is permitted which does not comply with these terms.
*Correspondence: Ingrid Kröncke, aW5ncmlkLmtyb2VuY2tlQHNlbmNrZW5iZXJnLmRl