- 1Department of Earth and Environmental Sciences, Rensselaer Polytechnic Institute, Troy, NY, United States
- 2MARUM - Center for Marine Environmental Sciences, University of Bremen, Bremen, Germany
- 3Department of Marine and Coastal Environmental Science, Texas A&M Galveston Campus, Galveston, TX, United States
- 4Department of Oceanography, Texas A&M University, College Station, TX, United States
- 5Max Planck Institute for Biogeochemistry, Jena, Germany
- 6ICBM-MPI Bridging Group for Marine Geochemistry, Institute for Chemistry and Biology of the Marine Environment, University of Oldenburg, Oldenburg, Germany
- 7International Atomic Energy Agency (IAEA) - Environment Laboratories, Monaco, Monaco
- 8Department of Earth Sciences, Utrecht University, Utrecht, Netherlands
- 9GEOMAR Helmholtz Centre for Ocean Research Kiel, Kiel, Germany
- 10Biogeochemistry Program, Japan Agency for Marine-Earth Science and Technology, Yokosuka, Japan
- 11Alfred Wegener Institute, Alfred Wegener Institute for Polar and Marine Research, Bremerhaven, Germany
- 12Department of Oceanography, Center for Oceanographic Research COPAS Sur-Austral, University of Concepción, Concepción, Chile
- 13Frontiers Science Center for Deep Ocean Multispheres and Earth System, and Key Laboratory of Marine Chemistry Theory and Technology, Ministry of Education, Ocean University of China, Qingdao, China
- 14Woods Hole Oceanographic Institution, Woods Hole, MA, United States
The vast majority of freshly produced oceanic dissolved organic carbon (DOC) is derived from marine phytoplankton, then rapidly recycled by heterotrophic microbes. A small fraction of this DOC survives long enough to be routed to the interior ocean, which houses the largest and oldest DOC reservoir. DOC reactivity depends upon its intrinsic chemical composition and extrinsic environmental conditions. Therefore, recalcitrance is an emergent property of DOC that is analytically difficult to constrain. New isotopic techniques that track the flow of carbon through individual organic molecules show promise in unveiling specific biosynthetic or degradation pathways that control the metabolic turnover of DOC and its accumulation in the deep ocean. However, a multivariate approach is required to constrain current carbon fluxes so that we may better predict how the cycling of oceanic DOC will be altered with continued climate change. Ocean warming, acidification, and oxygen depletion may upset the balance between the primary production and heterotrophic reworking of DOC, thus modifying the amount and/or composition of recalcitrant DOC. Climate change and anthropogenic activities may enhance mobilization of terrestrial DOC and/or stimulate DOC production in coastal waters, but it is unclear how this would affect the flux of DOC to the open ocean. Here, we assess current knowledge on the oceanic DOC cycle and identify research gaps that must be addressed to successfully implement its use in global scale carbon models.
Introduction
Containing roughly the same amount of carbon as terrestrial biomass, or CO2 in the atmosphere, the oceanic dissolved organic matter (DOM) pool represents one of Earth’s large organic carbon reservoirs (660 Pg-C; Hansell et al., 2009; Houghton, 2014). Global carbon cycling models generally consider the total ocean split into three “boxes” – the surface ocean (<200 m), the twilight or mesopelagic zone (200–1,000 m) and the interior ocean (>1,000 m). This compartmentalization of oceanic water masses is driven by our current understanding of how carbon is produced, cycled, and stored with depth. Oceanic DOM derives mainly from autochthonous production by phytoplankton in surface waters where it is rapidly recycled and serves as an energy source for heterotrophic microbes. A small portion of DOM produced in surface waters is eventually routed to the ocean’s interior, which houses the bulk of the oceanic dissolved organic carbon (DOC) pool and cycles on much longer timescales. However, mechanisms controlling the accumulation of DOM in the interior ocean remain poorly understood. The oceanic DOC cycle is an essential component of the global carbon cycle, thus imbalances among oceanic DOC pools would impact future climate scenarios. However, global scale carbon models lack a coherent implementation of DOC at present.
Surface, mesopelagic, and interior ocean DOM each exhibit distinct latitudinal trends (Carlson et al., 2010). The distribution of DOM among these ocean boxes is largely mediated by biological activity. The “biological carbon pump” is a conceptual framework that describes the cumulative processes involved in the transport of biogenic material from the surface to the deeper ocean, including the formation and settling of particulate organic matter (POM), the active migration of zooplankton and other mobile organisms and the physical overturning of ocean water, including its organic constituents (Sarmiento and Gruber, 2006; Lutz et al., 2007; Jiao et al., 2010). Taken together, these mechanisms result in the total export of ca. 0.2 Pg-C yr–1 as DOC from the surface and mesopelagic ocean (<1,000 m; 180 Pg-C) to the interior ocean (>1,000 m), contributing to the large, slowly cycling DOC pool that resides there (480 Pg-C; Hansell et al., 2009). However, balancing oceanic DOC budgets is challenging, particularly in the transitional mesopelagic zone where isopycnal gradients and microbial activity results in a DOM pool with a wide spectrum of reactivity (Carlson and Hansell, 2015). Labile DOM is taken up by heterotrophic organisms within hours to days in shallow ocean depths. Refractory DOM is largely resistant to microbial degradation and therefore cycles on millennial timescales. It is proposed that the continous and/or repeated reworking of freshly produced DOM in surface waters results in the accumulation of recalcitrant DOM (Jiao et al., 2010), which is comprised of molecular components that fall along a reactivity continuum (Carlson and Hansell, 2015). Radiocarbon and stoichiometric analyses provide evidence for the accumulation of apparently old (∼6,000 14C-years; Williams and Druffel, 1987) and carbon-enriched (Hopkinson and Vallino, 2005) DOM in the deep ocean. However, the distribution of labile and refractory DOM can also be explained by the “dilution hypothesis,” which argues that the degradation of DOM is controlled by its concentration rather than its intrinsic chemical reactivity (Jannasch, 1967; Arrieta et al., 2015). If the formation of the recalcitrant DOM pool is not strictly dependent upon microbial lability, then a holistic approach, one that considers environmental redox conditions, concentration, time, etc. is needed to further refine current and predict future oceanic carbon cycles (see section “DOM Recalcitrance Is an Emergent Property”).
Although contemporary carbon flux estimates have benefited from a two-component model approach involving labile and refractory fractions (Williams and Druffel, 1987; Druffel et al., 1992; Bauer et al., 1998), spatial mapping of DOM throughout the global oceans reveals a more dynamic system at play (Hansell et al., 2009). Oceanic DOC concentrations range from 40 to 80 μM-C where observed gradients are driven by vertical stratification and mixing, biological degradation, and terrigenous inputs (Hansell et al., 2009). Although the formation and degradation of recalcitrant DOM occurs on relatively long timescales, the spatial and temporal variability of this accumulated fraction of DOM has a major impact on the long-term storage of carbon in the ocean’s interior, thus affecting global carbon cycles and associated climate change. As our analytical capabilities continue to advance, so should our understanding of the fine-scale processes that govern the global distribution of oceanic DOC. In turn, a clearer view of fine-scale biogeochemistry will allow for improved oceanic representation in global carbon cycling and Earth system models. This review of oceanic fluxes focuses upon DOC only (see section “Current Understanding of Marine DOM”). While some information on the cycling of organic heteroatoms exist (Pohlabeln and Dittmar, 2015; Gomez-Saez et al., 2016; Ksionzek et al., 2016; Pohlabeln et al., 2017; Broek et al., 2019), data available for dissolved organic nitrogen, sulfur, and phosphorous are extremely limited compared to DOC measurements. Here, we synthesize both well and poorly characterized fluxes of oceanic DOC, consider what is meant by DOM “recalcitrance,” spotlight new isotopic approaches for probing the oceanic distribution of DOC, and discuss changes to DOM quantity and composition that are expected to occur in response to our changing climate.
Current Understanding of Marine Dom
Implementing oceanic DOC in the global carbon cycle and associated climate models requires an improved quantitative understanding of marine DOC. Here, we provide an overview of DOC sources and sinks as currently estimated for the surface and interior ocean. These fluxes are summarized in Figure 1. The apparent imbalance between sources and sinks can be ascribed to unconstrained loss estimates within the water column by either DOC remineralization or sorption to particles. In this section, we also highlight research gaps that must be addressed for a more accurate view of marine DOC cycling and how it may be altered with future climatic and oceanic change.
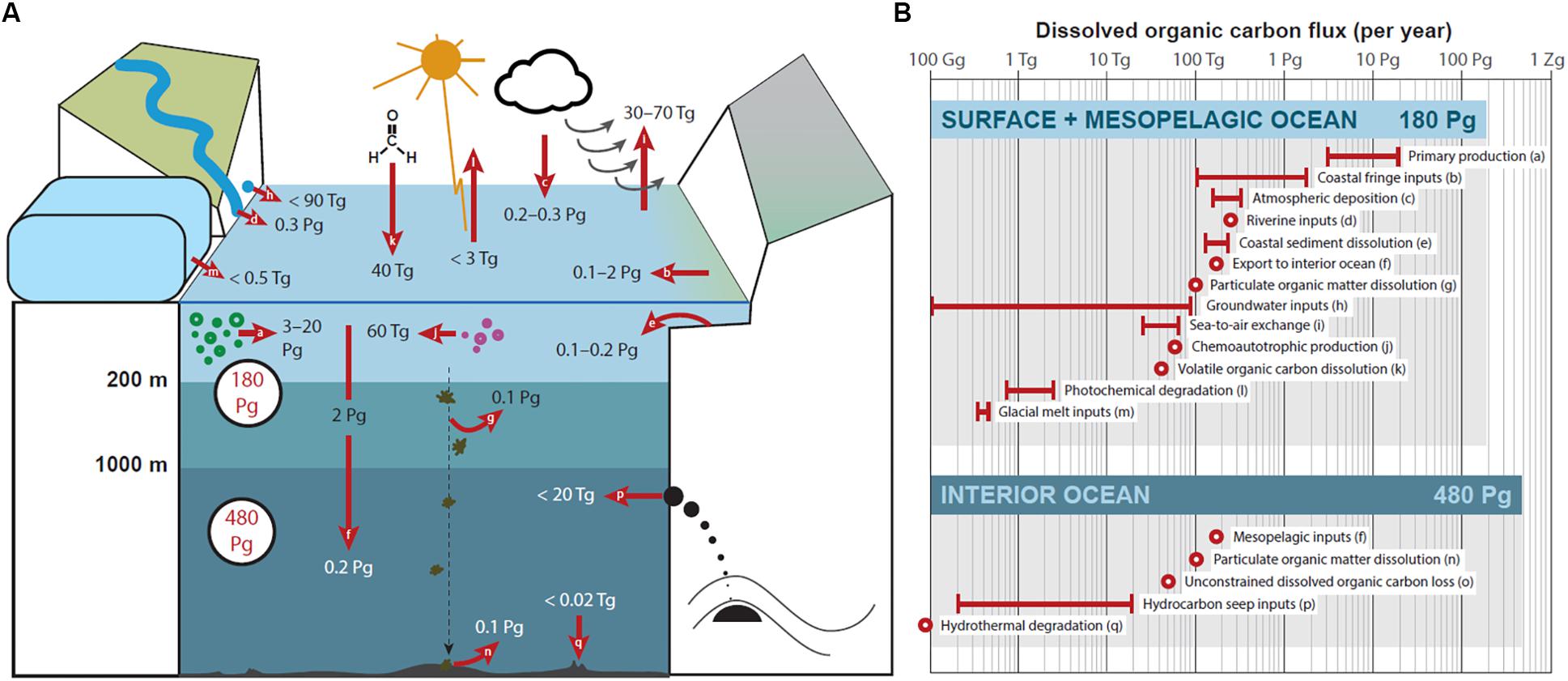
Figure 1. The current understanding of dissolved organic carbon (DOC) fluxes in the surface, mesopelagic, and interior ocean. In panel (A) oceanic DOC stocks are shown in black circles with red font and units are Pg-C. DOC fluxes are shown in black and white font and units are either Tg-C yr–1 or Pg-C yr–1. Letters in arrows and associated flux values correspond to descriptions displayed in (B), which lists sources and sinks of oceanic DOC described in the text (see section “Current Understanding of Marine DOM”).
Surface Ocean
Biotic Processes
Primary production (about 50 Pg-C yr–1) is the main source of DOC in oceanic surface waters (3–20 Pg-C yr–1) with significantly smaller contributions deriving from chemoautotrophic production (0.06 Pg-C yr–1) (Middelburg, 2011; Carlson and Hansell, 2015). Intracellular organic matter accumulates when cell division is limited. This excess material is subsequently discharged via extracellular release (Fogg, 1966; Engel et al., 2004). Specific mechanisms of release are yet not fully understood, but low molecular weight DOC can leak through the cell membrane directly (Fogg, 1966) and high molecular weight DOC is actively exudated via exocytosis or similar processes (Leppard, 1995). Extracellular release is a cellular overflow mechanism shedding excess carbon, where compounds released are depleted in biolimiting elements (Wood and Van Valen, 1990; Engel et al., 2002; Schartau et al., 2007). Thus, the extracellular release of carbon-enriched DOM represents a significant source for DOC in the upper ocean (Aluwihare et al., 1997) and partly explains seasonal variations observed for Redfield ratios (Williams, 1995; Søndergaard et al., 2000). DOC is also released when zooplankton feed on phytoplankton in surface waters. The production of DOC from “sloppy feeding” ranges from 3 to 70% of the total mass of phytoplankton carbon ingested and is generally a function of predator-to-prey size (Copping and Lorenzen, 1980; Bjornsen, 1988; Moller, 2005, 2007; Saba et al., 2011). The amount of DOC released during zooplankton grazing ranges from 2 to 10% of the total zooplankton carbon mass (Steinberg et al., 2000, 2002). Similarly, microzooplankton have been shown to ingest 20–50% of algal biomass carbon during grazing, the majority of which is subsequently released as DOC (Nagata et al., 2000). However, given the high degree of spatiotemporal variability of zooplankton feeding, global DOC flux values for grazing and sloppy feeding have not been adequately constrained (Steinberg and Landry, 2017).
Viral infections are a major cause of marine microbial mortality (Wilhelm and Suttle, 1999; Suttle, 2007) and result in cell lysis and release of DOM during cell death. An estimated 6–26% of annual marine primary production flows through the viral shunt producing 3–13 Pg-C as DOC per year (Wilhelm and Suttle, 1999; Carlson and Hansell, 2015). Compositionally, viral lysates are rich in dissolved amino acids, carbohydrates, and nucleic acids (Weinbauer and Peduzzi, 1995; Middelboe and Jorgensen, 2006; Holmfeldt et al., 2010). Most of the DOC produced in the surface ocean is then routed through microorganisms and remineralized to CO2 within an annual cycle (Azam et al., 1983). Only a small fraction of autotrophic DOC is converted to recalcitrant forms of DOC and survives long enough to be exported to the interior ocean (Gruber et al., 2006; Brophy and Carlson, 1989). Microbes utilize the diverse pool of organic compounds through direct uptake of small molecules or extracellular enzymatic hydrolysis to initiate decomposition (Weiss et al., 1991; Arnosti, 2004). The degradation of macromolecular structures is thereby linked enzymatic capabilities of the heterotrophic microbes.
Air-Sea Exchange
The transfer of organic matter from the atmosphere to the ocean occurs by dry deposition (settling of organic gases or particles) or by wet deposition (organic molecules dissolved in rainwater). Atmospheric deposition (wet plus dry) delivers 0.25 Pg-C yr–1 to the global surface ocean (Jurado et al., 2008; Kanakidou et al., 2012). However, atmospheric contributions to oceanic DOC depend largely upon the solubility of the deposited organic matter. Most wet deposition organics are presumed to be water soluble DOC (>90%; Kanakidou et al., 2012). Therefore, a lower limit for atmospheric DOC fluxes can be derived considering only wet deposition, which equates to 0.17 Pg-C yr–1, while an upper limit of 0.25 Pg-C yr–1 implies that all atmospherically deposited organic carbon dissolves within oceanic surface waters. Volatile organic molecules can also be transferred between the surface skin of the ocean and the overlying atmosphere (Liss et al., 2014). The efficiency of this ocean-to-air exchange is dependent upon molecular structure, current regimes, biological productivity, and light availability. Summed across all volatile organic compound classes, the ocean constitutes a net sink of short-lived gases [41 Tg-C yr–1; (Heikes et al., 2002; Marandino et al., 2005; Shim et al., 2007; Millet et al., 2008; Liss et al., 2014)]. Marine aerosols are produced from both primary (bursting bubbles and breaking waves) and secondary processes (nucleation and oxidative reactions) and are highly enriched in organic matter compared to seawater (Keene et al., 2007; O’Dowd and de Leeuw, 2007; Quinn et al., 2014). Model estimates suggest the ocean-air flux of organic matter from primary marine aerosol production (8–50 Tg-C yr–1) (Roelofs, 2008; Spracklen et al., 2008) can exceed that of organic matter transferred during secondary marine aerosol production (18 Tg-C yr–1) (Andreae and Rosenfeld, 2008). Primary marine organic aerosols are also comprised of roughly one third refractory carbon (Kieber et al., 2016; Beaupre et al., 2019). Thus, the atmospheric release of marine aerosols is a significant loss mechanism for recalcitrant DOM in the surface ocean.
Terrestrial Inputs
Globally, the export of riverine DOC to the coast is estimated to be 0.25 Pg-C yr–1 (Hedges et al., 1997; Raymond and Spencer, 2015). Within the last decade, efforts have been aimed to refine flux estimates from individual rivers. For example, due to increased temporal sampling resolution that now includes periods of high DOC export (spring freshet), estimated DOC fluxes for major Arctic Rivers have increased by ∼10 Tg-C yr–1 (Holmes et al., 2012). Rivers with high DOC yields export more carbon per unit area (e.g., the Amazon and Congo; Raymond and Spencer, 2015), and thus represent key targets for research seeking to constrain fluxes of terrestrial carbon to the ocean. Mountain glaciers export 0.58 Tg-C as DOC annually (Hood et al., 2015), but the majority of this glacial melt-derived DOC feeds primarily into fluvial systems and is thus captured by riverine DOC flux estimates and/or is highly bioavailable and degrades before reaching the ocean (Hemingway et al., 2019). At present, glacial melt represents a small source of terrestrial DOC to the coastal ocean (0.32–0.46 Tg-C yr–1; Priscu and Christner, 2004; Bamber et al., 2012; Hood et al., 2015). However, continued climate warming could unlock and mobilize a significant portion of the organic carbon currently stored in glacial ice (5.9 Pg-C) (Hood et al., 2015). Although glacial DOC is generally old, it is highly labile (Hood et al., 2009), which suggests the majority of glacier-derived DOC would be rapidly remineralized post-melt. However, more research is needed to estimate this potential loss of terrestrial DOC. In addition, it is unlikely that most riverine DOC would reach the open ocean due to efficient removal in estuaries and on the shelf (Fichot and Benner, 2014; Kaiser et al., 2017; Sharples et al., 2017).
Submarine groundwater flows from land to sea by gravity and is comprised of both a terrestrial component (meteoric groundwater) and a seawater component that circulates through the coastal aquifer through wave and tidal pumping (Burnett et al., 2003). Terrestrial DOM, which is transported directly by groundwater from inland watersheds, has global average DOC concentrations of 2.7 mg-C L–1 (McDonough et al., 2020). Thus, assuming fresh groundwater discharge equates to 0.3–10% of surface runoff, then terrestrial groundwater DOC inputs would account for 0.1–90 Tg-C yr–1 (Burnett et al., 2003; McDonough et al., 2020). However, coastal peatlands and marsh areas have been shown to spike local groundwater and recirculating seawater with DOC to increase concentrations up to 20-fold higher than the global average (Goñi and Gardner, 2003; Seidel et al., 2014). Therefore, subterranean inputs of terrestrial DOM may be much larger than currently assumed.
Coastal Fringe
Coastal fringe ecosystems are among the highest in productivity on Earth and include mangroves, salt marshes, and seagrass beds (Jennerjahn and Ittekkot, 2002). Intertidal sediments that house these highly productive coastal plant communities and their detritus are permanently flooded, which contributes to consistently high DOC yields. Combined, coastal fringe ecosystems are thought to export an estimated 0.1–1.9 Pg-C per year as DOC (Duarte, 2017). Since coastal fringe-derived DOC has been suggested to subsidize respiration in the open ocean (Barrón and Duarte, 2015), much of the DOC exported by these ecosystems may be rapidly remineralized by microbes. However, it is reported that as much as one third of the total DOC flux derived from coastal fringe ecosystems survives degradation and is eventually mobilized to the interior ocean (Krause-Jensen and Duarte, 2016). Further offshore, terrestrial and marine organic matter that is temporarily stored in coastal and continental margin sediments is laterally transported to deliver an additional 0.12–0.23 Pg-C yr–1 as DOC to open oceanic surface waters (Burdige et al., 1999; Burdige and Komada, 2015).
Photodegradation
The photomineralization of DOC in sunlit surface waters is an important, yet poorly understood, mechanism for the degradation of oceanic organic matter. Estimates currently suggest 0.7–2.7 Tg-C DOC is lost annually via photomineralization (Mopper and Kieber, 2002; Stubbins et al., 2006). The photomineralization of DOC depends upon several factors, including DOM composition, prior irradiation history, irradiation conditions, and the model used to fit the photobleaching kinetics (Mopper et al., 2014). DOM compounds exist along a continuum of varying photolability (Stubbins et al., 2010). For example, sulfur-containing compounds from sulfidic sediments are more photosensitive than bulk DOC (Gomez-Saez et al., 2017). Aromatic, refractory DOM is comprised of organic compounds that are most susceptible to photodegradation and have an estimated removal rate of up to 0.5 Tg-C yr–1 (Anderson and Williams, 1999; Stubbins et al., 2012). Partially degraded DOM compounds may be more bioavailable than organic molecules shielded from sunlight, thus coupled photo- and bio- degradation can remineralize more refractory DOC than photodegradation alone (Fichot and Benner, 2014; Shen and Benner, 2018). Degradation of recalcitrant DOC is likely also controlled by physical ocean circulation, specifically the rate at which deep water upwelling returns deep ocean DOC to the photic zone.
Interior Ocean
Net Losses
Of all the DOC that enters or is produced within surface waters each year, only 1.9 Pg-C yr–1 is exported to mesopelagic depths and a mere 0.18 Pg-C yr–1 survives long enough to reach the ocean’s interior (Carlson and Hansell, 2015). The exponential decrease in DOC export from the surface to deep ocean indicates the majority of exported DOC (∼99%) is respired in the water column, is incorporated into microbial biomass, or forms aggregates to sink and ultimately become stored in sediments as particulate organic carbon (POC). Respiration is the primary mode of DOC removal in surface waters, yet the complex cascade of processes that encompass a variety of biotic and abiotic mechanisms that control the formation of the recalcitrant DOM pool remains poorly understood. Upon entering the ocean’s interior, there is a net loss of refractory DOC (50 Tg-C yr–1), which cycles on millennial timescales (Hansell et al., 2009). The removal of refractory DOC from the deep ocean is ascribed to heterotrophic remineralization and/or sorption onto sinking particles, however, the relative contributions of these biogeochemical processes are largely unknown (Hansell et al., 2009).
Sorption and Dissolution
Organic matter-mineral interactions can lead to the ballasting and/or removal of DOC throughout the water column (Keil et al., 1994, 1997; Blattmann et al., 2018). The export of sediment from land to the ocean releases terrestrial organic matter, including labile compounds (Keil et al., 1994; Blattmann et al., 2018). The surfaces of terrestrial minerals are then repopulated with marine organic matter (Keil et al., 1997; Kennedy et al., 2002; Blattmann et al., 2018), an exchange process that depends upon sediment mineralogy (Blattmann et al., 2019). Since the degree of sorption is a function of surface area and DOC concentration (Henrichs, 1995; Kennedy et al., 2002), the removal of DOC from the water column likely scales with its concentration as well as availability of reactive sorption sites for organic compounds that persist in the interior ocean. While the loss and replacement of terrestrial and marine organic matter from mineral surfaces might pose no net sink or source of DOC, it may alter the composition and lability of the oceanic DOC pool. However, the abiotic exchange of organic matter between the operational POM and DOM fractions is a process that is not well understood (Carlson, 2002) and is in need of further research.
The imbalance of POM fluxes from the surface to depth and from depth to the seafloor (7.3 Pg-C yr–1) points to a likely source of DOC within the oceanic water column (Carlson and Hansell, 2015). POM loss processes are not well understood, but it is estimated that half is remineralized (3.6 Pg-C yr–1) and half is taken up by heterotrophic bacteria (3.6 Pg-C yr–1) (Carlson and Hansell, 2015). Sinking particles are enriched in organic and inorganic nutrients, thus representing energy hotspots in the open ocean. Microbes colonize the sinking particles and break down the POM into soluble components, releasing a trail of DOM that serves as a substrate to free floating microbes (Cho and Azam, 1988; Kiørboe, 2011). A small portion of this POM-derived DOC accumulates in the surface ocean at an estimated rate of 0.1 Pg-C yr–1 (Hansell, 2013; Carlson and Hansell, 2015). As particle flux decreases with depth, it is therefore unlikely that the flux of POM-derived DOC to the interior ocean is substantial. However, the dissolution of POM accumulated in ocean sediments contributes 0.1 Pg-C yr–1 (Dunne et al., 2007; Burdige and Komada, 2015), representing an important carbon and nutrient source to benthic microorganisms (Jones et al., 2014). A fraction of this sediment-derived DOC may also escape degradation and diffuse into deep waters overlying the seafloor, consistent with higher DOC concentrations observed in sediment porewaters compared to bottom waters (Burdige et al., 1992; Schmidt et al., 2011; Rossel et al., 2016). Consequently, sediment-derived DOC may subsidize benthic microbial communities and thus be an important source of the recalcitrant carbon observed in bottom waters (Burdige and Komada, 2015).
Seeps and Vents
Located along continental margins, hydrocarbon seeps represent an external source of DOC to the deep ocean. Current flux estimates (0.2–20 Tg-C yr–1) are based only upon data collected from known mud volcano seeps (Pohlman et al., 2010). These values likely underrepresent true DOC fluxes since current estimates omit inputs from other seep types, including seeps that have not yet been discovered. Depending upon the study, seep-derived DOC has been shown to either not accumulate or comprise up to 30% of DOC in the overlying water (Wang et al., 2001; Walker et al., 2017). Investigations that took place after the Deepwater Horizon oil spill (Gulf of Mexico) suggest microbial transformations of seep-derived hydrocarbons may result in the production of refractory DOC years after their release (Bianchi et al., 2014; Walker et al., 2017), thus contributing unknown quantities to the 14C-depleted DOC pool that resides in the deep ocean. Overall, hydrocarbon seeps likely represent a minor contribution to DOC in the ocean interior.
Concentrations of DOC in hydrothermal systems vary by an order of magnitude and are generally higher at low temperature diffusive systems compared to high temperature vents (Lang et al., 2006, 2010; Yamanaka et al., 2013; Hawkes et al., 2015). Hydrothermal circulation that exposes fluids to high temperatures (>400°C; Fontaine et al., 2009) is generally considered a sink of deep ocean DOC (Lang et al., 2006; Lin et al., 2012; Hawkes et al., 2015; Rossel et al., 2017), but these fluxes may be somewhat offset by cooler diffuse vent systems which are suggested to be a minor source of deep ocean DOC (Lang et al., 2006). Based upon data collected from DOC isolated by solid phase extraction, which is presumed to represent the less biologically labile fraction of marine DOC, high temperature fluids remove an estimated 0.02 Tg-C of refractory DOC per year (Hawkes et al., 2015). However, this flux estimate only considers the extractable portion of DOC, which becomes smaller with increased temperature (Hawkes et al., 2015, 2016) and largely excludes low molecular weight compounds (Klevenz et al., 2010; Lang et al., 2010; Fuchida et al., 2014; Reeves et al., 2014; McDermott et al., 2015; Longnecker et al., 2018). Hydrothermal vent-derived DOC may be a source of carbon for the deep ocean microbial communities (Winkel et al., 2014; Rossel et al., 2015), but fluxes have not yet been determined.
Dom Recalcitrance Is an Emergent Property
Dissolved organic matter is a heterogeneous pool of thousands, likely millions, of organic compounds. These compounds differ not only in composition and concentration (from pM to μM), but also originate from various organisms (phytoplankton, zooplankton, and bacteria) and environments (terrestrial vegetation and soils, coastal fringe ecosystems) and may have been produced recently or thousands of years ago. Moreover, even organic compounds deriving from the same source and of the same age may have been subjected to different processing histories prior to accumulating within the same pool of DOM.
Interior ocean DOM is a highly modified fraction that remains after years of exposure to sunlight, utilization by heterotrophs, flocculation and coagulation, and interaction with particles. Many of these processes within the DOM pool are compound- or class-specific. For example, condensed aromatic compounds are highly photosensitive (Stubbins et al., 2012), whereas proteins, carbohydrates, and their monomers are readily taken up by bacteria (Hodson et al., 1981; Hollibaugh and Azam, 1983; Ferguson and Sunda, 1984). Microbes and other consumers are selective in the type of DOM they utilize and typically prefer certain organic compounds over others. Consequently, DOM becomes less reactive as it is continually reworked. Said another way, the DOM pool becomes less labile and more refractory with degradation. As it is reworked, organic compounds are continually being added to the bulk DOM pool by physical mixing, exchange with particles, and/or production of organic molecules by the consumer community (Ogawa et al., 2001; Kaiser and Benner, 2008; Jiao et al., 2010; Shen and Benner, 2018). As such, the compositional changes that occur during degradation are more complex than the simple removal of more labile components and resultant accumulation of remaining, less labile compounds. The combined effect of the physicochemical and biological reworking of individual molecules on the overall molecular diversity and apparent recalcitrance of bulk interior ocean DOM is illustrated in Figure 2.
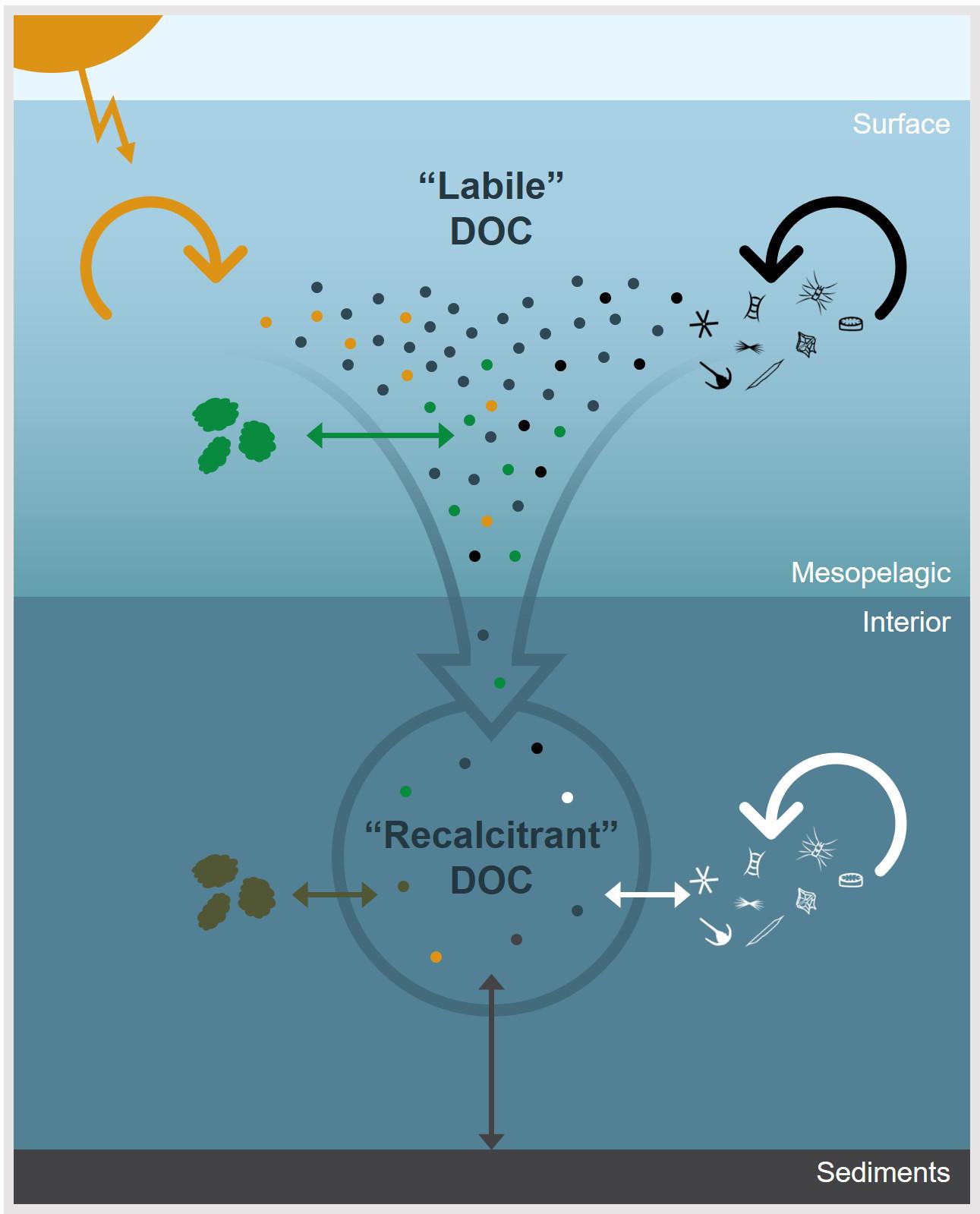
Figure 2. A conceptual diagram of some of the major environmental processes which control the apparent recalcitrance of oceanic DOC. The dots represent DOC molecules and arrows represent physicochemical and biological processes that impact DOC concentration and molecular composition. In the surface ocean, DOC derived from primary production is rapidly remineralized or transformed through microbial degradation (black arrow), photochemical degradation (yellow arrow), or particle exchange (green arrow). Labile components are removed down the water column and DOC becomes diluted by processes, such as particle exchange (brown arrow), sediment dissolution (gray arrow), and microbial reworking (white arrow), which continue to alter, add, and/or remove molecules from the bulk DOC pool. Thus, the apparent recalcitrance of DOC in the ocean’s interior is an emergent property that is largely controlled by environmental context.
Dissolved organic matter recalcitrance (i.e., its overall reactivity toward degradation and/or utilization) is therefore an emergent property. Thus, the perception of DOM recalcitrance changes during organic matter degradation and in conjunction with any other process that removes or adds organic compounds to the DOM pool under consideration. While it is possible to experimentally quantify the lability of very reactive, non-recalcitrant material on short time scales (Hodson et al., 1981; Hollibaugh and Azam, 1983; Ferguson and Sunda, 1984), we must infer DOM reactivities from chemical proxies, such as molecular composition, size distribution, and/or radiocarbon ages, for longer experimental temporal domains (i.e., more than 1 year). For example, older (14C-depleted) DOC is considered more refractory than young (14C-enriched) DOC and components with higher molecular mass are more reactive than components with lower molecular mass (Amon and Benner, 1994, 1996; Benner and Amon, 2015; Walker et al., 2016).
The reactivity of DOM is not only dependent upon intrinsic chemical characteristics of the organic compounds, but also depends upon the consumer community composition (e.g., microbes versus metazoans) (de Goeij et al., 2013). The availability of nutrients and biolimiting elements also comes into play, potentially limiting or promoting primary production and/or microbial consumption of DOM. Consumers must invest in structural or enzymatic machinery for harvesting certain compound classes. If target compound classes persist at very low concentration levels, the energetic gain from taking up these compounds may not be balanced by the cost of constructing the machinery required to utilize them. Consequently, even intrinsically labile DOM may not be readily degraded due to limited availability or access to such molecules (Jannasch, 1967; Arrieta et al., 2015). Thus, the apparent recalcitrance of DOM described by the dilution hypothesis is a property of the consumer or environmental context rather than the intrinsic chemical lability of substrate (Middelburg, 2015), yet provides a coherent explanation for the size and apparent age observed for the marine DOC pool (Mentges et al., 2019).
New Isotopic Approaches for Probing the Origin and Fate of Oceanic Doc
The marine DOC pool is molecularly complex, where compounds produced contemporaneously from the same source may have different degradation pathways and compounds that appear to be molecularly identical (i.e., have the same molecular formula or bulk isotopic composition) may have diverse sources and biogeochemical histories. Thus, in addition to analytical challenges, the flow of carbon through different organic molecules is highly convoluted, preventing a clear view of biotic drivers that control the production of recalcitrant DOC and its accumulation in the interior ocean. Natural abundance radiocarbon (14C) measurements are a powerful tool for assessing the age of oceanic DOC that cycles on centennial to millennial timescales (McNichol and Aluwihare, 2007), whereas stable carbon isotopic analyses (13C) supply information on carbon origins, degradation pathways, and transfer through marine food webs. At the bulk scale, radiocarbon data suggests oceanic DOC ranges in age from ca. 4,000 to ca. 6,000 14C-years with older ages in the interior ocean, although spatiotemporal resolution of these measurements is relatively limited (Beaupre, 2015). The World Ocean Circulation Experiment (WOCE) initiated a systematic increase in the resolution of dissolved inorganic carbon (DIC) 14C analyses, which has led to unprecedented insights into the dynamics of the marine carbon reservoir, particularly in the face of increasing atmospheric CO2 concentrations (McNichol et al., 2000). Thus, more extensive 14C analyses of DOC across our global oceans is needed to refine our understanding of DOC turnover and assess its role in modulating future climate scenarios. Bulk marine DOC is uniformly 13C-enriched and generally consistent with a marine phytoplankton source (Beaupre, 2015). However, compound-specific 13C analysis is an effective approach for discerning the source and fate of different DOM subcomponents, such as black carbon (Wagner et al., 2019). Similarly, 14C measurements on different DOC molecular fractions has revealed that the apparent age and lability is not evenly distributed throughout the marine DOM pool (Follett et al., 2014; Close, 2019; Ishikawa et al., 2019). Thus, targeted isotopic analyses are needed to unveil drivers of DOM accumulation and apparent recalcitrance in the interior ocean.
The enzymatic activity of heterotrophic microbes strongly affects the transformation of labile DOC into semi-labile and refractory DOC, thus altering the reactivity of DOC and modulating carbon fluxes in the ocean (Azam et al., 1983; Azam and Malfatti, 2007; Benner and Herndl, 2011). However, microbial activity is limited by both the accessibility of labile DOC components and nutrient availability. It is debated whether the balance between microbial growth and associated remineralization processes controls DOC cycling and whether this balance enhances or weakens the efficiency of the biological carbon pump (Azam and Malfatti, 2007). Biological carbon turnover is typically tracked by feeding molecular substrates (short-chain fatty acids, sugars, and amino acids) that have been uniformly labeled with stable (13C, 15N) or radiogenic (14C, 3H) isotopes (Ducklow et al., 1986; Jørgensen et al., 1993; Boschker et al., 1998; Keil and Kirchman, 1999; Boschker and Middelburg, 2002; Herndl et al., 2005). Some studies have utilized more complex isotopically labeled substrates, such as bacteria- or algae-derived DOC (Bronk and Glibert, 1993; Veuger et al., 2004; Bronk et al., 2007). However, bulk DOC does not permit distinction between the different carbon pools and uniformly labeled substrates omit the understanding of the fate of single carbon atoms within a molecule that are being differently used during uptake, biosynthesis, molecular transformation, and final release of DIC. Therefore, to specifically trace the microbial conversion of DOC to cellular biomolecules and metabolites or microbial remineralization of DOC to CO2, novel approaches based on position-specific and dual-isotope stable isotope probing (SIP), lipid radioisotope probing (RIP), and reversed isotope labeling (RIL) have been adapted from routine labeling techniques (Figure 3) (Wegener et al., 2012; Dippold and Kuzyakov, 2013; Kellermann et al., 2016; Dong et al., 2017, 2019; Evans et al., 2018, 2019; Aepfler et al., 2019).
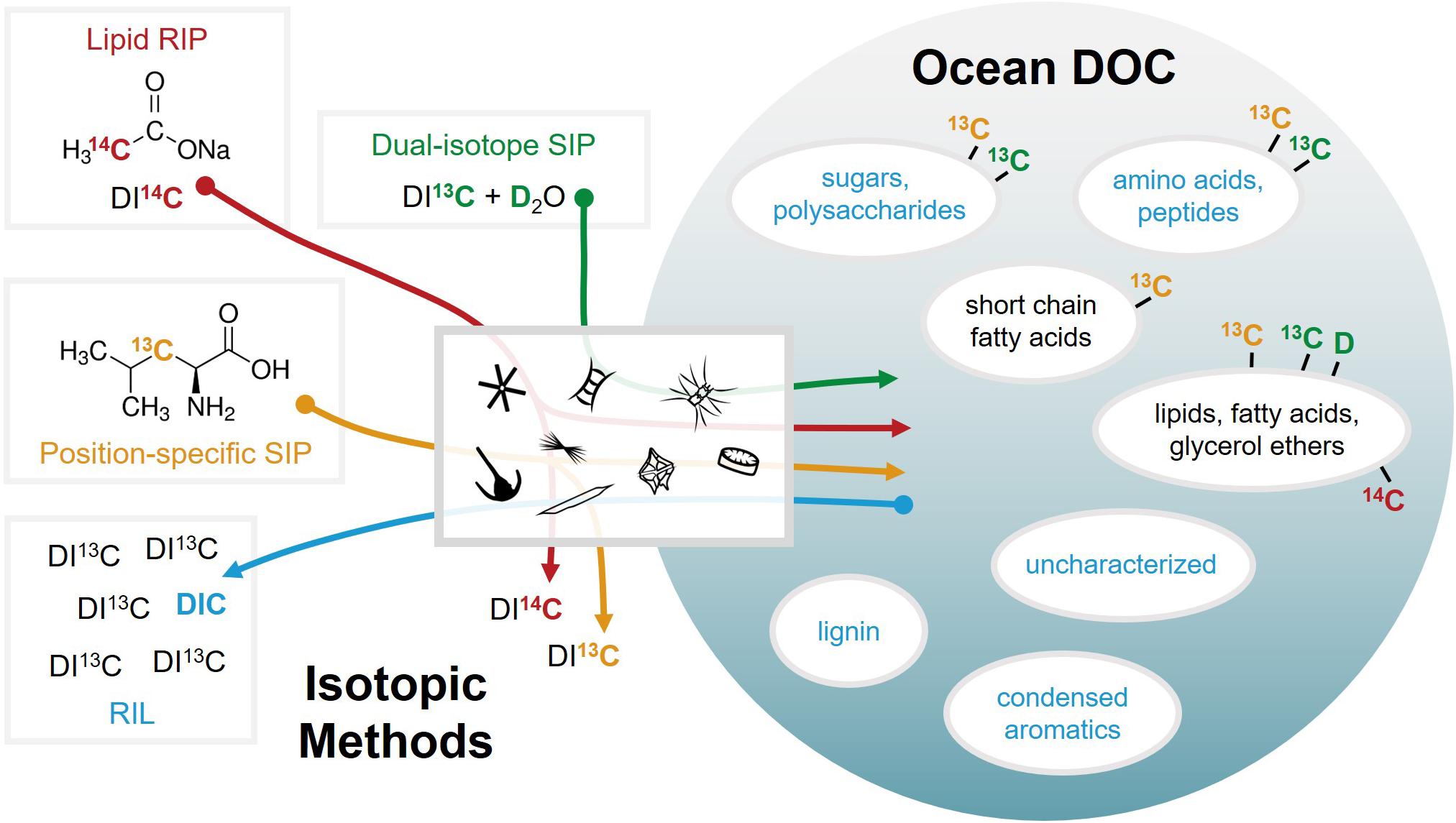
Figure 3. Emerging stable (13C) and radiocarbon (14C) isotopic techniques used to probe metabolic pathways for the biosynthesis and microbial turnover of DOC by planktonic communities in the ocean. These methods include position-specific and dual-isotope stable isotope probing (SIP), lipid radioisotope probing (RIP), and reversed isotope labeling (RIL). The sphere is shaded according to the apparent recalcitrance of DOC compound classes.
Position-specific SIP, where only one carbon position within a particular substrate molecule is labeled with 13C, has been applied extensively for revealing terrestrial carbon pathways (Kuzyakov and Galitsa, 1993; Fischer and Kuzyakov, 2010; Dijkstra et al., 2011; Dippold and Kuzyakov, 2013), but less so in the marine environment. Amino acids are one of the main substrates for heterotrophic bacteria and constitute a large fraction of their carbon demand (Jørgensen et al., 1993). Thus, the amino acids that occur as part of bulk DOC can be isotopically exploited to reveal key biosynthetic and metabolic pathways that link marine carbon and nitrogen cycles. Position-specific SIP has been used to reconstruct metabolic pathways of bacteria, identify substrate specificity of bacterial fatty acid biosynthesis, and quantify the release of metabolic products (e.g., short-chain fatty acids and DIC) (Aepfler et al., 2019). Dual-isotope lipid SIP, which combines D2O with 13C-labeled DIC, enables the direct quantitative tracking of both autotrophic carbon fixation and heterotrophic lipid production without having to provide an additional energy source in the form of excess carbon substrates (Wegener et al., 2016). This dual-isotopic approach has been utilized to investigate the metabolic activity of microbes in marine sediments, specifically for sulfate reducing bacteria (Wegener et al., 2012), the anaerobic oxidation of methane (Kellermann et al., 2012), and lipid biosynthesis therein (Kellermann et al., 2016). Lipid radioisotope probing has been similarly applied to better understand lipid biosynthetic pathways in marine planktonic archaea (Evans et al., 2018). Natural DOM and environmental organic compounds that are not available in a stable isotope or radiolabeled form are accessible to turnover studies using RIL, in which a pool of 13C-labeled DIC is continuously diluted during microbial degradation of a specific organic substrate or DOM at natural carbon isotope abundance (Dong et al., 2017, 2019). Thus, the change in the isotopic composition of DIC enables quantification of microbial remineralization by mass balance calculations.
Dom Future Projections
Concentrations of DOC in marine surface waters are closely linked to biogeochemical cycles and physical forcings. The oligotrophic subtropical gyres typically show higher concentrations (65–80 μM-C) of DOC than subpolar regions and the circumpolar Southern Ocean (40–50 μM-C) (Hansell, 2013). The accumulation and export of DOC in the subtropical gyres contributes to organic carbon flux from surface waters and supports the metabolic demand of heterotrophic microbes in the upper mesopelagic zone (Carlson et al., 2004). Ultimately, the production of DOC in the surface ocean is limited by the magnitude of primary production, which in turn depends on nutrient supply (Carlson and Hansell, 2015; Romera-Castillo et al., 2016). Within these constraints, an intimate feedback between climate systems, nutrient regimes, and community structure can modulate surface DOC inventories on seasonal and decadal time scales. A multiyear net increase of DOC inventories (∼300 mmol-C m2 yr–1) has been reported within the North Pacific Subtropical Gyre, primarily driven by decadal climate variations, along with concurrent shifts in the plankton community and transition from nitrogen- to phosphorus-limitation (Church et al., 2002). The majority of DOC accumulating in the surface ocean is rapidly remineralized in the upper mesopelagic zone (Abell et al., 2000; Kaiser and Benner, 2012). Since a large portion of net primary production is routed through active microbial food webs, the production of recalcitrant DOC should scale proportionally with DOC production (Karl, 1999; Jiao et al., 2010). Heterotrophic microbes transform ∼0.02% of primary production carbon into recalcitrant DOC (Benner and Herndl, 2011). Subtropical gyres cover ∼60% of the total ocean area (Eppley and Peterson, 1979) and may be expanding in size (Polovina et al., 2008; Sharma et al., 2019). Therefore, small changes to DOC concentrations in these regions can significantly change the size of ocean DOC inventories and funnel a greater fraction to the interior ocean as refractory DOC. Given the multivariate approach required, it is unclear how current oceanic DOC inventories may be altered in response to continued climate change. Here, we review predictions of how climate change will impact microbial and terrestrial DOC fluxes to the ocean and point out gaps in the current knowledge needed to refine our view of future oceanic DOM cycling.
Ocean Warming and Acidification
As a result of climate change, a concomitant rise in atmospheric temperatures and CO2 levels have directly contributed to the warming and acidification of global oceans (Doney et al., 2009; Legendre et al., 2015). Controlled experiments have consistently demonstrated enhanced primary production and release of DOC in response to elevated ambient temperatures and CO2 levels (Egge et al., 2009; Wohlers et al., 2009; Engel et al., 2011, 2013; Kim et al., 2011; Liu et al., 2017; Novak et al., 2018; Sugie et al., 2018). However, enhanced primary productivity may ultimately be balanced or exceeded by respiration rates in a warmer, more acidic ocean (Pomeroy and Wiebe, 2001; Vaquer-Sunyer et al., 2010). Enhanced DOC production under high CO2 conditions was often accompanied by increased activity of microbial consumers (Engel et al., 2013; Endres et al., 2014), thus counteracting DOC accumulation. Higher DOM degradation rates may be related to extracellular hydrolytic enzymes, which exhibit optimum activity rates under more acidic conditions (Piontek et al., 2010; Endres et al., 2013). However, recent findings suggest that DOC production and quality does not substantially change in the long-term, because either the local phytoplankton communities become nutrient-depleted or the newly produced organic matter is utilized by fast-adapting heterotrophic microbial communities (Zark et al., 2015, 2017; Aparicio et al., 2016; Moran et al., 2016; Kim et al., 2018; Viviani et al., 2018). Regardless of the future balance between production and respiration, modeling of oceanic DOC decay rates suggests that a uniform 1°C warming of the ocean would decrease the global DOC reservoir by ∼7 Pg-C (Lønborg et al., 2018). Given higher activation energies for refractory DOC compared to labile DOC, increased temperatures would also disproportionately impact the degradation of recalcitrant DOC presumed to accumulate in the interior ocean.
Thermohaline circulation in the Atlantic and Pacific Oceans is predicted to decrease within the next century (McPhaden and Zhang, 2002; Stocker et al., 2013). Modeling efforts that seek to couple biogeochemical fluxes to ocean circulation patterns suggest that density stratification will be strengthened as a result of ocean warming (Capotondi et al., 2012). It is unknown whether these combined effects would significantly affect losses of DOM by abiotic processes, such as photodegradation or outgassing (Legendre et al., 2015). However, a reduction in the rate at which deep waters are returned to the surface ocean would significantly impact nutrient availability and primary production and could therefore alter the amount and/or quality of DOM that accumulates at shallower depths or is shunted to the interior ocean. Based upon satellite data, warmer surface ocean temperatures have been shown to diminish the supply of nutrients needed to carry out photosynthesis, and thus reduce oceanic biomass and primary productivity (Behrenfeld et al., 2006; Doney, 2006). These observations are in opposition with results from controlled experiments described previously, which generally show warming and acidification to enhance primary production. This discrepancy spotlights the interdisciplinary and multivariate approach needed to accurately predict DOC production and turnover in the surface ocean.
Oxygen Minimum Zone Expansion
The persistence of organic carbon in the ocean is effectively regulated by O2 exposure time (Hartnett et al., 1998), which has been linked to the low density of metazoans (Jessen et al., 2017; Middelburg, 2018) and the oxidation reaction energetics of organic matter, which predict the persistence of complex, reduced organic molecules in anoxic settings (LaRowe and Van Cappellen, 2011). As oxygen minimum zones expand in the ocean due to decreased ventilation and increased temperature (Breitburg et al., 2018), the limited catabolic potential under such conditions could render specific molecular structures recalcitrant. Anoxia may have led to larger marine DOC reservoirs in the geological past, as tentatively suggested for the Precambrian and Eocene oceans (Rothman et al., 2003; Sexton et al., 2011; Ridgwell and Arndt, 2015). The deep waters of the Black Sea exhibit DOC concentrations that are 2.5-fold higher than DOC concentrations in the deep ocean (Ducklow et al., 2007; Margolin et al., 2016). It remains unclear whether the DOC accumulation is directly linked to terrestrial DOC inputs to this anoxic basin (Margolin et al., 2016) or is derived from a mixture of terrestrial, marine, and autochthonous DOC (Margolin et al., 2018). The East Japan Sea is a semi-enclosed marginal sea where DOC accumulation is attributed to decreased reactivity resulting from low bottom water temperatures (<1°C) (Kim et al., 2015). Therefore, although these two basins are imperfect modern analogs for future oxygen minimum zone expansion in the global ocean, the Black and East Japan Seas provide contrasting insights as to how the accumulation of marine DOC may increase under reduced O2 conditions. At present, DOC concentrations in oxygen minimum zones of the Eastern Tropical North Pacific and Eastern Tropical South Pacific Oceans are similar to concentrations observed for the open ocean and are consistent with general water circulation patterns1. However, rapid utilization of semi-labile DOM has been observed for the upper oxycline in waters of the Eastern Tropical South Pacific (Loginova et al., 2019), suggesting a high degree of diagenetic DOM alteration at shallow oxygen minimum zone depths compared to the open ocean and to deeper oxic waters. This may be explained by the upwelling of altered DOM and/or extensive heterotrophic DOM utilization. Based upon these observations it is unclear how changing O2 concentrations in the ocean would affect the size of DOC reservoirs and highlights a need for further research in this area.
Terrestrial Inputs
Riverine DOC fluxes will be closely tied to the effects of climate change within the watershed, particularly via enhanced warming and the increased frequency and severity of hydrological events. Climate change impacts will be enhanced in high latitude regions, where warming occurs at a rate twice that of the global average (Jeffries and Richter-Menge, 2012; Stocker et al., 2013). Glacial melt will not only increase freshwater runoff, but continued warming would also release the ∼6 Pg-C currently stored as DOC in glaciers globally (Hood et al., 2015). Most of the Earth’s soil organic carbon is stored in the Arctic, including permafrost (1,300 Pg-C of POC plus DOC) (Hugelius et al., 2014). Increased temperatures will unlock organic matter preserved in these soils, resulting in enhanced DOC export via rivers draining Arctic landscapes (Freeman et al., 2001; de Wit et al., 2016; Wild et al., 2019). In regions where permafrost carbon is a major contributor, riverine DOC may be quickly remineralized to CO2 before reaching the open ocean (Mann et al., 2015), potentially furthering climate change feedback. In areas of increased runoff, higher DOC concentrations paired with higher discharge will result in concomitantly higher DOC fluxes (Raymond et al., 2016). As such, decreased water residence times during hydrological events will quickly export sediment- and DOC-enriched river water to the coastal ocean. In conjunction with landscape alteration, hydrological events may also result in the enhanced mobilization of aged terrestrial carbon reservoirs (Marwick et al., 2015; Barnes et al., 2018).
The fate of terrestrial DOC also relies upon estuarine behavior and the resilience of coastal fringe ecosystems. These regions are among the highest in productivity on Earth and face increased pressure from anthropogenic and climatic forces (Erwin, 2009; Mitsch and Hernandez, 2013). With continued sea level rise, it is unknown how the migration and/or collapse of these coastal vegetation communities will impact the relative stability of organic matter stored in sediments (Kirwan et al., 2013; Sjøgaard et al., 2017; Ruiz-Fernández et al., 2018; Macreadie et al., 2019). Thus, the net flux of DOC from fringe ecosystems, particularly in tropical regions, is not well constrained. At high latitudes, long-term increases in DOC concentration in coastal surface waters have been observed (Worrall et al., 2004; Filella and Rodríguez-Murillo, 2014). However, when other factors, such as rainfall, land management, and salinity, are also considered, a net decrease in low latitude DOC concentrations is indicated (Regier et al., 2016). Large uncertainties associated with estimating the regional distribution and total land area for sea grasses, salt marshes, and mangrove forests is a major obstacle in constraining global DOC flux values for these coastal ecosystems (Duarte, 2017). These habitats face the fastest loss rates of any ecosystem (1–3% per year), largely as a result of anthropogenic impacts (Duarte, 2017). Therefore, spatial resolution of these ecosystems is critical for enhancing our current and future understanding of their role as an oceanic DOC source.
Anthropogenic Inputs
Poor spatiotemporal resolution of atmospherically deposited organic carbon prevents accurate global ocean flux estimates and highlights a critical area for future research. However, perhaps even more importantly, atmospheric deposition is also a major source of nutrients, such as nitrogen and phosphorus. Ocean stratification driven by a warming climate is expected to further enhance the relative impacts of atmospheric inputs (Kanakidou et al., 2012). Roughly half of all deposited organic nitrogen is derived from human activities (Kanakidou et al., 2012) and primary production has been shown to correlate with anthropogenic aerosol deposition globally (Wang et al., 2015). Paired with riverine export, the deposition of industrial aerosols in northwestern Pacific Ocean has converted surface waters from being nitrogen-limited to phosphorus-limited (Kim et al., 2011). The interplay of atmospheric deposition, ocean warming, and stratification is complex. However, net gains in primary production with continued industrialization and climate change would probably be significant (St-Laurent et al., 2017) and may enhance local DOC export.
Although wildfires are naturally occurring and have punctuated Earth’s history since the emergence of land plants (Scott and Glasspool, 2006), anthropogenic climate change, land use change, and fire suppression practices are enhancing the frequency and severity of vegetation fires (Flannigan et al., 2009; Krawchuk et al., 2009). Although the production of refractory charcoal during wildfires may help mitigate the release of CO2 globally during burn events (Jones et al., 2019), a considerable portion of the charred organic matter is carried by wind to coastal and offshore surface waters (Jurado et al., 2008). Studies investigating the composition of deposited pyrogenic aerosols are limited but appear to be enriched in nitrogen (Bauters et al., 2018), which may fuel primary production or alter local microbial communities. Despite increased occurrence of wildfire in coastal areas, little is known regarding the short- and long-term impacts and fluxes of deposited ash and smoke in oceanic surface waters.
Increased riverine nutrient concentrations resulting from agricultural development and urbanization contribute to coastal eutrophication and hypoxia (Rabalais et al., 2009; Gilbert et al., 2010). Increased primary productivity paired with O2 depletion in coastal waters may result in favorable conditions for the accumulation of excess organic matter in sediments. However, predicted increases in the frequency and severity of storm events (e.g., hurricanes), which oxygenate surface waters via mixing and freshwater inputs, could temporarily offset sedimentary organic matter storage. Given that coastal sediments are an intermediate reservoir for organic matter, lateral transport of accumulated carbon to the open ocean could increase as a result of nutrient inputs, but research is needed to confirm this. Finally, the input of microplastics to the ocean is estimated to be 1–4 Tg-C yr–1 (Jambeck et al., 2015). Exposure to sunlight can degrade microplastics, which undergo photodissolution to produce DOC, although photochemical conversion rates are very low (Zhu et al., 2020). Thus, contributions of plastic-derived DOM to the total oceanic DOC pool are likely negligible.
Conclusion
The total DOC flux from external sources is similar to the export of DOC to the mesopelagic and an order of magnitude greater than the export of DOC to the interior ocean. Therefore, even small variations in the amount or composition of external DOC exported to the ocean could ultimately impact the sum of DOC transferred from the surface to the interior ocean each year. However, at present, the slight imbalance between primary production and respiration in surface waters is the driver of recalcitrant DOC accumulation. Therefore, constraining oceanic DOC fluxes through the microbial food web relies upon both a comprehensive understanding of metabolic pathways and the spatiotemporally refined mapping of plankton and bacterial communities. Broad application of novel stable and radiocarbon isotopic techniques will pave the way for a coherent understanding of marine organic carbon cycling by revealing predominant biosynthetic and degradation pathways that control DOM production and turnover. Isotopic analyses, particularly compound-specific measurements, can be expensive and require large sample sizes in order to obtain robust isotopic signatures. An increased demand for DOC stable and radiocarbon isotopic measurements would continue to fuel ongoing analytical innovations that allow for lower analysis cost and sample size requirements. As we continue to accumulate isotopic measurements, these data should also be paired with less expensive, high throughput analyses (e.g., fluorescence spectroscopy) to allow for the extrapolation of stable and radiocarbon signatures more broadly throughout the global ocean. At present, the limited resolution of surface water plankton and bacterial populations, which vary significantly with latitude and coastal proximity (Fuhrman et al., 2008; Zinger et al., 2011; Sunagawa et al., 2015; Arteaga et al., 2016), prevents a globally relevant view of DOC production. Therefore, research efforts should also focus upon the in situ variability and activity of marine microbial communities, particularly in surface waters. A fine-scale approach, both molecularly and spatiotemporally, is necessary to refine the current view of oceanic DOC cycling so that we may accurately gauge the response of this major carbon store to anthropogenic perturbations and global climate change.
Author Contributions
SW, FS, KK, CH, HW, PR, RH, ME, JM, AE, TB, TC, SL, GG-S, SP-G, RB, and VG participated in formative discussions as part of the DOM Working Group while attending the Marine Organic Biogeochemistry workshop held at the Hanse-Wissenschaftskolleg Institute for Advanced Study (Delmenhorst, Germany) in April 2019. SW, FS, KK, CH, HW, PR, RH, ME, JM, AE, TB, TC, SL, GG-S, SP-G, RB, and VG wrote and/or provided feedback on sections of the manuscript. SW compiled and organized co-author contributions and wrote the final version of the manuscript. All authors have read and approved the submitted version.
Funding
This work was funded by the Deutsche Forschungsgemeinschaft (DFG, German Research Foundation) project number 422798570. The Hanse-Wissenschaftskolleg and the Geochemical Society provided funding for the conference. Additional support was provided by the National Science Foundation OCE #1756812 to SW. TB acknowledges funding from ETH Zürich and JAMSTEC. JM was supported by the Netherlands Earth System Science Centre. SP-G was funded by COPAS Sur-Austral (CONICYT PIA APOYO CCTE AFB170006). GG-S acknowledges funding from DFG, DI 842/6-1.
Conflict of Interest
The authors declare that the research was conducted in the absence of any commercial or financial relationships that could be construed as a potential conflict of interest.
Acknowledgments
The authors thank C. Arnosti, T. Dittmar, K.-U. Hinrichs, C. Lee, and S. Wakeham for organizing the Marine Organic Biogeochemistry workshop and leading thought-provoking discussions that resulted in the writing of this manuscript.
Footnotes
References
Abell, J., Emerson, S., and Renaud, P. (2000). Distributions of TOP, TON and TOC in the North Pacific subtropical gyre: implications for nutrient supply in the surface ocean and remineralization in the upper thermocline. J. Mar. Res. 58, 203–222. doi: 10.1357/002224000321511142
Aepfler, R., Bühring, S. I., and Elvert, M. (2019). Substrate characteristic bacterial fatty acid production based on amino acid assimilation and transformation in marine sediments. FEMS Microbiol. Ecol. 95:fiz13. doi: 10.1093/femsec/fiz131
Aluwihare, L. I., Repeta, D. J., and Chen, R. F. (1997). A major biopolymeric component of dissolved organic carbon in surface seawater. Nature 387, 166–169. doi: 10.1038/387166a0
Amon, R. M. W., and Benner, R. (1994). Rapid cycling of high molecular weight dissolved organic matter in the ocean. Nature 369, 549–552. doi: 10.1038/369549a0
Amon, R. M. W., and Benner, R. (1996). Bacterial utilization of different size classes of dissolved organic matter. Limnol. Oceanogr. 41, 41–51. doi: 10.4319/lo.1996.41.1.0041
Anderson, T. R., and Williams, P. J. (1999). A one-dimensional model of dissolved organic carbon cycling in the water column incorporating combined biological-photochemical decomposition. Glob. Biogeochem. Cycles 13, 337–349. doi: 10.1029/1999gb900013
Andreae, M. O., and Rosenfeld, D. (2008). Aerosol-cloud-precipitation interactions. Part 1. The nature and sources of cloud-active aerosols. Earth Sci. Rev. 89, 13–41. doi: 10.1016/j.earscirev.2008.03.001
Aparicio, F. L., Nieto-Cid, M., Borrull, E., Calvo, E., Pelejero, C., Sala, M. M., et al. (2016). Eutrophication and acidification: Do they induce changes in the dissolved organic matter dynamics in the coastal Mediterranean Sea? Sci. Total Environ. 56, 179–189. doi: 10.1016/j.scitotenv.2016.04.108
Arnosti, C. (2004). Speed bumps and barricades in the carbon cycle: substrate structural effects on carbon cycling. Mar. Chem. 92, 263–273. doi: 10.1016/j.marchem.2004.06.030
Arrieta, J. M., Mayol, E., Hansman, R. L., Herndl, G. J., Dittmar, T., and Duarte, C. M. (2015). Dilution limits dissolved organic carbon utilization in the deep ocean. Science 348, 331–333. doi: 10.1126/science.1258955
Arteaga, L., Pahlow, M., and Oschlies, A. (2016). Modeled Chl:C ratio and derived estimates of phytoplankton carbon biomass and its contribution to total particulate organic carbon in the global surface ocean. Glob. Biogeochem. Cycles 30, 1791–1810. doi: 10.1002/2016GB005458
Azam, F., Fenchel, T., Field, J. G., Gray, J. S., Meyer-Reil, L. A., and Thingstad, F. (1983). The ecological role of water-column microbes in the sea. Mar. Ecol. Prog. Ser. 10, 257–263. doi: 10.3354/meps010257
Azam, F., and Malfatti, F. (2007). Microbial structuring of marine ecosystems. Nat. Rev. Microbiol. 5, 782–791. doi: 10.1038/nrmicro1747
Bamber, J., van den Broeke, M., Ettema, J., Lenaerts, J., and Rignot, E. (2012). Recent large increases in freshwater fluxes from Greenland into the North Atlantic. Geophys. Res. Lett. 39:L19501.
Barnes, R. T., Butman, D. E., Wilson, H. F., and Raymone, P. A. (2018). Riverine export of aged carbon driven by flow path depth and residence time. Environ. Sci. Technol. 52, 1028–1035. doi: 10.1021/acs.est.7b04717
Barrón, C., and Duarte, C. M. (2015). Dissolved organic carbon pools and export from the coastal ocean. Glob. Biogeochem. Cycles 29, 1725–1738. doi: 10.1002/2014GB005056
Bauer, J. E., Druffel, E. R. M., Williams, P. M., Wolgast, D. M., and Griffin, S. (1998). Temporal variability in dissolved organic carbon and radiocarbon in the eastern North Pacific Ocean. J. Geophys. Res. Oceans 103, 2867–2881. doi: 10.1029/97JC02545
Bauters, M., Drake, T. W., Verbeeck, H., Bodé, S., Hervé-Fernández, P., Zito, P., et al. (2018). High fire-derived nitrogen deposition on central African forests. Proc. Nat. Acad. Sci. U.S.A. 115, 549–554. doi: 10.1073/pnas.1714597115
Beaupre, S. R. (2015). “The carbon and isotopic composition of marine DOC,” in Biogeochemistry of Marine Dissolved Organic Matter, 2nd Edn, eds D. A. Hansell and C. A. Carlson (Amsterdam: Elsevier Inc), 335–364.
Beaupre, S. R., Kieber, D. J., Keene, W. C., Long, M. S., Maben, J. R., Lu, X., et al. (2019). Oceanic efflux of ancient marine dissolved organic carbon in primary marine aerosol. Sci. Adv. 5:eaax6535. doi: 10.1126/sciadv.aax6535
Behrenfeld, M. J., O’Malley, R. T., Siegel, D. A., McClain, C. R., Sarmiento, J. L., Feldman, G. C., et al. (2006). Climate-driven trends in contemporary ocean productivity. Nat. Lett. 444, 752–755. doi: 10.1038/nature05317
Benner, R., and Amon, R. M. W. (2015). The size-reactivity continuum of major bioelements in the ocean. Annu. Rev. Mar. Sci. 7, 185–205. doi: 10.1146/annurev-marine-010213-135126
Benner, R., and Herndl, G. J. (2011). “Bacterially derived dissolved organic matter in the microbial carbon pump,” in Microbial Carbon Pump in the Ocean, eds N. Jiao, F. Azam, and S. Sanders (Washington, DC: Science/AAAS), 46–48.
Bianchi, T. S., Osburn, C., Shields, M. R., Yvon-Lewis, S., Young, J., Guo, L., et al. (2014). Deepwater Horizon oil in Gulf of Mexico waters after 2 years: transformation into the dissolved organic matter pool. Environ. Sci. Technol. 48, 9288–9297. doi: 10.1021/es501547b
Bjornsen, P. K. (1988). Phytoplankton exudation of organic matter: Why do healthy cells do it? Limnol. Oceanogr. 33, 151–154. doi: 10.4319/lo.1988.33.1.0151
Blattmann, T. M., Liu, Z., Zhang, Y., Zhao, Y., Haghipour, N., Montluçon, D. B., et al. (2019). Mineralogical control on the fate of continentally derived organic matter in the ocean. Science 366, 742–745. doi: 10.1126/science.aax5345
Blattmann, T. M., Zhang, Y., Zhao, Y., Wen, K., Lin, S., Li, J., et al. (2018). Contrasting fates of petrogenic and biospheric carbon in the South China Sea. Geophys. Res. Lett. 45, 9077–9086. doi: 10.1029/2018gl079222
Boschker, H. T. S., and Middelburg, J. J. (2002). Stable isotopes and biomarkers in microbial ecology. FEMS Microbiol. Ecol. 40, 85–95. doi: 10.1111/j.1574-6941.2002.tb00940.x
Boschker, H. T. S., Nold, S. C., Wellsbury, P., Bos, D., de Graaf, W., Pel, R., et al. (1998). Direct linking of microbial populations to specific biogeochemical processes by 13C-labeling of biomarkers. Nature 392, 801–805. doi: 10.1038/33900
Breitburg, D., Levin, L. A., Oschlies, A., Grégoire, M., Chavez, F. P., Conley, D. J., et al. (2018). Declining oxygen in the global ocean and coastal waters. Science 359:eaam7240. doi: 10.1126/science.aam7240
Broek, T. A. B., Bour, A. L., Ianiri, H. L., Guilderson, T. P., and McCarthy, M. D. (2019). Amino acid enantiomers in old and young dissolved organic matter: implications for a microbial nitrogen pump. Geochim. Cosmochim. Acta 247, 207–219. doi: 10.1016/j.gca.2018.12.037
Bronk, D. A., and Glibert, P. M. (1993). Application of a 15N tracer method to the study of dissolved organic nitrogen uptake during spring and summer in Chesapeake Bay. Mar. Biol. 115, 501–508. doi: 10.1007/bf00349849
Bronk, D. A., See, J. H., Bradley, P., and Killberg, L. (2007). DON as a source of bioavailable nitrogen for phytoplankton. Biogeosciences 4, 283–296. doi: 10.1016/j.scitotenv.2020.137837
Brophy, J. E., and Carlson, D. J. (1989). Production of biologically refractory dissolved organic carbon by natural seawater microbial populations. Deep Sea Res. I 36, 497–507. doi: 10.1016/0198-0149(89)90002-2
Burdige, D. J., Alperin, M. J., Homstead, J., and Martens, C. S. (1992). The role of benthic fluxes of dissolved organic carbon in oceanic and sedimentary carbon cycling. Geophys. Res. Lett. 19, 1851–1854. doi: 10.1029/92GL02159
Burdige, D. J., Berelson, W. M., Coale, K. H., McManus, J., and Johnson, K. S. (1999). Fluxes of dissolved organic carbon from California continental margin sediments. Geochim. Cosmochim. Acta 63, 1507–1515. doi: 10.1016/S0016-7037(99)00066-6
Burdige, D. J., and Komada, T. (2015). “Sediment pore waters,” in Biogeochemistry of Marine Dissolved Organic Matter, 2nd Edn, eds D. A. Hansell and C. A. Carlson (Amsterdam: Elsevier Inc), 535–577. doi: 10.1016/b978-0-12-405940-5.00012-1
Burnett, W. C., Bokuniewicz, H., Huettel, M., Moore, W. S., and Taniguchi, M. (2003). Groundwater and pore water inputs into the coastal zone. Biogeochemistry 66, 3–33. doi: 10.1023/b:biog.0000006066.21240.53
Capotondi, A., Alexander, M. A., Bond, N. A., Curchitser, E. N., and Scott, J. (2012). Enhanced upper-ocean stratification with climate change in the CMIP3 models. J. Geophys. Res. 117:C04031. doi: 10.1029/2011JC007409
Carlson, C. A. (2002). “Production and removal processes,” in Biogeochemistry of Marine Dissolved Organic Matter, eds D. A. Hansell and C. A. Carlson (San Diego, CA: Academic Press), 91–151. doi: 10.1016/b978-012323841-2/50006-3
Carlson, C. A., Giovannoni, S. J., Hansell, D. A., Goldberg, S. J., Parsons, R., and Vergin, K. (2004). Interactions among dissolved organic carbon, microbial processes, and community structure in the mesopelagic zone of the northwestern Sargasso Sea. Limnol. Oceanogr. 49, 1073–1083. doi: 10.4319/lo.2004.49.4.1073
Carlson, C. A., and Hansell, C. A. (2015). “DOM sources, sinks, reactivity, and budgets,” in Biogeochemistry of Marine Dissolved Organic Matter, 2nd Edn, eds D. A. Hansell and C. A. Carlson (Amsterdam: Elsevier Inc), 65–126. doi: 10.1016/b978-0-12-405940-5.00003-0
Carlson, C. A., Hansell, D. A., Nelson, N. B., Siegel, D. A., Smethie, W. M., Khatiwala, S., et al. (2010). Dissolved organic carbon export and subsequent remineralization in the mesopelagic and bathypelagic realms of the North Atlantic basin. Deep Sea Res. II 57, 1433–1445. doi: 10.1016/j.dsr2.2010.02.013
Cho, B. C., and Azam, F. (1988). Major role of bacteria in biogeochemical fluxes in the ocean’s interior. Nature 332, 441–443. doi: 10.1038/332441a0
Church, M. J., Ducklow, H. W., and Karl, D. M. (2002). Multi-year increases in dissolved organic matter inventories at station ALOHA in the North Pacific Subtropical Gyre. Limnol. Oceanogr. 47, 1–10. doi: 10.4319/lo.2002.47.1.0001
Close, H. G. (2019). Compound-specific isotope geochemistry in the ocean. Annu. Rev. Mar. Sci. 11, 27–56. doi: 10.1146/annurev-marine-121916-063634
Copping, A. E., and Lorenzen, C. J. (1980). Carbon budget of marine phytoplankton-herbivore system with carbon-14 as tracer. Limnol. Oceanogr. 25, 873–882. doi: 10.4319/lo.1980.25.5.0873
de Goeij, J. M., van Oevelen, D., Vermeij, M. J. A., Osinga, R., Middelburg, J. J., de Goeij, A. F. M. P., et al. (2013). Surviving in a marine desert: The sponge loop retains resources within coral reefs. Science 342, 108–110. doi: 10.1126/science.1241981
de Wit, H. A., Valinia, S., Weyhenmeyer, G. A., Futter, M. N., Kortelainen, P., Austnes, K., et al. (2016). Current browning of surface waters will be further promoted by wetter climate. Environ. Sci. Technol. 3, 430–435. doi: 10.1021/acs.estlett.6b00396
Dijkstra, P., Dalder, J. J., Selmants, P. C., Hart, S. C., Koch, G. W., Schwartz, E., et al. (2011). Modeling soil metabolic processes using isotopologue pairs of position-specific 13C-labeled glucose and pyruvate. Soil Biol. Biochem. 43, 1848–1857. doi: 10.1016/j.soilbio.2011.05.001
Dippold, M. A., and Kuzyakov, Y. (2013). Biogeochemical transformations of amino acids in soil assessed by position-specific labeling. Plant Soil 373, 385–401. doi: 10.1007/s11104-013-1764-3
Doney, S. C., Fabry, V. J., Feely, R. A., and Kleypas, J. A. (2009). Ocean acidification: the other CO2 problem. Annu. Rev. Mar. Sci. 1, 169–192.
Dong, X., Bäcker, L. E., Rahmatullah, M., Schunk, D., Lens, G., and Meckenstock, R. U. (2019). Quantification of microbial degradation activities in biological activated carbon filters by reverse stable isotope labelling. AMB Express 9:109. doi: 10.1186/s13568-019-0827-0
Dong, X., Jochmann, M. A., Elsner, M., Meyer, A. H., Backer, L. E., Rahmatullah, M., et al. (2017). Monitoring microbial mineralization using reverse stable isotope labeling analysis by mid-infrared laser spectroscopy. Environ. Sci. Technol. 51, 11876–11883. doi: 10.1021/acs.est.7b02909
Druffel, E. R. M., Williams, P. M., Bauer, J. E., and Ertel, J. R. (1992). Cycling of dissolved and particulate organic matter in the open ocean. J. Geophys. Res. 97, 15639–15659.
Duarte, C. M. (2017). Reviews and syntheses: hidden forests, the role of vegetated coastal habitats in the ocean carbon budget. Biogeosciences 14, 301–310. doi: 10.5194/bg-14-301-2017
Ducklow, H. W., Hansell, D. A., and Morgan, J. A. (2007). Dissolved organic carbon and nitrogen in the Western Black Sea. Mar. Chem. 105, 140–150. doi: 10.1016/j.marchem.2007.01.015
Ducklow, H. W., Purdie, D. A., Williams, P. J. L., and Davies, J. M. (1986). Bacterioplankton: a sink for carbon in a coastal marine plankton community. Science 232, 865–867. doi: 10.1126/science.232.4752.865
Dunne, J. P., Sarmiento, J. L., and Gnanadesikan, A. (2007). A synthesis of global particle export from the surface ocean and cycling through the ocean interior and on the seafloor. Glob. Biogeochem. Cycles 21:GB4006. doi: 10.1029/2006GB002907
Egge, J., Thingstad, T., Larsen, A., Engel, A., Wohlers, J., Bellerby, R. G. J., et al. (2009). Primary production during nutrient-induced blooms at elevated CO2 concentrations. Biogeosciences 6, 877–885. doi: 10.5194/bg-6-877-2009
Endres, S., Galgani, L., Riebesell, U., Schulz, K. G., and Engel, A. (2014). Stimulated bacterial growth under elevated pCO2: results from an off-shore mesocosm study. PLoS One 9:e99228. doi: 10.1371/journal.pone.0099228
Endres, S., Unger, J., Wannicke, N., Nausch, M., Voss, M., and Engel, A. (2013). Response of Nodularia spumigena to pCO2 – Part 2: exudation and extracellular enzyme activities. Biogeosciences 10, 567–582. doi: 10.5194/bg-10-567-2013
Engel, A., Borchard, C., Piontek, J., Schulz, K. G., Riebesell, U., and Bellerby, R. (2013). CO2 increases 14C-primary production in an Arctic plankton community. Biogeosciences 10, 1291–1308. doi: 10.5194/bg-10-1291-2013
Engel, A., Delille, B., Jacquet, S., Riebesell, U., Rochelle-Newall, E., Terbrüggen, A., et al. (2004). Transparent exopolymer particles and dissolved organic carbon production by Emiliania huxleyi exposed to different CO2 concentrations: a mesocosm experiment. Aquat. Microb. Ecol. 34, 93–104. doi: 10.3354/ame034093
Engel, A., Goldthwait, S., Passow, U., and Alldredge, A. (2002). Temporal decoupling of carbon and nitrogen dynamics in a mesocosm diatom bloom. Limnol. Oceanogr. 47, 753–761. doi: 10.4319/lo.2002.47.3.0753
Engel, A., Händel, N., Wohlers, J., Lunau, M., Grossart, H. P., Sommer, U., et al. (2011). Effects of sea surface warming on the production and composition of dissolved organic matter during phytoplankton blooms: results from a mesocosm study. J. Plankton Res. 33, 357–372. doi: 10.1093/plankt/fbq122
Eppley, R. W., and Peterson, B. J. (1979). Particulate organic flux and planktonic new production in the deep ocean. Nature 282, 677–680. doi: 10.1038/282677a0
Erwin, K. L. (2009). Wetlands and global climate change: the role of wetland restoration in a changing world. Wetl. Ecol. Manag. 17:71. doi: 10.1007/s11273-008-9119-1
Evans, T., Coffinet, S., Könneke, M., Lipp, J. S., Becker, K. W., Elvert, M., et al. (2019). Assessing the carbon assimilation and production of benthic archaeal lipid biomarkers using lipid-RIP. Geochim. Cosmochim. Acta 265, 431–442. doi: 10.1016/j.gca.2019.08.030
Evans, T., Könneke, M., Lipp, J. S., Adhikari, R. R., Taubner, H., Elvert, M., et al. (2018). Lipid biosynthesis of Nitrosopumilus maritimus dissected by lipid specific radioisotope probing (lipid-RIP) under contrasting ammonium supply. Geochim. Cosmochim. Acta 242, 51–63. doi: 10.1016/j.gca.2018.09.001
Ferguson, R. L., and Sunda, W. G. (1984). Utilization of amino acids by planktonic marine bacteria: importance of clean technique and low substrate additions. Limnol. Oceanogr. 29, 258–274. doi: 10.4319/lo.1984.29.2.0258
Fichot, C. G., and Benner, R. (2014). The fate of terrigenous dissolved organic carbon in a river-influenced ocean margin. Glob. Biogeochem. Cycles 28, 300–318. doi: 10.1002/2013GB004670
Filella, M., and Rodríguez-Murillo, J. C. (2014). Long-term trends of organic carbon concentrations in freshwaters: strengths and weaknesses of existing evidence. Water 6, 1360–1418. doi: 10.3390/w6051360
Fischer, H., and Kuzyakov, Y. (2010). Sorption, microbial uptake and decomposition of acetate in soil: transformations revealed by position-specific 14C-labeling. Soil Biol. Biochem. 42, 186–192. doi: 10.1016/j.soilbio.2009.10.015
Flannigan, M. D., Krawchuk, M. A., de Groot, W. J., Wotton, B. M., and Gowman, L. M. (2009). Implications of changing climate for global wildland fire. Int. J. Wildland Fire 18, 483–507. doi: 10.1080/10962247.2015.1040526
Fogg, G. E. (1966). The extracellular products of algae. Oceanogr. Mar. Biol. Annu. Rev. 4, 195–212.
Follett, C. L., Repeta, D. J., Rothman, D. H., Xu, L., and Santinelli, C. (2014). Hidden cycle of dissolved organic carbon in the deep ocean. Proc. Nat. Acad. Sci. U.S.A. 111, 16706–16711. doi: 10.1073/pnas.1407445111
Fontaine, F. J., Wilcock, W. S. D., Foustoukos, D. E., and Butterfield, D. A. (2009). A Si-Cl geothermobarometer for the reaction zone of high-temperature, basaltic-hosted mid-ocean ridge hydrothermal systems. Geochem. Geophys. Geosys. 10:Q05009. doi: 10.1029/2009GC002407
Freeman, C., Evans, C. D., Monteith, D. T., Reynolds, B., and Fenner, N. (2001). Export of organic carbon from peat soils. Nature 412:785. doi: 10.1038/35090628
Fuchida, S., Mizuno, Y., Masuda, H., Toki, T., and Makita, H. (2014). Concentrations and distributions of amino acids in black and white smoker fluids at temperatures over 200°C. Org. Geochem. 66, 98–106. doi: 10.1016/j.orggeochem.2013.11.008
Fuhrman, J. A., Steele, J. A., Hewson, I., Schwalbach, M. S., Brown, M. V., Green, J. L., et al. (2008). A latitudinal diversity gradient in planktonic marine bacteria. Proc. Nat. Acad. Sci. U.S.A. 105, 7774–7778. doi: 10.1073/pnas.0803070105
Gilbert, D., Rabalais, N. N., Diaz, R. J., and Zhang, J. (2010). Evidence for greater oxygen decline rates in the coastal ocean than in the open ocean. Biogeosciences 7, 2283–2296. doi: 10.5194/bg-7-2283-2010
Gomez-Saez, G. V., Niggemann, J., Dittmar, T., Pohlabeln, A. M., Lang, S. Q., Noowong, A., et al. (2016). Molecular evidence for abiotic sulfurization of dissolved organic matter in marine shallow hydrothermal systems. Geochim. Cosmochim. Acta 190, 35–52. doi: 10.1016/j.gca.2016.06.027
Gomez-Saez, G. V., Pohlabeln, A., Stubbins, A., Marsay, C. M., and Dittmar, T. (2017). Photochemical alteration of dissolved organic sulfur from sulfidic porewater. Environ. Sci. Technol. 51, 14144–14154. doi: 10.1021/acs.est.7b03713
Goñi, M. A., and Gardner, L. R. (2003). Seasonal Dynamics in dissolved organic carbon concentrations in a coastal water-table aquifer at the forest-marsh interface. Aquat. Geochem. 9, 209–232. doi: 10.1023/b:aqua.0000022955.82700.ed
Gruber, D. F., Simjouw, J. P., Seitzinger, S. P., and Taghon, G. L. (2006). Dynamics and characterization of refractory dissolved organic matter produced by pure bacterial culture in an experimental predator-prey system. Appl. Environ. Microbiol. 72, 4184–4191. doi: 10.1128/aem.02882-05
Hansell, D. A. (2013). “Recalcitrant dissolved organic carbon fractions,” in Annual Review of Marine Science, Vol. 5, eds C. A. Carlson and S. J. Giovannoni (Palo Alto, CA: Annual Reviews), 421–445. doi: 10.1146/annurev-marine-120710-100757
Hansell, D. A., Carlson, C. A., Repeta, D. J., and Schlitzer, R. (2009). Dissolved organic matter in the ocean: a controversy stimulates new insights. Oceanography 22, 202–211. doi: 10.5670/oceanog.2009.109
Hartnett, H. E., Keil, R. G., Hedges, J. I., and Devol, A. H. (1998). Influence of oxygen exposure time on organic carbon preservation in continental margin sediments. Nature 391, 572–574.
Hawkes, J. A., Hansen, C. T., Goldhammer, T., Bach, W., and Dittmar, T. (2016). Molecular alteration of marine dissolved organic matter under experimental hydrothermal conditions. Geochim. Cosmochim. Acta 175, 68–85. doi: 10.1016/j.gca.2015.11.025
Hawkes, J. A., Rossel, P. E., Stubbins, A., Butterfield, D., Connelly, D. P., Achterberg, E. P., et al. (2015). Efficient removal of recalcitrant deep-ocean dissolved organic matter during hydrothermal circulation. Nat. Geosci. 8, 856–860. doi: 10.1038/ngeo2543
Hedges, J. I., Keil, R. G., and Benner, R. (1997). What happens to terrestrial organic matter in the ocean? Org. Geochem. 27, 195–212. doi: 10.1016/s0146-6380(97)00066-1
Heikes, B. G., Chang, W., Pilson, M. E. Q., Swift, E., Singh, H. B., Guenther, A., et al. (2002). Atmospheric methanol budget and ocean implication. Glob. Biogeochem. 16:1133. doi: 10.1021/es302082p
Hemingway, J. D., Spencer, R. G. M., Podgorski, D. C., Zito, P., Sen, I. S., and Galy, V. (2019). Glacier meltwater and monsoon precipitation drive Upper Ganges Basin dissolved organic matter composition. Geochim. Cosmochim. Acta 244, 216–228. doi: 10.1016/j.gca.2018.10.012
Henrichs, S. M. (1995). Sedimentary organic matter preservation: an assessment and speculative synthesis - a comment. Mar. Chem. 49, 127–136. doi: 10.1016/0304-4203(95)00012-g
Herndl, G. J., Reinthaler, T., Teira, E., van Aken, H. M., Veth, C., Pernthaler, A., et al. (2005). Contribution of Archaea to total prokaryotic production in the deep Atlantic Ocean. Appl. Environ. Microbiol. 71, 2303–2309. doi: 10.1128/aem.71.5.2303-2309.2005
Hodson, R. E., Maccubbin, A. E., and Pomeroy, L. R. (1981). Dissolved adenosine triphosphate utilization by free-living and attached bacterioplankton. Mar. Biol. 64, 43–51. doi: 10.1007/bf00394079
Hollibaugh, J. T., and Azam, F. (1983). Microbial degradation of dissolved proteins in seawater. Limnol. Oceanogr. 28, 1104–1116. doi: 10.4319/lo.1983.28.6.1104
Holmes, R. M., Coe, M. T., Fiske, G. J., Gurtovaya, T., McClelland, J. W., Shiklomanov, A. I., et al. (2012). “Climate change impacts on the hydrology and biogeochemistry of Arctic Rivers,” in Climatic Change and Global Warming of Inland Waters: Impacts and Mitigation for Ecosystems and Societies, eds C. R. Goldman, M. Kumagai, and R. D. Robarts (Hoboken, NJ: John Wiley & Sons, Ltd), 1–26. doi: 10.1002/9781118470596.ch1
Holmfeldt, K., Titelman, J., and Riemann, L. (2010). Virus production and lysate recycling in different sub-basins of the Northern Baltic Sea. Microb. Ecol. 60, 572–580. doi: 10.1007/s00248-010-9668-8
Hood, E., Battin, T. J., Fellman, J., O’Neel, S., and Spencer, R. G. M. (2015). Storage and release of organic carbon from glaciers and ice sheets. Nat. Geosci. 8, 91–96. doi: 10.1038/ngeo2331
Hood, E., Fellman, J., Spencer, R. G. M., Hernes, P. J., Edwards, R., D’Amore, D., et al. (2009). Glaciers as a source of ancient and labile organic matter to the marine environment. Nature 462, 1044–1047. doi: 10.1038/nature08580
Hopkinson, C. S., and Vallino, J. J. (2005). Efficient export of carbon to the deep ocean through dissolved organic matter. Nature 433, 142–145. doi: 10.1038/nature03191
Houghton, R. A. (2014). “The contemporary carbon cycle,” in Treatise on Geochemistry, 2nd Edn, eds H. Holland, and K. Turekian (Oxford: Elsevier), 399–435. doi: 10.1016/B978-0-08-095975-7.00810-X
Hugelius, G., Strauss, J., Zubrzycki, S., Harden, J. W., Schuur, E. A. G., Ping, C. L., et al. (2014). Estimated stocks of circumpolar permafrost carbon with quantified uncertainty ranges and identified data gaps. Biogeosciences 11, 6573–6593. doi: 10.5194/bg-11-6573-2014
Ishikawa, N. F., Butman, D., and Raymond, P. A. (2019). Radiocarbon age of different photoreactive fractions of freshwater dissolved organic matter. Org. Geochem. 135, 11–15. doi: 10.1016/j.orggeochem.2019.06.006
Jambeck, J. R., Geyer, R., Wilcox, C., Siegler, T. R., Perryman, M., Andrady, A., et al. (2015). Plastic waste inputs from land into the ocean. Science 347, 768–771. doi: 10.1126/science.1260352
Jannasch, H. W. (1967). Growth of marine bacteria at limiting concentrations of organic carbon in seawater. Limnol. Oceanogr. 12, 264–271. doi: 10.4319/lo.1967.12.2.0264
Jeffries, M., and Richter-Menge, J. (2012). The Arctic, in: State of the Climate in 2011, B. Am. Meteorol. Soc. 93, 127–147.
Jennerjahn, T. C., and Ittekkot, V. (2002). Relevance of mangroves for the production and deposition of organic matter along tropical continental margins. Naturwissenschaften 89, 23–30. doi: 10.1007/s00114-001-0283-x
Jessen, G. L., Lichtschlag, A., Ramette, A., Pantoja, S., Rossel, P. E., Schubert, C. J., et al. (2017). Hypoxia causes preservation of labile organic matter and changes seafloor microbial community composition (Black Sea). Sci. Adv. 3:e1601897. doi: 10.1126/sciadv.1601897
Jiao, N., Herndl, G. J., Hansell, D. A., Benner, R., Kattner, G., Wilhelm, S. W., et al. (2010). Microbial production of recalcitrant dissolved organic matter: long-term carbon storage in the global ocean. Nat. Rev. Microbiol. 8, 593–599. doi: 10.1038/nrmicro2386
Jones, D. O. B., Yool, A., Wei, C. L., Henson, S. A., Ruhl, H. A., Watson, R. A., et al. (2014). Global reductions in seafloor biomass in response to climate change. Glob. Change Biol. 20, 1861–1872. doi: 10.1111/gcb.12480
Jones, M. W., Santin, C., van der Werf, G. R., and Doerr, S. H. (2019). Global fire emissions buffered by the production of pyrogenic carbon. Nat. Geosci. 12, 742–747. doi: 10.1038/s41561-019-0403-x
Jørgensen, N. O. G., Kroer, N., Coffin, R. B., Yang, X.-H., and Lee, C. (1993). Dissolved free amino acids, combined amino acids, and DNA as sources of carbon and nitrogen to marine bacteria. Mar. Ecol. Prog. Ser. 98, 135–148. doi: 10.3354/meps098135
Jurado, E., Dachs, J., Duarte, C. M., and Simo, R. (2008). Atmospheric deposition of organic and black carbon to the global oceans. Atmos. Environ. 42, 7931–7939. doi: 10.1016/j.atmosenv.2008.07.029
Kaiser, K., and Benner, R. (2008). Major bacterial contribution to the ocean reservoir of detrital organic carbon and nitrogen. Limnol. Oceanogr. 53, 99–112. doi: 10.4319/lo.2008.53.1.0099
Kaiser, K., and Benner, R. (2012). Organic matter transformations in the upper mesopelagic zone of the North Pacific: Chemical composition and linkages to microbial community structure. J. Geophys. Res. 117:C01023. doi: 10.1029/2011JC007141
Kaiser, K., Benner, R., and Amon, R. M. W. (2017). The fate of terrigenous dissolved organic carbon on the Eurasian shelves and export to the North Atlantic. J. Geophys. Res. Oceans 122, 4–22. doi: 10.1002/2016jc012380
Kanakidou, M., Duce, R. A., Prospero, J. M., Baker, A. R., Benitez-Nelson, C., Dentener, F. J., et al. (2012). Atmospheric fluxes of organic N and P to the global ocean. Glob. Biogeochem. 26:GB3026.
Karl, D. M. (1999). A sea of change: biogeochemical variability in the North Pacific Subtropical Gyre. Ecosystems 2, 181–214. doi: 10.1007/s100219900068
Keene, W. C., Maring, H., Maben, J. R., Kieber, D. J., Pszenny, A. A. P., Dahl, E. E., et al. (2007). Chemical and physical characteristics of nascent aerosols produced by bursting bubbles at a model air-sea interface. J. Geophys. Res. 112:D21202. doi: 10.1029/2007JD008464
Keil, R. G., and Kirchman, D. L. (1999). Utilization of dissolved protein and amino acids in the Northern Sargasso Sea. Aquat. Microb. Ecol. 18, 293–300. doi: 10.3354/ame018293
Keil, R. G., Mayer, L. M., Quay, P. D., Richey, J. E., and Hedges, J. I. (1997). Loss of organic matter from riverine particles in deltas. Geochim. Cosmochim. Acta 61, 1507–1511. doi: 10.1016/s0016-7037(97)00044-6
Keil, R. G., Montluçon, D. B., Prahl, F. G., and Hedges, J. I. (1994). Sorptive preservation of labile organic matter in marine sediments. Nature 370, 549–552. doi: 10.1038/370549a0
Kellermann, M. Y., Wegener, G., Elvert, M., Yoshinaga, M. Y., Lin, Y. S., Holler, T., et al. (2012). Autotrophy as a predominant mode of carbon fixation in anaerobic methane-oxidizing microbial communities. Proc. Nat. Acad. Sci. U.S.A. 109, 19321–19326. doi: 10.1073/pnas.1208795109
Kellermann, M. Y., Yoshinaga, M. Y., Wegener, G., Krukenberg, V., and Hinrichs, K.-U. (2016). Tracing the production and fate of individual archaeal intact polar lipids using stable isotope probing. Org. Geochem. 95, 13–20. doi: 10.1016/j.orggeochem.2016.02.004
Kennedy, M. J., Pevear, D. R., and Hill, R. J. (2002). Mineral surface control of organic carbon in black shale. Science 295, 657–660. doi: 10.1126/science.1066611
Kieber, D. J., Keene, W. C., Frossard, A. A., Long, M. S., Maben, J. R., Russell, L. M., et al. (2016). Coupled ocean-atmosphere loss of marine refractory dissolved organic carbon. Geophys. Res. Lett. 43, 2765–2772. doi: 10.1002/2016gl068273
Kim, E.-H., Kim, G., Lee, S.-A., and Dittmar, T. (2015). Extraordinary slow degradation of dissolved organic carbon (DOC) in a cold marginal sea. Sci. Rep. 5:13808. doi: 10.1038/srep13808
Kim, J. M., Lee, K., Suh, Y. S., and Han, I. S. (2018). Phytoplankton do not produce carbon-rich organic matter in high CO2 oceans. Geophys. Res. Lett. 45, 4189–4197. doi: 10.1029/2017gl075865
Kim, T. W., Lee, K., Najjar, R. G., Jeong, H. D., and Jeong, H. J. (2011). Increasing N abundance in the Northwestern Pacific Ocean due to atmospheric nitrogen deposition. Science 334, 505–509. doi: 10.1126/science.1206583
Kiørboe, T. (2011). How zooplankton feed: mechanisms, traits and trade-offs. Biol. Rev. 86, 311–339. doi: 10.1111/j.1469-185X.2010.00148.x
Kirwan, M. L., Langley, J. A., Guntenspergen, G. R., and Megonigal, J. P. (2013). The impact of sea-level rise on organic matter decay rates in Chesapeake Bay brackish tidal marshes. Biogeosciences 10, 1869–1876. doi: 10.5194/bg-10-1869-2013
Klevenz, V., Sumoondur, A., Ostertag-Henning, C., and Koschinsky, A. (2010). Concentrations and distributions of dissolved amino acids in fluids from Mid-Atlantic ridge hydrothermal vents. Geochem. J. 44, 387–397. doi: 10.1111/gbi.12026
Krause-Jensen, D., and Duarte, C. M. (2016). Substantial role of macroalgae in marine carbon sequestration. Nat. Geosci. 9, 737–742. doi: 10.1038/NGEO2790
Krawchuk, M. A., Moritz, M. A., Parisien, M. A., Van Dorn, J., and Hayhoe, K. (2009). Global pyrogeography: the current and future distribution of wildfire. PLoS One 4:e5102. doi: 10.1371/journal.pone.0005102
Ksionzek, K. B., Lechtenfeld, O. J., McCallister, S. L., Schmitt-Kopplin, P., Geuer, J. K., Geibert, W., et al. (2016). Dissolved organic sulfur in the ocean: biogeochemistry of a petagram inventory. Science 354, 456–459. doi: 10.1126/science.aaf7796
Kuzyakov, Y. A., and Galitsa, S. V. (1993). Kinetics of [2-14C] glycine decomposition and its incorporation into humus fractions of sierozem. Eur. Soil Sci. 25, 39–53.
Lang, S. Q., Butterfield, D. A., Lilley, M. D., Paul Johnson, H., and Hedges, J. I. (2006). Dissolved organic carbon in ridge-axis and ridge-flank hydrothermal systems. Geochim. Cosmochim. Acta 70, 3830–3842. doi: 10.1016/j.gca.2006.04.031
Lang, S. Q., Butterfield, D. A., Schulte, M., Kelley, D. S., and Lilley, M. D. (2010). Elevated concentrations of formate, acetate and dissolved organic carbon found at the Lost City hydrothermal field. Geochim. Cosmochim. Acta 74, 941–952. doi: 10.1016/j.gca.2009.10.045
LaRowe, D. E., and Van Cappellen, P. (2011). Degradation of natural organic matter: a thermodynamic analysis. Geochim. Cosmochim. Acta 75, 2030–2042. doi: 10.1016/j.gca.2011.01.020
Legendre, L., Rivkin, R. B., Weinbauer, M. G., Guidi, L., and Uitz, J. (2015). The microbial carbon pump concept: potential biogeochemical significance in the globally changing ocean. Prog. Oceanogr. 134, 432–450. doi: 10.1016/j.pocean.2015.01.008
Leppard, G. G. (1995). The characterization of algal and microbial mucilages and their aggregates in aquatic ecosystems. Sci. Total Environ. 165, 103–131. doi: 10.1016/0048-9697(95)04546-d
Lin, H. T., Cowen, J. P., Olson, E. J., Amend, J. P., and Lilley, M. D. (2012). Inorganic chemistry, gas compositions and dissolved organic carbon in fluids from sedimented young basaltic crust on the Juan de Fuca Ridge flanks. Geochim. Cosmochim. Acta 85, 213–227. doi: 10.1016/j.gca.2012.02.017
Liss, P. S., Marandino, C. A., Dahl, E. E., Helmig, D., Hintsa, E. J., Hughes, C., et al. (2014). “Short-lived trace gases in the surface ocean and the atmosphere,” in Ocean-Atmosphere Interactions of Gases and Particles, eds P. Liss and M. T. Johnson (Berlin: Springer), 1–54. doi: 10.1007/978-3-642-25643-1_1
Liu, X., Li, Y., Wu, Y., Huang, B., Dai, M., Fu, F., et al. (2017). Effects of elevated CO2 on phytoplankton during a mesocosm experiments in the southern eutrophicated coastal waters of China. Sci. Rep. 7:6868.
Loginova, A. N., Thomsen, S., Dengler, M., Lüdke, J., and Engel, A. (2019). Diapycnal dissolved organic matter supply into the upper Peruvian oxycline. Biogeosciences 16, 2033–2047. doi: 10.5194/bg-16-2033-2019
Lønborg, C., Álvarez-Salgado, X. A., Letscher, R. T., and Hansell, D. A. (2018). Large stimulation of recalcitrant dissolved organic carbon degradation by increasing ocean temperatures. Front. Mar. Sci. 4:436. doi: 10.3389/fmars.2017.00436
Longnecker, K., Sievert, S. M., Sylva, S. P., Seewald, J. S., and Kujawinski, E. B. (2018). Dissolved organic carbon compounds in deep-sea hydrothermal vent fluids from the East Pacific Rise at 9°50’N. Org. Geochem. 125, 41–49. doi: 10.1016/j.orggeochem.2018.08.004
Lutz, M. J., Caldeira, K., Dunbar, R. B., and Behrenfeld, M. J. (2007). Seasonal rhythms of net primary production and particulate organic carbon flux to depth describe the efficiency of biological pump in the global ocean. J. Geophys. Res. 112:C10011. doi: 10.1029/2006JC003706
Macreadie, P. I., Anton, A., Raven, J. A., Beaumont, N., Connolly, R. M., Friess, D. A., et al. (2019). The future of Blue Carbon science. Nat. Commun. 10:3998. doi: 10.1038/s41467-019-11693-w
Mann, P. J., Eglinton, T. I., McIntyre, C. P., Zimov, N., Davydova, A., Vonk, J. E., et al. (2015). Utilization of ancient permafrost carbon in headwaters of Arctic fluvial networks. Nat. Commun. 6:7856. doi: 10.1038/ncomms8856
Marandino, C. A., de Bruyn, W. J., Miller, S. D., Prather, M. J., and Saltzman, E. S. (2005). Oceanic uptake and the global atmospheric acetone budget. Geophys. Res. Lett. 32:L15806.
Margolin, A. R., Gerringa, L. J. A., Hansell, D. A., and Rijkenberg, M. J. A. (2016). Net removal of dissolved organic carbon in the anoxic waters of the Black Sea. Mar. Chem. 183, 13–24. doi: 10.1016/j.marchem.2016.05.003
Margolin, A. R., Gonnelli, M., Hansell, D. A., and Santinelli, C. (2018). Black Sea dissolved organic matter dynamics: insights from optical analyses. Limnol. Oceanogr. 63, 1425–1443. doi: 10.1002/lno.10791
Marwick, T. R., Tamooh, F., Teodoru, C. R., Borges, A. V., Darchambeau, F., and Boullion, S. (2015). The age of river-transported carbon: a global perspective. Glob. Biogeochem. Cycles 29, 122–137. doi: 10.1002/2014GB004911
McDermott, J. M., Seewald, J. S., German, C. R., and Sylva, S. P. (2015). Pathways for abiotic organic synthesis at submarine hydrothermal fields. Proc. Nat. Acad. Sci. U.S.A. 112, 7668–7672. doi: 10.1073/pnas.1506295112
McDonough, L. K., Santos, I. R., Andersen, M. S., O’Carroll, D., Rutlidge, H., Meredith, K., et al. (2020). Changes in global groundwater organic carbon driven by climate change and urbanization. Nat. Commun. 11:1279. doi: 10.1038/s41467-020-14946-1
McNichol, A. P., and Aluwihare, L. I. (2007). The power of radiocarbon in biogeochemical studies of the marine carbon cycle: insights from studies of dissolved and particulate organic carbon (DOC and POC). Chem. Rev. 107, 443–466. doi: 10.1021/cr050374g
McNichol, A. P., Schneider, R. J., von Reden, K. F., Gagnon, A. R., Elder, K. L., Key, R. M., et al. (2000). Ten years after – The WOCE AMS radiocarbon program. Nucl. Instrum. Methods Phys. Res. Sect. B 172, 479–484. doi: 10.1016/S0168-583X(00)00093-8
McPhaden, M. J., and Zhang, D. (2002). Slowdown of the meridional overturning circulation in the upper Pacific Ocean. Nature 415, 603–608. doi: 10.1038/415603a
Mentges, A., Feenders, C., Deutsch, C., Blasius, B., and Dittmar, T. (2019). Long-term stability of marine dissolved organic carbon emerges from a neutral network of compounds and microbes. Sci. Rep. 9:17780. doi: 10.1038/s41598-019-54290-z
Middelboe, M., and Jorgensen, N. O. G. (2006). Viral lysis of bacteria: an important source of dissolved amino acids and cell wall compounds. J. Mar. Biol. Assoc. 86, 605–612. doi: 10.1017/s0025315406013518
Middelburg, J. J. (2018). Reviews and syntheses: to the bottom of carbon processing at the seafloor. Biogeosci. 15, 413–427. doi: 10.5194/bg-15-413-2018
Millet, D. B., Jacob, D. J., Custer, T. G., de Gouw, J. A., Goldstein, A. H., Karl, T., et al. (2008). New constraints on terrestrial and oceanic sources of atmospheric methanol. Atmos. Chem. Phys. 8, 6887–6905. doi: 10.5194/acp-8-6887-2008
Mitsch, W. J., and Hernandez, M. E. (2013). Landscape and climate change threats to wetlands of North and Central America. Aquat. Sci. 75, 133–149. doi: 10.1007/s00027-012-0262-7
Moller, E. F. (2005). Sloppy feeding in marine copepods: prey-size-dependent production of dissolved organic carbon. J. Plankton Res. 27, 27–35. doi: 10.1093/plankt/fbh147
Moller, E. F. (2007). Production of dissolved organic carbon by sloppy feeding in the copepods Acartia tonsa, Centropages typicus, and Temora longicornis. Limnol. Oceanogr. 52, 79–84. doi: 10.4319/lo.2007.52.1.0079
Mopper, K., and Kieber, D. J. (2002). “The photochemistry and cycling of carbon, sulfur, nitrogen and phosphorus,” in Biogeochemistry of Marine Dissolved Organic Matter, eds D. A. Hansell and C. A. Carlson (San Diego, CA: Academic Press), 455–507. doi: 10.1016/b978-012323841-2/50011-7
Mopper, K., Kieber, D. J., and Stubbins, A. (2014). “Marine photochemistry of organic matter: processes and impacts,” in Biogeochemistry of Marine Dissolved Organic Matter, eds D. A. Hansell and C. A. Carlson (San Diego, CA: Academic Press), doi: 10.1016/C2012-0-02714-7
Moran, M. A., Kujawinski, E. B., Stubbins, A., Fatland, R., Aluwihare, L. I., Buchan, A., et al. (2016). Deciphering ocean carbon in a changing world. Proc. Nat. Acad. Sci. U.S.A. 113, 3143–3151. doi: 10.1073/pnas.1514645113
Nagata, T., Fukuda, H., Fukuda, R., and Koike, I. (2000). Bacterioplankton distribution and production in deep Pacific waters: large-scale geographic variations and possible coupling with sinking particle fluxes. Limnol. Oceanogr. 45, 426–435. doi: 10.4319/lo.2000.45.2.0426
Novak, T., Godrijan, J., Pfannkuchen, D. M., Djakovac, T., Mlakar, M., Baricevic, A., et al. (2018). Enhanced dissolved lipid production as a response to the sea surface warming. J. Mar. Sys. 180, 289–298. doi: 10.1016/j.jmarsys.2018.01.006
O’Dowd, C. D., and de Leeuw, G. (2007). Marine aerosol production: a review of the current knowledge. Philos. Trans. R. Soc. Lond. Ser. A. 365, 1753–1774. doi: 10.1098/rsta.2007.2043
Ogawa, H., Amagai, Y., Kioke, I., Kaiser, K., and Benner, R. (2001). Production of refractory dissolved organic matter by bacteria. Science 292, 917–920. doi: 10.1126/science.1057627
Piontek, J., Lunau, M., Händel, N., Borchard, C., Wurst, M., and Engel, A. (2010). Acidification increases microbial polysaccharide degradation in the ocean. Biogeosciences 7, 1615–1624. doi: 10.5194/bg-7-1615-2010
Pohlabeln, A. M., and Dittmar, T. (2015). Novel insights into the molecular structure of non-volatile marine dissolved organic sulfur. Mar. Chem. 168, 86–94. doi: 10.1016/j.marchem.2014.10.018
Pohlabeln, A. M., Gomez-Saez, G. V., Noriega-Ortega, B. E., and Dittmar, T. (2017). Experimental evidence for abiotic sulfurization of marine dissolved organic matter. Front. Mar. Sci. 4:364. doi: 10.3389/fmars.2017.00364
Pohlman, J. W., Bauer, J. E., Waite, W. F., Osburn, C. L., and Chapman, N. R. (2010). Methane hydrate-bearing seeps as a source of aged dissolved organic carbon to the oceans. Nat. Geosci. 4, 37–41. doi: 10.1038/ngeo1016
Polovina, J. J., Howell, E. A., and Abecassis, M. (2008). Ocean’s least productive waters are expanding. Geophys. Res. Lett. 35:L03618.
Pomeroy, L. R., and Wiebe, W. J. (2001). Temperature and substrates as interactive limiting factors for marine heterotrophic bacteria. Aquat. Microb. Ecol. 23, 187–204. doi: 10.3354/ame023187
Priscu, J. C., and Christner, B. C. (2004). “Earth’s icy biosphere,” in Microbial Diversity and Bioprospecting, ed. A. T. Bull (Washington, DC: ASM Press), 130–145. doi: 10.1128/9781555817770.ch13
Quinn, P. K., Bates, T. S., Schulz, K. S., Coffman, D. J., Frossard, A. A., Russell, L. M., et al. (2014). Contribution of sea surface carbon pool to organic matter enrichment in sea spray aerosol. Nat. Geosci. 7, 228–232. doi: 10.1038/ngeo2092
Rabalais, N. N., Turner, R. E., Diaz, R. J., and Justic, D. (2009). Global change and eutrophication of coastal waters. ICES J. Mar. Sci. 66, 1528–1537. doi: 10.1093/icesjms/fsp047
Raymond, P. A., Saiers, J. E., and Sobczak, W. V. (2016). Hydrological and biogeochemical controls on watershed dissolved organic matter transport: pulse-shunt concept. Ecology 97, 5–16. doi: 10.1890/14-1684.1
Raymond, P. A., and Spencer, R. G. M. (2015). “Riverine DOM,” in Biogeochemistry of Marine Dissolved Organic Matter, 2nd Edn, eds D. A. Hansell and C. A. Carlson (Amsterdam: Elsevier Inc), 509–525.
Reeves, E. P., McDermott, J. M., and Seewald, J. S. (2014). The origin of methanethiol in midocean ridge hydrothermal fluids. Proc. Nat. Acad. Sci. U.S.A. 111, 5474–5479. doi: 10.1073/pnas.1400643111
Regier, P., Briceno, H., and Jaffe, R. (2016). Long-term environmental drivers of DOC fluxes: Linkages between management, hydrology and climate in a subtropical coastal estuary. Estuar. Coast. Shelf Sci. 182, 112–122. doi: 10.1016/j.ecss.2016.09.017
Ridgwell, A., and Arndt, S. (2015). “Why dissolved organics matter: DOC in ancient oceans and past climate change,” in Biogeochemistry of Marine Dissolved Organic Matter, eds D. A. Hansell and C. A. Carlson (San Diego, CA: Academic Press), 1–20. doi: 10.1016/b978-0-12-405940-5.00001-7
Roelofs, G. J. (2008). A GCM study of organic matter in marine aerosol and its potential contribution to cloud drop activation. Atmos. Chem. Phys. 8, 709–719. doi: 10.5194/acp-8-709-2008
Romera-Castillo, C., Letscher, R. T., and Hansell, D. A. (2016). New nutrients exert fundamental control on dissolved organic carbon accumulation in the surface Atlantic Ocean. Proc. Nat. Acad. Sci. U.S.A. 113, 10497–10502. doi: 10.1073/pnas.1605344113
Rossel, P. E., Bienhold, C., Boetius, A., and Dittmar, T. (2016). Dissolved organic matter in pore 980 water of Arctic Ocean sediments: environmental influence on molecular composition. Org. Geochem. 97, 41–52. doi: 10.1016/j.orggeochem.2016.04.003
Rossel, P. E., Stubbins, A., Hach, P. F., and Dittmar, T. (2015). Bioavailability and molecular composition of dissolved organic matter from a diffuse hydrothermal system. Mar. Chem. 177, 257–266. doi: 10.1016/j.marchem.2015.07.002
Rossel, P. E., Stubbins, A., Rebling, T., Koschinsky, A., Hawkes, J. A., and Dittmar, T. (2017). Thermally altered marine dissolved organic matter in hydrothermal fluids. Org. Geochem. 110, 73–86. doi: 10.1016/j.orggeochem.2017.05.003
Rothman, D. H., Hayes, J. M., and Summons, R. E. (2003). Dynamics of the Neoproterozoic carbon cycle. Proc. Nat. Acad. Sci. U.S.A. 100, 8124–8129. doi: 10.1073/pnas.0832439100
Ruiz-Fernández, A. C., Carnero-Bravo, V., Sanchez-Cabeza, J. A., Pérez-Bernal, L. H., Amaya-Monterrosa, O. A., Bojórquez-Sánchez, S., et al. (2018). Carbon burial and storage in tropical salt marshes under the influence of sea level rise. Sci. Tot. Environ. 630, 1628–1640. doi: 10.1016/j.scitotenv.2018.02.246
Saba, G. K., Steinberg, D. K., and Bronk, D. A. (2011). The relative importance of sloppy feeding, excretion and fecal pellet leaching in the release of dissolved organic carbon and nitrogen by Acartia tonsa copepods. J. Exp. Mar. Biol. Ecol. 404, 47–56. doi: 10.1016/j.jembe.2011.04.013
Sarmiento, J. L., and Gruber, N. (2006). Ocean Biogeochemical Dynamics. Princeton, NJ: Princeton University Press.
Schartau, M., Engel, A., Schröter, J., Thoms, S., Völker, C., and Wolf-Gladrow, D. (2007). Modelling carbon overconsumption and the formation of extracellular particulate organic carbon. Biogeosciences 4, 433–454. doi: 10.5194/bg-4-433-2007
Schmidt, F., Koch, B. P., Elvert, M., Schmidt, G., Witt, M., and Hinrichs, K. (2011). Diagenetic transformation of dissolved organic nitrogen compounds under contrasting sedimentary redox conditions in the Black Sea. Environ. Sci. Technol. 45, 5223–5229. doi: 10.1021/es2003414
Scott, A. C., and Glasspool, I. J. (2006). The diversification of Paleozoic fire systems and fluctuations in atmospheric oxygen concentration. Proc. Nat. Acad. Sci. U.S.A. 103, 10861–10865. doi: 10.1073/pnas.0604090103
Seidel, M., Beck, M., Riedel, T., Waska, H., Suryaputra, I. G. N. A., Schnetger, B., et al. (2014). Biogeochemistry of dissolved organic matter in an anoxic intertidal creek bank. Geochim. Cosmochim. Acta 140, 418–434. doi: 10.1016/j.gca.2014.05.038
Sexton, P. F., Norris, R. D., Wilson, P. A., Palike, H., Westerhold, T., Rohl, U., et al. (2011). Eocene global warming events driven by ventilation of oceanic dissolved organic carbon. Nature 471, 349–352. doi: 10.1038/nature09826
Sharma, P., Marinov, I., Cabre, A., Kostadinov, T., and Singh, A. (2019). Increasing biomass in the warm oceans: unexpected new insights from SeaWiFS. Geophys. Res. Lett. 46, 3900–3910. doi: 10.1029/2018gl079684
Sharples, J., Middelburg, J. J., Fennel, K., and Jickells, T. (2017). What proportion of riverine nutrients reaches the open ocean? Glob. Biogeochem. Cycles 31, 39–58. doi: 10.1002/2016gb005483
Shen, Y., and Benner, R. (2018). Mixing it up in the ocean carbon cycle and the removal of refractory dissolved organic carbon. Sci. Rep. 8:2542. doi: 10.1038/s41598-018-20857-5
Shim, C., Wang, Y., Singh, H. B., Blake, D. R., and Guenther, A. B. (2007). Source characteristics of oxygenated volatile organic compounds and hydrogen cyanide. J. Geophys. Res. 112:D10305.
Sjøgaard, K., Treusch, A. H., and Valdemarsen, T. B. (2017). Carbon degradation in agricultural soils flooded with seawater after managed coastal realignment. Biogeosciences 14, 4375–4389. doi: 10.5194/bg-14-4375-2017
Søndergaard, M., leB Williams, P. J., Cauwet, G., Riemann, B., Robinson, C., Terzic, S., et al. (2000). Net accumulation and flux of dissolved organic carbon and dissolved organic nitrogen in marine plankton communities. Limnol. Oceanogr. 45, 1097–1111. doi: 10.4319/lo.2000.45.5.1097
Spracklen, D. V., Arnold, S. R., Sciare, J., Carslaw, K. S., and Pio, C. (2008). Globally significant oceanic source of organic carbon aerosol. Geophys. Res. Lett. 35:L12811. doi: 10.1029/2008GL033359
Steinberg, D. K., Carlson, C. A., Bates, N. R., Goldthwait, S. A., Madin, L. P., and Michaels, A. F. (2000). Zooplankton vertical migration and the active transport of dissolved organic and inorganic carbon in the Sargasso Sea. Deep Sea Res. I 47, 137–158. doi: 10.1016/s0967-0637(99)00052-7
Steinberg, D. K., Goldthwait, S. A., and Hansell, D. A. (2002). Zooplankton vertical migration and the active transport of dissolved organic and inorganic nitrogen in the Sargasso Sea. Deep Sea Res. I 49, 1445–1461. doi: 10.1016/s0967-0637(02)00037-7
Steinberg, D. K., and Landry, M. R. (2017). Zooplankton and the ocean carbon cycle. Annu. Rev. Mar. Sci. 9, 413–444. doi: 10.1146/annurev-marine-010814-015924
St-Laurent, P., Friedrichs, M. A. M., Najjar, R. G., Martins, D. K., Herrmann, M., Miller, S. K., et al. (2017). Impacts of atmospheric nitrogen deposition on surface waters of the Western North Atlantic mitigated by multiple feedbacks. J. Geophys. Res. Oceans 122, 8406–8426. doi: 10.1002/2017JC013072
Stocker, T. F., Qin, D., Plattner, G.-K., Tignor, M., Allen, S. K., Boschung, J., et al. (2013). Climate Change 2013: The Physical Science Basis. Contribution of Working Group I to the Fifth Assessment Report of the Intergovernmental Panel on Climate Change. Cambridge: Cambridge University Press.
Stubbins, A., Niggemann, J., and Dittmar, T. (2012). Photo-lability of deep ocean dissolved black carbon. Biogeosciences 9, 1661–1670. doi: 10.5194/bg-9-1661-2012
Stubbins, A., Spencer, R. G. M., Chen, H., Hatcher, P. G., Mopper, K., Hernes, P. J., et al. (2010). Illuminated darkness: molecular signatures of Congo River dissolved organic matter and its photochemical alteration as revealed by ultrahigh precision mass spectrometry. Limnol. Oceanogr. 55, 1467–1477. doi: 10.4319/lo.2010.55.4.1467
Stubbins, A., Uher, G., Law, C. S., Mopper, K., Robinson, C., and Upstill-Goddard, R. C. (2006). Open-ocean carbon monoxide photoproduction. Deep Sea Res. II 53, 1695–1705. doi: 10.1021/es502057n
Sugie, K., Yoshimura, T., and Wakita, M. (2018). Impact of CO2 on the elemental composition of the particulate and dissolved organic matter of marine diatoms emerged after nitrate depletion. Limnol. Oceanogr. 63, 1924–1943. doi: 10.1002/lno.10816
Sunagawa, S., Coelho, L. P., Chaffron, S., Kultima, J. R., Labadie, K., Salazar, G., et al. (2015). Structure and function of the global ocean microbiome. Science 348:1261359. doi: 10.1126/science.1261359
Suttle, C. A. (2007). Marine viruses—major players in the global ecosystem. Nat. Rev. Microbiol. 5, 801–812. doi: 10.1038/nrmicro1750
Vaquer-Sunyer, R., Duarte, C. M., Santiago, R., Wassmann, P., and Reigstad, M. (2010). Experimental evaluation of planktonic respiration response to warming in the European Arctic Sector. Polar Biol. 33, 1661–1671. doi: 10.1007/s00300-010-0788-x
Veuger, B., Middelburg, J. J., Boschker, H. T. S., Nieuwenhuize, J., van Rijswijk, P., Rochelle-Newall, E. J., et al. (2004). Microbial uptake of dissolved organic and inorganic nitrogen in Randers Fjord. Estuar. Coast. Shelf Sci. 61, 507–515. doi: 10.1016/j.ecss.2004.06.014
Viviani, D. A., Böttjer, D., Letelier, R. M., and Church, M. J. (2018). The influence of abrupt increases in seawater pCO2 on plankton productivity in the subtropical North Pacific Ocean. PLoS One 13:e0193405. doi: 10.1371/journal.pone.0193405
Wagner, S., Brandes, J., Spencer, R. G. M., Ma, K., Rosengard, S. Z., Moura, J. M. S., et al. (2019). Isotopic composition of oceanic dissolved black carbon reveals non-riverine source. Nat. Commun. 10:5064. doi: 10.1038/s41467-019-13111-7
Walker, B. D., Druffel, E. R. M., Kolasinski, J., Roberts, B. J., Xu, X., and Rosenheim, B. E. (2017). Stable and radiocarbon isotopic composition of dissolved organic matter in the Gulf of Mexico. Geophys. Res. Lett. 44, 8424–8434. doi: 10.1002/2017gl074155
Walker, B. D., Primeau, F. W., Beaupré, S. R., Guilderson, T. P., Druffel, E. R. M., and McCarthy, M. D. (2016). Linked changes in marine dissolved organic carbon molecular size and radiocarbon age. Geophys. Res. Lett. 43, 10385–10393. doi: 10.1002/2016GL070359
Wang, R., Balkanski, Y., Bopp, L., Aumont, O., Boucher, O., Ciais, P., et al. (2015). Influence of anthropogenic aerosol deposition on the relationship between oceanic productivity and warming. Geophys. Res. Lett. 42, 10745–10754. doi: 10.1002/2015GL066753
Wang, X.-C., Chen, R. F., Whelan, J., and Eglinton, L. (2001). Contribution of ‘old’ carbon from natural marine hydrocarbon seeps to sedimentary and dissolved organic carbon pools in the Gulf of Mexico. Geophys. Res. Lett. 28, 3313–3316. doi: 10.1029/2001gl013430
Wegener, G., Bausch, M., Holler, T., Thang, N. M., Prieto-Mollar, X., Kellermann, M. Y., et al. (2012). Assessing sub-seafloor microbial activity by combined stable isotope probing with deuterated water and 13C-bicarbonate. Environ. Microbiol. 14, 1517–1527. doi: 10.1111/j.1462-2920.2012.02739.x
Wegener, G., Kellermann, M. Y., and Elvert, M. (2016). Tracking activity and function of microorganisms by stable isotope probing of membrane lipids. Curr. Opin. Biotechnol. 41, 43–52. doi: 10.1016/j.copbio.2016.04.022
Weinbauer, M. G., and Peduzzi, P. (1995). Effect of virus-rich high molecular weight concentrates of seawater on the dynamics of dissolved amino acids and carbohydrates. Mar. Ecol. Prog. Ser. 127, 245–253. doi: 10.3354/meps127245
Weiss, M. S., Abele, U., Weckesser, J., Welte, W., Schiltz, E., and Schulz, G. E. (1991). Molecular architecture and electrostatic properties of a bacterial porin. Science 254, 1627–1630. doi: 10.1126/science.1721242
Wild, B., Andersson, A., Broder, L., Vonk, J., Hugelius, G., McClelland, J. W., et al. (2019). Rivers across the Siberian Arctic unearth the patterns of carbon release from thawing permafrost. Proc. Nat. Acad. Sci. U.S.A. 116, 10281–10285. doi: 10.1073/pnas.1811797116
Wilhelm, S. W., and Suttle, C. A. (1999). Viruses and nutrient cycles in the sea - Viruses play critical roles in the structure and function of aquatic food webs. Bioscience 49, 781–788. doi: 10.2307/1313569
Williams, P. J. B. (1995). Evidence for the seasonal accumulation of carbon-rich dissolved organic material, its scale in comparison with changes in particulate material and the consequential effect on net C/N assimilation ratios. Mar. Chem. 51, 17–29. doi: 10.1016/0304-4203(95)00046-t
Williams, P. M., and Druffel, E. R. M. (1987). Radiocarbon in dissolved organic matter in the central North Pacific Ocean. Nature 330, 246–248. doi: 10.1038/330246a0
Winkel, M., Pjevac, P., Kleiner, M., Littman, S., Meyerdierks, A., Rudolf, A., et al. (2014). Identification and activity of acetate-assimilating bacteria in diffusive fluids venting from deep-sea hydrothermal systems. FEMS Microbiol. Ecol. 90, 731–746. doi: 10.1111/1574-6941.12429
Wohlers, J., Engel, A., Zöllner, E., Breithaupt, P., Jürgens, K., Hoppe, H. G., et al. (2009). Changes in biogenic carbon flow in response to sea surface warming. Proc. Nat. Acad. Sci. U.S.A. 106, 7067–7072. doi: 10.1073/pnas.0812743106
Wood, M. A., and Van Valen, L. M. (1990). Paradox lost? On the release of energy-rich compounds by phytoplankton. Mar. Microb. Food Webs 4, 103–116.
Worrall, F., Burt, T., and Admason, J. (2004). Can climate change explain increases in DOC flux from upland peat catchments? Sci. Total Environ. 326, 95–112. doi: 10.1016/j.scitotenv.2003.11.022
Yamanaka, T., Maeto, K., Akashi, H., Ishibashi, J. I., Miyoshi, Y., Okamura, K., et al. (2013). Shallow submarine hydrothermal activity with significant contribution of magmatic water producing talc chimneys in the Wakamiko Crater of Kagoshima Bay, southern Kyushu, Japan. J. Volcanol. Geotherm. Res. 258, 74–84. doi: 10.1016/j.jvolgeores.2013.04.007
Zark, M., Broda, N. K., Hornick, T., Grossart, H. P., Riebesell, U., and Dittmar, T. (2017). Ocean acidification experiments in large-scale mesocosms reveal similar dynamics of dissolved organic matter production and biotransformation. Front. Mar. Sci. 4:271. doi: 10.3389/fmars.2017.00271
Zark, M., Riebesell, U., and Dittmar, T. (2015). Effects of ocean acidification on marine dissolved organic matter are not detectable over the succession of phytoplankton blooms. Sci. Adv. 1:e1500531. doi: 10.1126/sciadv.1500531
Zhu, L., Zhao, S., Bittar, T. B., Stubbins, A., and Li, D. (2020). Photochemical dissolution of buoyant microplastics to dissolved organic carbon: rates and microbial impacts. J. Hazard. Mater. 383:121065. doi: 10.1016/j.jhazmat.2019.121065
Keywords: dissolved organic carbon, global carbon cycle, recalcitrance, isotopic probing, climate change
Citation: Wagner S, Schubotz F, Kaiser K, Hallmann C, Waska H, Rossel PE, Hansman R, Elvert M, Middelburg JJ, Engel A, Blattmann TM, Catalá TS, Lennartz ST, Gomez-Saez GV, Pantoja-Gutiérrez S, Bao R and Galy V (2020) Soothsaying DOM: A Current Perspective on the Future of Oceanic Dissolved Organic Carbon. Front. Mar. Sci. 7:341. doi: 10.3389/fmars.2020.00341
Received: 28 February 2020; Accepted: 23 April 2020;
Published: 25 May 2020.
Edited by:
Stuart Wakeham, University of Georgia, United StatesReviewed by:
Dennis Arthur Hansell, University of Miami, United StatesRonald Benner, University of South Carolina, United States
Copyright © 2020 Wagner, Schubotz, Kaiser, Hallmann, Waska, Rossel, Hansman, Elvert, Middelburg, Engel, Blattmann, Catalá, Lennartz, Gomez-Saez, Pantoja-Gutiérrez, Bao and Galy. This is an open-access article distributed under the terms of the Creative Commons Attribution License (CC BY). The use, distribution or reproduction in other forums is permitted, provided the original author(s) and the copyright owner(s) are credited and that the original publication in this journal is cited, in accordance with accepted academic practice. No use, distribution or reproduction is permitted which does not comply with these terms.
*Correspondence: Sasha Wagner, d2FnbmVzM0BycGkuZWR1
†Present address: Roberta Hansman, Woods Hole Oceanographic Institution, Woods Hole, MA, United States