- 1Department of Arctic and Marine Biology, UiT – The Arctic University of Norway, Tromsø, Norway
- 2Faculty of Bioscience and Aquaculture, Nord University, Bodø, Norway
- 3Akvaplan-niva, FRAM – High North Research Centre for Climate and the Environment, Tromsø, Norway
- 4Department of Geosciences, UiT – The Arctic University of Norway, Tromsø, Norway
Cold-seep benthic communities in the Arctic exist at the nexus of two extreme environments; one reflecting the harsh physical extremes of the Arctic environment and another reflecting the chemical extremes and strong environmental gradients associated with seafloor seepage of methane and toxic sulfide-enriched sediments. Recent ecological investigations of cold seeps at numerous locations on the margins of the Arctic Ocean basin reveal that seabed seepage of reduced gas and fluids strongly influence benthic communities and associated marine ecosystems. These Arctic seep communities are mostly different from both conventional Arctic benthic communities as well as cold-seep systems elsewhere in the world. They are characterized by a lack of large specialized chemo-obligate polychetes and mollusks often seen at non-Arctic seeps, but, nonetheless, have substantially higher benthic abundance and biomass compared to adjacent Arctic areas lacking seeps. Arctic seep communities are dominated by expansive tufts or meadows of siboglinid polychetes, which can reach densities up to >3 × 105 ind.m–2. The enhanced autochthonous chemosynthetic production, combined with reef-like structures from methane-derived authigenic carbonates, provides a rich and complex local habitat that results in aggregations of non-seep specialized fauna from multiple trophic levels, including several commercial species. Cold seeps are far more widespread in the Arctic than thought even a few years ago. They exhibit in situ benthic chemosynthetic production cycles that operate on different spatial and temporal cycles than the sunlight-driven counterpart of photosynthetic production in the ocean’s surface. These systems can act as a spatio-temporal bridge for benthic communities and associated ecosystems that may otherwise suffer from a lack of consistency in food quality from the surface ocean during seasons of low production. As climate change impacts accelerate in Arctic marginal seas, photosynthetic primary production cycles are being modified, including in terms of changes in the timing, magnitude, and quality of photosynthetic carbon, whose delivery to the seabed fuels benthic communities. Furthermore, an increased northward expansion of species is expected as a consequence of warming seas. This may have implications for dispersal and evolution of both chemosymbiotic species as well as for background taxa in the entire realm of the Arctic Ocean basin and fringing seas.
Introduction
Photosynthesis is the main pathway of organic material production in the world’s oceans. Since this process relies on energy from sunlight, it is only spatially and temporally possible where and when there is enough light in the water column to stimulate photosynthetic activity (Harrison and Platt, 1986; Duarte and Cebrián, 1996). Generally, photic zones terminate at a maximum of 200 m water depth, and outside the tropics, photosynthetic production is highly seasonal (Sakshaug and Slagstad, 1991; Moran et al., 2012). Organisms living on the ocean floor outside the coastal zone largely rely on organic material sinking from the photic zone to the seabed, and the quality and quantity of organic material reaching the seafloor is correlated with water depth (Southward and Southward, 1982; Gage and Tyler, 1991; Morata et al., 2008). However, photosynthetic organisms and their carbon fixation activities in surface waters do not represent the entirety of the food supply to the benthos. Other sources of food are zooplankton and fecal pellets that become available to the benthos via pelagic–benthic coupling processes (Graf, 1989; Grebmeier and Barry, 1991). In benthic deep-sea systems, inorganic carbon fixed via prokaryotes (so-called “dark fixation”) may also provide significant carbon sources (Molari et al., 2013; Sweetman et al., 2019). Occasionally, large pulses of food arrive at the bottom of the ocean in the form of carcasses of large animals such as whale/shark falls, or mass mortality of jellyfish (e.g., Smith and Baco, 2003; Higgs et al., 2014; Dunlop et al., 2018) or even from terrestrial sources by wood-falls or large inputs of sediment (e.g., Dando et al., 1992; Bienhold et al., 2013; Holding et al., 2017; Sen et al., 2017).
Nonetheless, food availability on the seafloor is closely tied to photosynthetic primary production in surface waters (Rice et al., 1986Renaud et al., 2008; Morata et al., 2013). This pelagic–benthic coupling means that temporal and spatial patterns in surficial primary production regulates benthic food availability (Gooday et al., 1990; Wassmann et al., 2006; Morata et al., 2008). The most common manifestation of variable food input to the seafloor as a result of surface conditions is seasonality: benthic systems tend to be more food limited during the winter months when daylight is limited (Renaud et al., 2007; Ambrose et al., 2012; Morata et al., 2013). In polar regions, this seasonal disparity is particularly pronounced due to the phenomena of midnight sun and polar night (the absence of setting or rising of the sun above the horizon, respectively) (Berge et al., 2015). Summer biological blooms in polar regions are, furthermore, not limited to phytoplankton. Sea ice allows for the proliferation of ice algae during periods of unrestricted sunlight (Hegseth, 1998; McMahon et al., 2006; Søreide et al., 2006). The simultaneous presence of phytoplankton blooms and melting sea ice during Arctic spring and early summer results in a peak in both the quantity and quality of food supply to the benthos (Ambrose and Renaud, 1997; Wassmann et al., 2006; Renaud et al., 2008).
Cold seeps are locations on the seafloor where reduced compounds from subsurface hydrocarbon reserves enrich sediment fluids or emanate freely as gas from the seabed. Methane is often the predominant gas seeping up from sub-seabed reservoirs, however, fractions of heavier hydrocarbons can also occur (MacDonald, 1990; Hovland and Svensen, 2006; Andreassen et al., 2017). A consortium of sediment microbes oxidizes methane anaerobically through a process known as the anaerobic oxidation of methane (AOM) (Boetius et al., 2000). Sulfate reduction takes place concomitantly in this process, thereby generating another reduced compound, hydrogen sulfide. The enrichment of sediment porewater in compounds such as methane and sulfide provides the setting for chemosynthesis or the fixation of carbon by microbes with reduced compounds as the energy source. Chemosynthetic microbes can either be free living or reside within or on organisms with which they form a symbiotic relationship. Together, such autochthonous primary production at the seabed forms the base of a food chain and an ecosystem that revolves around chemical-based autotrophic processes (Fisher, 1996; Sibuet and Olu, 1998; Levin, 2005; Becker et al., 2013). Cold-seep systems, thus, function largely outside the paradigm of conventional benthic systems that rely on food supplied from the pelagic zone. They typically exhibit an uncharacteristically high biomass and abundance of organisms due to local energy sources and food production (Sibuet and Olu, 1998; Levin et al., 2016). However, sulfide that is produced as a result of seabed methane seepage and microbial activity is lethal even in low concentrations for most organisms because of its negative effect on oxidative respiration (Vismann, 1991; Bagarinao, 1992). Thus, despite high biomass at cold seeps, the presence of sulfide may limit biodiversity at seeps in comparison to non-reducing environments (Sibuet and Olu, 1998; Levin et al., 2003).
Until recently, Arctic seep-ecology research was nearly exclusively limited to the study of the Håkon Mosby mud volcano (HMMV) in the south-west Barents Sea at 72°N, 14°E (Figure 1). Concentrated efforts at HMMV proved insightful in understanding various aspects of this Arctic cold-seep system (e.g., Hjelsteun et al., 1999; Gebruk et al., 2003; Niemann et al., 2006; Jerosch et al., 2007; Decker et al., 2012). Yet, to extrapolate to larger scale processes and broader contexts requires investigation of multiple locations. In this review, we reveal how Arctic seep studies have progressed from merely viewing HMMV as a single anomaly to a more comprehensive understanding of Arctic seep ecology. We also discuss how Arctic seeps differ from seep ecology in lower latitudes.
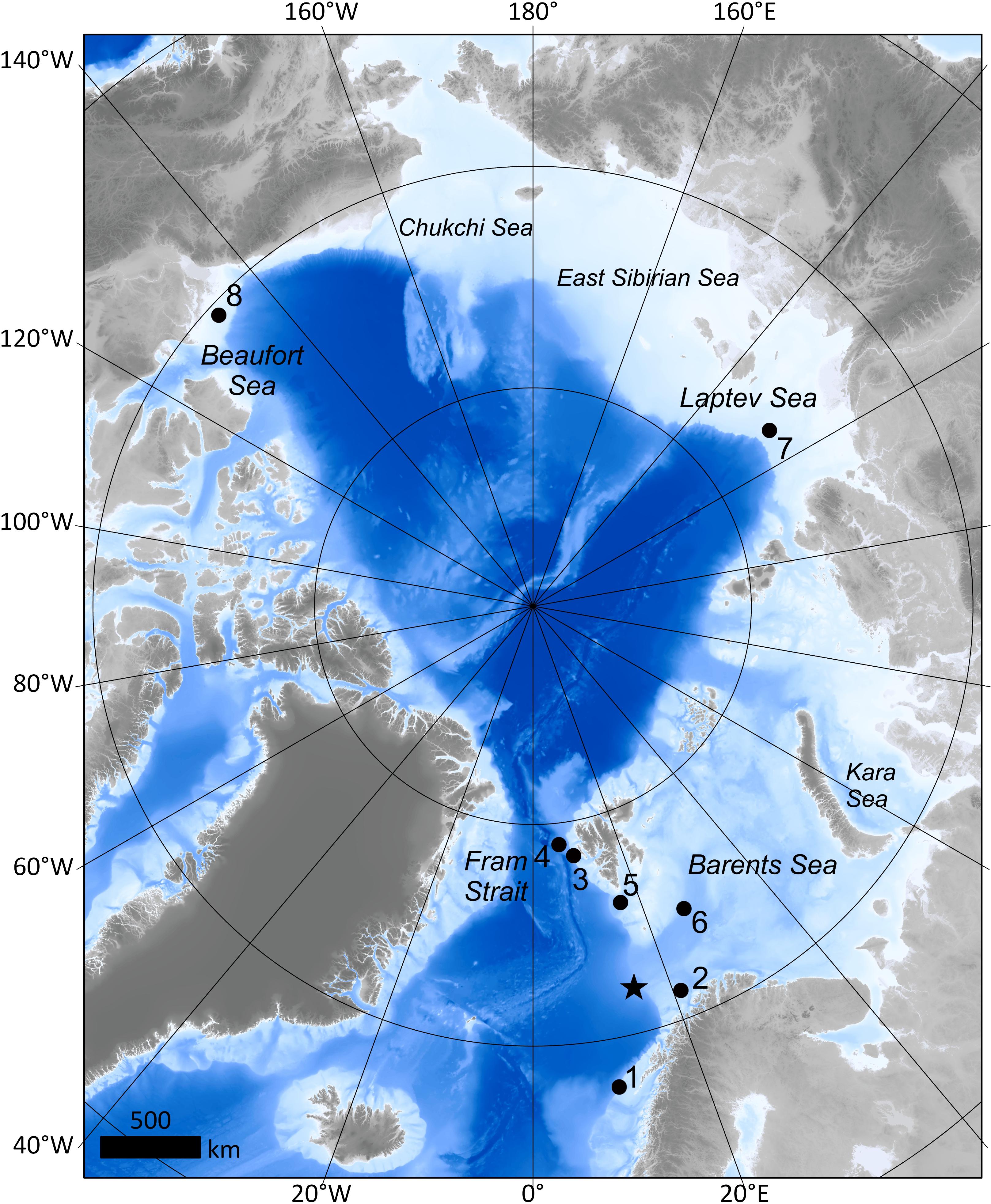
Figure 1. The Arctic and major seas. Seep sites discussed in this review are marked with black circles. Water depths of seep locations are presented in parentheses after the site name. The star marks the location of the Håkon Mosby mud volcano (HMMV) (1,250 m). 1: Lofoten canyons (750–800 m), 2: Svanfjell seep (380 m), 3: Prins Karls Forland (80–350 m), 4: Vestnesa Ridge (1,200 m), 5: Storfjordrenna seep and gas hydrate mounds/pingos (350–390 m), 6: Bjørnøyrenna seep and craters (330–360 m), 7: Laptev Sea (70 m), 8: Beaufort Sea mud volcanoes (280–740 m). Bathymetry for this map was obtained from IBCAO (Jakobsson et al., 2012).
The world’s oceans are in constant interaction of large-scale oceanographic and chemical processes, mitigating causes of climate changes through uptake of atmospheric CO2, other greenhouse gas equivalents, and excess heat. Consequences of such processes are warmer oceans affecting circulation and mixing, solubility of gases (i.e., reduced oxygen levels), changes in productivity, and ocean acidification (Levin and Bris, 2015; IPCC, 2018; Mora et al., 2018). The Arctic is no exception to these impacts; in fact, warming at the poles is occurring faster than elsewhere on Earth (Comiso and Hall, 2014), and the region is subjected to unprecedented changes as a result of the continuing rise of atmospheric greenhouse gases (IPCC, 2018). Even if global actions to reduce emissions are implemented immediately, climate change impacts will continue for at least the next few decades due to the time lag of effects (Steffen et al., 2018). It is, therefore, relevant and timely to examine Arctic seeps within the context of climate change.
In this review, we specifically focus on macrofaunal and megafaunal communities, i.e., taxa ≥ 0.5 cm and taxa visible with the naked eye from seafloor images (cm scale and larger) (e.g., Bowden et al., 2013; Amon et al., 2017; Sen et al., 2018a). Microbiology and meiofaunal communities are referred to only within the context of the ecology of larger animals. We discuss taxa and species inhabiting cold seeps using the following terminology: “Obligate chemosymbiotrophic” species reliant on nutritional chemosymbiosis; “obligate seep” species only present at seeps, “background” species occurring at seeps and non-seep sites, not matching the abovementioned criteria. Characteristically for many seeps worldwide are three groups of taxa: vestimentiferan siboglinids, vesicomyid clams, and bathymodioline mussels. We refer to these taxa as “hallmark seep” taxa. The geographical focus of this review is “the Arctic,” and we discuss the Arctic as the area above the Arctic Circle (66°33′N). We are aware that there are many different ways of defining the Arctic (Artcic Monitoring and Assessment Programme [AMAP], 1998), however, using the Arctic Circle makes this definition easily identifiable. Furthermore, this definition is biologically relevant due to the phenomena of midnight sun and polar night, which are strongly coupled to annual biological production cycles.
Past Arctic Cold-Seep Investigations
The Håkon Mosby Mud Volcano (HMMV)
The Håkon Mosby Mud volcano is a large, circular-shaped (1–2 km) mud volcano, located at 72°N at a water depth of about 1,250 m (Figure 1). Since its discovery in 1989, HMMV stood out as an unusual cold-seep system in the world’s oceans (Vogt et al., 1997). It lacks an association with either salt or plate tectonics, which are two of the primary means of providing migration conduits for seepage of gas-enriched fluids from within the seabed (Vogt et al., 1997). Additionally, the site is located within the Arctic and appears to have developed within glacial sediments. These unusual features prompted numerous scientific campaigns across various disciplines including geophysics, biogeochemistry, microbiology, and ecology to be carried out at HMMV (e.g., Vogt et al., 1997; Hjelsteun et al., 1999; Gebruk et al., 2003; Niemann et al., 2006; Jerosch et al., 2007; Lösekann et al., 2007; Decker et al., 2012; Rybakova et al., 2013). These studies provided the first insight into the structure and functioning of an Arctic cold-seep system.
Distinct habitats and species assemblages radiate concentrically from a central, visually uninhabited zone of fluid expulsion of HMMV (Gebruk et al., 2003; Jerosch et al., 2007; Rybakova et al., 2013). The zone just outward of the central zone is covered in mosaics of bacterial mats of various thicknesses. The most dominant forms among the mats are filamentous bacteria resembling sulfur-oxidizing strains such as Beggiatoa (Pimenov et al., 2000; Gebruk et al., 2003). Beyond the bacterial mats, there are zones dominated by siboglinid worms of two species: the moniliferan Sclerolinum contortum and the frenulate Oligobrachia haakonmosbiensis (Smirnov, 2008, 2014) (Figure 2). Though both species occur in high densities, forming large (up to meter square) tufts, S. contortum is the dominant species (biomass 435 g m–2 vs. 350 g m–2) (Gebruk et al., 2003).
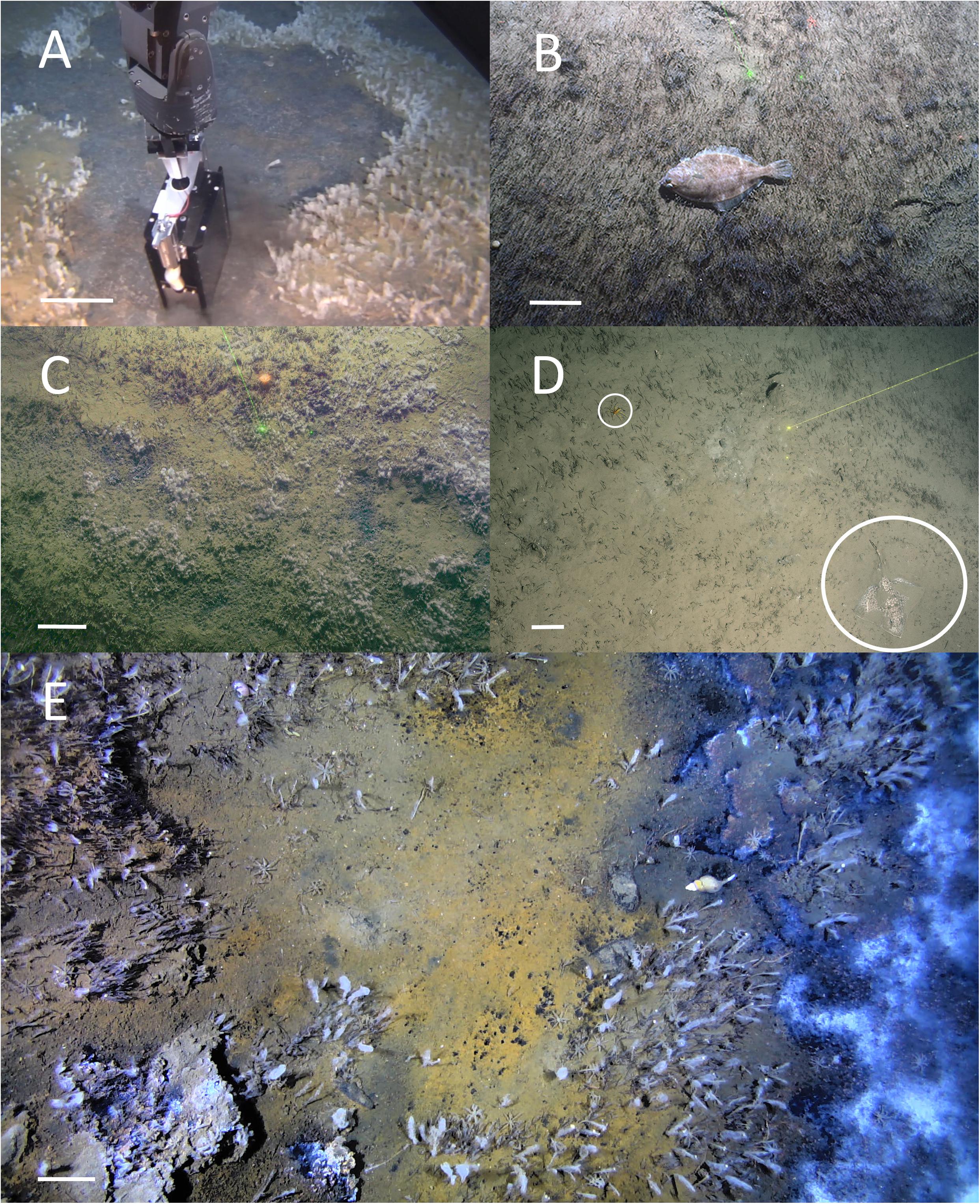
Figure 2. Tufts of siboglinids (mainly Oligobrachia spp.) from different Arctic cold-seep locations. From top left to right: (A) A large siboglinid field covered by filamentous bacteria in the “Bjørnøyrenna crater seep” Barents Sea (330 m) from Åström et al. (2019). (B) An American plaice (Hippoglossoides platessoides) in a dense meadow of siboglinids at the Storfjordrenna gas hydrate mounds/pingos, (380 m), from Åström et al. (2016). (C) Siboglinids and filamentous bacteria at the Storfjordrenna seep field (350 m), note also the bright orange anemone in the top middle, from Åström et al. (2019). (D) Siboglinid tufts at the deep Vestnesa seep (Fram Strait, 1,200 m); encircled is a half-buried Arctic skate (Amblyraja hyperborea) and a sea spider (Colossendeis sp.), from Åström et al. (2018). (E) Carbonate outcrops, microbial mats, and filamentous bacteria covering siboglinid tubes at the Lofoten canyon seeps (750 m); note the many sea spiders (Nymphon hirtipes) at the bottom, from Sen et al. (2019b). Scale bars indicate 20 cm.
The outermost zone is characterized by ophiuroids and a lack of chemosynthesis-based community members such as bacterial mats and siboglinid worms (Rybakova et al., 2013). In total, 80 in faunal and epifaunal taxa have been recorded at HMMV (Gebruk et al., 2003; Decker et al., 2012; Rybakova et al., 2013). Biomass is higher within the mud volcano compared to the outside and background benthos, while taxonomic richness does not differ significantly between inside and outside of the volcano (Figure 3; Rybakova et al., 2013). Gebruk et al. (2003) also noted that the background fauna (animals inhabiting the seafloor beyond the seep-influenced area) appeared to be considerably “poorer,” particularly with respect to biomass.
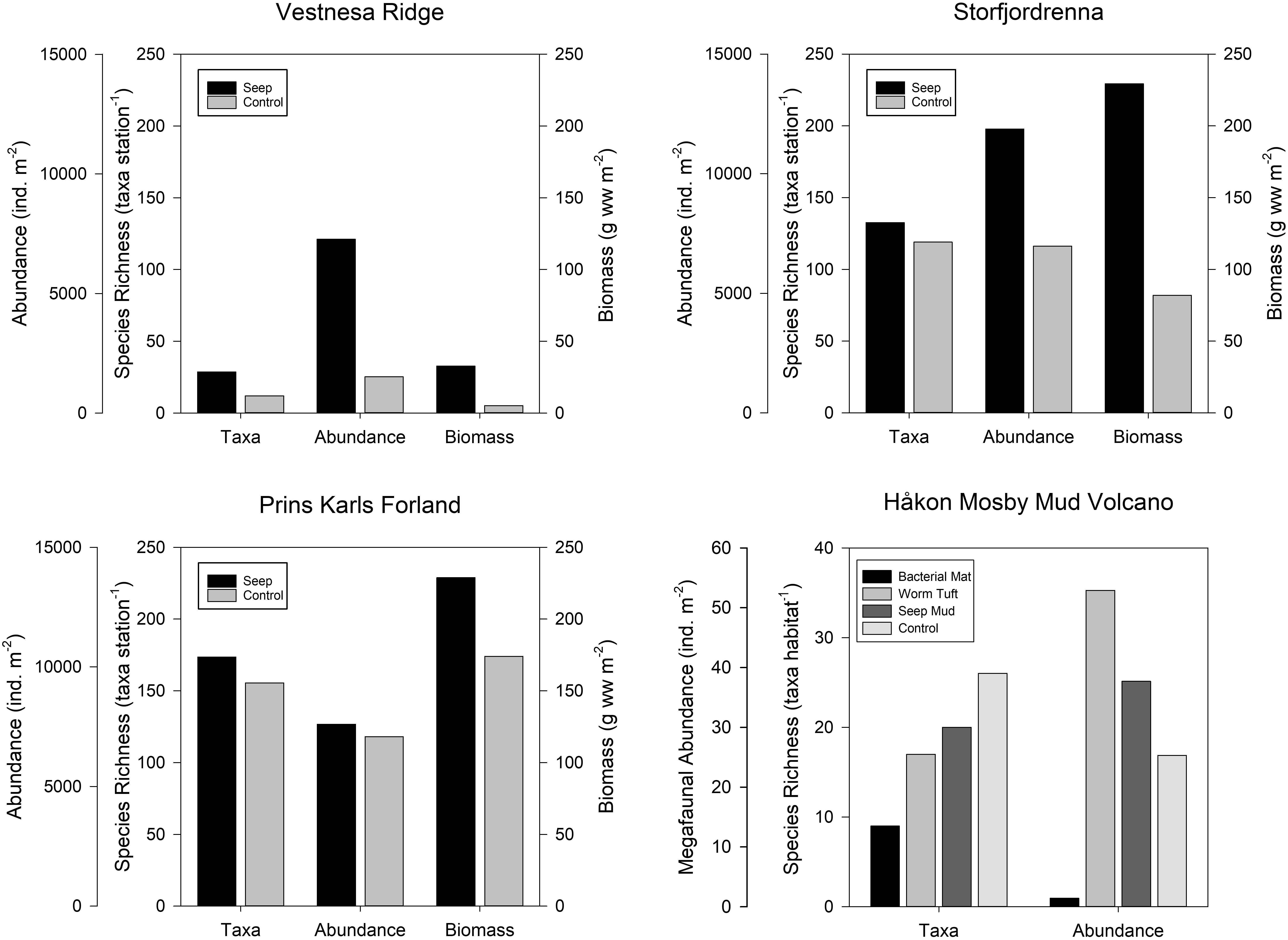
Figure 3. Community faunal parameters [species richness, abundance (density), and biomass] at cold seeps and control areas from four regions in the Arctic where quantitative faunal data are available. Vestnesa Ridge, Storfjordrenna seep, and Prins Karls Forland document macrofaunal infauna, while the Håkon Mosby Mud Volcano is mobile megafauna. Data are compiled from the following sources: Vestnesa Ridge (1,200 m) – Åström et al. (2018), Storfjordrenna (350–390 m) – Åström et al. (2016, 2019), Prins Karls Forland (85–350 m) – Åström et al. (2016), Håkon Mosby Mud Volcano (1,250 m) – Rybakova et al. (2013).
The ambient water around HMMV is characterized by subzero temperatures (De Beer et al., 2006; Portnova et al., 2011); however, sediment and fluid release at the mud volcano is accompanied by considerable heat release in the center (up to 40°C at 0.5 m below sediment surface) (Kaul et al., 2006; Feseker et al., 2008). The extent of heat, fluid, and mud release follows a concentric pattern where the greatest mud and fluid expulsion rates occur in the central zone, while the outer zones are less dynamic. Seepage of heated methane-rich porewater from deep sediments below HMMV causes unstable sediments as oversaturated mud is expulsed to the seafloor surface. The temperature variability in the surface sediments (-0.8 to + 25°C) (Kaul et al., 2006) at HMMV suggests dynamic systems with frequent eruptions and fluid flows in the center (Feseker et al., 2008). Such unstable sediments likely cause the observed faunally devoid area in the central zone (Gebruk et al., 2003; Van Gaever et al., 2006; Decker et al., 2012). High temperature has also been hypothesized as a possible cause of the conspicuous absence of fauna in addition to microbial mats (Gebruk et al., 2003; Decker et al., 2012). Indeed, the heat anomalies detected in the central zone (40°C) are some of the highest recorded at any cold-seep site (Feseker et al., 2008). High sulfide concentrations accompany the release of heat, which, together with the instability of the environment, may further hinder settlement and colonization of animals (Heyl et al., 2007; Rybakova et al., 2013; Feseker et al., 2014).
Distinctive Ecological Features of HMMV
Key ecological features of HMMV are an elevated biomass and a difference in faunal composition relative to the surrounding seafloor. This parallels what has been observed at seeps in other locations: seeps are known as biomass-rich “hotspots” (Carney, 1994; Weaver et al., 2004) on the seafloor, and community composition tends to differ substantially from the surrounding benthos due to the presence of obligate seep and chemosymbiotrophic fauna as well as opportunistic and vagrant background species (Levin et al., 2016). Local chemosynthesis-based primary production is usually cited to account for this trend since it provides an additional food-source to deep-sea seabed habitats. At HMMV, chemosynthetically derived carbon can be detected in the benthic food web and contributes significantly to the diet of some taxa such as Alvania gastropods, capitellid polychetes, amphipods, and pantopods (sea spiders) (Decker and Olu, 2012). Nonetheless, HMMV displays some characteristics that stand apart from cold seeps in other parts of the world.
Most striking is the absence of characteristic faunal groups associated with cold-seep ecosystems, e.g., vesicomyid clams, vestimentiferan worms, and bathymodioline mussels (hallmark seep fauna). These taxa tend to dominate seep systems and account for a large part of the biomass at cold seeps, presumably due to their ability to derive nutrition from seep fluids through endosymbiotic, chemosynthetic bacteria. The absence of such hallmark seep species at HMMV is particularly intriguing since these groups are known from North Atlantic locations both along the European and North American margin (e.g., Mayer et al., 1988; Rodrigues et al., 2013; Skarke et al., 2014) including marginal seas such as the Mediterranean (Olu-Le Roy et al., 2004; Loher et al., 2018).
Second, the taxonomic inventory of HMMV appears to consist entirely of background fauna (no obligate seep taxa) and two chemosymbiotrophic taxa (Sclerolinum moniliferans and Oligobrachia frenulates) (Figure 2) (Rybakova et al., 2013; Georgieva et al., 2015). Seeps tend to host taxa that are found only at seeps due to the toxicity of sulfide (Black et al., 1997; Schulze and Halanych, 2003). Even though these taxa are not the exclusive residents of seeps, they, nonetheless, make up at least some part of seep communities worldwide. Exceptions are shallow-water seeps (less than 200 m water depth) where greater availability of photosynthetic material at these locations and/or increased available substrate have been hypothesized to select against chemosymbiotic species and subsequently, seep obligate taxa (Sahling et al., 2003; Tarasov et al., 2005; Dando, 2010). At the time, the overall lack of obligate seep fauna at HMMV was considered the exception to the concept of deep-seep systems hosting specialized seep communities.
Third, HMMV does not appear to host a lower macrofaunal diversity community than the surrounding non-seep seafloor. Toxic sulfide is often considered the reason for low diversity and the presence of specialized taxa at seeps (Levin et al., 2003; Heyl et al., 2007). However, Rybakova et al. (2013) found that the HMMV community exhibited more diverse faunal assemblages than the surrounding seafloor community (Figure 3). Therefore, HMMV appears to attract and host a high number of background benthic species from the surrounding seafloor in contrast to non-Arctic seeps.
While these ecological characteristics at HMMV distinguished it from seep systems in other parts of the world, it was unknown at the time whether HMMV was simply a rarity or whether it was representative of Arctic seeps in general. Answering this question necessitated examining additional Arctic seeps. Such investigations were, however, not conducted until recently.
Recent Arctic Cold-Seep Investigations
Sources of Seafloor Seepage in the Arctic
Seafloor hydrocarbon seepage may occur because of migration of gas and fluids from sub-seabed reservoirs to the sediment surface. In the Arctic, though, a major contributor to seafloor seepage is the presence of subsurface gas hydrate reserves. Gas hydrates are frozen water structures (ice) within which gas molecules (usually methane) are trapped or “caged” (Koh and Sloan, 2007). These solid pieces of ice and gas are stable in sediments with high pressure and low temperature, a zone referred to as the Gas Hydrate Stability Zone (GHSZ) (Sloan, 1998). In most of the world’s oceans, conditions for maintaining the GHSZ (i.e., high pressure and low temperature) are met only in deep-water slope sediments and continental margins (Weaver and Stewart, 1982; Kvenvolden et al., 1993). Cold conditions in the Arctic, however, allow gas hydrates to be present in sediment at relatively shallow water depths (Kvenvolden et al., 1993). The Arctic Ocean is surrounded by continents with large, relatively shallow shelves and continental margins, and at these circumpolar margins, large quantities of hydrocarbon reservoirs and gas hydrates are expected to exist in the sediment because of the Arctic’s glacial history, hydrostatic pressure, and thermal gradients (Shakhova et al., 2010; Stranne et al., 2016; Andreassen et al., 2017). In short, the Arctic may hold substantial quantities of gas hydrates because of cold conditions and widespread, glacially influenced shelves, and therefore, there is a high potential for the development of seep ecosystems.
Subsequently, there is evidence for seeps being abundant in the Arctic. Large-scale bathymetric features such as mud volcanoes and pockmarks are present both at North American and European continental margins. Pockmarks, which commonly form due to the collapse of oversaturated gas or fluid-filled pore-spaces in sediments, are markers of past or present gas seepage from the seabed. They are common along the North Sea – Norwegian margin and the southern Barents Sea, and are sometimes observed in high densities, extending over several hundred km–2 (Hovland and Svensen, 2006; Chand et al., 2009; Rise et al., 2014). Seeps have also been identified along the Norwegian continental margin and the Western Svalbard shelf (Solheim and Elverhøi, 1993; Vogt et al., 1994, 1997; Lammers et al., 1995). In the Kara Sea, there are numerous locations where hydro acoustic gas flares have been documented (Portnov et al., 2013; Serov et al., 2015), and a large part of the Siberian shelves are predicted to hold substantial amounts of gas hydrates in the seabed (Stranne et al., 2016). Methane and gas hydrates are also believed to be present along the Alaskan, Canadian, and Greenland continental margins (Weaver and Stewart, 1982; Kvenvolden et al., 1993).
If either the temperature or the pressure criteria for the maintenance of gas hydrates is breached, large-scale dissociation of gas hydrates can occur. As a result, the limits of the GHSZ in the Arctic have in the past been closely linked to the glacial history of Arctic shelves (Shakhova et al., 2010; Stranne et al., 2016; Andreassen et al., 2017). Periods characterized by gas hydrate dissociation have shaped regions of the Arctic seafloor bathymetry, due to releases, some even catastrophic, creating structures such as mounds or blow-out craters on the seafloor (Andreassen et al., 2017; Serov et al., 2017). Warming conditions in the Arctic have, furthermore, raised concerns of gas hydrate dissociation in current times: shallow water gas hydrates, for example, could quickly lose stability with rising temperatures. Methane is a much more potent greenhouse gas than CO2, so methane reaching the atmosphere may have a disproportionate impact on the planet’s climate. The potential for the vast Arctic gas hydrate reserves to destabilize and expel large quantities of methane into the atmosphere is one of the reasons for an increase in Arctic seep research in recent years (Shakhova et al., 2010). Results, to date, indicate little evidence supporting a doomsday scenario of a massive efflux of a potent greenhouse gas from the ocean to the atmosphere (Berchet et al., 2016; James et al., 2016; Myhre et al., 2016), but such studies opened up the opportunity to conduct biological research at a number of Arctic seep sites.
We concentrate on the findings from eight locations in addition to HMMV where biological studies have been conducted (Figure 1). These recent investigations serve as the rationale for this review and the basis for this synthesis of the current state of knowledge on the biology and ecology of Arctic seeps.
Arctic Seep Sites
Lofoten Canyons (750–800 m Water Depth)
Offshore northern Norway and the Lofoten islands, more than 15 canyons are located on the continental slope at about 68°N (Figure 1). Some are deeply incised into the Cenozoic sedimentary succession (Rise et al., 2013). The two smallest canyons, approximately 2 km in length and up to 50 m deep, are located in water depths of about 750 m and were discovered to host cold-seep communities (Bellec, 2015). No free gas (bubbling of gas from the seafloor) has been observed at this location; however, sediment porewater fluids are enriched in dissolved gases of reduced compounds. Isotope analyses of porewater fluids indicate that dissolved methane is of biogenic origin, although the actual sources are still being investigated (Hong et al., 2019). In addition to methane seepage at the canyons, there is a freshwater discharge from submarine groundwater reservoirs, which is suggested to considerably influence the local circulation and ocean chemistry in the area (Hong et al., 2019).
Svanefjell (380 m Water Depth)
North of the Lofoten canyons, at about 72°N on the Norwegian continental shelf, lies the Svanefjell seep site at about 380 m water depth (Figure 1; Sen et al., 2019a). The surroundings of the Svanfjell seep consists of numerous seafloor pockmarks between 30 and 50 m in diameter (Rise et al., 2014; Sen et al., 2019a). Gas flares have been detected rising into the water column from a number of the pockmarks through multibeam surveys of the area, and seismic profiles of the sediment reveal anomalies that are indicative of subsurface hydrocarbon reserves.
Prins Karls Forland (PKF) (80–350 m Water Depth)
Prins Karls Forland is located on the shelf west of Svalbard (78°N) (Figure 1), where extensive gas seepage has been reported in the form of hydro-acoustically detected bubble plumes in water depths between 80 and 400 m (Berndt et al., 2014; Sahling et al., 2014; Åström et al., 2016). Additionally, high concentrations of methane have been detected in the water column at these locations (Berndt et al., 2014; Myhre et al., 2016; Pohlman et al., 2017). A cluster of sites exhibiting strong seafloor seepage at about 400 m water depth along the Prins Karls Forland shelf aligns with the predicted upper border of the GHSZ (∼400 m) (Portnov et al., 2016; Mau et al., 2017). Moreover, there is abundant seepage activity at considerably shallower depths at the Forland moraine complex (>80 m), and this shallower cluster of seeps is thought to be caused by gas migrating from deeper reservoirs through sub-seabed faults (Berndt et al., 2014; Sahling et al., 2014; Portnov et al., 2016).
Vestnesa Ridge (1,200 m Water Depth)
Northwest of PKF on the continental slope in the Fram Strait at 79°N lies Vestnesa Ridge (Figure 1), a ∼100 km long ultraslow spreading sediment-drift ridge (Johnson et al., 2015) at ∼1,200 m water depth. Along the ridge are numerous pockmarks associated with sub-seabed methane hydrate reservoirs (Vogt et al., 1994; Bünz et al., 2012). The sub-surface gas at these pockmarks is of both microbial and abiotic/thermogenic (Johnson et al., 2015). Multiple methane bubble plumes have been acoustically detected in geophysical surveys in the water column along the ridge, rising up to 800 m above the seafloor (Bünz et al., 2012).
The Storfjordrenna Seep and Storfjordrenna Pingos/Gas Hydrate Mounds (350–390 m Water Depth)
Prins Karls Forland and Vestnesa represent the western edge of one of the most well known and well studied of the Arctic seas, the Barents Sea. The bathymetry of the Barents Sea is largely influenced by the glacial history of northern margins and the coverage of the Barents Sea Ice Sheet (BSIS), and its deglaciation starting approximately 20,000 years BP (Rasmussen et al., 2007; Ingólfsson and Landvik, 2013; Patton et al., 2017). The present day average water depth of the Barents Sea is ∼230 m, and the shelf is characterized by extensive post-glacial features such as, troughs, plow marks, and mega-scale lineations (Patton et al., 2017). Located in the western Barents Sea and south of the Svalbard archipelago, Storfjordrenna is one of the larger troughs (∼250 km long). In the mouth of the trough, there are several locations of active methane seepage that align with the upper predicted limit of the GHSZ (∼350–400 m water depth) (Figure 1; Åström et al., 2016; Mau et al., 2017; Serov et al., 2017). One of these active methane seep sites is referred to simply as the “Storfjordrenna seep” (SR), located close to a glacial grounding zone wedge at approximately 350 m water depth (75°N). A few tens of kilometers north of the Storfjordrenna seep and within Storfjordrenna, is another area of highly active methane seepage located in similar water depths, at 76°N. This site is characterized by a cluster of several gas hydrate bearing mounds, or submarine “pingos,” which individually are a few hundred meters in diameter and rise approximately 10–15 m above the seabed (Hong et al., 2017; Serov et al., 2017). Seepage, identified by hydroacoustic flares has been observed from the tops of these pingos, with some flares reaching hundreds of meters above the seabed. This site is referred to as the Storfjordrenna pingo site or Storfjordrenna gas hydrate mound site.
Bjørnøyrenna Flare Area and Bjørnøyrenna Craters (330–360 m Water Depth)
Bjørnøyrenna is another large trough located south-east of Storfjordrenna, moving into the central Barents Sea. Within this trough, a large area (∼440 km2) with hundreds of seafloor depressions, craters, and crater–mound complexes is present at about 74°N (Figure 1; Solheim and Elverhøi, 1993). These craters and mounds are thought to be the result of massive blowouts of sub-surface gas deposits and subglacial gas hydrates during the deglaciation of the BSIS (Andreassen et al., 2017). The craters vary from approximately 100 m up to 1 km in diameter and are up to 30 m deep, whereas mounds rise up to 20 m above the seabed. Several of the craters and mounds are associated with methane seepage from the seabed (Andreassen et al., 2017), and the seafloor inside and around the craters and mounds is strikingly characterized by large angular blocks (Solheim and Elverhøi, 1993). This area, as a whole, is referred to as the Bjørnøyrenna crater area (e.g., Åström et al., 2016, 2019; Sen et al., 2018b; Ofstad et al., 2020).
Laptev Sea Seep (70 m Water Depth)
Along the Siberian Arctic, both terrestrial and sub-seabed permafrost layers in the ground are relics of past glacial history, and such features can store large quantities of methane gas and hydrates. In the Laptev Sea, located between the two marginal seas along the Siberian shelf, the Kara Sea and the East Siberian Sea, sub-seabed permafrost layers are estimated to reach 20–60 m down into the seabed. The second largest river in the Siberian Arctic, the Lena River, terminates into the Laptev Sea, and the river-delta system contributes large amounts of freshwater and suspended matter from the river catchment area. North of this river delta and at about 70 m water depth, a methane-seep site was recently discovered at 76.5°N, 127°E (Figure 1). This site represents one of the shallowest known seep sites in the Arctic (Demina and Galkin, 2018).
Beaufort Sea Mud Volcanoes (280–740 m Water Depth)
Most of the recent investigations of Arctic seeps have been carried out in the European Arctic and particularly within the Barents Sea. However, some biological data exist on seep systems in the North American Arctic. A number of mud volcanoes have been described and examined at water depths ranging from 282 to 740 m on the Canadian shelf and extending down to the slope, offshore the outflow of the Mackenzie River (Figure 1; Paull et al., 2007, 2015). These mud volcanoes are about 600 m to 1 km in diameter and rise up to 30 m above the seafloor. Coring into the shelf revealed the presence of ice-bonded permafrost and methane hydrates hundreds of meters to over a kilometer below the sediment surface. Though multiple mud volcanoes have been recorded and investigated in this region, one, at about 420 m water depth, has been targeted for biological studies (Paull et al., 2015; Lee D. H. et al., 2019, Lee Y. M. et al., 2019).
Energy Sources and Trophic Pathways at Arctic Seeps
Chemosynthesis-Based Primary Production
The seepage of methane from subsurface hydrocarbon reserves results in methane-enriched fluids emanating from the seabed at cold seeps. As methane seeps upward from subsurface sediment reserves, sulfate migrates downward in the sediment from the overlying water column. These two compounds are oxidized and reduced, respectively, in a tightly coupled, microbially driven process termed AOM (Boetius et al., 2000; Cavanaugh et al., 2006). This process releases hydrogen sulfide, which is why this compound, along with methane, is abundant in sediment porewater at seep locations. Both methane and hydrogen sulfide are reduced compounds; therefore, the energy release that accompanies their oxidation can be utilized by microbes for carbon fixation via chemosynthesis. A key difference between sulfur oxidizing and methane oxidizing chemosynthetic microbes is their inorganic carbon source. The former relies mostly on either dissolved inorganic carbon in seawater or in sediment porewater. In contrast, methane is an organic compound that provides microbes with both energy and inorganic carbon in a single source. Chemosynthetic microbes can either be free living or reside within or on organisms with which they form a symbiotic relationship. Together, they form the base of a food chain and an ecosystem that revolves around chemical-based autotrophic processes (Fisher, 1996; Sibuet and Olu, 1998; Levin, 2005; Becker et al., 2013).
When chemosynthetic bacteria form close symbiotic associations with animals, those animals essentially function as primary consumers within cold-seep ecosystems. At Arctic seeps, siboglinid worms (Oligobrachia frenulates and the moniliferan Sclerolinum contortum at HMMV, only the former at other sites) are the dominant species and the only confirmed chemosynthesis-based macrofauna and, thus, key players in the food web (Decker and Olu, 2012; Åström et al., 2019; Sen et al., 2019b). They form extensive tufts (sometimes grass like meadows) at Arctic seeps that are clearly visible in images (Figure 2). Though the frenulates (and moniliferans in the case of HMMV) represent the entirety of the macrofaunal chemosynthesis-based community presently known at Arctic seeps, small thyasirids (maximum 5 mm length) are also highly abundant at Arctic seeps (Åström et al., 2016, 2019). The family of Thyasiridae bivalves includes chemosymbiotrophic species associated with sulfur-oxidizing symbionts (Dufour, 2005; Dufour and Felbeck, 2006; Duperron et al., 2013), and a high abundance of thyasirids at seep sites is suggestive of these bivalves harboring chemosynthetic symbionts. Furthermore, thyasirids are extremely flexible regarding the nature of their symbiotic associations and exhibit a wide range of different dietary adaptations, from microbial syntrophy and chemosymbiosis to mixotrophy and heterotrophy (Dando and Spiro, 1993; Dufour, 2005; Taylor and Glover, 2010; Duperron et al., 2013). The two most abundant thyasirid species at Arctic seeps are Mendicula cf. pygmaea and Thyasira gouldi where the former is highly abundant both at Arctic seeps (up to 2,500 ind. m–2) and at non-seep, background locations (up to 2,125 ind. m–2) (Åström et al., 2019). Neither M. cf. pygmaea and T. gouldi are obligately chemosymbiotic (Oliver and Killeen, 2002; Dufour, 2005; Taylor and Glover, 2010), and it has not yet been possible to determine whether the populations of these thyasirids at Arctic seeps are symbiotic. Similarly, Sen et al. (2018a) suggested that Thenea sponges, which were abundant at the Storfjordrenna pingo/mound site, might contain chemosynthetic microbial symbionts. Thus, though carbon fixation and symbiotic chemosynthesis-based primary production is only presently confirmed for siboglinids, there are hints that this might also occur in more taxonomic groups.
Microbial Grazing and Secondary Consumption
Chemosynthetic microbes are not limited to symbiotic associations with animals. They can also be free living at the sediment surface, rocks, and carbonate crusts, or on top of other organisms (Cavanaugh et al., 2006). These strains can thrive at seep conditions to the extent that they form thick, clearly visible mats on the sediment surface (Boetius et al., 2000; Grünke et al., 2012). From a community perspective, such mats provide another pathway to chemosynthetically fixed carbon and organic material since organisms can actively graze microbial mats and sediment (i.e., microbial grazers). At Arctic seeps, this trophic level of microbial grazers appears to be represented most clearly by small (mm)-sized gastropods. At HMMV, the Lofoten canyons, and Vestnesa, rissoid snails (Alvania sp.) constitute this group (Gebruk et al., 2003; Decker et al., 2012; Sen et al., 2019b), whereas at the Bjørnøyrenna crater site, Hyalogyrina snails occupy this niche (Åström et al., 2019). Rissoids (the genus Alvania) have been recorded at non-chemosynthetic habitats in polar areas (Coyle et al., 2007; Meyer et al., 2013), whereas Hyalogyrina has only been reported from reducing environments such as wood-falls, vents, seeps, and whale falls (Marshall, 1988; Braby et al., 2007; Sasaki et al., 2010). At HMMV, Decker and Olu (2012) reported carbon isotope values as low as −46.6‰ in Alvania sp. located in microbial mats, while snails in the adjacent sediment had heavier values, of about −40.2‰. Both of these values are suggestive of chemosynthesis-based carbon (CBC) in their diets, and indeed, it was estimated based on a two-source food web model that 21–66% of the carbon uptake of these snails is from CBC (Decker and Olu, 2012). Carbon isotopic signatures of Hyalogyrina gastropods among mats of filamentous bacteria at Bjørnøyrenna crater seeps (δ13C = −23.8‰) (Åström et al., 2019) were heavier than in the alvanids at HMMV, but were also indicative of partial uptake of CBC in their diet (4.8–21.7%) based on a two-source food web model using local end-member values. In addition, these snails are highly abundant at Arctic seeps, particularly among microbial mats, which further suggests that they are grazing on the mats to a certain extent. In addition to small gastropods, other species and faunal groups likely graze on microbial mats as well, such as other mollusks and small polychetes (Levin et al., 2017). However, the link between chemosymbiotic primary producers (microbial associations) and primary consumers at cold seeps (i.e., small microbial mat grazers) is not well represented in seep food-web studies, so far, presumably because organisms in the latter niche are difficult to collect.
More conspicuous members of microbial mat grazers are large crustaceans that have been observed among microbial mats at certain Arctic seeps. For example, at the Storfjordrenna pingo/mound site, snow crabs (Chionoecetes opilio) were seen among mats in positions that suggest they are actively feeding on the bacteria (Sen et al., 2018a). Microbial grazing by crabs is known from seeps at the Costa Rica Subduction Zone where lithoid crabs intensely graze mats of Epsilon proteobacteria, and from seeps along the North American west coast, where tanner crabs (Chionoecetes tanneri) have been observed feeding on microbial mats (Niemann et al., 2013; Seabrook et al., 2019). For both species, compound-specific stable isotope analysis revealed that there was a partial microbial (and ultimately chemosynthesis based) input into their diet (Niemann et al., 2013; Seabrook et al., 2019). At seeps along the North American east coast, Turner et al. (2020) analyzed red crabs (Chaceon quinquedens) using bulk stable isotope analysis of carbon, and it was suggested that free-living chemsoymbiotic bacteria contributed to the crab’s chemosynthetically derived nutrition in addition to bathymodiolin mussels. Hence, it is likely that chemosynthesis-based carbon may play a role in the diets of snow crabs in areas where their distribution overlaps with that of cold seeps.
Secondary and higher-order consumers at Arctic seeps are diverse and represent various taxonomic groups (Decker and Olu, 2012; Sen et al., 2018a; Åström et al., 2019). Within the seep environment, some of these taxa appear to display specific preferences for seep-associated habitats such as microbial mats, siboglinid tufts, and methane-derived authigenic carbonates, i.e., carbonate outcrops (carbonate precipitates that form due to AOM). Pycnogonids (Nymphon hirtipes) were documented at the Lofoten canyon site (overall abundance for the canyons ∼35 ind.m–2), and they were concomitantly observed among microbial mats and siboglinid tufts (Sen et al., 2019b). In addition to N. hirtipes, other pycnogonids (Colossendeis sp.) have been documented in association with siboglinid tufts and carbonate outcrops at both Vestnesa seeps and the Storfjordrenna pingos/mounds, where they occur in higher densities in contrast to areas where such features are absent (Åström et al., 2018; Sen et al., 2018a). The northern shrimp Pandalus borealis has been observed aggregating among siboglinid tufts and microbial mats at several of the seeps in the Barents Sea (Sen et al., 2018a; Åström et al., 2019). From the perspective of both predators and scavengers, microbial mats and siboglinid tufts represent potential food-rich locations due to the presence of associated animals (Decker and Olu, 2012; Sen et al., 2018a; Åström et al., 2019). Individual siboglinid tubes have been seen to host a diverse array of fauna, from single-celled epibenthic foraminifera (Cibicidoides), to predatory caprellids and small polychetes (Decker et al., 2012; Sen et al., 2018a, b; Åström et al., 2019). Furthermore, worm tufts are sometimes overgrown by filamentous bacteria (Figures 2A,C,E; Sen et al., 2018b; Åström et al., 2019). Whether the animals associated with the tufts utilize them as substrate, refuges for hiding and/or resting (i.e., attracted by the seafloor heterogeneity that the tufts provide), or whether the tufts serve as feeding grounds for motile predators is an intriguing question. It is evident, however, that both siboglinid tufts and microbial mats attract certain organisms (from meiofauna to megafauna) at the Arctic seeps (Decker et al., 2012; Åström et al., 2018, 2019; Sen et al., 2018a, 2019b).
Chemosynthesis-based carbon (CBC) has also been found as a source of nutrition in predators at Arctic seeps. Carbon signatures (δ13C = −31.4‰) in predatory polychaetes (Nephtys sp.) suggest that CBC comprises up to 28% to 41% of their diet at Bjørnøyrenna crater seeps based on a two-source food web model (Åström et al., 2019). Likewise, carbon signatures (δ13C = −29.1‰) in Scoletoma fragilis from the Storfjordrenna gas hydrate mounds indicate partial input of CBC (18–32%) with the same two-source model (Åström et al., 2019). At HMMV, Gebruk et al. (2003) found depleted carbon isotope signals (δ13C = −44.9‰) in caprellids, indicating CBC in their diets. Moreover, they suggested that eelpouts (Lycodes sp.) prey directly on siboglinids based on depleted carbon isotopic signals in tissue (δ13C = −51.9‰) and stomach contents that included fragments of frenulate tubes. In addition to the reports at HMMV from Gebruk et al. (2003), Decker and Olu (2012) noted as well depleted carbon isotope signals in samples from sea spiders and amphipods, indicating substantial input of CBC (41–77%) for these taxa. There are a few studies of animal tissues at seep locations that detect chemosynthesis-based carbon beyond obligate chemosymbiotrophic animals and primary consumers (e.g., MacAvoy et al., 2003; Becker et al., 2013; Zapata-Hernández et al., 2014; Portail et al., 2016). Indeed, even at Arctic seeps, most of the assessed organisms have carbon isotopic signatures that indicate that most nutrition originates from photosynthetically derived carbon (Decker and Olu, 2012; Åström et al., 2019). Nevertheless, chemosynthetically derived carbon is detectable up the food chain at Arctic seeps (Gebruk et al., 2003; Decker and Olu, 2012; Åström et al., 2019). Advances in the use of bulk stable isotope analyses, combined with the application of compound-specific isotope and fatty acid analyses, have revealed new insights in benthic food webs (Reeburgh, 2007; Niemann et al., 2013; Seabrook et al., 2019) and could, thus, reveal new information about food web interactions, Arctic seep benthos, and carbon-source utilization.
Key Ecological Aspects of Arctic Seeps
Siboglinids at Arctic Seeps
In contrast with lower latitude seeps, there is an absence of hallmark chemosymbiotic species at Arctic seeps, such as vestimentiferan worms, vesicomyid clams, and bathymodioline mussels. At all Arctic seeps studied to date, chemosynthesis-based symbioses between macro/megafaunal taxa and bacteria are restricted to the siboglinid clade. At HMMV, both moniliferans and frenulates are present, with the former being the more abundant of the two (Gebruk et al., 2003). At other Arctic seeps, obligate chemosymbiotrophic fauna are restricted to frenulates and, specifically, to a complex of closely related Oligobrachia frenulates. Currently, across all Arctic seep sites, three morphologically cryptic species have been identified based on mitochondrial COI sequences: O. haakonmosbiensis (present at HMMV, the Lofoten canyons and Vestnesa), Oligobrachia sp. CPL-clade (present at Storfjordrenna pingos/mounds, Bjørnøyrenna craters, Laptev Sea, and the Beaufort Sea mud volcanoes) and a third, unnamed Oligobrachia species found at Vestnesa (Sen et al., 2018b, 2020; Lee Y. M. et al., 2019). Collections in the Bjørnøyrenna flare area have additionally revealed other frenulates, but no descriptions or identifications have been made for them (Åström et al., 2016, 2019). Therefore, among the multiple seep sites studied across the Arctic, merely three Oligobrachia frenulates and one moniliferan species have been confirmed to constitute the chemosynthesis-based megafauna, and dominant biomass (Table 1). This is a distinctive and highly visible feature of Arctic seeps and one that sets them apart from cold seeps in other parts of the world.
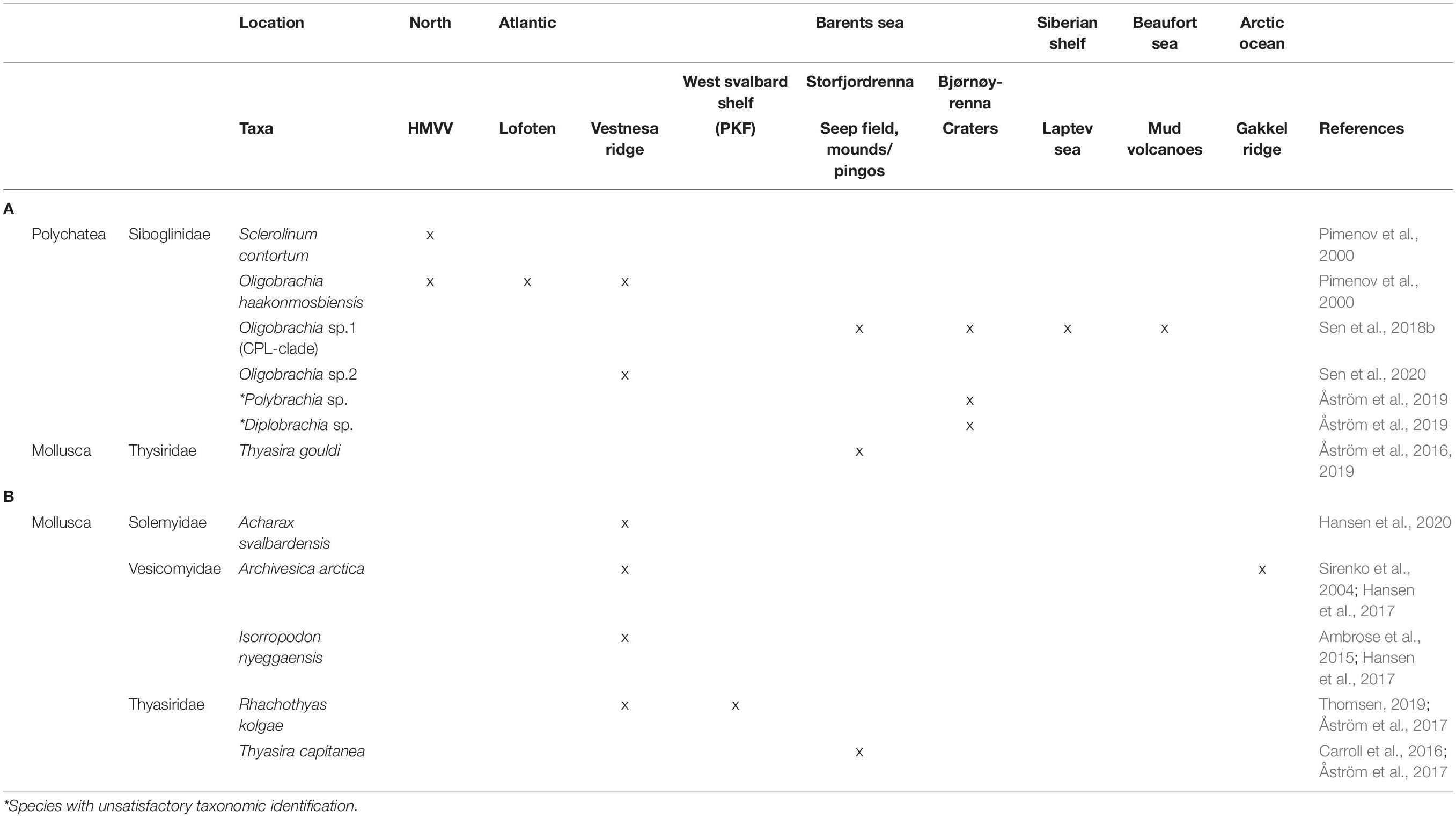
Table 1. (A) Modern symbiotrophic taxa identified at Arctic cold seeps; locations where taxa are present are marked as “x.” (B) Recent fossil (records younger than ∼20,000 years B.P.) of chemosymbiotic taxa identified from Arctic cold seep sediments.
A notable deviation from the generalized Arctic seep community pattern of chemosynthesis-based siboglinids and background benthic species are the shallow water seeps (∼80 m water depth) at Prins Karls Forland (PKF). No siboglinid worms have been observed at these sites, and overall community composition is different, characterized by high abundances and biomass of bivalves and echinoderms in relation to other Arctic seeps (Paull et al., 2015; Åström et al., 2016, 2019; Demina and Galkin, 2018). Shallow water sites tend to have fewer specialist species and chemosymbiotic taxa compared to deeper water seeps (Tarasov et al., 2005; Dando, 2010). Increased predatory pressure and higher input of photosynthetic material have been hypothesized to drive this difference and select against a dominance by specialist fauna and chemosynthesis-based taxa (Sahling et al., 2003; Dando, 2010). Despite a highly limited overall chemosymbiotic faunal inventory at Arctic seeps, this is, nonetheless, a reasonable explanation for the absence of siboglinid worms at shallow PKF seeps. Indeed, single records of siboglinids have been recovered from samples taken at deeper sites (240 m) at PKF (Åström et al., 2016). Oceanographic conditions at PKF, including high-velocity currents and sedimentary properties (gravelly, stony sediment versus soft-bottom sediments) could also account for the differences in PKF relative to other Arctic seeps. Detailed ecological assessments of all PKF sites where seepage is confirmed have not been conducted. Therefore, it is difficult to make comparisons with other Arctic seep sites and even along a depth gradient of the PKF shelf. In contrast to PKF, the shallow (70 m) Laptev Sea site shares a number of characteristic fauna with other Arctic seeps, including the high dominance of siboglinid worms (Åström et al., 2016; Demina and Galkin, 2018). Hence, for the Laptev Sea seep, depth alone is not an environmental barrier to exclude the presence of siboglinids (Demina and Galkin, 2018; Savvichev et al., 2018).
High Taxonomic Richness of Background Fauna
Arctic seeps often exhibit relatively higher taxonomic richness than the nearby surrounding non-seep benthos (Åström et al., 2018; Sen et al., 2018a, 2019a). Biomass and abundance are also higher at Arctic seeps compared to non-seep locations in similar areas (Carroll et al., 2008; Cochrane et al., 2012; Åström et al., 2019; Figure 3). The relatively high taxonomic richness of Arctic seeps in relation to background, non-seep locations is likely linked to the cold-seep communities being composed almost entirely of background fauna (i.e., no obligate seep taxa) that are typical for the Arctic region. With limited exceptions, including some of the Oligobrachia species that might potentially be obligate to seeps and some Alvania snails that have been suggested to possibly exclusively inhabit seep systems (Decker and Olu, 2012), taxa inhabiting Arctic seeps are extensions of the larger community of background Arctic benthos. This is a distinctive feature of Arctic seeps that contrasts with those at lower latitudes where seeps support a subset of the benthos taxa dominated by obligate seep or chemosymbiotrophic taxa. Arctic seeps, however, do not appear to host obligate seep fauna, and instead, are richly populated by diverse assemblages of benthic taxa at higher densities and abundances than the surrounding seafloor.
The Role of Carbonate Structures at Seeps
A key feature of many cold-seep systems is methane-derived authigenic carbonate outcrops. These features precipitate due to the AOM-sulfate reduction reactions that occur in the sediment. At cold seeps, carbonate outcrops generate complex spatial patterns on the seafloor (Cordes et al., 2010; Levin et al., 2017), and the 3D structures of carbonates provide refuges for organisms to hide or escape from predation (Levin et al., 2017; Åström et al., 2018). Carbonate outcrops contribute to enhanced biomass and megafaunal diversity of Arctic cold seeps. Several motile megafaunal taxa, such as red rockfish, eelpouts, wolfish and sea spiders, were commonly observed in and among the carbonate structures (Åström et al., 2018; Sen et al., 2019a), and the carbonates provide extensive hard surfaces for various sessile hard-bottom background species in a predominantly soft-bottom seafloor (Åström et al., 2018; Sen et al., 2018a; Figure 4). Ice rafted debris (drop stones) from icebergs and glacial deposits, which are randomly located on the Arctic seafloor, have been recorded to function as islands within Arctic soft-bottom habitats (Soltwedel et al., 2009; Schulz et al., 2010). In comparison to drop stones, carbonates provide much larger habitat areas and volumes. Furthermore, carbonate outcrops in combination with other large bathymetric features, such as pockmarks and mounds, can create refuges for sensitive, slow-growing hard-bottom fauna (e.g., corals and sponges), especially in areas with intense trawling activities (Webb et al., 2009; MacDonald et al., 2010; Clark et al., 2016). The habitat complexity and physical structures that carbonates provide at seeps cause a so-called “reef-effect” (Stone et al., 1979) where background motile organisms can aggregate regardless of seepage (Hovland, 2008; MacDonald et al., 2010). Since carbonates persist after seepage ceases, the effect of seeps on the seafloor can outlive the seep itself (Bowden et al., 2013; Levin et al., 2016). This is demonstrated by the Svanfjell seep site in the southern Barents Sea, where low levels of seepage activity suggest a senescent stage and limited chemosymbiotic-based production. Nonetheless, taxonomic richness and abundance are still considerably higher at the seep compared to the adjacent non-seep seafloor and appears to be linked to carbonate availability at the seep location (Sen et al., 2019a). Thus active, senescent, and even inactive seeps function as locations where benthic animals aggregate on the Arctic seafloor (Bowden et al., 2013; Sen et al., 2019a). These effects of carbonates are not unique to Arctic seeps (Bowden et al., 2013; Levin et al., 2016, 2017); for example, seep-derived carbonates have been documented to enhance the settlement of large reef-building animals such as cold water corals (Cordes et al., 2006, 2008; Becker et al., 2009). In the Arctic, though, this has particularly important implications, since Arctic seeps function as local diversity and biomass “hotspots” for Arctic benthos, and not only attract a specialized subset of taxa. Accordingly, this is another important consideration for ecosystem management because it means that seep locations, regardless of activity, have the potential to lead to increased benthic diversity and biomass on the Arctic seafloor (Webb et al., 2009; Åström et al., 2018; Sen et al., 2018a, 2019a).
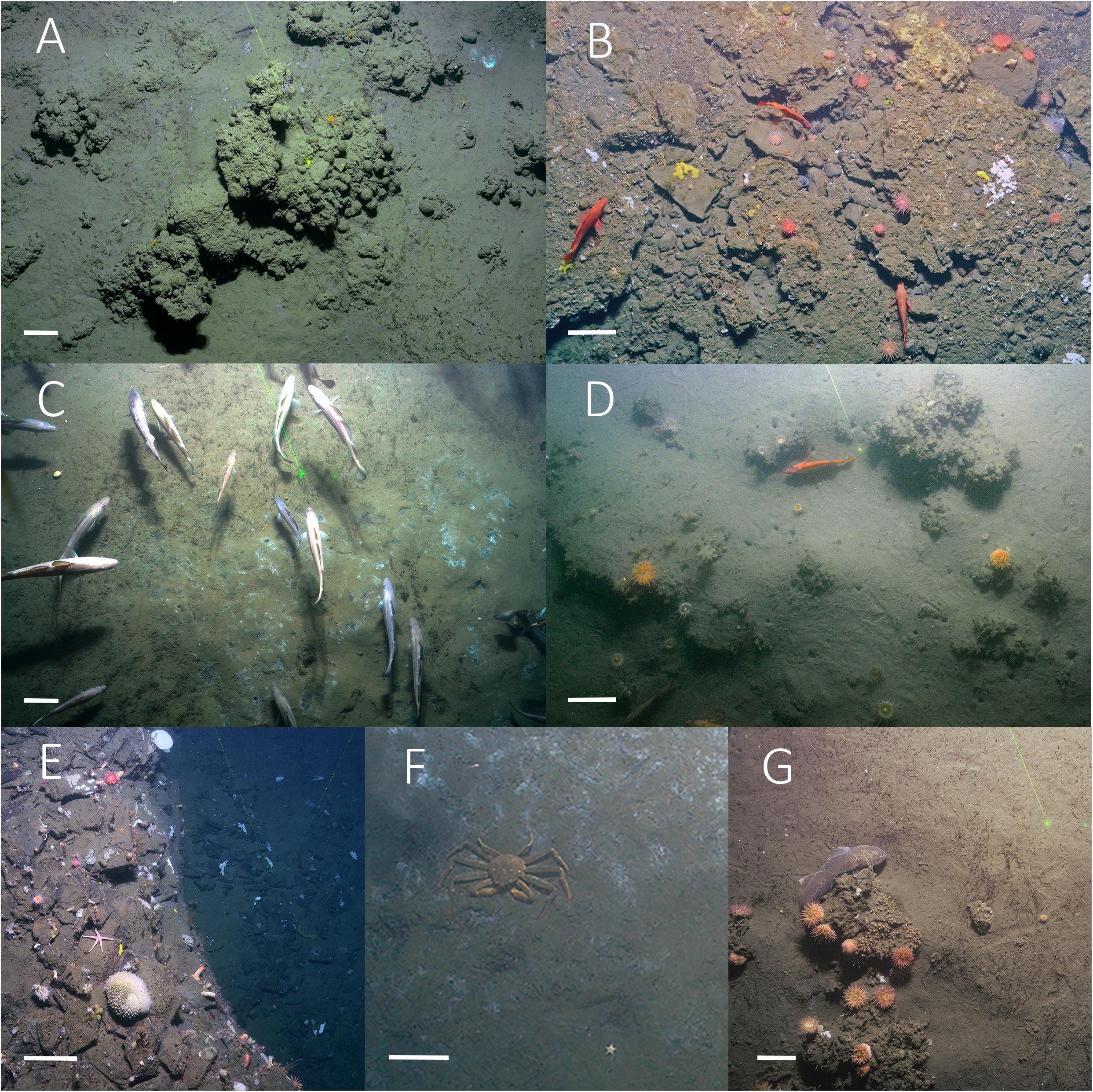
Figure 4. Arctic seep habitats; heterogeneity, carbonate outcrops, and commercial species, from top left to right. (A) Carbonate outcrops, siboglinid tufts, and microbial mats at the Vestnesa seep (1,200 m) from Åström et al. (2018). (B) At the Prins Karls Forland shelf (240 m); large carbonate outcrops and glacial debris provide hard surfaces for epifauna and a varied habitat for Red rock-fish (Sebastes sp.) and spotted wolffish (Anarhichas minor), from Åström (2018). (C) A school of cod (Gadhus morhua) swimming over mats of microbes and siboglinids at the Storfjordrenna gas hydrate mounds/pingos (380 m), from Åström (2018). (D) Red rock-fish (Sebastes sp.) and carbonate outcrops at Storfjordrenna seep field (350 m), from Åström et al. (2019). (E) Epifaunal organisms on a rocky slope into one of the craters at the Bjørnøyrenna crater seeps (330 m), from Åström et al. (2019). (F) A snow crab feeding in a microbial mat at Storfjordrenna gas hydrate mounds/pingos (380 m), from Sen et al. (2018a). (G) A spotted wolffish (A. minor) carbonate crust with epifaunal anemones, hydroids, and solitary corals surrounded by siboglinid tufts at Storfjordrenna gas hydrate mounds/pingos (380 m), from Åström et al. (2019). Scale bars indicate 20 cm.
Similarity of Seep Communities Across Geographic Regions
Despite a lack of obligate seep species, Arctic seep communities stand out as distinct from the surrounding benthic communities. Species composition alone does not contribute toward Arctic seep communities being different from background communities. It is the number of species present and the abundances of respective individual taxa that distinguish Arctic seeps from other non-seep locations. The influence of seepage to benthos is so distinctive that benthic communities at strongly influenced seep locations separated across large geographic areas are more similar to each other than to non-seep communities within the same geographic area (Åström et al., 2019). For example, seep-community composition and structure at the Barents Sea differ significantly from PKF seeps at W. Svalbard seep communities despite intense and active seepage at both locations (Sahling et al., 2014; Andreassen et al., 2017; Serov et al., 2017). At PKF seeps, the seep community is composed of background species and characterized by high densities of ophiuroids and high biomass of mollusks and various epifauna. In contrast, to Barents Sea seeps (pingos, mounds, and craters), host thousands of individuals of siboglinids and small thyasirid bivalves (Åström et al., 2016). The disparity in the community structure has been attributed mainly to differences in the oceanographic settings among the regions and depth; hence, significant differences in faunal composition is expected (Åström et al., 2016). However, within the Barents Sea, the distinct characteristics of Arctic seep communities override such large-scale geographic patterns. Storfjordrenna and Bjørnøyrenna sites are both located in the Barents Sea, south of Svalbard, yet their “background” benthic communities are distinct from one another despite similar water depths and sediment properties (Carroll et al., 2008; Cochrane et al., 2012; Åström et al., 2019). Conversely, the seep characteristics of Storfjordrenna and Bjørnøyrenna cold seeps are highly similar to each other, and strongly influenced seep locations are dominated by siboglinids and thyasirids. The Bjørnøyrenna crater seeps and the Storfjordrenna seep communities are separated by a distance over 300 km, yet the communities are regulated by a seepage signal strong enough to override faunal and biogeographical aspects within the Barents Sea. These distant seep communities, hence, are more similar to each other than they are to background benthic communities located just meters away from the impact of seepage (Åström et al., 2019).
Presence of Commercial Species
A noteworthy aspect of Arctic seep communities is the prevalence of several commercially important taxa. The Northern shrimp (Pandalus borealis), and fish such as Atlantic cod (Gadus morhua), saithe (Pollachius virens), haddock (Melanogrammus aeglefinus), Greenland halibut (Reinhardtius hippoglossides), red rockfish (Sebastes sp.), wolffish (Anarhichas), and even snow crabs (Chionoecetes opilio) have been recorded at Arctic seep sites (Åström et al., 2018, 2019; Sen et al., 2018a). Shrimp and cod are particularly numerous, especially at shelf seep sites (Sen et al., 2018a). Commercially harvested species have been recorded from cold seeps around the world (Bowden et al., 2013; Niemann et al., 2013; Zapata-Hernández et al., 2014; Higgs et al., 2016; Seabrook et al., 2019; Turner et al., 2020), but more often, single or few commercial species tend to be associated with a specific seep site or region. In the Arctic, multiple commercial species occur and overlap at individual seep locations (Figure 4). We suggest that the large-scale heterogeneity caused by the carbonate rocks (the “reef effect”) (Stone et al., 1979) is one reason for the high occurrence of individuals (Åström et al., 2018). Second, it is likely that these large motile and predatory organisms exploit the seeps because of localized and ample food resources. We, furthermore, suggest that the presence of multiple commercially important species co-occurring at the seeps, first and foremost at the shelves, is a consequence of the relatively shallow depths of the seeps and the rich and productive fishing grounds on the Barents Sea shelf (Kjesbu et al., 2014; Haug et al., 2017). It remains, however, unclear to what extent Arctic seeps contribute toward the maintenance of commercial stocks that inhabit the Arctic marginal seas.
Arctic Seeps Can Function as Nursery Grounds
The Lofoten canyon seep site was discovered to function as an egg case nursery for the Arctic skate, Amblyraja hyperborea (Sen et al., 2019b). The canyon setting alone was ruled out as the primary reason behind the Lofoten canyons serving as an egg case nursery ground. Egg cases were highly concentrated among seep locations and were notably rare or absent in areas with no visual evidence of seepage (presence of microbial mats or worm tufts). Surprisingly, egg cases were not associated with carbonate rocks, an observation that contrasts with observations at the Concepción seep offshore Chile, which is the only other seep recorded as being used as a skate egg case nursery ground (Treude et al., 2011). Ambient bottom water temperatures at the Lofoten canyons are lower than 0°C; therefore, a likely explanation is that seepage-related heat releases allow for the site to serve as a natural incubator for egg cases. Though seeps have earned the “cold” moniker because they do not exhibit the boiling temperatures of hydrothermal vents, they are neither particularly cold nor colder than the surrounding seafloor. In fact, the opposite is true: “cold seeps” can be slightly warmer than the background ambient bottom waters, albeit the temperature difference is small, typically less than 1°C (Sibuet and Olu, 1998). The link between temperature increase and nursery habitats has also been observed at low-temperature zones of a Galapagos hydrothermal vent, suggested to function as natural incubators for egg cases of deep water skates (Salinas-De-León et al., 2018). Ambient water temperature at the vent site was measured to ∼2.76°C, and the largest number of skate egg cases was observed in bottom water temperatures where there was an increase of ∼0.25°C. Such temperature anomalies closely match the low-scale temperature anomalies of seeps, which, in subzero conditions, are particularly relevant, first, because they would result in positive bottom water temperatures, and second, because even small increases in temperatures have been modeled to decrease the long incubation times of deep-water skates (Berestovskii, 1994; Hoff, 2010). Therefore, it is possible that the attraction of the Lofoten canyon seep site as a place for Arctic skates to deposit their egg cases might be the slightly warmer environment relative to the surrounding conditions. There are, furthermore, numerous reports of skates from HMMV (Rybakova et al., 2013), although whether this site also functions as an egg case nursery ground for skates is yet to be determined. Nonetheless, the ability for seeps to provide some degree of temperature increase could potentially benefit Arctic benthic fauna, especially for species with long incubation times, in ways previously not considered, such as serving as skate egg case nurseries.
Another hypothesis for high densities of elasmobranch egg cases and other egg-brooding deep-sea fauna in habitats such as seeps and vents, in addition to the higher bottom water temperatures, is the potential increase in available food resources and structures for shelter to hatched juveniles (Drazen et al., 2003; Treude et al., 2011; Turner et al., 2020). For the management of deep-sea ecosystems, in particular, for vulnerable species with long maturation and incubation times, deep-sea nursing habitats could be of high relevance for long-term monitoring and conservation (Drazen et al., 2003; Clark et al., 2016; Da Ros et al., 2019).
Arctic Seeps: Implications for Arctic Ecology
The ecological features discussed above have several implications for seep and Arctic research. The absence of living chemosymbiotic megafauna (e.g., hallmark taxa), the lack of obligate seep taxa, and the high diversity in addition to high biomass and abundance of Arctic seeps compared to background communities are features that are shared across Arctic seeps, though, different from seeps in other parts of the world. Therefore, Arctic seeps deviate from generalizations of cold-seep ecosystems and represent a novel aspect of seep ecological understanding (Sibuet and Olu, 1998; Vanreusel et al., 2009; Levin et al., 2016). The theoretical importance of Arctic seeps is not limited to seep or chemosynthesis-based research; it is also highly relevant to Arctic ecology. For example, the role of Arctic seeps as seafloor oases for a large variety of benthic species, not just for a small group of specialized animals, means that seeps impact the benthos in various ways and across different scales (Bowden et al., 2013; Levin et al., 2016). Simple differences in community structure to larger-scale processes such as food production, carbon transfer, storage, carbon cycling, etc., are all likely to be connected to seeps and need to be considered in the ecology of the Arctic (Figure 5). Furthermore, the presence of numerous commercial species means that Arctic seeps could also be important from a management perspective. In short, studies from these multiple Arctic seep sites indicate the importance of seeps for Arctic biology and ecology. We discuss below some of the implications of these results, and some questions that arise both from a theoretical perspective, as well as with respect to the Arctic and its future.
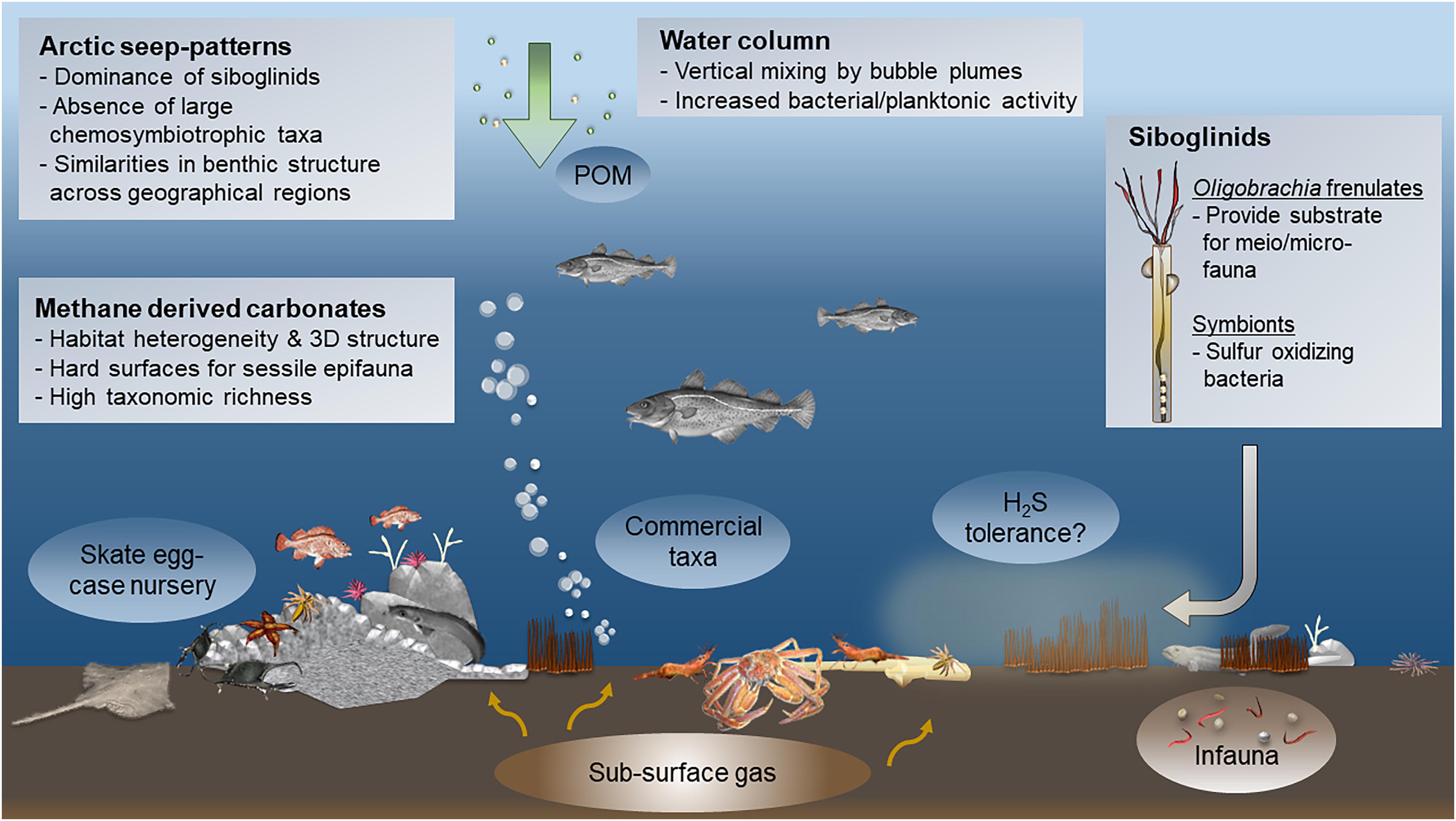
Figure 5. Graphic representation of the key elements of Arctic seeps; a schematic overview of community patterns and characteristics found in Arctic seep habitats. Note that not all patterns are necessarily observed within one single cold-seep site. POM - particulate organic matter.
How Do Seeps Fit Within the Highly Seasonal Arctic Paradigm?
Marine ecosystems in the high Arctic are driven by several processes that are absent outside the polar realm. The presence of sea ice during all or part of the year and intense seasonality in physicochemical features leads to strongly episodic production of organic material, with intense seasonal blooms of primary production in the euphotic zone interrupting extended periods of little or no new production (e.g., Wassmann et al., 2006). Benthic consumers are adapted to this feast-or-famine pattern through pelagic–benthic coupling and vertical transport of organic matter, with seabed community composition and ecological functioning reflecting the spatial and temporal dynamics of organic matter on the surface of the Arctic Ocean, as mitigated through consumers in the pelagic zone (Ambrose and Renaud, 1997; Renaud et al., 2008; Smith et al., 2012).
Chemosynthetic production associated with cold seeps occurs on different spatial and temporal scales than Arctic photosynthetic processes (Jerosch et al., 2007; Bowden et al., 2013; Campanyà-Llovet and Snelgrove, 2018). Such autochthonous production is largely independent of the vagrancies and seasonal cycles of the photosynthetic production at the surface ocean. To the extent that organic matter derived from chemosynthetic production makes its way out of the microbes and chemosymbiotic species to the community at large, this pathway provides a more temporally consistent supply of food resources throughout the year. Further, since locally produced at the seabed, chemosynthetic-based organic matter is not first available to the pelagic grazers (i.e., zooplankton and other planktonic grazers), whose efficiency at consuming and reprocessing surface production often leads to degraded organic material by the time it reaches the seabed. Hence, the chemosynthetic in situ sea bed production can have high relevance especially in systems with low photoautotrophic primary production (oligotrophy) (Carlier et al., 2010). Chemosynthesis-based production bypasses this pelagic filter and, therefore, provides a more-or-less temporally steady source of high-quality unprocessed food for the benthic community that can mitigate the seasonal fluctuations of food availability to Arctic benthic communities.
Are Populations of Hallmark Chemosymbiotic Seep Taxa Absent at Arctic Seeps?
A distinct feature of Arctic seeps is the absence of the hallmark chemosymbiotic species (e.g., vesicomyid clams, vestimentiferan worms, and bathymodioline mussels). No evidence has yet been found for the latter two groups at Arctic seeps in recent geological time (Hryniewicz et al., 2019). Vesicomyid shells have, however, been recovered from several Arctic seep locations (Hansen et al., 2017 and references herein) (Table 1). Dating of vesicomyid shells suggest that these chemosymbiotic bivalves disappeared from Arctic seeps approximately 15,000 years ago (Ambrose et al., 2015; Hansen et al., 2017, 2020). Since carbonates with preserved remains of frenulate tubes from Arctic seep sites have been dated to a minimum of 20,000 years old (Hong et al., 2019), the chemosymbiotic megafaunal communities of Arctic seeps in the past likely consisted of large bivalves along with siboglinids.
It is possible that an event occurred ∼15,000 years ago that led to the disappearance of large chemosymbiotic bivalves (including also solemyids and thyasirids in addition to vesicomyids) from Arctic seeps, leaving siboglinids unaffected (Ambrose et al., 2015; Hansen et al., 2017, 2020). A massive destabilization of gas hydrates triggered by deglaciation, accompanied by decreasing pressure at the seabed, could have resulted in the widespread release of gases. Such events are considered to have been responsible for topographical changes to the Arctic seafloor, including the creation of the blowout craters at Bjørnøyrenna and at the mound/pingos in Storfjordrenna (Andreassen et al., 2017; Serov et al., 2017). A problematic aspect of this hypothesis is the idea that these bivalves would be more vulnerable to such disturbances than the siboglinids, despite some groups such as vesicomyids, having behavioral adaptations for dealing with disruptions such as reorienting themselves in the sediment (Krylova and Von Cosel, 2011).
Another explanation for the absence of large chemosymbiotic bivalves could be the thermal barrier of low-bottom water temperatures in the Arctic. One hypothesis is that chemosymbiotic bivalves (vesicomyids and solemyids) colonized Arctic deep-water seeps during the Heinrich Stadial (HS1), after the most recent glacial period (Hansen et al., 2017, 2020). During this period of time, Arctic surface waters were generally colder, however, bottom water temperature was up to 2°C warmer than today (Rasmussen et al., 2007). When bottom water temperature decreased, resulting in negative bottom sea temperatures that persist at seeps today on the Arctic continental slope, this presumably caused an extinction of distinct chemosymbiotic vesicomyids and solemyids (Sztybor and Rasmussen, 2016; Hansen et al., 2017). This explanation does, however, fail to account for the perceived absence of large chemosymbiotic bivalves from seep sites on the shelf today, where temperatures are low, but nonetheless above 0°C (Åström et al., 2016, 2019; Hong et al., 2017). Vesicomyids have also been found at deep-water sites around the world where temperatures reach only a few degrees above freezing (Fisher, 1990; Taylor and Glover, 2010; Sen et al., 2017).
While it is currently impossible to pinpoint what factor(s) contributed to the disappearance of the large chemosymbiotic bivalves from Arctic seeps, their absence suggests that either the conditions that led to their extinction in the first place persist today, or barriers exist that have prevented recolonization. Since relatively warm, Atlantic water makes its way up past Svalbard and mixes with cold, Arctic water between Svalbard and mainland Norway (Loeng, 1991), dispersal, at least from the Atlantic to the Arctic, should not be limiting. Furthermore, Atlantic water inflow appears to be effective in transporting other species observed at Arctic seeps, namely, Oligobrachia worms (Sen et al., 2020). Additionally, the small thyasirids that have been collected from Arctic seeps today are also known to be present further south in the Atlantic (Oliver and Killeen, 2002; Decker et al., 2012; Åström et al., 2017).
Alternatively, some of these taxa might have re-populated Arctic seeps or never went extinct at all. A few shells of the large, presumably chemosymbiotic, species of thyasirids recovered from Storfjordrenna seeps reveal relatively modern and young ages (in comparison to the other large bivalves mentioned above), of about 500–1,300 years before present (Carroll et al., 2016; Åström et al., 2017). Therefore, certain taxa could have been present until just recently, and the question even arises as to whether they are currently present at Arctic seeps but have simply not yet been collected alive.
Are Seeps Stepping Stones for Arctic Benthic Dispersal?
At cold seeps, methane plays a key role in the process of chemosynthesis, although sulfide functions as the critical compound in shaping the benthic community. Sulfide is toxic to oxygen-breathing life forms (Vismann, 1991; Fisher and Childress, 1992), and tolerance to sulfide exposure requires special binding proteins that have evolved in animals specialized for a life in sulfide-rich habitats (Terwilliger, 1998). For motile animals, sulfide toxicity can be avoided by moving away from the most sulfidic areas while remaining in the seep vicinity. This behavioral strategy is not uncommon and is a possible explanation that no seep system in the world is populated solely by obligate seep species. As a result, vagrant, opportunistic background species are common residents around seeps (Bowden et al., 2013; Zapata-Hernández et al., 2014; Levin et al., 2016).
Less motile benthic animals populating Arctic seep sites may have acquired necessary physiological adaptations to protect them from the toxic effects of sulfide. Adaptations for prolonged sulfide exposure are, however, costly and, therefore, unexpected in background, non-seep obligate species (Sen et al., 2019b). Conversely, Arctic seasonality and the resulting episodic input of photosynthetic food to the benthic realm may have selected for adaptations in dealing with sulfide that are normally found in species endemic to, or specialized for, reducing environments (Sen et al., 2019b). This would infer an advantage in allowing them to utilize a food resource that is stable throughout the year (Åström et al., 2018). However, many Arctic seeps are relatively young in evolutionary terms, given the past glacial history of the Arctic and the Northern hemisphere (Stokes et al., 2015; Patton et al., 2017). The most recent deglaciation of the Arctic ice sheets started approximately 20,000 years cal. BP (Rasmussen et al., 2007; Patton et al., 2017; Margold et al., 2018), and a large part of the Arctic was not ice free until several thousand years later (Ingólfsson and Landvik, 2013; Andreassen et al., 2017; Margold et al., 2018). This is a short time frame for organisms to evolve both to the extremes of an Arctic environment and to cope with the presence of sulfide at seep habitats.
Given the ecological patterns of Arctic cold seeps, most benthic taxa found at seep sites in the Arctic are species that commonly occur at Arctic non-seep habitats (i.e., background fauna). Only a small subset of the species at Arctic cold seeps are chemosymbiotrophic or organisms recognized from other reduced or chemosynthetic habitats (Decker and Olu, 2012; Sen et al., 2018a; Åström et al., 2019). For chemosymbiotic species, seeps can function as stepping stones by linking the dispersal of chemosynthetic fauna from source populations at lower latitudes to locations of reduced habitats, seeps, and vents bordering the northern polar region (e.g., Mayer et al., 1988; Sahling et al., 2003; Kiel, 2016; Åström et al., 2017; Sen et al., 2020). In addition, reduced environments associated with large food falls can increase the connectivity between vents and seeps and serve as provisory habitats allowing a northward or circumpolar expansion of taxa (Georgieva et al., 2015; Smith et al., 2015). However, seeps may not only provide gateways of dispersal and connectivity for chemsoymbiotic taxa; the ample resources at seeps could also serve for background, migrating taxa. One sub-Arctic species that has rapidly expanded its geographical range in the Arctic is the snow crab (Chionoecetes opilio). In 1996, it was first documented in the Barents Sea (Kuzmin et al., 1998; Alvsvåg et al., 2009) and has since spread further west and north. Its current distribution reaches as far as northern Svalbard fjords and is currently an established and commercially harvested species in the Barents Sea system (Jørgensen et al., 2019). Snow crabs have been documented at cold seeps in the western Barents Sea where images indicate that they can feed on bacterial mats (Sen et al., 2018a). The presence of snow crabs at seeps and their observed behavior may imply that they are benefiting from resources provided by the seep. The motile snow crab is a typical example of an animal expanding its geographical boundary, while exploiting resources (food) at the seep habitat. Consequently, despite Arctic seeps being extreme habitats, they might play an important role in dispersal and connectivity of various Arctic biota.
How Might Climate Change Influence Arctic Seeps?
Key elements of photosynthetic primary production cycles will be affected as the Arctic warms, sea ice melts, and water mass and nutrient distributions change. This includes changes in the timing and location of surface blooms, the species that drive the blooms, and the match of such photosynthetic primary production to pelagic and benthic consumers (Stenseth and Mysterud, 2002; Wassmann et al., 2006) all with impacts on the availability of organic detritus at the seabed, which fuels benthic communities. Carroll and Carroll (2003) hypothesized that a warmer Arctic with less sea ice may favor pelagic communities at the expense of the benthos, due to the loss of sea-ice algal production. Sea-ice algae has been shown to play a crucial role in the export of organic matter to benthos (i.e., sympagic–benthic coupling) (McMahon et al., 2006; Sun et al., 2007), as large pieces of ice algae could bypass the pelagic filter (Boetius et al., 2013). Cold-seep communities may locally mitigate such a phenomenon by supporting benthic communities when surface-based organic matter is lacking or of poor quality. The existence of seep-related production may locally have a profound impact on the community structure, ecological interactions, and secondary production of the benthos as well as its coupling with the surface ocean (Pohlman et al., 2017; Åström et al., 2018, 2019; Ofstad et al., 2020). In this way, cold seeps can act as productive hotspots in the Arctic and function as a temporal bridge for benthic communities and associated ecosystems that may otherwise suffer from a lack of consistency in food quality from the surface ocean (McMahon et al., 2006). Indeed, by the combination of enhanced in situ organic material production, heterogeneous habitats from the carbonate outcrops, and function as stepping stones for dispersal of species (Smith et al., 2015), the entire vicinity of Arctic cold-seep habitats serve as an oasis for background species in contrast to non-seep locations sharing similar environmental characteristics (Åström et al., 2018).
As the Arctic warms at rates well above the world average, boreal and sub-arctic species are expanding their ranges northward, interacting with, and possibly even displacing, Arctic-adapted species (Fossheim et al., 2015; Frainer et al., 2017; Haug et al., 2017). Whether continued warming and the concomitant biogeographic changes predicted for pelagic and benthic systems will be valid for cold seeps as well is an open question. Cold seeps could act as refuges for Arctic-adapted species otherwise facing variability in the timing and quality of surface-based primary production outside the ranges previously experienced. Conversely, the reduction in the temporal variability in food supply at seeps may favor boreal and temperate species at the expense of Arctic-adapted benthic species that are used in highly episodic food inputs and sub-zero temperatures. Combined with warming sea temperatures, the functional adaptations of the more southerly species may infer a competitive advantage and favor their replacement of Arctic species. Even if Arctic seeps do not experience widespread replacements of boreal species, there is also a possibility of species replacements among Arctic species. If Arctic species are predisposed for dealing with seep conditions, then species whose ranges might be increasing either naturally, or due to human activities, could start colonizing and settling successfully at seeps outside their prior ranges, which could affect local community structures and dynamics.
Another consequence of climate warming is deoxygenation of marine systems as a result of increased pelagic stratification and decreased solubility of gases (Gruber, 2011). Moving from a sea ice-dominated system toward a more open-ocean Arctic combined with changes in seasonal primary production patterns, could lead to a trophic mis-match in pelagic–benthic coupling and eventually increase the input of organic matter degradation and oxygen consumption at the seafloor (Renaud et al., 2007; Sweetman et al., 2017; Durant et al., 2019). Hypoxic seafloor conditions can cause devoid areas, empty of larger life forms, and it is essential to maintain oxygen concentration above crucial levels in order to sustain seep faunal communities (Diaz, 2001; Vaquer-Sunyer and Duarte, 2010). Oxygen is also required for symbionts to carry out the sulfide and methane oxidation processes (Cavanaugh et al., 2006; Dubilier et al., 2008). If seafloor deoxygenation becomes a problem in the Arctic, particularly in enclosed water basins, fjords and estuaries, Diaz (2001) and Liira et al. (2019), one can speculate that organisms inhabiting seeps may have an advantage over others in less oxygenated systems (Pearson and Rosenberg, 1978, Levin et al., 2003; Sweetman et al., 2017). Some organisms, incapable of large-scale movements in and out of habitats can avoid hypoxia by modulating the surroundings to enhance oxygen circulation (i.e., re-working sediment or modulate their position in the sediment–water interface) (Hourdez and Lallier, 2007; Dando et al., 2008; Guillon et al., 2017). Others already have physiological adaptations to cope with such conditions such as increased gill size or oxygen-binding proteins (e.g., Hourdez and Lallier, 2007; Tobler et al., 2016). High densities of taxa that are known to inhabit locations with high organic matter degradation or low-oxygen habitats, at seeps compared to background sediments (Levin et al., 2003; Åström et al., 2016) could potentially indicate that these species may have a better ability to handle deoxygenation as a consequence of a warmer Arctic (Olsen et al., 2007; Norkko et al., 2019).
To summarize, cold seeps in the Arctic serve multiple ecological functions and provide critical ecosystem services (Figure 5). Recently, the Norwegian Environmental Agency recognized cold-seep habitats in the Norwegian marine sector as areas of special interest and vulnerable habitats with high conservation value (Miljødirektoratet, 2019). This recognition of cold-seep habitats, providing substrate and heterogeneity, alternative food resources, and possibly functioning as gateways for dispersal and connectivity of benthic taxa, including commercial species, clearly highlights the key role that Arctic cold seeps serve to the wider marine ecotone.
Conclusion
The physical extremes of the Arctic environment and the chemical extremes and strong environmental gradients of cold-seep systems are two primary drivers that interact in the Arctic, forming benthic communities that are distinct from not only adjacent Arctic benthic communities but also from lower-latitude seep communities.
Arctic cold seeps are conspicuously lacking in large and charismatic obligate chemosymbiotrophic faunal species that are characteristics of lower-latitude seeps. The dominant and distinct chemosymbiotic fauna at Arctic seeps is largely limited to Oligobrachia frenulates (with the addition of the moniliferan, S. contortum at HMMV). These frenulates, however, form incredibly dense tufts that are a defining biological feature of Arctic cold seeps. They alter the sediment stability, biogeochemistry, and are likely a key link between chemosynthetic and heterotrophic fauna.
Arctic seep communities support different community structure characteristics and have far-reaching trophic effects, as both diversity, abundance, and biomass are higher at Arctic seeps than at background communities (Levin et al., 2016). Importantly, this enhanced diversity and high abundance and biomass consists mainly of background, benthic species, and specific seep obligate fauna are almost entirely lacking.
The interaction of the few obligate chemosymbiotrophic species with the larger background benthic community is one of the key features of Arctic seep systems. It is the combination of autochthonous production and enhancement of the seabed heterogeneity that provides a dual basis for seeps’ ability to attract and accumulate biomass and diversity in a food and substrate-limited biome. The temporal consistency of chemosynthetic-based production is largely independent of the intense seasonality of the phytosynthetic-based production cycle, providing organic matter to the benthos in the larger parts of the year when it would otherwise be low. Arctic seeps, therefore, function as local diversity and biomass hotspots on the seafloor. These hotspots may also serve as geographic stepping stones for dispersal of both chemosynthetic and background species in the larger Arctic Ocean basin.
These features highlight the role of Arctic seeps and demonstrate important baseline insights both to science and to society. Since Arctic seep research is still fairly new, and these findings are known mostly to a niche audience, seeps have not yet been included in policy making or in modeling outcomes of a warming Arctic. It is clear, though, that these habitats are an important ecosystem resource of relevance for conservation and with broader implications for marine ecosystem functioning in the age of global climate change (Renaud et al., 2015; Haug et al., 2017). Recently, they have been granted the status of particularly valuable and vulnerable habitats by the Norwegian government (Miljødirektoratet, 2019).
Future Directions
Despite notable gains in the understanding of Arctic seep systems acquired through recent studies, significant gaps remain. Most Arctic seep research has been conducted in the European Arctic, particularly in Nordic waters, and large parts of the Arctic, despite seeps being present, have not been studied from a biological perspective. Furthermore, seeps fringing but outside the Arctic Circle (e.g., the North Atlantic–Norwegian Sea, Sea of Okhotsk, North Atlantic east, and west coast) have yet to be sufficiently studied (Sahling et al., 2003; Vaughn Barrie et al., 2011; Decker et al., 2012; Skarke et al., 2014). This is of importance since these locations experience some of the extreme phenomena that appear to drive current and past processes at Arctic seeps. Indeed, some of these seep sites also appear to share the community structure seen at Arctic seeps (Sahling et al., 2003; Krylova et al., 2011; Decker and Olu, 2012; Decker et al., 2012; Hansen et al., 2020).
Most biological seep studies address the impact of seeps on the Arctic benthos. However, Vinogradov and Semenova (2000) described the peculiarities of the mesoplankton distribution above HMMV, and Ofstad et al. (2020) measured different pelagic foraminifera and pteropod communities above Barents Sea seep sites. Furthermore, Pohlman et al. (2017) suggest that high methane concentrations and nearshore upwelling stimulate consumption of CO2 by photosynthetic phytoplankton at the shallow PKF seeps. Seasonal ecological studies at Arctic seeps are also lacking, despite the role of seasonality as a key driver of Arctic biological cycles, and the intriguing possibility that chemosynthetic production cycles can have strong effects mitigating such seasonality. Additionally, the investigation of specific trophic linkages between high-trophic level marine resources, their prey, and Arctic seep systems remains in its early stages. Finally, a focus on better understanding the ecosystem services associated with these unique habitats, specifically in an Arctic context, will provide stakeholders a basis of information to assess the possible conservation value of Arctic cold seeps.
With further investigation, we may begin to merge chemosynthesis-based systems in the Arctic and include them as an integral part of the Arctic marine ecosystem. Arctic vent communities seem to be more like Arctic seep communities than Atlantic vents. They share characteristics such as a lack of hallmark species and large symbiotrophic animals and are also mainly comprised of non-specialist, background species (Sweetman et al., 2013). Large “clouds” of planktonic animals have been observed above Arctic vent sites (Schander et al., 2010); therefore, the effects of vents might resemble those of Arctic seeps. The merging of seeps and vents into a single compartment may provide a basis for understanding processes at other Arctic systems where chemosymbiotrophic animals are observed, such as fjords, and generating new ecological perspectives and insights for the larger Arctic ecosystem.
Author Contributions
JC: manuscript initialization. EÅ and AS: conceptualization. AS and EÅ: drafting the manuscript. AS, EÅ, and MC: visualization. All authors: writing, revisions, and edits.
Funding
EÅ was a post-doctoral scholar funded by VISTA – a basic research program in collaboration between The Norwegian Academy of Science and Letters and Equinor (#6172). AS was a post-doctoral scholar at Nord University (Bodø, Norway). JC and MC contributions to this manuscript were funded by the Research Council of Norway (RCN #228107). The publication charges for this article have been funded by a grant from the publication fund of UiT – The Arctic University of Norway.
Conflict of Interest
The authors declare that the research was conducted in the absence of any commercial or financial relationships that could be construed as a potential conflict of interest.
Acknowledgments
We thank Dr. Bodil Bluhm, Dr. Henning Reis, and Dr. Paul Renaud for their support and guidance during the development of this publication. We are also grateful to Dr. Eve Southward and Dr. Paul Dando for sharing their knowledge on siboglinids and geochemistry through extensive discussions over the past few years. Part of the ecological research at the sites described in section 3.2.1 to 3.2.6 has been enabled through CAGE – The Center for Arctic Gas Hydrate, Environment and Climate, UiT – The Arctic University of Norway. Thanks to the two reviewers who helped refine the final manuscript.
References
Alvsvåg, J., Agnalt, A.-L., and Jørstad, K. E. (2009). Evidence for a permanent establishment of the snow crab (Chionoecetes opilio) in the Barents Sea. Biol. Invasions 11, 587–595.
Ambrose, W. G. J., Panieri, G., Schneider, A., Plaza-Faverola, A., Carroll, M. L., Åström, E. K. L., et al. (2015). Bivalve shell horizons in seafloor pockmarks of the last glacial-interglacial transition: a thousand years of methane emissions in the Arctic Ocean. Geochemistry, Geophys. Geosystems 16, 4108–4129. doi: 10.1002/2015GC005980
Ambrose, W. G. J., and Renaud, P. E. (1997). Does a pulsed food supply to the benthos affect polychaete recruitment patterns in the Northeast Water Polynya? J. Mar. Syst. 10, 483–495.
Ambrose, W. G. J., Renaud, P. E., Locke, V., Cottier, F. R., Berge, J., Carroll, M. L., et al. (2012). Growth line deposition and variability in growth of two circumpolar bivalves (Serripes groenlandicus, and Clinocardium ciliatum). Polar Biol. 35, 345–354.
Amon, D. J., Gobin, J., Van Dover, C. L., Levin, L. A., Marsh, L., and Raineault, N. A. (2017). Characterization of methane-seep communities in a deep-sea area designated for oil and natural gas exploitation off trinidad and tobago. Front. Mar. Sci. 4:342. doi: 10.3389/fmars.2017.00342
Andreassen, K., Hubbard, A., Winsborrow, M., Patton, H., Vadakkepuliyambatta, S., Plaza-Faverola, A., et al. (2017). Massive blow-out craters formed by hydrate-controlled methane expulsion from the Arctic seafloor. Science 356, 948–953. doi: 10.1126/science.aal4500
Artcic Monitoring and Assessment Programme [AMAP], (1998). AMAP Assessment Report: Arctic Pollution Issues. Norway: Arctic Monitoring and Assessment Programme, xii+859.
Åström, E. K., Carroll, M., Sen, A., Niemann, H., Ambrose, W., Lehmann, M., et al. (2019). Chemosynthesis influences food web and community structure in high-Arctic benthos. Mar. Ecol. Prog. Ser. 629, 19–42. doi: 10.3354/meps13101
Åström, E. K. L. (2018). Benthic Communities At High-Arctic Cold Seeps: Faunal Response To Methane Seepage In Svalbard. Ph. D. thesis, UiT-The Arctic University of Norway, Tromsø.
Åström, E. K. L., Carroll, M. L., Ambrose, W. G., Sen, A., Silyakova, A., and Carroll, J. (2018). Methane cold seeps as biological oases in the high-Arctic deep sea. Limnol. Oceanogr. 63, S209–S231. doi: 10.1002/lno.10732
Åström, E. K. L., Carroll, M. L., Ambrose, W. G. J., and Carroll, J. (2016). Arctic cold seeps in marine methane hydrate environments : impacts on shelf macrobenthic community structure offshore Svalbard. Mar. Ecol. Prog. Ser. 552, 1–18. doi: 10.3354/meps11773
Åström, E. K. L., Oliver, P. G., and Carroll, M. L. (2017). A new genus and two new species of Thyasiridae associated with methane seeps off Svalbard. Arctic Ocean. Mar. Biol. Res. 13, 402–416. doi: 10.1080/17451000.2016.1272699
Bagarinao, T. (1992). Sulfide as an environmental factor and toxicant: tolerance and adaptations in aquatic organisms. Aquat. Toxicol. 24, 21–62. doi: 10.1016/0166-445X(92)90015-F
Becker, E. L., Cordes, E. E., Macko, S. A., and Fisher, C. R. (2009). Importance of seep primary production to Lophelia pertusa and associated fauna in the Gulf of Mexico. Deep Sea Res. Part I Oceanogr. Res. Pap. 56, 786–800. doi: 10.1016/j.dsr.2008.12.006
Becker, E. L., Cordes, E. E., Macko, S. A., Lee, R. W., and Fisher, C. R. (2013). Using stable isotope compositions of animal tissues to infer trophic interactions in gulf of mexico lower slope seep communities. PLoS One 8:e74459. doi: 10.1371/journal.pone.0074459
Bellec, V. (2015). “The deep sea off lofoten, vesterålen and troms,” in The Norwegian Sea Floor - New Knowledge From MAREANO for Ecosystem-Based Management, eds H. Bhul-Mortenssen and T. Thorsnes, (Berlin: Springer), 61–79.
Berchet, A., Bousquet, P., Pison, I., Locatelli, R., Chevallier, F., Paris, J. D., et al. (2016). Atmospheric constraints on the methane emissions from the East Siberian Shelf. Atmos. Chem. Phys. 16, 4147–4157.
Berestovskii, E. G. (1994). Reproductive biology of skates of the family Rajidae in the seas of the far north. J. Ichthyol. Ikhtiologii. 34:2.
Berge, J., Renaud, P. E., Darnis, G., Cottier, F., Last, K., Gabrielsen, T. M., et al. (2015). In the dark: a review of ecosystem processes during the Arctic polar night. Prog. Oceanogr. 139, 258–271. doi: 10.1016/j.pocean.2015.08.005
Berndt, C., Feseker, T., Treude, T., Krastel, S., Liebetrau, V., Niemann, H., et al. (2014). Temporal constraints on hydrate-controlled methane seepage off svalbard. Science 343, 284–287. doi: 10.1126/science.1246298
Bienhold, C., Pop Ristova, P., Wenzhöfer, F., Dittmar, T., and Boetius, A. (2013). How deep-sea wood falls sustain chemosynthetic life. PLoS One 8:53590. doi: 10.1371/journal.pone.0053590
Black, M. B., Halanych, K. M., Maas, P. A. Y., Hoeh, W. R., Hashimoto, J., Desbruyères, D., et al. (1997). Molecular systematics of vestimentiferan tubeworms from hydrothermal vents and cold-water seeps. Mar. Biol. 130, 141–149. doi: 10.1007/s002270050233
Boetius, A., Albrecht, S., Bakker, K., Bienhold, C., Felden, J., Fernandez-Mendez, M., et al. (2013). Export of algal biomass from the melting arctic sea ice. Science 339, 1430–1432. doi: 10.1126/science.1231346
Boetius, A., Ravenschlag, K., Schubert, C. J., Rickert, D., Widdel, F., Gleeske, A., et al. (2000). A marine microbial consortium apparently mediating AOM. Nature 407, 623–626. doi: 10.1038/35036572
Bowden, D. A., Rowden, A. A., Thurber, A. R., Baco, A. R., Levin, L. A., and Smith, C. R. (2013). Cold Seep epifaunal communities on the hikurangi margin, new zealand: composition, succession, and vulnerability to human activities. PLoS One 8:e76869. doi: 10.1371/journal.pone.0076869
Braby, C. E., Rouse, G. W., Johnson, S. B., Jones, W. J., and Vrijenhoek, R. C. (2007). Bathymetric and temporal variation among Osedax boneworms and associated megafauna on whale-falls in Monterey Bay, California. Deep Sea Res. Part I Oceanogr. Res. Pap. 54, 1773–1791. doi: 10.1016/j.dsr.2007.05.014
Bünz, S., Polyanov, S., Vadakkepuliyambatta, S., Consolaro, C., and Mienert, J. (2012). Active gas venting through hydrate-bearing sediments on the vestnesa ridge, offshore w-svalbard. Mar. Geol. 332–334, 189–197. doi: 10.1016/j.margeo.2012.09.012
Campanyà-Llovet, N., and Snelgrove, P. V. R. (2018). Fine-scale infaunal community and food web patch mosaics from Barkley methane hydrates (British Columbia, Canada): the role of food quality. Deep Sea Res. Part I Oceanogr. Res. Pap. 140, 186–195. doi: 10.1016/j.dsr.2018.06.009
Carlier, A., Ritt, B., Rodrigues, C. F., Sarrazin, J., Olu, K., Grall, J., et al. (2010). Heterogeneous energetic pathways and carbon sources on deep eastern Mediterranean cold seep communities. Mar. Biol. 157, 2545–2565.
Carney, R. S. (1994). Consideration of the oasis analogy for chemosynthetic communities at Gulf of Mexico hydrocarbon vents. Geo. Mar. Lett. 14, 149–159. doi: 10.1007/BF01203726
Carroll, M. L., Åström, E. K. L., Ambrose, W. G. J., Locke, W., Oliver, G. P., Hong, W.-L., et al. (2016). “Shell growth and environmental control of methanophyllic Thyasirid bivalves from Svalbard cold seeps,” in Proceedings of the EGU General Assembly Conference Abstracts EGU General Assembly Conference Abstracts, Vienna.
Carroll, M. L., and Carroll, J. (2003). “The Arctic Seas,” in Biogeochemistry of Marine Systems, eds K. Black and G. Shimmield, (Oxford: Blackwell Publishing), 127–156.
Carroll, M. L., Denisenko, S. G., Renaud, P. E., and Ambrose, W. G. J. (2008). Benthic infauna of the seasonally ice-covered western Barents Sea: patterns and relationships to environmental forcing. Deep. Res. Part II Top. Stud. Oceanogr. 55, 2340–2351. doi: 10.1016/j.dsr2.2008.05.022
Cavanaugh, C. M., McKiness, Z. P., Newton, I. L. G., and Stewart, F. J. (2006). “Marine Chemosynthetic Symbioses,” in The Prokaryotes, eds M. Dworkin, S. Falkow, E. Rosenberg, K.-H. Schleifer, and E. Stackebrandt, (New York, NY: Springer), 475–507. doi: 10.1007/0-387-30741-9_18
Chand, S., Rise, L., Ottesen, D., Dolan, M. F. J., Bellec, V., and Bøe, R. (2009). Pockmark-like depressions near the goliat hydrocarbon field, barents sea: morphology and genesis. Mar. Pet. Geol. 26, 1035–1042.
Clark, M. R., Althaus, F., Schlacher, T. A., Williams, A., Bowden, D. A., and Rowden, A. A. (2016). The impacts of deep-sea fisheries on Benthic communities: a review. ICES J. Mar. Sci. J. Du Cons. 73, i51–i69.
Cochrane, S. K. J., Pearson, T. H., Greenacre, M., Costelloe, J., Ellingsen, I. H., Dahle, S., et al. (2012). Benthic fauna and functional traits along a Polar front transect in the Barents sea - advancing tools for ecosystem-scale assessments. J. Mar. Syst. 94, 204–217. doi: 10.1016/j.jmarsys.2011.12.001
Comiso, J. C., and Hall, D. K. (2014). Climate trends in the Arctic as observed from space. Wiley Interdiscip. Rev. Clim. Chang. 5, 389–409. doi: 10.1002/wcc.277
Cordes, E. E., Bergquist, D. C., Predmore, B. L., Jones, C., Deines, P., Telesnicki, G., et al. (2006). Alternate unstable states: convergent paths of succession in hydrocarbon-seep tubeworm-associated communities. J. Exp. Mar. Bio. Ecol. 339, 159–176. doi: 10.1016/j.jembe.2006.07.017
Cordes, E. E., Cunha, M. R., Galéron, J., Mora, C., Olu-Le Roy, K., Sibuet, M., et al. (2010). The influence of geological, geochemical, and biogenic habitat heterogeneity on seep biodiversity. Mar. Ecol. 31, 51–65. doi: 10.1111/j.1439-0485.2009.00334.x
Cordes, E. E., McGinley, M. P., Podowski, E. L., Becker, E. L., Lessard-Pilon, S., Viada, S. T., et al. (2008). Coral communities of the deep Gulf of Mexico. Deep Sea Res. Part I Oceanogr. Res. Pap. 55, 777–787. doi: 10.1016/j.dsr.2008.03.005
Coyle, K. O., Konar, B., Blanchard, A., Highsmith, R. C., Carroll, J., Carroll, M. L., et al. (2007). Potential effects of temperature on the benthic infaunal community on the southeastern Bering Sea shelf: possible impacts of climate change. Deep. Res. Part II Top. Stud. Oceanogr. 54, 2885–2905. doi: 10.1016/j.dsr2.2007.08.025
Da Ros, Z., Dell’Anno, A., Morato, T., Sweetman, A. K., Carreiro-Silva, M., Smith, C. J., et al. (2019). The deep sea: the new frontier for ecological restoration. Mar. Policy 108:103642. doi: 10.1016/j.marpol.2019.103642
Dando, P. R. (2010). “Biological communities at marine shallow-water vent and seep sites,” in The Vent and Seep Biota Topics in Geobiology, ed. S. Kiel, (Dordrecht: Springer), 333–378. doi: 10.1007/978-90-481-9572-5
Dando, P. R., Southward, A. F., Southward, E. C., Dixon, D. R., and Crawford, A. (1992). Shipwrecked tube worms. Nature 356, 667–667. doi: 10.1038/356667a0
Dando, P. R., Southward, A. J., Southward, E. C., Lamont, P., and Harvey, R. (2008). Interactions between sediment chemistry and frenulate pogonophores (Annelida) in the north-east Atlantic. Deep. Res. Part I Oceanogr. Res. Pap. 55, 966–996. doi: 10.1016/j.dsr.2008.04.002
Dando, P. R., and Spiro, B. (1993). Varying nutritional dependence of the thyasirid bivalves Thyasira sarsi and T. equalis on chemoautotrophic symbiotic bacteria, demonstrated by isotope ratios of tissue carbon and shell carbonate. Mar. Ecol. Prog. Ser. 92, 151–158. doi: 10.3354/meps092151
De Beer, D., Sauter, E., Niemann, H., Kaul, N., Foucher, J. P., Witte, U., et al. (2006). In situ fluxes and zonation of microbial activity in surface sediments of the Håkon Mosby Mud Volcano. Limnol. Oceanogr. 51, 1315–1331. doi: 10.4319/lo.2006.51.3.1315
Decker, C., Morineaux, M., Van Gaever, S., Caprais, J.-C. C., Lichtschlag, A., Gauthier, O., et al. (2012). Habitat heterogeneity influences cold-seep macrofaunal communities within and among seeps along the Norwegian margin. Part 1: macrofaunal community structure. Mar. Ecol. 33, 205–230. doi: 10.1111/j.1439-0485.2011.00503.x
Decker, C., and Olu, K. (2012). Habitat heterogeneity influences cold-seep macrofaunal communities within and among seeps along the Norwegian margin - Part 2: contribution of chemosynthesis and nutritional patterns. Mar. Ecol. 33, 231–245. doi: 10.1111/j.1439-0485.2011.00486.x
Demina, L. L., and Galkin, S. V. (2018). Ecology of the bottom fauna and bioaccumulation of trace metals along the Lena River–Laptev Sea transect. Environ. Earth Sci. 77:43. doi: 10.1007/s12665-018-7231-y
Drazen, J. C., Goffredi, S. K., Schlining, B., and Stakes, D. S. (2003). Aggregations of egg-brooding deep-sea fish and cephalopods on the gorda escarpment: a reproductive hot spot. Biol. Bull. 205, 1–7. doi: 10.2307/1543439
Duarte, C. M., and Cebrián, J. (1996). The fate of marine autotrophic production. Limnol. Oceanogr. 41, 1758–1766. doi: 10.4319/lo.1996.41.8.1758
Dubilier, N., Bergin, C., and Lott, C. (2008). Symbiotic diversity in marine animals: the art of harnessing chemosynthesis. Nat. Rev. Microbiol. 6, 725–740. doi: 10.1038/nrmicro1992
Dufour, S. C. (2005). Gill anatomy and the evolution of symbiosis in the bivalve family thyasiridae. Biol. Bull. 208, 200–212. doi: 10.2307/3593152
Dufour, S. C., and Felbeck, H. (2006). Symbiont abundance in thyasirids (Bivalvia) is related to particulate food and sulphide availability. Mar. Ecol. Prog. Ser. 320, 185–194. doi: 10.3354/meps320185
Dunlop, K. M., Jones, D. O. B., and Sweetman, A. K. (2018). Scavenging processes on jellyfish carcasses across a fjord depth gradient. Limnol. Oceanogr. 63, 1146–1155. doi: 10.1002/lno.10760
Duperron, S., Gaudron, S. M., Rodrigues, C. F., Cunha, M. R., Decker, C., and Olu, K. (2013). An overview of chemosynthetic symbioses in bivalves from the North Atlantic and Mediterranean Sea. Biogeosciences 10, 3241–3267. doi: 10.5194/bg-10-3241-2013
Durant, J. M., Molinero, J. C., Ottersen, G., Reygondeau, G., Stige, L. C., and Langangen, O. (2019). Contrasting effects of rising temperatures on trophic interactions in marine ecosystems. Sci. Rep. 9:15213. doi: 10.1038/s41598-019-51607-w
Feseker, T., Boetius, A., Wenzhöfer, F., Blandin, J., Olu, K., Yoerger, D. R., et al. (2014). Eruption of a deep-sea mud volcano triggers rapid sediment movement. Nat. Commun. 5, 1–8. doi: 10.1038/ncomms6385
Feseker, T., Foucher, J.-P., and Harmegnies, F. (2008). Fluid flow or mud eruptions? Sediment temperature distributions on Håkon Mosby mud volcano, SW Barents Sea slope. Mar. Geol. 247, 194–207. doi: 10.1016/j.margeo.2007.09.005
Fisher, C. R. (1990). Chemoautotrophic and methanotrophic symbiosis in marine invertebrates. Rev. Aquat. Sci 2, 399–436.
Fisher, C. R. (1996). “Ecophysiology of primary production at deep-sea vents and seeps,” in Deep-Sea and Extreme Shallow-Water Habitats: Affinities and Adaptations—Biosyste- matics and Ecology Series, eds F. Uiblein, J. Ott, and M. Stachowtisch, (Berlin: Springer), 313–336.
Fisher, C. R., and Childress, J. J. (1992). Organic carbon transfer from methanotrophic symbionts to the host hydrocarbon-seep mussel. Symbiosis 12, 221–235.
Fossheim, M., Primicerio, R., Johannesen, E., Ingvaldsen, R. B., Aschan, M. M., and Dolgov, A. V. (2015). Recent warming leads to a rapid borealization of fish communities in the Arctic. Nat. Clim. Chang. 5, 673–677. doi: 10.1038/nclimate2647
Frainer, A., Primicerio, R., Kortsch, S., Aune, M., Dolgov, A. V., Fossheim, M., et al. (2017). Climate-driven changes in functional biogeography of Arctic marine fish communities. Proc. Natl. Acad. Sci. 114, 12202–12207. doi: 10.1073/pnas.1706080114
Gage, J. D., and Tyler, P. A. (1991). Deep-Sea Biology: A Natural History Of Organisms At The Deep-Sea Floor. Cambridge: Cambridge University Press.
Gebruk, A. V., Krylova, E. M., Lein, A. Y., Vinogradov, G. M., Anderson, E., Pimenov, N. V., et al. (2003). Methane seep community of the Håkon Mosby mud volcano (the Norwegian Sea): composition and trophic aspects. Sarsia 88, 394–403. doi: 10.1080/00364820310003190
Georgieva, M. N., Wiklund, H., Bell, J. B., Eilertsen, M. H., Mills, R. A., Little, C. T. S., et al. (2015). A chemosynthetic weed: the tubeworm Sclerolinum contortum is a bipolar, cosmopolitan species. BMC Evol. Biol. 15:280. doi: 10.1186/s12862-015-0559-y
Gooday, A. J., Turley, C. M., and Allen, J. A. (1990). Responses by benthic organisms to inputs of organic material to the ocean floor: a review [and Discussion}. Philos. Trans. R. Soc. 331:119.
Graf, G. (1989). Benthic-pelagic coupling in a deep-sea benthic community. Nature 341, 437–439. doi: 10.1038/341437a0
Grebmeier, J. M., and Barry, J. P. (1991). The influence of oceanographic processes on pelagic-benthic coupling in polar regions : a benthic perspective. J. Mar. Syst. 2, 495–518.
Gruber, N. (2011). Warming up, turning sour, losing breath: ocean biogeochemistry under global change. Philos. Trans. R. Soc. A Math. Phys. Eng. Sci. 369, 1980–1996. doi: 10.1098/rsta.2011.0003
Grünke, S., Lichtschlag, A., De Beer, D., Felden, J., Salman, V., Ramette, A., et al. (2012). Mats of psychrophilic thiotrophic bacteria associated with cold seeps of the Barents Sea. Biogeosciences 9, 2947–2960. doi: 10.5194/bg-9-2947-2012
Guillon, E., Menot, L., Decker, C., Krylova, E., and Olu, K. (2017). The vesicomyid bivalve habitat at cold seeps supports heterogeneous and dynamic macrofaunal assemblages. Deep. Res. Part I Oceanogr. Res. Pap. 120, 1–13. doi: 10.1016/j.dsr.2016.12.008
Hansen, J., Ezat, M. M., Åström, E. K. L., and Rasmussen, T. L. (2020). New late pleistocene species of acharax from arctic methane seeps off svalbard. J. Syst. Palaeontol. 18, 197–212. doi: 10.1080/14772019.2019.1594420
Hansen, J., Hoff, U., Sztybor, K., and Rasmussen, T. L. (2017). Taxonomy and palaeoecology of two Late Pleistocene species of vesicomyid bivalves from cold methane seeps at Svalbard (79°N). J. Molluscan Stud. 83, 270–279. doi: 10.1093/mollus/eyx014
Harrison, W. G., and Platt, T. (1986). Photosynthesis-irradiance relationships in polar and temperate phytoplankton populations. Polar Biol. 5, 153–164. doi: 10.1007/BF00441695
Haug, T., Bogstad, B., Chierici, M., Gjøsaeter, H., Hallfredsson, E. H., Høines, A. S., et al. (2017). Future harvest of living resources in the Arctic Ocean north of the Nordic and Barents Seas: a review of possibilities and constraints. Fish. Res. 188, 38–57. doi: 10.1016/j.fishres.2016.12.002
Hegseth, E. N. (1998). Primary production of the northern Barents Sea. Polar Res. 17, 113–123. doi: 10.1111/j.1751-8369.1998.tb00266.x
Heyl, T. P., Gilhooly, W. P., Chambers, R. M., Gilchrist, G. W., Macko, S. A., Ruppel, C. D., et al. (2007). Characteristics of vesicomyid clams and their environment at the Blake Ridge cold seep, South Carolina, USA. Mar. Ecol. Prog. Ser. 339, 169–184. doi: 10.3354/meps339169
Higgs, N. D., Gates, A. R., and Jones, D. O. B. (2014). Fish food in the deep sea: revisiting the role of large food-falls. PLoS One 9:96016. doi: 10.1371/journal.pone.0096016
Higgs, N. D., Newton, J., and Attrill, M. J. (2016). Caribbean spiny lobster fishery is underpinned by trophic subsidies from chemosynthetic primary production. Curr. Biol. 26, 3393–3398. doi: 10.1016/j.cub.2016.10.034
Hjelsteun, B. O., Eldholm, O., Faleide, J. I., and Vogt, P. (1999). Regional settings of hakon mosby mud volcano, SE Barents Sea margin. Geo-Marine Lett. 19, 22–28. doi: 10.1007/s003670050089
Hoff, G. R. (2010). Identification of skate nursery habitat in the eastern Bering Sea. Mar. Ecol. Prog. Ser. 403, 243–254. doi: 10.3354/meps08424
Holding, J. M., Duarte, C. M., Delgado-Huertas, A., Soetaert, K., Vonk, J. E., Agustí, S., et al. (2017). Autochthonous and allochthonous contributions of organic carbon to microbial food webs in Svalbard fjords. Limnol. Oceanogr. 62, 1307–1323. doi: 10.1002/lno.10526
Hong, W.-L., Lepland, A., Himmler, T., Kim, J., Chand, S., Sahy, D., et al. (2019). Discharge of meteoric water in the eastern norwegian sea since the last glacial period. Geophys. Res. Lett. 46, 8194–8204. doi: 10.1029/2019gl084237
Hong, W.-L., Torres, M. E., Carroll, J., Crémière, A., Panieri, G., Yao, H., et al. (2017). Seepage from an arctic shallow marine gas hydrate reservoir is insensitive to momentary ocean warming. Nat. Commun. 8:15745. doi: 10.1038/ncomms15745
Hourdez, S., and Lallier, F. H. (2007). Adaptations to hypoxia in hydrothermal-vent and cold-seep invertebrates. Rev. Environ. Sci. Biotechnol. 6, 143–159. doi: 10.1007/s11157-006-9110-9113
Hovland, M. (2008). Deep-water Coral Reefs: Unique Biodiversity. Chichester, UK: Hotspots Praxis Publishing (Springer), 278.
Hovland, M., and Svensen, H. (2006). Submarine pingoes: indicators of shallow gas hydrates in a pockmark at Nyegga, Norwegian Sea. Mar. Geol. 228, 15–23. doi: 10.1016/j.margeo.2005.12.005
Hryniewicz, K., Amano, K., Bitner, M., Hagström, J., Kiel, S., Klompmaker, A., et al. (2019). A late Paleocene fauna from shallow-water chemosynthesis-based ecosystems, Spitsbergen, Svalbard. Acta Palaeontol. Pol. 64:554. doi: 10.4202/app.00554.2018
Ingólfsson, Ó, and Landvik, J. Y. (2013). The svalbard-barents sea ice-sheet - historical, current and future perspectives. Quat. Sci. Rev. 64, 33–60. doi: 10.1016/j.quascirev.2012.11.034
IPCC, (2018). Global Warming of 1.5°C: An IPCC Special Report on the Impacts of Global Warming of 1.5°C Above Pre-industrial Levels and Related Global Greenhouse Gas Emission Pathways, in the Context of Strengthening the Global Response to the Threat of Climate Chang. Geneva: Intergovernmental Panel on Climate Change.
Jakobsson, M., Mayer, L., Coakley, B., Dowdeswell, J. A., Forbes, S., Fridman, B., et al. (2012). The international bathymetric chart of the arctic ocean (IBCAO) version 3.0. Geophys. Res. Lett. 39:219. doi: 10.1029/2012GL052219
James, R. H., Bousquet, P., Bussmann, I., Haeckel, M., Kipfer, R., Leifer, I., et al. (2016). Effects of climate change on methane emissions from seafloor sediments in the Arctic Ocean: a review. Limnol. Oceanogr. 61, S283–S299. doi: 10.1002/lno.10307
Jerosch, K., Schlüter, M., Foucher, J.-P. P., Allais, A.-G. G., Klages, M., Edy, C., et al. (2007). Spatial distribution of mud flows, chemoautotrophic communities, and biogeochemical habitats at Håkon Mosby Mud Volcano. Mar. Geol. 243, 1–17. doi: 10.1016/j.margeo.2007.03.010
Johnson, J. E., Mienert, J., Plaza-Faverola, A., Vadakkepuliyambatta, S., Knies, J., Bünz, S., et al. (2015). Abiotic methane from ultraslow-spreading ridges can charge Arctic gas hydrates. Geology 43, 371–374. doi: 10.1130/G36440.1
Jørgensen, L. L., Primicerio, R., Ingvaldsen, R. B., Fossheim, M., Strelkova, N., Thangstad, T. H., et al. (2019). Impact of multiple stressors on sea bed fauna in a warming Arctic. Mar. Ecol. Prog. Ser. 608, 1–12. doi: 10.3354/meps12803
Kaul, N., Foucher, J. P., and Heesemann, M. (2006). Estimating mud expulsion rates from temperature measurements on Håkon Mosby Mud Volcano, SW Barents Sea. Mar. Geol. 229, 1–14. doi: 10.1016/j.margeo.2006.02.004
Kiel, S. (2016). A biogeographic network reveals evolutionary links between deep-sea hydrothermal vent and methane seep faunas. Proc. R. Soc. B Biol. Sci. 283:20162337. doi: 10.1098/rspb.2016.2337
Kjesbu, O. S., Bogstad, B., Devine, J. A., Gjøsaeter, H., Howell, D., Ingvaldsen, R. B., et al. (2014). Synergies between climate and management for Atlantic cod fisheries at high latitudes. Proc. Natl. Acad. Sci. 111, 3478–3483. doi: 10.1073/pnas.1316342111
Koh, C. A., and Sloan, E. D. (2007). Natural gas hydrates: recent advances and challenges in energy and environmental applications. AIChE J. 53, 1636–1643. doi: 10.1002/aic.11219
Krylova, E. M., Gebruk, A. V., Portnova, D. A., Todt, C., and Haflidason, H. (2011). New species of the genus Isorropodon (Bivalvia: Vesicomyidae: Pliocardiinae) from cold methane seeps at Nyegga (Norwegian Sea, Vøring Plateau, Storrega Slide). J. Mar. Biol. Assoc. U. K. 91, 1135–1144. doi: 10.1017/S002531541100004X
Krylova, E. M., and Von Cosel, R. (2011). A new genus of large vesicomyidae (Mollusca, Bivalvia, Vesicomyidae, Pliocardiinae) from the Congo margin, with the first record of the subfamily Pliocardiinae in the Bay of Biscay (northeastern Atlantic). Zoosystema 33, 83–99.
Kuzmin, S. A., Akhtarin, S. M., and Menis, D. T. (1998). The first findings of snow crab Chionoecetes opilio (Decapoda, Majidae) in the Barents Sea. Zool. J. 40, 490–492.
Kvenvolden, K. A., Ginsburg, G. D., and Soloviev, V. A. (1993). Worldwide distribution of subaquatic gas hydrates. Geo. Mar. Lett. 13, 32–40. doi: 10.1007/BF01204390
Lammers, S., Suess, E., and Hovland, M. (1995). A large methane plume east of Bear Island (Barents Sea): implications for the marine methane cycle. Geol. Rundschau. 84, 59–66. doi: 10.1007/BF00192242
Lee, D. H., Kim, J. H., Lee, Y. M., Jin, Y. K., Paull, C., Kim, D., et al. (2019). Chemosynthetic bacterial signatures in Frenulata tubeworm Oligobrachia sp. In an active mud volcano of the Canadian Beaufort Sea. Mar. Ecol. Prog. Ser. 628, 95–104. doi: 10.3354/meps13084
Lee, Y. M., Noh, H.-J., Lee, D.-H., Kim, J.-H., Jin, Y. K., and Paull, C. (2019). Bacterial endosymbiont of Oligobrachia sp. (Frenulata) from an active mud volcano in the Canadian Beaufort Sea. Polar Biol. 42, 2305–2312. doi: 10.1007/s00300-019-02599-w
Levin, L. (2005). “Ecology of cold seep sediments: interactions of fauna with flow, chemistry and microbes,” in Oceanography and Marine Biology - an Annual Review, eds R. Gibson, R. Atkinson, and J. Gordon, (Berlin: Springer), 1–46. doi: 10.1201/9781420037449.ch1
Levin, L., Ziebis, W., Mendoza, G., Growney, V., Tryon, M., Brown, K., et al. (2003). Spatial heterogeneity of macrofauna at northern California methane seeps: influence of sulfide concentration and fluid flow. Mar. Ecol. Prog. Ser. 265, 123–139. doi: 10.3354/meps265123
Levin, L. A., Baco, A. R., Bowden, D., Colaço, A., Cordes, E., Cunha, M. R., et al. (2016). Hydrothermal vents and methane seeps: rethinking the sphere of influence. Front. Mar. Sci. 3:72. doi: 10.3389/fmars.2016.00072
Levin, L. A., and Bris, N. L. (2015). The deep ocean under climate change. Science 350, 766–768. doi: 10.1126/science.aad0126
Levin, L. A., Mendoza, G. F., and Grupe, B. M. (2017). Methane seepage effects on biodiversity and biological traits of macrofauna inhabiting authigenic carbonates. Deep Sea Res. Part II Top. Stud. Oceanogr. 137, 26–41. doi: 10.1016/j.dsr2.2016.05.021
Liira, M., Noormets, R., Sepp, H., Kekišev, O., Maddison, M., and Olaussen, S. (2019). Sediment geochemical study of hydrocarbon seeps in Isfjorden and Mohnbukta : a comparison between western and eastern. Arktos 5, 49–62. doi: 10.1007/s41063-019-00067-67
Loeng, H. (1991). Features of the physical oceanograpic conditions of the Barents Sea. Polar Res. 10, 5–18. doi: 10.1111/j.1751-8369.1991.tb00630.x
Loher, M., Marcon, Y., Pape, T., Römer, M., Wintersteller, P., dos Santos Ferreira, C., et al. (2018). Seafloor sealing, doming, and collapse associated with gas seeps and authigenic carbonate structures at Venere mud volcano, Central Mediterranean. Deep Sea Res. Part I Oceanogr. Res. Pap. 137, 76–96. doi: 10.1016/j.dsr.2018.04.006
Lösekann, T., Knittel, K., Nadalig, T., Fuchs, B., Niemann, H., Boetius, A., et al. (2007). Diversity and abundance of aerobic and anaerobic methane oxidizers at the Haakon Mosby Mud Volcano, Barents Sea. Appl. Environ. Microbiol. 73, 3348–3362. doi: 10.1128/AEM.00016-17
MacAvoy, S. E., Macko, S. A., and Carney, R. S. (2003). Links between chemosynthetic production and mobile predators on the Louisiana continental slope: Stable carbon isotopes of specific fatty acids. Chem. Geol. 201, 229–237.
MacDonald, G. J. (1990). Role of methane clathrates in past and future climates. Clim. Change 16, 247–281. doi: 10.1007/BF00144504
MacDonald, I. R., Smith, M., and Huffer, F. W. (2010). Community structure comparisons of lower slope hydrocarbon seeps, northern Gulf of Mexico. Deep. Res. Part II Top. Stud. Oceanogr. 57, 1904–1915. doi: 10.1016/j.dsr2.2010.09.002
Margold, M., Stokes, C. R., and Clark, C. D. (2018). Reconciling records of ice streaming and ice margin retreat to produce a palaeogeographic reconstruction of the deglaciation of the Laurentide Ice Sheet. Quat. Sci. Rev. 189, 1–30. doi: 10.1016/j.quascirev.2018.03.013
Marshall, B. A. (1988). Skeneidae, Vitrinellidae and Orbitestellidae (Mollusca: Gastropoda) associated with biogenic substrata from bathyal depths off New Zealand and New South Wales. J. Nat. Hist. 22, 949–1004. doi: 10.1080/00222938800770631
Mau, S., Römer, M., Torres, M. E., Bussmann, I., Pape, T., Damm, E., et al. (2017). Widespread methane seepage along the continental margin off Svalbard-from Bjørnøya to Kongsfjorden. Sci. Rep. 7:42997. doi: 10.1038/srep42997
Mayer, L. A., Shor, A. N., Clarke, J. H., and Piper, D. J. W. (1988). Dense biological communities at 3850 m on the laurentian fan and their relationship to the deposits o f the 1929 G r and Banksearthquake. Deep Sea Res. Part A Oceanogr. Res. Pap. 35, 1235–1246.
McMahon, K. W., Ambrose, W. G. J., Johnson, B. J., Sun, M.-Y., Lopez, G. R., Clough, L. M., et al. (2006). Benthic community responses to ice algae and phytoplankton in Ny Alesund. Svalbard. Mar. Ecol. Prog. Ser. 310, 1–14. doi: 10.3354/meps310001
Meyer, K. S., Bergmann, M., and Soltwedel, T. (2013). Interannual variation in the epibenthic megafauna at the shallowest station of the HAUSGARTEN observatory (79° N, 6° E). Biogeosciences 10, 3479–3492. doi: 10.5194/bg-10-3479-2013
Miljødirektoratet, (2019). Saerlig Verdifulle Og Sårbare Områder - Faggrunnlag For Revisjon Og Oppdatering Av Forvaltningsplanene For Norske Havområder M-1303/2019. Available at: https://www.miljodirektoratet.no/globalassets/publikasjoner/m1303/m1303.pdf (accessed November 4, 2019).
Molari, M., Manini, E., and Dell’Anno, A. (2013). Dark inorganic carbon fixation sustains the functioning of benthic deep-sea ecosystems. Global Biogeochem. Cycles 27, 212–221. doi: 10.1002/gbc.20030
Mora, C., Spirandelli, D., Franklin, E. C., Lynham, J., Kantar, M. B., Miles, W., et al. (2018). Broad threat to humanity from cumulative climate hazards intensified by greenhouse gas emissions. Nat. Clim. Chang. 8, 1062–1071. doi: 10.1038/s41558-018-0315-316
Moran, S. B., Lomas, M. W., Kelly, R. P., Gradinger, R., Iken, K., and Mathis, J. T. (2012). Seasonal succession of net primary productivity, particulate organic carbon export, and autotrophic community composition in the eastern Bering Sea. Deep. Res. Part II Top. Stud. Oceanogr. 6, 84–97. doi: 10.1016/j.dsr2.2012.02.011
Morata, N., Michaud, E., and Włodarska-Kowalczuk, M. (2013). Impact of early food input on the arctic benthos activities during the polar night. Polar Biol. 38, 99–114. doi: 10.1007/s00300-013-1414-1415
Morata, N., Renaud, P. E., Brugel, S., Hobson, K. A., and Johnson, B. J. (2008). Spatial and seasonal variations in the pelagic- benthic coupling of the southeastern Beaufort Sea revealed by sedimentary biomarkers. Mar. Ecol. Prog. Ser. 371, 47–63. doi: 10.3354/meps07677
Myhre, C. L., Ferré, B., Platt, S. M., Silyakova, A., Hermansen, O., Allen, G., et al. (2016). Extensive release of methane from Arctic seabed west of Svalbard during summer 2014 does not influence the atmosphere. Geophys. Res. Lett. 43, 4624–4631. doi: 10.1002/2016GL068999.Received
Niemann, H., Linke, P., Knittel, K., MacPherson, E., Boetius, A., Brückmann, W., et al. (2013). Methane-carbon flow into the benthic food web at cold seeps – a case study from the costa rica subduction zone. PLoS One 8:e74894. doi: 10.1371/journal.pone.0074894
Niemann, H., Lösekann, T., de Beer, D., Elvert, M., Nadalig, T., Knittel, K., et al. (2006). Novel microbial communities of the Haakon Mosby mud volcano and their role as a methane sink. Nature 443, 854–858. doi: 10.1038/nature05227
Norkko, J., Pilditch, C. A., Gammal, J., Rosenberg, R., Enemar, A., Magnusson, M., et al. (2019). Ecosystem functioning along gradients of increasing hypoxia and changing soft-sediment community types. J. Sea Res. 153, 101781.
Ofstad, S., Meilland, J., Zamelczyk, K., Chierici, M., Fransson, A., Gründger, F., et al. (2020). Development, Productivity, and Seasonality of Living Planktonic Foraminiferal Faunas and Limacina helicina in an Area of Intense Methane Seepage in the Barents Sea. J. Geophys. Res. Biogeosciences 125, 1–24. doi: 10.1029/2019JG005387
Oliver, P. G., and Killeen, I. J. (2002). The Thyasiridae (Mollusca: Bivalvia) Of The British Continental Shelf And North Sea Oil Fields: An Identification Manual. Cardiff, CF: National Museums & Galleries of Wales.
Olsen, G. H., Carroll, M. L., Renaud, P. E., Ambrose, W. G. J., Olssøn, R., and Carroll, J. (2007). Benthic community response to petroleum-associated components in arctic versus temperate marine sediments. Mar. Biol. 151, 2167–2176. doi: 10.1007/s00227-007-0650-z
Olu-Le Roy, K., Sibuet, M., Fiala-Médioni, A., Gofas, S., Salas, C., Mariotti, A., et al. (2004). Cold seep communities in the deep eastern Mediterranean Sea: composition, symbiosis and spatial distribution on mud volcanoes. Deep. Res. Part I Oceanogr. Res. Pap. 51, 1915–1936. doi: 10.1016/j.dsr.2004.07.004
Patton, H., Hubbard, A., Andreassen, K., Auriac, A., Whitehouse, P. L., Stroeven, A. P., et al. (2017). Deglaciation of the eurasian ice sheet complex. Quat. Sci. Rev. 169, 148–172. doi: 10.1016/j.quascirev.2017.05.019
Paull, C. K., Dallimore, S. R., Caress, D. W., Gwiazda, R., Melling, H., Riedel, M., et al. (2015). Active mud volcanoes on the continental slope of the Canadian Beaufort Sea. Geochem. Geophys. Geosyst. 16, 3160–3181. doi: 10.1002/2015GC005928
Paull, C. K., Ussler, W., Dallimore, S. R., Blasco, S. M., Lorenson, T. D., Melling, H., et al. (2007). Origin of pingo-like features on the Beaufort Sea shelf and their possible relationship to decomposing methane gas hydrates. Geophys. Res. Lett. 34, 1–5. doi: 10.1029/2006GL027977
Pearson, T., and Rosenberg, R. (1978). Macrobenthic succession in relation to organic enrichment and pollution of the marine environment. Ocean Mar. Biol. Annu. Rev. 16, 229–311. doi: 10.1016/j.marpolbul.2006.09.008
Pimenov, N. V., Savvichev, A., Rusanov, I. I, Lein, A. Y., and Ivanov, M. V. (2000). Microbiological processes of the carbon and sulfur cycle in cold methane seeps in the North Atlantic. Mikrobiologiia 69, 831–843. doi: 10.1023/A:1026666527034
Pohlman, J. W., Greinert, J., Ruppel, C., Silyakova, A., Vielstädte, L., Casso, M., et al. (2017). Enhanced CO 2 uptake at a shallow arctic ocean seep field overwhelms the positive warming potential of emitted methane. Proc. Natl. Acad. Sci. U.S.A. 114, 5355–5360. doi: 10.1073/pnas.1618926114
Portail, M., Olu, K., Dubois, S. F., Escobar-Briones, E., Gelinas, Y., Menot, L., et al. (2016). Food-web complexity in guaymas basin hydrothermal vents and cold seeps. PLoS One 11:e0162263. doi: 10.1371/journal.pone.0162263
Portnov, A., Smith, A. J., Mienert, J., Cherkashov, G., Rekant, P., Semenov, P., et al. (2013). Offshore permafrost decay and massive seabed methane escape in water depths >20 m at the South Kara Sea shelf. Geophys. Res. Lett. 40, 3962–3967. doi: 10.1002/grl.50735
Portnov, A., Vadakkepuliyambatta, S., Mienert, J., and Hubbard, A. (2016). Ice-sheet-driven methane storage and release in the Arctic. Nat. Commun. 7:10314. doi: 10.1038/ncomms10314
Portnova, D., Mokievsky, V., and Soltwedel, T. (2011). Nematode species distribution patterns at the Håkon Mosby Mud Volcano (Norwegian Sea). Mar. Ecol. 32, 24–41. doi: 10.1111/j.1439-0485.2010.00403.x
Rasmussen, T. L., Thomsen, E., Ślubowska, M. A., Jessen, S., Solheim, A., and Koç, N. (2007). Paleoceanographic evolution of the SW Svalbard margin (76°N) since 20,000 14C yr BP. Quat. Res. 67, 100–114. doi: 10.1016/j.yqres.2006.07.002
Reeburgh, W. S. (2007). Oceanic methane biogeochemistry. Chem. Rev. 107, 486–513. doi: 10.1021/cr050362v
Renaud, P. E., Morata, N., Carroll, M. L., Denisenko, S. G., and Reigstad, M. (2008). Pelagic–benthic coupling in the western Barents Sea: processes and time scales. Deep. Res. Part II Top. Stud. Oceanogr. 55, 2372–2380. doi: 10.1016/j.dsr2.2008.05.017
Renaud, P. E., Riedel, A., Michel, C., Morata, N., Gosselin, M., Juul-Pedersen, T., et al. (2007). Seasonal variation in benthic community oxygen demand: a response to an ice algal bloom in the Beaufort Sea. Canadian Arctic? J. Mar. Syst. 67, 1–12. doi: 10.1016/j.jmarsys.2006.07.006
Renaud, P. E., Sejr, M. K., Bluhm, B. A., Sirenko, B., and Ellingsen, I. H. (2015). The future of Arctic benthos: expansion, invasion, and biodiversity. Prog. Oceanogr. 139, 244–257. doi: 10.1016/j.pocean.2015.07.007
Rice, A. L., Billett, D. S. M., Fry, J., John, A. W. G., Lampitt, R. S., Mantoura, R. F. C., et al. (1986). Seasonal deposition of phytodetritus to the deep-sea floor. Proc. R. Soc. Edinburgh. Sect. B. Biol. Sci. 88, 265–279. doi: 10.1017/S0269727000004590
Rise, L., Bellec, V. K., Ch, S., and Bøe, R. (2014). Pockmarks in the southwestern Barents Sea and Finnmark fjords. Nor. Geol. Tidsskr. 94, 263–282.
Rise, L., Bøe, R., Riis, F., Bellec, V. K., Laberg, J. S., Eidvin, T., et al. (2013). The Lofoten-Vesterålen continental margin, North Norway: canyons and mass-movement activity. Mar. Pet. Geol. 45, 134–149. doi: 10.1016/j.marpetgeo.2013.04.021
Rodrigues, C. F., Hilario, A., and Cunha, M. R. (2013). Chemosymbiotic species from the Gulf of Cadiz (NE Atlantic): distribution, life styles and nutritional patterns. Biogeosciences 10, 2569–2581. doi: 10.5194/bg-10-2569-2013
Rybakova, E., Galkin, S., Bergmann, M., Soltwedel, T., and Gebruk, A. (2013). Density and distribution of megafauna at the Håkon Mosby mud volcano (the Barents Sea) based on image analysis. Biogeosciences 10, 3359–3374. doi: 10.5194/bg-10-3359-2013
Sahling, H., Galkin, S. V., Salyuk, A., Greinert, J., Foerstel, H., Piepenburg, D., et al. (2003). Depth-related structure and ecological significance of cold-seep communities—a case study from the Sea of Okhotsk. Deep Sea Res. Part I Oceanogr. Res. Pap. 50, 1391–1409. doi: 10.1016/j.dsr.2003.08.004
Sahling, H., Römer, M., Pape, T., Bergès, B., dos Santos Fereirra, C., Boelmann, J., et al. (2014). Gas emissions at the continental margin west of Svalbard: mapping, sampling, and quantification. Biogeosciences 11, 6029–6046. doi: 10.5194/bg-11-6029-2014
Sakshaug, E., and Slagstad, D. (1991). Light and productivity of phytoplankton in polar marine ecosystems: a physiological view. Polar Res. 10, 69–86. doi: 10.3402/polar.v10i1.6729
Salinas-De-León, P., Phillips, B., Ebert, D., Shivji, M., Cerutti-Pereyra, F., Ruck, C., et al. (2018). Deep-sea hydrothermal vents as natural egg-case incubators at the Galapagos Rift. Sci. Rep. 8, 1–7. doi: 10.1038/s41598-018-20046-20044
Sasaki, T., Warén, A., Kano, Y., Okutani, T., and Fujikura, K. (2010). “Gastropods from recent hot vents and cold seeps: systematics, diversity and life strategies,” in The Vent and Seep Biota, ed. S. Kiel, (Berlin: Springer), 169–254. doi: 10.1007/978-90-481-9572-5_7
Savvichev, A. S., Kadnikov, V. V., Kravchishina, M. D., Galkin, S. V., Novigatskii, A. N., Sigalevich, P. A., et al. (2018). Methane as an organic matter source and the trophic basis of a laptev sea cold seep microbial community. Geomicrobiol. J. 35, 411–423. doi: 10.1080/01490451.2017.1382612
Schander, C., Rapp, H. T., Kongsrud, J. A., Bakken, T., Berge, J., Cochrane, S., et al. (2010). The fauna of hydrothermal vents on the Mohn Ridge (North Atlantic). Mar. Biol. Res. 6, 155–171. doi: 10.1080/17451000903147450
Schulz, M., Bergmann, M., von Juterzenka, K., and Soltwedel, T. (2010). Colonisation of hard substrata along a channel system in the deep Greenland Sea. Polar Biol. 33, 1359–1369. doi: 10.1007/s00300-010-0825-829
Schulze, A., and Halanych, K. M. (2003). Siboglinid evolution shaped by habitat preference and sulfide tolerance. Hydrobiologia 496, 199–205. doi: 10.1023/A:1026192715095
Seabrook, S., De Leo, F. C., and Thurber, A. R. (2019). Flipping for food: the use of a methane seep by tanner crabs (Chionoecetes tanneri). Front. Mar. Sci. 6:43. doi: 10.3389/fmars.2019.00043
Sen, A., Åström, E. K. L., Hong, W.-L., Portnov, A., Waage, M., Serov, P., et al. (2018a). Geophysical and geochemical controls on the megafaunal community of a high Arctic cold seep. Biogeosciences 15, 4533–4559. doi: 10.5194/bg-15-4533-2018
Sen, A., Duperron, S., Hourdez, S., Piquet, B., Léger, N., Gebruk, A., et al. (2018b). Cryptic frenulates are the dominant chemosymbiotrophic fauna at Arctic and high latitude Atlantic cold seeps. PLoS One 13:e0209273. doi: 10.1371/journal.pone.0209273
Sen, A., Chitkara, C., Hong, W.-L., Lepland, A., Cochrane, S., di Primio, R., et al. (2019a). Image based quantitative comparisons indicate heightened megabenthos diversity and abundance at a site of weak hydrocarbon seepage in the southwestern Barents Sea. PeerJ 7:e7398. doi: 10.7717/peerj.7398
Sen, A., Himmler, T., Hong, W. L., Chitkara, C., Lee, R. W., Ferré, B., et al. (2019b). Atypical biological features of a new cold seep site on the Lofoten-Vesterålen continental margin (northern Norway). Sci. Rep. 9:1762. doi: 10.1038/s41598-018-38070-38079
Sen, A., Dennielou, B., Tourolle, J., Arnaubec, A., Rabouille, C., and Olu, K. (2017). Fauna and habitat types driven by turbidity currents in the lobe complex of the Congo deep-sea fan. Deep. Res. Part II Top. Stud. Oceanogr. 142, 167–179. doi: 10.1016/j.dsr2.2017.05.009
Sen, A., Didriksen, A., Hourdez, S., Svenning, M. M., and Rasmussen, T. L. (2020). Frenulate siboglinids at high Arctic methane seeps and insight into high latitude frenulate distribution. Ecol. Evol. 10, 1339–1351. doi: 10.1002/ece3.5988
Serov, P., Portnov, A., Mienert, J., Semenov, P., and Ilatovskaya, P. (2015). Methane release from pingo-like features across the South Kara Sea shelf, an area of thawing offshore permafrost. J. Geophys. Res. Earth Surf. 120, 1515–1529. doi: 10.1002/2015JF003467
Serov, P., Vadakkepuliyambatta, S., Mienert, J., Patton, H., Portnov, A., Silyakova, A., et al. (2017). Postglacial response of Arctic Ocean gas hydrates to climatic amelioration. Proc. Natl. Acad. Sci. 114, 6215–6220. doi: 10.1073/pnas.1619288114
Shakhova, N., Semiletov, I., Leifer, I., Salyuk, A., Rekant, P., and Kosmach, D. (2010). Geochemical and geophysical evidence of methane release over the East Siberian Arctic Shelf. J. Geophys. Res. 115:C08007. doi: 10.1029/2009JC005602
Sibuet, M., and Olu, K. (1998). Biogeography, biodiversity and fluid dependence of deep-sea cold-seep communities at active and passive margins. Deep Sea Res. Part II Top. Stud. Oceanogr. 45, 517–567. doi: 10.1016/S0967-0645(97)00074-X
Sirenko, B., Denisenko, S., Deubel, H., and Rachor, E. (2004). Deep water communities of the laptev sea and adjacent parts of the arctic ocean. fauna ecosyst. laptev sea adjac. Deep waters Arctic. Part I. St.-petersbg. 54, 28–73.
Skarke, A., Ruppel, C., Kodis, M., Brothers, D., and Lobecker, E. (2014). Widespread methane leakage from the sea floor on the northern US Atlantic margin. Nat. Geosci. 7, 657–661.
Sloan, E. D. (1998). Gas hydrates: review of physical/chemical properties. Energy Fuels 12, 191–196. doi: 10.1021/ef970164
Smirnov, R. V. (2008). Morphological characters and classification of the subclass monilifera (Pogonophora) and the problem of evolution of the bridle in pogonophorans. Russ. J. Mar. Biol. 34, 359–368. doi: 10.1134/S1063074008060035
Smirnov, R. V. (2014). A revision of the Oligobrachiidae (Annelida: Pogonophora), with notes on the morphology and distribution of Oligobrachia haakonmosbiensis Smirnov. Mar. Biol. Res. 10, 972–982. doi: 10.1080/17451000.2013.872799
Smith, C. R., and Baco, A. R. (2003). Ecology of whale falls at the deep-sea floor. Oceanogr. Mar. Biol. Vol 41, 311–354.
Smith, C. R., De Master, D. J., Thomas, C., Sršen, P., Grange, L., Evrard, V., et al. (2012). Pelagic-benthic coupling, food banks, and climate change on the west Antarctic Peninsula shelf. Oceanography 25, 189–201. doi: 10.5670/oceanog.2012.94
Smith, C. R., Glover, A. G., Treude, T., Higgs, N. D., and Amon, D. J. (2015). Whale-fall ecosystems: recent insights into ecology, paleoecology, and evolution. Ann. Rev. Mar. Sci. 7, 571–596. doi: 10.1146/annurev-marine-010213-135144
Solheim, A., and Elverhøi, A. (1993). Gas-related sea floor craters in the Barents Sea. Geo. Mar. Lett. 13, 235–243. doi: 10.1007/BF01207753
Soltwedel, T., Jaeckisch, N., Ritter, N., Hasemann, C., Bergmann, M., and Klages, M. (2009). Bathymetric patterns of megafaunal assemblages from the arctic deep-sea observatory HAUSGARTEN. Deep. Res. Part I Oceanogr. Res. Pap. 56, 1856–1872. doi: 10.1016/j.dsr.2009.05.012
Søreide, J. E., Hop, H., Carroll, M. L., Falk-Petersen, S., and Hegseth, E. N. (2006). Seasonal food web structures and sympagic–pelagic coupling in the European Arctic revealed by stable isotopes and a two-source food web model. Prog. Oceanogr. 71, 59–87. doi: 10.1016/j.pocean.2006.06.001
Southward, A. J., and Southward, E. C. (1982). The role of dissolved organic matter in the nutrition of deep-sea Benthos. Am. Zool. 22, 647–658.
Steffen, W., Rockström, J., Richardson, K., Lenton, T. M., Folke, C., Liverman, D., et al. (2018). Trajectories of the earth system in the anthropocene. Proc. Natl. Acad. Sci. U.S.A. 115, 8252–8259. doi: 10.1073/pnas.1810141115
Stenseth, N. C., and Mysterud, A. (2002). Climate, changing phenology, and other life history traits: Nonlinearity and match-mismatch to the environment. Proc. Natl. Acad. Sci. U.S.A. 99, 13379–13381. doi: 10.1073/pnas.212519399
Stokes, C. R., Tarasov, L., Blomdin, R., Cronin, T. M., Fisher, T. G., Gyllencreutz, R., et al. (2015). On the reconstruction of palaeo-ice sheets: recent advances and future challenges. Quat. Sci. Rev. 125, 15–49. doi: 10.1016/j.quascirev.2015.07.016
Stone, R. B., Pratt, H. L., Parker, R. O., and Davis, G. E. (1979). A comparison of fish populations on an artificial and natural reef in the florida Keys. Mar. Fish. Rev. 41, 1–11.
Stranne, C., O’Regan, M., Dickens, G. R., Crill, P., Miller, C., Preto, P., et al. (2016). Dynamic simulations of potential methane release from East Siberian continental slope sediments. Geochem. Geophys. Geosyst. 17, 872–886.
Sun, M., Carroll, M. L., Ambrose, W. G. J., Clough, L. M., Zou, L., and Lopez, G. R. (2007). Rapid consumption of phytoplankton and ice algae by Arctic soft-sediment benthic communities : evidence using natural and 13 C-labeled food materials. J. Mar. Res. 65, 561–588.
Sweetman, A., Levin, L., Rapp, H., and Schander, C. (2013). Faunal trophic structure at hydrothermal vents on the southern Mohn’s Ridge, Arctic Ocean. Mar. Ecol. Prog. Ser. 473, 115–131. doi: 10.3354/meps10050
Sweetman, A. K., Smith, C. R., Shulse, C. N., Maillot, B., Lindh, M., Church, M. J., et al. (2019). Key role of bacteria in the short-term cycling of carbon at the abyssal seafloor in a low particulate organic carbon flux region of the eastern Pacific Ocean. Limnol. Oceanogr. 64, 694–713. doi: 10.1002/lno.11069
Sweetman, A. K., Thurber, A. R., Smith, C. R., Levin, L. A., Mora, C., Wei, C.-L., et al. (2017). Major impacts of climate change on deep-sea benthic ecosystems. Elem. Sci. Anth. 5:4. doi: 10.1525/elementa.203
Sztybor, K., and Rasmussen, T. L. (2016). Diagenetic disturbances of marine sedimentary records from methane- influenced environments in the Fram Strait as indications of variation in seep intensity during the last 35 000 years. Boreas 46, 212–228. doi: 10.1111/bor.12202
Tarasov, V. G., Gebruk, A. V., Mironov, A. N., and Moskalev, L. I. (2005). Deep-sea and shallow-water hydrothermal vent communities: two different phenomena? Chem. Geol. 224, 5–39. doi: 10.1016/j.chemgeo.2005.07.021
Taylor, J. D., and Glover, E. A. (2010). “Chemosymbiotic bivalves,” in The Vent and Seep Biota, ed. S. Kiel, (Göttingen: Springer), 107–135.
Terwilliger, N. B. (1998). Functional adaptations of oxygen-transport proteins. J. Exp. Biol. 201, 1085–1098.
Thomsen, E., Lander Rasmussen, T., Sztybor, K., Hanken, N.-M., Secher Tendal, O., and Uchman, A. (2019). Cold-seep fossil macrofaunal assemblages from Vestnesa Ridge, eastern Fram Strait, during the past 45 000 years. Polar Res. 38. doi: 10.33265/polar.v38.3310
Tobler, M., Passow, C. N., Greenway, R., Kelley, J. L., and Shaw, J. H. (2016). The evolutionary ecology of animals inhabiting hydrogen sulfide – rich environments. Annu. Rev. Ecol. Evol. Syst. 47, 239–262. doi: 10.1146/annurev-ecolsys-121415-132418
Treude, T., Kiel, S., Linke, P., Peckmann, J., and Goedert, J. L. (2011). Elasmobranch egg capsules associated with modern and ancient cold seeps: a nursery for marine deep-water predators. Mar. Ecol. Prog. Ser. 437, 175–181. doi: 10.3354/meps09305
Turner, P. J., Ball, B., Diana, Z., Fariñas-Bermejo, A., Grace, I., McVeigh, D., et al. (2020). Methane Seeps on the US atlantic margin and their potential importance to populations of the commercially valuable deep-sea red crab, Chaceon quinquedens. Front. Mar. Sci. 7:75. doi: 10.3389/fmars.2020.00075
Van Gaever, S., Moodley, L., de Beer, D., and Vanreusel, A. (2006). Meiobenthos at the Arctic Håkon Mosby Mud Volcano, with a parental-caring nematode thriving in sulphide-rich sediments. Mar. Ecol. Prog. Ser. 321, 143–155. doi: 10.3354/meps321143
Vanreusel, A., Andersen, A., Boetius, A., Connelly, D., Cunha, M. R., Decker, C., et al. (2009). Biodiversity of cold seep ecosystems along the European margins. Oceanography 22, 110–127.
Vaquer-Sunyer, R., and Duarte, C. M. (2010). Sulfide exposure accelerates hypoxia-driven mortality. Limnol. Oceanogr. 55, 1075–1082. doi: 10.4319/lo.2010.55.3.1075
Vaughn Barrie, J., Cook, S., and Conway, K. W. (2011). Cold seeps and benthic habitat on the Pacific margin of Canada. Cont. Shelf Res. 31, 85–92. doi: 10.1016/j.csr.2010.02.013
Vinogradov, G. M., and Semenova, T. N. (2000). Some peculiarities of the vertical distribution of mesoplankton in the Norwegian-Greenland Basin. Oceanology 40, 370–377.
Vismann, B. (1991). Sulfide tolerance: physiological mechanisms and ecological implications. Ophelia 34, 1–27.
Vogt, P., Crane, K., Sundvor, E., Max, M. D., and Pfirman, S. L. (1994). Methane-generated(?) pockmarks on young, thickly sedimented oceanic crust in the arctic: vestnesa ridge, fram strait. Geology 22, 255–258.
Vogt, P. R., Cherkashev, G., Ginsburg, G., Ivanov, G., Milkov, A., Crane, K., et al. (1997). Haakon Mosby mud volcano provides unusual example of venting. EOS Trans. Am. Geophys. Union 78, 549–557.
Wassmann, P., Reigstad, M., Haug, T., Rudels, B., Carroll, M. L., Hop, H., et al. (2006). Food webs and carbon flux in the Barents Sea. Prog. Oceanogr. 71, 232–287. doi: 10.1016/j.pocean.2006.10.003
Weaver, J. S., and Stewart, J. M. (1982). “In situ hydrates under the Beaufort Sea shelf,” in Proceedings of the 4th International Conferences on Permafrost, Ottawa, 312–319.
Weaver, P. P. E., Billett, D. S. M., Boetius, A., Danovaro, R., Freiwald, A., and Sibuet, M. (2004). Hotspot ecosystem research on Europe’s deep-ocean margins. Oceanography 17, 132–143. doi: 10.5670/oceanog.2004.10
Webb, K., Barnes, D. K. A., and Planke, S. (2009). Pockmarks: refuges for marine benthic biodiversity. Limnol. Oceanogr. 54, 1776–1788. doi: 10.4319/lo.2009.54.5.1776
Keywords: authigenic carbonates, chemosynthesis, commercial species, methane seepage, trophic structure
Citation: Åström EKL, Sen A, Carroll ML and Carroll J (2020) Cold Seeps in a Warming Arctic: Insights for Benthic Ecology. Front. Mar. Sci. 7:244. doi: 10.3389/fmars.2020.00244
Received: 30 November 2019; Accepted: 27 March 2020;
Published: 21 May 2020.
Edited by:
Jeroen Ingels, Florida State University, United StatesReviewed by:
Clara Rodrigues, University of Aveiro, PortugalCindy Lee Van Dover, Nicholas School of the Environment, United States
Copyright © 2020 Åström, Sen, Carroll and Carroll. This is an open-access article distributed under the terms of the Creative Commons Attribution License (CC BY). The use, distribution or reproduction in other forums is permitted, provided the original author(s) and the copyright owner(s) are credited and that the original publication in this journal is cited, in accordance with accepted academic practice. No use, distribution or reproduction is permitted which does not comply with these terms.
*Correspondence: Emmelie K. L. Åström, ZW1tZWxpZS5rLmFzdHJvbUB1aXQubm8=
†These authors have contributed equally to this work