- 1GEOMAR Helmholtz Centre for Ocean Research Kiel, Kiel, Germany
- 2Instituto Español de Oceanografía (IEO), Centro Oceanográfico de A Coruña, A Coruña, Spain
- 3Department of Oceanography, Center for Climate and Resilience Research (CR2), University of Concepción, Concepción, Chile
- 4Centre for Ocean and Atmospheric Sciences, School of Environmental Sciences, University of East Anglia, Norwich, United Kingdom
- 5National Institute of Water and Atmospheric Research, Wellington, New Zealand
- 6Department of Chemistry, University of Otago, Dunedin, New Zealand
- 7Plymouth Marine Laboratory, Plymouth, United Kingdom
- 8Department of Marine Chemistry, Leibniz Institute for Baltic Sea Research Warnemünde, Rostock, Germany
- 9Department of Earth, Ocean and Atmospheric Sciences – Department of Botany, The University of British Columbia, Vancouver, BC, Canada
- 10School of Natural and Environmental Sciences, Newcastle University, Newcastle upon Tyne, United Kingdom
- 11Daniel K. Inouye Center for Microbial Oceanography: Research and Education, University of Hawai’i at Mānoa, Honolulu, HI, United States
Nitrous oxide (N2O) is an important atmospheric trace gas involved in tropospheric warming and stratospheric ozone depletion. Estimates of the global ocean contribution to N2O emissions average 21% (range: 10 to 53%). Ongoing environmental changes such as warming, deoxygenation and acidification are affecting oceanic N2O cycling and emissions to the atmosphere. International activities over the last decades aimed at improving estimates of global N2O emissions, including (i) the MarinE MethanE and NiTrous Oxide database (MEMENTO) for archiving of quality-controlled data, and (ii) a recent large-scale inter-laboratory comparison by Working Group 143 of the Scientific Committee on Ocean Research (SCOR). To reduce uncertainties in oceanic N2O emission estimates and to characterize the spatial and temporal variability in N2O distributions in a changing ocean, we propose the establishment of a harmonized N2O Observation Network (N2O-ON) combining discrete and continuous data from various platforms. The network will integrate observations obtained by calibrated techniques, using time series measurements at fixed stations and repeated hydrographic sections on voluntary observing ships and research vessels. In addition to exploiting existing oceanographic infrastructure, we propose the establishment of central calibration facilities in selected international laboratories to improve accuracy, and ensure standardization and comparability of N2O measurements. Final data products will include a harmonized global N2O concentration and emission fields for use in model validation and projections of future oceanic N2O emissions, to inform the global research community and policy makers.
Introduction
Nitrous oxide (N2O; laughing gas) is an atmospheric trace gas, which accounts for 6% of tropospheric warming by greenhouse gasses, and is a major ozone-depleting compound in the stratosphere (Ravishankara et al., 2009; IPCC, 2013; WMO, 2014). Emission estimates indicate that the oceans may contribute 10 to 53% of combined natural and anthropogenic N2O sources (Anderson et al., 2010; Ciais et al., 2013). N2O has been measured in the water column of all major ocean basins, in most marginal seas and in numerous estuaries (Kock and Bange, 2015; Murray et al., 2015), with measurements from the surface mixed layer down to 9800 m in the deep Izu-Ogasawara Trench (Kawagucci et al., 2018). These and other studies show that N2O concentrations may vary over three orders of magnitude from the open ocean to coastal shelves and semi-enclosed basins. Concentrations range from <1 nmol L-1 in the permanently anoxic deep basin waters of the Black Sea and Cariaco Trench (Hashimoto et al., 1983; Westley et al., 2006) to ≈1000 nmol L-1 in coastal near-surface waters off Peru (Arévalo-Martínez et al., 2015) and ≈1500 nmol L-1 in the suboxic deep waters of the Baltic Sea (Rönner, 1983). Some estuaries may reach similarly high concentrations (Barnes and Upstill-Goddard, 2011).
While the oceans are clearly a major natural contributor to atmospheric N2O, quantitative estimates remain highly uncertain (Buitenhuis et al., 2018). This uncertainty reflects the low number of marine N2O measurements to date, as compared to, for example, CO2 [see e.g., (Bakker et al., 2016)], and the lack of information on (i) seasonal and inter-annual variability, (ii) land-ocean gradients, (iii) the effects of small scale/mesoscale features (Grundle et al., 2017) and (iv) extreme events such as storms (Naik et al., 2008). There is also uncertainty in the relative importance of the various biological processes driving the production and consumption of N2O in oceanic waters, and their potential responses to changing oceanic conditions (Bange et al., 2010). Likewise, the influence of sea ice on N2O emissions from high-latitude ecosystems is currently unknown (Vancoppenolle et al., 2013). Randall et al. (2012), for instance, showed that sea ice formation and melting cycles can reverse the direction of the N2O fluxes across the ocean/atmosphere interface. Yet, the overall impact of these processes on the annual cycle is still unclear.
Oceanic N2O production and consumption principally occurs in subsurface and deep waters. Microbial nitrification (N2O is a by-product of ammonia oxidation to nitrite), partial denitrification (reduction of nitrate to N2O), and nitrifier-denitrification (i.e., nitrifier switching to nitrite reduction under low O2 conditions) are considered to be the main oceanic N2O production pathways, whereas, the main N2O sink is via reduction to N2 by denitrification in anoxic waters (Bange et al., 2010). Extreme accumulation of N2O resulting from nitrification and/or denitrification has been found at oxic/anoxic boundaries within oxygen minimum zones (OMZ) of the eastern tropical North/South Pacific Ocean and the Arabian Sea, and also in coastal shelf waters (Bange et al., 2010). In addition, several studies indicate N2O production via nitrification in surface waters of the open ocean (Dore and Karl, 1996; Law and Ling, 2001; Morell et al., 2001) and in estuaries (Barnes and Upstill-Goddard, 2011), as well as its possible consumption during microbial N2O fixation (Farías et al., 2013; Cornejo et al., 2015).
Environmental changes such as ocean warming (and associated changes in stratification and ice coverage), acidification, deoxygenation, and eutrophication due to increasing anthropogenic inputs of nutrients (via rivers and atmospheric deposition), may significantly alter N2O production and consumption, its distribution patterns and, ultimately, its release to the atmosphere (Kroeze et al., 2005; Zhang et al., 2010; Suntharalingam et al., 2012; Rees et al., 2016; Myllykangas et al., 2017). Indeed, model projections that account for ocean warming and atmospheric nitrogen deposition show a net decrease of 4 to 24% in future global oceanic N2O emissions during the 21st century (Martinez-Rey et al., 2015; Landolfi et al., 2017; Battaglia and Joos, 2018). One model projection suggests that the decrease of N2O emissions in the 21st century might be followed by a substantial increase of the N2O emissions in the 22nd century (Battaglia and Joos, 2018). The large degree of uncertainty in future N2O emission projections results partly from the limitations of existing N2O concentration data used in model parameterizations and validation. These current data sets are not yet cross-calibrated (their comparability is limited due to missing standard measurement protocols), and are biased by poor spatio-temporal coverage of the ocean (Kock and Bange, 2015).
The importance of additional, routine oceanic N2O measurements is recognized by the Global Ocean Observing System (GOOS) program, which recently added N2O to its list of Essential Ocean Variables (EOV)1.
To reduce uncertainties in current global N2O marine emission estimates, better constrain and understand temporal and spatial variability, and improve future projections of N2O concentrations in a changing ocean, we propose the establishment of a harmonized Global N2O Ocean Observation Network (N2O-ON).
Observation Network Components
Measurement Techniques
The analysis of N2O at the sea surface and in the ocean interior differs in both measurement approach and the required analytical precision. While water column N2O concentrations are usually determined using discrete seawater samples, state-of-the-art surface water measurements increasingly use air-water equilibration systems coupled to optical sensors in a continuous mode. In this section we briefly review the development of marine N2O observations, discuss a coordinated approach to method calibration, and identify emerging technologies that should contribute to improved data quality and spatio-temporal coverage within N2O-ON.
Discrete Measurements
The first study of oceanic N2O distributions took place nearly 60 years ago in the South Pacific Ocean (Craig and Gordon, 1963), and was followed by measurements in the North Atlantic Ocean during the late 1960s/early 1970s (Junge and Hahn, 1971). A later study in the Sargasso and Caribbean Seas introduced the concept of “Δ(N2O)” [= cmeasured(N2O) – cequilibrium(N2O)], to quantify the difference between the observed and air equilibrium concentration of dissolved N2O, and thus examine net N2O production/consumption (Yoshinari, 1976). The development of a rigorously calibrated electron capture detector (ECD) coupled with gas chromatography (GC) facilitated precise and reliable N2O measurements (Rasmussen et al., 1976; Cohen, 1977; Elkins, 1980; Weiss et al., 1981). Since those pioneering studies, the increasing availability and comparatively low cost of such instrumentation facilitated a significant increase in data availability.
An important next step was the fundamental work on N2O solubility in seawater (Weiss and Price, 1980), which promoted the development of equilibration techniques for high-resolution surveys of the surface ocean (Weiss et al., 1992) (see section “Continuous Surface Measurements”) and water column N2O (Butler et al., 1989; Butler and Elkins, 1991). Today, GC-ECD analysis, coupled to headspace equilibration or purge-and-trap techniques, is used by the majority of laboratories worldwide for quantifying dissolved N2O in discrete seawater samples (Wilson et al., 2018). Even so, mass spectrometric analysis of N2O is becoming increasingly wide-spread (Capelle et al., 2015; Babbin et al., 2017; Bourbonnais et al., 2017) and may become increasingly important in the future.
An inter-laboratory comparison of oceanic N2O measurements was recently conducted by the Scientific Committee on Oceanic Research (SCOR) international Working Group (WG) 1432. Discrete water samples from the subtropical Pacific Ocean and the Baltic Sea were distributed to participating laboratories (Wilson et al., 2018) for a comparison of accuracy and precision. The samples represented a range of N2O concentrations, from low concentrations in the oligotrophic open ocean to high concentrations in highly productive and suboxic coastal waters. Recommendations arising from the inter-comparison include (Wilson et al., 2018):
(i) calibration of working gas standards against primary standards,
(ii) incorporation of internal controls (i.e., air-equilibrated seawater) alongside routine sample analysis, and
(iii) the production of high and low N2O concentration reference seawater for calibrating N2O measurements across the full range of seawater N2O concentrations.
Primary gas standard mixtures obtained from atmospheric monitoring agencies will ensure consistency between ocean observations and global atmospheric monitoring networks such as NOAA’s Earth System Research Laboratory/Global Monitoring Division (ESRL/GMD3), NASA’s Advanced Global Atmospheric Gasses Experiment (AGAGE4) and the European Integrated Carbon Observing System (ICOS5). With accompanying guidelines for discrete measurements in preparation, these recommendations should lead to significant advances in precision and accuracy, thereby improving the inter-comparability of dissolved N2O measurements and facilitating the detection of seasonal and inter-annual N2O variability in the near future. Detecting inter-annual N2O signals is a major goal of N2O-ON, and will require a precision of better than 0.02 nmol L-1 (<0.2%). This value is derived from the expected change in N2O solubility due to an annual surface ocean warming of 0.01°C, and an annual increase of 1 nmol mol-1 (ppb) in the atmospheric N2O dry mole fraction, setting the salinity to 35 assuming no changes in oceanic N2O sources and sinks.
Continuous Surface Measurements
In addition to the discrete analysis of N2O, measurements are also conducted by continuous sampling from the shipboard underway seawater supply. Such measurements are made at a fixed depth (generally between 2 and 10 m below the sea surface) and are often accompanied by atmospheric measurements. These underway measurements have benefited from recent technological advances in cavity-enhanced absorption spectroscopy (CEAS), which facilitate rapid and precise N2O detection at very low atmospheric mole fractions (i.e., in the sub-ppb range). CEAS analyzers coupled to continuous seawater/gas equilibrators (Arévalo-Martínez et al., 2013; Grefe and Kaiser, 2014; Erler et al., 2015; Zhan et al., 2018) are now frequently used to determine N2O temporal and spatial variability in surface layers of open and coastal oceans, see e.g., (Arévalo-Martínez et al., 2015; Brase et al., 2017; Grefe et al., 2018; Wells et al., 2018). In addition to CEAS, Fourier Transform Infrared (FTIR) analysis coupled to continuous seawater/gas equilibration (Müller D. et al., 2016) has been developed. A ship-board comparison of five analytical systems (incl. four CEAS systems and one FTIR system) for continuous dissolved N2O measurements was conducted in the Baltic Sea as part of the activities of SCOR WG 143, demonstrating good agreement between measurements obtained from the different systems. Only recently, a Pumped Profiling System (PPS), connected with a liquid degassing membrane coupled with CEAS has allowed real-time, high-resolution, vertically resolved measurements of sub-surface N2O (Troncoso et al., 2018). N2O-ON will encourage the wider use of these and emerging new technologies where they can contribute to improvements to data quality, measurement frequency and spatial resolution.
Measurements in the Marine Boundary Layer
Accurate estimates of N2O flux densities across the ocean/atmosphere interface require measurements of the N2O mole fraction in the atmospheric boundary layer above the ocean, as well as ocean surface N2O concentrations. Atmospheric dry mole fraction can be converted into seawater saturation concentration as a function of seawater temperature, salinity and ambient pressure using an established solubility equation (Weiss and Price, 1980). Atmospheric N2O dry mole fractions are often measured in parallel with continuous underway measurements on research vessels and on vessels of opportunity (VOS: also often referred to as “Voluntary Observing Ship” routes) (Arévalo-Martínez et al., 2013). As for seawater measurements, N2O-ON advocates the routine rigorous calibration and quality control of accompanying atmospheric data.
The relatively inert nature of the N2O molecule results in a long tropospheric residence time, leading to well-mixed and regionally invariant global mole fractions (Prather et al., 2015). Consequently, where high quality ship-based atmospheric measurements are unavailable, N2O-ON will encourage use of high quality data from land-based global atmospheric monitoring networks; for example tropospheric N2O dry mole fractions from ESRL/GMD (see text footnote 3) or AGAGE (see text footnote 4). This will enable the extrapolation of individual campaign results to regional or global scales. Satellite-based N2O measurements show promise to augment atmospheric data collection at land-based monitoring stations, but these remote sensing observations currently have intrinsically large measurement errors, making them unsuitable for quantifying air-sea N2O exchange (Xiong et al., 2014; Bernath et al., 2017). With further improvements, however, such approaches have the potential to inform N2O-ON in the future.
Future Enhancements
New CEAS-based instruments allow high quality N2O isotopolog measurements (Harris et al., 2013). N2O-ON will identify an observational framework that will facilitate deployment of these instruments on selected sustained observation lines to provide additional constraints on the global atmospheric N2O budget (Rahn and Wahlen, 2000; Bernard et al., 2006; Park et al., 2012), and to potentially provide greater insight into the mechanisms of oceanic N2O production and consumption (Sutka et al., 2006; Yamagishi et al., 2007).
Although the development of CEAS can considerably improve N2O monitoring capabilities (see above), the estimation of sea/air N2O flux densities remains challenging because of the intrinsic temporal and spatial variability in surface ocean N2O concentrations, and the variability of existing gas exchange parameterizations (Garbe et al., 2014), which reflect the complexity in environmental controls of air-sea gas exchange. Unraveling this complexity, and thereby refining gas exchange parameterizations, is the focus of considerable ongoing research beyond the scope of N2O-ON. However, techniques such as the eddy covariance (EC) method that directly evaluate air-sea fluxes circumvent the need for such parameterizations (Businger, 1986). Going forward, the use of direct flux techniques such as EC in combination with N2O analysis by CEAS will be encouraged by N2O-ON as a means of enhancing our understanding of N2O fluxes across the sea surface on a range of temporal and spatial scales.
Observation Platforms
N2O-ON will exploit established and new observation platforms to improve the characterization of spatial and temporal variability in oceanic N2O concentrations.
Research Vessels
To date, the majority of surface and water column N2O data have been obtained on board research vessels from discrete samples collected in Niskin bottles on a CTD Rosette (see section “Discrete Measurements”), or from underway surface measurements via a continuous seawater supply (see section “Continuous Surface Measurements”). While the significant contribution of research vessels is beyond question and will be supported by N2O-ON, such vessels have a limited spatial and temporal footprint, with most sampling campaigns not repeated regularly and mainly occurring during the summer. N2O-ON will address this limitation by promoting the use of additional measurement platforms and sustained observational campaigns.
Repeat Hydrographic Lines and Time-Series Stations
Repeat hydrographic sampling programs are important in evaluating variability at the ocean-basin scale and for establishing variability on timescales from seasonal to decadal. For example, N2O has been measured biannually since 2012 in repeat hydrographic/geochemistry surveys on GO-SHIP6 section A25 between Portugal and Greenland (de la Paz et al., 2017). The Atlantic Meridional Transect7 is an example of an annually repeated cruise on which N2O measurements have been made over two decades (Forster et al., 2009; Rhee et al., 2009; Grefe and Kaiser, 2014). N2O has been repeatedly measured during the annual Chinese Arctic and Antarctic Expeditions (CHINARE) to the Arctic and Southern Oceans, see e.g., (Zhan and Chen, 2009; Zhan et al., 2015, 2017). Beside these examples, there are few published time-series measurements of open ocean water column N2O distributions from repeat hydrographic sections (Nevison et al., 1995; Fenwick and Tortell, 2018). Extending and optimizing the distribution and sampling frequency of repeat hydrographic lines is an important future aspiration for N2O-ON, both for open-ocean and coastal regimes.
Temporal variability is also investigated through regular data collection at a small number of fixed time-series stations, which are usually located close to land. Examples include stations off Goa (India), in Saanich Inlet (Vancouver Island, British Columbia), off central Chile, off Hawai’i in the North Pacific subtropical gyre, in the Eckernförde Bay (southwestern Baltic Sea) and in the Strait of Gibraltar (Naqvi et al., 2010; de la Paz et al., 2015; Farías et al., 2015; Capelle and Tortell, 2016; Wilson et al., 2017; Capelle et al., 2018). Considering the important role of coastal regions in the global N2O cycle (Bange, 2006; Anderson et al., 2010; Ciais et al., 2013), extending the spatial coverage of fixed time-series stations within a coordinated network is a major aspiration of N2O-ON.
VOS Lines
Autonomous measurement systems on established, regular international VOS routes are restricted to near-surface measurements, and thus do not provide depth-resolved N2O data. Nevertheless, they do have the potential to deliver a comprehensive picture of the temporal and (limited) spatial variability in surface water N2O distributions. A pilot VOS line N2O study in the North Atlantic Ocean between Liverpool, United Kingdom, and Halifax, Canada, was conducted in January 2017 by GEOMAR for the EU InGOS program8. The EU BONUS INTEGRAL program9 will establish N2O surface measurements on two VOS lines in the Baltic Sea between Lübeck/Travemünde (Germany) and Helsinki (Finland) as well as to Kemi (Finland) at the northern tip of the Baltic Sea. The successful long-term operation of CEAS-based measurements of dissolved non-CO2 greenhouse gasses has already been demonstrated for methane in the Baltic Sea (Gülzow et al., 2011, 2013). Nevertheless, autonomously monitoring of N2O on VOS lines requires a clean and maintained seawater supply, the oversight of analytical and emergency systems, and rapid instrument turnaround and cleaning during port calls. Although this is logistically challenging, particularly in remote ocean regions, the increased spatio-temporal coverage offered by measurement of near-surface N2O on VOS routes should be encouraged as a component of N2O-ON.
Other Sampling Platforms
To date, there are no autonomous underwater sensors available for long-term in-situ N2O monitoring in either the open or coastal ocean. Addressing this gap will require small, robust (resistant to high-pressure, hydrogen sulfide and biofouling) rapid response sensors with low power requirements for long-term deployment. Once developed, these sensors have the capability to decipher oceanic N2O distributions with unprecedented spatio-temporal resolution. Potential sensor platforms include Bio-Argo floats10, gliders, coastal/deep sea moorings and mooring arrays, cabled observatories, drifting buoys and lander systems. We advocate a strong focus on the future development of such sensors and their subsequent integration into N2O-ON.
Data Management
MEMENTO
MEMENTO (The MarinE MethanE and NiTrous Oxide database11), launched in 2009 (Bange et al., 2009), archives quality-controlled N2O data from the open and coastal oceans (including estuaries, fjords etc.) (Kock and Bange, 2015). MEMENTO also publishes N2O data sets, making them publicly and freely available. Regular updates include new datasets, additional meta-information, and the implementation of improved data quality control. As MEMENTO expands, it will adopt best practices for quality control according to the recommendations resulting from inter-comparison exercises (Wilson et al., 2018) and in accordance to existing databases such as the Surface Ocean CO2 Atlas (SOCAT12) and the Global Ocean Data Analysis Project for Carbon (GLODAP13). N2O-ON and MEMENTO are clearly complementary and the routine archiving of quality-controlled data in MEMENTO is an intrinsic requirement of N2O-ON.
Ancillary Data
To evaluate the N2O data derived from N2O-ON, additional standard hydrographic data (i.e., water temperature, salinity, depth) are important. In addition, chemical (i.e., dissolved O2 and nutrient concentrations, and pH) and meteorological (i.e., air temperature, pressure, wind speed) data should ideally be collected. Most, if not all, of these variables are measured on a routine basis during research cruises, at some time-series stations and on some repeat hydrographic lines (see section “Observation Platforms”). VOS lines could be equipped with continuously operating systems such as the FerryBox14. N2O-ON will formally identify a suite of mandatory ancillary measurements and recommend appropriate measurement and/or sample collection alongside N2O where possible.
Baseline Measurements
Resource constraints (both financial and personnel) preclude the extensive monitoring of N2O concentrations across the entire global ocean. For this reason, a primary goal of N2O-ON is to develop a highly strategic sampling approach. In Figure 1, N2O seasonal distributions derived from MEMENTO clearly show severe under-sampling of many ocean regions during various seasons, and it is precisely these regions that should be the target of near-term sampling efforts within N2O-ON. The following regions were specifically identified:
- the North Atlantic during December – February,
- the South Atlantic Ocean during March – August,
- the North Pacific Ocean during September – February,
- the South Pacific Ocean during all seasons,
- the North and South Indian Ocean during all seasons,
- the Southern and Arctic Oceans during all seasons, and
- selected marginal seas and major estuaries.
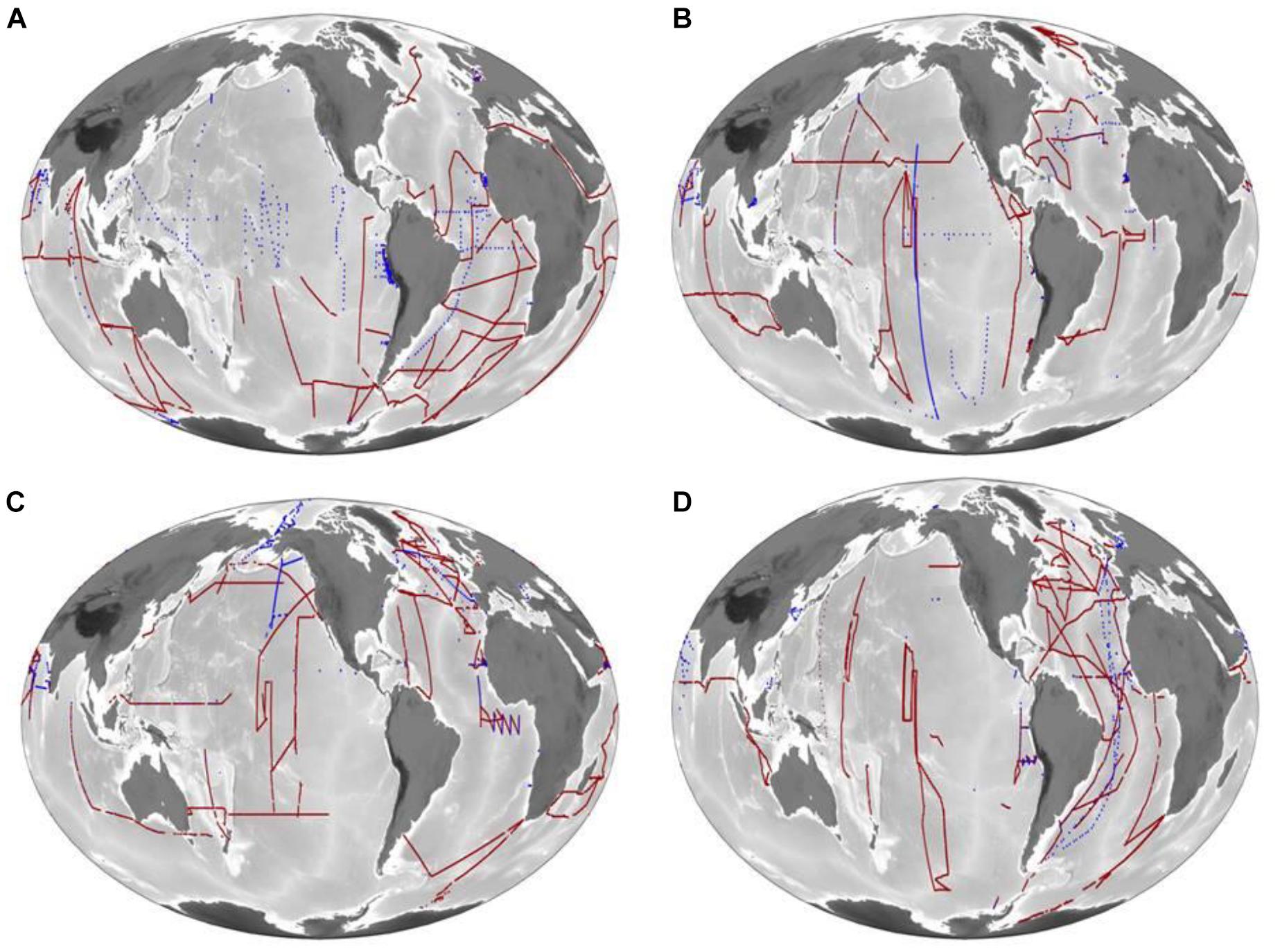
Figure 1. Maps of the distribution of N2O measurements in (A) December, January, February; (B) March, April, May; (C) June, July, August and (D) September, October, November. Red lines indicate continuous surface measurements. Blue dots indicate locations of N2O depth profiles. Data are from MEMENTO as of October 04, 2018 (https://memento.geomar.de/de).
N2O-ON will coordinate N2O baseline measurements on VOS, establishing these along major international shipping routes crossing the gyres of the major basins of the Atlantic, Pacific and Indian Oceans (Figure 2). We propose the establishment of repeat hydrographic lines using research vessels and/or VOS lines to measure N2O in the surface waters and water column of the Eastern Boundary Upwelling Systems (EBUS) and the Arabian Sea. This could exploit VOS lines transiting international shipping routes along the west coasts of North and South America, northwest and southwest Africa, and in the Arabian Sea. Moreover, routine N2O measurements should be incorporated into the FRAM Ocean Observing System (Soltwedel et al., 2013) in order to close some of the large data gaps in the Arctic Ocean (Figure 2).
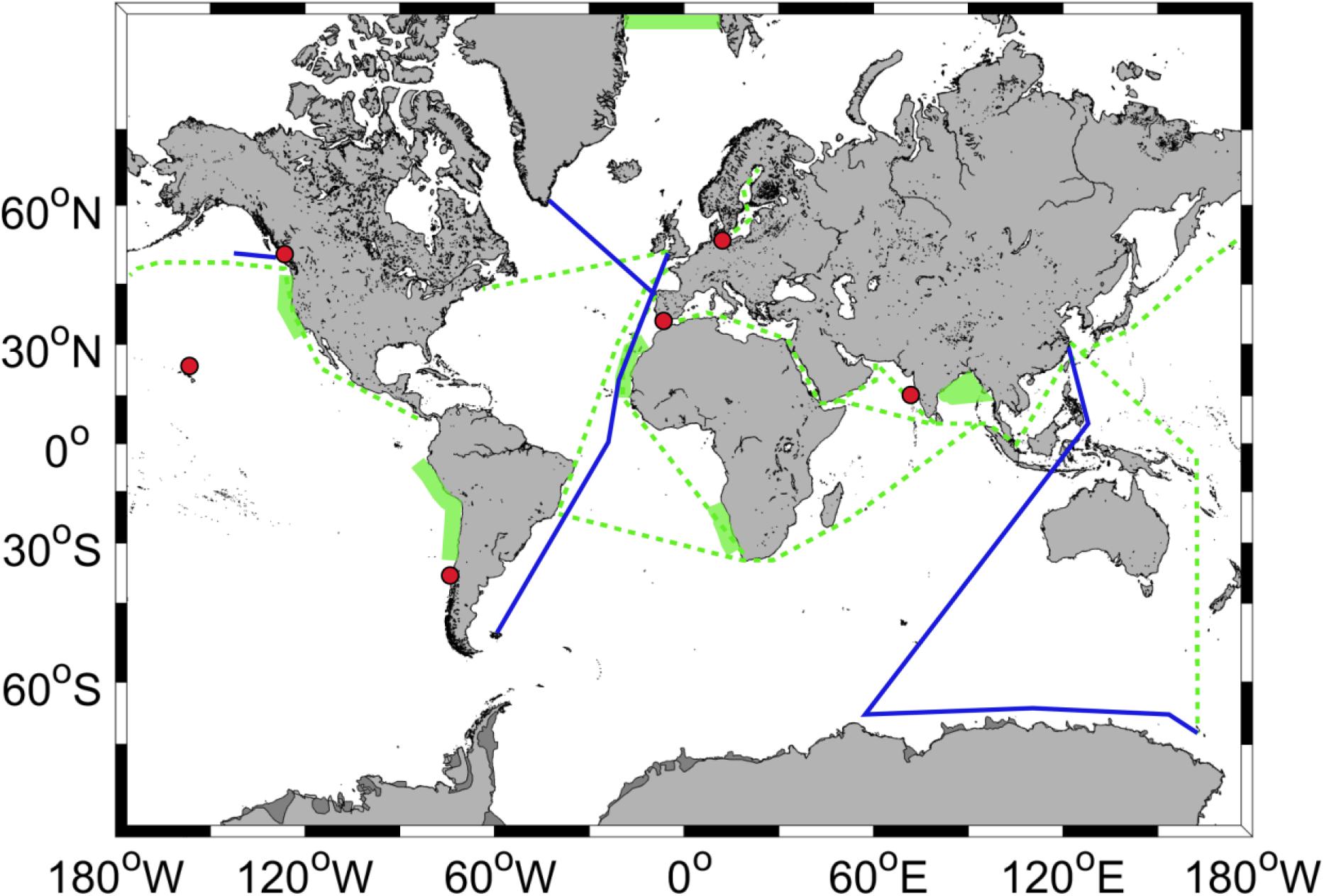
Figure 2. N2O baseline measurements proposed for N2O-ON. Blue lines/red points indicate currently active repeated oceanographic sections/fixed time-series stations (see text). Dashed green lines indicate prospective VOS lines to be equipped with systems for continuous measurements. Green shaded areas mark key regions for the establishment of new time-series stations.
Incorporating N2O into the suite of measurements of some established repeat hydrographic sections, such as GO-SHIP15 or GEOTRACES16, could provide a basin-scale approach to resolve N2O variability in the ocean interior, thus forming an important N2O-ON collaborative activity. N2O-ON will also encourage the regular monitoring of N2O in shelf areas and estuaries, which are prone to changes in redox-sensitive biogeochemistry due to enhanced anthropogenic and climatic impacts. Such activities would ideally be managed by local oceanographic institutes and/or relevant universities.
Established N2O time-series (see Section “VOS Lines”) at fixed station sites need to be continued. N2O-ON will identify additional sites to be established in the EBUS off Oregon/California, Peru, Mauritania and Namibia, in the northeast Indian Ocean (Bay of Bengal), and at some strategic coastal and enclosed basin sites to form a comprehensive and coordinated network. In addition to being important N2O sources to the atmosphere, these regions benefit from proximity to the necessary infrastructure provided by local/regional oceanographic institutes.
Summary and Outline of N2O-ON
Surface N2O concentration data can now be obtained with unprecedented precision. The inherent error in the CEAS technique is small relative to error in associated measurements (e.g., temperature correction to the seawater supply, non-steady state in the equilibration chamber, etc.). Even so, a harmonized data set requires a mechanism for inter-calibration, mutual agreement on metadata information and standard post-processing operations, as has been established for the global ocean surface CO2 network SOCAT (Pfeil et al., 2013). Enhancing the accuracy and consistency of discrete dissolved N2O concentration measurements requires the availability of liquid standards derived from strict preparation protocols, for example by the equilibration of seawater with air at known temperatures and salinity (Capelle et al., 2015; Wilson et al., 2018), or through the distribution of certified reference materials covering the range of concentrations expected in the oceanic environment (Wilson et al., 2018). The availability of a suitable reference material has been crucial in quantifying the oceanic carbon system (Dickson et al., 2007) with the required precision and accuracy to detect and evaluate long-term trends [e.g., (Müller J. D. et al., 2016)].
To improve and harmonize N2O measurements in a changing ocean, we suggest establishing a Global N2O Ocean Observation Network (N2O-ON) as outlined in Figure 3. In addition to exploiting existing oceanographic infrastructure (research vessels, VOS/repeat hydrographic lines etc.), we propose to establish central calibration facilities (CCF) in selected laboratories around the world to secure the comparability of N2O measurements, and provide data sets with maximum accuracy. The CCF will: (1) enable the precise calibration of N2O gas standards; (2) produce certified seawater reference material; (3) provide and maintain standard operating procedures for both surface and water column measurements; and (4) supply sampling bottles and equipment for research campaigns. Moreover, the N2O-ON calibration facilities will conduct regular internal comparison exercises to ensure long-term and high-level calibration performance. MEMENTO will archive all N2O data and make them publicly available following stringent quality checks. MEMENTO will also publish the N2O data sets with digital object identifiers (doi’s) to ensure appropriate referencing and tracking. Final N2O-ON data products, such as global N2O concentration maps, emissions, budgets and trends, will be used in modeling studies for projections of future trends in oceanic N2O emissions and advising policy makers and global climate assessments (Ciais et al., 2013). We advocate the establishment of regular workshops and courses to support all of these activities and to train the additional next generation of researchers who will be required to help realize the goals of N2O-ON.
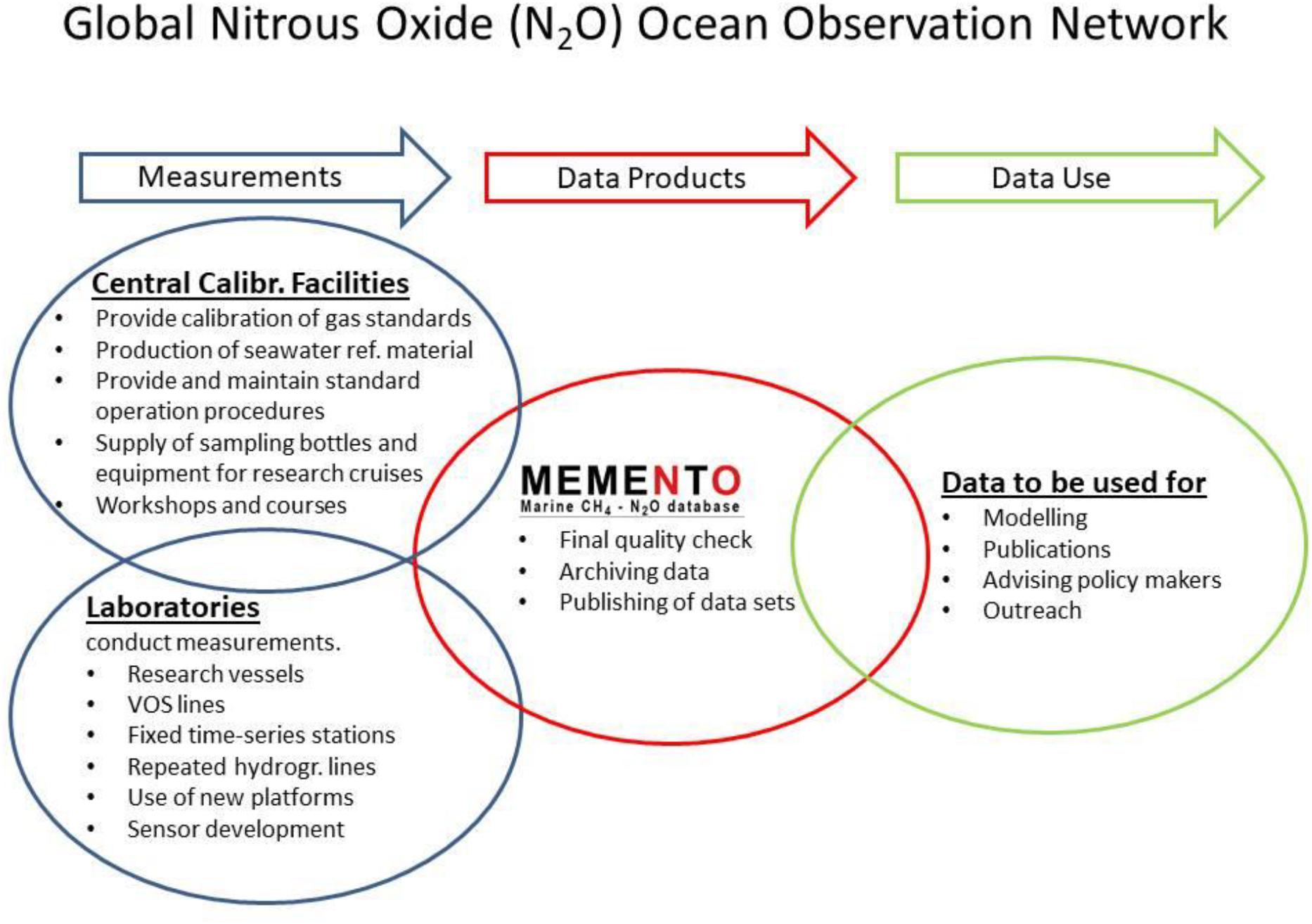
Figure 3. Proposed scheme for the Global N2O Ocean Observation Network. MEMENTO stands for “MarinE MethanE and NiTrous Oxide” database: https://memento.geomar.de/de.
Data Availability
No datasets were generated or analyzed for this study.
Author Contributions
All authors listed have made a substantial, direct and intellectual contribution to the work, and approved it for publication.
Funding
We thank SCOR for its generous support of WG 143 activities which were funded, in part, by a grant from the United States National Science Foundation (Grant OCE- 1840868) to SCOR. We also thank the EU FP7 project InGOS (Grant Agreement # 284274) for the support of the inter-laboratory comparison. LF was supported by the grants FONDAP 1511009 and FONDECYT N°1161138. MdlP received financial support from the INICIO project (CTM2015-74510-JIN). JK acknowledges support from grant NE/K002473/1 awarded by the United Kingdom Natural Environment Research Council (NERC). MEMENTO is currently supported by the Kiel Data Management Team at GEOMAR and the BONUS INTEGRAL Project which receives funding from BONUS (Art 185), funded jointly by the EU, the German Federal Ministry of Education and Research, the Swedish Research Council Formas, the Academy of Finland, the Polish National Centre for Research and Development, and the Estonian Research Council.
Conflict of Interest Statement
The authors declare that the research was conducted in the absence of any commercial or financial relationships that could be construed as a potential conflict of interest.
Acknowledgments
This article arose from meetings made possible by the SCOR WG 143 “Dissolved N2O and CH4 measurements: working toward a global network of ocean time series measurements of N2O and CH4.” We also thank Carolin Löscher for her comments on an early version of the manuscript and two reviewers for their helpful comments.
Footnotes
- ^www.goosocean.org
- ^https://scor-int.org/group/143/
- ^www.esrl.noaa.gov/gmd
- ^https://agage.mit.edu
- ^https://www.icos-ri.eu
- ^www.go-ship.org/
- ^www.amt-uk.org/
- ^www.ingos-infrastructure.eu
- ^www.io-warnemuende.de/integral-home.html
- ^http://biogeochemical-argo.org/
- ^https://memento.geomar.de
- ^www.socat.info
- ^www.glodap.info
- ^www.ferrybox.com
- ^www.goship.org
- ^www.geotraces.org/
References
Anderson, B., Bartlett, K., Frolking, S., Hayhoe, K., Jenkins, J., and Salas, W. (2010). Methane and Nitrous Oxide Emissions from Natural Sources. Washington, DC: United States Environmental Protection Agency.
Arévalo-Martínez, D. L., Beyer, M., Krumbholz, M., Piller, I., Kock, A., Steinhoff, T., et al. (2013). A new method for continuous measurements of oceanic and atmospheric N2O, CO and CO2: performance of off-axis integrated cavity output spectroscopy (OA-ICOS) coupled to non-dispersive infrared detection (NDIR). Ocean Sci. 9, 1071–1087. doi: 10.5194/os-9-1071-2013
Arévalo-Martínez, D. L., Kock, A., Löscher, C. R., Schmitz, R. A., and Bange, H. W. (2015). Evidence of massive nitrous oxide emissions from the tropical South Pacific Ocean. Nat. Geosci. 8, 530–533. doi: 10.1038/ngeo2469
Babbin, A. R., Peters, B. D., Mordy, C. W., Widner, B., Casciotti, K. L., and Ward, B. B. (2017). Multiple metabolisms constrain the anaerobic nitrite budget in the Eastern Tropical South Pacific. Glob. Biogeochem. Cycles 31, 258–271. doi: 10.1002/2016GB005407
Bakker, D. C. E., Pfeil, B., Landa, C. S., Metzl, N., and O’brien, K. M. (2016). A multi-decade record of high-quality fCO2 data in version 3 of the Surface Ocean CO2 Atlas (SOCAT). Earth Syst. Sci. Data 8, 383–413. doi: 10.5194/essd-8-383-2016
Bange, H. W. (2006). Nitrous oxide and methane in European coastal waters. Estuar. Coast. Shelf Sci. 70, 361–374. doi: 10.1016/j.ecss.2006.05.042
Bange, H. W., Bell, T. G., Cornejo, M., Freing, A., Uher, G., Upstill-Goddard, R. C., et al. (2009). MEMENTO: a proposal to develop a database of marine nitrous oxide and methane measurements. Environ. Chem. 6, 195–197. doi: 10.1071/EN09033
Bange, H. W., Freing, A., Kock, A., and Löscher, C. R. (2010). “Marine pathways to nitrous oxide,” in Nitrous Oxide and Climate Change, ed. K. Smith (London: Earthscan), 36–62.
Barnes, J., and Upstill-Goddard, R. C. (2011). N2O seasonal distribution and air-sea exchange in UK estuaries: implications for tropospheric N2O source from European coastal waters. J. Geophys. Res. 116:G01006. doi: 10.1029/2009JG001156
Battaglia, G., and Joos, F. (2018). Marine N2O emissions from nitrification and denitrification constrained by modern observations and projected in multimillenial global warming simulations. Glob. Biogeochem. Cycles 32, 92–121. doi: 10.1002/2017GB005671
Bernard, S., Röckmann, T., Kaiser, J., Barnola, J. M., Fischer, H., Blunier, T., et al. (2006). Constraints on N2O budget changes since pre-industrial time from new firn air and ice core isotope measurements. Atmos. Chem. Phys. 6, 493–503. doi: 10.5194/acp-6-493-2006
Bernath, P. F., Yousefi, M., Buzan, E., and Boone, C. D. (2017). A near-global atmospheric distribution of N2O isotopologues. Geophys. Res. Lett. 44, 10735–10743. doi: 10.1002/2017GL075122
Bourbonnais, A., Letscher, R. T., Bange, H. W., Échevin, V., Larkum, J., Mohn, J., et al. (2017). N2O production and consumption from stable isotopic and concentration data in the Peruvian coastal upwelling system. Glob. Biogeochem. Cycles 31, 678–698. doi: 10.1002/2016GB005567
Brase, L., Bange, H. W., Lendt, R., Sanders, T., and Dähnke, K. (2017). High resolution measurements of nitrous oxide (N2O) in the Elbe estuary. Front. Mar. Sci. 4:162. doi: 10.3389/fmars.2017.00162
Buitenhuis, E. T., Suntharalingam, P., and Le Quéré, C. (2018). Constraints on global oceanic emissions of N2O from observations and models. Biogeosciences 15, 2161–2175. doi: 10.5194/bg-15-2161-2018
Businger, J. A. (1986). Evaluation of the accuracy with which dry deposition can be measured with current micrometeorological techniques. J. Clim. Appl. Meteorol. 25, 1100–1124. doi: 10.1175/1520-0450(1986)025<1100:EOTAWW>2.0.CO;2
Butler, J. H., and Elkins, J. W. (1991). An automated technique for the measurements of dissolved N2O in natural waters. Mar. Chem. 34, 47–61. doi: 10.1016/0304-4203(91)90013-M
Butler, J. H., Elkins, J. W., Thompson, T. M., and Egan, K. B. (1989). Tropospheric and dissolved N2O of the west Pacific and east Indian Oceans during the El Nino Southern Oscillation Event of 1987. J. Geophys. Res. 94, 14865–14877. doi: 10.1029/JD094iD12p14865
Capelle, D. W., Dacey, J. W., and Tortell, P. D. (2015). An automated, high through-put method for accurate and precise measurements of dissolved nitrous-oxide and methane concentrations in natural waters. Limnol. Oceanogr. 13, 345–355. doi: 10.1002/lom3.10029
Capelle, D. W., Hawley, A. K., Hallam, S. J., and Tortell, P. D. (2018). A multi-year time-series of N2O dynamics in a seasonally anoxic fjord: saanich Inlet, British Columbia. Limnol. Oceanogr. 63, 524–539. doi: 10.1002/lno.10645
Capelle, D. W., and Tortell, P. D. (2016). Factors controlling methane and nitrous-oxide variability in the southern British Columbia coastal upwelling system. Mar. Chem. 179, 56–67. doi: 10.1016/j.marchem.2016.01.011
Ciais, P., Sabine, C., Bala, G., Bopp, L., Brovkin, V., Canadell, J., et al. (2013). “Carbon and other biogeochemical cycles,” in Climate Change 2013: The Physical Science Basis. Contribution of Working Gruop I to the Fifth Assessment Report of the Intergovernmental Panel on Climate Change, eds T. F. Stocker, D. Qin, G.-K. Plattner, M. Tignor, S. K. Allen, J. Boschung, et al. (Cambridge: Cambridge University Press), 465–570.
Cohen, Y. (1977). Shipboard measurement of dissolved nitrous oxide in seawater by electron capture gas chromatography. Anal. Chem. 49, 1238–1240. doi: 10.1021/ac50016a044
Cornejo, M., Murillo, A., and Farías, L. (2015). An unaccounted for N2O sink in the surface water of the eastern subtropical South Pacific ocean: physical versus biological mechanisms. Progr. Oceanogr. 137, 12–23. doi: 10.1016/j.pocean.2014.12.016
Craig, H., and Gordon, L. I. (1963). Nitrous oxide in the ocean and the marine atmosphere. Geochim. Cosmochim. Acta 27, 949–955. doi: 10.1016/0016-7037(63)90104-2
de la Paz, M., Garcia-Ibanez, M. I., Steinfeldt, R., Rios, A. F., and Perez, F. F. (2017). Ventilation versus biology: what is the controlling mechanism of nitrous oxide distribution in the North Atlantic? Glob. Biogeochem. Cycles 31, 745–760. doi: 10.1002/2016GB005507
de la Paz, M., Huertas, I. E., Flecha, S., Ríos, A. F., and Pérez, F. F. (2015). Nitrous oxide and methane in Atlantic and Mediterranean waters in the Strait of Gibraltar: air-sea fluxes and inter-basin exchange. Prog. Oceanogr. 138, 18–31. doi: 10.1016/j.pocean.2015.09.009
Dickson, A. G., Sabine, C. L., and Christian, J. R. (eds) (2007). Guide to Best Practices for Ocean CO2 Measurements. Sidney: PICES Special Publication.
Dore, J. E., and Karl, D. M. (1996). Nitrification in the euphotic zone as a source for nitrite, nitrate, and nitrous oxide at station ALOHA. Limnol. Oceanogr. 41, 1619–1628. doi: 10.4319/lo.1996.41.8.1619
Elkins, J. W. (1980). Determination of dissolved nitrous oxide in aquatic systems by gas chromatography using electron capture detection and multi phase equilibration. Anal. Chem. 52, 263–267. doi: 10.1021/ac50052a011
Erler, D. V., Duncan, T. M., Murray, R., Maher, D. T., Santos, I. R., Gatland, J. R., et al. (2015). Applying cavity ring-down spectroscopy for the measurement of dissolved nitrous oxide concentrations and bulk nitrogen isotopic composition in aquatic systems: correcting for interferences and field application. Limnol. Oceanogr. 13, 391–401. doi: 10.1002/lom3.10032
Farías, L., Besoain, V., and García-Loyola, S. (2015). Presence of nitrous oxide hotspots in the coastal upwelling area off central Chile: an anlysis of temproral variability based on ten years of a biogeochemiocal time series. Environ. Res. Lett. 10:044017. doi: 10.1088/1748-9326/10/4/044017
Farías, L., Faúndez, J., Fernandéz, C., Cornejo, M., Sanhueza, S., and Carrasco, C. (2013). Biological N2O fixation in the Eastern South Pacific Ocean and marine cynaobacterial cultures. PLoS One 8:e63956. doi: 10.1371/journal.pone.0063956
Fenwick, L., and Tortell, P. D. (2018). Methane and nitrous oxide distributions in coastal and open ocean waters of the Northeast Subarctic Pacific during 2015-2016. Mar. Chem. 200, 45–56. doi: 10.1016/j.marchem.2018.01.008
Forster, F. G., Upstill-Goddard, R. C., Gist, N., Robinson, C., Uher, G., and Woodward, E. M. S. (2009). Nitrous oxide and methane in the Atlantic Ocean between 50°N and 52°S: latitudinal distribution and sea-to-air flux. Deep Sea Res. Part 2 Top. Stud. Oceanogr. 56, 977–985. doi: 10.1016/j.dsr2.2008.12.002
Garbe, C. S., Rutgersson, A., Boutin, J., De Leeuw, G., Delille, B., and Fairall, C. W. (2014). “Transfer across the air-sea interface,” in Ocean-Atmosphere Interactions of Gases and Particles, eds P. S. Liss and M. T. Johnson (Heidelberg: Springer), 55–112.
Grefe, I., Fielding, S., Heywood, K. J., and Kaiser, J. (2018). Nitrous oxide variability at sub-kilometre resolution in the Atlantic sector of the Southern Ocean. PeerJ 6:e5100. doi: 10.7717/peerj.5100
Grefe, I., and Kaiser, J. (2014). Equilibrator-based measurements of dissolved nitrous oxide in the surface ocean using an integrated cavity output laser absorption spectrometer. Ocean Sci. 10, 501–512. doi: 10.5194/os-10-501-2014
Grundle, D. S., Löscher, C. R., Krahmann, G., Altabet, M. A., Bange, H. W., Karstensen, J., et al. (2017). Low oxygen eddies in the eastern tropical North Atlantic: implications for N2O cycling. Sci. Rep. 7:4806. doi: 10.1038/s41598-017-04745-y
Gülzow, W., Rehder, G., Schneider, B., and Von Deimling, J. S. (2011). A new method for continuous measurement of methane and carbon dioxide in surface waters using off-axis integrated cavity output spectroscopy (ICOS): an example from the Baltic Sea. Limnol. Oceanogr. Methods 9, 176–184. doi: 10.4319/lom.2011.9.176
Gülzow, W., Rehder, G., Von Deimling, J. S., Seifert, T., and Tóth, Z. (2013). One year of continuous measurements constraining methane emissions from the Baltic Sea to the atmosphere using a ship of opportunity. Biogeosciences 10, 81–99. doi: 10.5194/bg-10-81-2013
Harris, E., Nelson, D. D., Olszewski, W., Zahniser, M., Potter, K. E., Mcmanus, B. J., et al. (2013). Development of a spectroscopic technique for continuous online monitoring of oxygen and site-specific nitrogen isotopic composition of atmospheric nitrous oxide. Anal. Chem. 86, 1726–1734. doi: 10.1021/ac403606u
Hashimoto, L. K., Kaplan, W. A., Wofsy, S. C., and Mcelroy, M. B. (1983). Transformation of fixed nitrogen and N2O in the Cariaco Trench. Deep Sea Res. Part 2 Top. Stud. Oceanogr. 30, 575–590.
IPCC (2013). Climate Change 2013: The Physical Science Basis. Contribution of Working Group I to the Fifth Assessment Report of the Intergovernmental Panel on Climate Change. Cambridge: Cambridge University Press.
Junge, C., and Hahn, J. (1971). N2O measurements in the North Atlantic. J. Geophys. Res. 76, 8143–8146. doi: 10.1029/JC076i033p08143
Kawagucci, S., Makabe, A., Kodama, T., Matsui, Y., Yoshikawa, C., Ono, E., et al. (2018). Hadal water biogeochemistry over the Izu–Ogasawara Trench observed with a full-depth CTD-CMS. Ocean Sci. 14, 575–588. doi: 10.5194/os-14-575-2018
Kock, A., and Bange, H. W. (2015). Counting the ocean’s greenhouse gas emissions. EOS Earth Space Sci. News 96, 10–13. doi: 10.1029/2015EO023665
Kroeze, C., Dumont, E., and Seitzinger, S. P. (2005). New estimates of global emissions of N2O from rivers and estuaries. Environ. Sci. 2, 159–165. doi: 10.1080/15693430500384671
Landolfi, A., Somes, C. J., Koeve, W., Zamora, L. M., and Oschlies, A. (2017). Oceanic nitrogen cycling and N2O flux perturbations in the Anthropocene. Glob. Biogeochem. Cycles 31, 1236–1255. doi: 10.1002/2017GB005633
Law, C. S., and Ling, R. D. (2001). Nitrous oxide flux and response to increased iron availability in the Antarctic Circumpolar Current. Deep Sea Res. Part 2 Top. Stud. Oceanogr. 48, 2509–2527. doi: 10.1016/S0967-0645(01)00006-6
Martinez-Rey, J., Bopp, L., Gehlen, M., Tagliabue, A., and Gruber, N. (2015). Projections of oceanic N2O emissions in the 21st century using the IPSL Earth system model. Biogeosciences 12, 4133–4148. doi: 10.5194/bg-12-4133-2015
Morell, J. M., Capella, J., Mercado, A., Bauzá, J., and Corredor, J. E. (2001). Nitrous oxide fluxes in Caribbean and tropical Atlantic waters: evidence for near surface production. Mar. Chem. 74, 131–143. doi: 10.1016/S0304-4203(01)00011-1
Müller, D., Bange, H. W., Warneke, T., Rixen, T., Müller, M., Mujahid, A., et al. (2016). Nitrous oxide and methane in two tropical estuaries in a peat-dominated region of northwestern Borneo. Biogeosciences 13, 2415–2428. doi: 10.5194/bg-13-2415-2016
Müller, J. D., Schneider, B., and Rehder, G. (2016). Long-term alkalinity trends in the Baltic Sea and their implications for CO2-induced acidification. Limnol. Oceanogr. 61, 1984–2002. doi: 10.1002/lno.10349
Murray, R. H., Erler, D. V., and Eyre, B. D. (2015). Nitrous oxide fluxes in estuarine environments: response to global change. Glob. Chang. Biol. 21, 3219–3245. doi: 10.1111/gcb.12923
Myllykangas, J. P., Jilbert, T., Jakobs, G., Rehder, G., Werner, J., and Hietanen, S. (2017). Effects of the 2014 major Baltic inflow on methane and nitrous oxide dynamics in the water column of the central Baltic Sea. Earth Syst. Dyn. 8, 817–826. doi: 10.5194/esd-8-817-2017
Naik, H., Naqvi, S. W. A., Suresh, T., and Narvekar, P. V. (2008). Impact of a tropical cyclone on biogeochemistry of the central Arabian Sea. Glob. Biogeochem. Cycles 22:GB3020. doi: 10.1029/2007GB003028
Naqvi, S. W. A., Bange, H. W., Farías, L., Monteiro, P. M. S., Scranton, M. I., and Zhang, J. (2010). Marine hypoxia/anoxia as a source of CH4 and N2O. Biogeoscience 7, 2159–2190. doi: 10.5194/bg-7-2159-2010
Nevison, C. D., Weiss, R. F., and Erickson, D. J. III (1995). Global oceanic emissions of nitrous oxide. J. Geophys. Res. 100, 15809–15820. doi: 10.1029/95JC00684
Park, S., Croteau, P., Boering, K. A., Etheridge, D. M., Ferretti, D., Fraser, P. J., et al. (2012). Trends and seasonal cycles in the isotopic composition of nitrous oxide since 1940. Nat. Geosci. 5, 261–265. doi: 10.1038/ngeo1421
Pfeil, B., Olsen, A., Bakker, D. C. E., Hankin, S., Koyuk, H., Kozyr, A., et al. (2013). A uniform, quality controlled Surface Ocean CO2 Atlas (SOCAT). Earth Syst. Sci. Data 5, 125–143. doi: 10.5194/essd-5-125-2013
Prather, M. J., Hsu, J., Deluca, N. M., Jackman, C. H., Oman, L. D., and Douglass, A. R. (2015). Measuring and modeling the lifetime of nitrous oxide including its variability. J. Geophys. Res. Atmos. 120, 5693–5705. doi: 10.1002/2015JD023267
Rahn, T., and Wahlen, M. (2000). A reassessment of the global isotopic budget of atmospheric nitrous oxide. Glob. Biogeochem. Cycles 14, 537–543. doi: 10.1029/1999GB900070
Randall, K., Scarratt, M., Levasseur, M., Michaud, S., Xie, H. X., and Gosselin, M. (2012). First measurements of nitrous oxide in Arctic sea ice. J. Geophys. Res. Oceans 117:C00G15. doi: 10.1029/2011JC007340
Rasmussen, R. A., Krasnec, J., and Pierotti, D. (1976). N2O analysis in the atmosphere via electron capture-gas chromatography. Geophys. Res. Lett. 3, 615–618. doi: 10.1029/GL003i010p00615
Ravishankara, A. R., Daniel, J. S., and Portmann, R. W. (2009). Nitrous oxide (N2O): the dominant ozone-depleting substance emitted in the 21st century. Science 326, 123–125. doi: 10.1126/science.1176985
Rees, A. P., Brown, I. J., Jayakumar, A., and Ward, B. B. (2016). The inhibition of N2O production by ocean acidification in cold temperate and polar waters. Deep Sea Res. Part II Top. Stud. Oceanogr. 127, 93–101. doi: 10.1016/j.dsr2.2015.12.006
Rhee, T. S., Kettle, A. J., and Andreae, M. O. (2009). Methane and nitrous oxide emissions from the ocean: a reassessment using basin-wide observations in the Atlantic. J. Geophys. Res. 114:D12304. doi: 10.1029/2008JD011662
Rönner, U. (1983). Distribution, production and consumption of nitrous oxide in the Baltic Sea. Geochim. Cosmochim. Acta 47, 2179–2188. doi: 10.1016/0016-7037(83)90041-8
Soltwedel, T., Schauer, U., Boebel, O., Nöthig, E., Bracher, A., Metfies, K., et al. (2013). “FRAM – frontiers in Arctic marine monitoring visions for permanent observations in a gateway to the Arctic Ocean,” in Proceedings of the 2013 MTS/IEEE OCEANS, (Piscataway, NJ: IEEE), 1–7. doi: 10.1109/OCEANS-Bergen.2013.6608008
Suntharalingam, P., Buitenhuis, E., Le Quéré, C., Dentener, F., Nevison, C., Butler, J. H., et al. (2012). Quantifiying the impact of anthropogenic nitrogen deposition on oceanic nitrous oxide. Geophys. Res. Lett. 39:L07605. doi: 10.1029/2011GL050778
Sutka, R. L., Ostrom, N. E., Ostrom, P. H., Breznak, J. A., Gandhi, H., Pitt, A. J., et al. (2006). Distinguishing nitrous oxide production from nitrification and denitrification on the basis of isotopomer abundances. Appl. Environ. Microbiol. 72, 638–644. doi: 10.1128/AEM.72.1.638-644.2006
Troncoso, M., Garcia, G., Verdugo, J., and Farías, L. (2018). Toward high-resolution vertical measurements of dissolved greenhouse gases (nitrous oxide and methane) and nutrients in the eastern South Pacific. Front. Mar. Sci. 5:148. doi: 10.3389/fmars.2018.00148
Vancoppenolle, M., Meiners, K. M., Michel, C., Bopp, L., Brabant, F., Carnat, G., et al. (2013). Role of sea ice in global biogeochemical cycles: emerging views and challenges. Quat. Sci. Rev. 79, 207–230. doi: 10.1016/j.quascirev.2013.04.011
Weiss, R. F., Keeling, C. D., and Craig, H. (1981). The determination of tropospheric nitrous oxide. J. Geophys. Res. 86, 7197–7202. doi: 10.1029/JC086iC08p07197
Weiss, R. F., and Price, B. A. (1980). Nitrous oxide solubility in water and seawater. Mar. Chem. 8, 347–359. doi: 10.1016/0304-4203(80)90024-9
Weiss, R. F., Van Woy, F. A., and Salameh, P. K. (1992). Surface Water and Atmospheric Carbon Dioxide and Nitrous Oxide Observations by Shipboard Automated Gas Chromatography: Results From Expeditions Between 1977 and 1990. Oak Ridge: Oak Ridge National Laboratory.
Wells, N. S., Maher, D. T., Erler, D. V., Hipsey, M., Rosentreter, J. A., and Eyre, B. D. (2018). Estuaries as sources and sinks of N2O across a land use gradient in subtropical Australia. Glob. Biogeochem. Cycles 32, 877–894. doi: 10.1029/2017GB005826
Westley, M. B., Yamagishi, H., Popp, B. N., and Yoshida, N. (2006). Nitrous oxide cycling in the Black Sea inferred from stable isotope and isotopomer distributions. Deep Sea Res. Part 2 Top. Stud. Oceanogr. 53, 1802–1816. doi: 10.1016/j.dsr2.2006.03.012
Wilson, S. T., Bange, H. W., Arévalo-Martínez, D. L., Barnes, J., Borges, A. V., and Brown, I. (2018). An intercomparison of oceanic methane and nitrous oxide measurements. Biogeosciences 15, 5891–5907. doi: 10.5194/bg-15-5891-2018
Wilson, S. T., Ferron, S., and Karl, D. M. (2017). Interannual variability of methane and nitrous oxide in the North Pacific subtropical gyre. Geophys. Res. Lett. 44, 9885–9892. doi: 10.1002/2017GL074458
Xiong, X. Z., Maddy, E. S., Barnet, C., Gambacorta, A., Patra, P. K., Sun, F. Y., et al. (2014). Retrieval of nitrous oxide from atmospheric infrared sounder: characterization and validation. J. Geophys. Res. Atmos. 119, 9107–9122. doi: 10.1002/2013JD021406
Yamagishi, H., Westley, M. B., Popp, B. N., Toyoda, S., Yoshida, N., Watanabe, S., et al. (2007). Role of nitrification and denitrification on the nitrous oxide cycle in the eastern tropical North Pacific and Gulf of California. J. Geophys. Res. 112:G02015. doi: 10.1029/2006JG000227
Yoshinari, T. (1976). Nitrous oxide in the sea. Mar. Chem. 4, 189–202. doi: 10.1016/0304-4203(76)90007-4
Zhan, L., and Chen, L. (2009). Distributions of N2O and its air-sea fluxes in seawater along the cruise tracks between 30°S-67°S and in Prydz Bay, Anatarctica. J. Geophys. Res. 114:C03019. doi: 10.1029/2007JC004406
Zhan, L., Chen, L., Zhang, J., Yan, J., Li, Y., Wu, M., et al. (2015). Austral summer N2O sink and source characteristics and their impact factors in Prydz Bay. Antarctica. J. Geophys. Res. Oceans 120, 5836–5849. doi: 10.1002/2015JC010944
Zhan, L., Wu, M., Chen, L., Zhang, J., Li, Y., and Liu, J. (2017). The air-sea nitrous oxide flux along cruise tracks to the Arctic Ocean and Southern Ocean. Atmosphere 8:216. doi: 10.3390/atmos8110216
Zhan, L., Zhang, J., Li, Y., Wu, M., Liu, J., Lin, Q., et al. (2018). A fully automatic system for underway N2O measurements based on cavity ring-down spectroscopy. Int. J. Environ. Anal. Chem. 98, 709–724. doi: 10.1080/03067319.2018.1499902
Keywords: nitrous oxide, observation network, oceanic distribution, oceanic emissions, calibration
Citation: Bange HW, Arévalo-Martínez DL, de la Paz M, Farías L, Kaiser J, Kock A, Law CS, Rees AP, Rehder G, Tortell PD, Upstill-Goddard RC and Wilson ST (2019) A Harmonized Nitrous Oxide (N2O) Ocean Observation Network for the 21st Century. Front. Mar. Sci. 6:157. doi: 10.3389/fmars.2019.00157
Received: 24 October 2018; Accepted: 12 March 2019;
Published: 02 April 2019.
Edited by:
Minhan Dai, Xiamen University, ChinaReviewed by:
Xianhui Sean Wan, Xiamen University, ChinaMarlene Mark Jensen, Technical University of Denmark, Denmark
Copyright © 2019 Bange, Arévalo-Martínez, de la Paz, Farías, Kaiser, Kock, Law, Rees, Rehder, Tortell, Upstill-Goddard and Wilson. This is an open-access article distributed under the terms of the Creative Commons Attribution License (CC BY). The use, distribution or reproduction in other forums is permitted, provided the original author(s) and the copyright owner(s) are credited and that the original publication in this journal is cited, in accordance with accepted academic practice. No use, distribution or reproduction is permitted which does not comply with these terms.
*Correspondence: Hermann W. Bange, aGJhbmdlQGdlb21hci5kZQ== orcid.org/0000-0003-4053-1394
†orcid.org/0000-0003-2933-1586
‡orcid.org/0000-0002-9267-930X
§orcid.org/0000-0002-9479-1557
‖orcid.org/0000-0002-1553-4043
¶orcid.org/0000-0002-1017-6056
#orcid.org/0000-0002-7669-2475
Δorcid.org/0000-0003-3070-3447
♢orcid.org/0000-0002-0597-9989