- 1Horn Point Laboratory, University of Maryland Center for Environmental Science, Cambridge, MD, United States
- 2Department of Fisheries and Wildlife, Oregon State University, Corvallis, OR, United States
- 3Chesapeake Biological Laboratory, University of Maryland Center for Environmental Science, Solomons, MD, United States
Hypoxia, triggered in large part by eutrophication, exerts widespread and expanding stress on coastal ecosystems. Hypoxia is often specifically defined as water having dissolved oxygen (DO) concentrations < 2 mg L−1. However, DO concentration alone is insufficient to categorize hypoxic stress or predict impacts of hypoxia on zooplankton and fish. Hypoxic stress depends on the oxygen supply relative to metabolic demand. Water temperature controls both oxygen solubility and the metabolic demand of aquatic ectotherms. Accordingly, to assess impacts of hypoxia requires consideration of effects of temperature on both oxygen availability and animal metabolism. Temperature differences across ecosystems or across seasons or years within an ecosystem can dramatically impact the severity of hypoxia even at similar DO concentrations. Living under sub-optimum DO can reduce temperature-dependent metabolic efficiencies, prey capture efficiency, growth and reproductive potential, thus impacting production and individual zooplankton and fish fitness. Avoidance of hypoxic bottom water can reduce or eliminate low-temperature thermal refuges for organisms and increase energy demands and respiration rates, and potentially reduce overall fitness if alternative habitats are sub-optimal. Moreover, differential habitat shifts among species can shift predator-prey abundance ratios or interactions and thus modify food webs. For example, more tolerant zooplankton prey may use hypoxic waters as a refuge from fish predation. In contrast, zooplankton avoidance of hypoxic bottom waters can result in prey aggregations at oxyclines sought out by fish predators. Hypoxic conditions that affect spatial ecology can drive taxonomic and size shifts in the zooplankton community, affecting foraging, consumption and growth of fish. Advances in understanding the ecological effects of low DO waters on pelagic zooplankton and fish and comparisons among ecosystems will require development of generic models that estimate the oxygen demand of organisms in relation to oxygen supply which depends on both DO and temperature. We provide preliminary analysis of a metric (Oxygen Stress Level) which integrates oxygen demand in relation to oxygen availability for a coastal copepod and compare the prediction of oxygen stress to actual copepod distributions in areas with hypoxic bottom waters.
Introduction
Dissolved oxygen (DO) has been declining in coastal waters since the middle of the 20th century (Diaz and Rosenberg, 2008; Vaquer-Sunyer and Duarte, 2008; Rabalais et al., 2009; Breitburg et al., 2018). Hypoxia occurs naturally in many marine and freshwater systems that are characterized by high productivity and stratification (Rabalais et al., 2010). However, human activities such as intensive agriculture practices, land use changes, and point-source nutrient loading have caused the frequency, magnitude and extent of coastal hypoxia to increase (Turner et al., 2008; Bianchi et al., 2010).
Seasonal deoxygenation of sub-pycnocline coastal waters is driven primarily by nutrient stimulation of largely ungrazed phytoplankton blooms that decay below a stratified water column. These blooms sink or are eaten and processed by zooplankton, and are subsequently decomposed by microbial activity, consuming much of the available DO in the lower water column isolated from aeration (Malone, 1991; Nixon, 1995; Howarth et al., 1996; Diaz and Rosenberg, 2008). Since 1950, more than 500 sites in coastal waters have reported DO < 2 mg l−1, with fewer than 10 percent of these systems reporting such low DO values prior to 1950 (Diaz and Rosenberg, 2008; Vaquer-Sunyer and Duarte, 2008; Isensee et al., 2015). While more observations have likely contributed to this increase in reporting, a greater diversity of coastal hypoxic sites suggests that increased eutrophication is primarily responsible for expanding coastal hypoxia (Vaquer-Sunyer and Duarte, 2008). Coastal zooplankton and fish do not appear to have developed specific physiological adaptations to low DO in these new coastal systems experiencing seasonal hypoxia (Childress and Seibel, 1998; Dam, 2013; McBryan et al., 2013). Instead, these organisms suffer direct mortality, or reside in the low DO waters and tolerate some deleterious effects, or avoid low DO bottom waters through behavioral avoidance. Global warming can increase coastal hypoxia by increasing water column density stratification, decreasing oxygen solubility, and increasing the oxygen demand of ectotherms (Altieri and Gedan, 2014).
Hypoxia is often specifically defined as water having DO concentrations < 2 mg L−1 (at 18°C in seawater = 1.5 ml L−1 = 5.6 kPa oxygen partial pressure), although more biologically relevant definitions are required to define its impacts (Davis, 1975; Vaquer-Sunyer and Duarte, 2008; Breitburg et al., 2009; Ekau et al., 2010; Verberk et al., 2011; Elliott et al., 2013). Physiologists usually express DO in terms of partial pressure because oxygen availability to aquatic organisms is dependent on the rate of diffusion across integuments or gills and is controlled by the partial pressure of O2 (mm Hg or kPa). In contrast, aquatic ecologists and oceanographers usually express DO in terms of concentration (mg L−1 or ml L−1).
Temperature directly influences oxygen solubility in seawater and thus DO concentration and oxygen partial pressure as well as the metabolic demand of aquatic ectotherms, yet is often overlooked as a controlling factor in hypoxic assessments. A meta-analysis by Vaquer-Sunyer and Duarte (2011) suggests that the survival time of benthic macrofauna in low DO is reduced with increasing temperature and that the DO concentration which results in mortality increases. Thus, in order to effectively assess the impacts of hypoxic stress, it is necessary to consider the effects of temperature on both oxygen availability and animal metabolism. We contend that temperature is an essential component defining hypoxic conditions. Temperature differences across ecosystems or across seasons or years within an ecosystem can dramatically impact the severity of hypoxia even at similar DO concentrations.
Although hypoxic events have been shown to cause mortality of more sedentary benthic species and crabs (Grantham et al., 2004), less is known about the impact of hypoxia on pelagic species, which is the focus of this paper. Pelagic species have behavioral capabilities to generally avoid hypoxic water and must either move out of the way or suffer the consequences of reduced oxygen (Ekau et al., 2010). The physiological and ecological consequences of moving to non-hypoxic, perhaps adjacent habitats are important to understand broader impacts of hypoxia at the species and ecosystem level. Physiological consequences include changes in energy demand, respiration rates and overall fitness if adjacent habitats have different temperatures. Ecological consequences include shifts in densities and spatial overlaps of predators and prey that modify food webs.
Effects of Low Dissolved Oxygen on Zooplankton and Fish Metabolism and Vital Rates
Research on ecological effects of hypoxia in coastal systems typically has considered DO in terms of the concentration of oxygen in water (ml L−1 or mg L−1). However, it is also essential to consider the partial pressure and diffusivity of oxygen in water because these properties determine the rate of oxygen supply and, ultimately, the rate of oxygen uptake by organisms (Verberk et al., 2011). The solubility of oxygen in water (i.e., concentration at atmospheric equilibrium) declines at both higher temperatures and higher salinities. Considering potential biological and ecological effects of hypoxia on coastal zooplankton and fish, reduced rates of oxygen uptake are ultimately responsible for direct adverse effects such as reduced growth and increased mortality. Under low oxygen conditions (with oxygen supply low relative to oxygen demand), aerobic respiration may not be fully supported by the consequent lower oxygen uptake rates, and respiration becomes oxygen limited (Gnaiger, 1991; Childress and Seibel, 1998; McAllen et al., 1999; Pörtner and Knust, 2007; Seibel, 2011; Elliott et al., 2013). As a result, zooplankton and fish residing in hypoxic water must either implement specific adaptations to maintain the rate of oxygen uptake, make up the energy deficit through anaerobic respiration, or reduce their energy demand and oxygen requirements by lowering metabolic rate such as through reduced activity (Gnaiger, 1991; Childress and Seibel, 1998; McAllen et al., 1999; Perry, 2011; Seibel, 2011; Friedman et al., 2012; Elliott et al., 2013). In aquatic ectotherms, temperature controls respiration and metabolic rates and thus the ambient temperature of low oxygen water will affect the specific response rates of an organism.
Two useful metrics to assess the effects of low DO on zooplankton and fish are the critical (Pcrit) and lethal (Pleth) oxygen thresholds (Prosser and Brown, 1962; Connett et al., 1990; Gnaiger, 1991; Childress and Seibel, 1998; McAllen et al., 1999; Pörtner and Knust, 2007; Seibel, 2011; Elliott et al., 2013). When maximum potential respiration rate drops below an organism’s “target” respiration rate (Pcrit), respiration rate becomes limited by oxygen supply, and sub-lethal effects of hypoxia can be expected. When the oxygen supply drops below an organism’s “basal” respiration rate (Pleth), acute lethal effects of hypoxia can be expected. Both of these thresholds reflect the balance between oxygen supply to the organism, a function of the rate of molecular diffusion, and oxygen demand by the organism, a function of respiration rate (and ultimately metabolic rate). Typically, these are expressed in terms of oxygen partial pressures. According to Fick’s Law for diffusion across a membrane, oxygen uptake and maximum potential aerobic respiration rate will depend on oxygen supply as governed by the external (environmental) oxygen solubility, partial pressure, and diffusivity (Verberk et al., 2011). Thus, corresponding critical and lethal oxygen supply thresholds can be defined that account for oxygen solubility, partial pressure and diffusivity. These thresholds will depend on an organism’s non-oxygen-limited (target) respiration rate, and the lowest sustainable (basal) respiration rate, respectively (Elliott et al., 2013). For coastal zooplankton and fish lacking specialized adaptations to live under low environmental DO, oxygen uptake is more or less constant at the target level when pO2 is above Pcrit, then declines linearly as DO declines to below the Pcrit level (Figure 1). Because zooplankton and most newly hatched larval fish lack gills, their oxygen uptake is a function of diffusion through the body surface. Under low oxygen conditions relative to oxygen requirements, species with a higher surface/volume ratio would be favored because of their greater oxygen diffusion potential.
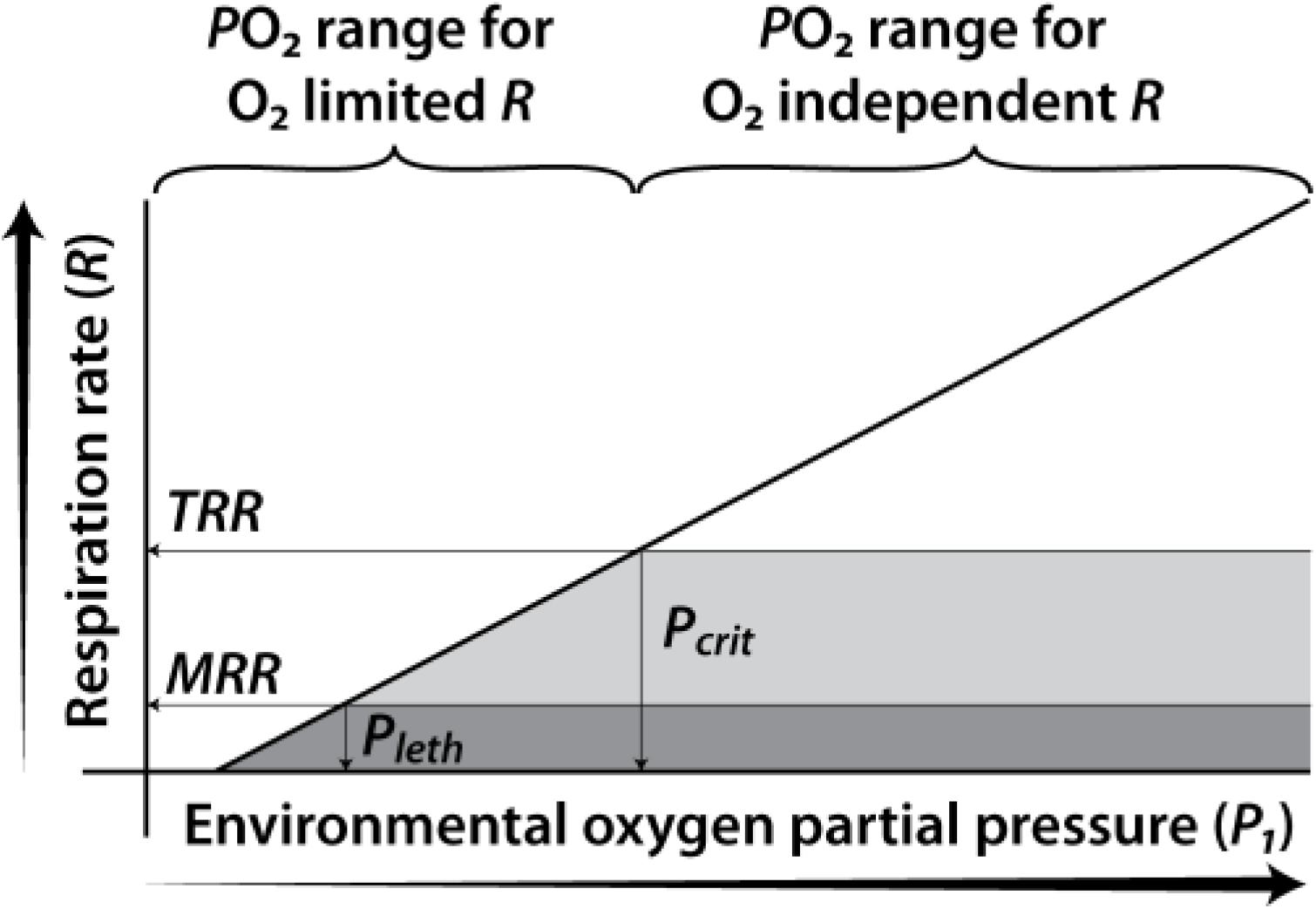
Figure 1. Adapted from Elliott et al. (2013). Relationship between an organism’s respiration rate and the environmental oxygen partial pressure. Target Respiration Rate (TRR); Minimum survivable Respiration Rate (MRR), critical oxygen partial pressure (Pcrit) and lethal oxygen partial pressure (Pleth) are shown.
It has been established for decades that there is a critical oxygen level for fishes at which metabolism and other processes are limited by oxygen. The use of Pcrit as a measure of the relative tolerance of a fish to low oxygen also has a long history (Prosser and Brown, 1962; Chapman et al., 2002; Nilsson and Östlund-Nilsson, 2008; Mandic et al., 2009; Richards, 2011; Speers-Roesch et al., 2013; and see review by Rogers et al., 2016). Early fish literature often referred to Pcrit as the ‘incipient limiting tension or level’ (Davis, 1975). The classic work of Fry (1957) concludes “Any reduction of the oxygen content below the level where the active metabolic rate begins to be restricted is probably unfavorable to the species concerned. From the ecological point of view, the ‘incipient limiting level’ (the critical level under conditions of activity) can be taken as the point where oxygen content becomes unsuitable.”
Specific adaptations that help maintain oxygen uptake under low DO (or increasing oxygen demand) include increased ventilation of the respiratory surfaces (e.g., gills), increased heart rate, reduced activity and production of high-affinity oxygen uptake molecules (Herreid, 1980; Childress and Seibel, 1998; Pörtner and Knust, 2007; Richards, 2009; Seibel, 2011). These types of responses are common among zooplankton and fishes in oceanic oxygen minimum zones, which likely have co-evolved with low DO concentrations for thousands of years. However, among coastal organisms, exposure to hypoxia is generally more ephemeral (seasonal) and in many cases is a relatively new stressor associated with eutrophication (Diaz and Rosenberg, 2008). Consequently, planktivorous fish and their zooplankton prey do not appear to have developed specific physiological adaptations to hypoxia in typical coastal systems (Childress and Seibel, 1998; Dam, 2013; McBryan et al., 2013). Instead, these organisms must either avoid hypoxia through behavioral mechanisms or reside in stressful hypoxic water.
With 33 times less oxygen and 3 × 105 times lower diffusion rates in water (at saturation) compared to air, DO can be the proximate cause of reduced growth in zooplankton and fish (e.g., Prosser and Brown, 1962; Pörtner, 2010; Bertrand et al., 2011; Verberk et al., 2011). Warming seas will result in increased oxygen demand by zooplankton and fishes which can result in shifts in spatial distributions, reduced body size and changes in species composition (e.g., Beaugrand et al., 2002; Pörtner and Knust, 2007; Cheung et al., 2012; Deutsch et al., 2015). Because warming temperatures and oxygen limitation are inextricably linked, investigating the impacts of low DO on coastal zooplankton and fishes can provide valuable insights into future ocean warming effects on pelagic ecosystems.
Low Oxygen Impacts on Coastal Zooplankton
The general observation of reduced copepod abundances (integrated over the entire water column) in hypoxic water columns (Roman et al., 1993; Keister et al., 2000; Kimmel et al., 2012) suggests lower population growth, greater copepod mortality, predation and/or emigration in water columns with hypoxic bottom waters. Laboratory experiments have demonstrated that copepod survival, growth rate, egg production and ingestion rate all decline with lowered oxygen availability. Low DO reduces the survival of copepods (Vargo and Sastry, 1977; Roman et al., 1993; Stalder and Marcus, 1997; Marcus et al., 2004; Richmond et al., 2006). In laboratory experiments, Marcus et al. (2004) and Richmond et al. (2006) demonstrated that Acartia egg production and population growth rate were reduced in low DO waters (0.7 and 1.5 ml O2 L−1) compared to normoxic (DO > 2 mg L−1) controls. Low DO conditions have also been shown to reduce the ingestion rates of copepods fed in laboratory experiments (Elliott et al., 2013), as well as reduce their escape response (Decker et al., 2004).
Hypoxia can directly affect the earliest life stages of copepods. Copepods can be divided into two groups with respect to reproduction: those that carry their eggs until hatching (brooders) and those that release their eggs (broadcast spawners). Copepod eggs are denser than seawater and thus sink to bottom waters where they can be affected by low DO conditions. Copepods in estuarine and coastal waters produce two types of eggs: diapause (resting) eggs which must complete a dormancy (refractory) period before hatching and non-diapause (subitaneous) eggs which hatch within hours-days of being spawned, depending on temperature (Grice and Marcus, 1981). Low DO has been shown in laboratory experiments to severely reduce the hatching success of non-diapause copepod eggs (Lutz et al., 1992; Roman et al., 1993; Marcus et al., 1994, 1997; Marcus and Lutz, 1994; Invidia et al., 2004; Richmond et al., 2006). The eggs of dominant coastal copepods have sinking rates which range from 15 to 35 m d−1 (Uye, 1980; Knutsen et al., 2001; Jiang et al., 2006). Thus, if hatching times are sufficiently long, non-diapause (subitaneous) copepod eggs may sink into low DO bottom waters in shallow systems (Tang et al., 1998; Jiang et al., 2006). Low DO conditions can sometimes induce dormancy in these non-diapause eggs, which is reversed with increases in DO (Katajisto, 2004). Thus, the potential exists for non-diapause eggs to hatch if hypoxia dissipates or they are resuspended into normoxic waters. The length of time that non-diapause eggs can withstand hypoxia/anoxia and remain viable to hatch varies with copepod species and abiotic conditions such as temperature and hydrogen sulfide (Katajisto, 2004; Hansen and Drillet, 2013; Broman et al., 2017). Copepod diapause eggs have an obligatory “refractory phase” and sink to the sediment which is typically anoxic below a depth of several millimeters. Diapause eggs can withstand considerable periods of anoxia and toxic hydrogen sulfide (Marcus, 2001). In general, non-diapause eggs are less able to withstand prolonged exposure to low oxygen than diapause eggs because of their higher metabolic demand (Hansen and Drillet, 2013). Coastal and estuarine waters which experience seasonal hypoxia in bottom waters may develop a significant “egg bank” of copepod eggs which, if reaerated, could hatch and make important contributions to copepod populations and their predators. A combined laboratory and modeling study by Broman et al. (2017) demonstrated that re-oxygenation of anoxic sediments activated copepod egg hatching, indicating that re-oxygenation could result in substantial contributions to copepod populations in the Baltic, and perhaps in other similar systems.
Generally, hypoxic bottom waters truncate zooplankton vertical migration behaviors, reducing the excursion distance (Roman et al., 1993, 2012; Pierson et al., 2009a, 2017; Keister and Tuttle, 2013). For example, zooplankton in the Gulf of Mexico avoided hypoxic near-bottom waters in their diel vertical migrations and the median depth of their daytime distribution was 7 m higher in the water column compared to daytime distributions of zooplankton in water columns with no hypoxic bottom waters (Roman et al., 2012). In the Chesapeake Bay, moderate levels of hypoxia (Pcrit > DO > Pleth) coincided with stronger migration responses than did lethal or fully oxygenated conditions (Pierson et al., 2017). Thus, along with food levels (Huntley and Brooks, 1982; Roman et al., 1988; Pearre, 2000; Hays et al., 2001) and predators (Ohman, 1988; Bollens and Frost, 1989; Frost and Bollens, 1992), the presence of hypoxic bottom waters can influence diel shifts in the vertical distribution of neritic copepods (e.g., Roman et al., 1993; Keister et al., 2000; Qureshi and Rabalais, 2001; North and Houde, 2004). Decker et al. (2003) reported that behavioral responses of copepods to hypoxia may differ depending on environmental history: Acartia tonsa from Chesapeake Bay appeared to avoid hypoxic bottom waters in laboratory mesocosms whereas A. tonsa from Florida, not typically exposed to hypoxia, did not avoid low-oxygen concentrations in the same mesocosms. Temporal analysis of vertical distributions of copepods has suggested that individuals display considerable variation in their vertical movements, often taking brief (hours) excursions between the surface mixed layer and sub-pycnocline depths (Pearre, 2000; Hays et al., 2001; Pierson et al., 2009a). Thus, it may be common for copepods in coastal waters with hypoxia to experience a range of oxygen concentrations over the day.
Zooplankton may change their vertical position in the water column to avoid low DO bottom waters. However, the vertical compression of their distribution to the upper water column can increase their vulnerability to predation by visually feeding fish and thus alter food-web processes (e.g., Pothoven et al., 2012). Vertical movements can increase zooplankton concentrations or visibility and move them into warmer temperatures with a different array of predators. In general, depth-stratified zooplankton sampling has shown that copepod abundances are higher in the surface mixed layer and within the pycnocline compared to hypoxic bottom water in coastal environments (Roman et al., 1993; Keister et al., 2000; North and Houde, 2004; Kimmel et al., 2009; Pierson et al., 2009b, 2017; Keister and Tuttle, 2013). However, this is not always the case (Qureshi and Rabalais, 2001; Taylor and Rand, 2003) and even if most zooplankton are above the oxycline, significant amounts can occur in hypoxic near-bottom water for part of the day (Roman et al., 1993; North and Houde, 2004; Taylor et al., 2007; Keister and Tuttle, 2013; Pierson et al., 2017). In most coastal and estuarine waters, copepods exhibit diel vertical migrations, presumably to reduce predation by visual feeders by residing at depth during the day, and returning to the surface layer at night (Cahoon, 1981; Roman et al., 1988). Bottom-water hypoxia can clearly disrupt vertical migration behavior. For example, when a wind event mixed the water column and re-aerated hypoxic bottom water in Chesapeake Bay, copepods migrated to a deeper depth during the day (Roman et al., 1993).
Low oxygen waters have been associated with changes in zooplankton species assemblages. These assemblage shifts could be due to direct effects of low DO on the metabolic functions of the animal or indirect effects due to selective predation by zooplanktivores. Long-term seasonal presence of bottom-water hypoxia may favor copepod species which brood their eggs as compared to broadcast spawners whose eggs would sink into anoxic/hypoxic bottom waters. For example, increased eutrophication and low oxygen bottom waters have resulted in an increase in the abundance of the small, egg-carrying copepod Oithona davisae in Tokyo Bay and decline in the occurrence of Acartia omorii and Paracalanus sp., copepods that release their eggs into the water column (Uye, 1994). A similar decline in the broadcast egg spawner, Acartia tonsa has been associated with the increase in bottom water hypoxia in Chesapeake Bay (Kimmel et al., 2012). Egg-carrying copepods in the Gulf of Mexico showed more relative abundance in water columns with hypoxic bottom waters compared to nearby well-oxygenated water columns (Elliott et al., 2012).
In general, smaller copepods have a higher surface to volume ratio which would favor their oxygen uptake over larger copepods in hypoxic waters and warmer waters where oxygen solubility is lower and oxygen demand (respiration) is higher. In laboratory experiments, Stalder and Marcus (1997) showed that the smaller copepod, Acartia tonsa, survived low oxygen conditions better than the larger Labidocera aestiva and Centropages hamatus. In similar types of laboratory experiments, Roman et al. (1993) found that the smaller copepod Oithona colcarva survived low oxygen conditions better than the larger Acartia tonsa. In addition to species differences there may be gender differences in tolerance to hypoxia. Pierson et al. (2017) reported that male Acartia tonsa were found in lower oxygen waters than females in Chesapeake Bay. Males are smaller (Pierson et al., 2017) and thus have a higher surface/volume ratio than females that may also have a higher respiratory demand because of egg production (e.g., Castellani and Altunbas, 2014).
Low Oxygen Impacts on Coastal Pelagic Fishes
Exploration of the impacts of low DO on fish date back over a century (e.g., Shelford and Allee, 1913; Wells, 1913) which, early on, documented that fish can often behaviorally avoid low DO and that the tolerance of fish to low DO can vary with species and body size (e.g., Fry, 1957; Brett, 1964, 1970). Research on the physiological response of fish to DO is extensive and research on the impact of hypoxia on fish in coastal ecosystems has exploded in the last few decades (see reviews by Pauly, 2010; Richards, 2011; Rogers et al., 2016). Hypoxia directly affects fish physiological rates and fish must either move out of the way or suffer the consequences of reduced DO. The consequences of moving to non-hypoxic environments, while often poorly understood, is a key to understanding hypoxic impacts at the species or ecosystem level. To predict/forecast how hypoxia-driven changes in habitat conditions will affect fish populations, we need to understand how vital habitat requirements of a species are related to hypoxia and other environmental drivers.
Physiological responses of fishes to low DO have been well studied under laboratory conditions (e.g., Rogers et al., 2016). Low DO has been shown to reduce fish consumption, growth, reproduction and recruitment in the laboratory (e.g., Aku and Tonn, 1997; Breitburg et al., 1999; Taylor and Miller, 2001; Shang and Wu, 2004; Craig and Crowder, 2005; Eby et al., 2005; Diaz and Breitburg, 2009; Brandt et al., 2011; Thomas and Rahman, 2012; Zhang et al., 2014) and increases susceptibility to other stressors (Breitburg et al., 2009). Reductions in abundance of sensitive, less-mobile fish species may occur due to fish kills (Graham et al., 2004; Thronson and Quigg, 2008). Effects may be direct via increased mortality through prolonged exposure to low DO (Breitburg et al., 1999, 2003; Turner, 2001; Diaz and Breitburg, 2009) or indirect via reduction of benthic (Turner, 2001) and water column (Wang, 1998; Breitburg et al., 1999; Chesney et al., 2000; Turner, 2001) habitat availability and alteration of food web structure (Graham, 2001). However, such research rarely considers the interactive effects of temperature and low DO on the fish.
If ambient DO is below the Pcrit level, fish can respond to lowered oxygen in a number of ways that range from avoiding the hypoxic region to increasing oxygen intake (increased ventilation) to physiological shifts (e.g., Gamperl and Driedzic, 2009; Richards, 2009; Wells, 2009; Speers-Roesch et al., 2012) to reducing metabolic costs (e.g., lowered appetite, reduced activity levels). Eggs and larvae have fewer options; eggs can only respond passively while larvae can migrate vertically or adjust their feeding and activity levels. Most responses to hypoxia will have consequences for consumption and growth rates (e.g., Wang et al., 2009) and thus ultimately survival rates and/or reproduction (Craig and Crowder, 2005; Beauchamp et al., 2007; Searcy et al., 2007; Daewel et al., 2008; Wu, 2009).
Hypoxia can drive changes in spatial distribution of coastal and estuarine fishes (Craig and Crowder, 2005; Hazen et al., 2009; Ludsin et al., 2009; Zhang et al., 2009). Hypoxia-induced changes in food webs may result from changes in the abundances of some species and/or the distributional overlap of predators and prey (Breitburg et al., 1997; Ekau et al., 2010). Diets of fishes can differ in hypoxic water as shown for Atlantic bumper in the Gulf of Mexico (Glaspie et al., 2018). Some fishes may even benefit from hypoxia if their prey are forced into more vulnerable predatory habitats as suggested for Chesapeake Bay where striped bass may benefit from concentration of bay anchovy prey in the well-oxygenated mixed layer (Costantini et al., 2008). Clearly, these effects differ across species, life stages, and ecosystems and are particularly dependent on the prevailing water temperatures and species-specific bioenergetics tolerances (Brandt et al., 2009).
Whether effects of hypoxia on fish populations are positive or negative is likely species-specific and ecosystem-dependent (Breitburg et al., 1997, 2001; Costantini et al., 2008) and also depend on the severity of the low oxygen coupled to the prevailing temperatures. For example, species less tolerant of hypoxia may be forced to reside where habitat (e.g., food, refuge from predators, and temperature) critical for growth, reproduction, and/or survival are suboptimal (Roman et al., 1993; Aku et al., 1997; Keister et al., 2000; Wannamaker and Rice, 2000; Costantini et al., 2008; Ludsin et al., 2009; Wu, 2009) which may reduce individual consumption and fitness, ultimately leading to lower population sizes (Aku and Tonn, 1997; Wu, 2002; Breitburg et al., 2003). In contrast, species more tolerant of low DO could prosper in hypoxic waters, using it as a refuge from predation or for feeding (Breitburg et al., 2001; Klumb et al., 2004; Prince and Goodyear, 2006; Prince et al., 2010; Vejøík et al., 2016). Some fish predators may benefit if low DO forces their prey to move into habitats easily exploited by the predators (e.g., Costantini et al., 2008; Brandt et al., 2011).
It has been demonstrated that year-class success in fishes is largely determined during the larval or early juvenile stage, and that variability in vital rates (e.g., growth and mortality rates), attributable to a wide variety of environmental stressors, including low DO, can lead to order of magnitude fluctuations in survival during early life (Cowan and Shaw, 2002; Houde, 2008). As such, even subtle changes in survival potential of early life stages may lead to declines in regional fish production if hypoxia affects the distribution and physiological ecology of a significant fraction of the population.
In coastal ecosystems, fish are likely to be most susceptible to the effects of hypoxia during their egg and larval stages (Breitburg, 2002). In coastal upwelling ecosystems subject to variable DO conditions, early life stages of pelagic fish species differ in their distributions, a reflection of species-specific tolerances to low DO (Ekau and Verheye, 2005; Geist et al., 2013, 2015). Pelagic fish eggs may have different exposures to bottom hypoxia, depending on the species, sinking rates and density structure of the water column (Nissling and Vallin, 1996; Ekau and Verheye, 2005). Larvae are generally planktonic and thus are primarily able to migrate vertically in response to hypoxia (Breitburg, 2002). Hypoxia may affect eggs and larvae through direct mortality or indirectly as a consequence of reduced growth and higher mortality resulting from altered spatial distributions, availability of suitable prey for larvae, and susceptibility to predators. Even small changes in daily growth and mortality rates of early life stages can have order of magnitude effects on levels of recruitment (Houde, 1989b, 2016).
Ecosystem Comparisons of Hypoxia Impacts on Coastal Zooplankton and Fish
Global comparisons of the effects of low DO on pelagic ecosystems need to consider and evaluate the role of temperature in controlling oxygen availability, metabolic demand, organism behavior, and the consequences of moving to alternative habitats. For example, temperatures of hypoxic (<2 mg L−1) bottom water in the Gulf of Mexico can exceed 28°C (Pierson et al., 2009b) in contrast to the Baltic Sea where hypoxic water temperatures are 8–10°C (Carstensen et al., 2014; Figure 2). Such differences in bottom temperatures affect oxygen availability (partial pressure and solubility) for organisms, and drive differences in respiratory demands (Q10 for respiration for most zooplankton is around 2). Perhaps equally important is the contrast in temperatures between hypoxic water and the overlying mixed layer (alternative habitats) which can be small (1–3°C in the Gulf of Mexico; Pierson et al., 2009b, East China Sea; Zhang et al., 2015) or large (up to 10°C) in the Baltic Sea (Carstensen et al., 2014), Hood Canal (Sato et al., 2016), and Lake Erie (Pothoven et al., 2012). Salinity also has an effect on oxygen solubility, but the effect of temperature per degree °C is nearly three times that of a change of one salinity unit.
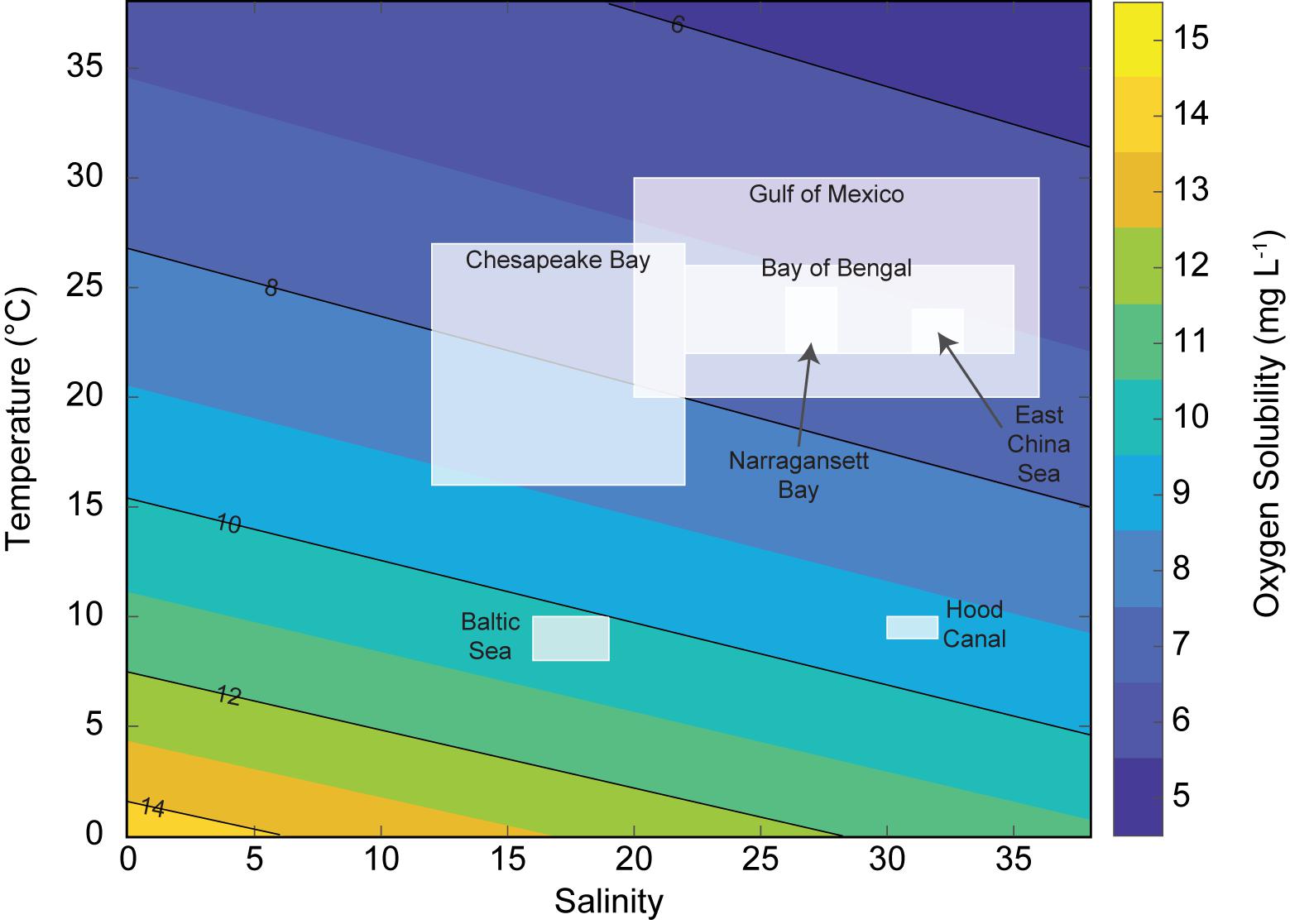
Figure 2. Oxygen solubility (mg L−1) is shown by the color scale at different salinity and temperature (°C) conditions, with boxes indicating the range of salinity and temperature during periods of seasonal deoxygenation for seven coastal and estuarine bodies of water around the world. Oxygen solubility calculated using the equations of Benson and Krause (1980, 1984), assuming surface water pressure.
Thus, all “hypoxic” bottom waters do not pose the same level of stress on zooplankton and zooplanktivorous fishes and can generate different responses in spatial ecology and predator-prey interactions. For example, using the predictive response to decreasing oxygen (Pcrit) developed for the copepod Acartia tonsa by Elliott et al. (2013), 2 mg L−1 bottom water in the Baltic Sea (9°C) would have an oxygen partial pressure of 4.24 kPa, which is slightly below the limiting Pcrit of A. tonsa predicted for this temperature (5.07 kPa). In contrast, 2 mg L−1 bottom water in the Gulf of Mexico (30°C) would have an oxygen partial pressure of 6.37 kPa, which is substantially below the limiting Pcrit = 18.31 kPa of A. tonsa predicted for this temperature and is approximately the same as the predicted lethal Pleth = 6.77 kPa oxygen partial pressure. These predicted differences in oxygen supply and demand provide a useful way to assess how low oxygen waters directly determine habitat availability for estuarine and coastal zooplankton. Field surveys have shown that zooplankton generally avoid the warmer low DO waters of the Gulf of Mexico (e.g., Qureshi and Rabalais, 2001; Roman et al., 2012) but reside in the colder low oxygen waters of the Baltic Sea for significant portions of the day, affording the zooplankton a potential refuge from predation (e.g., Appeltans et al., 2003; Webster et al., 2015). Because of the higher oxygen demand by coastal zooplankton and fish in warm tropical and subtropical waters, the loss of habitat space due to low oxygen waters is expected to be more severe in these regions.
Research in ecosystems such as the Great Lakes and Chesapeake Bay has identified water temperature as a critical factor in the assessment of hypoxia impacts for fish (e.g., Costantini et al., 2008; Brandt et al., 2009, 2011). Temperature is a fundamental driver of fish bioenergetics, behavior, and feeding and large-scale changes in ocean temperature trigger large-scale regime shifts in fish abundances (e.g., Cheung et al., 2013; Kilduff et al., 2014; Ito et al., 2015). The temperatures at which hypoxia occurs will determine the species most likely affected by the hypoxia. For example, if hypoxia occurs at temperatures that are largely unsuitable (too cold or too warm) to a particular species, then hypoxia is unlikely to directly impact that species. Also, if fish are forced to avoid hypoxic areas then temperatures of nearby alternative habitats may determine the consequences of shifts in distributions.
The direct consequences of lowered oxygen to fishes are also highly dependent on the prevailing water temperatures. Normally, the sensitivity of fish to low oxygen is higher at higher water temperatures due to higher metabolic demand. But the relationships are species-dependent, life stage-dependent and non-linear. For example, a set of laboratory experiments showed that juvenile striped bass growth was highly responsive to the interaction between oxygen and temperature (Figure 3). It is probable that striped bass would be more vulnerable to low DO in some ecosystems than others depending on respective temperature-DO levels (see Figure 2).
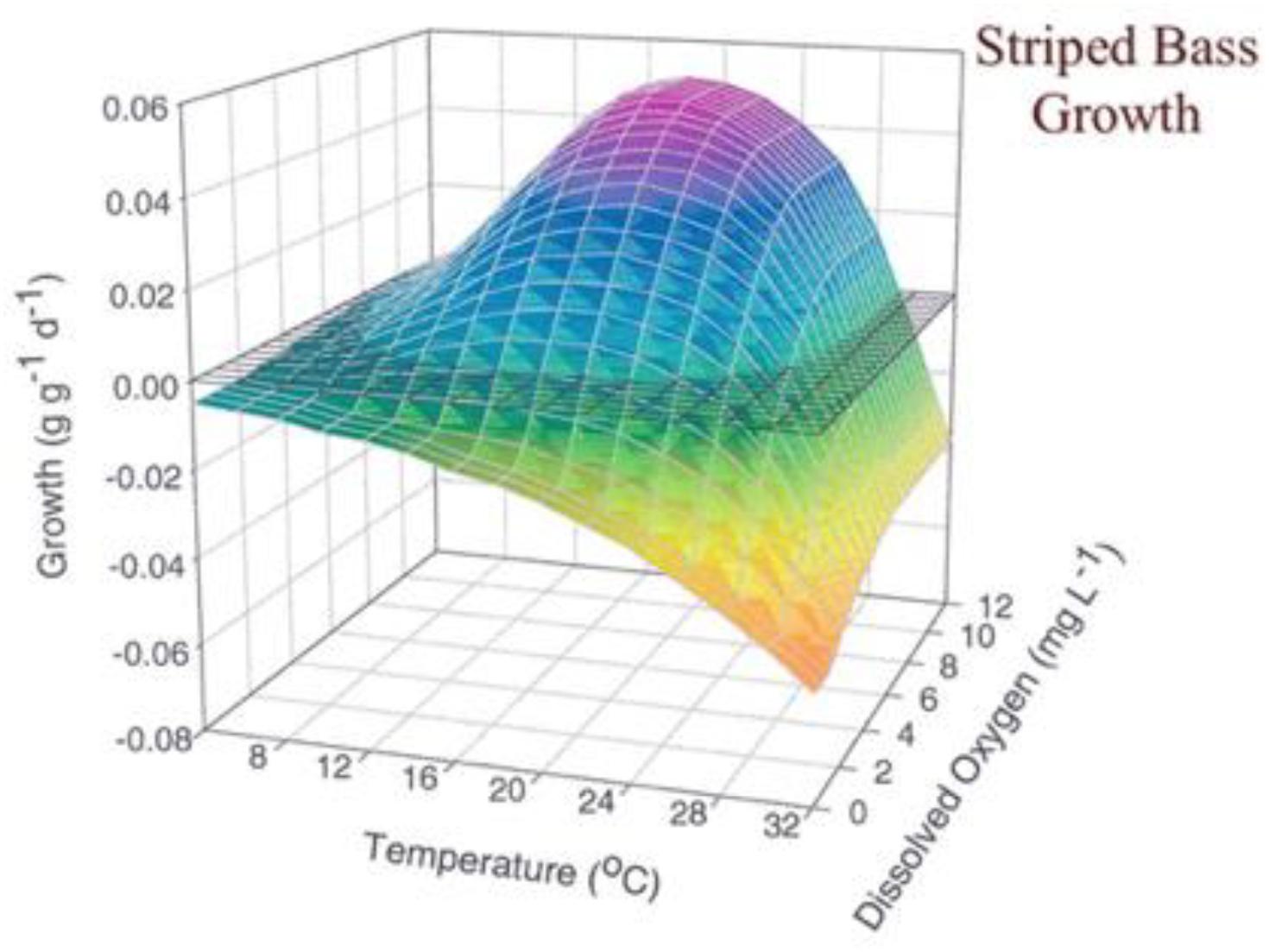
Figure 3. The relationships between striped bass growth rate, temperature, and dissolved oxygen from Brandt et al. (2009).
Zooplankton Respiration, Metabolism and Allometric Scaling
Oxygen availability to coastal zooplankton and fish is a function of the rate of diffusion across their integument or gills, thus allometric relationships for surface/volume, body mass, and gill area, among others, should be explored for similar groups of zooplankton and fish to predict oxygen limitation and its consequences. For zooplankton, copepods have been studied extensively and provide a robust example. The respiration of copepods is an exponential function of temperature (Ikeda, 1970). Whereas there are differences due to species, latitude and acclimation period, published Q10 values for copepod respiration range from 1.0 to 2.7 (Mauchline, 1998; Teuber et al., 2013). Temperature also influences the oxygen availability to copepods. While oxygen solubility in seawater decreases with temperature, oxygen partial pressure increases with temperature because of the greater kinetic energy of the oxygen molecules. As temperatures increase, the linear increase in partial pressure may initially satisfy the increased oxygen demand by copepods but, because respiration increases exponentially with respect to temperature, oxygen demand eventually exceeds supply and organisms become oxygen stressed (Verberk et al., 2011). An example of this is shown using the relationships from Elliott et al. (2013; Figure 4) for A. tonsa at a salinity of 25. The curve showing the relationship of Pcrit with temperature crosses the line describing the oxygen partial pressure for concentrations of 2 mg L−1 at approximately 10°C. The Pleth curve crosses at approximately 25°C. Consequently, above 10 and 25°C, DO concentrations even when above 2 mg L−1 may be below the critical or lethal thresholds, respectively, for A. tonsa.
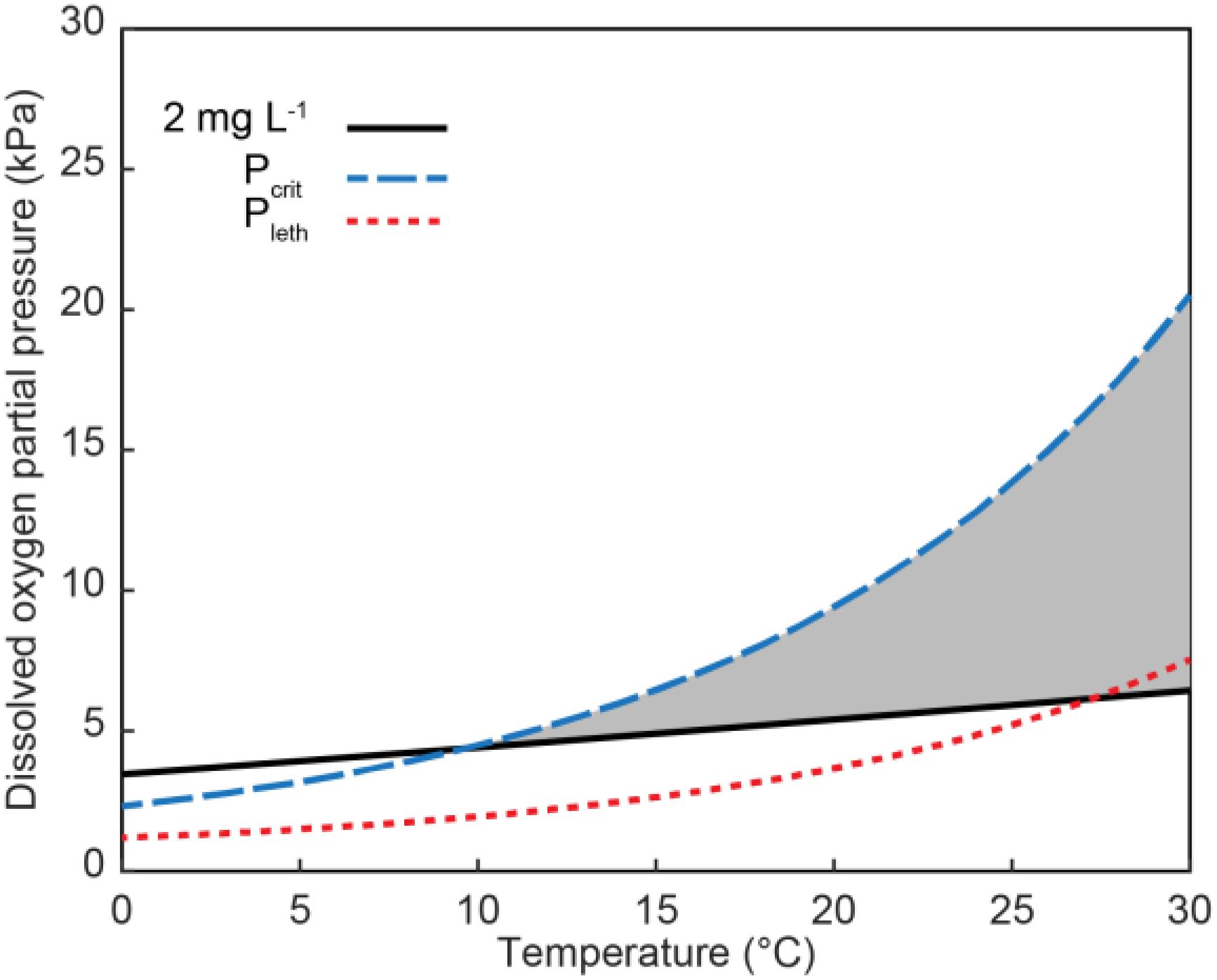
Figure 4. Partial pressure of dissolved oxygen over a range of temperatures for an oxygen concentration of 2 mg L−1 (solid black line), the Pcrit (dashed blue line) and Pleth (dotted red line) for the copepod A. tonsa (Elliott et al., 2013). Gray area shows region where values for Pcrit and Pleth are greater than the PO2 at 2 mg L−1, and thus where water may not be classified as hypoxic but DO would be below the critical or lethal threshold. The partial pressures of 2 mg L−1 were calculated as the percent saturation of O2 (Benson and Krause, 1980, 1984) at the given temperature (at assumed salinity of 25), and then multiplying that by 21.198 kPa, the assumed partial pressure of O2 at the surface.
The mass-specific metabolic activity and respiration of copepods decrease with body size (see reviews by Marshall, 1973; Hirst and Sheader, 1997; Mauchline, 1998). Thus, to compare respiration in different developmental stages of a particular species or to compare different zooplankton species over wide size ranges, investigators have developed empirical, allometric scaling models that predict respiration rates from body mass (Raymont and Gauld, 1951; Marshall, 1973; Ikeda, 1985). Within copepod species, body sizes in nature are inversely related to temperature (Deevey, 1960; McLaren, 1963; Uye, 1982), due largely to different temperature-dependent rates of growth and development (Miller et al., 1977; Forster et al., 2011). Since oxygen uptake by copepods takes place through the integument and surface to volume ratio decreases with increasing size, smaller copepods, with a higher surface area to volume ratio may be favored in warmer waters where oxygen solubility is lower but respiratory demand is higher. Similarly, in low-oxygen environments, smaller copepods may be favored because of their higher surface area to volume ratio and thus more efficient oxygen diffusion per unit mass (Pörtner, 2010).
Taken together, copepod body mass and habitat temperature can account for 72 – 96% of the variability in measured weight-specific copepod respiration rate (Ikeda, 1985; Ikeda et al., 2007; Bode et al., 2013; Castellani and Altunbas, 2014; Figure 5). Thus, for copepods, temperature dependence of growth rates and presumably respiration usually outweigh species-specific differences (Huntley and Lopez, 1992; Hirst and Sheader, 1997). To provide global assessments of seasonal hypoxia effects on coastal zooplankton, one could develop generic species models for copepods based solely on allometry and assess patterns of low DO effects based on size and ambient temperature.
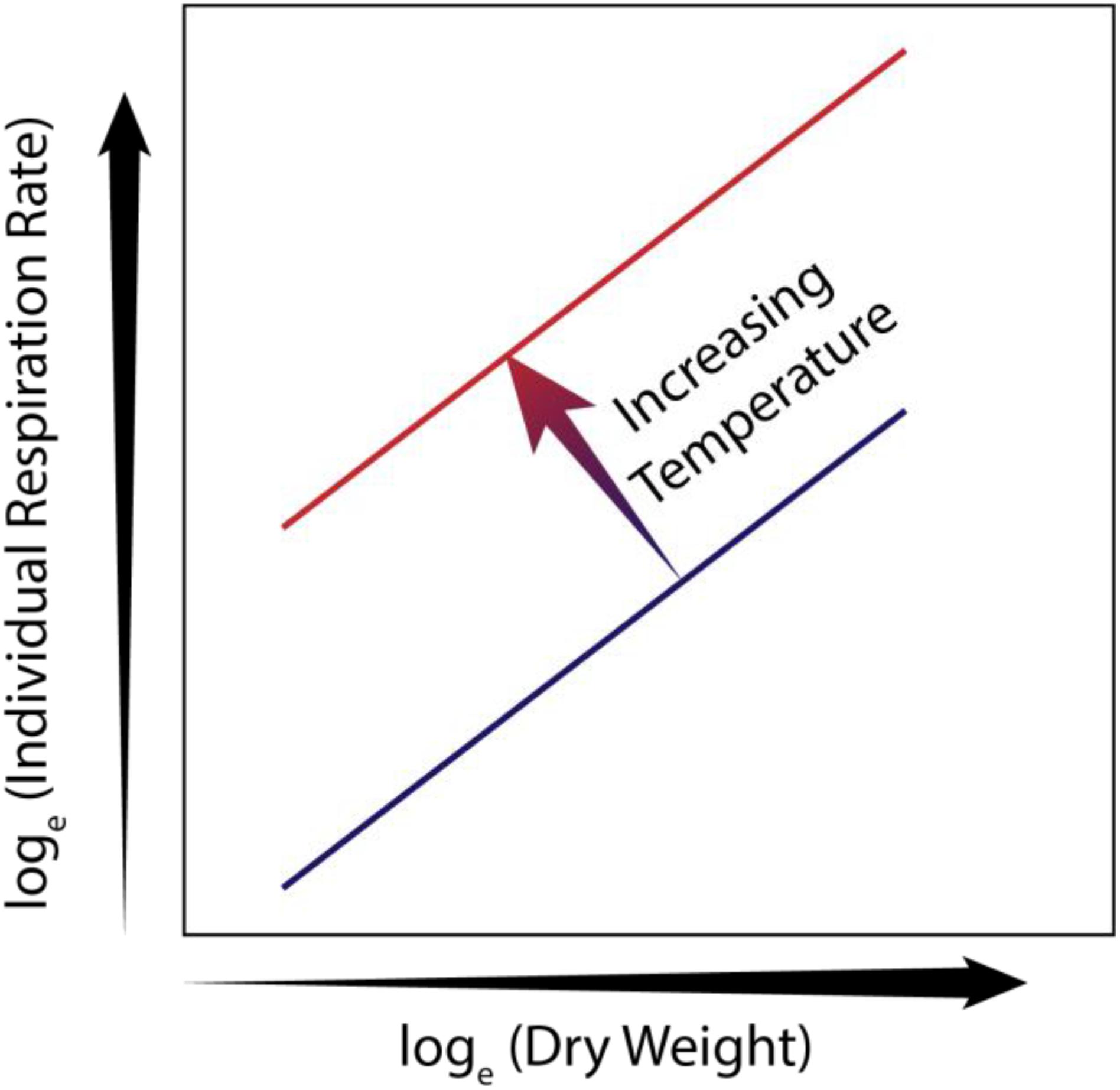
Figure 5. Conceptual figure showing natural log transformed respiration rate (ln R) of a copepod versus copepod dry weight (ln DW), and the effect of temperature on the relationship, based on data from Castellani and Altunbas (2014). Lines represent the relationship between ln R, ln DW for different temperatures. Note the slope remains the same but the overall respiration at a given size increases with temperature.
Planktivorous Fish Respiration, Metabolism and Allometric Scaling
There is an extensive body of literature demonstrating that metabolic rates of fish also are strongly dependent on both body mass and temperature (e.g., Fry, 1971; Clarke and Johnston, 1999; Claireaux and Lefrançois, 2007; Pauly, 2010; Peck et al., 2012; Evans et al., 2014). Within a species, the allometric relationship of metabolic demand to fish mass or weight is well established (Rombough, 1988; Clarke and Johnston, 1999). Larger fish have a higher demand for oxygen but the weight-specific rate of oxygen consumption decreases as weight increases. Thus warmer temperatures and lower DO would favor smaller fish (e.g., Ekau et al., 2010; Pauly, 2010; Verberk et al., 2011; Cheung et al., 2012).
Scaling relationships have been developed for body mass, metabolic rate and temperature in fishes and broader taxa categories (e.g., Gillooly et al., 2001; Brown et al., 2004). For example, Clarke and Johnston (1999) derived a generalized allometric relationship of standard (resting) metabolism for 69 species of post-larval fishes. The taxa covered 12 orders and 28 families of fishes ranging from Myctophidae, to Salmonidae, to Gobiidae to Ictaluridae. The mean coefficient b for allometric scaling (R = aMb) was 0.79 and the mean Q10 for the effect of temperature was 1.83. After adjusting for body mass, the relationship of metabolic rate (oxygen consumption) was a curvilinear relationship to temperature that the authors noted was “strikingly similar to relationships established previously for aquatic invertebrates” (Clarke, 1991). They also provided evidence that the relationship of metabolism to temperature had different mean rates but overall similar slopes across related species at the fish order level. Killen et al. (2010) examined the scaling of metabolic rate and fish body mass across temperature and fish ‘lifestyles’ among 89 species of fish and concluded that the metabolic scaling b exponent shifted with lifestyle changes from bathyal to benthic to benthopelagic to pelagic and the authors related this shift to energy requirements associated with swimming and predator-prey interactions. At a standardized temperature, oxygen consumption levels were generally high for pelagic fishes (46% higher than for benthic fishes; b = 0.698 benthic versus b = 0.856 pelagic). Highly active pelagic fishes may require more muscle mass and respiratory surface areas (Muir, 1969).
In larval-stage fishes, scaling relationships for respiration and metabolism also have been investigated and reviewed. Relationships may differ from those in juvenile and adult fishes, probably because of the shift from cutaneous to gill respiration during ontogeny (Rombough, 1988). The exponent in the allometric relationship between respiration rate and body mass is variable among taxa, generally lying in the range 0.6–1.2 (Houde and Zastrow, 1993; Peck et al., 2012), with some authors contending that the relationship is isometric (b = 1.0) in fish larvae (Giguere et al., 1988). As expected, temperature strongly affects respiration rates in embryonic and larval-stage fishes (Houde, 1989a; Houde and Zastrow, 1993). Peck et al. (2012) suggested there is an ontogenetic shift in the scaling relationship, with the power exponent in the allometric relationship declining as larvae transition to the juvenile stage. The Q10 for respiration rates in fish larvae may, in many cases, be lower than the 2.0–3.0 values typically seen in juvenile and adult fishes (Rombough, 1988). Houde (1989a) reported mean Q10 of 1.46 for marine fish larvae although Peck et al. (2012), with an expanded database, estimated a mean Q10 of 2.31, noting that taxa-specific Q10 always was < 1.70 for species living at > 25° C but was >2.60 for species living at ≤ 18°C.
Bioenergetics relationships in pelagic fishes have been investigated and feeding, energy budgets, oxygen uptakes and metabolic demand in relation to temperature, diet level, and body size are reasonably well understood for some taxa, for example anchovy life stages (Houde and Schekter, 1983; Houde et al., 1989; Vazquez and Houde, 1989). Bioenergetic models for bay anchovy (Luo and Brandt, 1993; Houde and Madon, 1995), based on our previous research, suggest that temperature-dependent, weight-specific metabolism of different anchovy species is similar (Vazquez and Houde, 1989; Figure 6), implying similar dependencies on ambient temperatures and DO.
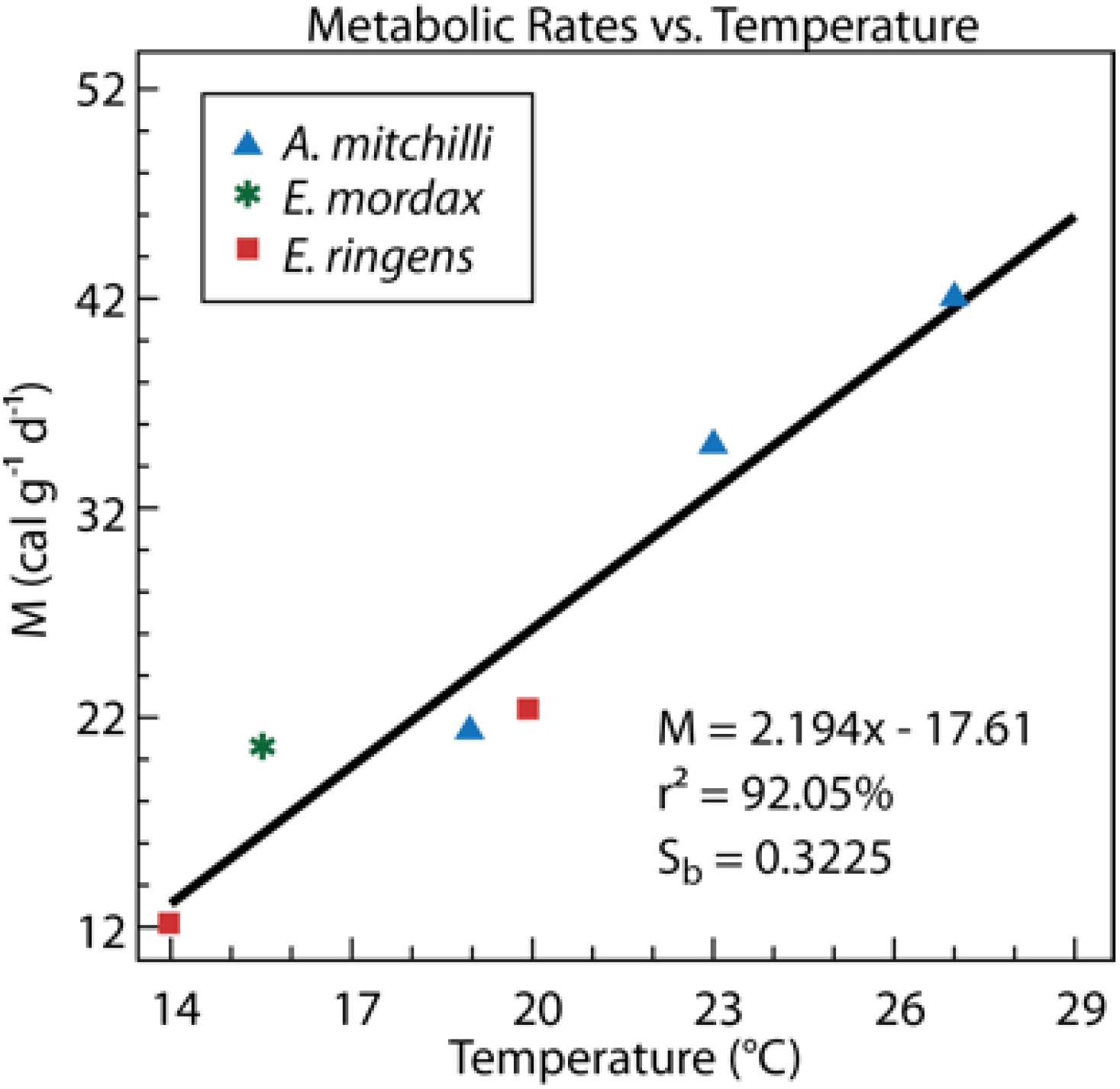
Figure 6. Weight-specific metabolic rates (M, calories g-1 day-1) at different temperatures (T, °C) for three anchovy species, A. mitchilli the bay anchovy, a coastal species; Engraulis mordax the northern anchovy from the California current; Engraulis ringens, the Peru anchoveta, from the Humboldt Current. Figure adapted from Vazquez and Houde (1989).
Comparing Hypoxia Impacts Across Ecosystems and Species
To assess effects of globally expanding, coastal low oxygen zones, we need to determine if generic models and unifying metrics can be developed to evaluate and predict how low DO imposes temporal and spatial limitations on metabolic functioning of zooplankton and their fish predators. An oxygen concentration of 2 mg L−1 has routinely been used to define the presence, areal extent, volume, duration and inter-annual variability of hypoxia within and across ecosystems. This concentration does not consider the important role of temperature in defining oxygen supply. Figure 2 demonstrates the large differences in ecosystems with similar oxygen concentrations. We recommend that habitat characterization be done using oxygen partial pressure to define hypoxia and the approach outlined in Figure 2 adopted to compare ecosystems.
Hypoxic stress must be considered in the context of oxygen supply relative to oxygen demand. Oxygen supply, being determined by rate of diffusion, will scale with temperature based on Fick’s Law (Verberk et al., 2011) and would be expected to scale linearly with body/gill surface area, or approximately to the 2/3 power with body volume or mass. Estimates of oxygen supply relative to demand can be derived from existing knowledge of an organism’s Pcrit (see Figure 4). Because Pcrit is essentially the external oxygen partial pressure at which supply equals demand, the rate of oxygen supply at this threshold will equal that of oxygen demand (i.e., the respiration rate). Values of Pcrit have been measured for copepods and fish of various body masses and respiration rates have been related to both temperature and body mass in numerous studies. Thus, ample data exist to derive estimates of the rate of oxygen supply and demand for zooplankton (mainly copepods) and fishes across a range of temperatures and body masses, allowing parameterization of the expected scaling relationships. Deutsch et al. (2015) developed an integrated “metabolic index” projected over the global ocean to predict how the supply of and demand for DO will change in the future with regard to all of the potential impacts of climate change on DO. Their findings suggest large decreases in the metabolic index over large swaths of the globe, meaning organisms in a particular region are likely to exist in less favorable conditions in the future. Although this research was directed to the open ocean, it is probable that coastal and estuarine systems experiencing seasonal hypoxia at increasing water temperatures are likely to have similar prospects for the future.
Similar to the metabolic index of Deutsch et al., 2015, the general, broadly applicable relationships of temperature and body mass with oxygen supply and demand can be parameterized to develop an Oxygen Stress Level (OSL, a dimensionless number), either for individual taxa or for groups of organisms. This ratio of oxygen supply to demand could be calculated for study regions as a simple metric indicating the predicted occurrence and severity of hypoxic stress. Oxygen stress levels can be defined as:
For OSL > 1, supply exceeds demand and no oxygen stress, sub-lethal or lethal effects would be predicted. Conversely, for OSL < 1, oxygen demand exceeds supply and sub-lethal or lethal effects of oxygen stress would be predicted. For OSL < 0 oxygen demand is below the basal respiration rate. Thus, OSL = 1 indicates DO conditions equal to the organism’s Pcrit and for OSL = 0 it indicates DO conditions equivalent to the organism’s Pleth.
As an example, we examined the spatial extent and temporal variability of OSL based on high resolution profiles of temperature, salinity and DO collected in July of each of 4 years along axial transects in Chesapeake Bay (Zhang et al., 2006). DO concentrations were converted to partial pressure by first calculating the DO solubility for each temperature and salinity value (Benson and Krause, 1984), and multiplying the percent saturation by 21.198, the partial pressure of oxygen in the air. Pcrit and Pleth for Acartia tonsa (Elliott et al., 2013) were calculated to determine OSL values for A. tonsa at each data point (Figure 7). The along-channel patterns in OSL are similar among years; however, 1996 was a wet year with high streamflow, which led to very low OSL in the upper Chesapeake Bay. In contrast, 1999 was a dry year with low streamflow, and OSL values were higher in the mid-bay and the lower-bay reaches than in any of the other years.
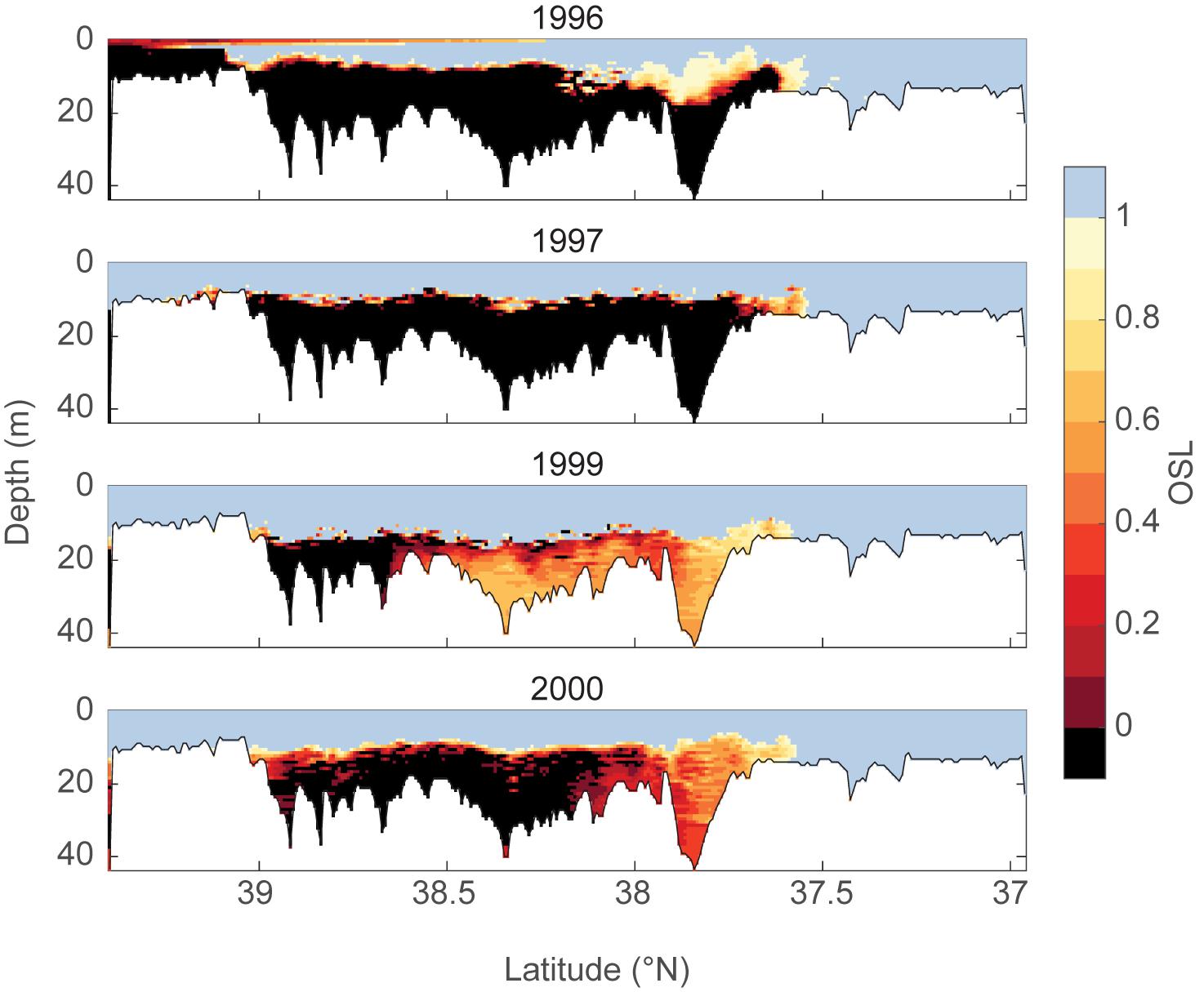
Figure 7. OSL index for the copepod A. tonsa in the main channel of the Chesapeake Bay for 4 years, calculated using high resolution hydrographic data (temperature, salinity, and dissolved oxygen) collected with a Scanfish (Zhang et al., 2006). Calculations of Pcrit and Pleth were made using equations from Elliott et al. (2013).
We also examined whether the concentration of A. tonsa varied with respect to OSL in this same system. Using data from zooplankton samples collected in summer of 2010 and 2011 from depth stratified net tows and simultaneously collected hydrographic data (Pierson et al., 2017), we determined the relationship between stage-specific A. tonsa concentrations and OSL using a regression tree analysis (Figure 8).
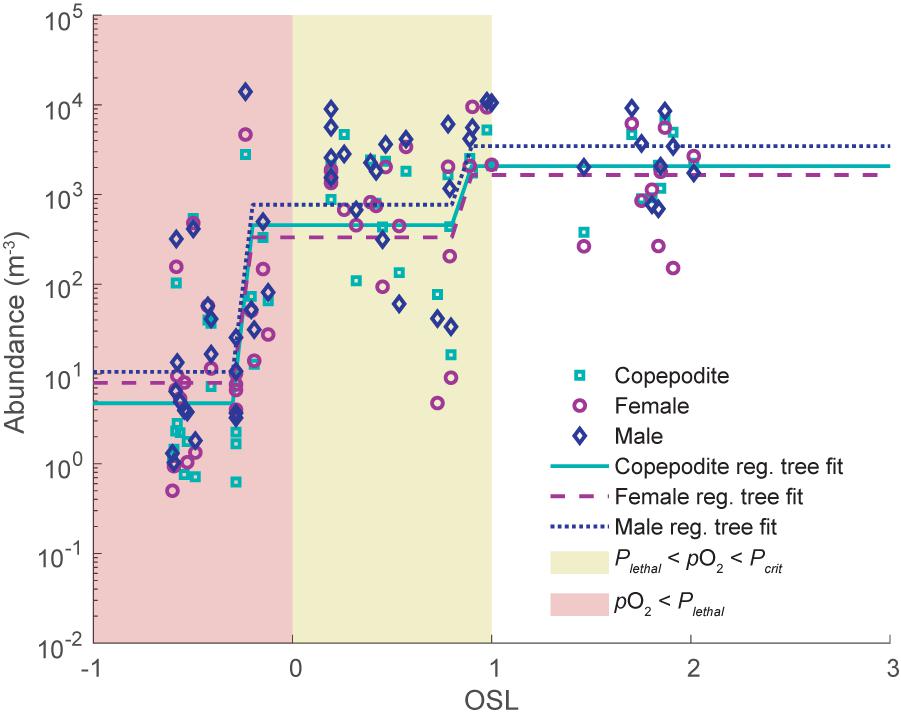
Figure 8. Concentration (m−3) of Acartia tonsa in the Chesapeake Bay at various values of OSL, determined from simultaneously collected vertically stratified zooplankton samples and hydrographic data. Green squares show data for copepodite stages, purple circles show data for adult females, and blue diamonds show data for adult males. Lines show results from regression tree analysis to examine the relationship between log10 transformed concentration and OSL, with solid green lines for copepodites, dashed purple lines for adult females, and dotted blue lines for adult males. Yellow shaded area shows where Pleth < pO2 < Pcrit, and pink shaded region shows where pO2 < Pleth. Regression trees were created in Matlab using the “fitrtree.m” function, with a minimum leaf size of 8 and pruned to a level of 2.
The A. tonsa data were sorted to include only data from samples collected in the oxycline and below the oxycline, and not in the surface, to avoid biases related to general patterns of depth distribution. In general, A. tonsa concentrations were lowest where OSL < 0 and highest where OSL > 1 (Figure 8). Specifically, the regression tree analysis identified cut points at OSL = −0.26 and OSL = 0.84 for all three stages. Mean concentrations values of copepodites, females, and males at each of the nodes ranged from 4.7 – 10.6 m−3 for OSL < −0.26, 333 – 770 m−3 for −0.26 < OSL < 0.84, and 1652 – 3467 m−3 for OSL > 0.84. This suggests that the OSL metric may be a useful tool to assess available and usable habitat.
In addition to this simple metric OSL, there are temperature-specific and allometric relationships describing Pcrit and Pleth. Because Pcrit and Pleth are direct reflections of the balance between oxygen supply and demand, these low oxygen thresholds will scale with temperature and body size in the same manner as OSL. Effects of temperature on these thresholds, through effects on respiration, can be described using specific respiratory Q10 values available from the literature (e.g., Rombough, 1988; Peck et al., 2012; Elliott et al., 2013). For calibration, absolute values of Pcrit and Pleth at various body masses can be taken or derived from the available literature using methods similar to Elliott et al. (2013). The methods of Killen et al. (2010) to derive Pcrit and temperature-oxygen specific respiration rates may be appropriate for pelagic planktivores which are likely to have similar energy requirements and oxygen demands (Clarke and Johnston, 1999; Killen et al., 2010). Results from published literature on fishes such as herring, sardines, anchovies and smelt (e.g., Kiørboe et al., 1987; Tanaka et al., 2006; Bernreuther et al., 2013) could be critically evaluated and combined to define generic relationships. Using the techniques employed by Elliott et al. (2013), one could convert vital rate data collected at different oxygen and temperature conditions to estimate the values of Pcrit and Pleth, and to determine OSL for fish taxa found in regions with hypoxic conditions. Such calculations are important because the relative impact of deoxygenation on an organism can be quantified and scaled to make it applicable to a wide variety of modeling efforts. de Mutsert et al. (2016) employed a similar approach to scale fish feeding to oxygen concentration and showed species-specific responses to hypoxia and to explicitly modeled drivers of hypoxia. Parameterizations of organismal responses to hypoxia for broader taxonomic (e.g., various zooplankton species) and ontogenetic stages (e.g., larvae and juveniles) as well as expanded parameterizations of the individual responses of organisms to hypoxia (e.g., reproductive output, predator avoidance) will further improve our predictive capabilities for ecosystem and food-web models.
Impacts of Hypoxia on Zooplankton-Fish Interactions
Low DO bottom waters can influence the spatial overlap and trophic coupling of pelagic zooplankton and fish populations. For example, more hypoxia-tolerant zooplankton may use low DO bottom waters as a refuge from fish predation (Appeltans et al., 2003; Taylor and Rand, 2003; Webster et al., 2015; Figure 9). In contrast, zooplankton avoidance of hypoxic bottom waters can result in prey aggregations at oxyclines where fish and invertebrate predators may aggregate (Qureshi and Rabalais, 2001; Taylor and Rand, 2003; Roman et al., 2012). As fish and zooplankton differentially distribute across oxygen gradients within the water column based on their DO tolerances, there will likely be a disruption in the food web as encounter rates among predators and prey will change (e.g., Vanderploeg et al., 2009; Pothoven et al., 2012).
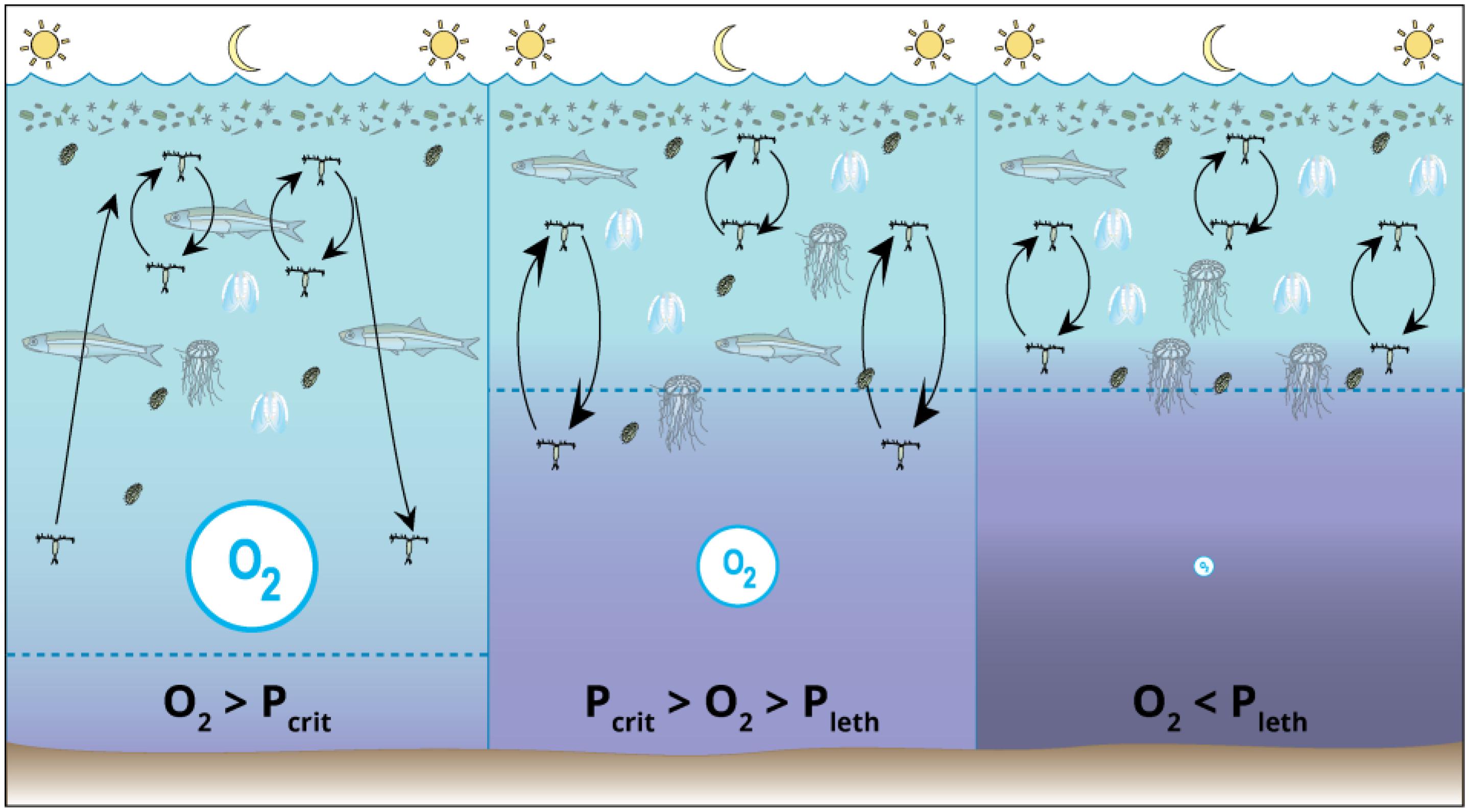
Figure 9. Conceptual diagram of food web changes that may occur over diel cycles between normoxic conditions (left), sub-lethal deoxygenation conditions (middle), and lethal conditions (right). The size of the symbol for O2 and the color indicates the relative amount of dissolved oxygen under each condition (with light blue indicating high oxygen concentration and purple indicating lower oxygen concentration). With decreasing oxygen concentration comes reduced habitat, truncated magnitude of copepod vertical migration (shown by length of arrows), increased gelatinous zooplankton abundance, and decreased forage fish abundance. Symbols Courtesy of the Integration and Application Network, University of Maryland Center for Environmental Science (ian.umces.edu/symbols/).
In coastal ecosystems with shears and differential flow between surface and deep layers, avoidance of low oxygen bottom waters can influence spatial dynamics of zooplankton and fish populations by altering emigration and immigration patterns, and residence times. As more information becomes available on the oxygen demand of dominant estuarine and coastal zooplankton and fish at different temperatures, we will be able to better assess if low oxygen bottom waters influence their trophic interactions.
Several lines of evidence suggest that hypoxic bottom waters do effect pelagic food-web interactions in coastal waters. Glaspie et al. (2018) found the diet of a pelagic fish, the Atlantic bumper (Chloroscombrus chrysurus), to differ significantly between hypoxic and non-hypoxic waters. The vertical distribution of DO within the water column affects spatial overlap between zooplankton and their fish and gelatinous zooplankton predators (e.g., Keister et al., 2000; Breitburg et al., 2003). Predators may make short excursions into hypoxic water to take advantage of aggregated prey (Taylor et al., 2007) and predation rates of fish and gelatinous zooplankton feeding on mesozooplankton are affected by DO concentrations (Breitburg et al., 1997; Decker et al., 2004).
Hypoxic conditions that affect spatial ecology and population dynamics can drive taxonomic and size shifts in the zooplankton community which will affect foraging, consumption and growth of fish species. Smaller zooplankton may be favored in low-oxygen conditions because their higher surface/volume ratio. If hypoxic conditions result in a community shift to smaller zooplankton, there may be negative consequences for fish that then must feed on smaller prey items. It is this type of shift in the Peru Current that is believed to control hypoxia-related species shifts that favor sardine over anchoveta (Bertrand et al., 2011). Similar food web consequences from shifts in zooplankton species could occur if there was a succession to favor copepods that carry their eggs in systems with hypoxic bottom waters (Uye, 1994). Furthermore, low oxygen waters may favor jellyfish over planktivorous fish (Figure 9) because of the higher oxygen requirements of fish. Shifts in the zooplankton food available to fish and greater competition by jellyfish can work together to alter pelagic food webs in estuarine and coastal waters experiencing seasonal bottom-water hypoxia.
An important issue related to bottom water hypoxia in estuarine and coastal waters is determining whether zooplankton use the low-DO areas as a critical habitat to avoid predation or must avoid it and remain in the upper water column where they may be subject to greater predation (Figure 9). In theory, zooplankton could reside in hypoxic bottom waters if the oxygen supply is greater than its critical oxygen demand (i.e., Pcrit, OSL > 1) but not if the oxygen availability is well below Pcrit or the lethal oxygen partial pressure (i.e., Pleth, OSL < 0). In both the Gulf of Mexico and Chesapeake Bay, the size distribution of zooplankton as measured with an Optical Plankton Counter (OPC) was shifted to smaller individuals in surface normoxic waters compared to bottom hypoxic waters where there was larger zooplankton (Kimmel et al., 2009). Kimmel et al. (2009) interpreted these patterns to be a consequence of larger copepods having been removed from surface waters by visual predators (fish) which did not forage in the hypoxic bottom waters. Analysis of depth-stratified zooplankton collections in the Gulf of Mexico confirmed OPC results, with larger copepod species more prevalent in low-oxygen bottom waters and smaller copepod species assemblages more prevalent in the oxygenated surface waters where selective predation by fish would likely reduce the abundance of larger copepod species (Elliott et al., 2012). A recent illustration of oxygen tolerances and predator-prey interactions is provided by Sato et al. (2016) for Hood Canal, a fjord in the Northwest U.S. The authors hypothesized that moderate 2–4 mg L−1 DO bottom waters would be avoided by fish but not zooplankton, providing a separation of predators and prey. However, field sampling did not support this hypothesis because daytime peak distributions of zooplankton (copepods and euphausiids) and fish (herring and hake) co-occurred in the low-DO bottom waters. The authors suggested that the cold (9°C) waters reduced fish metabolism such that the available oxygen was greater than their Pcrit.
The spatial relationships of relative stress (OSL < 1) between zooplankton and planktivorous fish can provide insight into potential effects on predator-prey interactions. Determining and comparing the Pcrit and Pleth for each will be important because the relative behaviors that control prey and predator interactions are likely to be responsive to Pcrit and Pleth. Habitats that have high potential stress for fishes but not for zooplankton may provide a refuge for the zooplankton. In contrast, habitats where DO is above the Pcrit for jellyfish but is below the Pcrit for zooplankton may increase predation rates on zooplankton as escape response is reduced in low DO (Decker et al., 2004). If Pcrit and Pleth differ substantially for copepods and their predators, this result can explain how predator-prey interactions are regulated by combined effects of DO and temperature and provide a means to map potential areas of relative predation risk.
Conclusion and Recommendations
The scientific literature does not clearly explain or define how hypoxia affects pelagic zooplankton and planktivorous fishes and their occurrences in hypoxic waters, nor have the interactive effects of temperature and hypoxia been addressed in the context of multiple stressors on organisms in coastal ecosystems. To assess effects of the globally expanding coastal low-oxygen zones, often termed “dead zones,” generic approaches and unifying metrics that include ambient water temperatures are needed to assess and predict when and how oxygen limits occurrence and metabolic functioning of zooplankton and their fish predators. Considering oxygen and temperature as a combined and interactive driver in coastal ecosystems will provide a unifying approach for ecosystem comparisons. The Pcrit, Pleth and OSL characterization of habitat provides a direct measure of habitat quality (or stress level) that should have broad appeal and direct applications in water quality monitoring and fisheries management. These metrics should be applicable to pelagic ecosystems in general, and once developed they can provide a quantitative and meaningful measure to define tipping points, evaluate external perturbations and resilience, nutrient reduction strategies, ecosystem physical dynamics, restoration potential, and impacts of a warming climate. Because oxygen availability is dependent on diffusion across integument or gills of aquatic organisms, allometric relationships such as surface area/volume, body mass, and gill area for similar groups of zooplankton and fishes offer a generalized approach to characterize effects of hypoxia and predict oxygen limitation. Allometric scaling models that predict temperature-dependent oxygen supply and demand from the perspectives of zooplankton and planktivorous fishes will lead to improved ability to assess effects of increasing coastal hypoxia on pelagic food webs and allow standardized, quantitative approaches to evaluate and compare hypoxia impacts across coastal ecosystems.
Author Contributions
MR had overall responsibility for manuscript preparation. SB and EH wrote the section on fish ecology and physiology and contributed sections on the fish-zooplankton interactions. JP prepared sections on zooplankton ecology and physiology.
Funding
The following grants supported our efforts to prepare this work: NOAA-NGOMEX NA06NOS4780148 and NA09NOS4780198 (SB, JP, and MR), NA16NOS4780202 (SB), NSF OCE-0961942 (EH, JP, and MR) and OCE-1259691 (MR and JP), and NAS- 2000006418 (MR, JP, and SB).
Conflict of Interest Statement
The authors declare that the research was conducted in the absence of any commercial or financial relationships that could be construed as a potential conflict of interest.
Acknowledgments
We are grateful to Catherine Fitzgerald who helped prepare the Figures and References for this manuscript.
References
Aku, P. M. K., Rudstam, L. G., and Tonn, W. M. (1997). Impact of hypolimnetic oxygenation on the vertical distribution of cisco (Coregonus artedi) in Amisk Lake, Alberta. Can. J. Fish. Aquat. Sci. 54, 2182–2195. doi: 10.1139/f97-124
Aku, P. M. K., and Tonn, W. M. (1997). Changes in population structure, growth, and biomass of cisco (Coregonus artedi) during hypolimnetic oxygenation of a deep, eutrophic lake, Amisk Lake, Alberta. Can. J. Fish. Aquat. Sci. 54, 2196–2206. doi: 10.1139/f97-118
Altieri, A. H., and Gedan, K. B. (2014). Climate change and dead zones. Glob. Change Biol. 21, 1395–1406. doi: 10.1111/gcb.12754
Appeltans, W., Hannouti, A., van Damme, S., Soetaert, K., Vanthomme, R., and Tackx, M. (2003). Zooplankton in the Schelde estuary (Belgium/The Netherlands). The distribution of Eurytemora affinis: effect of oxygen? J. Plankton. Res. 25, 1441–1445. doi: 10.1093/plankt/fbg101
Beauchamp, D. A., Cross, A. D., Armstrong, J. L., Myers, K. W., Moss, J. H., Boldt, J. L., et al. (2007). Bioenergetic responses by Pacific salmon to climate and ecosystem variation. North Pac. Anadromous Fish Commission Bull. 4,257–269.
Beaugrand, G., Reid, P. C., Ibañez, F., Lindley, J. A., and Edwards, M. (2002). Reorganization of North Atlantic marine copepod diversity and climate. Science 296, 1692–1694. doi: 10.1126/science.1071329
Benson, B., and Krause, D. (1980). The concentration and isotopic fractionation of gases dissolved in freshwater in equilibrium with the atmosphere. 1. Oxygen. Limnol. Oceanogr. 25, 662–671. doi: 10.4319/lo.1980.25.4.0662
Benson, B. B., and Krause, D. (1984). The concentration and isotopic fractionation of oxygen dissolved in freshwater and seawater in equilibrium with the atmosphere. Limnol. Oceanogr. 29, 620–632. doi: 10.4319/lo.1984.29.3.0620
Bernreuther, M., Herrmann, J. P., Peck, M. A., and Temming, A. (2013). Growth energetics of juvenile herring, Clupea harengus L.: food conversion efficiency and temperature dependency of metabolic rate. J. Appl. Ichthyol. 29, 331–340. doi: 10.1016/j.marpolbul.2010.02.011
Bertrand, A., Chaigneau, A., Peraltilla, S., Ledesma, J., Graco, M., Monetti, F., et al. (2011). Oxygen: a fundamental property regulating pelagic ecosystem structure in the coastal southeastern tropical pacific. PLoS One 6:e29558. doi: 10.1371/journal.pone.0029558
Bianchi, T. S., DiMarco, S. F., Cowan, J. H., Hetland, R. D., Chapman, P., Day, J. W., et al. (2010). The science of hypoxia in the northern Gulf of Mexico: a review. Sci. Total Environ. 408, 1471–1484. doi: 10.1016/j.scitotenv.2009.11.047
Bode, M., Schukat, A., Hagen, W., and Auel, H. (2013). Predicting metabolic rates of calanoid copepods. J. Exp. Mar. Biol. Ecol. 444, 1–7. doi: 10.1016/j.jembe.2013.03.003
Bollens, S. M., and Frost, B. W. (1989). Predator-induced diel vertical migration in a planktonic copepod. J. Plankton Res. 11, 1047–1065. doi: 10.1093/plankt/11.5.1047
Brandt, S. B., Costantini, M., Kolesar, S., Ludsin, S. A., Mason, D. M., Rae, C. M., et al. (2011). Does hypoxia reduce habitat quality for Lake Erie walleye (Sander vitreus)? A bioenergetics perspective. Can. J. Fish. Aquat. Sci. 68, 857–879. doi: 10.1139/f2011-018
Brandt, S. B., Gerken, M., Hartman, K. J., and Demers, E. (2009). Effects of hypoxia on food consumption and growth of juvenile striped bass (Morone saxatilis). J. Exp. Mar. Biol. Ecol. 381, S143–S149. doi: 10.1016/j.jembe.2009.07.028
Breitburg, D. (2002). Effects of hypoxia, and the balance between hypoxia and enrichment, on coastal fishes and fisheries. Estuaries 25, 767–781. doi: 10.1007/BF02804904
Breitburg, D., Levin, L. A., Oschlies, A., Grégoire, M., Chavez, F. P., Conley, D. J., et al. (2018). Declining oxygen in the global ocean and coastal waters. Science 359, 7240. doi: 10.1126/science.aam7240
Breitburg, D. L., Adamack, A., Rose, K. A., Kolesar, S. E., Decker, B., Purcell, J. E., et al. (2003). The pattern and influence of low dissolved oxygen in the Patuxent River, a seasonally hypoxic estuary. Estuaries 26, 280–297. doi: 10.1007/BF02695967
Breitburg, D. L., Hondorp, D. W., Davias, L. A., and Diaz, R. J. (2009). Hypoxia, nitrogen, and fisheries: integrating effects across local and global landscapes. Annu. Rev. Mar. Sci. 1, 329–349. doi: 10.1146/annurev.marine.010908.163754
Breitburg, D. L., Loher, T., Pacey, C. A., and Gerstein, A. (1997). Varying effects of low dissolved oxygen on trophic interactions in an estuarine food web. Ecol. Monogr. 67, 489–507. doi: 10.1890/0012-9615(1997)067[0489:VEOLDO]2.0.CO;2
Breitburg, D. L., Pihl, L., and Kolesar, S. E. (2001). “Effects of low dissolved oxygen on the behavior, ecology and harvest of fishes: a comparison of the chesapeake bay and baltic-kattegat systems,” in The Effects of Hypoxia on Living Resources, with Emphasis on the Northern Gulf of Mexico Coastal Hypoxia: Consequences for Living Resources and Ecosystems, eds N. C. Rabalais and E. Turner (Washington, DC: American Geophysical Union), 241–267.
Breitburg, D. L., Rose, K. A., and Cowan, J. H. (1999). Linking water quality to larval survival: predation mortality of fish larvae in an oxygen-stratified water column. Mar. Ecol. 178, 39–54. doi: 10.3354/meps178039
Brett, J. R. (1964). The respiratory metabolism and swimming performance of young sockeye salmon. J. Fish. Board Can. 21, 1183–1226. doi: 10.1139/f64-103
Broman, E., Brusin, M., Dopson, M., and Hylander, S. (2017). Oxygenation of anoxic sediment triggers hatching of copepod eggs. Proc. R. Soc. 282, 2015–2025. doi: 10.1098/rspb.2015.2025
Brown, J. H., Gillooly, J. F., Allen, A. P., Savage, V. M., and West, G. B. (2004). Toward a metabolic theory of ecology. Ecology 85, 1771–1789. doi: 10.1890/03-9000
Cahoon, L. B. (1981). Reproductive response of Acartia tonsa to variations in food ration and quality. Deep Sea Res. Part A Oceanogr. Res. Papers 28, 1215–1221. doi: 10.1016/0198-0149(81)90057-1
Carstensen, J., Andersen, J. H., Gustasson, B. G., and Conley, D. J. (2014). Deoxygenation of the Baltic Sea during the last century. Proc. Natl. Acad. Sci. U.S.A. 111, 5628–5633. doi: 10.1073/pnas.1323156111
Castellani, C., and Altunbas, Y. (2014). Seasonal change in acclimatized respiration rate of Temora longicornis. Mar. Ecol. Prog. Ser. 500, 83–101. doi: 10.3354/meps10661
Chapman, L. J., Chapman, C. A., Nordlie, F. G., and Rosenberger, A. E. (2002). Physiological refugia: swamps, hypoxia tolerance and maintenance of fish diversity in the Lake Victoria region. Comp. Biochem. Physiol. A 133, 421–437. doi: 10.1016/S1095-6433(02)00195-2
Chesney, E. J., Baltz, D. M., and Thomas, R. G. (2000). Louisiana estuarine and coastal fisheries and habitats: perspectives from a fish’s eye view. Ecol. Appl. 10, 350–366. doi: 10.1890/1051-0761(2000)010[0350:LEACFA]2.0.CO;2
Cheung, W. W. L., Sarmiento, J. L., Dunne, J., Frölicher, T. L., Lam, V. W. Y., Deng Palomares, M. L., et al. (2012). Shrinking of fishes exacerbates impacts of global ocean changes on marine ecosystems. Nat. Clim. Change 3, 254–258. doi: 10.1038/NCLIMATE1691
Cheung, W. W. L., Watson, R., and Pauly, D. (2013). Signature of ocean warming in global fish catch. Nature 497, 365–368. doi: 10.1038/nature12156
Childress, J. J., and Seibel, B. A. (1998). Life at stable low oxygen levels: adaptations of animals to oceanic oxygen minimum layers. J. Exp. Biol. 201, 1223–1232.
Claireaux, G., and Lefrançois, C. (2007). Linking environmental variability and fish performance: integration through the concept of scope for activity. Philos. Trans. R. Soc. B Biol. Sci. 362, 2031–2041. doi: 10.1098/rstb.2007.2099
Clarke, A. (1991). What is cold adaptation and how should we measure it? Am. Zool. 31, 81–92. doi: 10.1093/icb/31.1.81
Clarke, A., and Johnston, N. M. (1999). Scaling of metabolic rate with body mass and temperature in teleost fish. J. Anim. Ecol. 68, 893–905. doi: 10.1046/j.1365-2656.1999.00337.x
Connett, R. J., Honig, C. R., Gayeski, T. E., and Brooks, G. A. (1990). Defining hypoxia: a systems view of VO2, glycolysis, energetics, and intracellular PO2. J. Appl. Physiol. 68, 833–842. doi: 10.1152/jappl.1990.68.3.833
Costantini, M., Ludsin, S. A., Mason, D. M., Zhang, X., Boicourt, W. C., and Brandt, S. B. (2008). Effect of hypoxia on habitat quality of striped bass (Morone saxatilis) in Chesapeake Bay. Can. J. Fish. Aquat. Sci. 65, 989–1002. doi: 10.1139/f08-021
Cowan, J. H. Jr., and Shaw, R. F. (2002). “Recruitment,” in Fishery Science: The Unique Contributions of Early Life Stages, eds L. A. Fuiman and R. G. Werner (Bodmin: MPG Books), 88–111.
Craig, J. K., and Crowder, L. B. (2005). Hypoxia-induced habitat shifts and energetic consequences in Atlantic croaker and brown shrimp on the Gulf of Mexico shelf. Mar. Ecol. Prog. Ser. 294, 79–94. doi: 10.3354/meps294079
Daewel, U., Peck, M. A., Kühn, W., Michael, A., Alekseeva, I., and Schrum, C. (2008). Coupling ecosystem and individual-based models to simulate the influence of environmental variability on potential growth and survival of larval sprat (Sprattus sprattus L.) in the North Sea. Fish. Oceanogr. 17, 333–351. doi: 10.1111/j.1365-2419.2008.00482.x
Dam, H. G. (2013). Evolutionary adaptation of marine zooplankton to global change. Annu. Rev. Mar. Sci. 5, 349–370. doi: 10.1146/annurev-marine-121211-172229
Davis, J. C. (1975). Minimal dissolved oxygen requirements of aquatic life with emphasis on Canadian species: a review. J. Fish. Board Can. 32, 2295–2332. doi: 10.1139/f75-268
de Mutsert, K., Steenbeek, J., Lewis, K., Buszowski, J., Cowan, J. H. Jr., and Christensen, V. (2016). Exploring effects of hypoxia on fish and fisheries in the northern Gulf of Mexico using a dynamic spatially explicit ecosystem model. Ecol. Model 331, 142–150. doi: 10.1016/j.ecolmodel.2015.10.013
Decker, M. B., Breitburg, D. L., and Marcus, N. H. (2003). Geographical differences in behavioral responses to hypoxia: local adaptation to an anthropogenic stressor? Ecol. Appl. 13, 1104–1109. doi: 10.1890/1051-0761(2003)13[1104:GDIBRT]2.0.CO;2
Decker, M. B., Breitburg, D. L., and Purcell, J. E. (2004). Effects of low dissolved oxygen on zooplankton predation by the ctenophore Mnemiopsis leidyi. Mar. Ecol. Prog. Ser. 280, 163–172. doi: 10.3354/meps280163
Deevey, G. B. (1960). Relative effects of temperature and food on seasonal variations in length of marine copepods in some eastern American and western European waters. Bull. Bingham Oceanogr. Collect. 17, 54–86.
Deutsch, C., Ferrel, A., Seibel, B., Pörtner, H. O., and Huey, R. B. (2015). Climate change tightens a metabolic constraint on marine habitats. Science 348, 1132–1135. doi: 10.1126/science.aaa1605
Diaz, R. J., and Breitburg, D. L. (2009). The hypoxic environment. Fish Physiol. 27, 1–23. doi: 10.1016/S1546-5098(08)00001-0
Diaz, R. J., and Rosenberg, R. (2008). Spreading dead zones and consequences for marine ecosystems. Science 321, 926–929. doi: 10.1126/science.1156401
Eby, L. A., Crowder, L. B., McClellan, C. M., Peterson, C. H., and Powers, M. J. (2005). Habitat degradation from intermittent hypoxia: impacts on demersal fishes. Mar. Ecol. Prog. Ser. 291, 249–262. doi: 10.3354/meps291249
Ekau, W., Auel, H., Pörtner, H.-O., and Gilbert, D. (2010). Impacts of hypoxia on the structure and processes in pelagic communities (zooplankton, macro-invertebrates and fish). Biogeosciences 7, 1669–1699. doi: 10.5194/bg-7-1669-2010
Ekau, W., and Verheye, H. M. (2005). Influence of oceanographic fronts and low oxygen on the distribution of ichthyoplankton in the Benguela and southern Angola currents. Afr. J. Mar. Sci. 27, 629–639. doi: 10.2989/18142320509504123
Elliott, D. T., Pierson, J. J., and Roman, M. R. (2012). Relationship between environmental conditions and zooplankton community structure during summer hypoxia in the northern Gulf of Mexico. J. Plankton Res. 34, 602–613. doi: 10.1093/plankt/fbs029
Elliott, D. T., Pierson, J. J., and Roman, M. R. (2013). Predicting the effects of coastal hypoxia on vital rates of the planktonic copepod Acartia tonsa Dana. PLoS One 8:e63987. doi: 10.1371/journal.pone.0063987
Evans, D. H., Claiborne, J. B., and Currie, S. (eds). (2014). The Physiology of Fishes, 4th Edn. Boca Raton, FL: CRC Press.
Forster, J., Hirst, A. G., and Woodward, G. (2011). Growth and development rates have different thermal responses. Am. Nat. 178, 668–678. doi: 10.1086/662174
Friedman, J. R., Condon, N. E., and Drazen, J. C. (2012). Gill surface area and metabolic enzyme activities of demersal fishes associated with the oxygen minimum zone off California. Limnol. Oceanogr. 57, 1701–1710. doi: 10.4319/lo.2012.57.6.1701
Frost, B. W., and Bollens, S. M. (1992). Variability of diel vertical migration in the marine planktonic copepod Pseudocalanus newmani in relation to its predators. Can. J. Fish. Aquat. Sci. 49, 1137–1141. doi: 10.1139/f92-126
Fry, F. E. (1957). “The aquatic respiration of fish,” in The Physiology of Fishes, Vol. 1, ed. M. E. Brown (New York, NY: Academic Press), 1–63.
Fry, F. E. J. (1971). “The Effect of environmental factors on the physiology of fish,” in Fish Physiology, Vol. 6, eds W. S. Hoar and D. J. Randall (New York, NY: Academic Press), 1–98.
Gamperl, A. K., and Driedzic, W. R. (2009). Cardiovascular function and cardiac metabolism. Fish Physiol. 27, 301–360. doi: 10.1016/S1546-5098(08)00007-1
Geist, S. J., Ekau, W., and Kunzmann, A. (2013). Energy demand of larval and juvenile Cape horse mackerels, Trachurus capensis, and indications for hypoxia tolerance as benefit in a changing environment. Mar. Biol. 160, 3221–3232. doi: 10.1007/s00227-013-2309-2
Geist, S. J., Kunzmann, A., Verheye, H. M., Eggert, A., Schukat, A., and Ekau, W. (2015). Distribution, feeding behaviour, and condition of Cape horse mackerel early life stages, Trachurus capensis, under different environmental conditions in the northern Benguela upwelling ecosystem. ICES J. Mar. Sci. 72, 543–557. doi: 10.1093/icesjms/fsu087
Giguere, L. A., Côté, B., and St-Pierre, J.-F. (1988). Metabolic rates scale isometrically in larval fishes. Mar. Ecol. Prog. Ser. 50, 13–19. doi: 10.3354/meps050013
Gillooly, J. F., Brown, J. H., West, G. B., Savage, V. M., and Charnov, E. L. (2001). Effects of size and temperature on metabolic rate. Science 293, 2248–2251. doi: 10.1126/science.1061967
Glaspie, C. N., Clouse, M., Adamack, A. T., Cha, Y. K., Ludsin, S. A., Mason, D. M., et al. (2018). Effect of hypoxia on diet of Atlantic bumper Chloroscombrus chrysurus in the Northern Gulf of Mexico. Trans. Am. Fish. Soc. 147, 740–748. doi: 10.1002/tafs.10063
Gnaiger, E. (1991). “Animal energetics at very low oxygen: information from calorimetry and respirometry,” in Physiological Strategies for Gas Exchange and Metabolism, eds A. J. Woakes, M. K. Grieshaber, and C. R. Bridges (Cambridge: Cambridge University Press), 141–171.
Graham, B. A., Chan, F., Nielsen, K. J., Fox, D. S., Barth, J. A., Huyer, A., et al. (2004). Upwelling-driven nearshore hypoxia signals ecosystem and oceanographic changes in the northeast Pacific. Nature 429, 749–751. doi: 10.1038/nature02605
Graham, W. M. (2001). “Numerical increases and distributional shifts of Chrysaora quinquecirrha (Desor) and Aurelia aurita (Linné)(Cnidaria: Scyphozoa) in the northern Gulf of Mexico,” in Developments in Hydrobiology, Jellyfish Blooms: Ecological and Societal Importance, eds J. E. Purcell, W. M. Graham, and H. J. Dumont (Dordrecht: Kluwer Academic Publishers), 97–111.
Grantham, B. A., Chan, F., Nielsen, K. J., Fox, D. S., Barth, J. A., Huyer, A., et al. (2004). Upwelling-driven nearshore hypoxia signals ecosystem and oceanographic changes in the northeast Pacific. Nature 429, 749–754. doi: 10.1038/nature02605
Grice, G. D., and Marcus, N. H. (1981). Dormant eggs of marine copepods. Oceanogr. Mar. Biol. Annu. Rev. 19, 125–140.
Hansen, B. W., and Drillet, G. (2013). Comparative oxygen consumption rates of subitaneous and delayed hatching eggs of the calanoid copepod Acartia tonsa (Dana). J. Exp. Mar. Biol. Ecol. 442, 66–96. doi: 10.1016/j.jembe.2013.01.029
Hays, G. C., Kennedy, H., and Frost, B. W. (2001). Individual variability in diel vertical migration of a marine copepod: why some individuals remain at depth when others migrate. Limnol. Oceanogr. 46, 2050–2054. doi: 10.4319/lo.2001.46.8.2050
Hazen, E. L., Craig, J. K., Good, C. P., and Crowder, L. B. (2009). Vertical distribution of fish biomass in hypoxic waters on the Gulf of Mexico shelf. Mar. Ecol. Prog. Ser. 375, 195–207. doi: 10.3354/meps07791
Herreid, C. F. (1980). Hypoxia in invertebrates. Comp. Biochem. Physiol. A Physiol. 67, 311–320. doi: 10.1016/S0300-9629(80)80002-8
Hirst, A. G., and Sheader, M. (1997). Are in situ weight specific growth rates body-size independent in marine planktonic copepods? Are-ananlysis of the global syntheses and a new empirical model. Mar. Ecol. Prog. Ser. 154, 155–165. doi: 10.3354/meps154155
Houde, E. D. (1989a). Comparative growth, mortality, and energetics of marine fish larvae: temperature and implied latitudinal effects. Fish. Bull. 87, 471–495.
Houde, E. D. (1989b). Subtleties and episodes in the early life of fishes. J. Fish Biol. 35(Suppl. A), 29–38. doi: 10.1111/j.1095-8649.1989.tb03043.x
Houde, E. D. (2008). Emerging from Hjort’s shadow. J. Northwest Atlantic Fish. Sci. 41, 53–70. doi: 10.2960/J.v41.m634
Houde, E. D. (2016). “Recruitment variability,” in Reproductive Biology of Fishes: Implications for Assessment and Management, 2nd Edn, eds T. Jakobsen, M. Fogarty, B. Megrey, and E. Moksness (Hoboken, NJ: John Wiley & Sons, Ltd), 98–187. doi: 10.1002/9781118752739.ch3
Houde, E. D., Chesney, E. J., Newberger, T. A., Vazquez, A. V., Zastrow, C. E., Morin, L. G., et al. (1989). Population biology of bay anchovy in mid-Chesapeake Bay (Final Report to Maryland Sea Grant, Reference Number [UMCES]CBL 89-141). Solomons, MD: Chesapeake Biological Laboratory.
Houde, E. D., and Madon, S. P. (1995). Fish in MEERC: Top-Down Controls-Bioenergetics Models and Experimental Protocols. Cambridge, MD: University of Maryland Center for Environmental Science.
Houde, E. D., and Schekter, R. C. (1983). Oxygen uptake and comparative energetics among eggs and larvae of three subtropical marine fishes. Mar. Biol. 72, 283–293. doi: 10.1007/BF00396834
Houde, E. D., and Zastrow, C. E. (1993). Ecosystem-and taxon-specific dynamic and energetics properties of larval fish assemblages. Bull. Mar. Sci. 53, 290–335.
Howarth, R. W., Billen, G., Swaney, D., Townsend, A., Jaworski, N., Lajtha, K., et al. (1996). “Regional nitrogen budgets and riverine N and P fluxes for the drainages to the North Atlantic Ocean: Natural and human influences,” in Nitrogen Cycling in the North Atlantic Ocean and its Watersheds, ed. R. W. Howarth (Dordrecht: Kluwer Academic Publishers), 75–139.
Huntley, M., and Brooks, E. R. (1982). Effects of age and food availability on diel vertical migration of Calanus pacificus. Mar. Biol. 71, 23–31. doi: 10.1007/BF00396989
Huntley, M. E., and Lopez, M. D. (1992). Temperature-dependent production of marine copepods: a global synthesis. Am. Nat. 140, 201–242. doi: 10.1086/285410
Ikeda, T. (1970). Relationship between respiration rate and body size in marine plankton animals as a function of the temperature of habitat. Bull. Fac. Fish. Hokkaido Univ. 21, 91–112.
Ikeda, T. (1985). Metabolic rates of epipelagic marine zooplankton as a function of body mass and temperature. Mar. Biol. 85, 1–11. doi: 10.1007/BF00396409
Ikeda, T., Sano, F., and Yamaguchi, A. (2007). Respiration in marine pelagic copepods: a global-bathymetric model. Mar. Ecol. Prog. Ser. 339, 215–219. doi: 10.3354/meps339215
Invidia, M., Sei, S., and Gorbi, G. (2004). Survival of the copepod Acartia tonsa following egg exposure to near anoxia and to sulfide at different pH values. Mar. Ecol. Prog. Ser. 276, 187–196. doi: 10.3354/meps276187
Isensee, K., Levin, L. A., Breitburg, D., Gregoire, M., Garçon, V., and Valdés, L. (2015). The Ocean is Losing its Breath. Ocean and Climate, Scientific notes. Available at: www.ocean-climate.org
Ito, S.-I., Rose, K., Megrey, B. A., Schweigert, J. F., Hay, D. E., Werner, F. E., et al. (2015). Geographic variation in Pacific herring growth in response to regime shifts in the North Pacific Ocean. Prog. Oceanogr. 138, 331–347. doi: 10.1016/j.pocean.2015.05.022
Jiang, X. D., Wang, G. H., and Li, S. J. (2006). Population dynamics of Acartia pacifica (Copepods: calanoida) the importance of benthic-pelagic coupling. Acta Oceanol. Sin. 25, 88–98.
Katajisto, T. (2004). Effects of anoxia and hypoxia on the dormancy and survival of subitaneous eggs of Acartia bifilosa (Copepods: Calanoidia). Mar. Biol. 145, 751–757.
Keister, J. E., Houde, E. D., and Breitburg, D. L. (2000). Effects of bottom-layer hypoxia on abundances and depth distributions of organisms in Patuxent River, Chesapeake Bay. Mar. Ecol. Prog. Ser. 205, 43–59. doi: 10.3354/meps205043
Keister, J. E., and Tuttle, L. B. (2013). Effects of bottom-layer hypoxia on spatial distributions and community structure of mesozooplankton in a sub-estuary of Puget Sound, Washington, USA. Limnol. Oceanogr. 58, 667–680. doi: 10.4319/lo.2013.58.2.0667
Kilduff, D. P., Botsford, L. W., and Teo, S. L. H. (2014). Spatial and temporal covariability in early ocean survival of Chinook salmon (Oncorhynchus tshawytscha) along the west coast of North America. ICES J. Mar. Sci. 71, 1671–1682. doi: 10.1093/icesjms/fsu031
Killen, S. S., Atkinson, D., and Glazier, D. S. (2010). The intraspecific scaling of metabolic rate with body mass in fishes depends on lifestyle and temperature. Ecol. Lett. 13, 184–193. doi: 10.1111/j.1461-0248.2009.01415.x
Kimmel, D. G., Boynton, W. R., and Roman, M. R. (2012). Long-term decline in the calanoid copepod Acartia tonsa in central Chesapeake Bay, USA: An indirect effect of eutrophication? Estuar. Coast. Shelf Sci. 101, 76–85. doi: 10.1016/j.ecss.2012.02.019
Kimmel, D. G., Miller, W. D., Harding, L. W. Jr., Houde, E. D., and Roman, M. R. (2009). Estuarine ecosystem response captured using a synoptic climatology. Estuar. Coasts 32, 403–409. doi: 10.1007/s12237-009-9147-y
Kiørboe, T., Munk, P., and Richardson, K. (1987). Respiration and growth of larval herring Clupea harengus: relation between specific dynamic action and growth efficiency. Mar. Ecol. Prog. Ser. 40, 1–10. doi: 10.3354/meps040001
Klumb, R. A., Bunch, K. L., Mills, E. L., Rudstam, L. G., Brown, G., Knauf, C., et al. (2004). Establishment of a metalimnetic oxygen refuge for zooplankton in a productive Lake Ontario embayment. Ecol. Appl. 14, 113–131. doi: 10.1890/02-5054
Knutsen, T., Melle, W., and Calise, L. (2001). Determining the mass density of marine copepods and their eggs with a critical focus on some of the previously used methods. J. Plankton Res. 23, 859–873. doi: 10.1093/plankt/23.8.859
Ludsin, S. A., Zhang, X., Brandt, S. B., Roman, M. R., Boicourt, W. C., Mason, D. M., et al. (2009). Hypoxia-avoidance by planktivorous fish in Chesapeake Bay: implications for food web interactions and fish recruitment. J. Exp. Mar. Biol. Ecol. 381, S121–S131. doi: 10.1016/j.jembe.2009.07.016
Luo, J., and Brandt, S. B. (1993). Bay anchovy Anchoa mitchilli production and consumption in mid-Chesapeake Bay based on a bioenergetics model and acoustic measures of fish abundance. Mar. Ecol. Prog. Ser. 98, 223–223. doi: 10.3354/meps098223
Lutz, R. V., Marcus, N. H., and Chanton, J. P. (1992). Effects of low oxygen concentrations on the hatching and viability of eggs of marine calanoid copepods. Mar. Biol. 114, 241–247. doi: 10.1007/BF00349525
Malone, T. C. (1991). “River flow, phytoplankton production and oxygen depletion in Chesapeake Bay,” in Modern and Ancient Continental Shelf Anoxia: An Overview, Vol. 58, eds R. V. Tyson and T. H. Pearson (London: Geological Society of London Special Publications), 83–93. doi: 10.1144/GSL.SP.1991.058.01.06
Mandic, M., Todgham, A. E., and Richards, J. G. (2009). Mechanisms and evolution of hypoxia tolerance in fish. Proc. R. Soc. B Biol. Sci. 276, 735–744. doi: 10.1098/rspb.2008.1235
Marcus, N. H. (2001). “Zooplankton: responses to and consequences of hypoxia,” in Coastal Hypoxia: Consequences for Living Resources and Ecosystems, eds N. N. Rabalais and R. E. Turner (Washington, DC: American Geophysical Union), doi: 10.1029/CE058p0049
Marcus, N. H., and Lutz, R. V. (1994). Effects of anoxia on the viability of subitaneous eggs of planktonic copepods. Mar. Biol. 121, 83–87. doi: 10.1007/BF00349476
Marcus, N. H., Lutz, R., Burnett, W., and Cable, P. (1994). Age, viability, and vertical distribution of zooplankton resting eggs from an anoxic basin: evidence of an egg bank. Limnol. Oceanogr. 39, 154–158. doi: 10.4319/lo.1994.39.1.0154
Marcus, N. H., Lutz, R. V., and Chanton, J. P. (1997). Impact of anoxia and sulfide on the viability of eggs of three planktonic copepods. Mar. Ecol. Prog. Ser. 146, 291–295. doi: 10.3354/meps146291
Marcus, N. H., Richmond, C., Sedlacek, C., Miller, G. A., and Oppert, C. (2004). Impact of hypoxia on the survival, egg production and population dynamics of Acartia tonsa Dana. J. Exp. Mar. Biol. Ecol. 301, 111–128. doi: 10.1016/j.jembe.2003.09.016
Marshall, S. M. (1973). Respiration and feeding in copepods. Adv. Mar. Biol. 11, 57–120. doi: 10.1016/S0065-2881(08)60268-0
McAllen, R., Taylor, A. C., and Davenport, J. (1999). The effects of temperature and oxygen partial pressure on the rate of oxygen consumption of the high-shore rock pool copepod Tigriopus brevicornis. Comp. Biochem. Physiol. A Mol. Integr. Physiol. 123, 195–202. doi: 10.1016/S1095-6433(99)00050-1
McBryan, T. L., Anttila, K., Healy, T. M., and Schulte, P. M. (2013). Responses to temperature and hypoxia as interacting stressors in fish: implications for adaptation to environmental change. Integr. Comp. Biol. 53, 648–659. doi: 10.1093/icb/ict066
McLaren, I. (1963). Effects of temperature on growth of zooplankton and the adaptive value of vertical migration. J. Fish. Res. Board Can. 20, 685–727. doi: 10.1139/f63-046
Miller, C. B., Johnson, J. K., and Heinle, D. R. (1977). Growth rules in the marine copepod genus Acartia. Limnol. Oceanogr. 22, 326–335. doi: 10.4319/lo.1977.22.2.0326
Muir, B. S. (1969). Gill dimensions as a function of fish size. J. Fish. Res. Board. Can. 26, 165–170. doi: 10.1139/f69-018
Nilsson, G. E., and Östlund-Nilsson, S. (2008). Does size matter for hypoxia tolerance in fish? Biol. Rev. 83, 173–189. doi: 10.1111/j.1469-185X.2008.00038.x
Nissling, A., and Vallin, L. (1996). The ability of Baltic cod eggs to maintain neutral buoyancy and the opportunity for survival in fluctuating conditions in the Baltic Sea. J. Fish. Biol. 48, 217–227. doi: 10.1111/j.1095-8649.1996.tb01114.x
Nixon, S. W. (1995). Coastal marine eutrophication: a definition, social causes, and future concerns. Ophelia 41, 199–219. doi: 10.1080/00785236.1995.10422044
North, E. W., and Houde, E. D. (2004). Distribution and transport of bay anchovy (Anchoa mitchilli) eggs and larvae in Chesapeake Bay. Estuar. Coast. Shelf Sci. 60, 409–429. doi: 10.1016/j.ecss.2004.01.011
Pauly, D. (2010). Gasping Fish and Panting Squids: Oxygen, Temperature and the Growth of Water-Breathing Animals. International Ecology Institute. Excellence in Ecology, Vol. 22. Germany: Oldendorf/Luhe, 216.
Pearre, S. (2000). Long-term changes in diel vertical migration behavior: more ups and downs. Mar. Ecol. Prog. Ser. 197, 305–307. doi: 10.3354/meps197305
Peck, M. A., Huebert, K. B., and Llopiz, J. K. (2012). Intrinsic and extrinsic factors driving match-mismatch dynamics during the early life history of marine fishes. Adv. Ecol. Res. 47, 177–302. doi: 10.1016/B978-0-12-398315-2.00003-X
Perry, P. C. (2011). The Role of Compensatory Dynamics AND Influence of Environmental Factors Across Multiple Spatial Scales in Structuring Fish Populations (Order No. 1510259). Available from ProQuest Dissertations & Theses Global. (1015396170). Available at: https://search.proquest.com/docview/1015396170?accountid=28934
Pierson, J. J., Frost, B. W., Thoreson, D., Leising, A. W., Postel, J. R., and Nuwer, M. (2009a). Trapping migrating zooplankton. Limnol. Oceanogr. Methods 7, 334–346. doi: 10.4319/lom.2009.7.334
Pierson, J. J., Roman, M. R., Kimmel, D. G., Boicourt, W. C., and Zhang, X. (2009b). Quantifying changes in the vertical distribution of mesozooplankton in response to hypoxic bottom waters. J. Exp. Mar. Biol. Ecol. 381(Suppl.), S74–S79. doi: 10.1016/j.jembe.2009.07.013
Pierson, J. J., Slater, W. C. L., Elliott, D., and Roman, M. R. (2017). Synergistic effects of seasonal deoxygenation and temperature truncate copepod vertical migration and distribution. Mar. Ecol. Prog. Ser. 575, 57–68. doi: 10.3354/meps12205
Pörtner, H. O. (2010). Oxygen-and capacity-limitation of thermal tolerance: a matrix for integrating climate-related stressor effects in marine ecosystems. J. Exp. Biol. 213, 881–893. doi: 10.1242/jeb.037523
Pörtner, H. O., and Knust, R. (2007). Climate change affects marine fishes through the oxygen limitation of thermal tolerance. Science 315, 95–97. doi: 10.1126/science.1135471
Pothoven, S. A., Vanderploeg, H. A., Höök, T. O., and Ludsin, S. A. (2012). Hypoxia modifies planktivore-zooplankton interactions in Lake Erie. Can. J. Fish. Aquat. Sci. 69, 2018–2028. doi: 10.1139/cjfas-2012-0144
Prince, E. D., and Goodyear, C. P. (2006). Hypoxia-based habitat compression of tropical pelagic fishes. Fish. Oceanogr. 15, 451–464. doi: 10.1111/gcb.13799
Prince, E. D., Luo, J., Goodyear, C. P., Hoolihan, J. P., Snodgrass, D., Orbesen, E. S., et al. (2010). Ocean scale hypoxia-based habitat compression of Atlantic istiophorid billfishes. Fish. Oceanogr. 19, 448–462. doi: 10.1111/j.1365-2419.2010.00556.x
Prosser, C. L., and Brown, F. A. Jr. (1962). Comparative Animal Physiology. Philadelphia, PA: Saunders.
Qureshi, N. A., and Rabalais, N. N. (2001). “Distribution of zooplankton on a seasonally hypoxic continental shelf,” in Coastal Hypoxia: Consequences for Living Resources and Ecosystems, Vol. 58, eds N. N. Rabalais and R. E. Turner (Washington, DC: American Geophysical Union), 61–76. doi: 10.1029/CE058p0061
Rabalais, N. N., Diaz, R. J., Levin, L. A., Turner, R. E., Gilbert, D., and Zhang, J. (2010). Dynamics and distribution of natural and human-caused hypoxia. Mar. Ecol. Prog. Ser. 445, 75–95. doi: 10.5194/bg-7-585-2010
Rabalais, N. N., Turner, R. E., Díaz, R. J., and Justiæ, D. (2009). Global change and eutrophication of coastal waters. ICES J. Mar. Sci. 66, 1528–1537. doi: 10.1093/icesjms/fsp047
Raymont, J. E. G., and Gauld, D. T. (1951). The respiration of some planktonic copepods. J. Mar. Biol. Assoc. U. K. 29, 681–693. doi: 10.1017/S0025315400052863
Richards, J. G. (2009). “Chapter 10 metabolic and molecular responses of fish to hypoxia,” in Fish Physiology, Vol. 27, eds J. G. Richards, A. P. Farrell, and C. J. Brauner (Amsterdam: Elsevier), 443–485. doi: 10.1016/S1546-5098(08)00010-1
Richards, J. G. (2011). Physiological, behavioral and biochemical adaptations of intertidal fishes to hypoxia. J. Exp. Biol. 214, 191–199. doi: 10.1242/jeb.047951
Richmond, C., Marcus, N. H., Sedlacek, C., Miller, G. A., and Oppert, C. (2006). Hypoxia and seasonal temperature: short-term effects and long-term implications for Acartia tonsa dana. J. Exp. Mar. Biol. Ecol. 328, 177–196. doi: 10.1016/j.jembe.2005.07.004
Rogers, N. J., Urbina, M. A., Reardon, E. E., McKenzie, D. J., and Wilson, R. W. (2016). A new analysis on hypoxia tolerance in fishes using a database of critical oxygen level (Pcrit). Conserv. Physiol. 4:cow12. doi: 10.1093/conphys/cow012
Roman, M. R., Ashton, K. A., and Gauzens, A. L. (1988). “Day/night differences in the grazing impact of marine copepods,” in Developments in Hydrobiology: Biology of Copepods, eds G. Boxshall and H. K. Schminke (Dordrecht: Kluwer Academic Publishers), 21–30.
Roman, M. R., Gauzens, A. L., Rhinehart, W. K., and White, J. R. (1993). Effects of low oxygen waters on Chesapeake Bay zooplankton. Limnol. Oceanogr. 38, 1603–1614. doi: 10.4319/lo.1993.38.8.1603
Roman, M. R., Pierson, J. J., Kimmel, D. G., Boicourt, W. C., and Zhang, X. (2012). Impacts of hypoxia on zooplankton spatial distributions in the northern Gulf of Mexico. Estuar. Coasts 35, 1261–1269. doi: 10.1007/s12237-012-9531-x
Rombough, P. J. (1988). Growth, aerobic metabolism, and dissolved oxygen requirements of embryos and alevins of steelhead, Salmo gairdneri. Can. J. Zool. 66, 651–660. doi: 10.1139/z88-097
Sato, M., Horne, J. K., Parker-Stetter, S. L., Essington, T. E., Keister, J. E., Moriarty, P. E., et al. (2016). Impacts of moderate hypoxia on fish and zooplankton prey distributions in a coastal fjord. Mar. Ecol. Prog. Ser. 560, 57–72. doi: 10.3354/meps11910
Searcy, S. P., Eggleston, D. B., and Hare, J. A. (2007). Environmental influences on the relationship between juvenile and larval growth of Atlantic croaker Micropogonias undulatus. Mar. Ecol. Prog. Ser. 349, 81–88. doi: 10.3354/meps07124
Seibel, B. A. (2011). Critical oxygen levels and metabolic suppression in oceanic oxygen minimum zones. J. Exp. Biol. 214, 326–336. doi: 10.1242/jeb.049171
Shang, E. H., and Wu, R. S. (2004). Aquatic hypoxia is a teratogen and affects fish embryonic development. Environ. Sci. Technol. 38, 4763–4767. doi: 10.1021/es0496423
Shelford, V. E., and Allee, W. C. (1913). The reactions of fishes to gradients of dissolved atmospheric gases. J. Exp. Zool. 14, 207–266. doi: 10.1002/jez.1400140203
Speers-Roesch, B., Mandic, M., Groom, D. J. E., and Richards, J. G. (2013). Critical oxygen tensions as predictors of hypoxia tolerance and tissue metabolic responses during hypoxia exposure in fishes. J. Exp. Mar. Biol. Ecol. 449, 239–249. doi: 10.1016/j.jembe.2013.10.006
Speers-Roesch, B., Richards, J. G., Brauner, C. J., Farrell, A. P., Hickey, A. J., Wang, Y. S., et al. (2012). Hypoxia tolerance in elasmobranchs. I. Critical oxygen tension as a measure of blood oxygen transport during hypoxia exposure. J. Exp. Biol. 215, 93–102. doi: 10.1242/jeb.059642
Stalder, L. C., and Marcus, N. H. (1997). Zooplankton responses to hypoxia: behavioral patterns and survival of three species of calanoid copepods. Mar. Biol. 127, 599–607. doi: 10.1007/s002270050050
Tanaka, H., Aoki, I., and Ohshimo, S. (2006). Feeding habits and gill raker morphology of three planktivorous pelagic fish species off the coast of northern and western Kyushu in summer. J. Fish Biol. 68, 1041–1061. doi: 10.1111/j.0022-1112.2006.00988.x
Tang, K. W., Dam, H. G., and Feinberg, L. R. (1998). The relative importance of egg production rate, hatching success, hatching duration and egg sinking in population recruitment of two species of marine copepods. J. Plankton Res. 20, 1971–1987. doi: 10.1093/plankt/20.10.1971
Taylor, J. C., and Miller, J. M. (2001). Physiological performance of juvenile southern flounder, Paralichthys lethostigma (Jordan and Gilbert, 1884), in chronic and episodic hypoxia. J. Exp. Mar. Biol. Ecol. 258, 195–214. doi: 10.1016/S0022-0981(01)00215-5
Taylor, J. C., and Rand, P. S. (2003). Spatial overlap and distribution of anchovies (Anchoa spp.) and copepods in a shallow stratified estuary. Acoust. Fish. Aquat. Ecol. 16, 191–196. doi: 10.1016/S0990-7440(03)00012-3
Taylor, J. C., Rand, P. S., and Jenkins, J. (2007). Swimming behavior of juvenile anchovies (Anchoa spp.) in an episodically hypoxic estuary: implications for individual energetics and trophic dynamics. Mar. Biol. 152, 939–957. doi: 10.1007/s00227-007-0745-6
Teuber, L., Kiko, R., Séguin, F., and Auel, H. (2013). Respiration rates of tropical Atlantic copepods in relation to the oxygen minimum zone. J. Exp. Mar. Biol. Ecol. 448, 28–36. doi: 10.1371/journal.pone.0077590
Thomas, P., and Rahman, S. (2012). Extensive reproductive disruption, ovarian masculinization and aromatase suppression in Atlantic Croaker in the northern Gulf of Mexico hypoxic zone. Proc. R. Soc. B 1726, 28–38. doi: 10.1098/rspb.2011.0529
Thronson, A., and Quigg, A. (2008). Fifty-five years of fish kills in Coastal Texas. Estuar. Coasts. 31, 802–813. doi: 10.1007/sl2237-008-9056-5
Turner, R. E. (2001). “Some effects of nutrient loading and hypoxia on pelagic and demersal marine food webs,” in Coastal Hypoxia: Consequences for Living Resources and Ecosystems, Coastal and Estuarine Studies, Vol. 58, eds N. N. Rabalais and R. E. Turner (Washington, DC: American Geophysical Union), 371–398.
Turner, R. E., Rabalais, N. N., and Justic, D. (2008). Gulf of Mexico hypoxia: alternate states and a legacy. Environ. Sci. Technol. 42, 2323–2327. doi: 10.1021/es071617k
Uye, S. (1980). Development of neritic copepods Acartia clausi and A. steuri. 1. Some environmental factors affecting egg development and the nature of resting eggs. Bull. Plankton Soc. Jpn. 27, 1–9.
Uye, S. (1994). “Replacement of large copepods by small ones with eutrophication of embayments: cause and consequence,” in Developments in Hydrobiology: Ecology and Morphology of Copepods, Vol. 102, eds F. D. Ferrari and B. P. Bradley (Dordrecht: Kluwer Academic Publishers), 513–519. doi: 10.1007/978-94-017-1347-4_64
Uye, S. I. (1982). Length-weight relationships of important zooplankton from the Inland Sea of Japan. J. Oceanogr. Soc. Jpn. 38, 149–158. doi: 10.1007/BF02110286
Vanderploeg, H. A., Ludsin, S. A., Ruberg, S. A., Hook, T. O., Pothoven, S. A., Brandt, S. B., et al. (2009). Hypoxia affects spatial distributions and overlap of pelagic fish, zooplankton, and phytoplankton in Lake Erie. J. Exp. Mar. Biol. Ecol. 381, S92–S107. doi: 10.1016/j.jembe.2009.07.027
Vaquer-Sunyer, R., and Duarte, C. M. (2008). Thresholds of hypoxia for marine biodiversity. Proc. Natl. Acad. Sci. U.S.A. 105, 15452–15457. doi: 10.1073/pnas.0803833105
Vaquer-Sunyer, R., and Duarte, C. M. (2011). Temperature effects on oxygen thresholds for hypoxia in marine benthic organisms. Glob. Change Biol. 17, 1788–1797. doi: 10.1111/j.1365-2486.2010.02343.x
Vargo, S. L., and Sastry, A. N. (1977). Acute temperature and low dissolved oxygen tolerances of brachyuran crab (Cancer irroratus) larvae. Mar. Biol. 40, 165–171. doi: 10.1007/BF00396263
Vazquez, A. V., and Houde, E. D. (1989). “Chapter 6. Energetics of bay anchovy, Anchoa mitchilli: ration levels and temperature effects,” in Population biology of bay anchovy in mid-Chesapeake Bay, eds E. D. Houde et al. (Solomons, MD: Center for Environmental and Estuarine Studies, Chesapeake Biological Laboratory), 108–148.
Vejřík, L., Matějíčková, I., Jůza, T., Frouzová, J., Seïa, J., Blabolil, P., et al. (2016). Small fish use the hypoxic pelagic zone as a refuge from predators. Fresh. Biol. 61, 899–913. doi: 10.1111/fwb.12753
Verberk, W. C., Bilton, D. T., Calosi, P., and Spicer, J. I. (2011). Oxygen supply in aquatic ectotherms: partial pressure and solubility together explain biodiversity and size patterns. Ecology 92, 1565–1572. doi: 10.1890/10-2369.1
Wang, S. B. (1998). Comparative studies of the life history and production potential of bay anchovy Anchoa mitchilli and northern anchovy Engraulis mordax: an individual-based modeling approach. Diss. Abstr. Int. B Sci. Eng.59, 4555.
Wang, T., Lefevre, S., Thanh Huong, D. T., Cong, N., and van Bayley, M. (2009). “Chapter 8 The Effects of Hypoxia On Growth and Digestion,” in Fish Physiology, Vol. 27, eds J. G. Richards, A. P. Farrell, and C. J. Brauner (Amsterdam: Academic Press), 361–396.
Wannamaker, C. M., and Rice, J. A. (2000). Effects of hypoxia on movements and behavior of selected estuarine organisms from the southeastern United States. J. Exp. Mar. Biol. Ecol. 249, 145–163. doi: 10.1016/S0022-0981(00)00160-X
Webster, C. N., Hansson, S., Didrikas, T., Gorokhova, E., Peltonen, H., Brierley, A. S., et al. (2015). Stuck between a rock and a hard place: zooplankton vertical distribution and hypoxia in the Gulf of Finland. Baltic Sea. Mar. Biol. 162, 1429–1440. doi: 10.1007/s00227-015-2679-8
Wells, M. M. (1913). The resistance of fishes to different concentrations and combinations of oxygen and carbon dioxide. Biol. Bull. 25, 323–347. doi: 10.1086/BBLv25n6p323
Wells, R. M. (2009). “Blood-Gas Transport and Hemoglobin Function: Adaptations for Functional and Environmental Hypoxia,” in Fish Physiology, Vol. 27, eds J. G. Richards, A. P. Farrell, and C. J. Brauner (Amsterdam: Academic Press), 255–299.
Wu, R. S. (2002). Hypoxia: from molecular responses to ecosystem responses. Mar. Pollut. Bull. 45, 35–45. doi: 10.1016/S0025-326X(02)00061-9
Wu, R. S. (2009). “Effects of hypoxia on fish reproduction and development,” in Fish Physiology, Vol. 27, eds J. G. Richards, A. P. Farrell, and C. J. Brauner (Amsterdam: Academic Press), 79–141.
Zhang, C., Zhang, W., Ni, X., Zhao, Y., Huang, L., and Xiao, T. (2015). Influence of different water masses on planktonic ciliate distribution on the East China Sea shelf. J. Mar. Syst. 141, 98–111. doi: 10.1016/j.jmarsys.2014.09.003
Zhang, H., Ludsin, S. A., Mason, D. M., Adamack, A. T., Brandt, S. B., Zhang, X., et al. (2009). Hypoxia-driven changes in the behavior and spatial distribution of pelagic fish and mesozooplankton in the northern Gulf of Mexico. J. Exp. Mar. Biol. Ecol. 381, S80–S91. doi: 10.1016/j.jembe.2009.07.014
Zhang, H., Mason, D. M., Stow, C. A., Adamack, A. T., Brandt, S. B., Zhang, X., et al. (2014). Effects of hypoxia on habitat quality of pelagic planktivorous fishes in the northern Gulf of Mexico. Mar. Ecol. Prog. Ser. 505, 209–226. doi: 10.3354/meps10768
Keywords: deoxygenation, climate change, food-webs, stressor, metabolism
Citation: Roman MR, Brandt SB, Houde ED and Pierson JJ (2019) Interactive Effects of Hypoxia and Temperature on Coastal Pelagic Zooplankton and Fish. Front. Mar. Sci. 6:139. doi: 10.3389/fmars.2019.00139
Received: 03 June 2018; Accepted: 05 March 2019;
Published: 22 March 2019.
Edited by:
Arthur Capet, MAST – University of Liège, BelgiumReviewed by:
John Patrick Dunne, Geophysical Fluid Dynamics Laboratory (GFDL), United StatesAndrew Altieri, University of Florida, United States
José Lino Vieira De Oliveira Costa, Universidade de Lisboa, Portugal
Copyright © 2019 Roman, Brandt, Houde and Pierson. This is an open-access article distributed under the terms of the Creative Commons Attribution License (CC BY). The use, distribution or reproduction in other forums is permitted, provided the original author(s) and the copyright owner(s) are credited and that the original publication in this journal is cited, in accordance with accepted academic practice. No use, distribution or reproduction is permitted which does not comply with these terms.
*Correspondence: Michael R. Roman, cm9tYW5AdW1jZXMuZWR1