- 1National Oceanic and Atmospheric Administration (NOAA) Pacific Marine Environmental Laboratory (PMEL), Seattle, WA, United States
- 2Saildrone, Alameda, CA, United States
- 3Applied Physics Laboratory, University of Washington, Seattle, WA, United States
- 4Joint Institute for the Study of the Atmosphere and Ocean, University of Washington, Seattle, WA, United States
Partnership between the private sector and the ocean-observing community brings exciting opportunities to address observing challenges through leveraging the unique strengths of each sector. Here, we discuss a case study of a successful relationship between the National Oceanic and Atmospheric Administration (NOAA) Pacific Marine Environmental Laboratory (PMEL) and Saildrone to instrument an unmanned surface vehicle (USV) in order to serve shared goals. This case study demonstrates that a private company working with a federal laboratory has provided innovative ocean-observing solutions deployed at regional scale in only a few years, and we project that this model will be sustainable over the long term. An alignment of long-term goals with practical deliverables during the development process and integrating group cultures were key to success. To date, this effort has expanded NOAA’s interdisciplinary observing capabilities, improved public access to ocean data, and paved the way for a growing range of USV applications in every ocean. By emphasizing shared needs, complementary strengths, and a clear vision for a sustainable future observing system, we believe that this case study can serve as a blueprint for public and private partners who wish to improve observational capacity. We recommend that the international scientific community continues to foster collaborations between the private sector and regional ocean-observing networks. This effort could include regional workshops that build community confidence through independent oversight of data quality. We also recommend that an international framework should be created to organize public and private partners in the atmospheric and oceanographic fields. This body would coordinate development of observational technologies that adhere to best practices and standards for sensor integration, verification, data quality control and delivery, and provide guidance for unmanned vehicle providers. Last, we also recommend building bridges between the private sector, the ocean-observing community, and the operational forecast community to consider the future of this new private sector, with goals to determine targeted ocean-observing needs; assess the appropriateness of USVs as science platforms, sensors, and data format standards; and establish usage and data quality control and distribution protocols for ocean observing and operational forecasting.
Introduction
In 2009, the previous OceanObs conference ended with a call to action to significantly enhance global ocean observing. With over 600 participants across 36 countries and more than 100 papers submitted, the community put forward many recommendations. The key, consensus outcome of the global ocean science community represented by OceanObs’09 was the development of the Framework for Ocean Observing (FOO) (Lindstrom et al., 2012). The vision was aspirational: sustained, high-resolution observing networks that openly share data in near real time, distributed and quality controlled according to best practices and international standards, that support society’s needs for understanding and forecasting marine variability on a variety of scales. In order to achieve this vision, it was clear that development of new technologies would be essential, although the pathway forward was largely unclear.
In the intervening decade, technical progress has been made that has rapidly scaled the global ocean-observing system, sometimes through important partnerships with the private sector. Many industries, from insurance to agriculture, feel ill-equipped to plan for rapid environmental changes. As a result, private capital has been increasingly motivated to support the global science community to enhance earth observations.
Given these shared goals, public science entities and private partners can work together to make significant, high-quality, and rapid advances to the observing system by harnessing the strengths of each partner. Through the public sector, the scientific community provides guidance and shares expertise to ensure that any new assets or protocols meet data quality and access standards and target the public mission goals. The private sector can quickly harness capital and build assets, shouldering a significant amount of development and management risk. Together, these partners can quickly build, deploy, and apply high-quality scientific tools that both serve the public good and meet private sector needs.
Here, we present a public–private partnership (PPP) case study and model that has delivered innovative solutions to technological gaps and addressed requirements necessary for integration into sustained observing systems. For the purposes of this discussion, we define a PPP as an agreement between the U.S. government and a private corporate entity, each bearing the strengths and responsibilities suggested above. In 2014, a PPP was established between the U.S. National Oceanic and Atmospheric Administration’s (NOAA) Pacific Marine Environmental Laboratory (PMEL) and Saildrone. This partnership resulted in the successful development of the Saildrone USV1, an unmanned surface vehicle (USV) for ocean research, and has provided 4 years of validated data for demonstration missions that have been conducted on regional scales.
Based on these early successes, it seems clear that USVs could substantially expand the global ocean-observing network. One vision is to leverage private industry investment in the development of a variety of USVs coordinated in regional fleets. These focused networks would be adaptable to specific observing requirements and fill targeted regional observing gaps. To fulfill that vision, we recommend that the international community continue to foster close relationships between the private sector, the regional ocean-observing community, and the operational forecast community to determine these observing needs; assess the appropriateness of existing USVs, sensors, sampling schemes, and data format standards for achieving these needs; and establish usage and data distribution protocols for data generated by USVs at these scales. Establishing community confidence, transparency, and independent oversight of data quality will be critical to building on these early successes and achieving a robust role for USVs in the global observing system.
Public Infrastructure
At NOAA-PMEL, large-scale global observatories have traditionally evolved to address specific scientific needs such as providing time series data and forecasts for high-impact phenomenon like El Niño Southern Oscillation (ENSO), tsunamis, and carbon flux. Research programs are developed around each specific science requirement and encompass the entire observational effort from engineering development, sensor and platform procurement, data quality control (QC), and assurance, to ingestion of data into research models and products. If the research program’s data and products prove to be of significantly high impact, then the program is eventually transferred from the public research laboratory to a public operational entity. An example is the decade-long investment of PMEL research in developing an effective tsunami monitoring system that now delivers global data into NOAA National Weather Service forecast systems in real time to save lives and property (Bernard and Meinig, 2011).
This well-proven public observational data model has several benefits. Observational data generated by scientists through a rigorous scientific process are of high quality, and the model closely couples data users with data gatherers during the research development phase. Unfortunately, this public observational model also has several key weaknesses that negatively affect the scalability of the public observational arrays. When the research array transitions into operations, there is a considerable loss in flexibility and adaptability, and data users and data gatherers become decoupled. Implementing and maintaining the array come at considerable cost due to the need for skilled instrument technicians, seagoing personnel, and staff to manage the logistical and data pipelines. While this model generates isolated areas of specific expertise, such arrays are not easily ported, adopted, or adapted by other organizations that may have different cultures and funding priorities. Finally, arrays built in isolation from the private sector may be slow to develop and implement and, therefore, not well suited to the urgency of a rapidly changing world.
Private Capacity
The PPP with Saildrone offered the ability to leverage private capital and invest in complex, multiyear efforts. Saildrone possessed the capacity to scale up rapidly, and this streamlined purchasing and hiring. In return, NOAA received a large quantity of high-quality standardized observations of essential climate and ocean variables (ECVs and EOVs). From Saildrone’s perspective, NOAA provided expertise on the collection of observational data that were based on prior community development, including sensors, standards, and data quality management. Through the PPP, NOAA also serves as a potential customer for observational data.
The vision set forth in this paper is for distributed regional fleets of USVs that fill spatiotemporal data gaps in observing arrays, are adaptive to specific observing requirements, and serve as an end-to-end tool for the larger oceanographic community. Under the model presented here, a primary concern is the use of a private entity as a data provider. High-quality data provide the basis for decisions that affect weather, marine environment, fish stocks, climate, commerce, and security. For these reasons, a main driving force behind this PPP is the shared goal of making “fit for purpose” observations that have known and transparent QCs and, therefore, are trusted by the research community and, ultimately, by the public. To implement this vision, the roles of each partner must be defined, and the community must provide standards for data management and verification that are transparent and standardized. A cautionary lesson in the proper setting of data quality acceptance standards, availability, and archiving of contracted data is highlighted in an example from the ground-based Global Navigation Satellite System (GNSS) data (Serra et al., 2018). Because exact specifications for the data were not written into the vendor contract, the U.S. government must now pay for data produced by the private entity that it cannot use and cannot change. It is also imperative for the government to maintain in-house expertise to continually evaluate these data and act as a repository of expertise, should any commercial venture fail.
PPP: Saildrone as a Case Study
Public Interest
NOAA-PMEL has a long history of bringing innovations to ocean observations primarily through global-scale buoy arrays and sensor developments (McPhaden et al., 2010; Bernard and Meinig, 2011; Sutton et al., 2014). Saildrone’s founder spent ∼10 years perfecting highly innovative and robust wind-powered platforms. These innovative cultures mesh well, with each bringing unique strengths and shared goals to ocean-observing development. The team built momentum around shared values of “learning by doing” and, in the first year, tested prototypes with several integrated sensors and extended missions off central California, Gulf of Mexico, and the Bering Sea (Cokelet et al., 2015; Meinig et al., 2015).
Broader high-level impacts of interest to both parties for developing a scientific USV include the opportunity to address mission goals for oceanographic observing, as well as the opportunity to improve infrastructure and approach important scientific questions. NOAA’s mission is to understand and predict changes in climate, weather, oceans, and coasts and to share that information in support of the blue economy, environmental sustainability, and coastal management. The cutting-edge measurements that Saildrone USVs can provide may result in model and forecasting improvements that are central to this mission.
Saildrone USV
A goal of Saildrone is to address the scarcity of in situ ocean data by augmenting ship and buoy infrastructure with flexible and efficient autonomous technology. The core components of this network are the Saildrone USVs, which combine wind-powered vehicle technology with solar-powered meteorological and oceanographic sensors for long-range data collection missions in the toughest of ocean environments.
The Saildrone USV is an autonomous ocean-going data collection platform designed for long-range, long-duration missions of up to 12 months. The Saildrone USVs operate solely on renewable energy, using wind power for propulsion and solar power to run a suite of science-grade sensors. Each vehicle consists of a 7 m long narrow hull, a 5 m tall hard wing, and a keel with a 2.5 m draft. Each weighs approximately 750 kg and can be launched and recovered from a dock.
The Saildrone USV’s propulsion system is the result of a 10 years research effort in high-performance land sailing and consists of the tall hard wing, a longitudinal spar, and a vertical tail. The wing angle to the wind is adjusted by a trim-tab on the tail, similar to the way an elevator trim tab controls the pitch of the aircraft. Each vehicle travels at an average speed of 1.5–2.5 m/s and can reach top speeds above 4 m/s, enabling these USVs to cover large survey areas and reach most ocean locations within 30 days from the nearest shore.
The sensor payload consists of ∼20 sensors that measure key atmospheric and oceanographic environmental variables, with the goal of making climate quality measurements. These include solar irradiance, longwave radiation, atmospheric pressure, air temperature and humidity, wind speed and direction, ocean skin temperature, bulk water temperature, salinity, ocean color (Chl-a, CDOM), atmospheric and seawater partial pressure of CO2 (pCO2), dissolved oxygen, and pH. The vehicles have also been equipped with acoustic doppler current profilers (ADCP), scientific echosounders for biomass assessment and bathymetry surveys, and passive acoustic recorders for marine mammal studies (Mordy et al., 2017). The sensors are connected to onboard computers and transmit data in real time via satellite communications to shore-based data centers.
The Saildrone USVs transmit their position and health status via satellite, and the entire fleet is under the constant supervision of a centralized mission control, staffed by human pilots aided by sophisticated automation tools. The vehicles navigate autonomously from prescribed waypoint to waypoint, accounting for wind and currents, while staying within a user-defined safety corridor. To further ensure safe operation, each USV is equipped with an Automated Identification System (AIS) transceiver, navigation lights, radar reflector, high visibility wing colors, and four onboard cameras. Together, these measures have resulted in a strong track record of safe operations.
Saildrone Business Model
The Saildrone USV is just a small component of this new data collection infrastructure, and as such, the vehicles themselves are not for sale. Instead, Saildrone offers the data collected by its vehicles on a data-as-a-service basis, for a flat daily rate, without any upfront investment required from the user, while Saildrone takes care of all operational mission execution. This rate covers the lease of the vehicles and all of their core sensors, as well as integration, calibration, and testing; it also includes vehicle deployment and transit to the area of operation, 24/7 navigation, and piloting; data retrieval via satellite link, and its visualization via a robust data portal; and the delivery of high-resolution data in NetCDF2 format (minute-level subsampled data in near real-time and raw data at mission end for full transparency and further QC).
The private sector has, so far, provided all upfront capital expenses (over $100M has been contributed to date), which ensures that this new infrastructure can become operational without the delays associated with large and complex public investment and also ensures that each user’s budget is fully directed toward its specific data collection efforts while still leveraging the full power of the overall infrastructure.
Furthermore, in cases where multiple stakeholders with overlapping data interests can be served by the same regional USV fleet, operating expenses can be distributed among the group, further increasing cost-efficiencies for all. This mode of operation is analogous to a satellite array managed by a command-and-control center and serviced by a global operations team of engineers serving a global user base. The long-term nature of observations ensures the financial sustainability of the infrastructure over a long horizon.
Forming the PPP
The PMEL and Saildrone partnership started in 2014 with an exploration of what value a long-endurance USV outfitted with numerous scientific sensors could bring to PMEL mission areas from the Arctic to the tropics. After a prototype Saildrone USV (without sensors) proved it could cross the Pacific from San Francisco, CA, to Honolulu, HI, the teams met several times to discuss forming a partnership to integrate PMEL sensors and leverage experience from decades of operating global buoy arrays. Common organizational goals were (1) high-quality surface and subsurface ocean observations in near real time; (2) highly reliable, long-endurance USVs that can operate globally; (3) elimination of shiptime for operations; and (4) a large, multidisciplinary payload capacity.
Based on common interest in developing a scientific USV, the teams established a Cooperative Research and Development Agreement (CRADA). A CRADA is an agreement under which a U.S. government laboratory contributes personnel, services, facilities, equipment, or other resources – but not funding – toward the conduct of specified research or development efforts. The CRADA business partner contributes any necessary funding to the project as well as personnel, services, facilities, equipment, or other resources. A CRADA is intended to speed the transfer and commercialization of technology by optimizing resources and protecting the private company’s intellectual property (IP) rights. Additionally, the CRADA contains high-level goals for the partnership and lays out the basic framework for the development effort.
Once benchmark standards of data quality have been assured, the PPP between NOAA and Saildrone has the potential to improve efficiency and cost-effectiveness as follows: (1) private investment is able to capitalize on cost savings through economy of scale, (2) consolidation of expertise by the private sector allows scientific investigators to focus on data ingestion and results, and (3) easily accessible data ensure that the data products generated are provided to multiple investigators, maximizing impact.
Challenges Met by This PPP
In a resource-limited environment, the FOO (Lindstrom et al., 2012) encouraged increased coordination and leveraging of existing observing assets, but a pathway to the development of the necessary technological advancements through increasing readiness levels (RL) was a slow, multiyear endeavor. However, our PPP was able to significantly accelerate this process.
At the start of the PPP, the Saildrone USV as an ocean science platform had an RL of 3 as defined by Lindstrom et al. (2012). Four years later, the Saildrone USV (Figure 1) has 20 sensor packages integrated into the platform, with near real-time data delivery (Figure 2) and an RL range of 7–9. The system, including all subsystem components and processes, was successfully tested as part of this PPP. Saildrone has completed over 200,000 nautical miles of missions and continues to work closely with NOAA-PMEL (Figure 3) and other entities to demonstrate that the mission data are of high quality and can meet the requirements of the community.
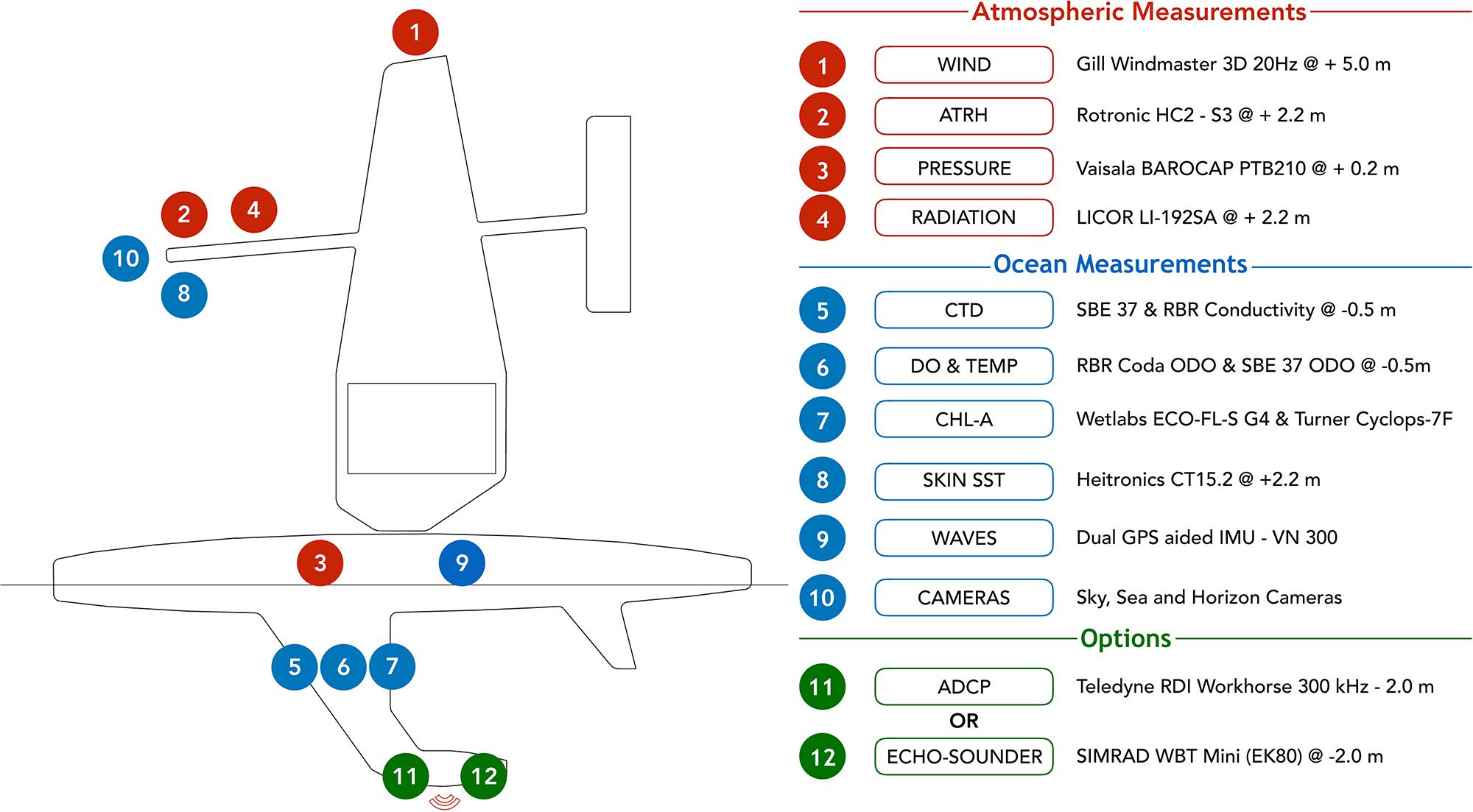
Figure 2. Saildrone USV surface and subsurface core sensor suite. Additional application-specific sensors have routinely been added to the core suite to measure parameters such as atmospheric & dissolved pCO2, short and long wave solar radiation, passive acoustic recordings, and other specialized measurements.
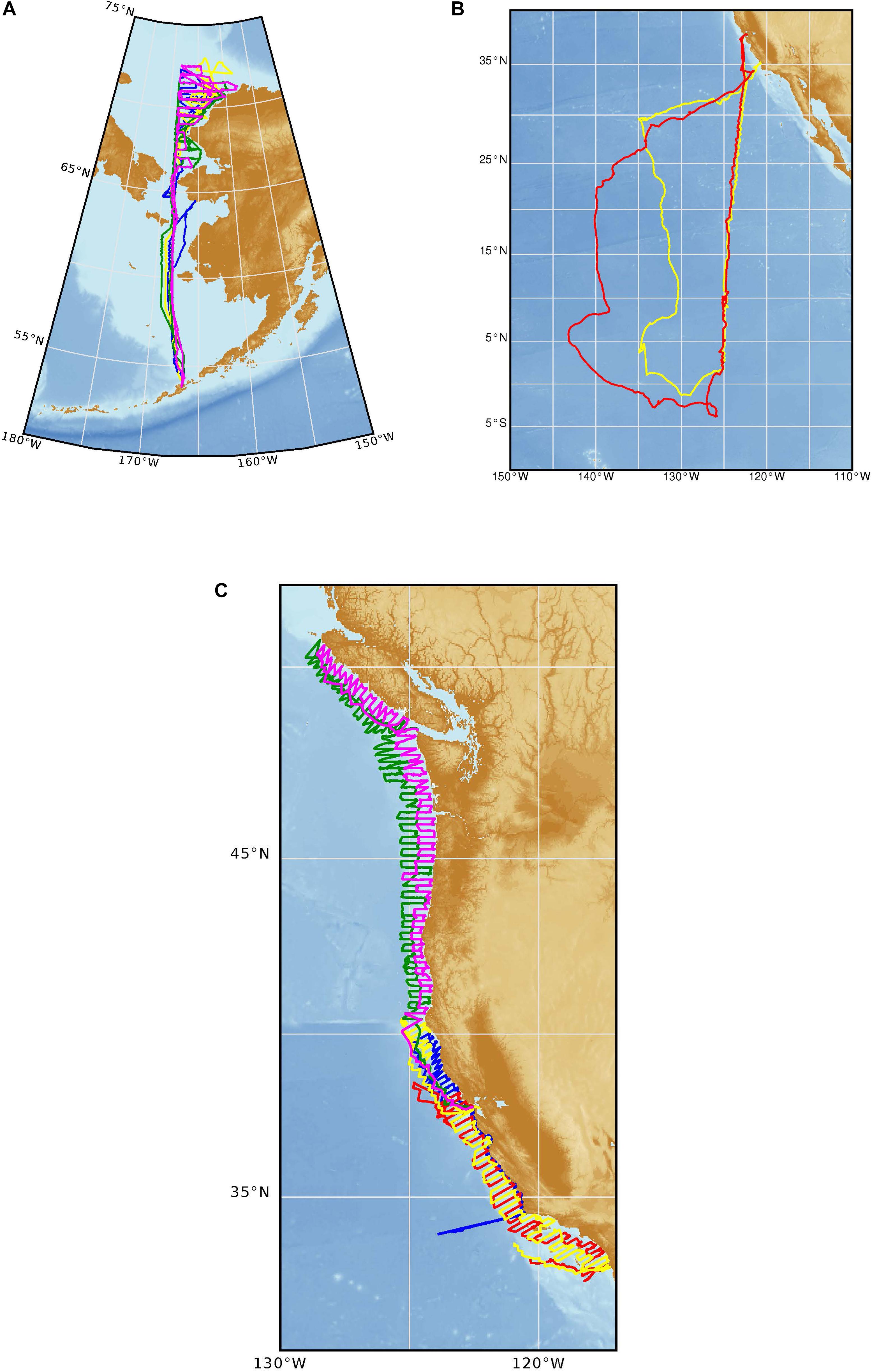
Figure 3. Saildrone USV tracks from (A) four vehicles during a four-month mission in the Bering and Chukchi Seas in 2018, (B) two vehicles during a ∼nine-month equatorial mission in 2017–2018, and (C) five vehicles during a four-month fisheries mission on the North American West Coast.
These successes would not have been possible without acknowledging and carefully planning to face several important challenges. From the beginning, both parties shared a vision for a large fleet and an entirely new scale of data production. We also noted that these new platform and survey capabilities would likely lead to a demand for new sensor development in the future, although a relatively low demand has historically limited this market. Each of these issues on their own had historically caused many headaches for the scientific community. Here, we share some of our insights based on these three difficult issues and how they informed our planning and the vision for a large-scale Saildrone fleet.
Observing Network
A Saildrone USV network can provide data at an entirely new scale, including an unprecedented new flexibility. However, this scale requires operating a large fleet of data collection platforms. Historically, stitching together disparate data sets from individual campaigns has been a major challenge. Many programs rely on postcorrecting and synthesizing data to generate global data sets and climatological products [e.g., Surface Ocean CO2 Atlas (SOCAT) Program; Lauvset et al., 2018].
When envisioning the activity of a Saildrone USV fleet, we did our best to consider the scientific community’s long experience with these challenges by thinking through how to plan surveys that would be easily intercomparable. Part of this is an innovative survey design that not only includes calibration and intercomparison opportunities and overlapping tracks but also suggests that the fleet should carry standard sensors, use consistent calibration methods, and set clear QC metrics.
The fleet refresh rate was also a critical point to consider: because of issues like biofouling and recalibration, deployments would likely be limited to approximately 1 year. This could be less in some regions: for instance, as a sailing vehicle that depends on sunlight to power sensors, in high latitudes, Saildrone USVs have an operational limit based on solar availability, freezing sea spray, and ice cover. However, with a scaled fleet, year-round persistence outside of extreme latitudes will be possible by swapping vehicles on location periodically.
Data
Multidisciplinary observational platforms like the Saildrone USV generate huge amounts of data, especially when integrated with complex motion-corrected instrumentation such as echosounders, ADCPs, and three-dimensional (3D) wind measurements. After the first scientific mission in 2015 generated several terabytes of scientific data in 3 months, PMEL and Saildrone formed a data management working group and began to develop a data management and delivery pathway. This effort focused on the automated delivery of a climate and forecast (CF)-compliant, self-documented, NetCDF files with goals of making data readily available to the broad oceanographic community and enabling researchers to focus directly on data analysis and investigation results.
In the days of Amazon, Apple, Facebook, and Google-size data sets, tools exist to manage data at scale. However, this scale is new for in situ oceanographic data and means that Saildrone must rely on modern big data methodologies as data sets grow exponentially. Saildrone is working not just to implement large-scale storage and computation but also to manage data quality and metadata at very large scale. The science-driven technology development PPP method laid out in this paper provides an outline for addressing the very important concerns of data quality and chain of custody when a private company is involved in the collection and processing of data sets.
New Sensor Development
Oceanographic sensor development has been slow because the market size for such sensors is small when compared to sensor markets such as laboratory, industrial, and commercial monitoring, measurement, and testing equipment. Additionally, the oceanographic sensor market is dispersed over both a wide range and number of public, academic, and private institutions with stringent and often unique sensor requirements. Building an array of hundreds of USV vehicles equipped with standardized sensors has the potential to contribute significantly to market share and generate enough focused demand to drive the commercial development of new specialized sensors.
One example of new sensor development is the Saildrone–PMEL autonomous surface vehicle pCO2 (ASVCO2) system. This system is based on the MApCO2 technology (Sutton et al., 2014), which was transitioned from PMEL to Battelle Memorial Institute and sold commercially as the Seaology pCO2 monitoring system. Over the last decade, Battelle sold ∼120 systems worldwide. This lack of demand relative to the commercial demand of other Battelle products has resulted in the stagnation of technical development, obsolescence issues, and, ultimately, the abandonment of this sensor as a commercial product. Despite these commercial challenges, there is a significant scientific demand for a pCO2 sensor based on ocean-observing needs. Targeting key gaps in the Surface Ocean CO2 NETwork (SOCONET) array could reduce uncertainties in the ocean sink of anthropogenic CO2 (Wanninkhof et al., 2019). If the PMEL–Saildrone partnership can build and deliver codeveloped pCO2 systems that collect climate-quality surface ocean pCO2 data (<2 μatm), this opens a new market to fill in major observing gaps in SOCONET.
Development of a Drone Into a Science Tool
Considering these questions helped inform the design of a large-scale USV fleet and what the key operational design challenges would be. However, we first needed to develop the Saildrone USV into a scientific tool. In order to make high-quality measurements of ECVs and EOVs, many factors need to be considered, including details about the sensor’s initial quality, drift, calibration, and platform effects. Engineers and scientists need to build and maintain tight feedback loops so that each sensor and essential variable is properly understood and calibration and validation is tracked during hardware and software design cycles. Critically, the overall development needs to include not only laboratory testing but also at-sea comparisons. These experiments need to be properly designed with detailed knowledge of temporal and spatial variabilities of each essential variable in the operating environment so that new sensors and platforms such as the Saildrone USV can be properly compared with established standards, which are usually ships or buoys.
Sensor Integration
The team collaboratively chose an incremental sensor integration strategy to gain some early “easy” successes based on science requirements before proceeding to more difficult sensors. Figure 4 shows a progression of testing and validation for several sensors suites. In general, the team progressed from basic atmospheric (solar irradiance, wind, barometric pressure, air temperature, and relative humidity) and ocean physics measurements (water temperature and salinity) to biogeochemical measurements (ocean color, dissolved oxygen, pH, and pCO2) to complex active acoustics (fisheries, ADCP, charting) and broadband passive acoustics (marine mammals, subsea volcanoes).
This incremental approach divided the integration effort into discrete, year-long, sensor suite developments, which contained the following steps:
(1) Determine variables to measure based on ECVs, EOVs, and NOAA research priorities.
(2) Identify sensors to integrate and sensor sampling protocols based on science specifications.
(3) Integrate identified sensors onto the Saildrone USV platform with the goal of producing high-quality data.
(4) Validate the suite of instrumentation through carefully designed sensor intercomparisons.
Codevelopment Working Groups
To ensure the delivery of high-quality data, the PMEL–Saildrone effort was structured around joint science–engineering working groups. Within these groups the PMEL engineers, who were embedded with the scientists at the research laboratory, facilitated the flow of information and communicated science and engineering requirements/realities across the culture gap that existed between the government scientists and the private company engineers. This communication structure has proven very successful in both development and validation. Because of the multidisciplinary nature of the Saildrone USV platform, these working groups shared a common core of PMEL and Saildrone engineers. Working groups varied in size and scope from large groups containing scientific expertise from all of PMEL’s major research programs, to small and highly focused groups of measurement and data specialists. An important aspect of these working groups was their flexibility. As new sensors were integrated and data processes developed, new working groups were formed or the focus of existing groups was modified.
In order to establish scientific confidence in observations outside of PMEL’s expertise, the PMEL–Saildrone team sought out and developed external partnerships with established experts in the fields of fisheries acoustics, 3D wind flux, and wave measurement. These relationships varied in scope and included research partnerships that resulted in multidisciplinary scientific USV missions, joint peer-reviewed journal publications on observational methods, contracts for specific sensor development, and informal review and advice on sensor integration and validation methodologies.
Working groups for bulk surface measurements and specialized sensor measurement groups for ADCP, 3D wind flux, and echosounder established scientific confidence in the Saildrone USV sensor measurements and sampling protocols. Additional working groups addressing pre- and postmission sensor calibration requirements, CF compliant data formats, and a drone-to-desktop data delivery pathway ensure data quality and chain of custody.
Validation of Observations
Detailed comparisons have been run in several oceans for EOVs and ECVs to understand the effects and corrections of a moving Saildrone USV in a variety of sea states. Saildrone and PMEL engineers and scientists collaboratively design the validation experiments and view the data in real time to catch biases and work out difficult sensing problems. As an example, underway ADCP measurements require extremely accurate heading information and precise sensor installation geometry so that ping-by-ping corrections can be made without introducing bias. These types of comparisons are typically intensive during the early development cycles but continue as spot checks for all missions. To date, EOV comparisons have been done with (1) Saildrone USV ADCP and an upward-looking moored ADCP off California deployed specifically for the intercomparison purpose; (2) Saildrone USV surface air and sea measurements against shipboard and surface moored buoys during science missions in the Arctic/Bering Sea, the tropical Pacific, the U.S. West Coast, and the Tasman Sea; and (3) Saildrone USV and shipboard fisheries acoustic sounders. Figure 5 is an example of wind speed validation between Saildrone USVs, ship, and buoy in the Bering Sea.
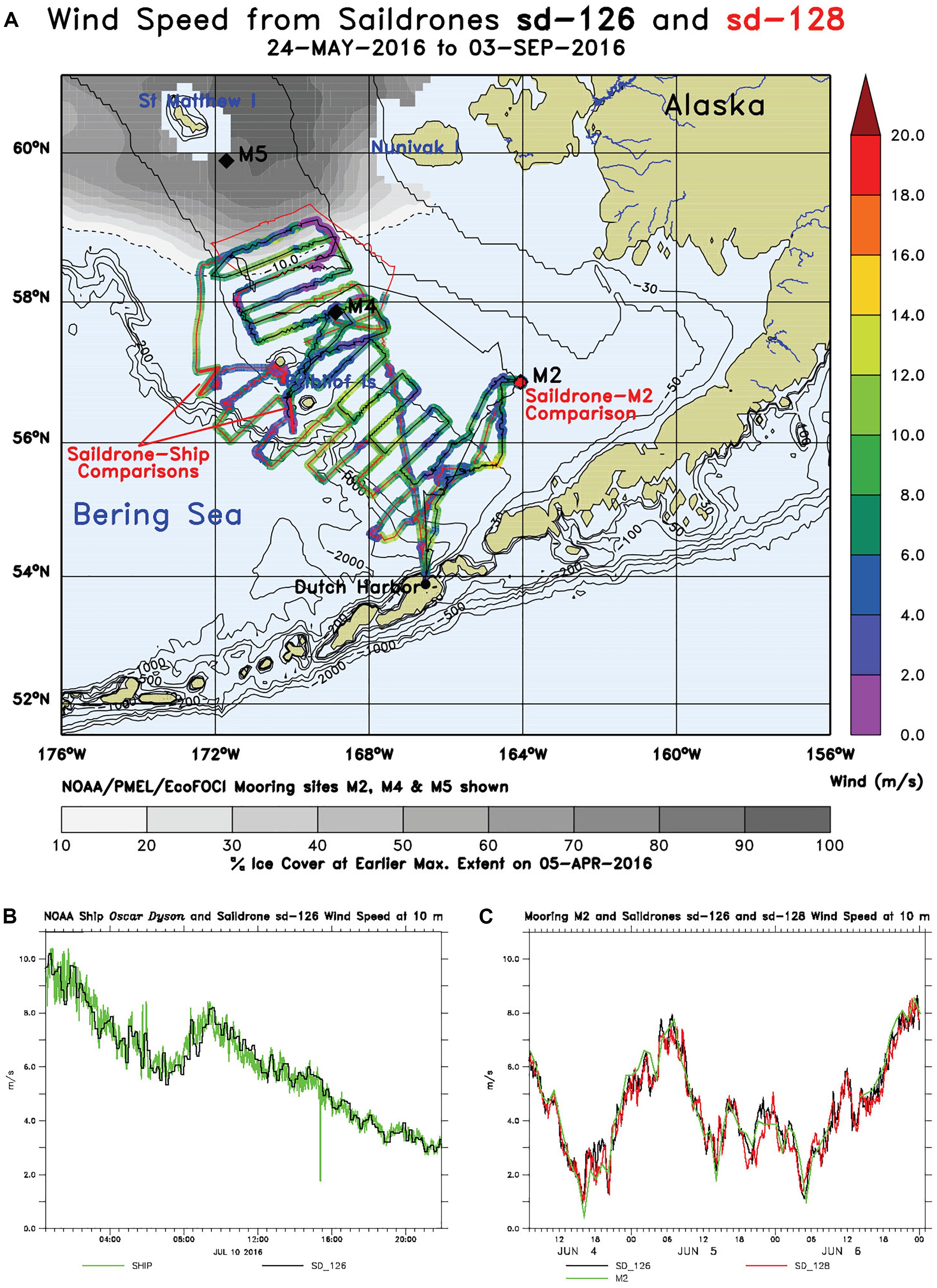
Figure 5. (A) Map of the wind speed (m/s) along the tracks of two Saildrone USVs in the Bering Sea, 24 May–3 September 2016. Saildrone USV sd-126’s track line is black, and sd-128’s is red. During this 103-day mission, the mean wind speed was 5.7 m/s with peak gusts to 23 m/s. Also shown are the sites of the comparisons between the Saildrone USVs and NOAA Ship Oscar Dyson and NOAA/PMEL research mooring M2. (B) The wind speed as measured by the ship (green) and Saildrone USV sd-126 (black). The comparison is good with an rms difference of 0.44 m/s. (C) The wind speed as measured by mooring M2 (green) and Saildrone USVs sd-126 (black) and sd-128 (red). The rms differences between the M2 and Saildrone USV winds were 0.49 and 0.54 m/s, respectively.
To further establish community-wide confidence in the validity of the Saildrone USV data, the results of these validation studies are in the process of being published in peer-reviewed journals. Currently, there are papers in early stages of development addressing 3D wind flux, USV carbon measurements, bulk surface met and ocean measurements, and fisheries acoustics (De Robertis et al., 2019; Zhang et al., 2019).
Data Delivery
Saildrone assembles data into two delivery modes, real time and postmission. The real-time datastream delivers averaged data samples during the mission to the mission owner via the Saildrone mission portal and as data files. Meteorological and oceanographic parameters are averaged to 1 min samples and delivered every 2 h to the mission operator as self-documenting, CF metadata-compliant, NetCDF Discrete Sampling Geometry (DSG) files that meet the underway observation feature type. For the postmission data, a NetCDF template is defined for the delivery of a larger selection of scientific and engineering data. These data are averaged to 1 and 10 Hz and delivered as a single file per day. Under this scheme, the data volume is ∼6 GB per month per vehicle. These data are not quality controlled at present.
Data Acceptance and Reprocessing
The objective of the data acceptance workflow is rapid data ingestion so that the data are available to scientists within 5 min after delivery to the mission operator (PMEL). As Saildrone delivers data in fully documented NetCDF files, minor data reformatting is required to make these data available through interoperable data services. Data are transferred to PMEL by FTP, where timed processes move the data from the FTP server to an internal server (Figure 6). Derived parameters (e.g., wind speed, direction, and USV separation distances) are computed and added to the NetCDF files.
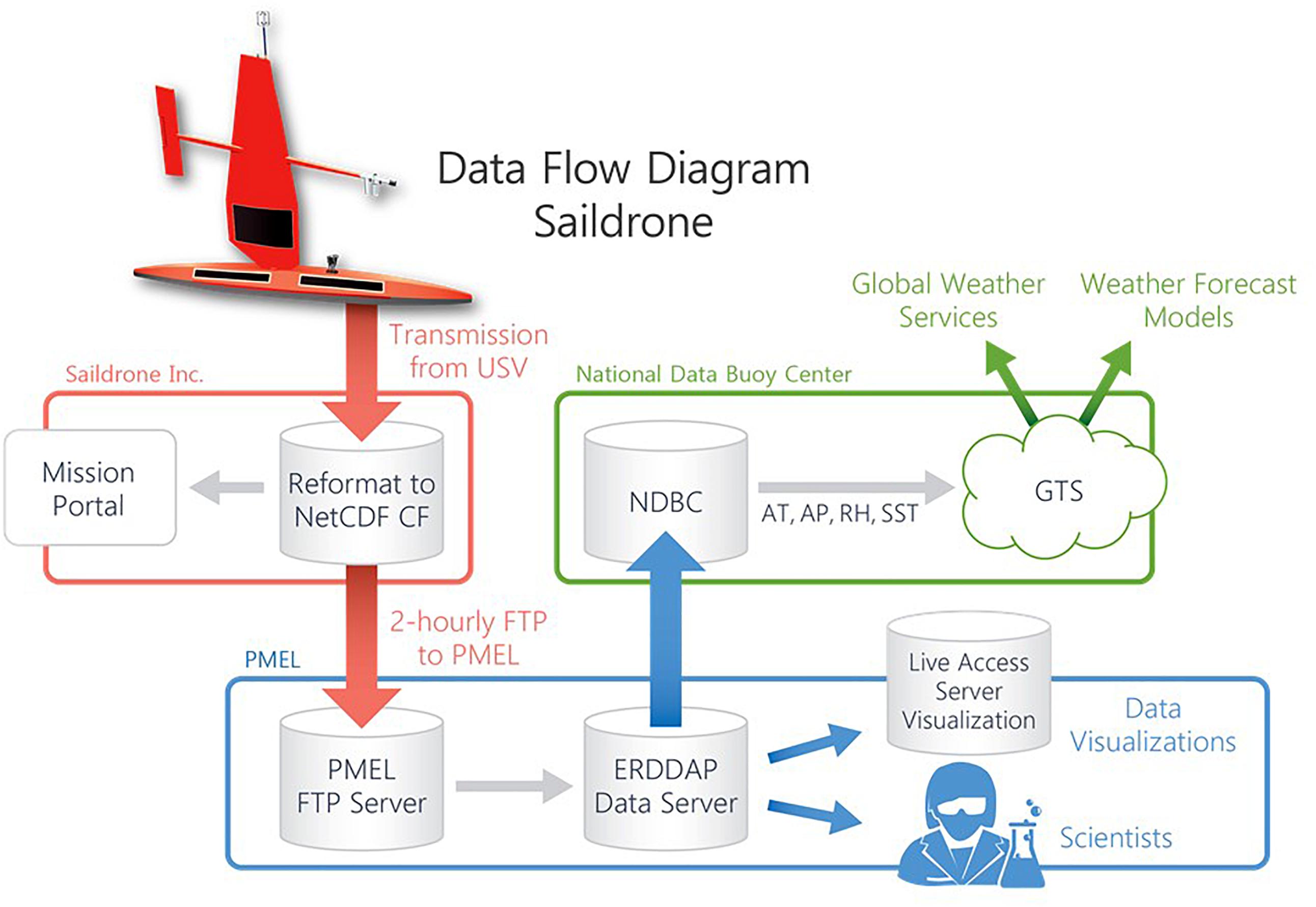
Figure 6. Near real-time data workflow between Saildrone Inc., PMEL, and operational data dissemination network (GTS).
Operational use of measurements from Saildrone USVs requires these data to be available to weather forecast centers via the World Meteorological Organization (WMO) Global Telecommunications System (GTS). The GTS is a system of dedicated and shared network connections organized to distribute meteorological and oceanographic data to operational forecast centers.
By leveraging a pilot project undertaken by PMEL, the OpenGTS project3, some variables from Saildrone USV platforms are placed on to the GTS every 10 min in highly structured formats that require a minimum amount of metadata. A Binary Universal Form for the Representation of meteorological data4 (BUFR) template specific to unmanned surface platforms, such as Saildrone USV, is now being defined.
QC is essential to the long-term viability of this effort, and groups 1 and 2 QC will be applied to these data using methods developed for the Integrated Ocean Observing System (IOOS) Quality Assurance for Real Time Oceanographic Data (QARTOD) as the guidance (Willis et al., 2015).
Data Interoperability
The Environmental Research Division Data Access Platform5 (ERDDAP) data server is used to provide interoperable access to the data. ERDDAP ingests data in many formats and outputs aggregated data sets in more than 30 formats via a graphical user interface and through a machine-to-machine Representational State Transfer (REST) interface. This REST interface to ERDDAP’s rich functionality can be leveraged by other applications to provide the user with specialized services, such as visualizations or data analysis tools.
ERDDAP’s machine-to-machine services provide users with powerful data access functions with applications that use ERDDAP as a middle-tier data broker. In general, data access is increasingly steering away from graphically rich “data portals” to data platforms that provide a broad set of services through which data can be accessed by other applications to serve a particular science community.
Current Regional Saildrone Projects
The current fleet of ∼70 Saildrone USVs is composed of a collection of regional fleets, with each fleet ultimately deploying between 20 and 50 vehicles from a local operating hub (e.g., Dutch Harbor, AK, for U.S. Arctic operations). Each section below includes a brief summary of work to date that has been completed in each region.
Arctic Fleet
Since 2015, a Saildrone USV fleet has been conducting annual surveys of the U.S. Arctic (Bering and Chukchi Seas) (Cokelet et al., 2015; Mordy et al., 2017). These surveys have focused on the following activities: meteorological observations to improve forecasting, multisensor improved SST (MISST) for calibration of remotely sensed data, surveys of carbon flux using ASVCO2 systems, ADCP surveys of ocean circulation to understand basin–basin and open ocean–shelf exchange of water masses and nutrients, acoustic surveys of Arctic cod (Boreogadus saida) and walleye pollock (Gadus chalcogrammus), bathymetric data, focal follows of tagged northern fur seals (Callorhinus ursinus), passive acoustics, and measurements focused on the marginal ice zone (MIZ).
Tropical Pacific Fleet
Missions to the tropical Pacific began in 2017 to test the Saildrone USV’s ability to make climate-quality meteorological, oceanic, and biogeochemical observations as part of Tropical Pacific Observing System (TPOS) 2020 project (Cravatte et al., 2016; Smith et al., 2019). These pilot study surveys (Zhang et al., 2019) have included field intercomparisons of a full suite of meteorological, biogeochemical, and oceanic sensors used to estimate the wind stress and the air–sea exchanges of heat and CO2. These pilot study surveys have also tested Saildrone USV performance under challenging tropical Pacific conditions, including low wind, strong currents, and strong air–sea interaction at fronts. The ability to adaptively sample and measure multiscale variability make the Saildrone USV a powerful tool for monitoring evolving ENSO. A scientific focus of these missions has been to resolve mesoscale air–sea interactions because of their potential importance in ENSO development and rectification into large-scale air–sea interaction and teleconnections. These small-scale processes are neither adequately observed by the current TPOS nor are they resolved or correctly simulated in state-of-science coupled models.
Southern Ocean Fleet
Following initial work conducted in the Southern Ocean based out of Hobart, Australia, in partnership with the Commonwealth Scientific and Industrial Research Organisation (CSIRO), an inaugural Saildrone USV fleet has launched to circumnavigate Antarctica. The Southern Ocean fleet is optimized for the high wind and heavy sea state prevalent in the Southern Ocean and is equipped to measure CO2 flux using ASVCO2 systems, conduct acoustic krill surveys using science-grade echosounders, track tagged animal migrations, conduct marine mammal studies using passive acoustic recorders, and measure currents (ADCP).
Coastal Fleet
Saildrone USVs are highly capable of detailed coastal surveys. In 2018, Saildrone completed a full survey of the North American West Coast from Vancouver Island, Canada, to San Diego, CA, using five Saildrone USVs to complete a grid of 40 nautical mile transects spaced 10 nautical miles apart over 100 days (Figure 3C). Coastal surveys can significantly augment existing assets such as ships and moorings, and Saildrone USV fleets have been deployed for acoustic fish stock and bathymetry surveys, maritime domain awareness (AIS and smart cameras), meteorological data for improved weather forecasting, environmental monitoring (surface hydrocarbon detection and MIZ studies), and methane and CO2 seep detection near carbon sequestration sites.
Saildrone is committed to long-term PPPs to ensure this technology meets the scientific community’s needs at scale. By marrying the best science from the ocean-observing community with the private sector’s capability to invest in next-generation infrastructure, new high-accuracy and large-scale data sets can be obtained in the very short term to help address the current scarcity of in situ ocean observations. This partnership works primarily due to a shared commitment to operational excellence and high data quality standards. As this fleet of USVs expands, it is critical to expand engagement with the broader community around needs and requirements.
Vision
The promise of autonomous technology has always been to lower the data acquisition cost by an order of magnitude, something modeled by the Argo float network and expanded on by unmanned technologies such as Saildrone USVs. In turn, this reduction in cost enables very large-scale geospatial coverage for persistent or adaptive observations.
The main obstacle for implementing such networks has traditionally been the high level of upfront capital expenditures required. Some, such as Argo, successfully rallied national funding agencies to make large-scale investments, while others, such as underwater gliders, have built more localized networks.
As funding for infrastructure has become more limited, a PPP may serve as a pipeline to ensure continuity and serve new demands in ocean observations. The private sector is now taking a more involved role in setting up this type of infrastructure, in large part because it is a key beneficiary of the insights obtained and because the present era of evolving global weather and climate patterns has a substantial impact on the private sector. This creates an unprecedented alignment of global observation needs, technology advancement and cost-effectiveness, and private sector investment capacity and interest. However, to reap the scientific benefits of such efforts, communication and involvement of the regional-based and observation-based communities, beyond government agencies, are key requirements.
Observations are about “Systems of Systems,” and success depends on community involvement to ensure the right mix of technologies for each domain while not stifling innovation. USVs would not meet the community’s needs in isolation, but instead community input can ensure that the unique strengths of different technology solutions are leveraged to enable the System of Systems to have the maximum impact. For example, when unmanned systems augment ship-based surveys, they may provide reconnaissance, allow for adaptive sampling, and enable the ship to focus on high-value use cases, such as on-board science. USVs also can augment buoys by providing measurements in areas that are impractical for buoy deployment, provide in situ validation to enhance the value of remote sensing data sets, and measure air–sea interactions to augment subsurface (e.g., the Argo network) and atmospheric measurements (e.g., remote sensing and moored fixed-point time series measurements).
Optimizing the effectiveness of this type of System of Systems requires design input from the community to ensure that the network of USVs can address the most pressing questions at scale as it continues to extend its operating area to cover the world’s oceans.
Vision Structure and Framework
This case study has demonstrated that USVs can provide high-quality atmospheric and oceanographic data for individual projects on a regional scale. Specifically, the committed and cooperative working relationship between NOAA and Saildrone has led to scientific confidence in the quality and accuracy of valuable measurements. Similar outcomes have been achieved by partnerships with other national and international agencies through regional deployments distributed in a variety of ocean basins. The experience, to date, compels us to consider the potential for a coordinated international effort to establish a global network of USVs that ultimately could contribute to the Global Ocean Observing System (GOOS) and the Global Climate Observing System (GCOS).
The evolution and ongoing success of the Argo float program is a natural place to begin a discussion of how a global USV system might be achieved. While there are similarities with Argo, such as the concept of global network of autonomous platforms, several important differences need to be considered. Operational Argo floats were developed from community experience with the ALACE floats used for the 1990–1997 World Ocean Circulation Experiment (WOCE). In contrast, the existing USVs have been developed largely by private industry. Argo floats are designed to be expendable, while most USVs are designed to be retrieved and redeployed multiple times. Other technological contrasts include the added complexity of making measurements at the ocean surface as well as the increased number and variety of potential sensors. A complete treatment of the issues relevant to designing and implementing a global USV observing system is beyond the scope of this paper. Here, we seek to highlight some of the important issues and to provide a broad outline of the steps that would be necessary to evaluate the possibility of implementing a global network of USVs.
The history of how the Argo program evolved can provide valuable guidance for evaluating the need and practicality of a global USV observing network. The major components that led to the development of Argo were outlined in a presentation at the 13th meeting of the Argo Steering Committee in 2012 (Roemmich, 2012). These elements included the following:
• A statement of requirements
• Appropriate, cost-effective technology
• Consensus among user groups on its value
• Entrainment of agency sponsors and collaborators
• International scientific collaboration
• Intergovernmental coordination
• Commercial partnerships
Argo is an independent and voluntary entity administered by the Argo Steering Committee and is a major contributor to GOOS, which is under the Intergovernmental Oceanographic Commission (IOC) of UNESCO. As noted above, the idea of a global array of ocean profiling floats was an outcome of WOCE. Community consensus included endorsement by the Global Ocean Data Assimilation Experiment and the CLIVAR Upper Ocean Panel. Following these endorsements, a formal statement of requirements was developed at an international workshop. U.S. agency participation followed and was led by NOAA, with Argo seen as a global compliment to the Tropical Atmosphere Ocean array. A pilot project through the National Ocean Partnership Program, sponsored by NOAA and the Office of Naval Research, led to the multi-institution U.S. Argo Float Consortium. International partners (Japan, India, United Kingdom, France, Australia, and SOPAC) were engaged through contacts at the agency level. In addition to the Steering Committee, Argo includes a Data Management Team and runs science, technical, and training workshops. Argo is managed by the Argo Project Office, with each participating country responsible for funding and determining priorities for their own deployments.
Over the past several years, the potential for USVs to contribute to the global ocean-observing network has gained significant interest. For example, the Data Buoy Cooperation Panel (DBCP), under the Joint Technical Commission for Oceanography and Marine Meteorology (JCOMM), has an ongoing effort to evaluate USVs as a compliment, or even a cost-effective alternative, to moored buoys. DBCP states that such an evaluation will require detailed examination of data quality, reliability over extend deployments, and operating costs. Our experience and other reports using a variety of high-endurance (>6 months) surface platforms have demonstrated the endurance and versatility of currently available USVs. These can be categorized by their means of propulsion as either wave or wind driven. In addition to Saildrone USVs, currently available wind-propelled USVs include the C-Enduro, Sailbuoy (Ghani et al., 2014), Ocean Aero, and Harborwing. Wave-propelled USVs include the Wave Glider (Hine et al., 2009) and the Autonaut (Johnston and Poole, 2017).
In addition to providing many of the functions of surface moorings, the ability of USVs to be remotely piloted allows them to operate as a movable array, alternating between station keeping and transect survey mode. Thus, USVs can provide an extension and enhancement of fixed arrays through sampling on spatial and temporal scales that are not currently captured. The flexibility and independence from ship operations for deployment and recovery provide the ability for USVs to fill gaps in observing system arrays, such as the existing need for moored observations in the western equatorial Pacific or other emerging needs, such as air–sea flux measurements to capture ENSO precursors. The TPOS 2020 project, tasked with evaluating all elements that contribute to the existing TPOS, considers USVs to be at the pilot project RL and is evaluating their potential contributions. There are also several international efforts to evaluate USVs for extended observations such as the flux glider project sponsored by JAMSTEC and the United Kingdom-based Marine Autonomous Systems in Support of Marine Observations (MASSMO) through the National Oceanography Centre (NOC).
In summary, experience over the past few years has demonstrated pilot level technology for individual projects at the regional scale. Private industry investment in the development of a variety of USVs has allowed this new technology to evolve much more rapidly than would be possible through government-funded research alone. The scientific and operational communities need to decide how to take advantage of this unprecedented opportunity for expansion of the ocean-observing network, potentially to the global scale. For example, Cronin et al. (2019) have proposed deploying a global fleet of USVs to produce an in situ air–sea flux product with a 10° × 10° resolution. The community needs to determine gaps in the existing network that USVs can address, prioritize resources, and establish sensor and data standards perhaps through existing organizations such as the DBCP. For companies with mission-as-service business models, the community needs to explore how national and international agency sponsorship can be engaged since, in general, there is no precedent for this type of PPPs at the global scale. A plan should be developed to establish community confidence in the capability of USVs to provide accurate measurements, and an organizational structure should be established to ensure ongoing QC for data that would be widely distributed. A starting point would be to convene an international workshop to address these questions by involving current users, potential beneficiaries, manufacturers, and governing agencies.
Vision – Saildrone Fleets
The current fleet of 70 Saildrone USVs is composed of a collection of regional fleets, with each fleet ultimately deploying between 20 and 50 vehicles from a local operating hub. Saildrone is planning a fleet expansion of ∼200 USVs by the end of 2019 divided into regional fleets. In the coming years, Saildrone’s target is to deploy ∼1,000 vehicles, capable of real-time data collection across all oceans at a target resolution of 6° by 6° and operated from ∼20 regional hubs. The data generated from this large set of sensors (20 per USV or ∼20,000 total) will continue to be subject to a strict QC framework developed and managed on an ongoing basis in close partnership with the global science community, following the successful blueprint of established systems such as the Argo network.
Vision – Data
Delivery
In a rapidly changing climate, the efficient delivery of in situ ocean observations at a high spatial and temporal resolution will become increasingly important. Data handling services should allow data from USVs to be delivered via cloud computing to mission operators in near real time for immediate interoperable access (Buck et al., 2019). Ease of access will need to be balanced with IT system protections to ensure data integrity. As illustrated by the cases described in this paper, it is imperative that the scientific community work closely with commercial cloud providers and platform operators to ensure services are in line with scientific best practices (Pearlman et al., 2019; Vance et al., 2019).
Quality Control
An important distinction in the case of the Saildrone USV is that the platform cannot be purchased, deployed, or operated by the end user of the data. Saildrone’s business model is based on a fee-for-service structure in which the vendor provides and maintains both the platform and all instrumentation, including calibration, data QC, and data distribution. A major advantage of this model, as noted above, is that infrastructure costs are provided through investment by private industry. From a data quality point of view, this model has worked well for the individual regionally focused projects to date since the end users have been intimately involved. When considering extension to a global network with open data access, a natural concern is how to establish community confidence in the data quality.
This observing platform model will only succeed if confidence and trust are established in the quality and veracity of the data. To establish such community data confidence, the private industry and the science community should jointly develop data quality verification processes that are transparent and standardized. We recommend the formation of a stakeholder group, derived from the meteorological and oceanographic communities, to provide an international framework for developing USV observation guidelines and standards. This could be a working group of the DBCP, a joint body of the WMO and IOC. Focus areas should include existing best practices and standards for sensor integration, validation, QC, and data delivery. Guidance documents from this group should be reviewed by subject matter experts to establish best practices documents.
The QC processes for USV data should take into account the unique characteristics of each platform. For example, acoustic backscatter measurements are affected by platform orientation. Community-wide distributed QC as a Service (QCaaS) capability should be established, where centers of excellence address the QC requirements for targeted parameters. Collectively, a wealth of QC capability could be leveraged to benefit a much broader community.
Discovery and Access
Observational data should be made available in an easily digestible format within minutes of the observation to fully utilize a USV’s capability to respond to changing conditions in real time. Interoperable access would allow the users to access the data in a format that best suits their needs. Focus should be on a services-based approach that will empower communities to establish data portals that cater to their requirements.
Observational data should be discoverable and formatted for assimilation by modeling centers. This would require cooperation and would advance the scientific objectives of both observationalists and modelers. Information on data access points should be published at international-level discovery portals provided by the IOC and WMO. Data discovery functionality should be independent of the data hosting platform. New data discovery technologies and capabilities are becoming available, and leveraging tools such as Google’s Dataset Search and the NOAA ERDDAP broker can assist the oceanographic community, and USV operators, to improve data discovery and data access services.
Roadmap
Vision Statement #1:
Continue fostering close collaboration between the private sector and the regional ocean-observing community to leverage strengths of each sector to incorporate USVs to fill regional observing and forecast gaps. Additional PPPs will form interdisciplinary teams that will define the focus, drive innovation, and result in rapid deployments to fill observing system gaps.
Recommendation #1:
Convene regional workshops that include the ocean-observing community and private sector engineers and scientists to establish regional teams that focus on all aspects of observing from development to data delivery. To build community confidence, PPPs must have transparent processes for continued verification. An independent oversight of data quality is necessary for confidence in the business model for “Mission as Service.” Communicate early successes through public engagement and communication.
Key Results #1:
Convene five regional workshops by 2022; establish oversight and data quality criteria on at least 10 ECVs and EOVs delivered through “Mission as Service” from industry, and attract >$300 million in private capital to form PPPs to address regional overserving gaps.
Vision Statement #2:
Provide an international framework for coordinating and developing observation technologies from USVs within the meteorological and oceanographic communities.
Recommendation #2:
Form a USV working group of the long-established DBCP. Focus areas could include best practices and standards for sensor integration, verification and data delivery, and guidance for unmanned vehicle data providers.
Key Results #2:
Expand the terms of reference of the DBCP to include other met-ocean observation platforms and establish a USV task team by 2021.
Vision Statement 3:
Over the next decade, USVs will be incorporated into the ocean-observing system on a global scale for both the research and operational forecast community, providing new insight into phenomena at finer spatial and temporal scales than currently possible.
Recommendation 3:
We recommend that an international workshop be convened to establish community consensus on the needs and priorities for USVs in the global ocean-observing network and their contributions to forecast models. Important questions include the appropriateness of existing USVs for ocean-observing, including their complexity and cost-effectiveness and how to best ingest data from these moving platforms. Various models for PPPs should be explored. The workshop should address the issues of sensor and data format standards as well as an open access data distribution and access infrastructure. Calibration and validation methodologies should be developed to ensure community confidence in the data quality.
Key Results #3:
Convene three international workshops by 2025; establish oversight and data quality criteria; determine model forecast value on at least five ECVs and EOVs in coordination with GCOS and GOOS; and attract >$1billion in private capital in additional USV PPP’s.
Conclusion
As new technologies continue to rapidly increase observing assets and expand the international ocean-observing network, protecting the integrity of the global array is essential. In our view, the most efficient way to ensure rapid development that meets global standards is by encouraging close collaborations between the ocean-observing community and private developers. Private capacity can streamline capitalization and hiring, allowing development to scale up rapidly. Public involvement in private development can ensure that data quality, delivery, and access meet important community benchmarks and target key parts of the global observing array. According to our experience working together, NOAA and Saildrone suggest that the success of these relationships is based on close alignment of long-term goals that focus on practical, short-term deliverables. However, integrating workflows alone is insufficient: an essential element for achieving these successes is harmonizing group cultures. Good collaborations are based on relationships where both sectors understand and value their respective strengths and share goals that easily dovetail. By describing this case study in close detail here, we hope to provide the international community with a blueprint for collaborating successfully with private industry partners.
We see the potential for the rapid expansion of private participation in regional and global ocean observing, particularly with USVs. While this will be dependent, in part, on individual teams working together, it is our view that the future of the industry could greatly benefit from a coordinating infrastructure on the international scale. This body could help provide oversight of this community by determining best practices and transparent data quality standards for using USVs and other new platforms for ocean observing. This would provide crucial guidance to private participants and build community confidence in these new assets. This body also has a role to play in driving the community forward by bringing the regional ocean-observing community together with operational forecasters to determine observing requirements, assessing the appropriateness of existing USVs, sensors, sampling schemes, QC, and assurance and data format standards for achieving these needs, and establish usage and data distribution protocols for data generated by USVs at these scales. Including private partners in this body will also help them efficiently identify and target community demand for new assets and development.
In this case study, we suggest one vision that would leverage private industry investment in the development of a variety of USVs coordinated in regional fleets, adapted to specific observing requirements, and targeted to fill regional observing gaps. We are confident that the international community will be able to use the information we have provided here to suggest a variety of other operating modes. We look forward to avid discussion with our colleagues and partners about the far-reaching opportunities that PPPs provide for the global ocean-observing community.
Author Contributions
CM provided the overall guidance and executive editing for the manuscript, and wrote the sections “Introduction,” “Public Infrastructure,” “Private Capacity,” “Forming the PPP,” and “Roadmap.” All authors provided text input and editing. RJ, SdH, and NC provided Figures 1, 2; EFB, Figures 3, 6; CM, Figure 4; and EDC, Figure 5.
Funding
All authors gratefully acknowledge the support of their home institutions. CM, EFB, EDC, MFC, JNC, NL-S, AJS, and CZ are supported by PMEL and NOAA’s Office of Oceanic and Atmospheric Research. ATJ was supported by APL-UW. CWM and DZ were partially funded by the Joint Institute for the Study of the Atmosphere and Ocean (JISAO) under NOAA Cooperative Agreement NA15OAR4320063.
Conflict of Interest Statement
NOAA-PMEL and Saildrone have a CRADA since 2014 that covers joint development work and in-kind contributions from Saildrone, in the form of USV testing days. PMEL researchers (CM, EFB, EDC, MFC, JNC, NL-S, AJS, and CZ) have benefitted from additional vehicle days-at-sea, and Saildrone (RJ, SdH, and NC) has benefitted from the sensor development and data validation. University of Washington JISAO researchers (CWM and DZ) have benefitted from the additional data collected during Arctic and Tropical Pacific missions. ATJ has no formal relationship with either NOAA-PMEL or Saildrone.
Acknowledgments
The success of this PMEL-Saildrone PPP case study involved a large team of dedicated technicians, engineers, and scientists including R. Bott, C. Berchok, M. Casari, J. Crance, A. DeRobertis, J. Keene, C. Kuhn, S. Maenner, H. Tabisola, K. O’Brien, D. Peacock, J. Shanley, T. Walton, I. Wangen, and C. Wilson. This study is a PMEL contribution 4898 and JISAO contribution 2018-0180.
Footnotes
- ^ In this publication, “Saildrone” refers to the company name, and “Saildrone USV” refers to company’s unmanned surface vehicle.
- ^ https://www.unidata.ucar.edu/software/netcdf/
- ^ http://www.opengts.org/
- ^ A Guide to the Code Form FM-94 BUFR (see https://www.wmo.int/pages/prog/www/WDM/Guides/Guide-binary-1A.html).
- ^ https://coastwatch.pfeg.noaa.gov/erddapinfo/index.html.
References
Bernard, E., and Meinig, C. (2011). “History and future of deep-ocean tsunami measurements”. in Proceedings of Oceans ‘11 MTS/IEEE, Kona, (Piscataway, NJ: IEEE).
Buck, J. J. H., Bainbridge, S. J., Burger, E. F., Kraberg, A. C., Casari, M., Casey, K. S., et al. (2019). Ocean data product integration through innovation—the next level of data interoperability. Front. Mar. Sci. 6:32 doi: 10.3389/fmars.2019.00032.
Cokelet, E. D., Jenkins, R., Meinig, C., Lawrence-Slavas, N., Mordy, C. W., Stabeno, P. J., et al. (2015). “The use of saildrones to examine spring conditions in the bering sea: instrument comparisons, sea ice meltwater and yukon river plume studies. in Proceedings of the Oceans’15 MTS/IEEE, Marine Technology Society and Institute of Electrical and Electronics Engineers, Washington, DC, 19–22.
Cravatte, S., Kessler, W. S., Smith, N., Wijffels, S., Ando, K., Cronin, M., et al. (2016). First Report of TPOS 2020. GOOS-215. Available at: http://TPOS2020.org/first-report/ (accessed April 15, 2017).
Cronin, M. F., Gentemann, C. L., Edson, J., Ueki, I., Bourassa, M., Brown, S., et al. (2019). Air–sea fluxes with a focus on heat and momentum. OceanObs’19. Front. Mar. Sci 6:430, doi: 10.3389/fmars.2019.00430
De Robertis, A., Lawrence-Slavas, N., Jenkins, R., Wangen, I., Mordy, C. W., Meinig, C., et al. (2019). Long-term measurements of fish backscatter from Saildrone unmanned surface vehicles and comparison with observations from a noise-reduced research vessel. ICES J. Mar. Sci. fsz124, doi: 10.1093/icesjms/fsz124
Ghani, M. H., Hole, L. R., Fer, I., Kourafalou, V. H., Wienders, N., Kang, H., et al. (2014). The SailBuoy remotely controlled unmanned vessel: measurements of near surface temperature, salinity and oxygen concentration in the Northern gulf of mexico. Methods Oceanogr. 10, 104–121. doi: 10.1016/j.mio.2014.08.001
Hine, R., Willcox, S., Hine, G., and Richardson, T. (2009). “The Wave glider: a wave-powered autonomous marine vehicle”. in Proceedings of the OCEANS ‘09, (Biloxi, MS: IEEE). doi: 10.23919/OCEANS.2009.5422129
Johnston, P., and Poole, M. (2017). “Marine surveillance capabilities of the autonaut wave-propelled unmanned surface vessel (USV)”.in Proceedings of the OCEANS. (Aberdeen: IEEE). doi: 10.1109/OCEANSE.2017.8084782
Lauvset, S. K., Olsen, A., Gehlen, M., and Sommer, A. (2018). Surface Carbon EOV Report. AtlantOS Deliverable, D7.10. (Kiel: AtlantOS). doi: 10.3289/AtlantOS-D7.10
Lindstrom, E., Gunn, J., Fischer, A., McCurdy, A., and Glover, L. K. (2012). “A framework for ocean observing,” in By the Task Team for an Integrated Framework for Sustained Ocean Observing. (Paris: UNESCO).
McPhaden, M. J., Ando, K., Bourlès, B., Freitag, H. P., Lumpkin, R., Masumoto, Y., et al. (2010). The global tropical moored buoy array. in Proceedings of the “OceanObs’09: Sustained Ocean Observations and Information for Society Vol. 2. eds J. Hall, D. E., Harrison, and D. Stammer (Venice: ESA Publication).
Meinig, C., Jenkins, R., Lawrence-Slavas, N., and Tabisola, H. (2015). “The use of Saildrones to examine spring conditions in the Bering Sea: vehicle specification and mission performance,” in Oceans’15 MTS/IEEE, Marine Technology Society and Institute of Electrical and Electronics Engineers (Washington, DC).
Mordy, C. W., Cokelet, E. D., DeRobertis, A., Jenkins, R., Kuhn, C. E., Lawrence-Slavas, N., et al. (2017). Advances in ecosystem research: saildrone surveys of oceanography, fish, and marine mammals in the Bering Sea. Oceanography 30, 113–115. doi: 10.5670/oceanog.2017.230
Pearlman, J., Bushnell, M., Coppola, L., Karstensen, J., Buttigieg, P. L., Pearlman, F., et al. (2019). Evolving and sustaining ocean best practices and standards for the next decade. Front. Mar. Sci. 6:277. doi: 10.3389/fmars.2019.00277
Roemmich, D. (2012). “On the beginnings of Argo: ingredients of an ocean observing system”, in Proceedings of the 13th Meeting of the Argo Steering Committee, Paris.
Serra, Y. L., Haase, J. S., Adams, D. K., Fu, Q., Ackerman, T. P., Alexander, M. J., et al. (2018). The risks of contracting the acquisition and processing of the nation’s weather and climate data to the private sector. Bull. Am. Meteorol. Soc. 99, 869–870. doi: 10.1175/BAMS-D-18-0034.1
Smith, N., Kessler, W. S., Cravatte, S., Sprintall, J., Wijffels, S., Cronin, M. F., et al. (2019). Tropical Pacific observing system. Front. Mar. Sci. 6:31. doi: 10.3389/fmars.2019.00031
Sutton, A. J., Sabine, C. L., Maenner-Jones, S., Lawrence-Slavas, N., Meinig, C., Feely, R. A., et al. (2014). A high-frequency atmospheric and seawater pCO2 data set from 14 open ocean sites using a moored autonomous system. Earth Syst. Sci. Data 6, 353–366. doi: 10.5194/essd-6-353-2014
Vance, T. C., Wengren, M., Burger, E. F., Hernandez, D., Kearns, T., Merati, N., et al. (2019). From the oceans to the cloud: opportunities and challenges for data, models, computation and workflows. Front. Mar. Sci. 6:211. doi: 10.3389/fmars.2019.00211
Wanninkhof, R., Pickers, P. A., Omar, A. M., Sutton, A., Murata, A., Olsen, A., et al. (2019). A surface ocean CO2 reference network, SOCONET and associated marine boundary layer CO2 measurements. Front. Mar. Sci. 6:400. doi: 10.3389/fmars.2019.00400
Willis, Z., Swaykos, J., and Paternostro, C. (2015). Manual for the Real Time Quality Control of In-Situ Current Observations. (Silver Spring, MD: IOOS).
Keywords: unmanned surface vehicle, Saildrone, National Oceanic and Atmospheric Administration, Pacific Marine Environmental Laboratory, surface essential ocean variables, essential climate variables, ocean observations, public–private partnership
Citation: Meinig C, Burger EF, Cohen N, Cokelet ED, Cronin MF, Cross JN, de Halleux S, Jenkins R, Jessup AT, Mordy CW, Lawrence-Slavas N, Sutton AJ, Zhang D and Zhang C (2019) Public–Private Partnerships to Advance Regional Ocean-Observing Capabilities: A Saildrone and NOAA-PMEL Case Study and Future Considerations to Expand to Global Scale Observing. Front. Mar. Sci. 6:448. doi: 10.3389/fmars.2019.00448
Received: 30 November 2018; Accepted: 05 July 2019;
Published: 13 August 2019.
Edited by:
Fei Chai, State Oceanic Administration, ChinaReviewed by:
Wayne Homer Slade, Sequoia Scientific, United StatesIan Walsh, Sea-Bird Scientific, United States
Copyright © 2019 Meinig, Burger, Cohen, Cokelet, Cronin, Cross, de Halleux, Jenkins, Jessup, Mordy, Lawrence-Slavas, Sutton, Zhang and Zhang. This is an open-access article distributed under the terms of the Creative Commons Attribution License (CC BY). The use, distribution or reproduction in other forums is permitted, provided the original author(s) and the copyright owner(s) are credited and that the original publication in this journal is cited, in accordance with accepted academic practice. No use, distribution or reproduction is permitted which does not comply with these terms.
*Correspondence: Christian Meinig, Q2hyaXN0aWFuLk1laW5pZ0Bub2FhLmdvdg==