- 1Jet Propulsion Laboratory, California Institute of Technology, Pasadena, CA, United States
- 2EOAS & COAPS, Florida State University, Tallahassee, FL, United States
- 3College of Earth, Ocean and Atmospheric Sciences, Oregon State University, Corvallis, OR, United States
- 4Upper Ocean Processes Group, Woods Hole Oceanographic Institution, Woods Hole, MA, United States
- 5Department of Electrical and Computer Engineering, Brigham Young University, Provo, UT, United States
The Winds and Currents Mission (WaCM) is a proposed approach to meet the need identified by the NRC Decadal Survey for the simultaneous measurements of ocean vector winds and currents. WaCM features a Ka-band pencil-beam Doppler scatterometer able to map ocean winds and currents globally. We review the principles behind the WaCM measurement and the requirements driving the mission. We then present an overview of the WaCM observatory and tie its capabilities to other OceanObs reviews and measurement approaches.
1. Introduction
Air-sea interaction is a critical component of the Earth's weather and climate systems and also plays an important role in ocean biology. Ocean surface winds couple the ocean and atmosphere, driving ocean circulation, and influencing fluxes across the air-sea interface. Ocean surface currents determine horizontal and vertical transport of heat, nutrients, and gases near the ocean surface, and also modulate the atmospheric wind forcing. Over the polar regions, both winds and currents play determining roles in the motion of sea ice and fresh water released by melting ice sheets. Since they form a tightly coupled dynamic system, surface winds and currents must be observed together at appropriate space and time scales. The joint measurement of these two essential climate variables (ECVs) has been recommended as a targeted observable for the next decade of NASA spaceborne observations by the 2018 Decadal Review (National Academies of Sciences, Engineering, and Medicine, 2018). Here, we present a conceptual measurement approach for a Winds and Currents Mission (WaCM) capable of meeting the observational goals outlined by the Decadal Review.
Radar altimeters have revolutionized monitoring large-scale geostrophic ocean currents (e.g., Stammer and Cazenave, 2017), but limited coverage by the altimetry constellation restricts the resolution to spatial scales ~ 200 km and temporal scales of about a month. The ocean contains significant variability at smaller scales and the NASA/CNES SWOT mission (Durand et al., 2010) will soon provide high spatial resolution measurements of small mesoscale features. SWOT will provide significant insights into small scale Sea Surface Height (SSH) variability, but its limited swath restricts its ability for forming temporal averages of spatial derivatives to compute geostrophic velocity and double derivatives to compute vorticity (Chelton et al., 2018) (see Figure 1).
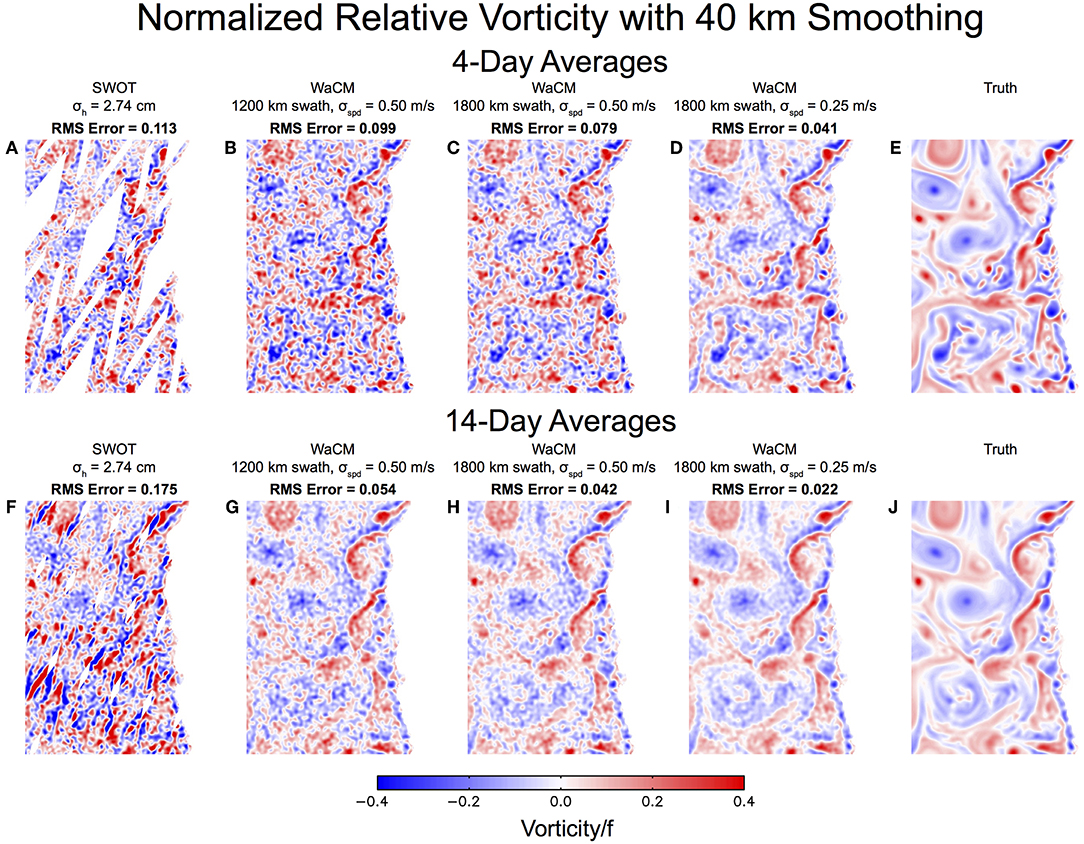
Figure 1. Simulated relative vorticity fields in the California Current System averaged for 4 (E) and 14 (J) days. On the left (A,F) are the same average fields sampled by the NASA/CNES SWOT mission, showing the problems with small swath coverage. The same fields sampled by WaCM assuming an 1,800 km swath and 50 cm/s (C,H) or 40 cm/s speed noise at 5 km sampling. The impact of swath width is shown in B,G, to be compared with C,H, where only a 1,200 km swath was used for sampling. D,I show the impact of reducing measurement noise from 50 cm/s to 25 cm/s. These results are taken from Chelton et al. (2018).
Even if geostrophic currents were determined precisely, surface currents contain additional contributions from Ekman (Lagerloef et al., 1999) and inertial currents (Alford et al., 2014) (both related to winds), tidal currents, and near surface currents driven by wind and wave induced instabilities (McWilliams, 2016). Although there have been efforts to compliment geostrophic currents by adding a wind driven Ekman component (Bonjean and Lagerloef, 2002), probing smaller scales requires coincident data and the inclusion of additional physics beyond a simple Ekman layer. Surface current divergence, an indicator of vertical circulation and mixing, may be resolvable by a total current WaCM sensor (Chelton et al., 2018), but cannot be computed from the geostrophic currents estimated from SWOT data. It is therefore necessary to develop sensors that are sensitive directly to the total surface current velocity, not just the geostrophic current.
Radar scatterometers, such as NASA's QuikSCAT or EUMETSAT's ASCAT, have demonstrated the capability to retrieve stress-equivalent winds (de Kloe et al., 2017) globally. Although the ASCAT constellation is operational, and complemented in by scatterometers launched by India (ScatSat-1) and China (HY2A, HY2B, CFOSAT), the sampling currently available (concentrated at ~9a.m./9p.m. or ~6a.m./6p.m., with systematic daily gaps in the tropics and mid-latitudes, ~25 km spatial resolution) is not sufficient to provide measurements of global winds/stress and wind/stress derivatives at appropriate space-time sampling, which, as we discuss below require both wide-swath coverage and high spatial resolution. To achieve these two requirements, the WaCM mission will collect both winds and currents from the same platform.
For WaCM, we propose to use Doppler scatterometry, described in section 2, to obtain simultaneous measurements of total ocean surface currents and winds. Meeting appropriate sampling and performance requirements, reviewed in section 3, is key for the viability of WaCM. How these requirements are met by a Doppler scatterometer system using current technology is reviewed in section 4. Finally, section 5 ties WaCM to the science goals and measurement concepts outlined by other contributions to 2019 OceanObs survey.
2. Doppler Scatterometry
Our science goals require an instrument that can provide simultaneous measurements of winds and ocean surface currents: we examine the state of retrieving these from space.
2.1. Measuring Winds
The estimation of ocean vector winds through the radar cross section measured by scatterometers, such as NASA's Ku-band QuikSCAT and ESA's C-band ASCAT, is a mature technology. Over the next decade both EUMETSAT and ISRO will likely continue to operate scatterometers, and China's scatterometer data products may be validated for science use and become publicly available. These sensors will complement the wind and current measurements of WaCM.
One of the key issues in air-sea interaction is the measurement of both vector winds and currents at ocean fronts (Chelton et al., 2004), which can be quite sharp as spatial scales decrease (McWilliams, 2016). Pencil-beam scatterometers can provide adequate wind directions at scales of about 25 km, although wind speeds at higher resolution can be estimated using super-resolution techniques (Plagge et al., 2009). Improved processing of ASCAT data (Vogelzang and Stoffelen, 2016), can also improve the spatial posting, although the Spatial Response Function (SRF) (Lindsley et al., 2016) limits spectral resolution; this may be improved in the future SCA EUMETSAT instrument (Lin et al., 2017). Although finer resolution can be achieved with traditional scatterometers, it comes at the cost of higher noise. Since the wind stress curl is essential to understanding wind-current interactions, we seek a system that can improve the spatial resolution of existing scatterometers at low-noise performance. This improvement can be accomplished by using Ka-band (~35 GHz) radars, which will reduce the ground azimuth footprint by a factor of ~3 relative to Ku-band, for a given antenna size. The azimuth resolution can be further improved by increasing the antenna size. Although there have been no spaceborne Ka-band scatterometer systems, the sensitivity of Ka-band to wind speed and direction has been established by radar measurements from towers (Yurovsky et al., 2016) and airplanes (Masuko et al., 1986; Rodriguez et al., 2018). All field measurements are consistent in showing Ka-band sensitivities to both wind speed and direction that are at least as good as are observed at Ku-band.
2.2. Measuring Surface Currents
The direct measurement of ocean surface velocities is achieved through measurements of the Doppler shift of the radar returns, which is proportional to the component of the ocean surface velocity along the line of sight. This technique was first demonstrated by airborne radars using along-track interferometry (ATI) (Goldstein et al., 1989). The surface current along the line of sight can be obtained, given the wind speed and direction, by removing the known phase speed of the resonant Bragg waves and contamination from brightness variations along surface gravity waves. Chapron et al. (2005) realized that some surface current information could be obtained by using the Doppler anomalies in a single-antenna radar system, and they have demonstrated retrievals over multiple ocean targets (Johannessen et al., 2008; Rouault et al., 2010). To go from radial velocity to vector velocity measurements, one needs to observe the radial velocity along different azimuth directions. Recently, several teams (Bao et al., 2015; Rodriguez et al., 2018) have proposed using a pencil-beam approach, such as the one on QuikSCAT, to obtain surface velocity estimates, and Rodriguez et al. (2018) have demonstrated the principle using airborne data.
The last decade has also seen the maturing of the theoretical basis for the geophysical algorithms required to separate the current from the Bragg wave and large-scale wave motions (e.g., Johannessen et al., 2008). Although helpful in guiding the understanding of the underlying physics, theory is not yet at the stage where it can be used to remove the contamination due to surface waves. For the moment, an approach based on a geophysical model function (GMF) that parametrizes the surface wave contamination as a function of wind speed and direction has been proposed and demonstrated using both airborne (Rodriguez et al., 2018) and tower data (Yurovsky et al., 2018). Although successful in removing much of the surface wave contamination, an empirical correlation approach can remove true surface current components, such as Stokes drift, that are directly correlated with the wind speed and direction and which may also be of geophysical interest.
Another issue with Doppler measurements is that they are sensitive to velocities at the actual ocean surface, and not to the more commonly used velocities at depths of order 10 m: current shear with depth must be accounted for when relating the two (Morey et al., 2018). Recently, Clarke and Van Gorder (2018) have examined empirically the contribution of Stokes drift and concluded that it is mainly driven by short waves generated by the local wind, so that the Stokes drift can be estimated from the wind stress measured by the scatterometer, highlighting again the need for simultaneous wind and current measurements. Both the GMF and current shear issue will need to be addressed in greater detail, both experimentally and theoretically, to mature the Doppler current concept to its full potential.
Note that the Doppler velocity concept also applies to tracking of sea ice, where greater radar brightness and no wave motion results in a more accurate measurement of velocity than over the ocean.
3. Resolution and Accuracy Requirements
The mission sampling requirements are not set merely by the accuracy and temporal resolution of the surface wind and current velocities. Since the curl of the wind stress and surface current are both important in air-sea interaction, one must consider the requirements for sampling velocity field derivatives, as well the fields themselves (Bourassa and McBeth-Ford, 2010). It is well-known that the derivative operator amplifies the errors of the measured variable.
3.1. Spatial Coverage
Global coverage, including the polar ocean, requires high inclination orbits. Most scatterometer missions are sun-synchronous (orbit inclination ~98°) which is sufficient to meet our goals, provided sun-synchronous signals, such as tides, can be removed reliably. While the elevation changes due to tides are well-known in the deep ocean (Stammer and Cazenave, 2017), their surface velocity expression has been less validated. Other high-inclination non-sun-synchronous orbits in the range between 82° and 98°, which may have better diurnal and tidal sampling, would also meet our observation requirements.
3.2. Space-Time Sampling
Temporal sampling of surface currents drives the mission design. In the tropics, temporal scales may be adequately sampled by observations separated by a few days. Elsewhere, small mesoscale features (30 to 100 km) not resolved by the altimeter constellation have lifetimes that range between 1 day and less than 1 week. To resolve synoptic surface wind variability and the sub-inertial ocean response or weak-wind or deep-mixed-layer conditions, one must consider time scales associated with the atmosphere-ocean coupling on the order of days to a week at scales of 100–200 km. For WaCM, simultaneous winds and surface currents will be at a frequency of 1–2 times per day (mitigating aliasing from tides and inertial motions), but temporal averages over several days are required to resolve relative vorticity features for the smallest scales.
Appropriate temporal sampling of coincident winds and currents is a major observational requirement (Wentz et al., 2017). Simultaneous observations are desired to study wind and current coupling, and the simultaneous measurements collected by WaCM will avoid temporal sampling. WaCM winds could complement, and be complemented by ongoing operational platforms, such as EUMETSAT's ASCAT, ISRO's SCATSAT-1, China's HY series, CFOSAT and WindRad.
An additional space-time coverage issue is the ability to gain synoptic views of the ocean circulation so that derivatives (such as vorticity) can be calculated and an assessment can be made of the temporal evolution of the two dimensional field (Chelton et al., 2018). Figure 1 compares simulated temporally averaged relative vorticity fields from the 120 km-swath NASA SWOT mission and those from the wide-swath WaCM scatterometer described below. Even though the instantaneous SWOT data have smaller random noise, the distortion in the time-averaged fields due to measurement gaps and the rapid evolution of small-scale features dominates the relative vorticity synoptic map errors. This is the case for short (4-day) averages and is even more of an issue when the temporal averages are conducted over 2 weeks.
Care must also be taken that wind-driven inertial motions not be aliased into the low-frequency signal. The period of inertial motions varies with latitude. In the tropics, the inertial period is long (e.g., 69 h at 10° latitude) and should not present a major sampling problem. However, the inertial period becomes shorter than one day at latitudes higher than 30° and appropriate sampling requires several observations per day. Current wide-swath radar scatterometers can achieve this sampling up to mid-latitudes, but it is possible that some of the inertial signals might alias at higher latitudes. At these latitudes, the use of models provides a means for removing the inertial motion contributions. To demonstrate the feasibility of this approach, we have examined the coherence of in situ inertial current measurements with an internal-wave admitting global ocean simulation (Rocha et al., 2016) driven by ECMWF atmospheric analysis and found there is significant coherence between simulated and observed inertial currents. This suggests that the effects of aliasing of near-inertial currents could be reduced by modeling and removing the inertial signal or by fitting for it, given sufficient duration and known oscillation periods. This is an area of active study.
3.3. Spatial Resolution
The spatial resolution of the measurements is driven by: avoiding contamination due to land and rain; the need to compute spatial derivatives (e.g., wind stress curl); the desire to resolve smaller features (wind and current) that may appear at higher latitudes or in coastal regions; and, consistency between wind and current estimates. High resolution is also required in the polar oceans or in the coastal regions to discriminate between land/ice and water. Based on previous scatterometer experience near land and rain, these requirements imply the need for spatial resolution of about 5 km, or a factor of ~5 improvement over the existing capabilities. Although the scatterometer signal for both Ku and Ka-band scatterometers is strongly attenuated by rain, we expect the significantly smaller resolution cell of WaCM will help in rejecting rain cells and cover the areas around them, improving on Ku-band scatterometer rain contamination. We also expect that the joint backscatter and Doppler signatures will allow for the simultaneous estimation of winds and rain, building on Draper and Long (2004).
3.4. Measurement Accuracy
The accuracy requirements are driven by the atmosphere-ocean coupling target. Using classical Ekman and bulk mixed-layer models to characterize the ageostrophic surface current component, accuracy requirements on stress can be derived. Experience with existing satellite scatterometer systems indicates that a precision of 0.02 N m−2 (equivalent to about 1.5 m/s wind speed) for surface stress is adequate to characterize the local wind field (Bourassa et al., 2019).
Computing the surface current vorticity and divergence places the most stringent requirements on the surface current accuracies. Chelton et al. (2018) have examined the resolutions that can be achieved for the velocity and vorticity fields in the California Current System (CCS) as a function of current component noise, temporal averaging, and swath width. They conclude that an 1,800 km swath and speed error of 50 cm/s for 5 km samples can resolve wavelength scales 45 km in the velocity and 70 km in the vorticity, assuming averaging over 4 days. Reducing the speed error to 25 cm/s further improves these resolution capabilities to about 20 and 45 km, respectively. As shown by Chelton et al. (2018; Appendix B), the feature diameter corresponds to about 1/2 of the resolved wavelengths, so one could resolve eddies whose diameter is 10 km (velocity) or 22.5 km (vorticity), which starts to probe ocean submesoscales. Figure 1 illustrates the resolution capabilities for different speed errors and swath widths, showing that the small mesoscale field will be appropriately sampled by a Doppler scatterometer system that can achieve an 1,800 km swath and speed accuracies between 25 and 50 cm/s sampled at 5 km.
4. Observing System
The measurement requirements lead to a sensor that has the following characteristics: ability to measure currents and winds simultaneously; large swath (~1, 800 km); high spatial resolution (< 5 km); continuous spatial coverage without significant gaps; current speed errors better than 50 cm/s. Rodriguez (2018) has proposed a design approach for WaCM that meets these requirements. Some highlights include:
• A pencil-beam scanning antenna architecture with a ~56° radar incidence angle. For orbits in the 700–800 km altitude range (i.e., OSCAT to QuikSCAT orbits), swaths between 1,700 and 1,900 km will be achieved, consistent with the spatial coverage and temporal sampling above and the recommendations in Chelton et al. (2018).
• A Ka-band, vertically polarized, Doppler scatterometer with a long (~5 m) skinny (~0.3 m) rotating antenna. The antenna length, which is substantially longer and narrower than the one in past scatterometers, has multiple benefits: (a) The azimuth resolution will be <3 km (8 times better than QuikSCAT), enabling the computation of current velocity derivatives with sufficient accuracy (Chelton et al., 2018) and leading to significant improvements in resolution that will be of importance at the ice edge and at the coasts. (b) Increases in signal-to-noise ratios, leading to improvements in random error performance that will meet or exceed the accuracy requirements in section 3 (see Figure 2). The narrow antenna dimension produces a large footprint in the range direction, so that continuous coverage is achieved at lower antenna rotation speeds than for circular antennas.
• A pulse repetition frequency (PRF) that varies with azimuth angle, which optimizes the pulse separation and energy per pulse, resulting in the surface velocity errors in Figure 2. The variable PRF significantly increases the imaged range ambiguity-free swath, and results in continuous coverage without need for interpolation. This in contrast with high-PRF systems (e.g., Ardhuin et al., 2018), where a limited range swath requires filling in voids using an interpolation scheme.
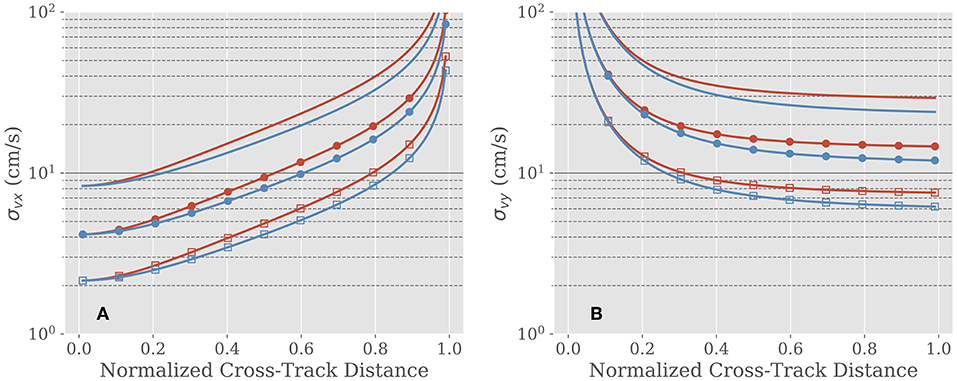
Figure 2. Velocity component error as a function of the normalized cross-track distance from nadir in the (A) along-track (σvx) direction (roughly North) and (B) across-track (σvy) direction (roughly East),for antenna lengths of 4 m (read), and 5 m (blue). The peak output power is 100 Watts (solid line), 400 Watts (circles), and 1.5 kWatts (squares). From Rodriguez (2018).
The WaCM errors quoted above assume the availability of off-the-shelf components with known performance for most of the instrument. Although not standard, the antenna assumed here is similar to a light, deployable reflectarray antenna developed by NASA's JPL for the SWOT mission (Hodges and Zawadzki, 2012), whose modification for WaCM is currently under study. One of the mission cost drivers is the radar RF source, since power drives the size and complexity of the spacecraft, so, in lieu of a detailed cost estimate at present, we show in Figure 2 the performance for several options spanning possible RF sources, and note that the threshold measurement objectives are met even for the lowest power solution, although additional power will enhance the science returns significantly.
5. Science Objectives and Relationship to Other OceanObs Reviews
WaCM would offer the first global data set of simultaneous measurements of ocean surface currents, winds, and sea ice sampled nearly twice per day with 5 km footprint. These capabilities are expected to make contributions in three broad areas:
• Ocean-Atmosphere Interactions: By measuring total surface currents, WaCM will provide a unique capability to monitor the non-geostrophic equatorial oceans, which play a key role in ocean heat uptake and carbon outgassing and are key in understanding the ocean's meridional heat transport (Villas Boas et al., 2019). At higher latitudes, WaCM would contribute to an improved understanding of wind- and current-driven ocean upwelling mechanisms (Gaube et al., 2015), wind work and the influence of ocean currents on the atmosphere (Chelton et al., 2004; Chelton and Xie, 2010; O'Neill et al., 2010; Frenger et al., 2013; Renault et al., 2016b, 2018).
• Ocean-Atmosphere-Biosphere Interactions: Wind-driven ocean upwelling and mesoscale/submesoscale features play an important role in the availability of nutrients in the mixed layer, and, therefore on ocean productivity and ecosystems (Gaube et al., 2014; Renault et al., 2016a). Interactions of orographic jets and ocean currents can also impact ocean productivity (Xie et al., 2005). Combining WaCM surface currents and winds with ocean color data will advance our understanding of these interactions.
• Ocean-Atmosphere-Cryosphere Interactions: Fresh water melting from ice sheets that occurs in the upper layer of the ocean and its pathway into lower latitudes will depend on synoptic winds. The dynamics of sea ice will reflect and influence the circulation of the polar oceans as sea-ice cover continues to evolve. By measuring surface currents, winds, and sea ice motion, WaCM will make a unique contribution to understanding the evolving cryosphere.
These applications are a subset of the many identified in other white papers in this OceanObs review (Villas Boas et al., 2019). WaCM shares some similarities with SKIM (Ardhuin, 2019) and SEASTAR, also in this OceanObs review. SKIM will have smaller random errors, but, due to a narrow swath and gaps, will have different resolution capabilities than WaCM (Ardhuin et al., 2018). Unlike WaCM, SKIM will not measure winds, but provides estimates of surface currents and surface wave spectra. SEASTAR will have high spatial resolution and accuracy, but its coverage will be limited to coastal regions. It is clear that the three mission concepts will be highly complimentary.
In addition to purely scientific uses, we expect the data provided by WaCM to be of use for many operational, civil, and commercial applications. As detailed in Bourassa et al. (2019), scatterometers are a vital input to global numerical weather forecasting. While the scatterometer constellation continues to grow, the community recommendation for sampling sufficient to characterize diurnal and semi-diurnal observations has not been achieved. The data provided by WaCM will help improve the sampling, especially if it is not in a sun-synchronous orbit. Marine debris (Maximenko et al., 2019) is another area of application that will benefit greatly by the availability of readily available surface currents and winds. Marine debris, and other marine pollution, such as oil spills, pose an environmental challenge that is worsening in a rapidly industrializing world. Debris is hard to detect using remote sensing, and it is expected that, since its dispersal is governed by surface winds and currents, availability of the variables on a regular basis will improve greatly the ability to forecast debris and surfactant trajectories and accumulation points. The monitoring of coastal winds and currents plays an important role in shipping and coastal safety (Chang et al., 2009), and in the assessment and management of coastal fisheries. The very high resolution winds provided by WaCM in the coastal region will fill a significant gap, since current systems are generally restricted to distances from shore that can be as large as 25 km. Finally, the ability to provide wide swath imagery together with radial velocity measurements will provide a significant benefit to monitoring of the rapidly changing sea ice cover and help in the tracking of icebergs, which present a danger to shipping and are also of interest for climate monitoring (Long, 2016).
Author Contributions
ER led the writing, and editing of the paper. All authors contributed equally to writing sections and revising the manuscript. All authors have approved the submitted version.
Funding
ER was funded under NASA grant NNN13D462T. DC was funded under NASA grant NNX10AO98G. JF was funded under NASA grants NNX14AM71G and NNX16AH76G. DL was funded under NASA grant NNX14AM67G. DP-M was funded under NASA grant NNH13ZDA001N. RS was funded under NASA grant NNX14AM66G.
Conflict of Interest Statement
The authors declare that the research was conducted in the absence of any commercial or financial relationships that could be construed as a potential conflict of interest.
Acknowledgments
The research was partially carried out at the Jet Propulsion Laboratory, California Institute of Technology, under a contract with the National Aeronautics and Space Administration (80NM0018D0004). Copyright 2018 California Institute of Technology. U.S. Government sponsorship acknowledged.
References
Alford, M. H., MacKinnon, J. A., Simmons, H., and Nash, J. (2014). Near-inertial internal gravity waves in the ocean. Annu. Rev. Mar. Sci. 8, 1–29. doi: 10.1146/annurev-marine-010814-015746
Ardhuin, F. (2019). SKIM, an ESA satellite mission for exploring global ocean currents and waves. Front. Mar. Sci. 6:209. doi: 10.3389/fmars.2019.00209
Ardhuin, F., Aksenov, Y., Benetazzo, A., Bertino, L., Brandt, P., Caubet, E., et al. (2018). Measuring currents, ice drift, and waves from space: the Sea surface KInematics Multiscale monitoring (SKIM) concept. Ocean Sci. 14, 337–354. doi: 10.5194/os-14-337-2018
Bao, Q., Dong, X., Zhu, D., Lang, S., and Xu, X. (2015). The feasibility of ocean surface current measurement using pencil-beam rotating scatterometer. IEEE J. Sel. Top. Appl. Earth Observ. Remote Sens. 8, 3441–3451. doi: 10.1109/JSTARS.2015.2414451
Bonjean, F., and Lagerloef, G. (2002). Diagnostic model and analysis of the surface currents in the tropical Pacific Ocean. J. Phys. Oceanogr. 32, 2938–2954. doi: 10.1175/1520-0485(2002)032<2938:DMAAOT>2.0.CO;2
Bourassa, M. A., and McBeth-Ford, K. (2010). Uncertainty in scatterometer-derived vorticity. J. Atmos. Oceanic Technol. 27, 594–603. doi: 10.1175/2009JTECHO689.1
Bourassa, M. A., Meissner, T., Cerovecki, I., Chang, P., De Chiara, G., Donlon, C., et al. (2019). Remotely sensed winds and wind stresses for marine forecasting and ocean modeling. Front. Mar. Sci. 6:443. doi: 10.3389/fmars.2019.00443
Chang, P. S., Jelenak, Z., Sienkiewicz, J. M., Knabb, R., Brennan, M. J., Long, D. G., et al. (2009). Operational use and impact of satellite remotely sensed ocean surface vector winds in the marine warning and forecasting environment. Oceanography 22, 194–207. doi: 10.5670/oceanog.2009.49
Chapron, B., Collard, F., and Ardhuin, F. (2005). Direct measurements of ocean surface velocity from space: interpretation and validation. J. Geophys. Res. Oceans 110. doi: 10.1029/2004JC002809
Chelton, D. B., Schlax, M. G., Freilich, M. H., and Milliff, R. F. (2004). Satellite measurements reveal persistent small-scale features in ocean winds. Science 303, 978–983. doi: 10.1126/science.1091901
Chelton, D. B., Schlax, M. G., Samelson, R. M., Farrar, J. T., Molemaker, M. J., McWilliams, J. C., et al. (2018). Prospects for future satellite estimation of small-scale variability of ocean surface velocity and vorticity. Prog. Oceanogr. 173, 256–350. doi: 10.1016/j.pocean.2018.10.012
Chelton, D. B., and Xie, S.-P. (2010). Coupled ocean-atmosphere interaction at oceanic mesoscales. Oceanography 23, 52–69. doi: 10.5670/oceanog.2010.05
Clarke, A., and Van Gorder, S. (2018). The relationship of near-surface flow, stokes drift and the wind stress. J. Geophys. Res. Oceans 123, 4680–4692. doi: 10.1029/2018JC014102
de Kloe, J., Stoffelen, A., and Verhoef, A. (2017). Improved use of scatterometer measurements by using stress-equivalent reference winds. IEEE J. Sel. Top. Appl. Earth Observ. Remote Sens. 10, 2340–2347. doi: 10.1109/JSTARS.2017.2685242
Draper, D. W., and Long, D. G. (2004). Evaluating the effect of rain on SeaWinds scatterometer measurements. J. Geophys. Res. Oceans 109. doi: 10.1029/2002JC001741
Durand, M., Fu, L.-L., Lettenmaier, D., Alsdorf, D., Rodriguez, E., and Esteban-Fernandez, D. (2010). The surface water and ocean topography mission: observing terrestrial surface water and oceanic submesoscale eddies. Proc. IEEE 98, 766–779. doi: 10.1109/JPROC.2010.2043031
Frenger, I., Gruber, N., Knutti, R., and Münnich, M. (2013). Imprint of southern ocean eddies on winds, clouds and rainfall. Nat. Geosci. 6:608. doi: 10.1038/ngeo1863
Gaube, P., Chelton, D. B., Samelson, R. M., Schlax, M. G., and O'Neill, L. W. (2015). Satellite observations of mesoscale eddy-induced ekman pumping. J. Phys. Oceanogr. 45, 104–132. doi: 10.1175/JPO-D-14-0032.1
Gaube, P., McGillicuddy, D. J., Chelton, D. B., Behrenfeld, M. J., and Strutton, P. G. (2014). Regional variations in the influence of mesoscale eddies on near-surface chlorophyll. J. Geophys. Res. Oceans 119, 8195–8220. doi: 10.1002/2014JC010111
Goldstein, R. M., Barnett, T. P., and Zebker, H. A. (1989). Remote sensing of ocean currents. Science 246, 1282–1285. doi: 10.1126/science.246.4935.1282
Hodges, R., and Zawadzki, M. (2012). “Ka-band reflectarray for interferometric SAR altimeter,” in Antennas and Propagation Society International Symposium (APSURSI), 2012 IEEE (Monterey, CA: IEEE), 1–2.
Johannessen, J., Chapron, B., Collard, F., Kudryavtsev, V., Mouche, A., Akimov, D., et al. (2008). Direct ocean surface velocity measurements from space: improved quantitative interpretation of Envisat ASAR observations. Geophys. Res. Lett. 35. doi: 10.1029/2008GL035709
Lagerloef, G., Mitchum, G., Lukas, R., and Niiler, P. (1999). Tropical Pacific near-surface currents estimated from altimeter, wind, and drifter data. J. Geophys. Res. Oceans 104, 23313–23326. doi: 10.1029/1999JC900197
Lin, C.-C., Lengert, W., and Attema, E. (2017). Three generations of c-band wind scatterometer systems from ers-1/2 to metop/ascat, and metop second generation. IEEE J. Sel. Top. Appl. Earth Observ. Remote Sens. 10, 2098–2122. doi: 10.1109/JSTARS.2016.2616166
Lindsley, R. D., Anderson, C., Figa-Saldaña, J., and Long, D. G. (2016). A parameterized ASCAT measurement spatial response function. IEEE Trans. Geosci. Remote Sens. 54, 4570–4579. doi: 10.1109/TGRS.2016.2544835
Long, D. G. (2016). Polar applications of spaceborne scatterometers. IEEE J. Sel. Top. Appl. Earth Observ. Remote Sens. 10, 2307–2320. doi: 10.1109/JSTARS.2016.2629418
Masuko, H., Okamoto, K., Shimada, M., and Niwa, S. (1986). Measurement of microwave backscattering signatures of the ocean surface using x band and ka band airborne scatterometers. J. Geophys. Res. Oceans 91, 13065–13083. doi: 10.1029/JC091iC11p13065
Maximenko, N., et al. (2019). Towards the integrated marine debris observing system. Front. Mar. Sci. 6:447. doi: 10.3389/fmars.2019.00447
McWilliams, J. (2016). Submesoscale currents in the ocean. Proc. R. Soc. A 472:20160117. doi: 10.1098/rspa.2016.0117
Morey, S., Wienders, N., Dukhovskoy, D., and Bourassa, M. (2018). Measurement characteristics of near-surface currents from ultra-thin drifters, drogued drifters, and HF radar. Remote Sens. 10:1633. doi: 10.3390/rs10101633
National Academies of Sciences, Engineering, and Medicine (2018). Thriving on Our Changing Planet: A Decadal Strategy for Earth Observation from Space. Washington, DC: The National Academies Press.
O'Neill, L. W., Chelton, D. B., and Esbensen, S. K. (2010). The effects of SST-induced surface wind speed and direction gradients on midlatitude surface vorticity and divergence. J. Clim. 23, 255–281. doi: 10.1175/2009JCLI2613.1
Plagge, A., Vandemark, D., and Long, D. (2009). Coastal validation of ultra-high resolution wind vector retrieval from QuikSCAT in the Gulf of Maine. IEEE Geosci. Remote Sens. Lett. 6, 413–417. doi: 10.1109/LGRS.2009.2014852
Renault, L., Deutsch, C., McWilliams, J. C., Frenzel, H., Liang, J.-H., and Colas, F. (2016a). Partial decoupling of primary productivity from upwelling in the California Current system. Nat. Geosci. 9, 505–508. doi: 10.1038/ngeo2722
Renault, L., McWilliams, J. C., and Gula, J. (2018). Dampening of submesoscale currents by air-sea stress coupling in the Californian Upwelling System. Sci. Rep. 8:13388. doi: 10.1038/s41598-018-31602-3
Renault, L., Molemaker, J., McWilliams, J., Shchepetkin, A., Lemarie, F., Chelton, D., et al. (2016b). Modulation of wind work by oceanic current interaction with the atmosphere. J. Phys. Oceanogr. 46, 1685–1704. doi: 10.1175/JPO-D-15-0232.1
Rocha, C. B., Chereskin, T. K., Gille, S. T., and Menemenlis, D. (2016). Mesoscale to submesoscale wavenumber spectra in Drake Passage. J. Phys. Oceanogr. 46, 601–620. doi: 10.1175/JPO-D-15-0087.1
Rodriguez, E. (2018). On the optimal design of Doppler scatterometers. Remote Sens. 10:1765. doi: 10.3390/rs10111765
Rodriguez, E., Wineteer, A., Perkovic-Martin, D., Gal, T., Stiles, B., Niamsuwan, N., et al. (2018). Estimating ocean vector winds and currents using a Ka-band pencil-beam doppler scatterometer. Remote Sens. 10:576. doi: 10.3390/rs10040576
Rouault, M. J., Mouche, A., Collard, F., Johannessen, J. A., and Chapron, B. (2010). Mapping the Agulhas Current from space: An assessment of ASAR surface current velocities. J. Geophys. Res. Oceans 115. doi: 10.1029/2009JC006050
Stammer, D., and Cazenave, A. (2017). Satellite Altimetry Over Oceans and Land Surfaces. Boca Raton, FL: CRC Press.
Villas Boas, A. B., Ardhuin, F., Ayet, A., Bourassa, M. A., Brandt, P., Chapron, B., et al. (2019). Integrated observations and modeling of winds, currents, and waves: requirements and challenges for the next decade. Front. Mar. Sci. 6:425. doi: 10.3389/fmars.2019.00425
Vogelzang, J., and Stoffelen, A. (2016). ASCAT Ultrahigh-resolution wind products on optimized grids. IEEE J. Sel. Top. Appl. Earth Observ. Remote Sens. 10, 2332–2339. doi: 10.1109/JSTARS.2016.2623861
Wentz, F., Ricciardulli, L., Rodriguez, E., Stiles, B., Bourassa, M., Long, D., et al. (2017). Evaluating and extending the ocean wind climate data record. IEEE J. Sel. Top. Appl. Earth Observ. Remote Sens. 10, 2165–2185. doi: 10.1109/JSTARS.2016.2643641
Xie, S.-P., Xu, H., Kessler, W., and Nonaka, M. (2005). Air–sea interaction over the Eastern Pacific Warm Pool: gap winds, thermocline dome, and atmospheric convection. J. Clim. 18, 5–20. doi: 10.1175/JCLI-3249.1
Yurovsky, Y., Kudryavtsev, V. N., Grodsky, S. A., and Chapron, B. (2016). Ka-band dual copolarized empirical model for the sea surface radar cross section. IEEE Geosci. Remote Sens. 55, 1–19. doi: 10.1109/TGRS.2016.2628640
Keywords: surface currents, surface winds, Doppler, scatterometer, air-sea interaction, sea ice, relative vorticity
Citation: Rodríguez E, Bourassa M, Chelton D, Farrar JT, Long D, Perkovic-Martin D and Samelson R (2019) The Winds and Currents Mission Concept. Front. Mar. Sci. 6:438. doi: 10.3389/fmars.2019.00438
Received: 15 November 2018; Accepted: 05 July 2019;
Published: 24 July 2019.
Edited by:
Laura Lorenzoni, University of South Florida, United StatesReviewed by:
Ad Stoffelen, Royal Netherlands Meteorological Institute, NetherlandsPatrice M. Klein, Institut Français de Recherche pour l'Exploitation de la Mer (IFREMER), France
Copyright © 2019 Rodríguez, Bourassa, Chelton, Farrar, Long, Perkovic-Martin and Samelson. This is an open-access article distributed under the terms of the Creative Commons Attribution License (CC BY). The use, distribution or reproduction in other forums is permitted, provided the original author(s) and the copyright owner(s) are credited and that the original publication in this journal is cited, in accordance with accepted academic practice. No use, distribution or reproduction is permitted which does not comply with these terms.
*Correspondence: Ernesto Rodríguez, ZXJuZXN0by5yb2RyaWd1ZXpAanBsLm5hc2EuZ292