- 1Department of Ecology, Evolution, and Marine Biology, University of California, Santa Barbara, Santa Barbara, CA, United States
- 2Marine Science Institute, University of California, Santa Barbara, Santa Barbara, CA, United States
Corallivory is the predation of coral mucus, tissue, and skeleton by fishes and invertebrates, and a source of chronic stress for many reef-building coral species. Corallivores often prey on corals repeatedly, and this predation induces wounds that require extensive cellular resources to heal. The effects of corallivory on coral growth, reproduction, and community dynamics are well-documented, and often result in reduced growth rates and fitness. Given the degree of anthropogenic pressures that threaten coral reefs, it is now imperative to focus on understanding how corallivory interacts with anthropogenic forces to alter coral health and community dynamics. For example, coral bleaching events that stem from global climate change often reduce preferred corals species for many corallivorous fishes. These reductions in preferred prey may result in declines in populations of more specialized corallivores while more generalist corallivores may increase. Corallivory may also make corals more susceptible to thermal stress and exacerbate bleaching. At local scales, overfishing depletes corallivorous fish stocks, reducing fish corallivory and bioerosion, whilst removing invertivorous fishes and allowing population increases in invertebrate corallivores (e.g., urchins, Drupella spp.). Interactive effects of local stressors, such as overfishing and nutrient pollution, can alter the effect of corallivory by increasing coral-algal competition and destabilizing the coral microbiome, subsequently leading to coral disease and mortality. Here, we synthesize recent literature of how global climate change and local stressors affect corallivore populations and shape the patterns and effect of corallivory. Our review indicates that the combined effects of corallivory and anthropogenic pressures may be underappreciated and that these interactions often drive changes in coral reefs on scales from ecosystems to microbes. Understanding the ecology of coral reefs in the Anthropocene will require an increased focus on how anthropogenic forcing alters biotic interactions, such as corallivory, and the resulting cascading effects on corals and reef ecosystems.
Introduction
Reef-building corals are declining at an unprecedented rate worldwide as a result of anthropogenic forcing (Hughes et al., 2017a). From local impacts including overfishing and terrestrial runoff to global-scale phenomena such as ocean acidification and rising sea surface temperatures (Fabricius, 2011; Mora et al., 2011; Hoegh-Guldberg et al., 2017; Hughes et al., 2017a, 2018), anthropogenic stressors cause coral mortality and alter coral reef communities (Jackson et al., 2014; Hughes et al., 2017b). These shifts in coral density and reef community compromise both the resilience of coral reefs (Hughes et al., 2003; Putnam et al., 2017) and the ecological goods and services they provide (Moberg and Folke, 1999). The effects of individual human stressors on corals, such as warming-induced bleaching, are often well-documented (Fabricius, 2005; Mumby and Steneck, 2008; Mora et al., 2011; Hoegh-Guldberg et al., 2017; Hughes et al., 2018). However, it is also critical to understand how anthropogenic stressors alter important biotic interactions, such as predation by coral consumers (e.g., corallivory), that further impact coral growth and fitness and shape coral community dynamics.
Predation on tropical reef corals is common, with corallivores consuming coral mucus, tissue, and/or skeleton. Corallivores encompass a wide range of taxa including fishes, echinoids, crustaceans, mollusks, and annelids. The diversity of corallivore taxa is reflected in the diverse foraging behaviors and strategies (Figure 1), including: (1) mucus feeders, such as Trapezia and Tetralia crabs, that use specialized feeding appendages to consume mostly coral mucus along with some coral tissue while leaving the skeleton intact, (2) browsers that remove only coral tissue (e.g., butterflyfishes and Acanthaster spp.), (3) scrapers such as parrotfishes, that scrape live tissue from the surface of corals while also removing a thin layer of skeleton, and (4) excavators (bioeroders) that remove large amounts of coral skeleton while removing coral tissue (e.g., pufferfishes and some parrotfishes) [reviewed by Rotjan and Lewis (2008a)].
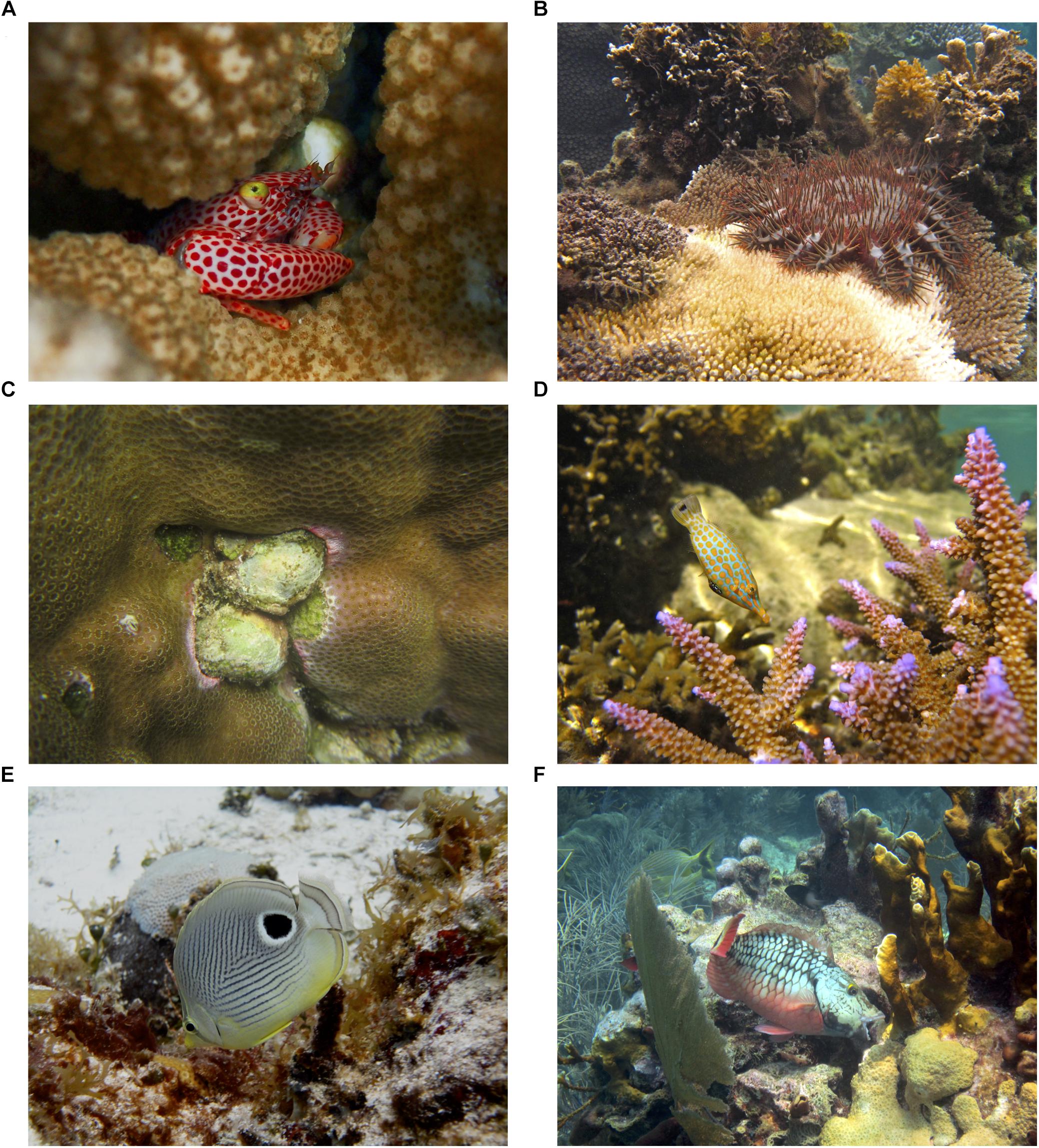
Figure 1. Common corallivores that exhibit a broad range of foraging behaviors. (A) Trapezia crab within the branches of a Pocillopora coral feeding on coral mucus and tissue (image by Corinne Fuchs). (B) Substantial tissue removal by Acanthaster spp. on an Acropora colony in Fiji (image by Cody Clements). (C) Corallivorous snail Coralliophila violacea removing massive Porites tissue and inducing an immune response (note the pink margin on the feeding scar) (image by Cody Clements). (D) The filefish Oxymonacanthus longirostris removing coral mucus and tissue from an Acropora sp. (image by Cody Clements). (E) The facultative butterflyfish Chaetodon capistratus consuming coral polyps (image by Cody Clements). (F) An excavating terminal phase Sparisoma viride removes chunks of coral skeleton in the Florida Keys (image by Corinne Fuchs).
Corallivory is an important determinant of health and fitness for individual corals and can impact coral community dynamics. Predation on corals can inhibit coral growth and sexual reproduction through tissue and/or skeleton removal (Henry and Hart, 2005) and can even cause widespread mortality of corals (Turner, 1994; Kayal et al., 2012; Saponari et al., 2018). For example, predation by crown-of-thorns starfish (Acanthaster spp.) was identified as the second largest contributor of coral mortality on the Great Barrier Reef between 1985 and 2012 (De’ath et al., 2012). Yet, corallivores play an integral role in coral communities since they can mediate coral-coral and coral-algal competition (Cox, 1986; Miller and Hay, 1998; Kayal et al., 2011; Johnston and Miller, 2014), foster asexual reproduction in corals (Enochs and Glynn, 2017), and even deter more efficient coral predators (Glynn, 1976). Given that the impacts of corallivory on corals and community dynamics can be diverse in direction and magnitude of effect (Mumby, 2009), there is a critical need to understand how, when, and to what extent predation on corals may interact with the changing biotic and abiotic template of reefs in the Anthropocene.
A number of reviews have documented corallivore feeding behavior and their impacts on coral growth, fitness, and community dynamics (e.g., Robertson, 1970; Cole et al., 2008; Rotjan and Lewis, 2008a; Enochs and Glynn, 2017; Rotjan and Bonaldo, 2018). However, our goal is to synthesize more recent work examining how anthropogenic stressors shape the consequences of corallivory. This topic is timely due to pervasive anthropogenic effects on both the abundance of corallivores and how corals respond to predation. Specifically, we synthesize recent information about how global (i.e., increasing sea surface temperatures and ocean acidification) and local stressors (i.e., overfishing, terrestrial runoff, nutrient pollution) alter the relationship between corals and their predators. These impacts span scales ranging from coral communities down to coral microbiomes. Additionally, we identify key gaps in our knowledge that may help guide future work on corallivory in the Anthropocene.
Effects of Corallivory on the Individual
The impact of corallivory on the wound healing, growth, and fitness of individual corals is well-documented (Henry and Hart, 2005; Cole et al., 2008; Rotjan and Lewis, 2008a). Here, we briefly discuss the effects of corallivory on individual corals in order to set the stage for the interactions between corallivory and anthropogenic forcing. We focus on more recent research to highlight current advances where possible.
Wound Healing Mechanisms
Corallivores can inflict damage to coral that initiate the host’s immune responses involved in wound healing. Upon injury, several immune pathways are activated: (1) the TOLL-like pathway to fight pathogens and recolonize commensal bacteria, (2) melanin synthesis for tissue regeneration, (3) the complement system resulting in apoptosis of damaged cells and tissue regeneration, and (4) cell activation to move amoebocytes to the wound (reviewed by Toledo-Hernandez and Ruiz-Diaz, 2014). Amoebocytes, cells responsible for phagocytosis, are involved in cnidarian tissue regeneration (Toledo-Hernandez and Ruiz-Diaz, 2014) and melanin synthesis (i.e., production of new tissue) (Palmer and Traylor-Knowles, 2012). Tissue regeneration and its associated immune pathways require extensive cellular resources (e.g., carbon products and amoebocytes) to be translocated to the wound (Henry and Hart, 2005).
Corals that are better able to transfer these resources from healthy tissue to lesions will fare better in response to predation. For example, wound healing for imperforate corals (e.g., pocilloporids) is restrained to polyps adjacent to the wound (Henry and Hart, 2005). Yet, perforate corals with deep tissue (e.g., poritids, fungids, and acroporids) can translocate resources across the colony to wounds (Jokiel et al., 1993; Edmunds, 2008). In either case, polyps bordering wounds are essential to translocating cellular resources for tissue regeneration (Henry and Hart, 2005). Yet this can drain neighboring polyps of metabolites and amoebocytes, directly affecting growth and reproduction.
Growth
The extent of damage often mediates the effects of corallivory on coral growth. Cameron and Edmunds (2014) observed that massive Porites and Pocillopora meandrina growth rates declined with more damaging modes of simulated fish corallivory (growth rates: browsed > scraped > excavated). Yet protection from corallivores with various foraging strategies can increase coral growth (Cox, 1986; Lenihan et al., 2011; Shantz et al., 2011), suggesting that even browsing corallivory has observable effects on coral growth (Shaver et al., 2017; Clements and Hay, 2018; Hamman, 2018). In fact, the gastropod Coralliophila violacea creates energy sinks as neighboring polyps translocate carbon products to the site of injury, allowing these snails to feed for extended periods of time (Oren et al., 1998). These energy sinks decrease coral growth rates as C. violacea size (Clements and Hay, 2018) and density increases (Hamman, 2018). Furthermore, mucus and tissue feeding Trapezia ectosymbionts can reduce coral calcification (Doo et al., 2018), potentially decreasing coral growth. Thus, different corallivore foraging strategies can directly alter coral growth, yet the degree of damage can mediate these effects.
Reproduction
Corals use the same finite cellular resources for both tissue regeneration and reproduction (Henry and Hart, 2005), creating a tradeoff between these metabolic processes (Rinkevich, 1996). Immediately following damage, polyps neighboring lesions experience a limited supply of cellular resources, reducing local reproductive output within the colony as resources are shunted toward wound healing (Kojis and Quinn, 1981; Van Veghel and Bak, 1994; Rotjan and Lewis, 2008b). Neighboring polyps likely exhaust resources on wound healing, ultimately reducing their reproductive potential and creating within-colony heterogeneity in reproductive output.
Yet the effects of corallivory on coral reproductive potential may depend on corals reproductive methods (i.e., asexual vs. sexual reproduction). For example, the pufferfish Arothron meleagris feeds intensively on Pocillopora damicornis, often resulting in fragmented branches (Guzman and Lopez, 1991; Palacios et al., 2014) that may result in propagules that disperse across the reef and grow into new colonies via asexual reproduction (Enochs and Glynn, 2017). However, fragmentation by corallivores can also have detrimental effects on coral sexual reproduction. For instance, ∼55% of regenerating fragmented Stylophora pistillata colonies resembling pufferfish predation were sterile during the reproductive season and had an order magnitude less larvae released compared to intact colonies (Rinkevich and Loya, 1989). Moreover, parrotfish in Belize selectively preyed on Montastrea annularis polyps containing more gonads, which reduced coral reproductive effort (Rotjan and Lewis, 2008b). Thus, the mode of reproduction mediates the influence of corallivory on coral reproductive output.
Global Stressors and Their Interactions With Corallivory
Rising Sea Surface Temperatures
Global ocean warming has been occurring the past >40 years due to increased human activities (Allen et al., 2014). As a consequence, the frequency and severity of regional-scale mass bleaching events has been increasing the past several decades (Hughes et al., 2018). These thermal anomalies have dire consequences for reef communities as it induces thermal stress and can result in massive coral mortality (Hughes et al., 2018). Thermal stress can drain coral energy reserves (Schoepf et al., 2015), likely compromising corals’ ability to meet the energy demands required to heal corallivory wounds. However, there are no clear patterns in how elevated temperatures affect tissue regeneration in scleractinians. For instance, warmer temperatures have little effect on tissue regeneration in Porites and Pocillopora (Edmunds and Lenihan, 2010; Lenihan and Edmunds, 2010; Traylor-Knowles, 2016) while increasing wound healing rates in cold water corals (Burmester et al., 2017). Yet elevated temperatures reduce wound healing in Indo-Pacific Acropora spp. (Denis et al., 2013; Bonesso et al., 2017) and mounding Caribbean corals (Meesters and Bak, 1993). However, the mechanisms behind why there appear to be taxa-specific impacts of temperature stress on wound healing are currently unclear.
The depletion of cellular resources due to chronic corallivory wounds may also affect corals’ ability to withstand or recover from thermal stress. In Caribbean brain corals, corallivorous snails increased coral bleaching severity as compared to corals without snails, with the densest snail aggregations causing corals to bleach completely (Shaver et al., 2018). Further, the highest densities of snails also led to less recovery from bleaching and complete colony mortality in many corals. Similarly, chronic corallivory by parrotfishes hindered the recovery of Symbiodinium populations in Orbicella spp. following bleaching events and also resulted in a change in the community composition of Symbiodinium within the corals (Rotjan et al., 2006). Moreover, some corallivores may transmit the bacterium Vibrio shiloi, which causes bleaching in a narrow range of coral taxa (Sussman et al., 2003; Moreira et al., 2014). Thus, chronic predation may exacerbate stress from thermal anomalies and reduce the resilience to and recovery from coral bleaching.
Coral bleaching also affects corallivore ecology with the response to bleaching depending on the degree of dietary specialization of the corallivore. If preferred corals become rare then specialized corallivores (e.g., butterflyfishes) often increase feeding on remaining healthy conspecifics (Cole et al., 2009). Conversely, generalist predators (e.g., wrasses and muricid snails) tend to prey more intensely on bleached colonies (Cole et al., 2009; Tsang and Ang, 2014) or other healthy coral species (Pratchett et al., 2004; Hoeksema et al., 2013; Zambre and Arthur, 2018). These alterations to feeding behavior have indirect effects on corallivorous fish populations. For instance, specialized butterflyfishes are projected to disappear from reefs as preferred prey species decline in response to bleaching whilst generalist butterflyfish populations are expected to remain stable (Berumen and Pratchett, 2006; Graham, 2007; Graham et al., 2009; Emslie et al., 2011; Wilson et al., 2014). However, diet plasticity in some specialized corallivores (e.g., Chaetodon trifasciatus) in response to bleaching may allow local populations to persist (Zambre and Arthur, 2018). Altogether, these data suggest that bleaching events can reduce population sizes of specialized corallivorous fishes but also concentrate corallivory on the remaining corals after a bleaching event, potentially slowing coral recovery.
Ocean Acidification
Ocean acidification (OA) reduces coral calcification rates, and consequently, coral growth (Kroeker et al., 2013). In fact, decreasing pH has already slowed coral growth on some reefs (Albright et al., 2018). To maintain growth rates under low pH conditions, corals can decrease skeletal density, which can promote destruction by bioeroders, like parrotfishes, that favor lower-density substrates (Hoegh-Guldberg et al., 2007). Corals prone to bioerosion will experience increased energy expenditures to repair skeletal damage, which is energetically costlier under low pH conditions (Doney et al., 2009). Yet this response may depend on coral life history strategies and the degree of damage. For example, end-of-century pH conditions (∼7.6) compromise wound healing rates for some mounding and branching corals more than faster-growing corals (Renegar et al., 2008; Horwitz and Fine, 2014; Hall et al., 2015; Edmunds and Yarid, 2017), although these effects appear variable depending on taxa. As pH continues to decline, there might be clear “losers” and “winners” for coral species under future OA conditions and concurrent corallivory (Renegar et al., 2008; Horwitz and Fine, 2014; Hall et al., 2015; Edmunds and Yarid, 2017). Research investigating the interactive effects of low pH and the different modes of corallivory (i.e., browser, scraper, and excavator) on corals with varying life history strategies would be especially valuable given that one would expect OA to compromise recovery from scraping and excavating corallivores much more than for browsing corallivores.
Some corallivores may also be subject to the effects of OA. Recent work suggests that the effects of OA on Acanthaster spp. may differ across life history stages. Low pH conditions (∼7.6) have dire effects during the early life history stages by hindering Acanthaster spp. fertilization, larval development, and settlement rates (Uthicke et al., 2013). However, juvenile Acanthaster spp. may maintain growth under projected OA scenarios, which is likely due to increased grazing rates on crustose coralline algae (Kamya et al., 2016, 2017). Thus, the magnitude of Acanthaster predation under future ocean conditions is likely to be governed by this taxa’s response during the developmental and juvenile stages.
Local Stressors and Their Interactions With Corallivory
Overfishing
Coral reef fishes play essential roles in reef community dynamics as predators, herbivores, and recyclers of nutrients (Sale, 2013). However, the overexploitation of reef fishes can significantly affect these ecological processes. For example, overfishing directly affects corallivory by reducing the biomass and species richness of corallivorous fishes (Guillemot et al., 2014), particularly larger parrotfish species (Bellwood et al., 2011). Parrotfishes are key herbivores, corallivores, and bioeroders on coral reefs worldwide (Hoey and Bonaldo, 2018). Yet, they are also a prime target of fisheries with some populations clearly threatened according to the IUCN Red List (i.e., Bolbometopon muricatum: vulnerable, Scarus guacamaia: near threatened, and Scarus trispinosus: endangered) (Chan and Donaldson, 2012; Choat et al., 2012; Padovani-Ferreira et al., 2012). The removal of these critically important fishes substantially reduces bioerosion and corallivory on fished reefs (Hoey and Bellwood, 2008; Bellwood et al., 2011). In fact, even at low densities B. muricatum is responsible for ∼88% of the corallivory and bioerosion on outer-shelf reefs in the Great Barrier Reef (Hoey and Bellwood, 2008). However, it disappears from reefs even at relatively low human densities and modest fishing pressure (Figure 2), drastically reducing bioerosion and corallivory rates (Dulvy and Polunin, 2004; Bellwood et al., 2011). Despite this dramatic example, the effect of overfishing corallivorous fishes on coral communities has received little attention (Bellwood et al., 2011).
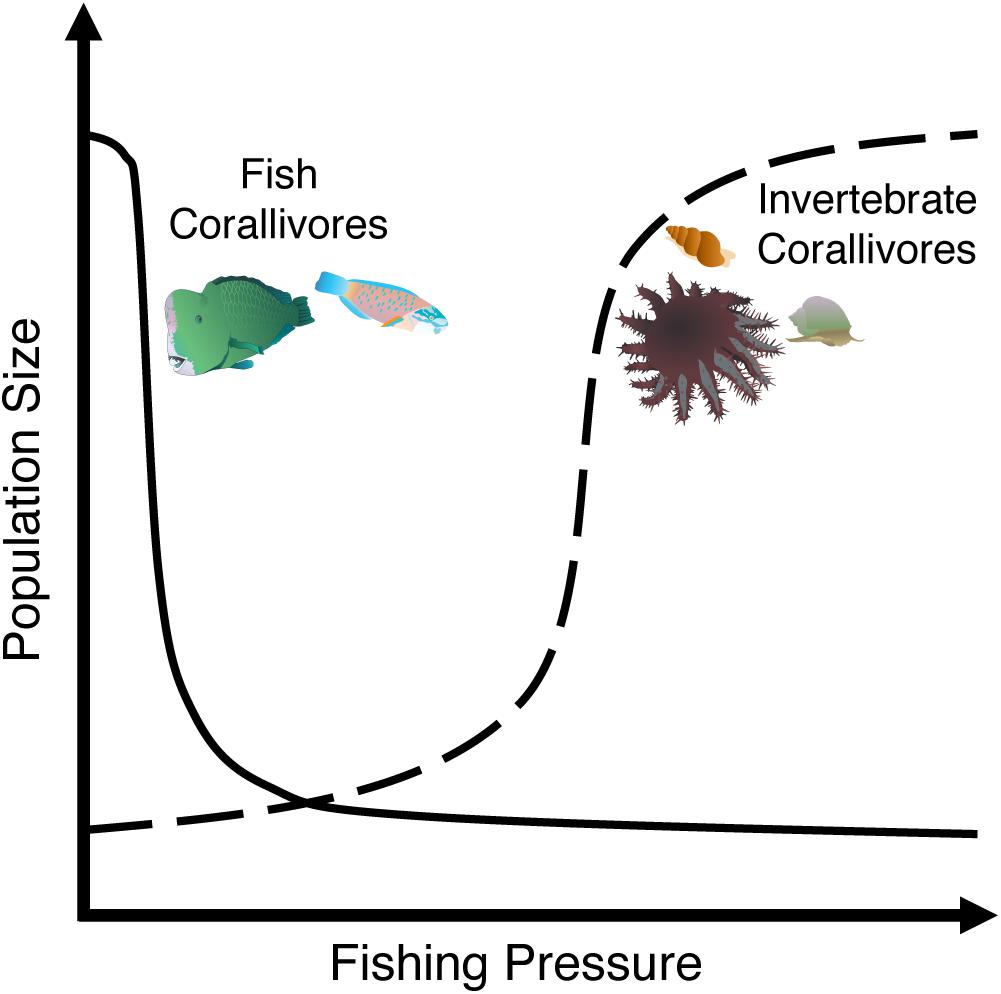
Figure 2. As fishing pressure increases, the population of large corallivorous fishes (e.g., parrotfishes) often show a steep initial decline followed by marginal decreases in population size. Conversely, fishing pressure may release invertebrate corallivores from predation allowing these corallivores to increase logistically up to fivefold on fished reefs.
In addition, the depletion of predatory fishes alters corallivory indirectly by releasing invertebrate corallivores from top-down control, resulting in increased invertebrate corallivore populations that cause widespread coral mortality (Figure 2; McClanahan, 1994; Dulvy et al., 2004; Sweatman, 2008). For example, densities of the gastropods Drupella cornus and Coralliophila violacea, as well as the crown-of-thorns sea star Acanthaster spp. and the sea urchin Echinometra mathaei, were greater on heavily fished reefs with low predator densities compared to marine protected areas (MPAs) with higher predator densities (McClanahan and Muthiga, 1989; McClanahan, 1994; Sweatman, 2008; Clements and Hay, 2017). Similarly, densities of Acanthaster spp. throughout Fiji’s Lau Islands were lower on islands with minimal fishing pressure (Dulvy et al., 2004). However, warmer temperatures and nutrient pollution that increase phytoplankton concentrations likely also facilitate Acanthaster outbreaks by benefitting larval and juvenile success (Pratchett et al., 2014; Uthicke et al., 2015; Brodie et al., 2017; Kamya et al., 2018). Thus, overfishing and nutrient pollution may work in concert to facilitate Acanthaster outbreaks. Indirect effects of overfishing on coral-corallivore interactions can also lead to changes in corallivore behavior. In Fiji, small, coral-dominated MPAs had ∼2–3.4 times greater densities of Acanthaster spp. than adjacent fished reefs dominated by macroalgae – likely due to migration of Acanthaster spp. from fished reefs into MPAs (Clements and Hay, 2017).
One consequence of overfishing on reefs is the rise of macroalgae as large herbivorous fishes are removed (Hughes et al., 2007; Mumby and Steneck, 2008; Holbrook et al., 2016). Competition with macroalgae may modify the effect of corallivory in corals several ways. In some cases, macroalgae can exacerbate predation. For example, the green calcareous alga, Halimeda opuntia, can facilitate corallivory by the fireworm, Hermodice carunculata (Wolf and Nugues, 2013). The alga creates sites of mortality on corals that attracts H. carunculata, which may also vector coral disease and further exacerbate coral mortality (Wolf and Nugues, 2013).
Conversely, macroalgae may benefit corals by providing an associational refuge from corallivorous invertebrates (Clements and Hay, 2015) and fishes (Venera-Ponton et al., 2011; Bulleri et al., 2013; Brooker et al., 2016, 2017). For instance, dense stands of the brown alga Sargassum polycystum significantly reduced growth of Montipora hispida, but also hindered attacks by crown-of-thorns sea star (Clements and Hay, 2015). Additionally, coral-feeding butterflyfishes and the obligate corallivore, Oxymonacanthus longirostris, avoided preying on corals that had been in physical contact with macroalgae (Brooker et al., 2013, 2017). Thus, some coral-algal interactions create a trade-off where corals are protected against predation yet may experience reduced growth as a consequence (Venera-Ponton et al., 2011; Clements and Hay, 2015). Given the rise of algae on many reefs due to anthropogenic forcing, it is increasingly important to examine how these algae will modify existing interactions between corals and corallivores.
Nutrient Pollution
The global use of phosphate- and nitrogen-rich fertilizers has increased by over 300 and 800%, respectively, since 1961 (Food and Agriculture Organization of the United Nations, 2014), resulting in a drastic increase of nutrient inputs into coral reefs and other coastal ecosystems (Fabricius, 2005). Increased nitrogen and phosphorus (P) loading has direct and indirect negative effects on coral fitness and physiology from suppressing growth rates to increasing susceptibility to bleaching and disease (D’Angelo and Wiedenmann, 2014; Shantz and Burkepile, 2014). Some of these effects may compound the negative effects of corallivory. For instance, corals exposed to nutrients from river discharge have more porous skeletons (Mwachireya et al., 2016), likely due to P enrichment increasing coral skeleton porosity (Koop et al., 2001; Dunn et al., 2012) and weakening the skeletal matrix (Caroselli et al., 2011). These effects on coral skeletons could exacerbate the effects of corallivores as elevated nutrients magnify the damage of microborers and parrotfishes on calcium carbonate blocks, possibly due to weakened skeletal structure (Chazottes et al., 2017). By extension, corals subjected to nutrient enrichment may be more susceptible to mechanical damage from predation and may experience reduced wound healing as a consequence (Koop et al., 2001; Renegar et al., 2008). However, these questions have received little attention so far.
The interaction between corallivory and nutrients may depend on the type of corallivore and the degree of damage to the coral. For example, Zaneveld et al. (2016) showed that corallivory by scraping and excavating parrotfish interacted with nutrient exposure to increase Porites mortality by 62% as compared to corals that were preyed on under nutrient poor conditions. Changes in the coral microbiome may have driven this increase in mortality as opportunistic Proteobacteria increased on corals bitten under nutrient replete conditions as compared to corals bitten under nutrient poor conditions (Figure 3). Conversely, predation by the snail, Coralliophila abbreviata, resulted in ∼33% mortality in Acropora cervicornis while nutrient exposure did not affect mortality but did increase the abundance of opportunistic bacteria in the coral microbiome (Shaver et al., 2017). Yet, the interaction between predation and nutrient exposure did not affect A. cervicornis mortality rates or coral microbiomes (Figure 3; Shaver et al., 2017). These results suggest that bioeroding corallivory may interact with nutrients in fundamentally different ways than browsing corallivory. Bioeroders remove significant amounts of skeletal material that may provide more area for colonization by opportunistic bacteria. More direct comparisons investigating the response of corals to various modes of corallivory (i.e., tissue removal vs. skeletal damage) and the potential interactions with nutrient exposure are needed to better understand the effects on coral condition and associated microbial communities.
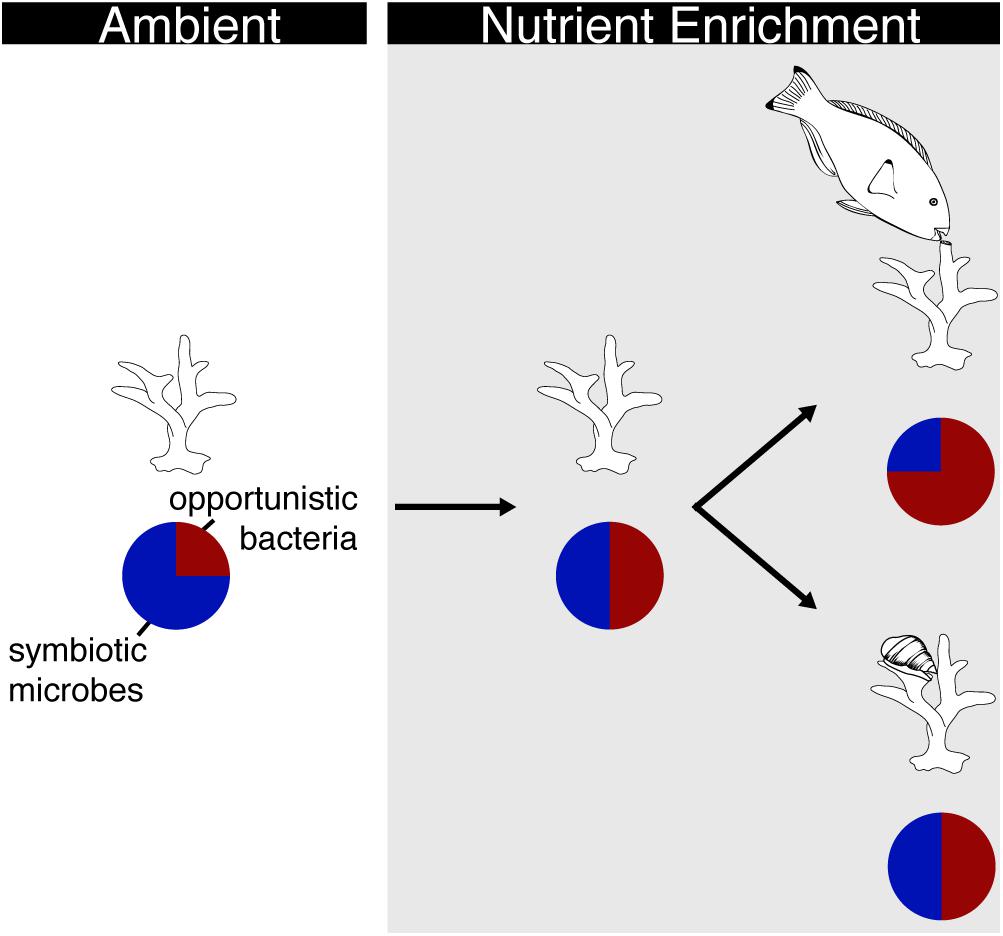
Figure 3. Schematic of how different modes of corallivory interact with nutrient enrichment to affect the coral microbiome. Under ambient nutrient conditions, the microbiome of healthy corals is dominated by symbiotic microbes (blue portion of pie chart) with few opportunistic bacteria (red portion of pie chart). Upon nutrient enrichment (gray box), more opportunistic bacteria colonize the microbial community of corals. In this scenario, host corals have less stable microbiomes and are more prone to concurrent stressors. The microbiome of corals experiencing nutrient enrichment and simultaneous parrotfish corallivory becomes dominated by opportunistic bacteria. Conversely, there is no shift in the microbial community of corals exposed to nutrients and snail corallivory.
Sedimentation
Coastal development, agriculture, and dredging are some of the leading contributors of sediment accumulation on reefs (Rogers, 1990; Fabricius, 2005). Sediment increases turbidity, resulting in suppressed photosynthesis and growth in corals that ultimately results in lower fecundity and survivorship (Rogers, 1990; Fabricius, 2005). Sediment accumulation also depletes lipid and energy reserves in corals due to the energy expended to shed particles via mucus production (Sheridan et al., 2014). These energetically compromised corals may be more susceptible to other stressors, such as corallivory. In fact, wounds caused by corallivorous fishes healed slower at sites characterized by high sediment compared to low sediment sites (Cróquer et al., 2002). Importantly, sedimentation and high turbidity suppressed the recovery of simulated parrotfish bites more than simulated butterflyfish corallivory (Cróquer et al., 2002). Corallivore feeding mode and the degree of structural damage induced by corallivores, in this case excavated vs. browsed bites, appears to determine the extent to which sedimentation hinders wound healing.
Some small invertebrate corallivores benefit corals by removing sediments from their host corals. Acropora and Pocillopora corals housing corallivorous ectosymbionts that feed on coral tissue and mucus (i.e., trapeziid crabs and alpheid shrimps) are less likely to be smothered by sediment and have higher survival rates (Stewart et al., 2006, 2013; Stier et al., 2012). In fact, housing Trapezia crabs makes juvenile Pocillopora corals five times less likely to be smothered by sediment and increases juvenile coral survival by ∼35% at sites with high sedimentation (Stewart et al., 2013). Moreover, trapeziid crabs and alpheid shrimps also help coral hosts maintain growth rates when hosts are covered with vermetid gastropod mucus nets (Stier et al., 2010) and protect hosts from Acanthaster spp. and Drupella predation (Glynn, 1976; Samsuri et al., 2018). Although these small corallivores do feed directly on coral tissue, these symbionts can increase coral growth (Stewart et al., 2006), demonstrating a net overall benefit to corals hosting these corallivorous ectosymbionts.
One area of research that has received little attention is how sediment type (i.e., fine vs. coarse-grained sediments) may mediate both the response of corals to predation and corallivore feeding behavior. Terrigenous fine sediments composed of clay and silt have higher organic content and smaller grain sizes, resulting in higher turbidity and greater reduction in light availability that invokes more physiological stress in corals compared to larger, coarser sediments (Fabricius, 2005; Weber et al., 2006; Erftemeijer et al., 2012). Although corals are able to shed smaller grain sizes (Stewart et al., 2006), doing so drastically depletes energy reserves (Sheridan et al., 2014), likely leaving corals ill-equipped to heal wounds following corallivory. Thus, corals’ response to corallivory may be fundamentally different in the presence of finer vs. coarser sediments.
In regard to the relationship between sediment type and corallivore feeding behavior, we know relatively little. Coarse sediments reduce herbivory rates for Scarus rivulatus, a facultative corallivore, more than fine sediments (Gordon et al., 2016). Similar trends may be observed for corallivory rates, but this has not been tested. Conversely, it seems plausible that finer sediments may deter invertebrate predators because smaller grain sizes may interfere with the proboscis feeding structure used by muricid gastropods. For example, the freshwater gastropod Potamopyrgus antipodarum is nearly four times more likely to be found on clean surfaces than surfaces with fine sediment (Suren, 2005). The same may hold true for reef gastropods but no data currently exist on this topic. The effects of different sediment types on corallivory, corallivore behavior, and coral condition is clearly an important area of research that needs attention.
Sewage Output
Human sewage is the largest contributor of global coastal pollution with most wastewater entering tropical coastal waters without prior treatment (Islam and Tanaka, 2004; Wear and Thurber, 2015). Sewage contains high concentrations of inorganic compounds, toxins, heavy metals, and pathogens, all of which can increase coral bleaching and mortality rates, reduce fecundity, and induce coral disease (Pantsar-Kallio et al., 1999). Pathogens from human sewage can destabilize the coral holobiont, providing invasive microbes the opportunity to colonize and potentially facilitate the vectoring of pathogens to corals by corallivores (Sutherland et al., 2010, 2011).
Sutherland et al. (2010) found that the enterobacterium strain Serratia marcescens PDR60, which is found in sewage, is also found in the obligate corallivorous snail Coralliophila abbreviata. Anthropogenic sources of sewage wastewater input this strain into the Florida Keys reef tract (Sutherland et al., 2011), infecting Acropora palmata with white pox disease. Moreover, C. abbreviata is a reservoir for S. marcescens because it preferentially consumes A. palmata (Miller, 2001) and can transmit the strain, and thus coral disease, to uninfected Acropora colonies (Sutherland et al., 2010). The propagation of this disease decreases live coral tissue and concentrates snail predators on remaining live coral, creating a positive feedback loop between muricid snail density and Acropora spp. predation (see Figure 6 in Williams and Miller, 2005). The interactions among sewage, corallivores, and coral disease is likely not exclusive to the Florida Keys as many tropical regions have little to no treatment of sewage and many species of corallivores may serve as vectors for pathogens that propagate disease. Examining how widespread these interactions are could be critical for understanding the full effects of sewage on coral reef communities.
Future Directions for Corallivory Research
How Does Anthropogenic Forcing Affect Coral Dynamics?
Much of the existing literature on the interactions of corallivory and anthropogenic stressors focuses on the impact on individual corals. There has been much less focus on how these stressors may change the impact of corallivory at the level of reef communities. For example, there has been a strong effort to understand how MPAs help maintain herbivory and the direct effects on algae and indirect effects on corals (Mumby et al., 2006, 2007; MacNeil et al., 2015), yet there has been little focus on the consequences to corallivory. We know the processes that parrotfishes strongly influence (e.g., bioerosion and corallivory) decline with increasing fishing pressure in Indo-Pacific reefs (Bellwood et al., 2011). Yet it is unclear if these patterns hold true in other regions where fewer corallivore species occur (i.e., Eastern Pacific, Caribbean). Conversely, we also know relatively little about how robust populations of these large corallivores impact coral populations and communities, especially on reefs where corals are declining. For example, parrotfishes in the Florida Keys, United States switch to preying on less favored coral species as preferred prey species become rare, which ultimately increases corallivory rates overall when coral cover is low and parrotfish density is high (Burkepile, 2012). This trend is a concerning one and understanding if it is a general phenomenon is important.
There is also limited knowledge on how corallivory interacts with local and/or global stressors to alter benthic community composition on reefs. The presence of select corallivores can reverse the competitive dominance amongst corals (Cox, 1986; Colgan, 1987; Kayal et al., 2011), altering community composition. For instance, when the corallivore Chaetodon unimaculatus is present, the competitive dominance shifts from Montipora verrucosa to Porites compressa because M. verrucosa is preferentially preyed upon (Cox, 1986). This reversed competitive hierarchy may be reinforced under different anthropogenic regimes. For example, Acropora and Pocillopora corals are preferred prey in the Indo-Pacific (Cole et al., 2008) and compete for space on the benthos. Under future ocean acidification scenarios, the competitive dominance shifts from Pocillopora to Acropora (Horwitz et al., 2017). Rapid Acropora growth rates (Anderson et al., 2017) may allow Acropora to outcompete Pocillopora, further reinforcing the reversed competitive hierarchy under low pH. Understanding these complex feedbacks will require both time series data of coral community dynamics as well as targeted experimentation to understand the mechanisms of interspecific interactions and predation patterns.
What Are the Interactions of Different Corallivore Feeding Modes and Anthropogenic Forcing?
The effects of corallivory can differ in magnitude depending on anthropogenic stressors. For example, browsing corallivory (e.g., tissue removal) does not exacerbate the effects of nutrient enrichment or sedimentation while excavating corallivory clearly does (Cróquer et al., 2002; Zaneveld et al., 2016; Shaver et al., 2017). Conversely, prudent gastropods that exert chronic predation and act as photosynthate energy sinks (e.g., Coralliophila spp.) (Oren et al., 1998) may exacerbate thermal stress, bleaching, and post-bleaching mortality (Shaver et al., 2018) more so than excavating corallivores that likely have more ephemeral effects on individual colonies (e.g., spot biting). Given that corallivore feeding mode has contrasting effects on corals under different anthropogenic templates, we need to better understand how these foraging strategies affect coral survivorship and energy budgets under both present-day conditions and future ocean scenarios. This question is especially important as corallivores with different feeding modes may have fundamentally different population trajectories under increasing anthropogenic forcing (Figure 2), potentially resulting in very different corallivore regimes in future oceans.
Both corallivory and anthropogenic stressors often have independent negative effects on coral fecundity (Van Veghel and Bak, 1994; Rotjan and Lewis, 2008b; Jones et al., 2015). However, how these forces interact to modulate reproductive potential in corals remains largely unknown. Tissue regeneration occurs at the expense of somatic growth and gametogenesis because these processes compete for the same cellular resources (Henry and Hart, 2005). Thus, the suppression of fitness likely scales with the degree of corallivory and the need for tissue regeneration. Yet it is unclear if anthropogenic stressors act additively or synergistically with various modes of corallivory to further reduce coral fitness. These interactions could serve as crucial negative effects on coral reproduction that lower the resilience of reefs in the face of increasing disturbances.
What Are the Effects of Corallivory at Molecular and Microbial Scales?
Anthropogenic pressures (e.g., sedimentation and seawater warming) often cause corals to upregulate immune response pathways, suggesting that corals may be robust to extrinsic stressors (Sheridan et al., 2014; van de Water et al., 2015b) and maintain the ability to regenerate tissue during thermal stress (van de Water et al., 2015a,c; Bonesso et al., 2017). However, research investigating the cellular mechanisms that govern tissue regeneration under other anthropogenic regimes (e.g., nutrient pollution) is surprisingly lacking. Studies that quantify the upregulation of enzymes and genes involved in immune response pathways are necessary to better understand host defense in response to these extrinsic factors and assess if local and global stressors act additively or synergistically.
Beyond affecting corals through mechanical damage, corallivores provide entry points for opportunistic bacteria (e.g., coral diseases) and can aid in parasite transmission on the reef. For instance, the coral-feeding butterflyfish Chaetodon multicinctus plays an implicit role in the dynamics of trematodiasis infection in some Porites spp., by serving as a final host in the life cycle of the trematode Podocotyloides stenometra (Aeby, 1998, 2002). Similarly, some corallivores can transmit disease pathogens to corals, and four invertebrate corallivores have been empirically linked to coral disease transmission (see Table 1 in Nicolet et al., 2018a). It is likely that this mechanism of disease transmission may be more common than currently appreciated. Similarly, butterflyfishes prey on diseased coral tissue (Aeby and Santavy, 2006; Chong-Seng et al., 2011; Nicolet et al., 2013, 2018b) with coral disease correlating positively with butterflyfish abundance (Raymundo et al., 2009). Corallivorous fishes may transmit disease pathogens orally or through feces (Aeby and Santavy, 2006), however, more research is needed to demonstrate if there is a causal link between corallivorous fishes (e.g., butterflyfishes and parrotfishes) and coral disease transmission (Nicolet et al., 2018a). Fish acting as vectors may be an important driver structuring coral-microbial communities, particularly for excavating corallivores, like parrotfishes, that create larger wounds in corals. Thus, there is a significant need for studies that examine the causal link between corallivores, coral microbiome dynamics, and coral disease propagation.
In the context of anthropogenic forcing, corallivory may act synergistically to destabilize the coral microbiome, leading to compromised host immunity (Zaneveld et al., 2017) and increased mortality (Zaneveld et al., 2016). Moreover, compared to MPAs, overfished reefs have a higher prevalence of coral disease (Raymundo et al., 2009) and larger populations of invertebrate corallivores capable of transmitting opportunistic bacteria (McClanahan, 1994; Dulvy et al., 2004; Sweatman, 2008; Nicolet et al., 2018a). These local processes may further fuel coral disease propagation via corallivory. Understanding how anthropogenic pressures might shape the effects of corallivores on coral microbiomes will be a fruitful and important area of research.
Conclusion
In the Anthropocene, the convergence of multiple interacting stressors is altering the natural interactions among corals and corallivores. Although a small suite of corallivores have beneficial effects on corals, the negative effects of most corallivores appear to be exacerbated by anthropogenic stressors. As anthropogenic forcing strengthens, the rich biodiversity characterized by pristine reefs will decline (Figure 4A). Global stressors, such as ocean warming, will continue to induce bleaching and mortality in thermally sensitive coral species while local stressors, such as nutrient pollution and sedimentation, exacerbate coral disease, fundamentally altering coral community composition. Concurrently, local stressors such as overfishing is removing both large corallivorous fishes that impact coral community composition as well as the predators of corallivorous invertebrates, relaxing top-down control on these corallivores (Figure 4B).
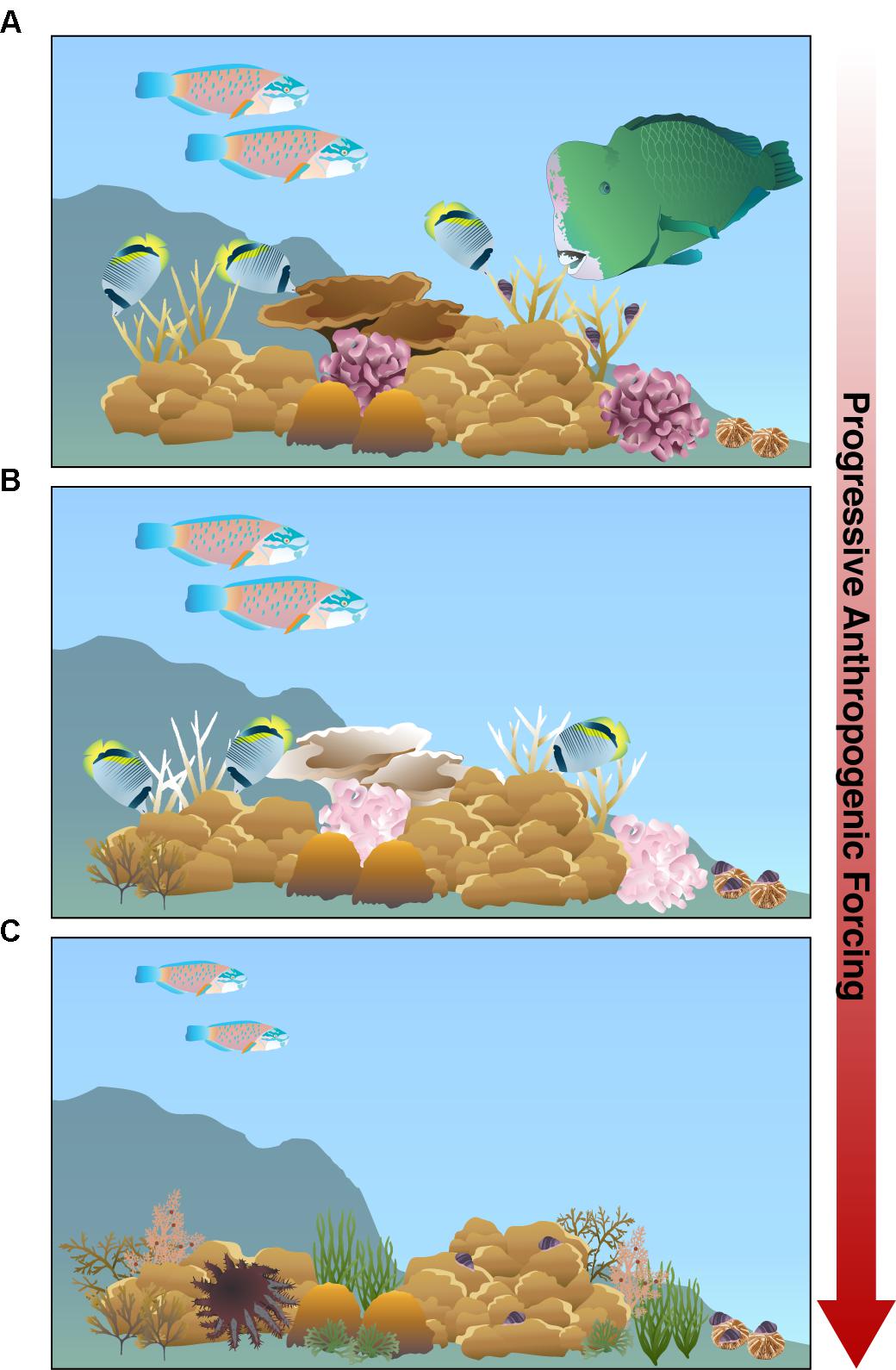
Figure 4. Modern scleractinian reefs with progressive anthropogenic pressure will experience a shift from (A) pristine reefs with a high biodiversity of reef-building corals and fishes to degraded reefs. (B) Under moderate anthropogenic forcing, temperature-sensitive corals (e.g., Acropora and Pocillopora) experience bleaching, inducing obligate butterflyfishes and corallivorous gastropods, Drupella spp., to shift prey preferences to massive Porites and fungiid corals, respectively. The large excavating parrotfish Bolbometopon muricatum is removed due to fishing pressure, reducing the overall corallivorous fish biomass on the reef. (C) As anthropogenic pressures increase, coral composition shifts to more tolerant coral species, like massive Porites. Specialized, obligate corallivorous fishes disappear from the reef because preferred coral species die out. Overfishing removes larger fishes, including invertivores and terminal phase parrotfishes, resulting in smaller average sized parrotfishes and giving way for increased macroalgae colonization. Moreover, overfishing may release invertebrate corallivores from predation pressure, increasing corallivorous gastropod and Acanthaster spp. populations that may hinder remaining coral recovery and compromise reef resilience to these synergistic stressors.
As the pressure from anthropogenic forcing accumulates, thermally sensitive coral species will likely disappear from reefs along with their specialized corallivores (Figure 4C). The corals that remain will likely have compromised wound healing, growth, and fecundity due to chronic thermal stress and the increasingly unfavorable energetics under declining ocean pH. The cumulative effects of local stressors, such as overfishing and nutrient pollution, will lower corallivorous fish biomass on reefs, reduce average parrotfish size, and fuel invertebrate corallivore population growth that may prevent coral recovery. The increase in nutrient pollution, sedimentation, and algal abundance on these future reefs will likely contribute to corallivore-mediated propagation of coral diseases.
However, the mechanisms underlying many of the interactions between corallivory and anthropogenic stressors are still unclear. As coral cover continues to decline globally, the effect of corallivory on coral health and reef community dynamics will become more imperative to understand. Given that ∼60% of global coral reef management agencies are controlling corallivore populations to some extent (Shaver et al., 2018), investigating the feedbacks between corallivory and increasing stressors is a high priority for informing coral reef conservation and management. Moving forward, we need to improve our understanding of how anthropogenic forcing changes coral-corallivore trophic interactions across scales from coral community dynamics down to coral microbiomes.
Author Contributions
All authors contributed to manuscript writing and revision.
Funding
Funding was provided by a National Science Foundation Graduate Research Fellowship to MR (#1650114) and a Swiss National Science Foundation Fellowship (#P2SKP3171764) to LE. This work was also supported by a National Science Foundation CAREER Grant OCE-1547952 to DB.
Conflict of Interest Statement
The authors declare that the research was conducted in the absence of any commercial or financial relationships that could be construed as a potential conflict of interest.
Acknowledgments
We thank two reviewers for their valuable comments that helped to improve our manuscript. We thank the Integration and Application Network, University of Maryland Center for Environmental Science (ian.umces.edu/imagelibrary/) for the use of images for the conceptual figures. We also thank Corinne Fuchs for insightful comments on the manuscript.
References
Aeby, G. S. (1998). A digenean metacercaria from the reef coral, Porites compressa, experimentally identified as Podocotyloides stenometra. J. Parasitol. 84, 1259–1261. doi: 10.2307/3284684
Aeby, G. S. (2002). Trade-offs for the butterflyfish, Chaetodon multicinctus, when feeding on coral prey infected with trematode metacercariae. Behav. Ecol. Sociobiol. 52, 158–165. doi: 10.1007/s00265-002-0490-2
Aeby, G. S., and Santavy, D. L. (2006). Factors affecting susceptibility of the coral Montastraea faveolata to black-band disease. Mar. Ecol. Prog. Ser. 318, 103–110. doi: 10.3354/meps318103
Albright, R., Takeshita, Y., Koweek, D. A., Ninokawa, A., Wolfe, K., Rivlin, T., et al. (2018). Carbon dioxide addition to coral reef waters suppresses net community calcification. Nature 555, 516–519. doi: 10.1038/nature25968
Allen, M. R., Barros, V. R., Broome, J., Cramer, W., Christ, R., Church, J. A., et al. (2014). IPCC Fifth Assessment Synthesis Report-Climate Change 2014 Synthesis Report. Geneva: IPCC.
Anderson, K. D., Cantin, N. E., Heron, S. F., Pisapia, C., and Pratchett, M. S. (2017). Variation in growth rates of branching corals along Australia’s Great Barrier Reef. Sci. Rep. 7:13. doi: 10.1038/s41598-017-03085-1
Bellwood, D. R., Hoey, A. S., and Hughes, T. P. (2011). Human activity selectively impacts the ecosystem roles of parrotfishes on coral reefs. Proc. R. Soc. B Biol. Sci. 279, 1621–1629. doi: 10.1098/rspb.2011.1906
Berumen, M. L., and Pratchett, M. S. (2006). Recovery without resilience: persistent disturbance and long-term shifts in the structure of fish and coral communities at Tiahura Reef, Moorea. Coral Reefs 25, 647–653. doi: 10.1007/s00338-006-0145-2
Bonesso, J. L., Leggat, W., and Ainsworth, T. D. (2017). Exposure to elevated sea-surface temperatures below the bleaching threshold impairs coral recovery and regeneration following injury. PeerJ 5:21. doi: 10.7717/peerj.3719
Brodie, J., Devlin, M., and Lewis, S. (2017). Potential enhanced survivorship of crown of thorns starfish larvae due to near-annual nutrient enrichment during secondary outbreaks on the central mid-shelf of the Great Barrier Reef. Aust. Divers. 9, 17. doi: 10.3390/d9010017
Brooker, R. M., Brandl, S. J., and Dixson, D. L. (2016). Cryptic effects of habitat declines: coral-associated fishes avoid coral-seaweed interactions due to visual and chemical cues. Sci. Rep. 6:8. doi: 10.1038/srep18842
Brooker, R. M., Jones, G. P., and Munday, P. L. (2013). Within-colony feeding selectivity by a corallivorous reef fish: foraging to maximize reward? Ecol. Evol. 3, 4109–4118. doi: 10.1002/ece3.778
Brooker, R. M., Sih, T. L., and Dixson, D. L. (2017). Contact with seaweed alters prey selectivity in a coral-feeding reef fish. Mar. Ecol. Prog. Ser. 580, 239–244. doi: 10.3354/meps12317
Bulleri, F., Couraudon-Reale, M., De Loma, T. L., and Claudet, J. (2013). Variability in the effects of macroalgae on the survival and growth of corals: the consumer connection. PLoS One 8:7. doi: 10.1371/journal.pone.0079712
Burkepile, D. E. (2012). Context-dependent corallivory by parrotfishes in a Caribbean reef ecosystem. Coral Reefs 31, 111–120. doi: 10.1007/s00338-011-0824-5
Burmester, E. M., Finnerty, J. R., Kaufman, L., and Rotjan, R. D. (2017). Temperature and symbiosis affect lesion recovery in experimentally wounded, facultatively symbiotic temperate corals. Mar. Ecol. Prog. Ser. 570, 87–99. doi: 10.3354/meps12114
Cameron, C. M., and Edmunds, P. J. (2014). Effects of simulated fish predation on small colonies of massive Porites spp. and Pocillopora meandrina. Mar. Ecol. Prog. Ser. 508, 139–148. doi: 10.3354/meps10862
Caroselli, E., Prada, F., Pasquini, L., Nonnis Marzano, F., Zaccanti, F., Falini, G., et al. (2011). Environmental implications of skeletal micro-density and porosity variation in two scleractinian corals. Zoology 114, 255–264. doi: 10.1016/j.zool.2011.04.003
Chan, S. T., and Donaldson, T. J. (2012). “Bolbometopon muricatum”. The IUCN Red List of Threatened Species. Available at: http://www.iucnredlist.org
Chazottes, V., Hutchings, P., and Osorno, A. (2017). Impact of an experimental eutrophication on the processes of bioerosion on the reef: one tree Island, Great Barrier Reef, Australia. Mar. Pollut. Bull. 118, 125–130. doi: 10.1016/j.marpolbul.2017.02.047
Choat, J. H., Feitosa, C., Ferreira, C. E., Gaspar, A. L., Padovani-Ferreira, B., and Rocha, L. A. (2012). Scarus guacamaia”. The IUCN Red List of Threatened Species 2012. Available at: http://www.iucnredlist.org
Chong-Seng, K. M., Cole, A. J., Pratchett, M. S., and Willis, B. L. (2011). Selective feeding by coral reef fishes on coral lesions associated with brown band and black band disease. Coral Reefs 30, 473–481. doi: 10.1007/s00338-010-0707-1
Clements, C. S., and Hay, M. E. (2015). Competitors as accomplices: seaweed competitors hide corals from predatory sea stars. Proc. R. Soc. B Biol. Sci. 282, 221–229. doi: 10.1098/rspb.2015.0714
Clements, C. S., and Hay, M. E. (2017). Size matters: predator outbreaks threaten foundation species in small marine protected areas. PLoS One 12:14. doi: 10.1371/journal.pone.0171569
Clements, C. S., and Hay, M. E. (2018). Overlooked coral predators suppress foundation species as reefs degrade. Ecol. Appl. 28, 1673–1682. doi: 10.1002/eap.1765
Cole, A. J., Pratchett, M. S., and Jones, G. P. (2008). Diversity and functional importance of coral-feeding fishes on tropical coral reefs. Fish Fish. 9, 286–307. doi: 10.1111/j.1467-2979.2008.00290.x
Cole, A. J., Pratchett, M. S., and Jones, G. P. (2009). Effects of coral bleaching on the feeding response of two species of coral-feeding fish. J. Exp. Mar. Biol. Ecol. 373, 11–15. doi: 10.1016/j.jembe.2009.02.016
Colgan, M. W. (1987). Coral-reef recovery on Guam (Micronesia) after catastrophic predation by Acanthaster planci. Ecology 68, 1592–1605. doi: 10.2307/1939851
Cox, E. F. (1986). The effects of a selective corallivore on growth rates and competition for space between two species of Hawaiian corals. J. Exp. Mar. Biol. Ecol. 101, 161–174. doi: 10.1016/0022-0981(86)90047-X
Cróquer, A., Villamizar, E., and Noriega, N. (2002). Environmental factors affecting tissue regeneration of the reef – building coral Montastraea annularis (Faviidae) at Los Roques National Park. Venezuela. Rev. Biol. Trop. 50, 1055–1065.
D’Angelo, C., and Wiedenmann, J. (2014). Impacts of nutrient enrichment on coral reefs: new perspectives and implications for coastal management and reef survival. Curr. Opin. Environ. Sustain. 7, 82–93. doi: 10.1016/j.cosust.2013.11.029
De’ath, G., Fabricius, K. E., Sweatman, H., and Puotinen, M. (2012). The 27-year decline of coral cover on the Great Barrier Reef and its causes. Proc. Natl. Acad. Sci. U.S.A. 109, 17995–17999. doi: 10.1073/pnas.1208909109
Denis, V., Guillaume, M. M., Goutx, M., De Palmas, S., Debreuil, J., Baker, A. C., et al. (2013). Fast growth may impair regeneration capacity in the branching coral Acropora muricata. PLoS One 8:e72618. doi: 10.1371/journal.pone.0072618
Doney, S. C., Fabry, V. J., Feely, R. A., and Kleypas, J. A. (2009). Ocean acidification: the other CO2 problem. Annu. Rev. Mar. Sci. 1, 169–192. doi: 10.1146/annurev.marine.010908.163834
Doo, S. S., Carpenter, R. C., and Edmunds, P. J. (2018). Obligate ectosymbionts increase the physiological resilience of a scleractinian coral to high temperature and elevated pCO2. Coral Reefs 37, 997–1001. doi: 10.1007/s00338-018-1731-9
Dulvy, N. K., Freckleton, R. P., and Polunin, N. V. C. (2004). Coral reef cascades and the indirect effects of predator removal by exploitation. Ecol. Lett. 7, 410–416. doi: 10.1111/j.1461-0248.2004.00593.x
Dulvy, N. K., and Polunin, N. V. C. (2004). Using informal knowledge to infer human-induced rarity of a conspicuous reef fish. Anim. Conserv. 7, 365–374. doi: 10.1017/s1367943004001519
Dunn, J. G., Sammarco, P. W., and Lafleur, G. (2012). Effects of phosphate on growth and skeletal density in the scleractinian coral Acropora muricata: a controlled experimental approach. J. Exp. Mar. Biol. Ecol. 411, 34–44. doi: 10.1016/j.jembe.2011.10.013
Edmunds, P. J. (2008). The effects of temperature on the growth of juvenile scleractinian corals. Mar. Biol. 154, 153–162. doi: 10.1007/s00227-008-0910-6
Edmunds, P. J., and Lenihan, H. S. (2010). Effect of sub-lethal damage to juvenile colonies of massive Porites spp. under contrasting regimes of temperature and water flow. Mar. Biol. 157, 887–897. doi: 10.1007/s00227-009-1372-1
Edmunds, P. J., and Yarid, A. (2017). The effects of ocean acidification on wound repair in the coral Porites spp. J. Exp. Mar. Biol. Ecol. 486, 98–104. doi: 10.1016/j.jembe.2016.10.001
Emslie, M. J., Pratchett, M. S., and Cheal, A. J. (2011). Effects of different disturbance types on butterflyfish communities of Australia’s Great Barrier Reef. Coral Reefs 30, 461–471. doi: 10.1007/s00338-011-0730-x
Enochs, I. C., and Glynn, P. W. (2017). “Corallivory in the Eastern Pacific,” in Coral Reefs of the Eastern Tropical Pacific: Persistence and Loss in a Dynamic Environment, eds P. W. Glynn, D. P. Manzello, and I. C. Enochs (Dordrecht: Springer), 315–337. doi: 10.1007/978-94-017-7499-4-10
Erftemeijer, P. L. A., Riegl, B., Hoeksema, B. W., and Todd, P. A. (2012). Environmental impacts of dredging and other sediment disturbances on corals: a review. Mar. Pollut. Bull. 64, 1737–1765. doi: 10.1016/j.marpolbul.2012.05.008
Fabricius, K. E. (2005). Effects of terrestrial runoff on the ecology of corals and coral reefs: review and synthesis. Mar. Pollut. Bull. 50, 125–146. doi: 10.1016/j.marpolbul.2004.11.028
Fabricius, K. E. (2011). “Factors determining the resilience of coral reefs to eutrophication: a review and conceptual model,” in Coral Reefs: An Ecosystem in Transition, eds Z. Dubinsky and N. Stambler (Dordrecht: Springer), 493–505. doi: 10.1007/978-94-007-0114-4_28
Food and Agriculture Organization of the United Nations (2014). “FAOSTAT”, in: Fertilizers. Rome: FAO.
Glynn, P. W. (1976). Some physical and biological determinants of coral community structure in the eastern Pacific. Ecol. Monograp. 46, 431–456. doi: 10.2307/1942565
Gordon, S. E., Goatley, C. H. R., and Bellwood, D. R. (2016). Low-quality sediments deter grazing by the parrotfish Scarus rivulatus on inner-shelf reefs. Coral Reefs 35, 285–291. doi: 10.1007/s00338-015-1374-z
Graham, N. A. J. (2007). Ecological versatility and the decline of coral feeding fishes following climate driven coral mortality. Mar. Biol. 153, 119–127. doi: 10.1007/s00227-007-0786-x
Graham, N. A. J., Wilson, S. K., Pratchett, M. S., Polunin, N. V. C., and Spalding, M. D. (2009). Coral mortality versus structural collapse as drivers of corallivorous butterflyfish decline. Biodivers. Conserv. 18, 3325–3336. doi: 10.1007/s10531-009-9633-3
Guillemot, N., Chabanet, P., Kulbicki, M., Vigliola, L., Leopold, M., Jollit, I., et al. (2014). Effects of fishing on fish assemblages in a coral reef ecosystem: from functional response to potential indicators. Ecol. Indicat. 43, 227–235. doi: 10.1016/j.ecolind.2014.02.015
Guzman, H. M., and Lopez, J. D. (1991). Diet of the corallivorous pufferfish Arothron meleagris (Pisces, Tetraodontidae) at Gorgona Island, Colombia. Rev. Biol. Trop. 39, 203–206.
Hall, E. R., Degroot, B. C., and Fine, M. (2015). Lesion recovery of two scleractinian corals under low pH conditions: implications for restoration efforts. Mar. Pollut. Bull. 100, 321–326. doi: 10.1016/j.marpolbul.2015.08.030
Hamman, E. A. (2018). Aggregation patterns of two corallivorous snails and consequences for coral dynamics. Coral Reefs 37, 851–860. doi: 10.1007/s00338-018-1712-z
Henry, L.-A., and Hart, M. (2005). Regeneration from injury and resource allocation in sponges and corals – a review. Int. Rev. Hydrobiol. 90, 125–158. doi: 10.1002/iroh.200410759
Hoegh-Guldberg, O., Mumby, P. J., Hooten, A. J., Steneck, R. S., Greenfield, P., Gomez, E., et al. (2007). Coral reefs under rapid climate change and ocean acidification. Science 318, 1737–1742. doi: 10.1126/science.1152509
Hoegh-Guldberg, O., Poloczanska, E. S., Skirving, W., and Dove, S. (2017). Coral reef ecosystems under climate change and ocean acidification. Front. Mar. Sci. 4:158. doi: 10.3389/fmars.2017.00158
Hoeksema, B. W., Scott, C., and True, J. D. (2013). Dietary shift in corallivorous Drupella snails following a major bleaching event at Koh Tao. Gulf of Thailand. Coral Reefs 32, 423–428. doi: 10.1007/s00338-012-1005-x
Hoey, A. S., and Bellwood, D. R. (2008). Cross-shelf variation in the role of parrotfishes on the Great Barrier Reef. Coral Reefs 27, 37–47. doi: 10.1007/s00338-007-0287-x
Hoey, A. S., and Bonaldo, R. M. (2018). Biology of Parrotfishes. Boca Raton, FL: CRC Press. doi: 10.1201/9781315118079
Holbrook, S. J., Schmitt, R. J., Adam, T. C., and Brooks, A. J. (2016). Coral reef resilience, tipping points and the strength of herbivory. Sci. Rep. 6:11. doi: 10.1038/srep35817
Horwitz, R., and Fine, M. (2014). High CO2 detrimentally affects tissue regeneration of Red Sea corals. Coral Reefs 33, 819–829. doi: 10.1007/s00338-014-1150-5
Horwitz, R., Hoogenboom, M. O., and Fine, M. (2017). Spatial competition dynamics between reef corals under ocean acidification. Sci. Rep. 7:13. doi: 10.1038/srep40288
Hughes, T. P., Anderson, K. D., Connolly, S. R., Heron, S. F., Kerry, J. T., Lough, J. M., et al. (2018). Spatial and temporal patterns of mass bleaching of corals in the Anthropocene. Science 359, 80–83. doi: 10.1126/science.aan8048
Hughes, T. P., Baird, A. H., Bellwood, D. R., Card, M., Connolly, S. R., Folke, C., et al. (2003). Climate change, human impacts, and the resilience of coral reefs. Science 301, 929–933. doi: 10.1126/science.1085046
Hughes, T. P., Barnes, M. L., Bellwood, D. R., Cinner, J. E., Cumming, G. S., Jackson, J. B. C., et al. (2017a). Coral reefs in the Anthropocene. Nature 546, 82–90. doi: 10.1038/nature22901
Hughes, T. P., Kerry, J. T., Alvarez-Noriega, M., Alvarez-Romero, J. G., Anderson, K. D., Baird, A. H., et al. (2017b). Global warming and recurrent mass bleaching of corals. Nature 543, 373–377. doi: 10.1038/nature21707
Hughes, T. P., Rodrigues, M. J., Bellwood, D. R., Ceccarelli, D., Hoegh-Guldberg, O., Mccook, L., et al. (2007). Phase shifts, herbivory, and the resilience of coral reefs to climate change. Curr. Biol. 17, 360–365. doi: 10.1016/j.cub.2006.12.049
Islam, M. S., and Tanaka, M. (2004). Impacts of pollution on coastal and marine ecosystems including coastal and marine fisheries and approach for management: a review and synthesis. Mar. Pollut. Bull. 48, 624–649. doi: 10.1016/j.marpolbul.2003.12.004
Jackson, J., Donovan, M., Cramer, K., and Lam, V. (2014). Status and trends of Caribbean coral reefs. Gland: Global Coral Reef Monitoring Network, IUCN. Available at http://cmsdata.iucn.org/downloads/caribbean_coral_reefs___status_report_1970_2012.pdf
Johnston, L., and Miller, M. W. (2014). Negative indirect effects of neighbors on imperiled scleractinian corals. Coral Reefs 33, 1047–1056. doi: 10.1007/s00338-014-1176-8
Jokiel, P. L., Hunter, C. L., Taguchi, S., and Watarai, L. (1993). Ecological impact of a fresh-water “reef kill” Kaneohe Bay, Oahu, Hawaii. Coral Reefs 12, 177–184. doi: 10.1007/bf00334477
Jones, R., Ricardo, G. F., and Negri, A. P. (2015). Effects of sediments on the reproductive cycle of corals. Mar. Pollut. Bull. 100, 13–33. doi: 10.1016/j.marpolbul.2015.08.021
Kamya, P. Z., Byrne, M., Graba-Landry, A., and Dworjanyn, S. A. (2016). Near-future ocean acidification enhances the feeding rate and development of the herbivorous juveniles of the crown-of-thorns starfish, Acanthaster planci. Coral Reefs 35, 1241–1251. doi: 10.1007/s00338-016-1480-6
Kamya, P. Z., Byrne, M., Mos, B., and Dworjanyn, S. A. (2018). Enhanced performance of juvenile crown of thorns starfish in a warm-high CO2 ocean exacerbates poor growth and survival of their coral prey. Coral Reefs 37, 751–762. doi: 10.1007/s00338-018-1699-5
Kamya, P. Z., Byrne, M., Mos, B., Hall, L., and Dworjanyn, S. A. (2017). Indirect effects of ocean acidification drive feeding and growth of juvenile crown-of-thorns starfish, Acanthaster planci. Proc. R. Soc. B Biol. Sci. 284:8. doi: 10.1098/rspb.2017.0778
Kayal, M., Lenihan, H. S., Pau, C., Penin, L., and Adjeroud, M. (2011). Associational refuges among corals mediate impacts of a crown-of-thorns starfish Acanthaster planci outbreak. Coral Reefs 30, 827–837. doi: 10.1007/s00338-011-0763-1
Kayal, M., Vercelloni, J., Lison De Loma, T., Bosserelle, P., Chancerelle, Y., Geoffroy, S., et al. (2012). Predator crown-of-thorns starfish (Acanthaster planci) outbreak, mass mortality of corals, and cascading effects on reef fish and benthic communities. PLoS One 7:e47363. doi: 10.1371/journal.pone.0047363
Kojis, B. L., and Quinn, N. J. (1981). Aspects of sexual reproduction and larval development in the shallow-water hermatypic coral, Goniastrea australensis (Edwards and Haime, 1857). Bull. Mar. Sci. 31, 558–573.
Koop, K., Booth, D., Broadbent, A., Brodie, J., Bucher, D., Capone, D., et al. (2001). ENCORE: the effect of nutrient enrichment on coral reefs. Synthesis of results and conclusions. Mar. Pollut. Bull. 42, 91–120. doi: 10.1016/s0025-326x(00)00181-8
Kroeker, K. J., Kordas, R. L., Crim, R., Hendriks, I. E., Ramajo, L., Singh, G. S., et al. (2013). Impacts of ocean acidification on marine organisms: quantifying sensitivities and interaction with warming. Glob. Chang. Biol. 19, 1884–1896. doi: 10.1111/gcb.12179
Lenihan, H. S., and Edmunds, P. J. (2010). Response of Pocillopora verrucosa to corallivory varies with environmental conditions. Mar. Ecol. Prog. Ser. 409, 51–63. doi: 10.3354/meps08595
Lenihan, H. S., Holbrook, S. J., Schmitt, R. J., and Brooks, A. J. (2011). Influence of corallivory, competition, and habitat structure on coral community shifts. Ecology 92, 1959–1971. doi: 10.1890/11-0108.1
MacNeil, M. A., Graham, N. A. J., Cinner, J. E., Wilson, S. K., Williams, I. D., Maina, J., et al. (2015). Recovery potential of the world’s coral reef fishes. Nature 520, 341–344. doi: 10.1038/nature14358
McClanahan, T. R. (1994). Coral-eating snail Drupella cornus population increases in Kenyan coral-reef lagoons. Mar. Ecol. Prog. Ser. 115, 131–137. doi: 10.3354/meps115131
McClanahan, T. R., and Muthiga, N. A. (1989). Patterns of predation on a sea urchin, Echinometra mathaei (de Blainville), on Kenyan coral reefs. J. Exp. Mar. Biol. Ecol. 126, 77–94. doi: 10.1016/0022-0981(89)90125-1
Meesters, E. H., and Bak, R. P. (1993). Effects of coral bleaching on tissue regeneration potential and colony survival. Mar. Ecol. Prog. Ser. 96, 189–198. doi: 10.3354/meps096189
Miller, M. W. (2001). Corallivorous snail removal: evaluation of impact on Acropora palmata. Coral Reefs 19, 293–295. doi: 10.1007/pl00006963
Miller, M. W., and Hay, M. E. (1998). Effects of fish predation and seaweed competition on the survival and growth of corals. Oecologia 113, 231–238. doi: 10.1007/s004420050373
Moberg, F., and Folke, C. (1999). Ecological goods and services of coral reef ecosystems. Ecol. Econ. 29, 215–233. doi: 10.1016/s0921-8009(99)00009-9
Mora, C., Aburto-Oropeza, O., Bocos, A. A., Ayotte, P. M., Banks, S., Bauman, A. G., et al. (2011). Global human footprint on the linkage between biodiversity and ecosystem functioning in reef fishes. PLoS Biol. 9:e1000606. doi: 10.1371/journal.pbio.1000606
Moreira, A. P. B., Tonon, L. A. C., Pereira, C. D. P., Alves, N., Amado, G. M., Francini, R. B., et al. (2014). Culturable heterotrophic bacteria associated with healthy and bleached scleractinian Madracis decactis and the fireworm Hermodice carunculata from the remote St. Peter and St. Paul Archipelago, Brazil. Curr. Microbiol. 68, 38–46. doi: 10.1007/s00284-013-0435-1
Mumby, P. J. (2009). Herbivory versus corallivory: are parrotfish good or bad for Caribbean coral reefs? Coral Reefs 28, 683–690. doi: 10.1007/s00338-009-0501-0
Mumby, P. J., Dahlgren, C. P., Harborne, A. R., Kappel, C. V., Micheli, F., Brumbaugh, D. R., et al. (2006). Fishing, trophic cascades, and the process of grazing on coral reefs. Science 311, 98–101. doi: 10.1126/science.1121129
Mumby, P. J., Harborne, A. R., Williams, J., Kappel, C. V., Brumbaugh, D. R., Micheli, F., et al. (2007). Trophic cascade facilitates coral recruitment in a marine reserve. Proc. Natl. Acad. Sci. U.S.A. 104, 8362–8367. doi: 10.1073/pnas.0702602104
Mumby, P. J., and Steneck, R. S. (2008). Coral reef management and conservation in light of rapidly evolving ecological paradigms. Trends Ecol. Evol. 23, 555–563. doi: 10.1016/j.tree.2008.06.011
Mwachireya, S. A., McClanahan, T. R., Cote, I. M., and Hartwick, B. E. (2016). Increased terrestrial perturbations modify skeletal properties and mechanical strength of hard corals. Environ. Nat. Resourc. Res. 6:153. doi: 10.5539/enrr.v6n4p153
Nicolet, K. J., Chong-Seng, K. M., Pratchett, M. S., Willis, B. L., and Hoogenboom, M. O. (2018a). Predation scars may influence host susceptibility to pathogens: evaluating the role of corallivores as vectors of coral disease. Sci. Rep. 8:5258. doi: 10.1038/s41598-018-23361-y
Nicolet, K. J., Hoogenboom, M. O., Pratchett, M. S., and Willis, B. L. (2018b). Selective feeding by corallivorous fishes neither promotes nor reduces progression rates of black band disease. Mar. Ecol. Prog. Ser. 594, 95–106. doi: 10.3354/meps12525
Nicolet, K. J., Hoogenboom, M. O., Gardiner, N. M., Pratchett, M. S., and Willis, B. L. (2013). The corallivorous invertebrate Drupella aids in transmission of brown band disease on the Great Barrier Reef. Coral Reefs 32, 585–595. doi: 10.1007/s00338-013-1010-8
Oren, U., Brickner, I., and Oya, Y. (1998). Prudent sessile feeding by the corallivore snail Coralliophila violacea on coral energy sinks. Proc. R. Soc. B Biol. Sci. 265, 2043–2050. doi: 10.1098/rspb.1998.0538
Padovani-Ferreira, B., Floeter, S., Rocha, L. A., Ferreira, C. E., Francini-Filho, R., Moura, R., et al. (2012). “Scarus trispinosus”. The IUCN Red List of Threatened Species 2012. Gland: IUCN.
Palacios, M. M., Muñoz, C. G., and Zapata, F. A. (2014). Fish corallivory on a pocilloporid reef and experimental coral responses to predation. Coral Reefs 33, 625–636. doi: 10.1007/s00338-014-1173-y
Palmer, C. V., and Traylor-Knowles, N. (2012). Towards an integrated network of coral immune mechanisms. Proc. R. Soc. B Biol. Sci. 279, 4106–4114. doi: 10.1098/rspb.2012.1477
Pantsar-Kallio, M., Mujunen, S. P., Hatzimihalis, G., Koutoufides, P., Minkkinen, P., Wilkie, P. J., et al. (1999). Multivariate data analysis of key pollutants in sewage samples: a case study. Anal. Chim. Acta 393, 181–191. doi: 10.1016/s0003-2670(99)00287-1
Pratchett, M. S., Caballes, C. F., Rivera-Posada, J. A., and Sweatman, H. P. A. (2014). “Limits to understanding and managing outbreaks of crown-of-thorns starfish (Acanthaster spp.),” in Oceanography and Marine Biology: An Annual Review, Vol. 52, eds R. N. Hughes, D. J. Hughes, and I. P. Smith (Boca Raton, FL: Crc Press-Taylor & Francis Group), 133–199. doi: 10.1201/b17143-4
Pratchett, M. S., Wilson, S. K., Berumen, M. L., and Mccormick, M. I. (2004). Sublethal effects of coral bleaching on an obligate coral feeding butterflyfish. Coral Reefs 23, 352–356. doi: 10.1007/s00338-004-0394-x
Putnam, H. M., Barott, K. L., Ainsworth, T. D., and Gates, R. D. (2017). The vulnerability and resilience of reef-building corals. Curr. Biol. 27, R528–R540. doi: 10.1016/j.cub.2017.04.047
Raymundo, L. J., Halford, A. R., Maypa, A. P., and Kerr, A. M. (2009). Functionally diverse reef-fish communities ameliorate coral disease. Proc. Natl. Acad. Sci. U.S.A. 106, 17067–17070. doi: 10.1073/pnas.0900365106
Renegar, D. A., Blackwelder, P. L., and Moulding, A. L. (2008). “Coral ultrastructural response to elevated pCO2 and nutrients during tissue repair and regeneration,” in 11th International Coral Reef Symposium, (Fort Lauderdale, FL).
Rinkevich, B. (1996). Do reproduction and regeneration in damaged corals compete for energy allocation? Mar. Ecol. Prog. Ser. 143, 297–302. doi: 10.3354/meps143297
Rinkevich, B., and Loya, Y. (1989). Reproduction in regenerating colonies of the coral Stylophora pistillata. Environ. Q. Ecosyst. Stabil. 4, 257–265.
Robertson, R. (1970). Review of the predators and parasites of stony corals, with special reference to symbiotic prosobranch gastropods. Pac. Sci. 24, 43–54.
Rogers, C. S. (1990). Responses of coral reefs and reef organisms to sedimentation. Mar. Ecol. Prog. Ser. 62, 185–202. doi: 10.3354/meps062185
Rotjan, R. D., and Bonaldo, R. M. (2018). “The good, the bad, and the ugly: parrotfishes as coral predators,” in Biology of Parrotfishes, ed. A. S. Hoey (Boca Raton, FL: CRC Press), 207–224.
Rotjan, R. D., Dimond, J. L., Thornhill, D. J., Leichter, J. J., Helmuth, B., Kemp, D. W., et al. (2006). Chronic parrotfish grazing impedes coral recovery after bleaching. Coral Reefs 25, 361–368. doi: 10.1007/s00338-006-0120-y
Rotjan, R. D., and Lewis, S. M. (2008a). Impact of coral predators on tropical reefs. Mar. Ecol. Prog. Ser. 367, 73–91. doi: 10.3354/meps07531
Rotjan, R. D., and Lewis, S. M. (2008b). Predators selectively graze reproductive structures in a clonal marine organism. Mar. Biol. 156, 569–577. doi: 10.1007/s00227-008-1108-7
Samsuri, A. N., Kikuzawa, Y. P., Taira, D., Sam, S. Q., Sim, W. T., Ng, C. S. L., et al. (2018). The effectiveness of Trapezia cymodoce in defending its host coral Pocillopora acuta against corallivorous Drupella. Mar. Biol. 165:8. doi: 10.1007/s00227-018-3330-2
Saponari, L., Montalbetti, E., Galli, P., Strona, G., Seveso, D., Dehnert, I., et al. (2018). Monitoring and assessing a 2-year outbreak of the corallivorous seastar Acanthaster planci in Ari Atoll, Republic of Maldives. Environ. Monitor. Assess. 190:12. doi: 10.1007/s10661-018-6661-z
Schoepf, V., Grottoli, A. G., Levas, S. J., Aschaffenburg, M. D., Baumann, J. H., Matsui, Y., et al. (2015). Annual coral bleaching and the long-term recovery capacity of coral. Proc. R. Soc. B Biol. Sci. 282:9. doi: 10.1098/rspb.2015.1887
Shantz, A. A., and Burkepile, D. E. (2014). Context-dependent effects of nutrient loading on the coral-algal mutualism. Ecology 95, 1995–2005. doi: 10.1890/13-1407.1
Shantz, A. A., Stier, A. C., and Idjadi, J. A. (2011). Coral density and predation affect growth of a reef-building coral. Coral Reefs 30, 363–367. doi: 10.1007/s00338-010-0694-2
Shaver, E. C., Burkepile, D. E., and Silliman, B. R. (2018). Local management actions can increase coral resilience to thermally-induced bleaching. Nat. Ecol. Evol. 2, 1075–1079. doi: 10.1038/s41559-018-0589-0
Shaver, E. C., Shantz, A. A., McMinds, R., Burkepile, D. E., Thurber, R. L. V., and Silliman, B. R. (2017). Effects of predation and nutrient enrichment on the success and microbiome of a foundational coral. Ecology 98, 830–839. doi: 10.1002/ecy.1709
Sheridan, C., Grosjean, P., Leblud, J., Palmer, C. V., Kushmaro, A., and Eeckhaut, I. (2014). Sedimentation rapidly induces an immune response and depletes energy stores in a hard coral. Coral Reefs 33, 1067–1076. doi: 10.1007/s00338-014-1202-x
Stewart, H. L., Holbrook, S. J., Schmitt, R. J., and Brooks, A. J. (2006). Symbiotic crabs maintain coral health by clearing sediments. Coral Reefs 25, 609–615. doi: 10.1007/s00338-006-0132-7
Stewart, H. L., Price, N. N., Holbrook, S. J., Schmitt, R. J., and Brooks, A. J. (2013). Determinants of the onset and strength of mutualistic interactions between branching corals and associate crabs. Mar. Ecol. Prog. Ser. 493, 155–163. doi: 10.3354/meps10525
Stier, A. C., Gil, M. A., Mckeon, C. S., Lemer, S., Leray, M., Mills, S. C., et al. (2012). Housekeeping mutualisms: do more symbionts facilitate host performance? PLoS One 7:6. doi: 10.1371/journal.pone.0032079
Stier, A. C., Mckeon, C. S., Osenberg, C. W., and Shima, J. S. (2010). Guard crabs alleviate deleterious effects of vermetid snails on a branching coral. Coral Reefs 29, 1019–1022. doi: 10.1007/s00338-010-0663-9
Suren, A. M. (2005). Effects of deposited sediment on patch selection by two grazing stream invertebrates. Hydrobiologia 549, 205–218. doi: 10.1007/s10750-005-5323-7
Sussman, M., Loya, Y., Fine, M., and Rosenberg, E. (2003). The marine fireworm Hermodice carunculata is a winter reservoir and spring-summer vector for the coral-bleaching pathogen Vibrio shiloi. Environ. Microbiol. 5, 250–255. doi: 10.1046/j.1462-2920.2003.00424.x
Sutherland, K. P., Porter, J. W., Turner, J. W., Thomas, B. J., Looney, E. E., Luna, T. P., et al. (2010). Human sewage identified as likely source of white pox disease of the threatened Caribbean elkhorn coral. Acropora palmata. Environ. Microbiol. 12, 1122–1131. doi: 10.1111/j.1462-2920.2010.02152.x
Sutherland, K. P., Shaban, S., Joyner, J. L., Porter, J. W., and Lipp, E. K. (2011). Human pathogen shown to cause disease in the threatened eklhorn coral Acropora palmata. PLoS One 6:e23468. doi: 10.1371/journal.pone.0023468
Sweatman, H. (2008). No-take reserves protect coral reefs from predatory starfish. Curr. Biol. 18, R598–R599. doi: 10.1016/j.cub.2008.05.033
Toledo-Hernandez, C., and Ruiz-Diaz, C. P. (2014). The immune responses of the coral. Invertebrate Surviv. J. 11, 319–328.
Traylor-Knowles, N. (2016). Distinctive wound-healing characteristics in the corals Pocillopora damicornis and Acropora hyacinthus found in two different temperature regimes. Mar. Biol. 163:231. doi: 10.1007/s00227-016-3011-y
Tsang, R. H. L., and Ang, P. (2014). Cold temperature stress and predation effects on corals: their possible roles in structuring a nonreefal coral community. Coral Reefs 34, 97–108. doi: 10.1007/s00338-014-1210-x
Turner, S. J. (1994). “The biology and population outbreaks of the corallivorous gastropod Drupella on Indo-Pacific reefs,” in Oceanography and Marine Biology, Vol. 32: An Annual Review, eds A. D. Ansell, R. N. Gibson, and M. Barnes (London: U C L Press Ltd.), 461–530.
Uthicke, S., Logan, M., Liddy, M., Francis, D., Hardy, N., and Lamare, M. (2015). Climate change as an unexpected co-factor promoting coral eating seastar (Acanthaster planci) outbreaks. Sci. Rep. 5:8. doi: 10.1038/srep08402
Uthicke, S., Pecorino, D., Albright, R., Negri, A. P., Cantin, N., Liddy, M., et al. (2013). Impacts of ocean acidification on early life-history stages and settlement of the coral-eating sea star Acanthaster planci. PLoS One 8:9. doi: 10.1371/journal.pone.0082938
van de Water, J. A., Ainsworth, T. D., Leggat, W., Bourne, D. G., Willis, B. L., and Van Oppen, M. J. (2015a). The coral immune response facilitates protection against microbes during tissue regeneration. Mol. Ecol. 24, 3390–3404. doi: 10.1111/mec.13257
van de Water, J. A., Lamb, J. B., Van Oppen, M. J., Willis, B. L., and Bourne, D. G. (2015b). Comparative immune responses of corals to stressors associated with offshore reef-based tourist platforms. Conserv. Physiol. 3:cov032. doi: 10.1093/conphys/cov032
van de Water, J. A, Leggat, W., Bourne, D. G., Van Oppen, M. J. H., Willis, B. L., and Ainsworth, T. D. (2015c). Elevated seawater temperatures have a limited impact on the coral immune response following physical damage. Hydrobiologia 759, 201–214. doi: 10.1007/s10750-015-2243-z
Van Veghel, M. L. J., and Bak, R. P. M. (1994). Reproductive characteristics of the polymorphic Caribbean reef building coral Montastrea annularis. III. Reproduction in damaged and regenerating colonies. Mar. Ecol. Prog. Ser. 109, 229–233. doi: 10.3354/meps109229
Venera-Ponton, D. E., Diaz-Pulido, G., Mccook, L. J., and Rangel-Campo, A. (2011). Macroalgae reduce growth of juvenile corals but protect them from parrotfish damage. Mar. Ecol. Prog. Ser. 421, 109–115. doi: 10.3354/meps08869
Wear, S. L., and Thurber, R. V. (2015). “Sewage pollution: mitigation is key for coral reef stewardship,” in Year in Ecology and Conservation Biology, eds A. G. Power and R. S. Ostfeld (Oxford: Blackwell Science Publ.), 15–30. doi: 10.1111/nyas.12785
Weber, M., Lott, C., and Fabricius, K. E. (2006). Sedimentation stress in a scleractinian coral exposed to terrestrial and marine sediments with contrasting physical, organic and geochemical properties. J. Exp. Mar. Biol. Ecol. 336, 18–32. doi: 10.1016/j.jembe.2006.04.007
Williams, D. E., and Miller, M. W. (2005). Coral disease outbreak: pattern, prevalence and transmission in Acropora cervicornis. Mar. Ecol. Prog. Ser. 301, 119–128. doi: 10.3354/meps301119
Wilson, S. K., Graham, N. A. J., and Pratchett, M. S. (2014). “Susceptibility of butterflyfish to habitat disturbance: do ’chaets’ ever prosper?,” in Biology of Butterflyfishes, eds M. S. Pratchett, M. L. Berumen, and B. G. Kapoor (Boca Raton, FL: Crc Press-Taylor & Francis Group), 226–245.
Wolf, A. T., and Nugues, M. M. (2013). Synergistic effects of algal overgrowth and corallivory on Caribbean reef-building corals. Ecology 94, 1667–1674. doi: 10.1890/12-0680.1
Zambre, A. M., and Arthur, R. (2018). Foraging plasticity in obligate corallivorous Melon butterflyfish across three recently bleached reefs. Ethology 124, 302–310. doi: 10.1111/eth.12733
Zaneveld, J. R., Burkepile, D. E., Shantz, A. A., Pritchard, C. E., McMindss, R., Payet, J. P., et al. (2016). Overfishing and nutrient pollution interact with temperature to disrupt coral reefs down to microbial scales. Nat. Commun. 7:11833. doi: 10.1038/ncomms11833
Keywords: corallivory, overfishing, nutrient pollution, global climate change, parrotfishes, butterflyfishes, Acanthaster spp.
Citation: Rice MM, Ezzat L and Burkepile DE (2019) Corallivory in the Anthropocene: Interactive Effects of Anthropogenic Stressors and Corallivory on Coral Reefs. Front. Mar. Sci. 5:525. doi: 10.3389/fmars.2018.00525
Received: 31 May 2018; Accepted: 22 December 2018;
Published: 11 January 2019.
Edited by:
Michael Sweet, University of Derby, United KingdomReviewed by:
Douglas Fenner, Independent Researcher, Pago Pago, American SamoaCaroline V. Palmer, Guanacaste Dry Forest Conservation Fund (GDFCF), United States
Copyright © 2019 Rice, Ezzat and Burkepile. This is an open-access article distributed under the terms of the Creative Commons Attribution License (CC BY). The use, distribution or reproduction in other forums is permitted, provided the original author(s) and the copyright owner(s) are credited and that the original publication in this journal is cited, in accordance with accepted academic practice. No use, distribution or reproduction is permitted which does not comply with these terms.
*Correspondence: Mallory M. Rice, bWFsbG9yeW1yaWNlQGdtYWlsLmNvbQ==