- 1Key Laboratory of Experimental Marine Biology, Center for Ocean Mega-Science, Institute of Oceanology, Chinese Academy of Sciences, Qingdao, China
- 2Laboratory for Marine Biology and Biotechnology, Pilot National Laboratory for Marine Science and Technology (Qingdao), Qingdao, China
- 3Marine Science and Engineer College, Qingdao Agricultural University, Qingdao, China
- 4Shenghang Aquatic Science and Technology Co., Ltd., Weihai, China
Artificial tetraploid induction is one of the important techniques of fish chromosome manipulation, and it is the first step for triploid breeding. There are a few reports to artificial induction of tetraploid in marine fish. The induction and survival rates were usually low. We firstly optimized the tetraploid induction conditions in turbot Scophthalmus maximus, one of the most important maricultural fish in China and Europe. For the initiate time of treatment, which is the most important factor in tetraploid induction, the first cleavage index (FCI) was used to reduce the influences of genetic origin and environment factors. Overall, the optimal initiation time for pressure shock was 15 min before the first cleavage at 14.8–15.5°C. The optimal treatment pressure and treatment duration were 67.5 MPa and 6 min. The regression equation prediction model was: The optimal initiation time = 0.982 FCI − 12.182 or the optimal initiation time = 0.85 FCI. Then two tetraploid induction (4n1 and 4n2) populations were obtained under the optimal conditions with diploid controls (2n1 and 2n2). The induction rates in tetraploid induction (4n) populations at hatched larvae stage could reach 100%. The genetic structure of these two 4n populations was also studied. Two to four alleles in each locus were detected in diploid (2n) and 4n populations, respectively. Private alleles were only appeared at locus Sma-USC21, with two alleles lost in 4n populations. Eleven and fourteen loci in 2n and 4n populations respectively showed a negative genetic deviation index. 3D-FCA analysis showed that the two 2n and two 4n populations have obvious differences. The numbers of locus deviating from Hardy–Weinberg equilibrium in 2n1, 4n1, 2n2, and 4n2 populations were 6, 9, 12, and 7, respectively. Overall, 12 loci in either 2n or 4n population deviated from Hardy–Weinberg equilibrium. Tetraploid induction population showed lower heterozygosity and higher heterozygote deletion.
Introduction
Polyploidization results in a wide variety of beneficial effects for genetic breeding. The most common chromosome manipulation in fish is to use a physical shock, usually a pressure or heat shock, to induce retention of the second polar body during meiosis, resulting in triploid fish. An alternative method for producing triploids is to first make tetraploids by suppression of early cell division in the zygote (Chourrout, 1984; Zhang and Onozato, 2004; Zhu et al., 2017) and then mate the fertile tetraploids with normal diploids to generate triploid offspring. The tetraploid-derived triploids are not exposed to the trauma of the induction shock and show higher survival rate than induced triploids. Generation of tetraploid-derived triploids has been achieved in rainbow trout (Oncorhynchus mykiss) (Chourrout et al., 1986; Blanc et al., 1993). However, tetraploid-derived triploid was unavailable in marine fish until now, due to the low induction rate of tetraploid.
Research on chromosome set doubling has been going on since the first part of the last century. Tetraploid has been induced in many finfish species, including rainbow trout (Thorgaard et al., 1981; Chourrout et al., 1986), channel catfish (Ictalurus punctatus) (Bidwell et al., 1985), tilapia (Oreochromis aureus) (Don and Avtalion, 1988) and silver crucian carp (Carassius auratus) (Gui et al., 1993). In marine fishes, induction of tetraploid has been reported in European sea bass (Dicentrarchus labrax) (Peruzzi and Chatain, 2003), yellow perch (Perca flavescens) (Malison et al., 1993), olive flounder (Paralichthys olivaceus) (Yi et al., 2012), and half-smooth tongue sole (Cynoglossus semilaevis) (Li et al., 2012). However, the optimal values of treatment parameters, such as initiate treatment time, treatment intensity (temperature or pressure) and treatment duration, differ greatly among species. We also studied the artificial induction of tetraploid turbot (Scophthalmus maximus) (Wu et al., 2014), but the result was not stable and the induction rate was low. The viability of tetraploids was low in most instances and even unviable in several cases (Rothbard et al., 1997). The low yield of tetraploids, high frequency of abnormality and mosaicism, and especially the relatively low fertility of tetraploid females have prevented the establishment of tetraploid broodstock (Myers and Hershberger, 1991). Some possible mechanisms for the occurrence of mosaicism have been proposed by several investigators. Mosaics may be produced from tetraploid by conversion of some of tetraploid to diploid cells resulting from tetrapolar division (Yamaki et al., 1999) or somatic meiosis (Zhang and Onozato, 2004), or may be generated from the start by the simultaneous appearance of both types of ploidy cells after treatment. Maybe it is a reason to lead to the ploidy instability of artificial induced tetraploid. So, most reports on the production of tetraploid finfish have based their claims on analyses of early embryos or fry, and successful examples have been reported in a limited number of freshwater fish as mentioned above.
Induction of tetraploid requires the precise timing of the application of the shock treatment, usually a pressure shock. In salmonid, the embryo development lasts a long period. The initiation time of tetraploid induction is based on a percentage of the first cleavage interval (FCI), which is the time span between insemination and first cleavage of the zygote (Chourrout, 1984; Myers et al., 1986). The initiation time was also expressed as the relative dimensionless unit τ0, which is equivalent to the duration of one mitotic cycle during synchronous cell divisions in the initial stages of embryogenesis (Cherfas et al., 1993). By comparison, in turbot and most marine fish, the embryo development was relatively short. It seems that the optimal initiation time is easier to ascertain. The initiation time of tetraploid was mostly calculated by minutes after fertilization or minutes before the first cleavage. However, the precise timing of the shock treatment was hard to get due to the different embryo development speed caused by different genetic origin and breeding temperature.
Tetraploid usually shows higher heterozygosity because it has four sets of chromosome. It could help to produce relatively large fish (Chourrout et al., 1986). The tetraploid also plays an important role in evolution due to its high heterozygosity (Amores et al., 2004; Pasquier et al., 2017). In artificial induced tetraploid, all the chromosome sets come from the diploid parents. The genetic diversity in tetraploid should be equal to the diploid full sib family in theory. Or the genetic diversity should be even lower in tetraploid because of the loss of some genetic information in induction process. However, how the genetic diversity varied in artificial induced tetraploid is still unknown.
In this study, one of our goals was to determine the most effective and repeatable timing of hydrostatic pressure shock for suppression of the mitotic cleavage of the embryo in turbot, an important maricultured fish in China and Europe. Subsequently, tetraploid turbot with high tetraploid rates were produced. Finally, the genetic structure of tetraploid populations was also studied.
Materials and Methods
Brood Stock Management and Gamete Collection
The turbot broodstocks used in the present study were the cultured stocks introduced from Europe. Turbot broodstocks were cultured in the fish farm of Shenghang Aquatic Science and Technology Co., Ltd., Weihai, China under controlled conditions (photoperiod 16 h light: 8 h dark; temperature, 14 ± 1°C). The eggs were stripped from each female turbot and stored at wet box under dark condition. The eggs were divided into small subsamples before fertilization so that each parameter could be tested with one batch (∼5,000 eggs per batch). Semen was drawn from mature male by the application of gentle pressure to their abdomens and was transferred into 5 mL EP tubes to be stored on ice until required. Semen contaminated with water or urine was discarded.
Artificial Fertilization
Before artificial fertilization, the quality of sperms and eggs were checked under microscope. The sperm motility was Grades IV–V (Valdebenito et al., 2013). The unfertilized eggs were buoyant, transparent, spherical in shape, and 1 mm in average diameter with a single oil globule. The gametes below standards were discarded. The artificial fertilization procedure was as follows: 0.5 mL sperm was activated using 15–20 mL filtered seawater at 15°C and then was added to plastic beakers each containing 5,000 eggs. After a further 30 s of gentle agitation, 50–100 mL filtered seawater was added and the eggs were left undisturbed.
Tetraploid Induction
The appropriate conditions for the production of tetraploid turbot were examined by varying the moment of hydrostatic pressure shock after fertilization and by altering the pressure and durations of hydrostatic pressure shock. Hydrostatic pressure induction was carried out in manual hydrostatic pressure chamber. There were three experiments, including five groups in each experiment: (1) to determine the optimal moment of shock induction with a single treatment of pressure shock at 65 MPa for 6 min at 5, 10, 15, 20, or 25 min before the appearance of the cleavage furrow; (2) to determine the appropriate pressure intensity of shock with a single treatment of pressure shock at 15 min before the appearance of the cleavage furrow for 6 min at 55, 60, 65, 70, or 75 MPa; (3) to determine the appropriate pressure duration of shock with a single treatment of pressure shock at 15 min before the appearance of the cleavage furrow at 65 MPa for 4, 5, 6, 7, or 8 min. The preset pressure was achieved in 15 s, and recovered to normal pressure in 30 s after shock. Temperature was constantly monitored throughout the experimentation. After treatments, shocked eggs were acclimated to 15 ± 0.2°C water for incubation. All experiments were replicated up to five times using egg batches derived from different five males and females, respectively. Eggs without hydrostatic pressure shock were used as diploid control.
Fertilization rate in each group was determined at blastocyst stage by examining ∼200 floating eggs. Hatching rate was assessed at 6 h after hatching. Induction rate was the percentage of tetraploid larvae in hatched larvae.
The FCI and mitotic interval (τ0) were calculated in diploid controls. The FCI was defined as the time at which more than 80% of the zygotes had reached first cleavage, and was calculated according to Hershberger and Hostuttler (2005). The τ0 was also calculated according to Shelton et al. (1997). The FCI and τ0 were calculated and recorded in each group of each experiment.
Assessment of Ploidy Level
The ploidy of control and treatment groups was determined by cellular DNA content and chromosome preparation. Flow cytometric analysis was performed to detect average cellular DNA contents of larvae in hatched control and treated groups with a PARTEC cell counter analyzer CCA-II (PARTEC, Germany), and 30 hatched larvae were sampled and prepared each group (You et al., 2001; Luckenbach et al., 2004). Diploid larvae were used as a diploid standard for the calibration of the cytometer. Chromosomal metaphases were prepared from embryos at gastrula stage by regular air-dried method after colchicine and hypotonic pretreatments. Slides were stained in 15% Giemsa for 15 min and observed under the light microscope after drying (You et al., 1991).
Induction of Tetraploid Populations and Embryo Development Observation
According to the results of induction conditions of tetraploid turbot, we carried out a massive tetraploid induction of turbot. About 200 mL eggs acquired from three to four female turbot were fertilized with 5 mL sperms from three to four male ones, and then shocked under the optimal parameters. Then eggs were incubated in net cages under 15 ± 0.2°C. The hatched larvae were reared in indoor tanks with flow-through sea water at a temperature of 18–21°C and fed with rotifer, artemia, and commercial dry feed (salinity 28–30, pH 7.8–8.2, dissolved oxygen > 6 mg/L, water exchange rate was 50% for fry, 100% for larvae, and >800% for juveniles). Two tetraploid induction (4n1 and 4n2) populations with different parental origin were induced, respectively. The control diploid (2n1 and 2n2) populations were also conducted.
The embryo development was observed under stereoscope. The morphological character and embryonic development time were calculated in each tetraploid induction (4n) and diploid control (2n) population.
Genetic Structure Analysis
The genetic diversity of 4n induction populations was measured using microsatellite markers. More than 50 new hatched larvae in each 2n or 4n population were sampled and stored in 100% ethanol under −20°C until DNA extraction. Total genomic DNA was extracted using the rapid salt-extraction of genomic DNA according to Aljanabi and Martinez (1997). Extracted DNA was checked using 0.8% agarose gel electrophoresis and then stored at −20°C for PCR amplification.
Twenty out of fifty-five previously published microsatellite loci were screened to be analyzed (Table 1, Iyengar et al., 2000; Castro et al., 2003; Chen et al., 2007; Pardo et al., 2010). Primers were synthesized by Sangon Biological Engineering Technology & Services Co., Ltd. (Shanghai, China). PCR was conducted in a volume of 25 μL containing 100 ng of template DNA, 0.4 μmol/L of each primer, 1.5 mmol/L of MgCl2, 100 μmol/L of dNTPs, 1 U of Taq polymerase, and 1 × PCR buffer (Promaga). Reactions were processed as follows: an initial denaturation step of 5 min at 94°C, followed by 1 min at 94°C, 30 s at annealing temperature and 45 s at 72°C for 35 cycles with a final 5 min extension at 72°C. Amplification products (5 μL load) were separated by electrophoresis through a 12% non-denaturing polyacrylamide gel in 1 × TBE buffer. Detection of microsatellite alleles was achieved by silver staining method. DNA fragments were visualized with a modified silver stain method described by Xu et al. (2002). Alleles were designated according to the PCR product size relative to a molecular size marker (M1041 50–500 bp, Dongsheng Biotech, China) in combination with Quantity One software (Bio-Rad). More than 2% PCR products with representative alleles in each population were screened out, and were blindly scored after electrophoresis on the same plate to reduce genotyping errors.
The genetic diversities of the two 4n induction populations were calculated separately and integrally, as well as the two 2n populations. Measurements of genetic diversity were conducted within population: standard genetic diversity parameters, including the number of alleles (A), effective alleles (Ae), observed heterozygosity (Ho), and expected heterozygosity (He), were calculated using GENALEX v. 6. Deviations from Linkage and Hardy–Weinberg Equilibrium (HWE) for each microsatellite locus were tested using the package GENEPOP v. 4.0, and the significance was adjusted by applying the sequential Bonferroni correction. Linkage disequilibrium and HWE exact tests were tested using the Markov chain method (10,000 dememorization steps, 100 batches, 5,000 iterations). The Discriminant Analysis of Principal Components (DAPC) was used to cluster genotypes independently of a priori haplotype designation using the R package adegenet v. 1.4.2.
Statistical Analysis
Data from control diploid and tetraploid induction groups were analyzed with the SPSS package for Windows (Version 15.0, SPSS, Chicago, IL, United States). Data are shown as mean ± SD. And the regression analyses of optimal initiation time, τ0 and FCI in nine groups were conducted by linear regression analysis in the SPSS package. Distributions were examined for departures from normality by the Kolmogorov–Smirnov test and the homogeneity of variances was verified by the Levene’s test. Significant differences were determined using One-way ANOVA tests followed by Duncan’s multiple comparison tests at the probability level of 0.05.
Results
The Optimal Conditions of Pressure Shock Induction
The optimal conditions of pressure shock induction were evaluated according to the survival rate of new hatched larvae (hatching rate × tetraploid rate). Overall, the optimal initiation time for pressure shock was 15 min before the first cleavage at 14.8–15.5°C. The optimal treatment pressure and treatment duration were 67.5 MPa and 6 min (Figure 1). The initiation time for pressure shock was the most important factor for the survival of tetraploid induction. The peak of survival rate was obtained when the shock started around 15 min (12.5–17.5 min) before the appearance of the cleavage furrow, which was higher than those obtained from shocks applied at earlier or later time. The induction rate was determined by cellular DNA content of hatched larvae and was verified by chromosome preparation at gastrula stage (Figure 2). The induction rate ranged from 70 to 100% under the optimal condition.
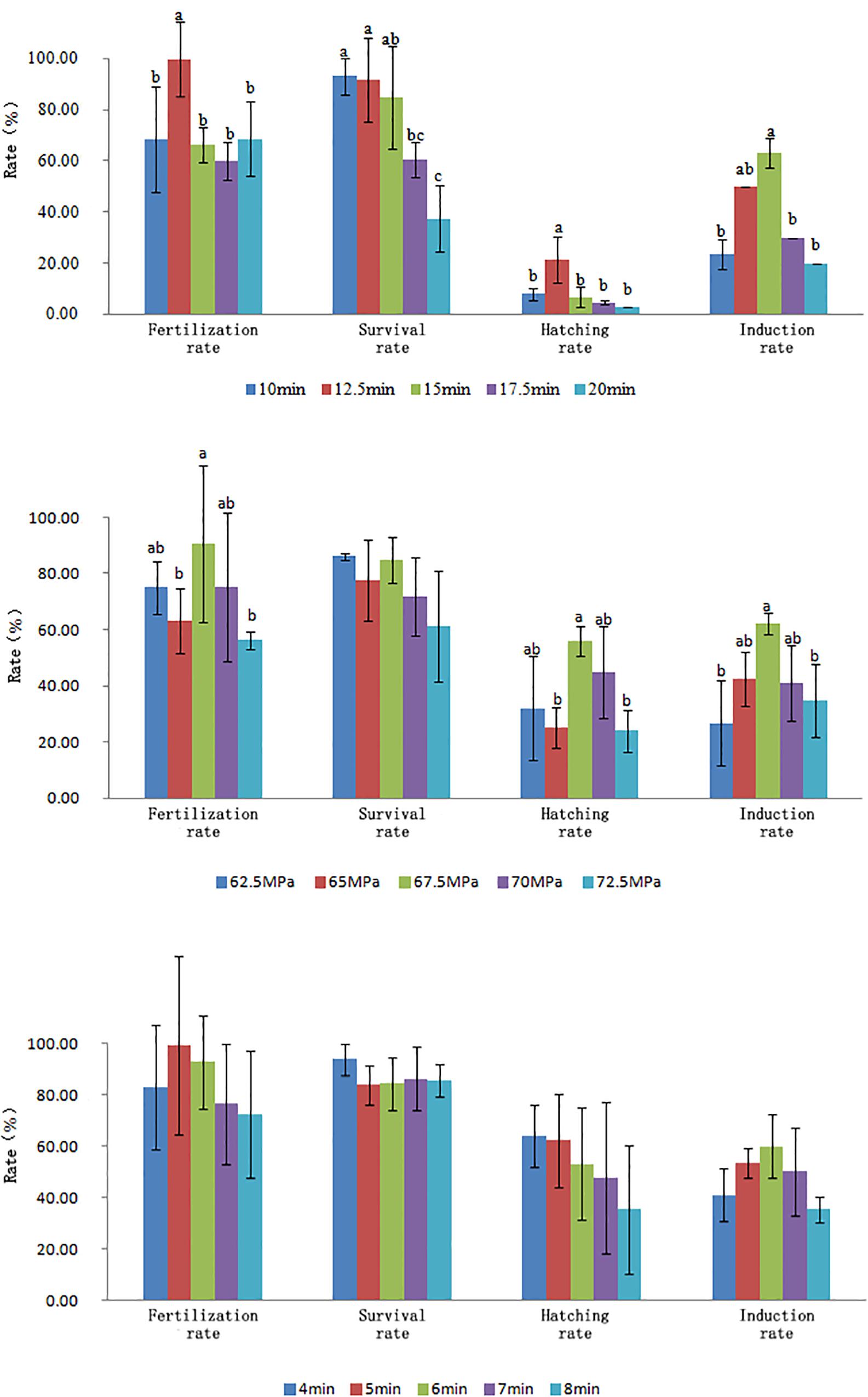
Figure 1. Effects of initiation treatment time, treatment pressure, and treatment duration on fertilization rate, survival rate at gastrula stage, hatching rate, and induction rate.
The Regression Analysis of Optimal Initiation Time, FCI, and τ0
The variation in FCI and τ0 among the populations tested in this study was fairly large (Table 2). On the other hand, the coefficient of variation within the groups was rather small, suggesting homogeneity among the eggs within each group.
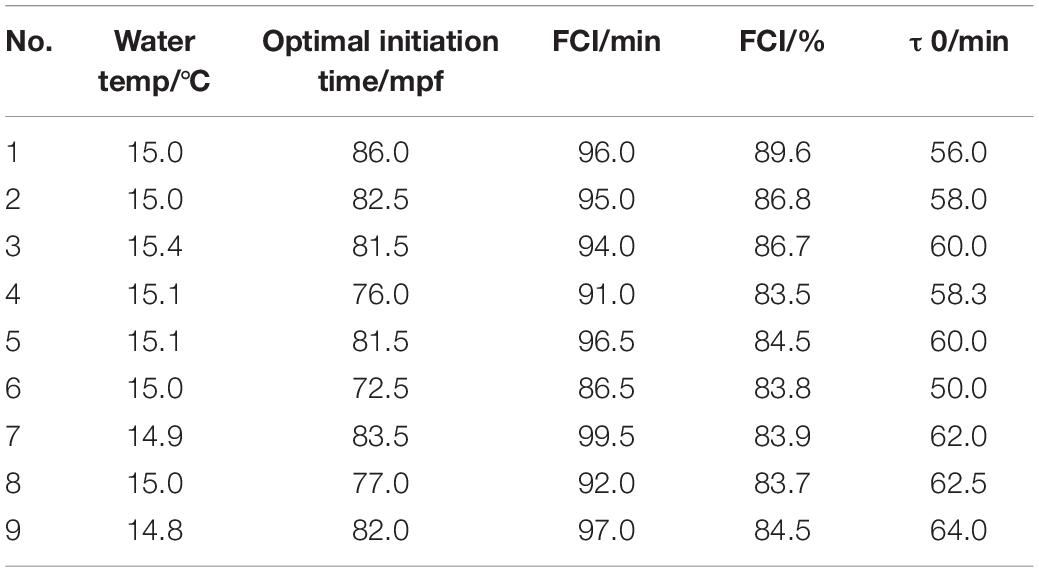
Table 2. The optimal initiation time, FCI, and τ0 in nine different tetraploid induction (4n) groups.
The FCI had extremely significant correlation with the optimal initiation time (P = 0.002). Meanwhile, there was no significant correlation between τ0 and the optimal initiation time (Table 3). Therefore, the factor τ0 could be excluded, and the regression analyses of optimal initiation time and FCI were conducted. The FCI had extremely significant correlation with the optimal initiation time (P = 0.001, Table 3). A regression equation prediction model was as below:
Or
Tetraploid Induction Population Establishment and Embryo Development Observation
According to the optimal induction conditions of tetraploid turbot and the prediction model of optimal initiation time described above, we carried out a massive tetraploid induction of turbot, and obtained two 4n induction populations. The ploidy levels in these two populations were tested by FCM at 1 dph. The 1 dph diploid larvae were also tested as control. The tetraploid rates in both 4n induction populations were 100%. The observation of embryo development showed that the 2n embryos took about 100 h to hatch under 15°C. Hydrostatic pressure shock induced higher deformity rate of embryos which could not survive to hatching stage. The rest embryos showed no difference in morphology with diploid embryo and larvae, and had a prolonged hatching period (110 h). About 8 g (7,200–8,800) embryos in each population were moved into a 1.5 m3 indoor tank before hatching. The hatching rates in 4n1 and 4n2 induction populations were 70 and 75%, respectively. The hatching rates in 2n1 and 2n2 populations were slightly higher (81 and 88%, respectively). In embryo development, two mortality peaks were observed at stages gastrula and hatching in 4n induction populations. Some embryos in 2n populations also died in these two stages, but the mortality rates were much lower (Figure 3).
Genetic Structure of Tetraploid Induction Populations
The population genetic analysis with 20 microsatellite markers showed that two to four alleles in each locus were respectively detected in 2n and 4n populations (Figure 4). Private alleles were only appeared at locus Sma-USC21, with low allele frequencies. Two alleles in locus Sma-USC21 lost in 4n induction populations. The genetic diversity indexes were shown in Table 4. Eleven and fourteen loci in 2n and 4n populations showed a negative genetic deviation index (D). The numbers of locus deviating from Hardy–Weinberg equilibrium in 2n1, 4n1, 2n2, and 4n2 populations were 6, 9, 12, and 7, respectively. Overall, 12 loci in 2n and 4n populations, respectively deviated from Hardy–Weinberg equilibrium (Table 5).
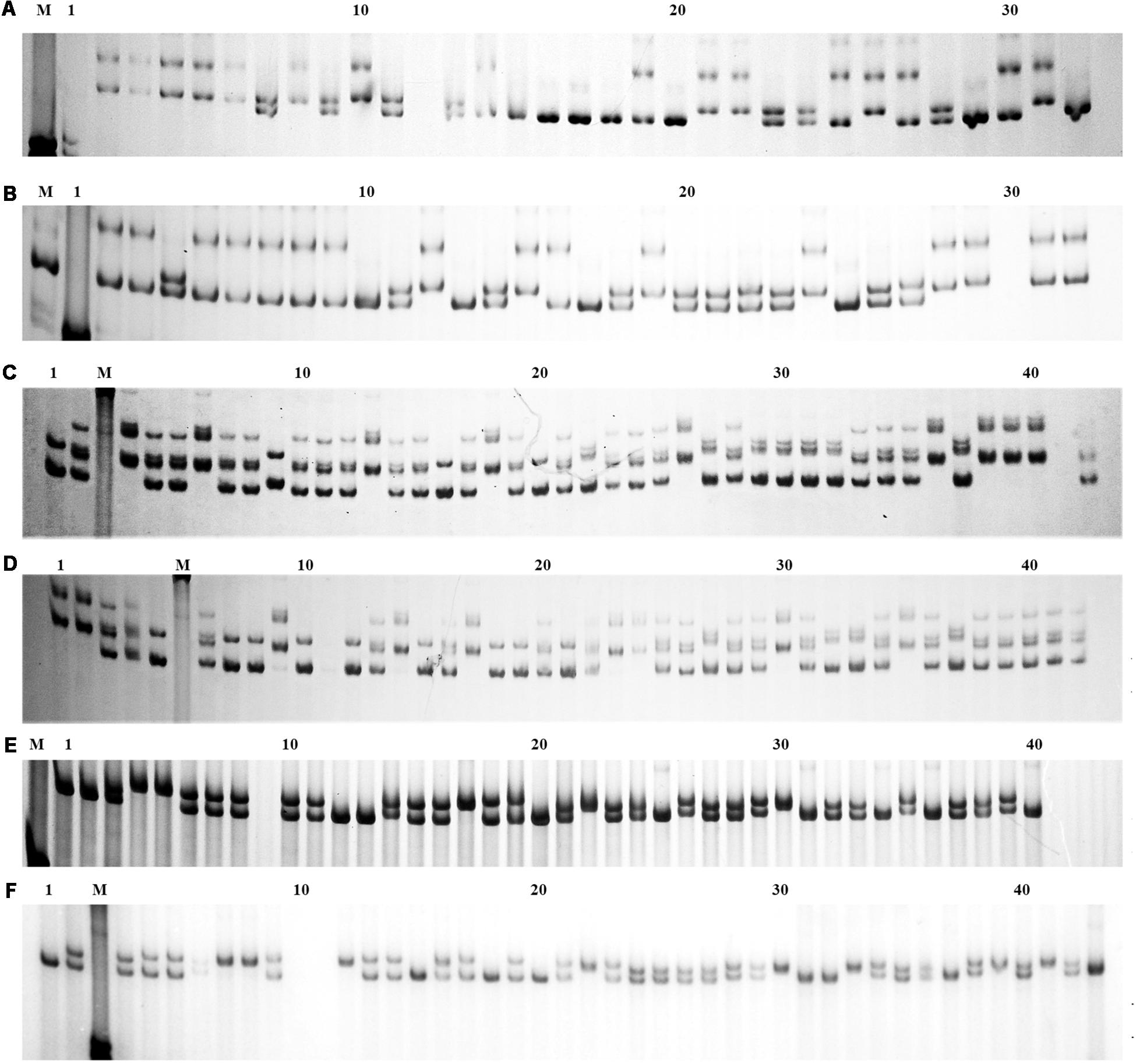
Figure 4. The electrophoresis patterns of some primers in 2n and 4n populations. M, DNA 2000 plus maker. (A) Locus Smac-05 in 2n populations; (B) Locus Smac-05 in 4n populations; (C) Locus Sma3-129INRA in 2n populations; (D) Locus Sma3-129INRA in 4n populations; (E) Locus Sma-USC23 in 2n populations; (F) Locus Sma-USC23 in 4n populations.
The scatter plots from the DAPC clearly showed four major clusters. In the 3D-FCA, the plots of 4n1 scatted more widely than those of 2n1 population, meanwhile plots of 4n2 showed narrower distribution range than those of 2n2 population (Figure 5).
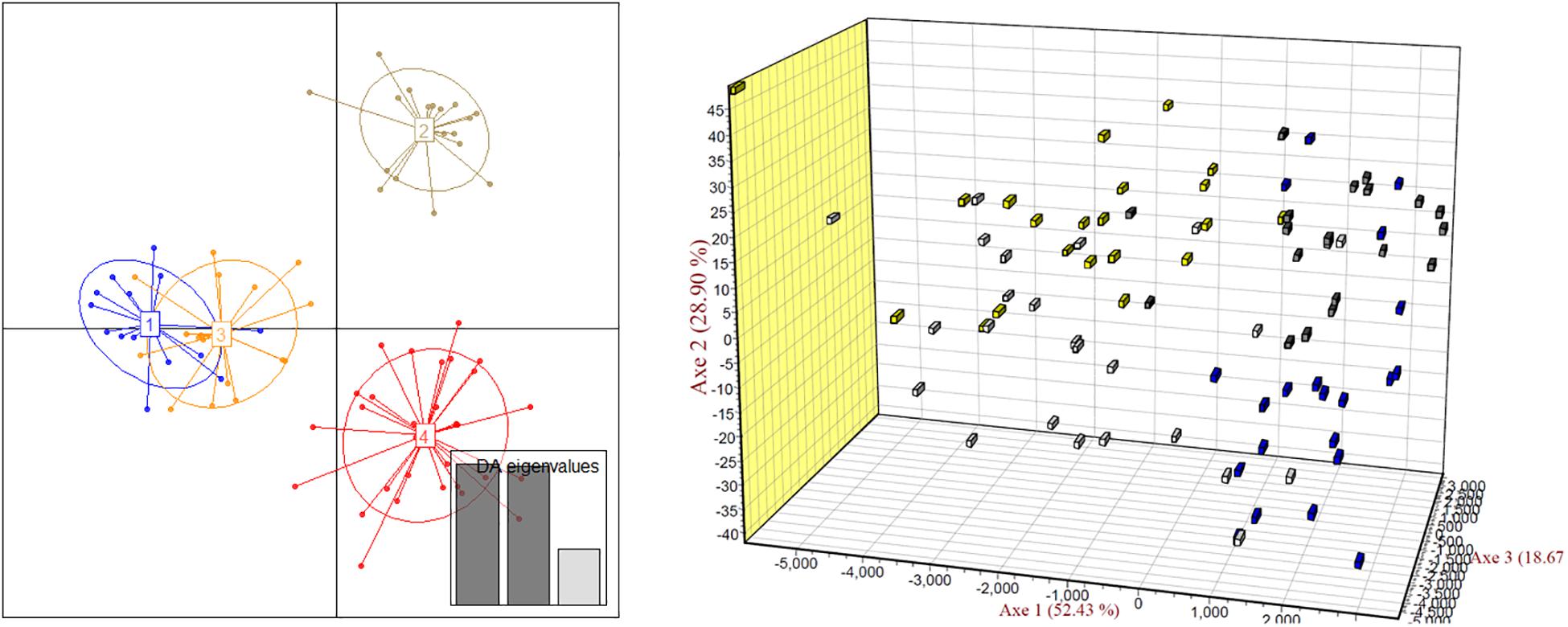
Figure 5. Discriminant analysis of principal components (DAPC, left) and three-dimensional factorial correspondence analysis (3D-FCA, right) showing the relationships among turbot individuals of two 2n and two 4n populations. Yellow block, 2n1; white block, 4n1; blue block, 2n2; gray block, 4n2.
Discussion
Tetraploid could benefit in triploid breeding, because it can avoid the inductive effect in artificial triploid induction. However, tetraploid-derived triploids were only acquired in a few fish, such as rainbow trout (Chourrout et al., 1986; Weber et al., 2014) and Atlantic salmon (Salmo salar) (Benfey, 2016). In some ancient tetraploid fresh water fish, triploids could also be acquired by distant hybridization, such as female Japanese crucian carp (Carassius cuvieri) and male blunt snout bream (Megalobrama amblycephala) (Hu et al., 2018), and female grass carp (Ctenopharyngodon idellus) and male topmouth culter (Erythroculter ilishaeformis) (Wu et al., 2019). In turbot and other marine fish, no report was found about the tetraploid-derived triploids. The main reason is the difficulty in acquirement of tetraploid parent fish because of the low survival and unstable ploidy of artificial induced tetraploid.
Hydrostatic treatment is the most frequently used method of tetraploid induction in marine fish. Usually, the effect of hydrostatic treatment is mainly affected by three factors: initiate treatment time, treatment pressure intensity and treatment duration (Peruzzi and Chatain, 2003). The optimal values of these parameters differ greatly among species. Especially the initiate treatment time could range from 6 to 70 min after fertilization (Peruzzi and Chatain, 2003; Li et al., 2012; Yi et al., 2012). Our results showed a longer optimal initiate treatment time (about 85 min after fertilization) in turbot, which may concern with the longer FCI. There were significant differences in fertilization rate, hatching rate and induction rate among induction groups at different initiate time and pressure (P < 0.05); but there were no significant differences at different treatment time, which indicated that the effect of treatment time on the experiment results was small, and the determination of appropriate treatment time and pressure were more important. Hydrostatic pressure doubles the chromosomes to induce tetraploid fish by inhibiting cleavage of fertilized eggs (Zhou and Gui, 2017).
Generally, before the first cleavage, the low synchronization of fertilized eggs, the complexity of cleavage regulation and environmental changes will affect the development of fertilized eggs, making it difficult to determine the optimal treatment time (Jeuthe et al., 2016). If treated in advance, the fertilized eggs will be shocked before they reach the expected level, which greatly reduces the induction efficiency. If the treatment is postponed, the fertilized eggs will begin to cleavage, which will affect their inhibition effect on cleavage, leading to the generation of aneuploidy and an increase in mortality (Sakao et al., 2006). In salmonids, early studies suggested that small changes in the moment of shock induction could have large effects on success of tetraploid induction (Chourrout, 1984; Chourrout et al., 1986; Zhang et al., 2005). Hershberger and Hostuttler (2007) found that applying pressure 15 min late could decrease the tetraploid induction rate from 100 to 0%. It was also found that the variation in embryo development significantly affected the tetraploid induction in terms of both tetraploid induction rate and viability of tetraploid progeny (Weber and Hostuttler, 2012). Thereafter, the FCI index was used to alleviate the effect of asynchronism in embryo development. And the induction success rate was increased (Weber and Hostuttler, 2012). The temperature-dependent measure of τ0 can also help to standardize chromosome manipulation in fish eggs (Shelton et al., 1997). It is the duration of one mitotic cycle during early embryonic cleavage stage when the cleavage is synchronous. τ0 is affected by temperatures, and is also a reliable indicator of developmental rates of egg incubation. So it can be used to estimate the optimal times for chromosome manipulation. Both the FCI and τ0 were widely used in Salmonids, paddlefish (Polyodon spathula) and shovelnose sturgeon (Scaphirhynchus platorynchus) (Shelton et al., 1997). It is mainly because the optimal moment of treatment in fish is long, and the embryo development is hard to find out. In flatfish, the embryo development was far shorter than that in Salmonids, so it was believed that the deviation from the actual initial time to the optimal initial time was small. The optimal initiate time of treatment was conducted as minutes after the fertilized time or before the appearance of the first cleavage furrow (Lin et al., 2016). In the present study, we found that FCI and τ0 varied in a fairly large range for the optimal initiation time for pressure shock (Table 2). These indexes could be affected by the water temperature and genetic background. Due to the buoyant trait of turbot eggs, they may also be affected by the air temperature. Besides, our previous study showed that about 5 min derived from the optimal moment of shock induction would sharply decrease the induction rate (Zhu et al., 2017). Therefore, the relatively short embryo development also shortens the time window of initiation time for success tetraploid induction. It needs to determine the initiation time more precisely. In order to determine the treatment time accurately and reduce the genetic and environment influences, the relation among optimal initiate time of treatment, FCI and τ0 was calculated. We found that the FCI is the best index to determine the optimal initiate time of treatment in turbot. In this way, the influences of genetic origin and environmental factors such as water temperature and air temperature can be reduced. Under the optimal conditions, even fully tetraploid population could be induced, which is far better than the original method.
In present study, we also tried to find out how the artificial tetraploid induction affected the genetic diversity in turbot. The genetic structure of natural or spontaneous tetraploid fish, such as Amazon molly (Poecilia formosa) (Lampert et al., 2008) and minnow (Squalius alburnoides) (Crespo-López et al., 2007), has been reported before. The number of alleles and heterozygosity were higher in tetraploid populations than those in diploid populations in these fishes due to their hybrid origin. However, the genetic structure of artificial induced tetraploids was different. Overall, the number of effective alleles in the 2n population of turbot was basically the same as that in the 4n induction population. The observed heterozygosity was slightly lower and the expected heterozygosity was slightly higher in 2n population than those in the 4n induction population. The average observed heterozygosity Ho of 2n and 4n populations were 0.553 and 0.569, respectively. The average expected heterozygosity He of 2n and 4n populations were 0.527 and 0.519, respectively. Yu et al. (2009) analyzed seven artificial breeding families of turbot, and the average heterozygosity was 0.7206. Gu et al. (2009) used self-developed microsatellite markers to analyze the expected heterozygosity of 31 turbot individuals ranging from 0.5061 to 0.8995. It was found that the heterozygosity of turbot populations in the present study were lower than those has been reported. The screened out microsatellite markers were reported to have more alleles in the literature, but the number of alleles in this study is only 2–4. Heterozygote deletion was also found at many loci. It indicates that inbreeding may occur in parent turbot breeding, and it is necessary to introduce parents from different sources to improve their genetic diversity. By comparing the allele frequencies of turbot, two alleles of Sma-USC21 were deleted in the 4n population, suggesting the existence of recessive lethal genes. Tetraploid induction population showed lower heterozygosity and higher heterozygote deletion. We also, respectively, analyzed the genetic diversity of two 4n populations, and the scatter plots from the DAPC clearly showed four major clusters. In the 3D-FCA, the plots of 4n1 population scatted more widely than those of 2n1 population, meanwhile plots of 4n2 population showed narrower distribution range than those of 2n2 population. Since the 4n population was obtained by inhibiting the cleavage doubling of the 2n population, its genetic diversity level should theoretically be the same as that in the 2n population (Ferris and Whitt, 1980). Besides, this induction process can be regarded as artificial selection of population. Some individuals with specific alleles or genotypes are sensitive to high-pressure induction and cannot survive, resulting in the deletion or frequency reduction of the allele. Such phenomena could also be found in artificial induced triploid (Liu et al., 2018). Therefore, it is easy to explain the decrease of genetic diversity in 4n2 population compared to that in 2n2 population. However, it is hard to explain the wider expand of scatter of 4n1 population than that of 2n1 population. The 4n1 and 4n2 induction populations came from different parents. Weather the genetic origin affected the genetic diversity in tetraploid induction is still unknown. Further analysis is needed in combination with genotypes.
In conclusion, the tetraploid induction rate of turbot can be increased to be more than 90% or even 100% under the optimized conditions by using FCI. The optimal initiation time is 0.982 FCI − 12.182 or the optimal initiation time is 0.85 FCI. And the optimal treatment pressure and treatment duration were 67.5 MPa and 6 min. Then two tetraploid induction populations were obtained under the optimal conditions. They showed lower heterozygosity and higher heterozygote deletion. Besides, it seems that the hydrostatic pressure has different effects on turbot with different genetic origin. The exact mechanism still needs further study. The results would help in tetraploid induction and relatively studies in marine fish.
Data Availability Statement
All datasets generated for this study are included in the manuscript/supplementary files.
Ethics Statement
The animal study was reviewed and approved by Institutional Animal Care and Use Committee of the Institute of Oceanology, Chinese Academy of Sciences.
Author Contributions
ZW and FY designed the study and wrote the manuscript. ZW, LW, YL, XZ, and XY conducted the field work. ZW generated the data. LW performed the data analyses. All authors read, commented, and agreed on the manuscript.
Funding
This work was supported by the National Natural Science Foundation of China (Nos. 31502156 and 41276171), the Key Research and Development Plan of Shandong Province (No. 2017CXGC0101), and the National Key R&D Program of China (No. 2018YFD0901202).
Conflict of Interest
XY was employed by Shenghang Aquatic Science and Technology Co., Ltd., Weihai, China.
The remaining authors declare that the research was conducted in the absence of any commercial or financial relationships that could be construed as a potential conflict of interest.
Acknowledgments
We are grateful to Mr. Yan Li for his help in the laboratory.
References
Aljanabi, S., and Martinez, I. (1997). Universal and rapid salt-extraction of high quality gnomic DNA for PCR-based techniques. Nucleic Acids Res. 25, 4692–4693. doi: 10.1093/nar/25.22.4692
Amores, A., Suzuki, T., Yan, Y. L., Pomeroy, J., Singer, A., Amemiya, C., et al. (2004). Developmental roles of pufferfish hox clusters and genome evolution in ray-fin fish. Genome Res. 14, 1–10. doi: 10.1101/gr.1717804
Benfey, T. J. (2016). Effectiveness of triploidy as a management tool for reproductive containment of farmed fish: atlantic salmon (Salmo salar) as a case study. Aquaculture 8, 264–282. doi: 10.1111/raq.12092
Bidwell, C. A., Chrisman, C. L., and Libey, G. S. (1985). Polyploidy induced by heat-shock in channel catfish. Aquaculture 51, 25–32. doi: 10.1016/0044-8486(85)90237-6
Blanc, J. M., Poisson, H., Escaffre, A. M., Aguirre, P., and Vallee, F. (1993). Inheritance of fertilizing ability in male tetraploid rainbow-trout (Oncorhynchus mykiss). Aquaculture 110, 61–70. doi: 10.1016/0044-8486(93)90434-Z
Castro, J., Bouza, C., Sánchez, L., Cal, R. M., Piferrer, F., and Martínez, P. (2003). Gynogenesis assessment using microsatellite genetic markers in turbot (Scophthalmus maximus). Mar. Biotechnol. 5, 584–592. doi: 10.1007/s10126-003-0004-x
Chen, S. L., Ma, H. Y., Jiang, Y., Liao, X. L., and Meng, L. (2007). Isolation and characterization of polymorphic microsatellite loci from an EST library of turbot (Scophthalmus maximus) and cross-species amplification. Mol. Ecol. Resour. 5, 215–217. doi: 10.1111/j.1471-8286.2007.01725.x
Cherfas, N. B., Gomelsky, B., Peretz, Y., Bendom, N., Hulata, G., and Moav, B. (1993). Induced gynogenesis and polyploidy in the Israeli common carp line Dor-70. Isr. J. Aquac. 45, 59–72.
Chourrout, D. (1984). Pressure-induced retention of second polar body and suppression of first cleavage in rainbow trout: production of all-triploids, all-tetraploids, and heterozygous and homozygous diploid gynogenetics. Aquaculture 36, 111–126. doi: 10.1016/0044-8486(84)90058-9
Chourrout, D., Chevassus, B., Krieg, F., Happe, A., Burger, G., and Renard, P. (1986). Production of second generation triploid and tetraploid rainbow trout by mating tetraploid males and diploid females - Potential of tetraploid fish. Theor. Appl. Genet. 72, 193–206. doi: 10.1007/Bf00266992
Crespo-López, M. E., Pala, I., Duarte, T. L., Dowling, T. E., and Coelho, M. M. (2007). Genetic structure of the diploid-polyploid fish Squalius alburnoides in southern Iberian basins Tejo and Guadiana, based on microsatellites. J. Fish Biol. 71, 423–436. doi: 10.1111/j.1095-8649.2007.01688.x
Don, J., and Avtalion, R. R. (1988). Production of viable tetraploid tilapias using the cold shock technique. Isr. J. Aquac. 40, 17–21.
Ferris, S. D., and Whitt, G. S. (1980). Genetic variability in species with extensive gene duplication: the tetraploid catostomid fishes. Am. Nat. 115, 650–666. doi: 10.1086/283590
Gu, Y., Guo, Z. B., Gu, J. J., Mao, R. X., Lu, C. Y., and Sun, X. W. (2009). Isolation of novel microsatellite markers from genome library and SSR polymorphic loci identification in turbot Scophthalmus maximus. J. Dalian Fish. University 24, 366–370.
Gui, J. F., Liang, S. C., Zhu, L. F., and Jiang, Y. G. (1993). Discovery of 2 different reproductive development modes of the eggs of artificial multiple tetraploid allogynogenetic silver crucian carp. Chin. Sci. Bull. 38, 332–341. doi: 10.1016/0167-8809(93)90098-A
Hershberger, W. K., and Hostuttler, M. A. (2005). Variation in time to first cleavage in rainbow trout Oncorhynchus mykiss embryos: a major factor in induction of tetraploids. J. World Aquac. Soc. 36, 96–102. doi: 10.1111/j.1749-7345.2005.tb00135.x
Hershberger, W. K., and Hostuttler, M. A. (2007). Protocols for more effective induction of tetraploid rainbow trout. North Am. J. Aquac. 69, 367–372. doi: 10.1577/a06-022.1
Hu, F., Wu, C., Zhou, Y., Cao, L., Xiao, J., Wang, S., et al. (2018). Production of androgenetic, triploid and tetraploid hybrids from the interspecific hybridization of female Japanese crucian carp and male blunt snout bream. Aquaculture 491, 50–58. doi: 10.1016/j.aquaculture.2018.03.014
Iyengar, A., Piyapattanakorn, S., Heipel, D. A., Stone, D. M., Howell, B. R., Child, A. R., et al. (2000). A suite of highly polymorphic microsatellite markers in turbot (Scophthalmus maximus L.) with potential for use across several flatfish species. Mol. Ecol. 9, 368–371. doi: 10.1046/j.1365-294x.2000.00874-3.x
Jeuthe, H., Brännäs, E., and Nilsson, J. (2016). Effects of variable egg incubation temperatures on the embryonic development in Arctic charr Salvelinus alpinus. Aquacu. Res. 47, 3753–3764. doi: 10.1111/are.12825
Lampert, K., Lamatsch, D. K., Fischer, P., and Schartl, M. (2008). A tetraploid amazon molly. Poecilia formosa. J. Heredity 99, 223–226. doi: 10.1093/jhered/esm102
Li, W. L., Chen, S. L., Ji, X. S., Xie, M. S., Xu, Y., and Deng, H. (2012). Induction and identification of tetraploid fry in Cynoglossus semilaevis. J. Fish. Sci. China 19, 196–201. doi: 10.3724/SP.J.1231.2011.17344
Lin, Z., Zhu, X., Zhang, T., You, F., Wu, Z., and Cao, Y. (2016). Effects of hydrostatic pressure on microtubule organization and nucleus changes in gynogenetically activated eggs of olive flounder (Paralichthys olivaceus). Theriogenology 85, 1610–1624. doi: 10.1016/j.theriogenology.2016.01.020
Liu, H., Wu, Z., Zhu, X., Song, Z., Hu, J., Wang, L., et al. (2018). Comparative performance of growth, vertebral structure and muscle composition in diploid and triploid Paralichthys olivaceus. J. Fish Dis. 41, 1495–1504. doi: 10.1111/jfd.12846
Luckenbach, J. A., Godwin, J., Daniels, H. V., Beasley, J. M., Sullivan, C. V., and Borski, R. J. (2004). Induction of diploid gynogenesis in southern flounder (Paralichthys lethostigma) with homologous and heterologous sperm. Aquaculture 237, 499–516. doi: 10.1016/j.aquaculture.2004.05.005
Malison, J. A., Kayes, T. B., Held, J. A., Barry, T. P., and Amundson, C. H. (1993). Manipulation of ploidy in yellow perch (Perca flavescens) by heat-shock, hydrostatic-pressure shock, and spermatozoa inactivation. Aquaculture 110, 229–242. doi: 10.1016/0044-8486(93)90371-5
Myers, J. M., and Hershberger, W. K. (1991). Early growth and survival of heat-shocked and tetraploid-derived triploid rainbow trout (Oncorhynchus mykiss). Aquaculture 96, 97–107. doi: 10.1016/0044-8486(91)90142-T
Myers, J. M., Hershberger, W. K., and Iwamoto, R. N. (1986). The induction of tetraploidy in Salmonids. J. World Aquac. Soc. 17, 1–7. doi: 10.1111/j.1749-7345.1986.tb00546.x
Pardo, B. G., Hermida, M., Fernández, C., Bouza, C., Pérez, M., Llavona, A., et al. (2010). A set of highly polymorphic microsatellites useful for kinship and population analysis in turbot (Scophthalmus maximus L.). Aquac. Res. 37, 1578–1582. doi: 10.1111/j.1365-2109.2006.01600.x
Pasquier, J., Braasch, I., Batzel, P., Cabau, C., Montfort, J., Nguyen, T., et al. (2017). Evolution of gene expression after whole-genome duplication: new insights from the spotted gar genome. J. Exp. Zool. Part B Mol. Dev. Evol. 328, 709–721. doi: 10.1002/jez.b.22770
Peruzzi, S., and Chatain, B. (2003). Induction of tetraploid gynogenesis in the European sea bass (Dicentrarchus labrax L.). Genetica 119, 225–228. doi: 10.1023/a:1026077405294
Rothbard, S., Shelton, W. L., Kulikovsky, Z., Rubinshtein, I., Hagani, Y., and Moav, B. (1997). Chromosome set manipulations in the black carp. Aquac. Int. 5, 51–64. doi: 10.1007/Bf02764787
Sakao, S., Fujimoto, T., Kimura, S., Yamaha, E., and Arai, K. (2006). Drastic mortality in tetraploid induction results from the elevation of ploidy in masu salmon Oncorhynchus masou. Aquaculture 252, 147–160. doi: 10.1016/j.aquaculture.2005.06.048
Shelton, W., Mims, S., Clark, J. A., Hiott, A. E., and Wang, C. (1997). A temperature-dependent index of mitotic interval (τ0) for chromosome manipulation in paddlefish and shovelnose sturgeon. Progress. Fish 59, 229–234. doi: 10.1577/1548-8640(1997)059<0229:atdiom>2.3.co;2
Thorgaard, G. H., Jazwin, M. E., and Stier, A. R. (1981). Polyploidy induced by heat shock in rainbow trout. Trans. Am. Fish. Soc. 110, 546–550. doi: 10.1577/1548-8659(1981)110<546:pibhsi>2.0.co;2
Valdebenito, I., Gallegos, P. C., and Roldán, B. (2013). Gamete quality in fish: evaluation parameters and determining factors. Zygote 23, 1–21. doi: 10.1017/S0967199413000506
Weber, G. M., and Hostuttler, M. A. (2012). Factors affecting the first cleavage interval and effects of parental generation on tetraploid production in rainbow trout (Oncorhynchus mykiss). Aquaculture 34, 231–238. doi: 10.1016/j.aquaculture.2012.03.017
Weber, G. M., Hostuttler, M. A., Cleveland, B. M., and Leeds, T. D. (2014). Growth performance comparison of intercross-triploid, induced triploid, and diploid rainbow trout. Aquaculture 433, 85–93. doi: 10.1016/j.aquaculture.2014.06.003
Wu, C., Huang, X., Hu, F., Ouyang, Y., Zhao, L., Wang, S., et al. (2019). Production of diploid gynogenetic grass carp and triploid hybrids derived from the distant hybridization of female grass carp and male topmouth culter. Aquaculture 504, 462–470. doi: 10.1016/j.aquaculture.2018.12.056
Wu, Z. H., You, F., Song, Z. C., Hu, J. W., Wang, L. J., Zhu, X. P., et al. (2014). Induction of tetraploid in turbot Scophthalmus maximus. Oceanol. Limnol. Sinica 45, 657–662.
Xu, S. B., Tao, Y. F., Yang, Z. Q., and Chu, J. Y. (2002). A simple and rapid methods used for silver staining and gel preservation. Hereditas 24, 335–336.
Yamaki, M., Satou, H., Taniura, K., and Arai, K. (1999). Progeny of the diploid-tetraploid mosaic amago salmon. Nippon Suisan Gakkai. 65, 1084–1089. doi: 10.2331/suisan.65.1084
Yi, Q. L., Yu, H. Y., Wang, X. L., Wang, Z. G., Qi, J., and Zhang, Q. Q. (2012). Production of viable tetraploid olive flounder (Paralichthys olivaceus) by hydrostatic pressure shock. Oceanol. Limnol. Sinica 43, 382–388.
You, F., Liu, J., Wang, X. C., Xu, Y. L., Huang, R. D., and Zhang, P. J. (2001). Study on embryonic development and early growth of triploid and gynogenetic diploid left-eyed flounder. Paralichthys olivaceus (T. et S.). Chin. J. Oceanol. Limnol. 19, 147–151. doi: 10.1007/bf02863039
You, F., Sha, X. S., and Ruan, H. C. (1991). Preliminary study on induced triploidy in Sparus microcephalus (Basilewsky). Oceanol. Limnol. Sinica 22, 489–491.
Yu, F., Wang, W. J., Kong, J., and Ruan, X. H. (2009). The application of microsatellite markers for genealogical identification in selective breeding program of turbot (Scophthalmus maximus L.). Acta Oceanol. Sinica 31, 127–136.
Zhang, X., Mutsukawa, K., and Onozato, H. (2005). Correlation between delay in the earlier cleavage stage and the tetraploidization rate in rainbow trout Oncorhynchus mykiss embryos treated with heat or hydrostatic pressure shock during the first cell cycle. Fish. Sci. 71, 239–241. doi: 10.1111/j.1444-2906.2005.00954.x
Zhang, X. L., and Onozato, H. (2004). Hydrostatic pressure treatment during the first mitosis does not suppress the first cleavage but the second one. Aquaculture 240, 101–113. doi: 10.1016/j.aquaculture.2004.07.004
Zhou, L., and Gui, J. F. (2017). Natural and artificial polyploids in aquaculture. Aquacu. Fish. 2, 103–111. doi: 10.1016/j.aaf.2017.04.003
Keywords: turbot Scophthalmus maximus, tetraploid, artificial induction, first cleavage index, genetic diversity
Citation: Wu Z, Wang L, Lu Y, Zhu X, Yue X and You F (2019) Artificial Induction and Genetic Structure Analysis of Tetraploid Turbot Scophthalmus maximus. Front. Mar. Sci. 6:637. doi: 10.3389/fmars.2019.00637
Received: 25 May 2019; Accepted: 30 September 2019;
Published: 16 October 2019.
Edited by:
Dongdong Xu, Marine Fisheries Research Institute of Zhejiang, ChinaReviewed by:
Jie Mei, Huazhong Agricultural University, ChinaTian Yongsheng, National Astronomical Observatories (CAS), China
Haiyang Yu, Ocean University of China, China
Copyright © 2019 Wu, Wang, Lu, Zhu, Yue and You. This is an open-access article distributed under the terms of the Creative Commons Attribution License (CC BY). The use, distribution or reproduction in other forums is permitted, provided the original author(s) and the copyright owner(s) are credited and that the original publication in this journal is cited, in accordance with accepted academic practice. No use, distribution or reproduction is permitted which does not comply with these terms.
*Correspondence: Feng You, eW91ZmVuZ0BxZGlvLmFjLmNu