- 1Section for Applied Marine Ecology and Modelling, Department of Bioscience, Aarhus University, Roskilde, Denmark
- 2Departamento de Biologia and CESAM, Universidade de Aveiro, Aveiro, Portugal
- 3Centre for Ocean and Atmospheric Sciences, School of Environmental Sciences, University of East Anglia, Norwich, United Kingdom
- 4CSIC, Instituto de Investigacións Mariñas, Vigo, Spain
The marine dissolved organic carbon (DOC) pool is an important player in the functioning of marine ecosystems. DOC is at the interface between the chemical and the biological worlds, it fuels marine food webs, and is a major component of the Earth’s carbon system. Here, we review the research showing impacts of global change stressors on the DOC cycling, specifically: ocean warming and stratification, acidification, deoxygenation, glacial and sea ice melting, changed inflow from rivers, changing ocean circulation and upwelling, and wet/dry deposition. A unified outcome of the future impacts of these stressors on the global ocean DOC production and degradation is not possible, due to regional differences and differences in stressors impacts, but general patterns for each stressor are presented.
Introduction
The ocean dissolved organic carbon (DOC) pool represents one of the largest reservoirs of organic matter on the Earth’s surface, containing a similar amount of carbon as atmospheric carbon dioxide (CO2; Hedges, 2002). Therefore, changes in DOC cycling due to global change, can result in alterations in the air-sea exchange of CO2, and have the potential to create important feedbacks onto global change itself. In the coming decades to centuries global change will impact the ocean biogeochemical cycles. Climate models have predicted global impacts on ocean biogeochemistry, but these are linked with large uncertainties and unknowns, depending on the model setups and constrained by the lack of data (Bopp et al., 2013; Burd et al., 2016). Furthermore, local, regional, and seasonal differences limit our ability to make general statements and predictions.
This manuscript will focus on global change impacts on DOC cycling in the future ocean on large scales. Specifically, we will describe impacts of global change stressors on DOC cycling in the future ocean. These stressors include: ocean warming and stratification, acidification, deoxygenation, glacial and sea ice derived material, changed inflow from rivers, ocean circulation and upwelling, and wet and dry deposition. These global change stressors are expected to have large impacts on ecosystem functioning and biogeochemistry and, consequently, on DOC cycling. While some of these stressors have been investigated in some detail (e.g., Zark et al., 2017; Lønborg et al., 2018), others have not, thus some of the suggested impacts mentioned in this manuscript will be theoretically based and will need further evaluation. Additionally, some potential global change factors such as cloud cover (Norris et al., 2016), have not been included, as there is currently no evidence of its impact on DOC cycling.
In the following sections we first describe the general properties and cycling of DOC in the oceans (see sections “Marine organic matter”, “Sources and sinks of DOC”) followed by discussions on the impacts of various aspects of global change (see sections “Ocean Warming”, “Ocean Acidification”, “Ocean Deoxygenation”, “Melting glaciers and sea ice”, “River derived material”, “Changes in ocean circulation and upwelling patterns” and “Wet and dry depositions”), and finish with concluding statements (see section “Conclusion”).
Marine Organic Matter
In nature organic compounds can be found in diverse chemical forms and molecular sizes spanning from the simplest organic molecule methane (CH4) to highly complex natural compounds and man-made organic pollutants (Dachs and Méjanelle, 2010). A simple definition of organic compounds is therefore difficult. In marine systems, organic matter (OM) is commonly defined as compounds acting as electron and proton donors and hence a source of metabolic energy, and sometimes nitrogen and phosphorus, for organism growth. But OM has other important roles including: (1) binding the majority of metals (e.g., iron) required for organism growth which is termed organic complexation (Morel and Price, 2003), (2) absorbing UV and visible light, thereby “protecting” marine microbes from harmful light and controlling primary production (West et al., 1999; Klug, 2002), and (3) reacting with free radicals which otherwise would be harmful to marine organisms due to their antioxidant properties (Romera-Castillo and Jaffé, 2015).
Typically, OM in marine systems is differentiated by its filtration behavior, with material retained on a filter (pore size between 0.2 and 0.7 μm) referred to as particulate organic matter (POM), whereas OM that passes the filter is termed dissolved organic matter (DOM). This distinction is operational (Verdugo, 2012), but has consequences for the biogeochemistry: POM can be suspended in the water column or sink to the sediments, while DOM is transported as a solute and generally remains in the water column. Therefore, the residence time of DOM in the water column is much longer than that of POM. The POM pool contains a minor part of living biomass (e.g., phytoplankton) and a major fraction of detritus (e.g., dead cells), while the DOM pool is largely lifeless, although it includes some prokaryotes and viruses (Hedges, 2002). The living parts of the POM pool have a fairly well-defined chemical composition mainly made of proteins (∼45%) complemented by lipids (∼17%), carbohydrates (∼25%), nucleic acids (∼12%), and pigments (∼2%; Anderson, 1995; Fraga et al., 1998). In contrast, the detrital POM and DOM pools have a very heterogeneous composition, but generally contain less proteins and nucleic acids and are supplemented with compounds from various sources, different degradation histories and reactivity (Middelburg, 2019).
On average, in marine systems the DOM concentrations are about an order of magnitude greater than that of POM in the euphotic zone of the ocean, while in sediments POM concentrations are commonly three orders of magnitude higher than those of DOM (Middelburg, 2019). The biological degradation pathways are also different. DOM is primarily degraded by microbes, while both microbes and larger organisms are able to degrade the POM pool.
The DOM molecules contain carbon (DOC), nitrogen (DON), phosphorus (DOP), sulfur (DOS), organically complexed trace-metals (e.g., iron), toxins and contaminants such as mercury (Hg), and most other biological relevant elements (Maranger and Pullin, 2003; Qualls and Richardson, 2003; Ravichandran, 2004). Most studies of DOM have focused on the DOC pool mainly because: (1) the analytical methods used to measure DOC are more advanced and fairly straight forward compared to the other pools, (2) the carbon fraction is the largest component of the DOM pool, and (3) from a global change perspective DOC is very important because the ocean DOC pool contains comparable amounts of carbon to atmospheric CO2 (Hedges, 2002). Therefore, small changes in DOC cycling can have large implications for atmospheric CO2 levels and influence the cooling and warming of the Earth’s surface over geological timescales (millions of years) (Peltier et al., 2007; Swanson-Hysell et al., 2010; Sexton et al., 2011). On shorter timescales (years) the amounts of DOC injected into the deep ocean [∼2000 million metric tons (Tg) yr–1; Hansell et al., 2009] are equal to the amounts of CO2 entering the ocean from the atmosphere each year; therefore changes in ocean DOC cycling could impact ocean and atmospheric CO2 levels (Gruber et al., 2019).
The DOC pool is often operationally divided into different fractions depending on its physical state; a part exists as a gaseous fraction in the water and is referred to as air-sea exchangeable DOC (EDOC), while the remaining fraction is divided in the low-molecular-weight (LMW < 1000 Da) and the high-molecular-weight (HMW > 1000 Da) components (Dachs et al., 2005; Benner and Amon, 2015) some of which may be colloidal rather than truly dissolved. Previous studies have suggested that in some areas (e.g., subtropical Atlantic ocean) EDOC may represent up to one-third of the DOC in surface waters (Dachs et al., 2005; Ruiz-Halpern et al., 2010). Generally HMW and LMW fractions constitute around 25 and 75%, respectively of DOC (Benner and Amon, 2015). From a biological point of view distinguishing between LMW and HMW is relevant as prokaryotes can usually only transport molecules in the LMW size range across the cell membrane (600–1000 Da; Weiss et al., 1991), while HMW-substrate requires enzymatic cleavage prior to uptake. However, some prokaryotes have been shown to use transporters to take up intact proteins and larger oligosaccharides (up to 69,000 Da) through the cell membrane without previous enzymatic cleavage (Arnosti and Repeta, 1994; Cuskin et al., 2015). The extent to which these mechanisms are widespread and important in the ocean remains to be determined.
Sources and Sinks of DOC
In marine systems DOC originates from either autochthonous or allochthonous sources (Figure 1). Autochthonous DOC is produced within the system, primarily by plankton organisms (Kawasaki and Benner, 2006; Lønborg et al., 2009) and in coastal waters additionally by benthic microalgae, benthic fluxes, and macrophytes (Wada et al., 2007), whereas allochthonous DOC is mainly of terrestrial origin (see sections “Melting glaciers and sea ice” and “River derived material”), supplemented by groundwater and atmospheric inputs (see section “Wet and dry depositions”) (Willey et al., 2000; Raymond and Spencer, 2015). In addition to soil derived humic material (see section “River derived material”), terrestrial DOC also includes material leached from plants exported during rain events, emissions of plant materials to the atmosphere and deposition in aquatic environments (e.g., volatile organic carbon and pollens), and also thousands of synthetic human-made organic chemicals that can be measured in the ocean at “trace” concentrations (Dachs and Méjanelle, 2010; Raymond and Spencer, 2015). Phytoplankton produces DOC by extracellular release commonly accounting between 5 and 30% of their total primary production (Karl et al., 1998), although this varies from species to species (Wetz and Wheeler, 2007). Nonetheless, this release of extracellular DOC is enhanced under high light and low nutrient levels, and thus should increase relatively from eutrophic to oligotrophic areas, probably as a mechanism for dissipating cellular energy (Thornton, 2014). Phytoplankton can also produce DOC by autolysis during physiological stress situations e.g., nutrient limitation (Boekell et al., 1992). Other studies have demonstrated DOC production in association with meso- and macro-zooplankton feeding on phytoplankton and bacteria (Hygum et al., 1997). Zooplankton-mediated release of DOC occurs through sloppy feeding, excretion and defecation which can be important energy sources for microbes (Lampert, 1978; Hygum et al., 1997). Such DOC production is largest during periods with high food concentration and dominance of large zooplankton species (Jumars et al., 1989). Bacteria are often viewed as the main consumers of DOC, but they can also produce DOC during cell division and viral lysis (Iturriaga and Zsolnay, 1981; Ogawa et al., 2001; Kawasaki and Benner, 2006). The biochemical components of bacteria are largely the same as other organisms, but some compounds from the cell wall are unique and are used to trace bacterial derived DOC (e.g., peptidoglycan). These compounds are widely distributed in the ocean, suggesting that bacterial DOC production could be important in marine systems (McCarthy et al., 1997). Viruses are the most abundant life forms in the oceans infecting all life forms including algae, bacteria and zooplankton (Suttle, 2005). After infection, the virus either enters a dormant (lysogenic) or productive (lytic) state (Weinbauer, 2004). The lytic cycle causes disruption of the cell(s) and release of DOC (Lønborg et al., 2013).
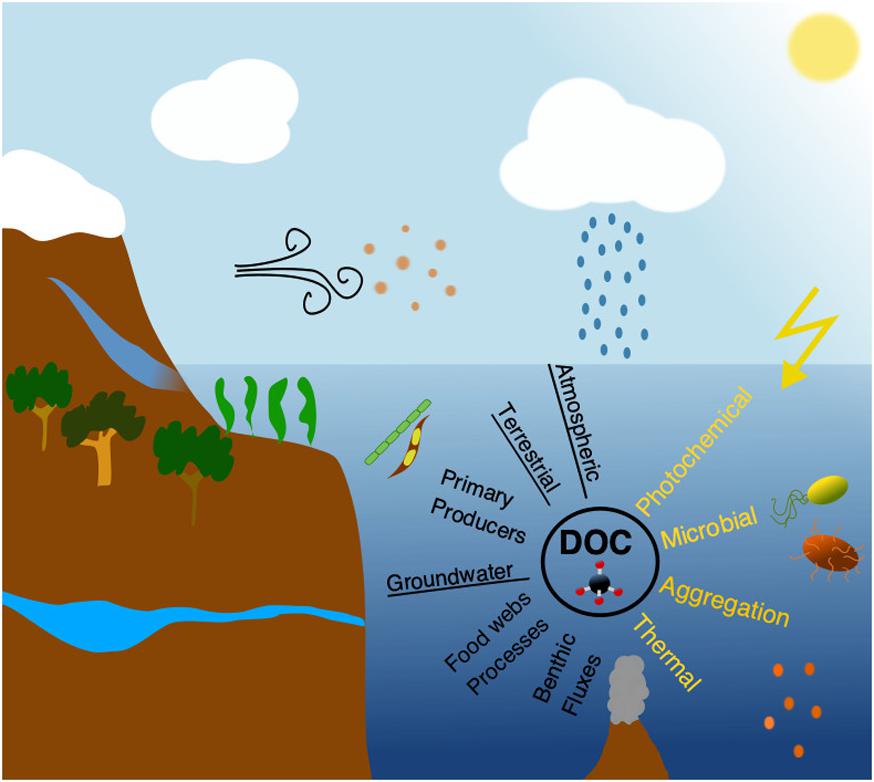
Figure 1. Simplified view of the main sources (black text; underlined are the allochthonous sources) and sinks (yellow text) of the oceanic dissolved organic carbon (DOC) pool. Most commonly referred sources of DOC are: atmospheric (e.g., rain and dust), terrestrial (e.g., rivers), primary producers (e.g., microalgae, cyanobacteria, macrophytes), groundwater, food chain processes (e.g., zooplankton grazing), and benthic fluxes (exchange of DOC across the sediment-water interface but also from hydrothermal vents). The four main processes removing DOC from the water column are: photodegradation (most notably UV-radiation; it should be noted that sometimes photodegradation “transforms” rather than “removes” DOC, ending up in higher molecular weight complex molecules), microbial (mainly by prokaryotes), aggregation (primarily when river and seawater mixes) and thermal degradation (in e.g., hydrothermal systems).
Marine macrophytes (i.e., macroalgae and seagrass) are highly productive and extend over large areas in coastal waters but their production of DOC has not received much attention. Macrophytes release DOC during growth with a conservative estimate (excluding release from decaying tissues) suggesting that macroalgae release between 1-39% of their gross primary production (Brilinsky, 1977; Pregnall, 1983), while seagrasses release less than 5% as DOC of their gross primary production (Penhale and Smith, 1977). The released DOC has been shown to be rich in carbohydrates, with rates depending on temperature and light availability (Barrón et al., 2014; Barrón and Duarte, 2015). Globally the macrophyte communities have been suggested to produce ∼160 Tg C yr–1 of DOC, which is approximately half the annual global river DOC input (250 Tg C yr–1; Barrón et al., 2014).
Marine sediments represent the main sites of OM degradation and burial in the ocean, hosting microbes in densities up to 1000 times higher than found in the water column (e.g., Hewson et al., 2001). The DOC concentrations in sediments are often an order of magnitude higher than in the overlying water column (Burdige and Gardner, 1998). This concentration difference results in a continued diffusive flux and suggests that sediments are a major DOC source releasing 350 Tg C yr–1, which is comparable to the input of DOC from rivers (Burdige and Komada, 2014). This estimate is based on calculated diffusive fluxes and does not include resuspension events which also releases DOC (Komada and Reimers, 2001) and therefore the estimate could be conservative. Also, some studies have shown that geothermal systems and petroleum seepage contribute with pre-aged DOC to the deep ocean basins (Dittmar and Koch, 2006; Dittmar and Paeng, 2009), but consistent global estimates of the overall input are currently lacking. Globally, groundwaters account for an unknown part of the freshwater DOC flux to the oceans (Burnett et al., 2006). The DOC in groundwater is a mixture of terrestrial, infiltrated marine, and in situ microbially produced material (Longnecker and Kujawinski, 2011). This flux of DOC to coastal waters could be important, as concentrations in groundwater are generally higher than in coastal seawater (Webb et al., 2019), but reliable global estimates are also currently lacking.
The main processes that remove DOC from the ocean water column (Figure 1) are: (1) Thermal degradation in e.g., submarine hydrothermal systems (Lang et al., 2006), (2) bubble coagulation and abiotic flocculation into microparticles (Kerner et al., 2003) or sorption to particles (Chin et al., 1998); (3) abiotic degradation via photochemical reactions (Moran and Zepp, 1997; Mopper et al., 2015); and (4) biotic degradation by marine heterotrophic prokaryotes (Lønborg and Álvarez-Salgado, 2012). It is suggested that the combined effects of photochemical and microbial degradation represent the major sinks of DOC (Carlson and Hansell, 2015). Thermal degradation of DOC has been found at high-temperature hydrothermal ridge-flanks, where outflow DOC concentrations are lower than in the inflow. While the global impact of these processes has not been investigated, current data suggest it is a minor DOC sink (e.g., Lang et al., 2006). Abiotic DOC flocculation is often observed during rapid (minutes) shifts in salinity when fresh and marine waters mix (Sholkovitz, 1976). Flocculation changes the DOC chemical composition, by removing humic compounds and reducing molecular size, transforming DOC to particulate organic flocs which can sediment and/or be consumed by grazers and filter feeders, but it also stimulates the bacterial degradation of the flocculated DOC (Tranvik and Sieburth, 1989). The impacts of flocculation on the removal of DOC from coastal waters are highly variable with some studies suggesting it can remove up to 30% of the DOC pool (Sholkovitz, 1976; Mulholland, 1981; Powell et al., 1996), while others find much lower values (3–6%; Sholkovitz et al., 1978). Such differences could be explained by seasonal and system differences in the DOC chemical composition, pH, metallic cation concentration, microbial reactivity, and ionic strength (Sholkovitz, 1976; Volk et al., 2000).
The colored fraction of DOC (CDOM) absorbs light in the blue and UV-light range and therefore influences plankton productivity both negatively by absorbing light, that otherwise would be available for photosynthesis, and positively by protecting plankton organisms from harmful UV-light (Williamson et al., 1996, 2015). However, as the impact of UV damage and ability to repair is extremely variable, there is no consensus on how UV-light changes might impact overall plankton communities (Jeffrey et al., 1996; Rhode et al., 2001). The CDOM absorption of light initiates a complex range of photochemical processes, which can impact nutrient, trace metal and DOC chemical composition, and promote DOC degradation (Mopper et al., 2015). Photodegradation involves the transformation of CDOM into smaller and less colored molecules (e.g., organic acids), or into inorganic carbon (CO, CO2), and nutrient salts (NH, HPO) (Miller and Zepp, 1995; Moran and Zepp, 1997; Moran et al., 2000). Therefore, it generally means that photodegradation transforms recalcitrant into labile DOC molecules that can be rapidly used by prokaryotes for biomass production and respiration. However, it can also increase CDOM through the transformation of compounds such as triglycerides, into more complex aromatic compounds (Kieber et al., 1997; Berto et al., 2016), which are less degradable by microbes. Moreover, UV radiation can produce e.g., reactive oxygen species, which are harmful to microbes (Hudson et al., 2003). The impact of photochemical processes on the DOC pool depends also on the chemical composition (Benner et al., 2004), with some studies suggesting that recently produced autochthonous DOC becomes less bioavailable while allochthonous DOC becomes more bioavailable to prokaryotes after sunlight exposure, albeit others have found the contrary (e.g., Obernosterer and Herndl, 1995; Benner and Ziegler, 1999; Sulzberger and Durisch-Kaiser, 2009). Photochemical reactions are particularly important in coastal waters which receive high loads of terrestrial derived CDOM, with an estimated ∼20–30% of terrestrial DOC being rapidly photodegraded and consumed (Miller and Moran, 1997). Global estimates also suggests that in marine systems photodegradation of DOC produces ∼180 Tg C yr–1 of inorganic carbon, with an additional 100 Tg C yr–1 of DOC made more available to microbial degradation (Moran and Zepp, 1997; Stubbins et al., 2006). Another attempt at global ocean estimates also suggest that photodegradation (210 Tg C yr–1) is approximately the same as the annual global input of riverine DOC (250 Tg C yr–1; Miller et al., 2002), while others suggest that direct photodegradation exceeds the riverine DOC inputs (Andrews and Zafiriou, 2000; Wang et al., 2004).
Due to the continuous production and degradation in natural systems the DOC pool contains a spectrum of reactive compounds, each with their own reactivity (e.g., Vahatalo et al., 2010) that have been divided into the following pools, from labile to recalcitrant, depending on the turnover times: (1) from hours to days (labile pool: DOCL < 200 Tg C), (2) weeks to months (semi-labile fraction: DOCSL; ∼600 Tg C), (3) over decades (semi-recalcitrant fraction: DOCSR; ∼1400 Tg C), (4) over thousands of years (recalcitrant fraction: DOCR; ∼63000 Tg C), and (5) a pool resistant to removal for tens of thousands of years (Hansell, 2013). This wide range in degradation times has been linked with the chemical composition, structure and molecular size (Amon and Benner, 1996; Benner and Amon, 2015), but degradation also depends on the environmental conditions (e.g., nutrients), prokaryote diversity, redox state, iron availability, mineral-particle associations, temperature, sun-light exposure, biological production of recalcitrant compounds, and the effect of priming or dilution of individual molecules (e.g., Amon and Benner, 1996; Thingstad et al., 1999; Del-Giorgio and Davies, 2003; Bianchi, 2011; Kattner et al., 2011; Keil and Mayer, 2014). For example, lignin can be degraded in aerobic soils but is relatively recalcitrant in anoxic marine sediments (Bianchi et al., 2018). This example shows bioavailability varies as a function of the ecosystem’s properties. Accordingly, even normally ancient and recalcitrant compounds (e.g., petroleum, carboxyl-rich alicyclic molecules) can be degraded in the appropriate environmental setting (e.g., Ward et al., 2013; Myers-Pigg et al., 2015).
Overall, the marine DOC pool is an important player in the functioning of marine ecosystems, because it is at the interface between the chemical and the biological worlds, it fuels marine food webs, and is a major component of the Earth’s carbon cycling. It is therefore important to understand how global changes will impact the ocean DOC cycling.
Global Change stressors
Ocean Warming
The best-known global change stressor is warming. The ocean absorbs around ∼90% of the heat generated by greenhouse gas emissions, predicted to increase the average sea surface temperature between 1 and 4°C over the next decades (Meehl et al., 2011). The degree of warming varies spatially with the Southern (south of 30°S) and Pacific oceans showing a higher warming than the Atlantic and Indian oceans (Cheng et al., 2019). The overall warming rates of coastal waters are also higher by up to an order of magnitude compared to the open ocean (Amos et al., 2003). However, warming also varies over time, which is partly linked with the rising heat accumulation in the deep ocean, resulting in around 30% of the current increase in ocean heat occurring at depths between 700 and 2000 m (Whitney et al., 2007; Meehl et al., 2011; Balmaseda et al., 2013).
In combination with a distinct long-term warming signal, the frequency and intensity of extreme warm sea surface temperatures (“marine heatwaves”) that persist for days to months and can extend over thousands of square kilometers, have increased, with prediction of higher frequency for the western tropical Pacific and Arctic ocean (Coumou and Rahmstorf, 2012). Marine heatwaves have been shown to cause loss of kelp forests, seagrass beds, coral reefs and increase the mortality of finfish, shellfish, seabirds, benthic invertebrates and mammals (Smale et al., 2019). While these extreme events will clearly impact the ecosystem structure and function, the underlying impacts on biogeochemical processes remains to be studied, but mass mortalities can be expected to lead to a short-term DOC release. Temperature is a major environmental driver controlling metabolic rates, and studies have shown that warming will lead to decreases in phytoplankton and prokaryote cell size (Morán et al., 2010; Morán et al., 2017), accelerated phytoplankton blooming (Sommer and Lengfellner, 2008), decreased total plankton biomass and changed community composition (Flombaum et al., 2013), and increase the release and change the composition of DOC produced by primary producers (Engel et al., 2011; Huete-Stauffer et al., 2017). Microbial respiration, growth, and reproductive rates also respond to temperature, with warming generally increasing the rates (Gillooly et al., 2001; Brown et al., 2004). These processes might change at the species level, although the overall community and rates might remain the same. Warming will only stimulate process rates up to the species optimum temperature and thereafter it can either have no or a negative impact. The overall response of marine ecosystems to warming is not straight forward but is expected to greatly affect the rates and mechanisms for both DOC production and degradation. As an example, it is predicted that a community of smaller-sized phytoplankton and prokaryotes will lead to more carbon regeneration within the surface layers and less efficient carbon sequestration to the deep ocean.
The impacts of temperature on biochemical reaction rates can be described by the Boltzmann factor or the Van’t Hoff-Arrhenius relationship, adapted from physical chemistry. This relationship argues that for a reaction to take place, reactants must first have enough energy, called the activation energy (E), to react (Arrhenius, 1889). This E value has been used to calculate the factor by which a rate increases with a 10°C increase (Q10).
The temperature sensitivity of microbial OM degradation has received attention in soils (Davidson et al., 2000; Davidson and Janssens, 2006), and suggests that warming will stimulate the microbial degradation of more recalcitrant OM fractions. This suggests that substrate bioavailability can be defined by changes in E, with more recalcitrant substrate having higher values and the microbial degradation process therefore having higher temperature sensitivity (Bosatta and Ågren, 1999; Sierra, 2011).
In marine systems, some studies have demonstrated that ocean DOC degradation follows a similar pattern to soils, with the degradation of more recalcitrant compounds having higher temperature sensitivity (Lønborg et al., 2016, 2018; Brewer and Peltzer, 2017). These findings have been used to predict that a 1°C ocean warming would increase the microbial degradation rates of the labile, semi-labile and semi-recalcitrant DOC pools by 4–6, 7–12, and 15–18%, respectively, resulting in an overall decrease of 700 ± 100 Tg C (∼15%) in the DOCSL + DOCSR pools (Lønborg et al., 2018). A subsequent study showed that warming increased the DOC degradation, but this enhanced microbial degradation of DOC, was mainly respired rather than used for biomass production (Lønborg et al., 2019).
In addition to the direct effects of temperature on microbial process, warming will also increase surface water stratification (Figure 2), particularly in subtropical gyres, which will also expand (Sarmiento et al., 2004; Polovina et al., 2008). This increased stratification prevents the exchange of dissolved materials across the pycnocline (Cermeño et al., 2008) and will intensify in the future (Burd et al., 2016). Increased stratification affects the cycle of recalcitrant DOC in surface ocean waters of temperate and subtropical areas in different ways. The first effect in permanently stratified waters is related to the compression of the surface mixed layer and the strengthening of the thermocline (Hutchins and Fu, 2017), which decreases the vertical exchange of dissolved materials or passive particles (e.g., phytoplankton). In seasonally stratified waters, the stratification period is followed by winter convective mixing. Enhanced water column heat content produces a decline of the winter mixed layer depth (Sarmiento et al., 2004), unless the frequency and strength of winter storms increases. Strengthening of permanent or seasonal stratification and shoaling of the winter mixed layer means a reduction of both the upward flux of inorganic nutrients and the downward flux of DOC. An overall decline of the nitrate flux implies a proportional reduction of global new primary production between 6 and 8% by 2090 (Codispoti et al., 1986; Sweeney et al., 2000; Yool et al., 2013) and, therefore, sinking of POM is predicted to decrease by around 4% by 2090 (Yool et al., 2017). Under these conditions, the amount and chemical composition of recalcitrant DOC changes. On the one hand, since the accumulation of recalcitrant DOC in the surface layer is controlled by the nitrate flux (Romera-Castillo et al., 2016), a reduction of the DOC pool is expected. However, the ratio of DOC accumulation to nitrate flux increases and, therefore, recalcitrant DOC represents a larger fraction of the new primary production (Thornton, 2014). On the other hand, increased stratification modifies the chemical composition of the accumulated DOC, which becomes carbon richer because of the extra exudation of polysaccharides by phytoplankton under nitrogen limitation (Thornton, 2014; Saad et al., 2016). Additionally, the lack of nutrients contributes to DOC accumulation in the surface mixed layer because of the “malfunctioning” of the microbial loop under these deprived conditions (Cotner et al., 1997; Thingstad et al., 1997). Apart from stratification, the temperature increase itself enhances the release of DOC by phytoplankton (Thornton, 2014) and favors the biodegradation of the recalcitrant DOC accumulated in the stratified surface waters during the winter mixing period, when nutrients are not limiting. Therefore, the primary effects of global warming on DOC in surface ocean waters, particularly in permanently stratified oligotrophic waters (Figure 2), are: (1) a reduction in DOC levels in response to the decline in the nitrate flux; (2) an increase of the C:N:P ratio; and (3) an increased importance of DOM to the biological carbon pump compared with sinking POM. Furthermore, both temperature and stratification contribute to boosting the microbial carbon pump, i.e., the microbial generation of recalcitrant DOC from labile OM (Jiao et al., 2010), as compared with the biological pump (Legendre et al., 2015). Conversely, in seasonally stratified areas where extreme winter storms have increased over the last decades, a deepening of the winter mixed layer and the corresponding increase of the nutrient flux occurs (Somavilla et al., 2017; Neale and Smyth, 2018) partially counteracting the effect of increased seasonal stratification.
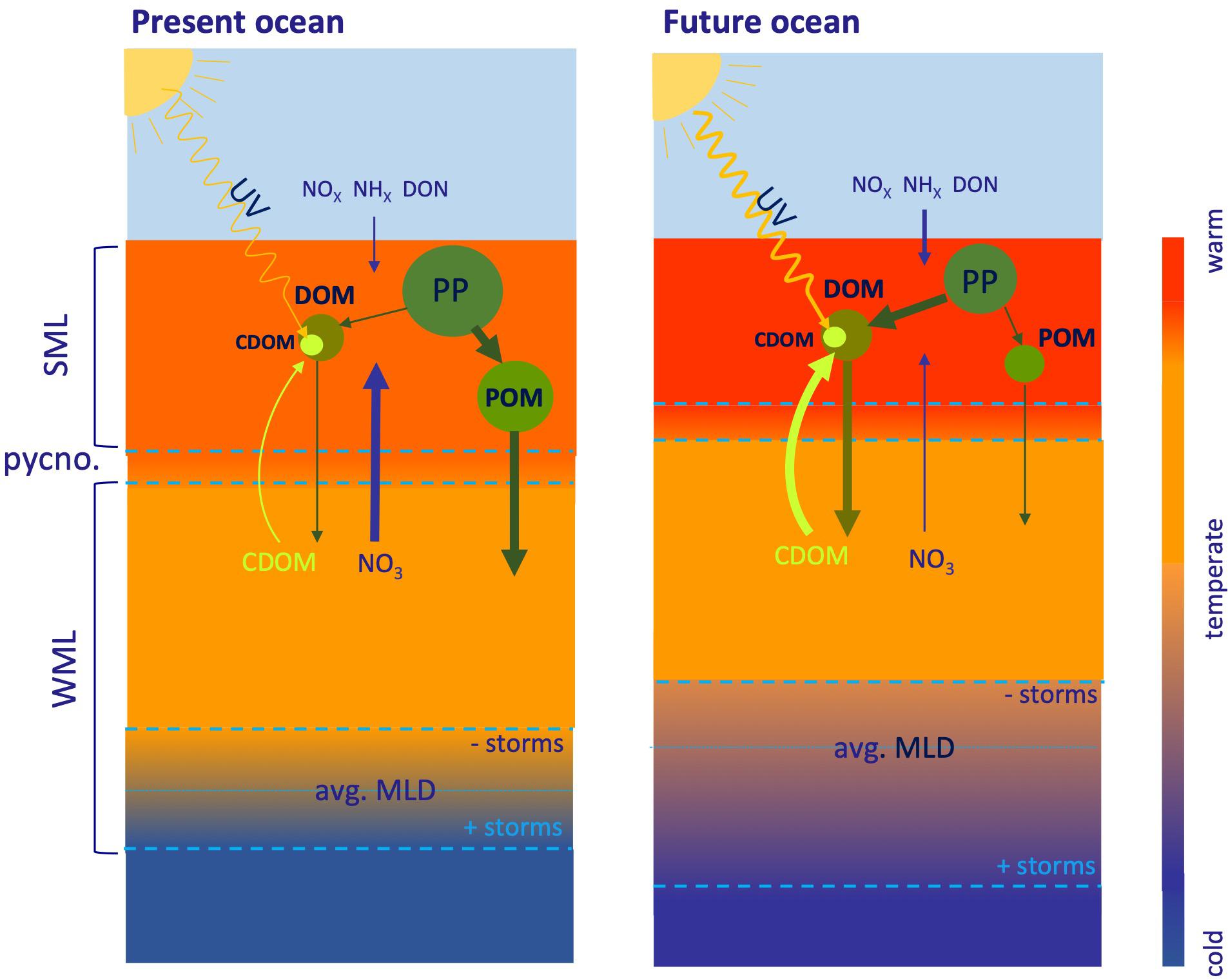
Figure 2. Illustration of dissolved organic matter (DOM) cycle in open Ocean stratified water. The stratified surface mixed layer (SML) will be shallower, warmer and more isolated from the subsurface waters (stronger pynocline) in the future ocean. The future decline of the upward flux of NO across the pycnocline could be partially compensated by the increased N atmospheric inputs in the future ocean. As a consequence of enhanced stratification, primary production (PP) will decline and the partition between DOM and particulate organic matter (POM) will be more favorable to the dissolved form compared with the present ocean. Particularly, the optically active fraction of DOM (CDOM) will play a more important role in the DOM cycling of the future ocean. In seasonally stratified open ocean waters, winter convective mixing occurs. The thickness of the winter mixed layer (WML) in the future ocean will be controlled by the relative importance of global warming and winter storm events. Whereas warming will tend to reduce the WML depth, winter storm events tend to increase it. The deeper the WML in the future ocean, the closer the DOM cycling will be to the present ocean conditions. Thickness of the arrows represents the relative importance of each process in the present and future ocean.
The second effect of global warming in the stratified surface ocean is related to the impact of the natural UV radiation on CDOM (see section “Sources and sinks of DOC”). Compression of the surface mixed layer and strengthening of the thermocline together with a present and future increase of the Earth’s surface UV radiation (Herman, 2010; Watanabe et al., 2011) has consequences for the CDOM pool dynamics (Figure 2). Increased stratification causes a decrease of the CDOM production because of the overall decline of new primary production (Nelson et al., 1998; Iuculano et al., 2019). CDOM in the surface mixed layer also decreases because increased stratification limits upward transport of CDOM across the seasonal thermocline. On the contrary, warming during winter mixing stimulate CDOM production by the microbial food web. The compression of the surface mixed layer in parallel with the increase of UV radiation intensifies the UV exposure of the CDOM accumulated in the surface ocean and, therefore, stimulates its photodegradation. Furthermore, photodegradation of CDOM is also stimulated by warming (Porcal et al., 2015). At this point the different dynamics of the recalcitrant DOC and CDOM pools should be noted: whereas DOC accumulates in the surface mixed layer and is transported downwards, CDOM is transported upwards and consumed in the surface layer. In summary, the effect of global warming on the CDOM pool in the ocean surface mixed layer is an overall reduction of the pool because of: (1) decreased net primary production and import from the ocean interior; and (2) the increased photodegradation of CDOM because of the compression of the surface mixed layer and the increase of UV radiation on the Earth’s surface.
Overall, the direct effects of ocean warming on microbial processes are expected to cause a faster turnover of DOC, reduce export to the deep ocean and consequently a net loss of carbon from the ocean DOC pool to the atmosphere. The warming related increase in stratification, will cause a reduction in DOC levels in response to the decline in the nitrate flux and increased photodegradation of CDOM. However detailed studies still need to address the direct and combined impacts of ocean warming and increased stratification on the cycling of DOC, in particular how this impacts the export to the deep ocean. Furthermore, as DOC exposure to sunlight is expected to increase with increased stratification, it is crucial to understand the impact of photo-chemical degradation on DOC concentration and composition.
Ocean Acidification
Current atmospheric CO2 levels are about 40% higher than before the industrial revolution (from 278 to 400 ppm), as a result of combustion of fossil fuels, cement production and land use changes (Gattuso et al., 2015). Around one third of the anthropogenic emitted CO2 has been dissolved in the ocean (Sabine et al., 2004; Gruber et al., 2019), lowering the pH (known as “ocean acidification”), currently by 0.1 units (from 8.2 to 8.1, on the total pH scale), below pre-industrial levels (RoyalSociety, 2005). If CO2 emissions continue to increase at similar rates, it is suggested that surface ocean pH will fall to 7.8 in 2100 (Gattuso et al., 2015). The magnitude of this decrease is difficult to predict precisely as it is expected that ocean warming will lower CO2 solubility, thus decreasing ocean uptake of anthropogenic CO2, while increased vertical stratification, will slow down physical transport of CO2 into the ocean interior (Doney, 2010). Nonetheless, surface CO2 has slowly spread downward into the deep ocean, with post-industrial CO2 already being detectable at 1000–3000 m depth (Gruber, 2011; Gruber et al., 2019).
Many experimental studies have investigated the impacts of ocean acidification, and these have mostly focused on single species experiments over short timescales (Alvarez-Fernandez et al., 2018). These studies have suggested positive, negative and neutral effects of ocean acidification on species physiology, demography and ecology (Harvey et al., 2013; Kroeker et al., 2013). Studies investigating impacts of decreasing pH on marine microbial communities show changes in phytoplankton and bacterial community composition, growth and cell size (Grossart et al., 2006; Piontek et al., 2010; Alvarez-Fernandez et al., 2018; James et al., 2019). However, the impacts on the plankton community are not uniform. For example, some phytoplankton groups, such as those producing calcium carbonate shells, could have lower growth rates, whereas phytoplankton primary production in general might not be impacted as they are not limited by current CO2 levels (Beardall and Raven, 2004). Furthermore, natural pH fluctuations in aquatic ecosystems, larger than the projected pH increases, already occur naturally (Joint et al., 2010). So overall as responses to increasing CO2 vary between communities and environments, general impact statements are difficult to make.
As anthropogenic CO2 enters the ocean mainly through its surface it is expected that acidification will primarily impact the DOC cycling in surface waters (Piontek et al., 2013). However, the observed impacts of ocean acidification on the DOC pool are variable. Some studies show increased carbon fixation by phytoplankton, with enhanced production and DOC accumulation (Engel et al., 2013), while others have found no effect but instead suggest enhanced production of transparent exopolymeric particles (TEP) (Engel et al., 2013; Zark et al., 2015). Another study where the composition of phytoplankton produced DOC was followed in mesocosm experiments exposed to a range of CO2 levels, showed that increases in DOC concentration and changes in the chemical composition were independent of CO2 (Zark et al., 2017). Such differences between studies might be explained by: (1) varying organic carbon partitioning between the particulate and dissolved phase which depends on community diversity and food web structure (Wetz and Wheeler, 2007), (2) quick microbial degradation of the additional DOC released, (3) loss of additional organic carbon as TEP to the sediment, and/or (4) different species and associated processes producing different responses, and degrees of resilience to changes in pH. However, generally in most environments, DOC concentrations seem to increase with elevated CO2 levels without clear impacts on the molecular composition (Zark et al., 2015; Bergen et al., 2016; Zark et al., 2017). An additional factor may be that the bioavailability and concentration of potentially limiting micronutrients (e.g., iron) have been shown to increase with ocean acidification (Gledhill et al., 2015), and this may result in a change in both primary production and species composition which in turn could affect DOC cycling.
Few studies have investigated the direct impact of pH on photochemical and microbial DOC degradation. One study has suggested that photochemical processes are pH dependent, with larger impacts at higher pH, suggesting that acidification could potentially reduce photochemical degradation (Timko et al., 2015). Earlier studies have clearly demonstrated impacts of decreased pH on enzymatic reactions (Arrhenius, 1889), but different extracellular enzymes have distinct acidification optima under natural conditions making it difficult to evaluate overall impacts (e.g., Grossart et al., 2006). Some studies showed enhanced rates of extracellular enzyme activity as a function of CO2 suggesting an increased DOC degradation (e.g., Teira et al., 2012; Piontek et al., 2013). Also, direct measurements of the microbial degradation of DOC showed increases with elevated CO2, with the additional degraded carbon mainly directed for respiration rather than biomass production (James et al., 2017). Such a stimulation in degradation could decrease the ocean DOC storage. Determining ocean wide impacts are difficult as both DOC production and degradation increase with acidification. Given that there is such a variable response per species (increase, decrease, no response) regarding DOC degradation and production, a first approach could include targeted studies on key species and individual source to understand acidification impacts on DOC cycling.
Ocean Deoxygenation
Oxygen (O2) is fundamental for most life on Earth and it functions as the main electron acceptor during DOC degradation in the ocean water column. Over the past 50 years oxygen minimum zones (OMZs) have expanded as a result of global warming directly (e.g., decreased oxygen solubility) and indirectly (e.g., increased respiration, decreased ocean ventilation), reducing the overall O2 content (this process is known as “ocean deoxygenation”; Keeling et al., 2010; Robinson, 2019). Approximately 15% of the global decline in open ocean O2 has been attributed to decreased solubility in response to ocean warming, with the remaining 85% due to intensified stratification (Helm et al., 2011). Furthermore, in coastal waters respiration of OM is the main contributor to deoxygenation (Diaz et al., 2019). Global models have suggested that overall ocean O2 levels will decline between 1.5 and 4% by 2090 (Ciais et al., 2013).
OMZs have lower O2 content relative to over- and under-lying waters, occur in depths between 100 and 1000 m, and are primarily found in open ocean waters adjacent to coastal upwelling regions. In these upwelling areas the productivity in surface waters is high, which together with the subsequent sinking OM, leads to elevated heterotrophic activity in deeper waters, thus lowering O2 levels (Bograd et al., 2008). Coastal waters can also contain low O2 and over the past centuries some studies have argued that there has been a ten-fold increase in the number of these low O2 regions. These have mainly been linked with eutrophication induced increases in OM production which lead to hypoxia or dead zones during degradation (Diaz et al., 2019).
The impacts of ocean deoxygenation are predicted to be minor in open ocean regions already experiencing low O2 levels (tropical and subtropical thermocline), while the largest change will likely happen in areas close to outcrops of the thermocline and deep ocean (Schmidtko et al., 2017). These effects will be amplified in coastal waters, because of a future global predicted doubling in both nutrient loadings compared to current levels and enhanced respiration rates (Foley et al., 2005; Foley, 2017). Coastal waters will therefore be more impacted by deoxygenation than open ocean systems (Gilbert et al., 2010). These changes in O2 concentration could lead to the disappearance of higher trophic organisms (e.g., fish) in some systems and change the food-web structure toward a microbial dominated system impacting the overall biogeochemical cycling (Gruber, 2011). When O2 levels drop from fully saturated (“oxic”) to low (“dysoxic” 20–90 μmol O2 l–1 and “suboxic” 1–20 μmol O2 l–1) and near zero (“anoxic” < 1 μmol O2 l–1) levels (Wright et al., 2012), the degradation of DOC shifts from an O2 dominated respiration, and towards other available electron acceptors, with the most dominant being nitrate (denitrification) and sulfate (sulfate reduction) (Canfield et al., 2005). Studies in marine sediments, where the use of these two alternative electron acceptors can dominate, have suggested that the degradation of OM under anoxic conditions is slower and less efficient (Jessen et al., 2017), which is consistent with observed higher OM concentrations in anoxic than in oxic pore water (e.g., Middelburg et al., 1993). Nonetheless, some studies have also suggested that under oxic and anoxic conditions, OM degradation is similar (e.g., Westrich and Berner, 1984; Lee, 1992). A slower and less efficient degradation of OM under anoxic conditions in sediments might also be explained by: (1) anaerobic prokaryotes normally degrading the recalcitrant OM that remains after aerobic degradation in surface sediments, (2) a lack of grazers which under oxic conditions would graze on prokaryotes releasing nutrients to aid the degradation process (Middelburg et al., 1993), (3) the free energy provided by the degradation of OM declines from aerobic to anaerobic respiration resulting in a less dense community and slower degradation, and (4) specific reactions are slower or not possible without O2 (Zehnder and Stumm, 1988; Fenchel and Finlay, 1995). Additionally the declining O2 levels will also lead to the biological removal of nitrate (Neubacher et al., 2011), and the release of phosphorus and iron from sediments (Scholz et al., 2014; Watson et al., 2018), potentially affecting primary production and DOC production.
Few studies have investigated how decreasing O2 impacts DOC degradation in the ocean water column. A study showed that the optical properties in a OMZ off the Peruvian coast were different and dominated by marine humic-like compounds in the anoxic compared with the oxic layers, suggesting differences in the chemical composition and a more recalcitrant nature of the DOC pool in the anoxic waters (Loginova et al., 2016). Another study showed that suboxic conditions do not reduce enzymatic degradation of OM and prokaryote production (Pantoja et al., 2009), whereas others show that the combined effects of eutrophication and low O2 on heterotrophic prokaryote activity favor DOC accumulation due to slower degradation (Maßmig et al., 2019a, b). However, these studies are performed using short-term experiments. Bastviken et al. (2004) followed the OM (e.g., fulvic acid) degradation under oxic and anoxic conditions in freshwater samples over an extended period (426 days) and found that most OM (68-78%) degraded faster under oxic conditions, although a small fraction (16–18%) degraded faster under anoxic conditions. The authors also suggest that over the short term (days) the degradation rates are similar in oxic and anoxic conditions.
Overall, decreasing ocean O2 levels, could lead to the disappearance of higher trophic organisms and change the food web structure. The prokaryote community structure would be expected to change and shift from an O2 dominated respiration, toward other available electron acceptors which would decrease the overall energy yield (Figure 3). Consequently, this change would impact the DOC chemical composition and is suggested to cause slower OM degradation and therefore DOC accumulation (Figure 3). Although previous studies have shown the role of O2 in controlling OM cycling, studies with a DOC focus are clearly lacking. As deoxygenation in coastal waters and the open ocean are controlled differently, studies should determine how this difference impacts DOC fluxes. Furthermore, it is essential to determine the impact of deoxygenation on the DOC degradation rates.
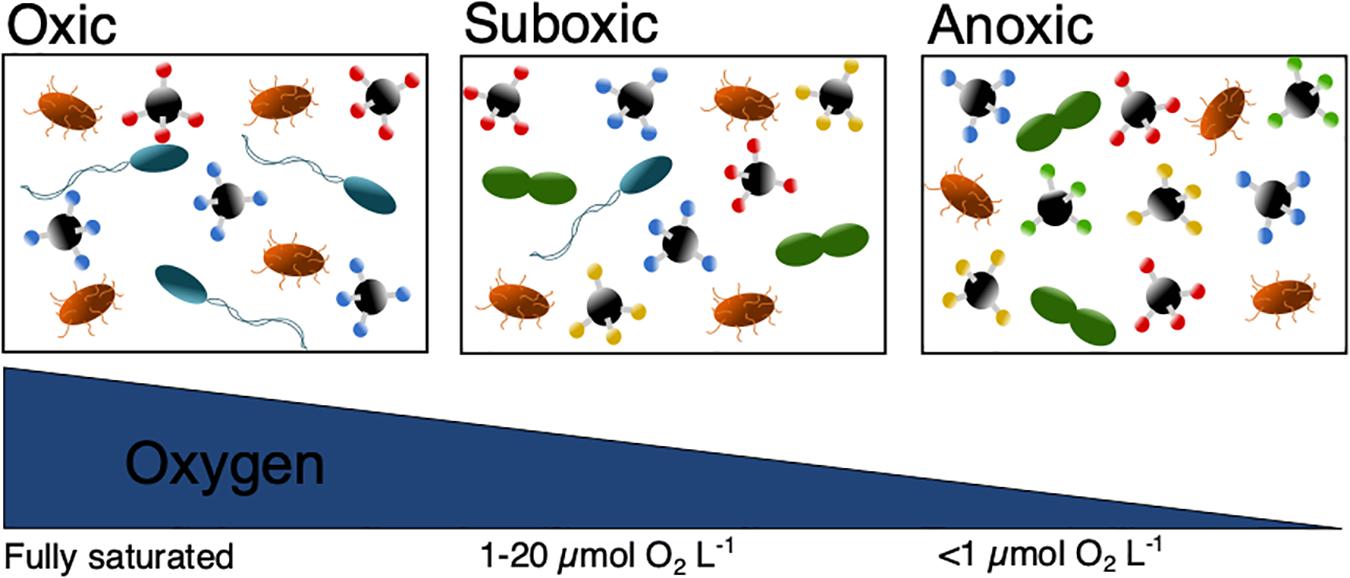
Figure 3. Scheme showing how decreasing ocean O2 levels could (1) slow down the prokaryote degradation and therefore lead to an accumulation of DOC (illustrated by increasing amounts of chemical compounds), (2) change its chemical composition (illustrated by changed color of chemical compounds), (3) change the prokaryote community composition (illustrated by changed prokaryote color and shape) and (4) decrease their energy yield (fewer prokaryotes). Please note that for convenience we have used the terms “oxic,” “suboxic,” and “anoxic” despite their definition not being fixed (Canfield and Thamdrup, 2009).
Melting Glaciers and Sea Ice
Glacial ecosystems (glaciers and ice sheets) contain 70% of the world’s freshwater and cover roughly 10% of the Earth’s surface (Hood et al., 2009; Boetius et al., 2015). Global Warming is expected to cause melting of mountain glaciers and ice caps with projections indicating an average total volume loss of 21% (some areas up to 75%) by 2100 (Radić and Hock, 2011). Also, under the RCP8.5 future emission scenario, it is very likely that we will see a seasonally ice-free Arctic before 2050 (Notz and Stroeve, 2016). Due to this melting the global mean sea-level rise is expected to be between 0.5 and 1.2 m higher by 2100, relative to pre-industrial levels (Church et al., 2013).
Glacial ecosystems were previously not thought to host an active microbial community, but with the discovery of high bacterial abundances (up to 108 cells ml–1; Boetius et al., 2015) that actively cycle carbon (Hood et al., 2009) this view is changing. Microbial colonization occurs initially through atmospheric deposition, but over time, the microbial communities becomes distinct, for example, bacterial cells tend to be larger (few μm in diameter) compared to those in the water column (Boetius et al., 2015). These communities are typically dominated by bacteria (including cyanobacteria), viruses, microalgae and fungi (Hodson et al., 2008; Boetius et al., 2015). Essentially two ecosystems exist: the supraglacial where inorganic carbon is fixed via photosynthesis, and the subglacial ecosystem where chemoautotrophy dominates. Hood et al. (2009) showed that the DOM of glacial origin is highly labile, and linked with glacial microbial production. Furthermore, two studies (Musilova et al., 2017; Smith et al., 2017) showed, in Antarctica and Greenland, a net production of highly labile DOC (from photosynthetic bacteria) that was exported during the melt season ultimately feeding the receiving coastal ecosystems (Lawson et al., 2014). Although DOC from glacial ecosystems is highly bioavailable, it is ancient (Hood et al., 2009). The source of this ancient carbon has partly been linked with aerosols derived from fossil fuel burning (Stubbins et al., 2012).
Cryoconite holes are particularly active habitats formed in the supraglacial ecosystems. Accumulation of dark-colored (inorganic and organic) particles, including microbes, in glacial ice in the ablation zone, causes the ice to melt, hence forming these water pools (Hodson et al., 2008; Boetius et al., 2015). Lawson et al. (2014) found DOC concentrations in cryoconite holes in excess of 554 μM C (average 285 μM C), with higher carbon fixation in the cryoconite sediment, compared to the overlying waters (3329 vs. 36 ng C l–1 d–1) (Foreman et al., 2007). As has been found in glacial waters, the OM in these holes have a microbial origin (Pautler et al., 2013). One estimate combining Svalbard, Greenland and European Alps, suggests they can fix up to 0.64 Tg C yr–1, indicating glaciers are autotrophic systems (Anesio et al., 2009).
Estimates of the DOC contribution from glacial runoffs to the world’s oceans are small (1 Tg DOC y–1; Raymond and Spencer, 2015) compared to e.g., rivers (250 Tg DOC yr–1; Raymond and Spencer, 2015). However the content is very labile, thus with a shorter turnover time. For example, runoff from the Gulf of Alaska glaciers show a DOC annual export of 0.13 Tg yr–1, of which about 0.10 Tg (77%) were degraded within days (Hood et al., 2009). Overall, by 2050 it is expected that 15 Tg of glacial DOC will be exported to coastal waters due to global change (Hood et al., 2015).
Glacial melting could have additional impacts. One report showed an increase in phytoplankton productivity as a result of inputs of iron (Fe) from melting glaciers in the Amundsen Sea (Alderkamp et al., 2012). Another study estimated a glacial runoff of bioavailable Fe of 0.40–2.54 Tg yr–1 and 0.06–0.17 Tg yr–1 in Greenland and Antarctica, respectively (Hawkings et al., 2014), which is expected to increase with melting glaciers. Global warming could, therefore, briefly increase the phytoplankton productivity, if Fe is limiting in the receiving coastal waters, and thus could result in a temporary negative feedback to anthropogenic CO2 emissions (Alderkamp et al., 2012).
With global warming, glacial DOC and Fe exports might increase temporarily, but will eventually vanish when no new glaciers are formed (Milner et al., 2017). Fellman et al. (2014) has suggested that, as glaciers retreat, forests and wetlands will replace these ecosystems shifting the DOC pool from a protein-like, labile compounds (microbially derived), to a plant-derived, humic-like and less labile compounds. Overall, this is expected to increase DOC concentrations, but decrease its lability (Fellman et al., 2014; Raymond and Spencer, 2015). This difference in the concentration and type of DOC could exert several changes in coastal waters. For example, if higher concentrations of humic-like compounds reach coastal waters it could: (1) protect the microbial food web from UV radiation, (2) reduce PAR radiation and thus primary production, and (3) provide labile DOC resulting from photodegradation.
Yearly, sea ice covers an area equivalent to approximately 5% of the total global ocean surface area (Arrigo, 2014). The phytoplankton community living in sea ice produce globally around 70 Tg C y–1 of organic carbon, contributing with 15 and 4% of the total primary production (seawater and ice) in Arctic and Antarctic waters, respectively. Around 11% (8 Tg) of this primary production is released as DOC, increasing the DOC concentrations up to 3 orders of magnitude compared with the surrounding seawater (Vancoppenolle et al., 2013). Furthermore, when the sea ice melts this material is released to the surrounding waters (Norman et al., 2011). The DOC in the Artic has a large river borne contribution, while in the Southern Ocean it is mainly autochthonous in origin (Retamal et al., 2007; Norman et al., 2011) and so the impacts of sea ice DOC may be different.
Global changes are already causing decreases in summer minimum sea-ice extent in the Arctic (up to 45%; Arrigo, 2014), while in the Antarctic impacts are more variable, with declines (up to 41%) in the Western and no change in the East Antarctic Peninsula over the last several decades (Ducklow et al., 2012; Stammerjohn and Maksym, 2017). In the Artic not only the extent of sea ice is changing, but it is also thinning (Lindsay and Schweiger, 2015), and the amount of multiyear ice that persist for at least one summer has significantly reduced (Kwok, 2018). The loss in sea-ice in the period between 1998 and 2012 in some Arctic areas, has been shown to increase primary productivity by up to 35%, which has been linked with higher light availability due to ice melt, while other areas have not shown any increase (Fernández-Méndez et al., 2015; Frey et al., 2017). Such regional differences could be linked with differences in the supply of nutrients by advection or upwelling (Aksenov et al., 2011; Kinney et al., 2014). Overall, while sea ice primary and DOC production will decline in the future, the retreat of sea ice could lead to higher DOC production with predicted increases in the microbial processing of this material.
In summary, increased glacial DOC could initially increase phytoplankton productivity in coastal waters, however, when most glaciers have melted it is expected an increase in terrestrial DOC. Additionally, the melting sea ice will lead to higher DOC production in receiving waters, and consequently increases in overall concentrations. It is thus critical to determine the impact of DOC released from melting glaciers and sea ice on the receiving ecosystems. Most importantly determining the response of the microbial communities to a possible change of DOC composition and its consequence to the food web structure, once the ice has melted requires further study.
River Derived Material
In the ocean, terrestrial derived DOC (allochthonous) constitutes around 10% of the total DOC pool (Benner, 2004). Rivers are the main source of this material and therefore are one of the largest contributors to the ocean’s DOC “mass balance,” delivering around 250 Tg DOC yr–1 (total DOC + POC inflow ∼350 Tg C yr–1; Raymond and Spencer, 2015). This DOC is exported together with nutrients (nitrogen: 37–66 Tg yr–1; phosphorus: 4–11 Tg yr–1; silicate; 340–380 Tg yr–1; Seitzinger et al., 2010; Beusen et al., 2016), fueling new DOC production, mainly in coastal waters.
DOC concentrations in rivers are mainly controlled by soil carbon stocks, mean catchment slope, wetland cover, land use, river floodplain extent and precipitation (Atkinhead-Peterson et al., 2003; Mulholland, 2003; Harrison et al., 2005). Elevated export of river DOC to the ocean is therefore generally observed during wet seasons, isolated rainfall, or storm events (e.g., Yoon and Raymond, 2012; Raymond et al., 2016).
Large river exports and their impacts are evident in the Arctic Ocean, receiving around 10% of the global riverine DOC, thus leading to the highest DOC concentrations and terrestrial DOC proportion of all ocean basins (Opsahl et al., 1999). This region is also particularly vulnerable to temperature rise which is occurring in the Arctic at a rate twice the global average (Blunden and Arndt, 2012). Most DOC in Artic rivers is derived from soils, and with extensive thawing of frozen soils and permafrost which contain a vast store of organic carbon (∼1.7 × 106 Tg organic carbon) there will be an increased mobilization and export of DOC to the ocean (Tarnocai et al., 2009; Hugelius et al., 2014).
Photochemical and microbial degradation are the main sinks for allochthonous DOC in the Arctic Ocean (e.g., Benner and Opsahl, 2001; Hernes and Benner, 2002), but parts of this DOC is relatively stable to photochemical degradation (Osburn et al., 2009). While early studies suggested that river derived DOC was largely “non-degradable,” it is now clear that terrestrial material is degraded in coastal waters (Coble, 2007), as it becomes more bioavailable in aquatic environments (Ward et al., 2013). The DOC exported by rivers contain labile components (Findlay and Sinsabaugh, 1999), but generally the DOC is more prone to photodegradation and is less bioavailable than phytoplankton exudates (Moran and Hodson, 1994; Mann and Wetzel, 1996). Whether terrestrial DOC is degraded in coastal waters or exported to the open ocean will depend on the reactivity and system flushing time (Lønborg and Álvarez-Salgado, 2012). For example, because Arctic and temperate systems are further away from the Equator (compared to tropical systems), they have a decreased Coriolis force. This results in longer flushing time on shelfs allowing longer time for the degradation to take place (Sharples et al., 2016).
Future projections on the fluxes of DOC and nutrients (including DON) from rivers to the ocean are unclear, some indicate increase, others decreases (Rabalais et al., 2009; Seitzinger et al., 2010; Yates et al., 2019). Increases have been linked with enhanced leaching and permafrost thawing, while decreases have been linked with declining extent of wetlands and lower river discharge (Harrison et al., 2005). Currently there are regional differences in export of DOC into the ocean and potential climate impacts. As an example, increases in precipitation over land north of 30°N since 1901 have been observed, while between 10°S and 30°N it has decreased since the 1970s (Dore, 2005). Such changes in precipitation and associated soil leaching could have increased DOC export in regions with increased precipitation, while decreasing exports in regions with droughts. Regional increases in freshwater DOC concentrations have been found in northern Europe and America undergoing a “browning” due to rising concentrations of allochthonous CDOM (Figure 4; Monteith et al., 2007; Solomon et al., 2015) and associated Fe (Björnerås et al., 2017). These increases have been linked with factors, such as: (1) increased precipitation leading to shorter residence and larger “flushing” of soil derived DOC into rivers and lakes (Roulet and Moore, 2006), (2) reduced atmospheric acid deposition which changes pH and increases soil DOC release (Evans et al., 2006), and (3) increasing vegetation cover (“greening”) of the northern hemisphere leading to higher terrestrial DOC production (Finstad et al., 2016). In the receiving systems, these DOC increases have been shown to: (1) impact primary production positively (supply nutrients; Tanentzap et al., 2014) and negatively (attenuate light), (2) enhance the importance of photochemical processes in the degradation of DOC, and (3) provide additional substrate, thus stimulating bacterial production (Hessen et al., 1990). Overall, such increased CDOM levels will decrease water clarity and benthic primary production, and due to increased substrate availability, switch from algal to bacterial dominance (Hessen, 1998).
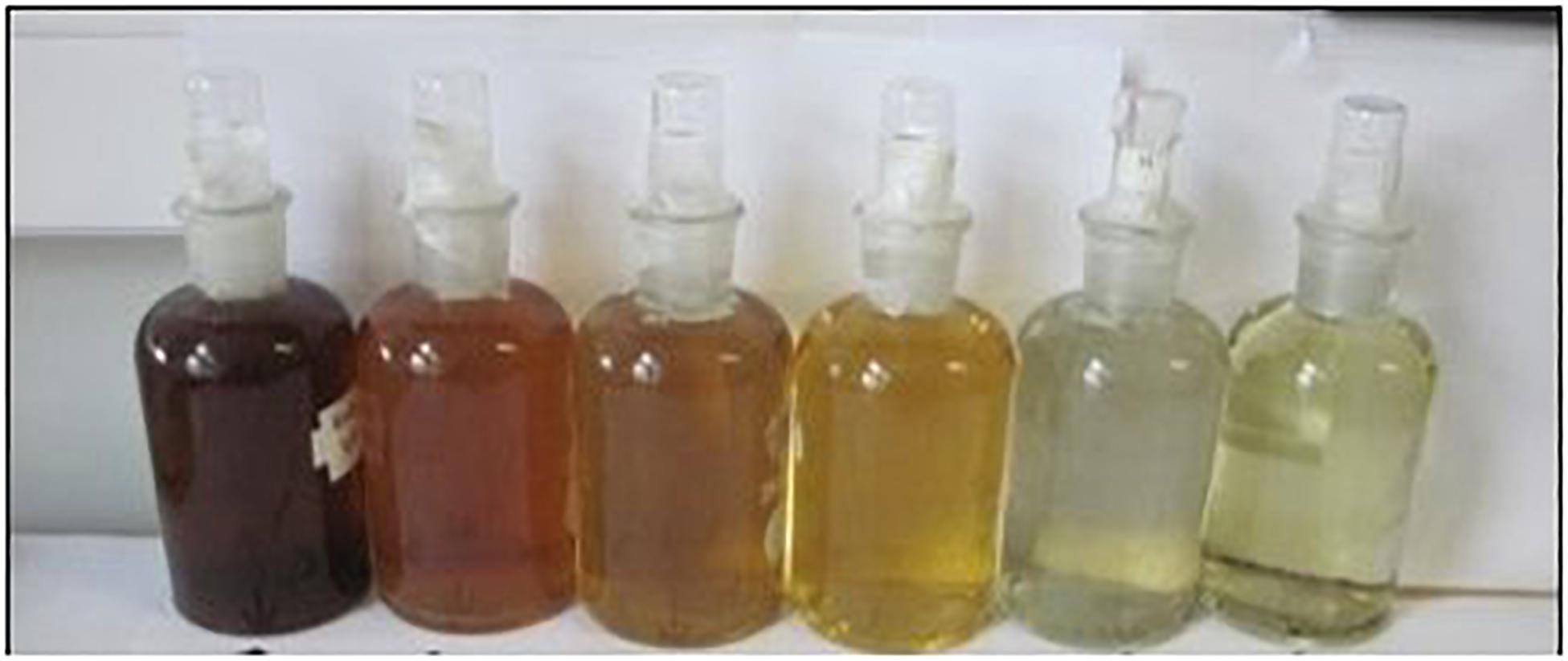
Figure 4. Photograph showing filtered (0.2 μm) coastal marine waters collected at various locations in the United Kingdom. The differences in color is due to the range of soil-derived carbon input to the coastal water, with dark brown (left) indicating a high soil-derived carbon contribution and near-clear water (right) indicating a low soil-derived carbon contribution (Credit: C. Lønborg).
Global change is predicted to increase the frequency, intensity and duration of storms, floods and droughts in different regions (Huntington, 2006; Knutson et al., 2010; Kyselı et al., 2012; Lehmann et al., 2015). Extreme events such as tropical cyclones are predicted to increase in the future with the linked precipitation increasing by 7% per °C warming (Prein et al., 2017). A framework termed the Pulse-Shunt concept suggests that OM is pulsed from catchments into streams during extreme hydrologic events (pulse), such as tropical cyclones, resulting in a rapid “flushing” of soil DOC to coastal waters (shunt; Raymond et al., 2016). One study demonstrated that within a few days after a tropical storm around 40% of the average annual DOC was exported (Yoon and Raymond, 2012; Bianchi et al., 2013), with the degradation of this DOC in the receiving coastal waters taking place over weeks to months after the event (Osburn et al., 2019). In addition, the source, chemical composition and reactivity of DOM have been shown to change in storm events (Eckard et al., 2017). The effects of storm events on DOC and nutrients export and impacts is probably more evident for small watershed but global estimates of the ocean wide impacts, are currently lacking.
Droughts have also been predicted to increase in some regions, such as the Mediterranean, regions in Africa (Sahel) and Asia (Mesopotamia and China’s loess), which together have been identified as drought and “land degradation” (Desertification) hotspots (Dregne, 1986). These changes are caused by longer periods of droughts, wildfires, higher temperatures, and changes in land cover, such as deforestation and grazing (D’Odorico et al., 2013; Prăvălie, 2016). Periods of drought may reduce land runoff, allowing terrestrial OM to accumulate. These periods can then often be followed by intense precipitation and flooding events (Paerl et al., 2019), boosting episodic DOC exports to coastal waters.
Another anthropogenic factor that will have major impacts on the flow of river derived material to the ocean is the construction of dams, which is expected to double over the next 15–20 years (Lehner et al., 2011; Zarfl et al., 2015). In the catchment areas these dams have been shown to: (1) increase flooding and degradation of biomass and soil OM, (2) increase water residence time, (3) improve underwater light conditions and hence increase primary production, (4) enhance nutrient retention and sediment trapping, and (5) promote the burial of OM (Maavara et al., 2017). Considering that dams represent a sink for riverine OM, largely caused by settling and burial of POM behind the dams, one study suggests that by the year 2000 dams had already decreased OM export to the global coastal waters by 13%, while the DOC leaving the dams will increase until 2030 and then decrease slightly until 2050 (Maavara et al., 2017). With an expected increase in the number of dams, riverine DOC export could increase in the near future, especially in major dam construction areas of Asia, South America, and Africa.
Predicting global trends in river DOC delivery is challenging due to large regional variations in how global stressors will impact the export of DOC to the ocean. Regionally, in the Arctic, northern Europe and America increasing DOC rivers deliveries are expected. These increases are predicted to lead to larger importance of photo-chemical processes in the degradation of DOC in receiving coastal waters. Extreme events, such as storms and droughts, will cause regional large episodic exports of DOC to coastal waters. The construction of large dams in Asia, South America, and Africa could lead to regional increases in DOC delivery. Despite decades of research, we still need to understand how the interaction between catchment properties and weather events (e.g., storms) impacts the DOC composition and cycling along the river-ocean continuum. In such studies it will be essential to determine regional differences.
Changes in Ocean Circulation and Upwelling Patterns
The recalcitrant fractions of DOC produced in the surface ocean are prone to accumulate in the stratified surface layer of oligotrophic gyres (Hansell et al., 2009; Romera-Castillo et al., 2016) or to be transported horizontally by surface ocean currents (Letscher et al., 2013; Wu et al., 2015). Horizontal export of recalcitrant DOC also occurs in equatorial (Archer et al., 1997; Hansell et al., 1997) and coastal upwelling areas (Alvarez-Salgado et al., 2007; Lovecchio et al., 2018), eventually accumulating in the adjacent oligotrophic ocean. Deep convection at high latitudes injects DOC into the deep ocean (Hansell et al., 2002, 2009; Fernández-Castro et al., 2019). Once in the deep ocean, the less recalcitrant fractions of DOC are typically removed with increasing water mass age over timescales of decades and longer (Carlson et al., 2010; Hansell et al., 2012; Fontela et al., 2016).
Global warming is already affecting major ocean currents such as the Gulf Stream and the associated Atlantic Meridional Overturning Circulation (AMOC), which has apparently weakened over the last decades (Caesar et al., 2018). Future climate scenarios indicate that this weakening will continue during the 21st century (Chen et al., 2013). Global warming has also affected the wind regime of coastal upwelling areas (Sydeman et al., 2014; Bakun et al., 2015; Wang et al., 2015). Forecasting of future climate scenarios indicates that summertime winds at higher latitudes (about 35°–45° N or S) will intensify, while at lower latitudes (about 10°–20°N or S) they will weaken (Rykaczewski et al., 2015). Strong reduction of the equatorial upwelling in the Eastern Pacific, and moderate reduction in the Atlantic, is expected by the end of this century because of weakening of trade winds (Terada et al., 2020). These changes, together with increasing stratification (Rykaczewski and Dunne, 2010; Sousa et al., 2020; Terada et al., 2020), also have implications for the production, vertical advection and horizontal export of dissolved materials in these highly productive areas.
The present and future weakening of the AMOC (Chen et al., 2013; Caesar et al., 2018) causes a reduction of the amount of recalcitrant DOC that is injected in the deep waters of the North Atlantic. This recalcitrant DOC is partly produced in the Subpolar North Atlantic and partly transported northward from subtropical latitudes by the North Atlantic Current (Fernández-Castro et al., 2019). This DOC is transported downwards by the overturning circulation during North Atlantic Deep Water formation (Carlson et al., 2010; Fontela et al., 2016). The suggested decrease of the AMOC by 15% since the 1950’s, would produce at least a proportional decrease of the amount of DOC injected in the deep ocean. For the worst climate scenario (RCP8.5) a further reduction between 15% and 60% is expected by the end of this century (Caesar et al., 2018). Furthermore, It has been observed in coastal (e.g., Lønborg and Álvarez-Salgado, 2012) and open ocean waters (e.g., Fernández-Castro et al., 2019) a close inverse relationship between water residence time and quantity and quality of the DOM. Therefore, we hypothesize that the longer water residence time in the Subpolar North Atlantic would reduce the amount and increase the C:N ratio of the recalcitrant DOM accumulated in the surface layer and, further reduce the downward flux of DOC in the area. This means that: (1) the contribution of DOC to the biological pump would reduce; and (2) the microbial populations of the deep North Atlantic would receive a lower amount of a poorer quality substrate from the Subpolar North Atlantic.
Future projections of the Southern Ocean circulation also predict a weakening of the subduction rates of the Subantarctic Mode Water by 8% and the Antarctic Intermediate Water by 23% at the end of the 21st century in response to buoyancy gain and shoaling of the winter mixed layer (Downes et al., 2010). Furthermore, the formation of Antarctic Bottom Water and its northward sinking toward the global abyss is also expected to weaken in response to temperature-driven decreases in density (Lago and England, 2019).
Generally, upwelling favorable winds have intensified in the major coastal upwelling systems of the world ocean in response to global warming (Figure 5; Sydeman et al., 2014; García-Reyes et al., 2015; Wang et al., 2015). Forecasting results indicate that this increase will continue through this century at the high latitudinal range of the major coastal upwelling systems (Rykaczewski et al., 2015). Conversely, winds will weaken at the low latitudinal range. Intensification of coastal winds is not the only effect in coastal upwelling systems: global warming will also increase the stratification (see section “Ocean Warming”; Rykaczewski and Dunne, 2010; Varela et al., 2018; Sousa et al., 2020). Therefore, two opposite forces must be considered. Strengthening of coastal upwelling intensifies the vertical nitrate flux to the surface mixed layer, proportionally increasing new production, the accumulation of recalcitrant DOM with relatively lower C:N ratio, and its horizontal export to the adjacent open ocean (Figure 5). The increased vertical nitrate flux is not only due to the larger volume of upwelled water but also to the higher concentration of nitrate. Source waters in upwelling systems have experienced pH (see section “Ocean Acidification”) and O2 (see section “Ocean Deoxygenation”) decrease and nitrate gain over the last decades (Bograd et al., 2008, 2015), that it is also predicted to occur in the future (Bakun et al., 2015). Further pH and O2 decline and nitrate gain occurs in bottom shelf waters because of local OM mineralization, making surface waters nitrate-richer, but O2 poorer and more acidic. Whereas nitrate has a direct impact on DOM cycling (Figure 5), it is not expected that the low O2 have a distinct influence. Fast exchange with the atmosphere and stimulation of O2 production by phytoplankton fertilized with nitrate-rich waters will rapidly increase the O2 levels of upwelled waters in the surface layer. This enhanced phytoplankton production will also consume quickly the CO2 excess of upwelled waters.
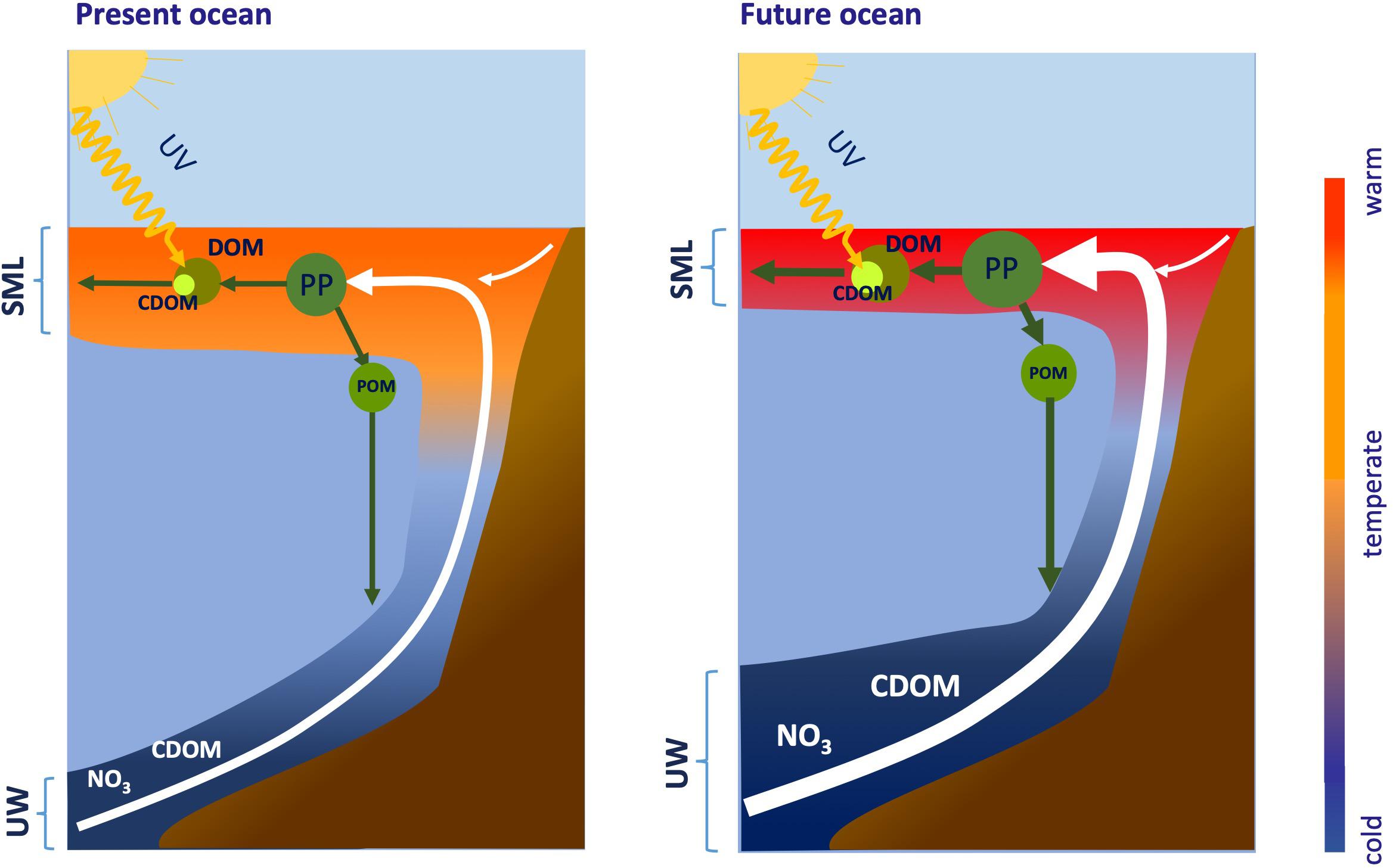
Figure 5. Intensification of summertime winds by global warming will increase upwelling strength at high latitudes within coastal upwelling regions. Global warming will also increase stratification of the surface mixed layer (SML), which will be warmer and shallower in the future ocean. Strengthening of coastal upwelling will intensify the vertical fluxes of nitrate (NO) and colored dissolved organic matter (CDOM) to the SML, proportionally increasing new production, the accumulation of DOM and its horizontal export to the adjacent open ocean. Nitrate and CDOM fluxes will increase because both the upwelling rate and the concentration will rise. The downward flux of particulate organic matter (POM) to shelf bottom sediments will intensify in parallel to new production increase, the horizontal export of DOM to the adjacent ocean will increase because of the increase of outwelling rates and DOM concentrations in the SML, and the photochemical decomposition of the upwelled CDOM will be stimulated by a shallower SML. Predicted weakening of upwelling at the low latitudinal range of the major coastal upwelling system and the Atlantic and Pacific equatorial upwelling regions will produce the opposite effect.
Concomitantly, the horizontal DOC export is amplified because both the concentration of recalcitrant DOC and the mass flux of upwelled water increase in response to global warming. Intensified coastal upwelling conditions combined with increasing stratification (see section “Ocean Warming”) favor a compression of the surface mixed layer in response to the vertical water displacement, a gentler injection of the upwelled nutrients in the surface mixed layer and a more efficient use of them by the plankton assemblages in the surface mixed layer. Predicted weakening of upwelling at the low latitudinal range of the major coastal upwelling system (Rykaczewski et al., 2015) and the Atlantic and Pacific equatorial upwelling regions (Terada et al., 2020) will produce a decline of the nitrate flux and an increase of the stratification and flushing time of the surface mixed layer, leading to a decline of the accumulation of recalcitrant DOC and its export to the adjacent ocean.
In conclusion, predicted changes in the Southern Ocean and AMOC will all cause a reduction in the amount of recalcitrant DOC exported to the deep ocean. Intensified coastal upwelling conditions are predicted for higher latitudes, while at lower latitudes they are projected to weaken. This will at higher latitudes lead to increasing new production and accumulation of recalcitrant DOC, and subsequent increased export to the adjacent open ocean, while the contrary is predicted for lower latitudes. DOC dynamics in deep and intermediate water mass formation areas as well as in coastal upwelling systems have not been studied in the detail that they deserve. The first and main gap is the scarcity and poor spatial and temporal resolution of DOC data. The elemental, isotopic and molecular composition of DOC and how global change stressors impact them should also be studied. Finally, DOC cycling should be properly included in ocean forecasting models.
Wet and Dry Depositions
Air-sea exchange processes depend substantially on both precipitation, which drives the wet deposition of material to the oceans, and on wind speed, which influences the emission and deposition of aerosols (i.e., fine particles or liquids) and gases (e.g., CO2) at the sea surface. Air-sea exchange increases rapidly with increasing wind speed (IPCC, 2013). The exchange of aerosols and gases has to pass through the sea surface microlayer, an interface enriched in DOC and able to modulate the air-sea exchange (Carpenter and Nightingale, 2015). All of these processes are likely to change in the future due to global change, although the extent of change is uncertain, since the pattern and direction of change for storm tracks, wind speed and rainfall will vary geographically (e.g., Kirtman et al., 2013). These exchanges of both aerosols and gasses will in turn feedback and affect global change itself (IPCC, 2013). Because the changes in these physical forcing processes with global change vary regionally, it is not possible to derive a global prediction of how they will affect ocean DOC cycling, but the potential for impacts are real as discussed below. The exchanges of aerosols and gases in both directions across the air-sea interface are closely linked, but for clarity in this section, the impact of atmospheric deposition on the ocean productivity and DOC cycling is considered first, followed by a discussion of the impact of the ocean DOC cycling on the atmosphere.
The atmosphere is a significant source of nutrients to the ocean, particularly for nitrogen (39 Tg N yr–1) and Fe (16 Tg Fe yr–1), although only a fraction of this Fe is soluble and readily bioavailable (e.g., Ito et al., 2019). Hence nutrient deposition from the atmosphere affects ocean productivity, CO2 uptake and DOC cycling (Jickells et al., 2005; Jickells and Moore, 2015; Jickells et al., 2017). The extent of atmospheric nutrient transport is sensitive to changes in nutrient emissions to the atmosphere, as well as changes in atmospheric transport and transformation pathways. Atmospheric transport pathways are vulnerable to global change, and hence the geographic distribution of atmospheric deposition to the ocean may change; for instance, the jet stream transport (the fast high level flow that bounds the polar and temperate air masses in the North Atlantic) may shift poleward (Kirtman et al., 2013). In addition, emissions to the atmosphere are likely to change in the future. In the case of Fe inputs to the atmosphere, there are some important anthropogenic Fe emissions, such as from coal combustion and metal smelting, but the main source is desert dust, and these emissions are sensitive to global change (Jickells et al., 2005; Ito et al., 2019). The scale of future changes in desert dust emissions is uncertain, since it depends on wind intensity and direction, temperature and most critically rainfall patterns in the source regions (Mahowald et al., 2011). Fe is only very sparingly soluble at ocean pHs and its bioavailability in seawater is regulated by complexation with OM (enhancing Fe solubility and ocean residence time) (Tagliabue et al., 2017). This complexing OM is itself a component of the ocean DOC. Organic complexation of Fe probably also plays a role in its atmospheric transport, deposition and bioavailability (Cheize et al., 2012).
The main sources of nitrogen emissions to the atmosphere are now anthropogenic and hence directly influenced by human activity. The total nitrogen emissions are projected to remain stable globally over the coming decades, but the global distribution of emission and deposition will probably change with increasing inputs downwind of Asia balanced by decreases elsewhere, and an increase in ammonium deposition and a decrease in nitrate. This latter change reflects the increasing controls on oxidized nitrogen emissions, due to the use of catalytic converters on cars, and the less regulated emissions of ammonia, which comes primarily from an increasing agricultural production sector, with the reduced/oxidized nitrogen deposition ratio projected to change from about 0.7 to 1.0 from 2005 to 2050 (Jickells et al., 2017). Atmospheric nitrogen deposition to the oceans includes a substantial contribution (25%; Jickells et al., 2017) from DON. This organic nitrogen is of uncertain bioavailability and contains a wide variety of organic compounds from terrestrial and marine sources. Sources are many but poorly characterized, and include anthropogenic and natural sources, as well as biomass burning, which may be both natural and anthropogenic (Cape et al., 2011; Jickells et al., 2017).
Given that, some studies suggest that 50% of ocean export production is regulated by atmospheric Fe supply, and only a few percent by atmospheric nitrogen supply (Krishnamurthy et al., 2010), it seems reasonable to assume that variations in atmospheric Fe supply to the ocean with global change, particularly changes in desert dust emissions and transport, have the greater potential to influence ocean productivity and DOC cycling, compared to changes in nitrogen deposition.
Globally the atmosphere is a direct DOC source (∼100 Tg C yr–1) to the oceans (Willey et al., 2000; Jurado et al., 2008; Carlson and Hansell, 2015). While this contribution only equals approximately half of estimated riverine inputs, the influence on the ocean is different, as all atmospherically transported material will directly reach the open ocean, while a variable proportion of riverine inputs will be degraded in coastal waters and not reach the open ocean (see section “River Derived Material”). This atmospheric transport of DOC will include the organic nitrogen compounds noted above. The global magnitude of this atmospheric DOC source to the oceans is poorly characterized. This partly reflects the very limited measurements of rainwater and aerosol DOC and uncertainty over emission sources; in addition, a part of the DOC within the atmosphere may in fact be recycled from seawater via sea spray production (injection of seawater into the atmosphere by breaking waves). Indeed, this latter process has been argued to be an important sink for ocean DOC, particularly recalcitrant components (Kieber et al., 2016; Beaupré et al., 2019). The proposed mechanism is that recalcitrant marine DOC is injected into the atmosphere associated with bursting bubbles. This aerosol may be enriched in sea spray relative to sea salt due to the higher surface activity of the DOC itself, meaning the DOC is concentrated on the surface of rising bubbles both in the bulk water column and the microlayer. The resulting aerosol containing DOC will reside in the atmosphere for days where it will be subject to high sunlight conditions, and hence may be at least partly photodegraded to CO2 and/or converted into more labile DOC (see section “Marine organic matter”) before re-deposition to the ocean or transfer to land. This newly proposed air-sea exchange mechanism of ocean DOC cycling clearly merits further study. The production of marine aerosol and its re-deposition is a non-linear function of wind speed (e.g., Hoppel et al., 2002), and hence sensitive to global change induced changes in wind speed which are projected to vary geographically (McInnes et al., 2011). Hence, again it is not simple to derive an estimate of how this process will affect ocean DOC on a global scale, although (Beale et al., 2014) suggest that the overall effect of global warming will be a reduction in poleward temperature gradients and hence a reduction in wind speeds (see also section “Changes in Ocean Circulation and Upwelling Patterns”).
In addition to the transfer of relatively involatile organic compounds from the ocean to atmospheric aerosol, the photodegradation of DOC in the water column results in the production of a wide range of volatile trace gases in the water column (part of EDOC see section “Marine organic matter”). These gases include methanol, acetone, ethane, carbon monoxide, carbonyl sulfide and some low molecular weight sulfur and halogen gases, while some other gases, such as dimethyl sulfide, are produced by direct biological processes in the marine surface waters (Beale et al., 2014; Carpenter and Nightingale, 2015). Many of these gases are degraded in ocean surface waters. However, a fraction of these gases escapes the ocean by air-sea exchange into the atmosphere before degradation. The air-sea exchange of these gases plays a very important role in the chemistry of the atmosphere and thereby the climate and is again subject to a non-linear relationship to wind speed and to temperature (Beale et al., 2014; Carpenter and Nightingale, 2015). Ocean acidification may also affect the production and emission of these gases from the ocean (Hopkins et al., 2020).
Hence it is clear that the atmosphere influences ocean productivity and DOC cycling, which in turn can influence atmospheric chemistry. There is a strong dependence of air-sea exchange processes on climatic drivers, particularly wind speed and rainfall. These drivers will vary with global change making the air-sea exchanges themselves sensitive to global change. However, there is no single global unidirectional change for these climatic drivers predicted with changing climate, but rather strong regional differences in the extent and direction of change.
We lack data to validate fluxes of organic carbon both to and from the ocean, and compositional information on this organic carbon. Linking these fluxes to ocean, atmosphere and land processes will allow models to be developed to predict how these fluxes will change under global change scenarios. The sensitivity of the biologically driven trace gas emissions from the ocean to acidification (Hopkins et al., 2020) means that research in this area would be particularly valuable in order to understand the implications of changes in these fluxes on the atmosphere.
Conclusion
Understanding how global change stressors impact the ocean DOC cycling is a challenging task as many processes act at the same time and are impacted by a wide range of other processes varying temporally and spatially. Therefore, these stressors might show impacts in some circumstances but not in others.
In this manuscript, we explored some of the possible global change stressors that could impact future DOC cycling, however, there also are unknowns and uncertainties in the above sections. This makes straightforward predictions challenging, but some general patterns emerged (Table 1):
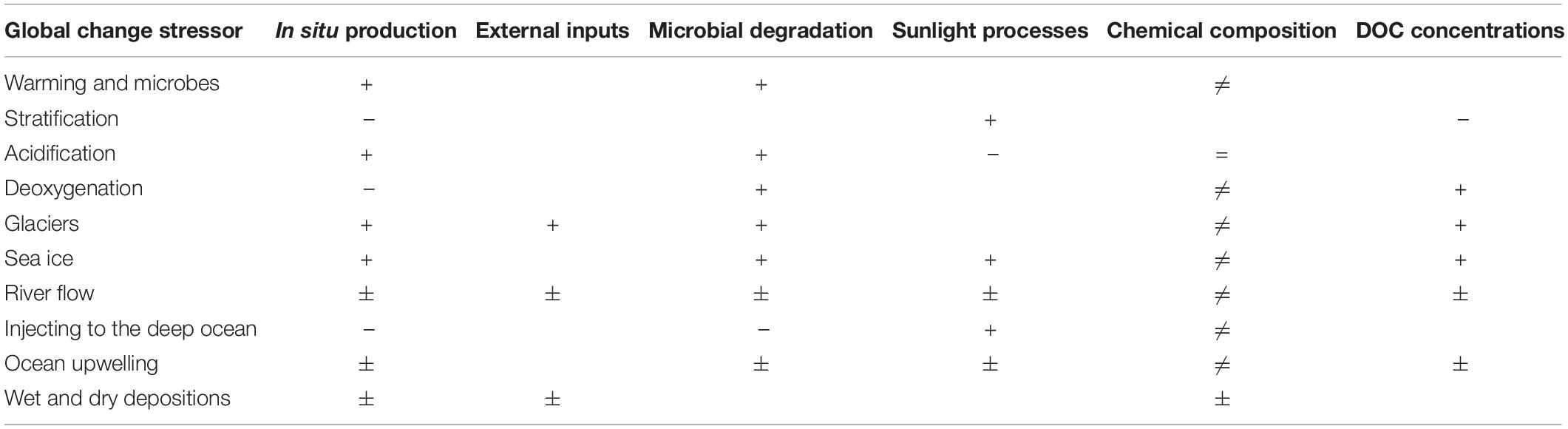
Table 1. Summary of the possible impacts of global change stressors on the DOC cycling in the future ocean over large scales. Please note that these possible impacts in some cases are theoretically based and will need further testing to quantify their specific impacts. The symbols represent: + for increase; – for decrease; ± for no overall suggested changes; = for similar composition; ≠ for changed composition.
(1) Ocean warming: Warming will directly impact microbial processes and is expected to cause an increase in production and degradation of DOC. However, the increase in the stratification strength and duration due to warming, could reduce DOC production and levels in response to the decline in the nitrate flux and increased impact of photodegradation. In seasonally stratified waters, deeper winter mixing layer depths in response to more intense winter storm events, would partly counteract the effect of increased seasonal stratification.
(2) Ocean acidification: Overall acidification could increase both the production and microbial degradation of DOC and potentially decrease the importance of photodegradation processes. It may also change the emission fluxes of climatically important trace gases from the ocean.
(3) Ocean deoxygenation: Decreasing O2 levels could reduce DOC production and lead to slower degradation and change its chemical composition. Combined, these changes could increase the DOC concentrations.
(4) Glacial and Sea Ice: Increased glacial DOC inputs could, initially increase phytoplankton productivity, however, later when most glaciers have melted, a shift toward larger terrestrial inputs are expected. The melting sea ice is expected to lead to higher DOC production and levels. Both glacial and sea ice melting would change the DOC chemical composition.
(5) River inputs: In the Arctic, northern Europe and America, increasing deliveries of river derived DOC are expected. Extreme events, such as storms and droughts, could cause large regional episodic exports of DOC to coastal waters. In Asia, South America, and Africa the construction of large dams could lead to temporary regional increases in DOC delivery. Combined, these could change the DOC chemical composition and flux.
(6) Ocean currents and upwelling: Forecasted weakening of intermediate and deep-water formation in the Arctic and Antarctic oceans could decrease the injection of DOC in the ocean interior. Intensified coastal upwelling conditions could lead to increasing production and degradation of DOC in those areas, with overall increases in concentrations and export to the adjacent oligotrophic ocean. Changes in ocean current and upwelling could increase the impact of photodegradation processes and change the DOC chemical composition.
(7) Atmospheric dry and wet deposition: There is no single global unidirectional change in atmospheric depositions with changing climate, but rather strong regional differences in the extent and direction of change are expected.
From the above points (Table 1) it is evident that it is difficult to conclude on a unified outcome of the future impacts of the global stressors mentioned regarding ocean DOC cycling. While most of the stressors have a global impact, distinct regional differences are evident as seen, e.g., on the river inflow of DOC or the latitude range of coastal upwelling systems. Also, ocean warming will likely reduce production in already stratified low and temperate latitudes, but might enhance production at high latitudes. Ocean acidification appears to cause most problems at high latitudes, where waters have a natural limited capacity to buffer against pH changes.
Our current understanding of many of the global change stressors reviewed here are mainly based on results from laboratory and mesocosm studies, which are difficult to extrapolate to the real world, as these experiments might miss some processes and organism interactions, and the experimental timescales do not normally allow for a potential adaptation to the stressor. But it should also be considered that global change stressors are linked together, e.g., respiration is expected to increase with warming, which in turn will reduce O2 levels and increase acidity.
Obtaining further knowledge of ocean DOC cycling and global change is important for our ability to accurately understand and forecast how the ocean will respond to global changes. The ocean DOC pool is very large and small changes in inputs and removal, could result in substantial net changes in the ocean carbon cycle and climate.
Author Contributions
All authors listed have made a substantial, direct and intellectual contribution to the work, and approved it for publication.
Funding
Thanks are due to FCT/MCTES for the financial support to CESAM (UIDP/50017/2020 + UIDB/50017/2020), through national funds. CC was supported by Fundação para a Ciência e a Tecnologia (FCT; SFRH/BPD/117746/2016). XÁ-S was funded by the Spanish MICINN (CTM2015-69392-C3-2-R), co-funded by FEDER.
Conflict of Interest
The authors declare that the research was conducted in the absence of any commercial or financial relationships that could be construed as a potential conflict of interest.
The reviewer DH declared a past co-authorship with the authors XÁ-S and CL to the handling editor.
Acknowledgments
We thank the three reviewers for their detailed comments and useful suggestions which helped improve the manuscript. We would also like to thank M. J. Pazo for help with some of the drawings.
References
Aksenov, Y., Ivanov, V. V., Nurser, A. J. G., Bacon, S., Polyakov, I. V., Coward, A. C., et al. (2011). The Arctic circumpolar boundary current. J. Geophys. Res. 116:C09017. doi: 10.1029/2010JC006637
Alderkamp, A.-C., Mills, M. M., Dijken, G. L. V., Laan, P., Thuróczy, C.-E., Gerringa, L. J. A., et al. (2012). Iron from melting glaciers fuels phytoplankton blooms in the Amundsen Sea (SouthernOcean): phytoplankton characteristics and productivity. Deep Sea Res. II 71-76, 32–48. doi: 10.1016/j.dsr2.2012.03.005
Alvarez-Fernandez, S., Bach, L. T., Taucher, J., Riebesell, U., Sommer, U., Aberle, N., et al. (2018). Plankton responses to ocean acidification: the role of nutrient limitation. Prog. Oceanogr. 165, 11–18. doi: 10.1016/j.pocean.2018.04.006
Alvarez-Salgado, X. A., Arıstegui, J., Barton, E. D., and Hansell, D. A. (2007). Contribution of upwelling filaments to offshore carbon export in the subtropical Northeast Atlantic Ocean. Limnol. Oceanogr. 52, 1287–1292. doi: 10.4319/lo.2007.52.3.1287
Amon, R. M. W., and Benner, R. (1996). Bacterial utilization of different size classes of dissolved organic matter. Limnol. Oceanogr. 41, 41–51. doi: 10.4319/lo.1996.41.1.0041
Amos, C. L., Rashidi, T. A., Rakha, K., El-Gamily, H., and Nichlls, R. (2003). Sea surface temperature trends in the coastal ocean. Curr. Dev. Oceanogr. 6, 1–13.
Anderson, L. A. (1995). On the hydrogen and oxygen content of marine phytoplankton. Deep Sea Res. I 42, 1675–1680. doi: 10.1016/0967-0637(95)00072-E
Andrews, S. S., and Zafiriou, O. C. (2000). Photochemical oxygen consumption in marine waters: a Major soink for colored dissolved organic matter? Limnol. Oceanogr. 45, 267–277. doi: 10.4319/lo.2000.45.2.0267
Anesio, A. M., Hodson, A. J., Fritz, A., Psenner, R., and Sattler, B. (2009). High microbial activity on glaciers: importance to the global carbon cycle. Glob. Change Biol. 15, 955–960. doi: 10.1111/j.1365-2486.2008.01758.x
Archer, D., Peltzer, E. T., and Kirchman, D. L. (1997). A timescale for dissolved organic carbon production in equatorial Pacific surface waters. Glob. Biogeochem. Cycles 11, 435–452. doi: 10.1029/97GB01196
Arnosti, C., and Repeta, D. J. (1994). Extracellular enzyme activity in anaerobic bacterial cultures evidence of pullulanase activity among mesophilic marine bacteria. Appl. Environ. Microbiol. 60, 840–846. doi: 10.1128/AEM.60.3.840-846.1994
Arrhenius, S. Z. (1889). Über die reaktionsgeschwindigkeit bei der inversion von rohrzucker durch säuren. Phys. Chem. 4, 226–248. doi: 10.1515/zpch-1889-0416
Arrigo, K. R. (2014). Sea ice ecosystems. Annu. Rev. Mar. Sci. 6, 439–467. doi: 10.1146/annurev-marine-010213-135103
Atkinhead-Peterson, J. A., Mcdowell, W. H., and Neff, J. C. (2003). “Sources, production and regulation of allochthonous dissolved organic matter inputs to surface waters,” in Aquatic Ecosystems, Interactivity of Dissolved Organic Matter, eds S. E. G. Findley and R. L. Sinsabaugh (San Diego, CA: Academic Press), 26–70. doi: 10.1016/B978-012256371-3/50003-2
Bakun, A., Black, B. A., Bograd, S. J., García-Reyes, M., Miller, A. J., Rykaczewski, R. R., et al. (2015). Anticipated effects of climate change on coastal upwelling ecosystems. Curr. Clim. Change Rep. 1, 85–93. doi: 10.1007/s40641-015-0008-4
Balmaseda, M. A., Trenberth, K. E., and Källén, E. (2013). Distinctive climate signals in reanalysis of global ocean heat content. Geophys. Res. Lett. 40, 1754–1759. doi: 10.1002/grl.50382
Barrón, C., Apostolaki, E. T., and Duarte, C. M. (2014). Dissolved organic carbon fluxes by seagrass meadows and macroalgal beds. Front. Mar. Sci. 1:42. doi: 10.3389/fmars.2014.00042
Barrón, C., and Duarte, C. M. (2015). Dissolved organic carbon pools and export from the coastal ocean. Glob. Biogeochem. Cycles 29, 1725–1738. doi: 10.1002/2014GB005056
Bastviken, D., Persson, L., Odham, G., and Tranvik, L. (2004). Degradation of dissolved organic matter in oxic and anoxic lake water. Limnol. Oceanogr. 49, 109–116. doi: 10.4319/lo.2004.49.1.0109
Beale, R., Johnson, M., Liss, P. S., and Nightingale, P. D. (2014). “Air–sea exchange of marine trace gases,” in Treatise on Geochemistry, 2nd Edn, Vol. 8, eds M. J. Mottl and H. Elderfield (Oxford: Elsevier), 53–92. doi: 10.1016/B978-0-08-095975-7.00603-3
Beardall, J., and Raven, J. A. (2004). The potential effects of globalclimate change on microalgal photosynthesis, growth and ecology. Phycologia 43, 26–40. doi: 10.2216/i0031-8884-43-1-26.1
Beaupré, S. R., Kieber, D. J., Keene, W. C., Long, M. S., Maben, J. R., Lu, X., et al. (2019). Oceanic efflux of ancient marine dissolved organic carbon in primary marine aerosol. Sci. Adv. 5:eaax6535. doi: 10.1126/sciadv.aax6535
Benner, R. (2004). What happens to terrestrial organic matter in the ocean? Mar. Chem. 92, 307–310. doi: 10.1016/j.marchem.2004.06.033
Benner, R., and Amon, R. M. (2015). The size-reactivity continuum of major bioelements in the ocean. Ann. Rev. Mar. Sci. 7, 185–205. doi: 10.1146/annurev-marine-010213-135126
Benner, R., Benitez-Nelson, B., Kaiser, K., and Amon, R. M. W. (2004). Export of young terrigenous dissolved organic carbon from rivers to the Arctic Ocean. Geophys. Res. Lett. 31:L05305. doi: 10.1029/2003GL019251
Benner, R., and Opsahl, S. (2001). Molecular indicators of the sources and transformations of dissolved organic matter in the Mississippi river plume. Org. Geochem. 32, 597–611. doi: 10.1016/S0146-6380(00)00197-2
Benner, R., and Ziegler, S. (1999). “Do photochemical transformations of dissolved organic matter produce biorefractory as well as bioreactive substrates?” in Proceedings of the 8th International Symposium on Microbial Ecology, eds C. R. Bell, M. Brylinsky, and P. Johnson-Green (Port Aransas, TX: University of Texas at Austin).
Bergen, B., Endres, S., Engel, A., Zark, M., Dittmar, T., Sommer, U., et al. (2016). Acidification and warming affect prominent bacteria in two seasonal phytoplankton bloom mesocosms. Environ. Microbiol. 18, 4579–4595. doi: 10.1111/1462-2920.13549
Berto, S., Laurentiis, E. D., Tota, T., Chiavazza, E., Daniele, P. G., Minella, M., et al. (2016). Properties of the humic-like material arising from the phototransformation of L-tyrosine. Sci. Total Environ. 546, 434–444. doi: 10.1016/j.scitotenv.2015.12.047
Beusen, A. H. W., Bouwman, A. F., Beek, L. P. H. V., Mogollón, J. M., and Middelburg, J. J. (2016). Global riverine N and P transport to ocean increased during the 20th century despite increased retention along the aquatic continuum. Biogeosciences 13, 2441–2451. doi: 10.5194/bg-13-2441-2016
Bianchi, T. S. (2011). The role of terrestrially derived organic carbon in the coastal ocean: a changing paradigm and the priming effect. Proc. Natl. Acad. Sci. U.S.A. 108, 19473–19481. doi: 10.1073/pnas.1017982108
Bianchi, T. S., Cui, X., Blair, N. E., Burdige, D. J., Eglinton, T. I., and Galy, V. (2018). Centers of organic carbon burial and oxidation at the land-ocean interface. Org. Geochem. 115, 138–155. doi: 10.1016/j.orggeochem.2017.09.008
Bianchi, T. S., Garcia-Tigreros, F., Yvon-Lewis, S. A., Shields, M., Mills, H. J., Butman, D., et al. (2013). Enhanced transfer of terrestrially derived carbon to the atmosphere in a flooding event. Geophys. Res. Lett. 40, 116–122. doi: 10.1029/2012GL054145
Björnerås, C., Weyhenmeyer, G. A., Evans, D., Gessner, M. O., Grossart, H.-P., Kangur, K., et al. (2017). Widespread increases in iron concentration in European and North American freshwaters. Glob. Biogeochem. Cycles 31, 1488–1500. doi: 10.1002/2017GB005749
Blunden, J., and Arndt, D. S. (2012). State of the Climate in 2011. Bull. Am. Meteorol. Soc. 93, S1–S264. doi: 10.1175/2012BAMSStateoftheClimate.1
Boekell, W. H. M. V., Hansen, F. C., Riegman, R., and Bak, R. P. M. (1992). Lysis-induced decline of a Phaeocystis spring bloom and coupling with the microbial foodweb. Mar. Ecol. Prog. Ser. 81, 269–276. doi: 10.3354/meps081269
Boetius, A., Anesio, A. M., Deming, J. W., Mikucki, J. A., and Rapp, J. Z. (2015). Microbial ecology of the cryosphere: sea ice and glacial habitats. Nat. Rev. Microbiol. 13, 677–690. doi: 10.1038/nrmicro3522
Bograd, S. J., Castro, C. G., Di Lorenzo, E., Palacios, D. M., Bailey, H., Gilly, W., et al. (2008). Oxygen declines and the shoaling of the hypoxic boundary in the California Current. Geophys. Res. Lett. 35:L12607. doi: 10.1029/2008GL034185
Bograd, S. J., Pozo Buil, M., Dilorenzo, E., Castro, C. G., Schroeder, I. D., Goericke, R., et al. (2015). Changes in source waters to the Southern California Bight. Deep Sea Res. II 112, 42–52. doi: 10.1016/j.dsr2.2014.04.009
Bopp, L., Resplandy, L., Orr, J. C., Doney, S. C., Dunne, J. P., Halloran, M. G. P., et al. (2013). Multiple stressors of ocean ecosystems of the 21st century: projections with CMIP5 models. Biogeosciences 10, 6225–6245. doi: 10.5194/bg-10-6225-2013
Bosatta, E., and Ågren, G. (1999). Soil organic matter quality interpreted thermodynamically. Soil Biol. Biochem. 31, 1889–1891. doi: 10.1016/S0038-0717(99)00105-4
Brewer, P. G., and Peltzer, E. T. (2017). Depth perception: the need to report ocean biogeochemical rates as functions of temperature, not depth. Philos. Trans. R. Soc. A 375:20160319. doi: 10.1098/rsta.2016.0319
Brilinsky, M. (1977). Release of dissolved organic matter by some marine macrophytes. Mar. Biol. 39, 213–220. doi: 10.1007/BF00390995
Brown, J. H., Gillooly, J. F., Allen, A. P., Savage, V. M., and West, G. B. (2004). Towards a metabolic theory of ecology. Ecology 85, 1771–1789. doi: 10.1890/03-9000
Burd, A. B., Frey, S., Cabre, A., Ito, T., Levine, N. M., et al. (2016). Terrestrial and marine perspectives on modeling organic matter degradation pathways and controls. Glob. Change Biol. 22, 121–136. doi: 10.1111/gcb.12987
Burdige, D. J., and Gardner, K. G. (1998). Molecular weight distribution of dissolved organic carbon in marine sediment pore waters. Mar. Chem. 62, 45–64. doi: 10.1016/S0304-4203(98)00035-8
Burdige, D. J., and Komada, T. (2014). “Sediment pore waters,” in Biogeochemistry of Marine Dissolved Organic Matter, eds D. A. Hansen and C. A. Carlson (Cambridge, MA: Academic Press), 535–577. doi: 10.1016/B978-0-12-405940-5.00012-1
Burnett, W. C., Aggarwal, P. K., Aureli, A., Bokuniewicz, H., Cable, J. E., Charette, M. A., et al. (2006). Quantifying submarine groundwater discharge in the coastal zone via multiple methods. Sci. Total Environ. 367, 498–543. doi: 10.1016/j.scitotenv.2006.05.009
Caesar, L., Rahmstorf, S., Robinson, A., Feulner, G., and Saba, V. (2018). Observed fingerprint of a weakening Atlantic Ocean overturning circulation. Nature 556, 191–196. doi: 10.1038/s41586-018-0006-5
Canfield, D. E., and Thamdrup, B. (2009). Towards a consistent classification scheme for geochemical environments, or, why we wish the term ‘suboxic’ would go away. Geobiology 7, 385–392. doi: 10.1111/j.1472-4669.2009.00214.x
Canfield, D. E., Thamdrup, B., and Kristensen, E. (2005). Aquatic Geomicrobiology. Amsterdam: Elsevier Academic Press. doi: 10.1515/9781501509551
Cape, J. N., Cornell, S. E., Jickells, T. D., and Nemitz, E. (2011). Organic nitrogen in the atmosphere — Where does it come from? A review of sources and methods. Atmos. Res. 102, 30–48. doi: 10.1016/j.atmosres.2011.07.009
arlson, C. A., and Hansell, D. A. (2015). “DOM sources, sinks, reactivity, and budgets,” in Biogeochemistry of Marine Dissolved Organic Matter, eds C. A. Carlson and D. A. Hansell (San Diego, CA: Academic Press), 65–126. doi: 10.1016/B978-0-12-405940-5.00003-0
Carlson, C. A., Hansell, D. A., Nelson, N. B., Siegel, D. A., Smethie, W. M., Khatiwala, S., et al. (2010). Dissolved organic carbon export and subsequent remineralization in the mesopelagic and bathypelagic realms of the North Atlantic basin. Deep Sea Res. II 57, 1433–1445. doi: 10.1016/j.dsr2.2010.02.013
Carpenter, L. J., and Nightingale, P. D. (2015). Chemistry and release of gases from eth surface ocean. Chem. Rev. 115, 4015–4034. doi: 10.1021/cr5007123
Cermeño, P., Dutkiewicz, S., Harris, R. P., Follows, M., Schofield, O., and Falkowski, P. G. (2008). The role of nutricline depth in regulating the ocean carbon cycle. Proc. Natl. Acad. Sci. U.S.A. 105, 20344–20349. doi: 10.1073/pnas.0811302106
Cheize, M., Sarthou, G., Croot, P. L., Bucciarelli, E., Baudoux, A.-C., and Baker, A. R. (2012). Iron organic speciation determination in rainwater using cathodic stripping voltammetry. Anal. Chim. Acta 736, 45–54. doi: 10.1016/j.aca.2012.05.011
Chen, W., Chiang, J., and Zhang, D. (2013). Atlantic Meridional Overturning Circulation (AMOC) in CMIP5 models: RCP and Historical Simulations. J. Clim. 26, 7187–7197. doi: 10.1175/JCLI-D-12-00496.1
Cheng, L., Zhu, J., Abraham, J., Trenberth, K. E., Fasullo, J. T., Zhang, B., et al. (2019). 2018 Continues record global ocean warming. Adv. Atmos. Sci. 36, 249–252. doi: 10.1007/s00376-019-8276-x
Chin, W. C., Orellana, M. V., and Verdugo, P. (1998). Spontaneous assembly of marine dissolved organic matter into polymer gels. Nature 391, 568–572. doi: 10.1038/35345
Church, J. A., Clark, P. U., Cazenave, A., Gregory, J. M., Jevrejeva, S., Levermann, A., et al. (2013). “Sea level change,” in Climate Change 2013: The Physical Science Basis. Contribution of Working Group I to the Fifth Assessment Report of the Intergovernmental Panel on Climate Change, eds T. F. Stocker, D. Qin, G.-K. Plattner, M. Tignor, S. K. Allen, J. Boschung, et al. (Cambridge: Cambridge University Press).
Ciais, P., Sabine, C., Bala, G., Bopp, L., Brovkin, V., Canadell, J., et al. (2013). “Carbon and other biogeochemical cycles,” in Climate Change 2013: The Physical Science Basis. Contribution of Working Group I to the Fifth Assessment Report of the Intergovernmental Panel on Climate Change, eds T. F. Stocker, D. Qin, G. Plattner, K. M. Tignor, S. K. Allen, J. Boschung, et al. (Cambridge: Cambridge University Press).
Coble, P. G. (2007). Marine optical biogeochemistry: the chemistry of ocean color. Chem. Rev. 107, 402–418. doi: 10.1021/cr050350+
Codispoti, L. A., Friederich, G. E., Packard, T. T., Glover, H. E., Kelly, P. J., Spinrad, R. W., et al. (1986). High nitrite levels off Northern Peru: a signal of instability in the marine denitrification rate. Science 233, 1200–1202. doi: 10.1126/science.233.4769.1200
Cotner, J. B., Ammerman, J. A., Peele, E. R., and Bentzen, E. (1997). Phosphorus limited bacterioplankton growth in the Sargasso Sea. Aquat. Microb. Ecol. 13, 141–149. doi: 10.3354/ame013141
Coumou, D., and Rahmstorf, S. (2012). A decade of weather extremes. Nat. Clim. Change 2, 491–496. doi: 10.1038/nclimate1452
Cuskin, F., Lowe, E. C., Temple, M. J., Zhu, Y., Cameron, E. A., Pudlo, N. A., et al. (2015). Human gut Bacteroidetes can utilize yeast mannan through a selfish mechanism. Nature 517, 165–186. doi: 10.1038/nature13995
Dachs, J., Calleja, M. L., Duarte, C. M., Vento, S. D., Turpin, B., Polidori, A., et al. (2005). High atmosphere-ocean exchange of organic carbon in the NE subtropical Atlantic. Geophys. Res. Lett. 32:L21807. doi: 10.1029/2005GL023799
Dachs, J., and Méjanelle, L. (2010). Organic pollutants in coastal waters, sediments, and biota: a relevant driver for ecosystems during the anthropocene? Estuarines Coasts 33, 1–14. doi: 10.1007/s12237-009-9255-8
Davidson, E. A., and Janssens, I. A. (2006). Temperature sensitivity of soil carbon decomposition and feedbacks to climate change. Nature 440, 165–173. doi: 10.1038/nature04514
Davidson, E. A., Trumbore, S. E., and Amundson, R. (2000). Soil warming and organic carbon content. Nature 408, 789–790. doi: 10.1038/35048672
Del-Giorgio, P., and Davies, J. (2003). “Patterns of dissolved organic matter lability and consumption across aquatic ecosystems,” in Aquatic Ecosystems: Interactivity of Dissolved Organic Matter, eds S. E. G. Findlay and R. L. Sinsabaugh (San Diego, CA: Academic Press), 399–424. doi: 10.1016/B978-012256371-3/50018-4
Diaz, R. J., Rosenberg, R., and Sturdivant, K. (2019). “Hypoxia in estuaries and semi-enclosed seas,” in Ocean Deoxygenation: Everyone’s Problem - Causes, Impacts, Consequences and Solutions, eds D. Laffoley and J. M. Baxter (Gland: IUCN Global Marine and Polar Programme), 85–102.
Dittmar, T., and Koch, B. P. (2006). Thermogenic organic matter dissolved in the abyssal ocean. Mar. Chem. 102, 208–217. doi: 10.1016/j.marchem.2006.04.003
Dittmar, T., and Paeng, J. (2009). A heat-induced molecular signature in marine dissolved organic matter. Nat. Geosci. 2, 175–179. doi: 10.1038/ngeo440
D’Odorico, P., Bhattachan, A., Davis, K. F., Ravi, S., et al. (2013). Global desertification: drivers and feedbacks. Adv. Water Resour. 51, 326–344. doi: 10.1016/j.advwatres.2012.01.013
Doney, S. C. (2010). The growing human footprint on coastal and open-ocean biogeochemistry. Science 328, 1512–1516. doi: 10.1126/science.1185198
Dore, M. H. I. (2005). Climate change and changes in global precipitation patterns: what do we know? Environ. Int. 31, 1167–1181. doi: 10.1016/j.envint.2005.03.004
Downes, S., Bindoff, N. L., and Rintoul, S. R. (2010). Changes in the subduction of southern ocean water masses at the end of the twenty-first century in eight IPCC mode. J. Clim. 23, 6526–6541. doi: 10.1175/2010JCLI3620.1
Dregne, H. E. (1986). “Desertification of arid lands,” in Physics of Desertification, eds F. El-Baz and M. H. A. Hassan (London: Kluwer Academic Press), 4–21. doi: 10.1007/978-94-009-4388-9_2
Ducklow, H. W., Clarke, A. W., Dickhut, R., Doney, S. C., Geisz, H., Huang, K., et al. (2012). “The Marine Ecosystem of the West Antarctic Peninsula,” in Antarctica: An Extreme Environment in a Changing World, eds A. Clarke, N. M. Johnston, E. J. Murphy, and A. D. Rogers (London: Wiley-Blackwell), 121–159. doi: 10.1002/9781444347241.ch5
Eckard, R. S., Pellerin, B. A., Bergamaschi, B. A., Bachand, P. A. M., Bachand, S. M., Spencer, R. G. M., et al. (2017). Dissolved organic matter compositional change and biolability during two storm runoff events in a small agricultural watershed. J. Geophys. Res. Biogeosci. 122, 2634–2650. doi: 10.1002/2017JG003935
Engel, A., Borchard, C., Piontek, J., Schulz, K. G., Riebesell, U., and Bellerby, R. (2013). CO2 increases 14C primary production in an Arctic plankton community. Biogeosciences 10, 1291–1308. doi: 10.5194/bg-10-1291-2013
Engel, A., Händel, N., Wohlers, J., Lunau, M., Grossart, H.-P., Sommer, U., et al. (2011). Effects of sea surface warming on the production and composition of dissolved organic matter during phytoplankton blooms: results from a mesocosm study. J. Plankton Res. 33, 357–370. doi: 10.1093/plankt/fbq122
Evans, C. D., Chapman, P. J., Clark, J. M., Monteith, D. T., and Cresser, M. S. (2006). Alternative explanations for rising dissolved organic carbon export from organic soils. Glob. Change Biol. 12, 2044–2053. doi: 10.1111/j.1365-2486.2006.01241.x
Fellman, J. B., Hood, E., Spencer, R. G. M., Stubbins, A., and Raymond, P. A. (2014). Watershed glacier coverage influences dissolved organic matter biogeochemistry in coastal watersheds of southeast Alaska. Ecosystems 17, 1014–1025. doi: 10.1007/s10021-014-9777-1
Fenchel, T., and Finlay, B. J. (1995). Ecology and Evolution in Anoxic Worlds. Oxford: Oxford University Press.
Fernández-Castro, B., Álvarez, M., Nieto-Cid, M., Zunino, P., Mercier, H., and Álvarez-Salgado, X. A. (2019). Net production and export of dissolved organic nitrogen in the subpolar North Atlantic. Geophys. Res. Lett. 46, 3832–3842. doi: 10.1029/2018GL080284
Fernández-Méndez, M., Katlein, C., Rabe, B., Nicolaus, M., Peeken, I., Bakker, K., et al. (2015). Photosynthetic production in the central Arctic Ocean during the record sea-ice minimum in 2012. Biogeosciences 12, 3525–3549. doi: 10.5194/bg-12-3525-2015
Findlay, S., and Sinsabaugh, R. L. (1999). Unravelling the sources and bioavailability of dissolved organic matter in lotic aquatic ecosystems. Mar. Freshw. Res. 50, 781–790. doi: 10.1071/MF99069
Finstad, A. G., Andersen, T., Larsen, S., Tominaga, K., Blumentrath, S., Wit, H. A. D., et al. (2016). From greening to browning: catchment vegetation development and reduced S-deposition promote organic carbon load on decadal time scales in Nordic lakes. Sci. Rep. 6:31944. doi: 10.1038/srep31944
Flombaum, P., Gallegos, J. L., Gordillo, R. A., Rincón, J., Zabala, L. L., Jiao, N., et al. (2013). Present and future global distributions of the marine cyanobacteria Prochlorococcus and Synechococcus. Proc. Natl. Acad. Sci. U.S.A. 110, 9824–9829. doi: 10.1073/pnas.1307701110
Foley, J. (2017). Living by the lessons of the planet. Science 356, 251–251. doi: 10.1126/science.aal4863
Foley, J. A., Defries, R., Asner, G. P., Barford, C., Bonan, G., Carpenter, S. R., et al. (2005). Global consequences of land use. Science 309, 570–574. doi: 10.1126/science.1111772
Fontela, M., García-Ibáñez, M. I., Hansell, D. A., Mercier, H., and Pérez, F. F. (2016). Dissolved organic carbon in the North Atlantic Meridional Overturning Circulation. Sci. Rep. 6:26931. doi: 10.1038/srep26931
Foreman, C. M., Sattler, B., Mikucki, J. A., Porazinska, D. L., and Priscu, J. C. (2007). Metabolic activity and diversity of cryoconites in the Taylor Valley, Antarctica. J. Geophys. Res. 112:G04S32. doi: 10.1029/2006JG000358
Fraga, F., Ríos, A. F., Pérez, F. F., and Figueiras, F. G. (1998). Theoretical limits of oxygen: carbon and oxygen:nitrogen ratios during photosynthesis and mineralization of organic matter in the sea. Sci. Mar. 62, 161–168. doi: 10.3989/scimar.1998.62n1-2161
Frey, K. E., Comiso, J. C., Cooper, L. W., Eisner, L. B., Gradinger, R. R., Gremeier, J. M., et al. (2017). Arctic Ocean Primary Productivity. In Arctic Report Card 2017. Available online at: http://www.arctic.noaa.gov/Report-Card (accessed February 21, 2020).
García-Reyes, M., Sydeman, W. J., Schoeman, D. S., Rykaczewski, R. R., Black, B. A., Smit, A. J., et al. (2015). Under pressure: climate change, upwelling, and eastern boundary upwelling ecosystems. Front. Mar. Sci. 2:109. doi: 10.3389/fmars.2015.00109
Gattuso, J.-P., Magnan, A., Billé, R., Cheung, W. W. L., Howes, E. L., Joos, F., et al. (2015). Contrasting futures for ocean and society from different anthropogenic CO2 emissions scenarios. Science 349:aac4722. doi: 10.1126/science.aac4722
Gilbert, D., Rabalais, N. N., Díaz, R. J., and Zhang, J. (2010). Evidence for greater oxygen decline rates in the coastal ocean than in the open ocean. Biogeosciences 7, 2283–2296. doi: 10.5194/bg-7-2283-2010
Gillooly, J. F., Brown, J. H., West, G. B., Savage, V. M., and Charnov, E. L. (2001). Effects of size and temperature on metabolic rate. Science 293, 2248–2251. doi: 10.1126/science.1061967
Gledhill, M., Achterberg, E. P., Li, K., Mohamed, K. N., and Rijkenberg, M. J. A. (2015). Influence of ocean acidification on the complexation of iron and copper by organic ligands in estuarine waters. Mar. Chem. 177, 421–433. doi: 10.1016/j.marchem.2015.03.016
Grossart, H. P., Allgaier, M., Passow, U., and Riebesell, U. (2006). Testing the effect of CO2 concentration on the dynamics of marine heterotrophic bacterioplankton. Limnol. Oceanogr. 51, 1–11. doi: 10.4319/lo.2006.51.1.0001
Gruber, N. (2011). Warming up, turning sour, losing breath: ocean biogeochemistry under global change. Philos. Trans. R. Soc. A 369, 1980–1996. doi: 10.1098/rsta.2011.0003
Gruber, N., Clemen, D., Carter, B. R., Feely, R. A., Heuven, S. V., Hoppema, M., et al. (2019). The oceanic sink for anthropogenic CO2 from 1994 to 2007. Science 363, 1193–1199. doi: 10.1126/science.aau5153
Hansell, D. A. (2013). Recalcitrant dissolved organic carbon fractions. Ann. Rev. Mar. Sci. 5, 421–445. doi: 10.1146/annurev-marine-120710-100757
Hansell, D. A., Carlson, C. A., Bates, N. R., and Poisson, A. (1997). Horizontal and vertical removal of organic carbon in the equatorial Pacific Ocean: a mass balance assessment. Deep Sea Res. II 44, 2115–2130. doi: 10.1016/S0967-0645(97)00021-0
Hansell, D. A., Carlson, C. A., Repeta, D. J., and Schlitzer, R. (2009). Dissolved organic matter in the ocean: new insights stimulated by a controversy. Oceanography 22, 202–211. doi: 10.5670/oceanog.2009.109
Hansell, D. A., Carlson, C. A., and Schlitzer, R. (2012). Net removal of major marine dissolved organic carbon fractions in the subsurface ocean. Glob. Biogeochem. Cycles 26:GB1016. doi: 10.1029/2011GB004069
Hansell, D. A., Carlson, C. A., and Suzuki, S. (2002). Dissolved organic carbon export with North Pacific Intermediate Water formation. Glob. Biogeochem. Cycles 16, 77–84. doi: 10.1029/2000GB001361
Harrison, J. A., Caraco, N., and Seitzinger, S. P. (2005). Global patterns and sources of dissolved organic matter export to the coastal zone: results from a spatially explicit, global model. Glob. Biogeochem. Cycles 19:GB4S04. doi: 10.1029/2005GB002480
Harvey, B. P., Gwynn-Jones, D., and Moore, P. J. (2013). Meta-analysis reveals complex marine biological responses to the interactive effects of ocean acidification and warming. Ecol. Evol. 3, 1016–1030. doi: 10.1002/ece3.516
Hawkings, J. R., Wadham, J. L., Tranter, M., Raiswell, R., Benning, L. G., Statham, P. J., et al. (2014). Ice sheets as a significant source of highly reactive nanoparticulate iron to the oceans. Nat. Commun. 5:3929. doi: 10.1038/ncomms4929
Hedges, J. I. (2002). “Why Dissolved organics matter,” in Biogeochemistry of Marine Dissolved Organic Matter, eds D. Hansell and C. Carlson (New York, NY: Academic Press), 1–33. doi: 10.1016/B978-012323841-2/50003-8
Helm, K. P., Bindoff, N. L., and Church, J. A. (2011). Observed decreases in oxygen content of the global ocean. Geophys. Res. Lett. 38:L23602. doi: 10.1029/2011GL049513
Herman, J. R. (2010). Global increase in UV irradiance during the past 30 years (1979–2008) estimated from satellite data. Geophys. Res. Lett. 115:D04203. doi: 10.1029/2009JD012219
Hernes, P. J., and Benner, R. (2002). Transport and diagenesis of dissolved and particulate terrigenous organic matter in the North Pacific Ocean. Deep Sea Res. I 49, 2119–2132. doi: 10.1016/S0967-0637(02)00128-0
Hessen, D. O. (1998). “Food webs and carbon cycling in humic lakes,” in Aquatic Humic Substances, eds Hessen and Tranvik (Berlin: Springer-Verlag), 285–315. doi: 10.1007/978-3-662-03736-2_12
Hessen, D. O., Andersen, T., and Lyche, A. (1990). Carbon metabolism in a humic lake—Pool sizes and cycling through zooplankton. Limnol. Oceanogr. 35, 84–99. doi: 10.4319/lo.1990.35.1.0084
Hewson, I., O’neil, J. M., Fuhrman, J. A., and Dennison, W. C. (2001). Virus-like particle distribution and abundance in sediments and overlying waters along eutrophication gradients in two subtropical estuaries. Limnol. Oceanogr. 46, 1734–1746. doi: 10.4319/lo.2001.46.7.1734
Hodson, A., Anesio, A. M., Tranter, M., Fountain, A., Osborn, M., Priscu, J., et al. (2008). Glacial ecosystems. Ecol. Monogr. 78, 41–67. doi: 10.1890/07-0187.1
Hood, E., Battin, T. J., Fellman, J., O’neel, S., and Spencer, R. G. M. (2015). Storage and release of organic carbon from glaciers and ice sheets. Nat. Geosci. 8, 91–96. doi: 10.1038/ngeo2331
Hood, E., Fellman, J., Spencer, R. G. M., Hernes, P. J., Edwards, R., D’amore, D., et al. (2009). Glaciers as a source of ancient and labile organic matter to the marine environment. Nature 462, 1044–1047. doi: 10.1038/nature08580
Hopkins, F. E., Suntharalingam, P., Gehlen, M., Andrews, O., Archer, S. D., Bopp, L., et al. (2020). The impacts of ocean acidification on marine trace gases and the implications for atmospheric chemistry and climate. Proc. R. Soc. A. 476:20190769. doi: 10.1098/rspa.2019.0769
Hoppel, W. A., Frick, G. M., and Fitzgerald, J. W. (2002). Surface source function for sea-salt aerosol and aerosol dry deposition to the ocean surface. J. Geophys. Res. 107:4382. doi: 10.1029/2001JD002014
Hudson, J. J., Dillon, P. J., and Somers, K. M. (2003). Long-term patterns in dissolved organic carbon in boreal lakes: the role of incident radiation, precipitation, air temperature, southern oscillation and acid deposition. Hydrol. Earth Syst. Sci. 7, 390–398. doi: 10.5194/hess-7-390-2003
Huete-Stauffer, T. M., Arandia-Gorostidi, N., González-Benítez, N., Díaz-Pérez, L., Calvo-Díaz, A., and Morán, X. A. G. (2017). Large plankton enhance heterotrophy under experimental warming in a temperate coastal ecosystem. Ecosystems 21, 1139–1154. doi: 10.1007/s10021-017-0208-y
Hugelius, G., Strauss, J., Zubrzycki, S., Harden, J. W., Schuur, E. A. G., Ping, C.-L., et al. (2014). Estimated stocks of circumpolar permafrost carbon with quantified uncertaintyranges and identified data gaps. Biogeosciences 11, 6573–6593. doi: 10.5194/bg-11-6573-2014
Huntington, T. G. (2006). Evidence for intensification of the global water cycle: review and synthesis. J. Hydrol. 319, 83–95. doi: 10.1016/j.jhydrol.2005.07.003
Hutchins, D. A., and Fu, F. (2017). Microorganisms and ocean global change. Nat. Microbiol. 2:17058. doi: 10.1038/nmicrobiol.2017.58
Hygum, B. H., Petersen, J. W., and Søndergaard, M. (1997). Dissolved organic carbon released by zooplankton grazing activity- a high quality substrate pool for bacteria. J. Plankton Res. 19, 97–111. doi: 10.1093/plankt/19.1.97
IPCC (2013). “Clouds and aerosols,” in Climate Change 2013: The Physical Science Basis. Contribution of Working Group I to the Fifth Assessment Report of the Intergovernmental Panel on Climate Change, eds O. Boucher, D. Randall, P. Artaxo, C. Bretherton, G. Feingold, P. Forster, et al. (Cambridge: Cambridge University Press).
Ito, A., Myriokefalitakis, S., Kanakidou, M., Mahowald, N. M., Scanza, R. A., Hamilton, D. S., et al. (2019). Pyrogenic iron: the missing link to high iron solubility in aerosols. Sci. Adv. 5:eaau7671. doi: 10.1126/sciadv.aau7671
Iturriaga, R., and Zsolnay, A. (1981). Transformation of some dissolved organic compounds by a natural heterotrophic population. Mar. Biol. 62, 125–129. doi: 10.1007/BF00388174
Iuculano, F., Álvarez-Salgado, X. A., Otero, J., Catalá, T. S., Sobrino, C., Duarte, C. M., et al. (2019). Patterns and drivers of chromophoric dissolved organic matter in the open epipelagic ocean. Front. Mar. Sci. 6:320. doi: 10.3389/fmars.2019.00320
James, A. K., Kelly, L. W., Nelson, C. E., Wilbanks, E. G., and Carlson, C. A. (2019). Elevated pCO2 alters marine heterotrophic bacterial community composition and metabolic potential in response to a pulse of phytoplankton organic matter. Environ. Microbiol. 21, 541–555. doi: 10.1111/1462-2920.14484
James, A. K., Passow, U., Brzezinski, M. A., Parsons, R. J., Trapani, J. N., and Carlson, C. A. (2017). Elevated pCO2 enhances bacterioplankton removal of organic carbon. PLoS One 12:e0173145. doi: 10.1371/journal.pone.0173145
Jeffrey, W. H., Aas, P., Lyons, M. M., Coffin, R. B., Pledger, R. J., and Mitchell, D. L. (1996). Ambient solar radiation-induced photodamage in marine bacterioplankton. Photochem. Photobiol. 64, 419–427. doi: 10.1111/j.1751-1097.1996.tb03086.x
Jessen, G. L., Lichtschlag, A., Ramette, A., Pantoja, S., Rossel, P. E., Schubert, C. J., et al. (2017). Hypoxia causes preservation of labile organic matter and changes seafloor microbial community composition (Black Sea). Sci. Adv. 3:e1601897. doi: 10.1126/sciadv.1601897
Jiao, N., Herndl, G. J., Hansell, D. A., Benner, R., Kattner, G., Wilhelm, S. W., et al. (2010). Microbial production of recalcitrant dissolved organic matter: long-term carbon storage in the global ocean. Nat. Rev. Microbiol. 8, 593–599. doi: 10.1038/nrmicro2386
Jickells, T., and Moore, C. M. (2015). The importance of atmospheric deposition for ocean productivity. Annu. Rev. Ecol. Evol. Syst. 46, 481–501. doi: 10.1146/annurev-ecolsys-112414-054118
Jickells, T. D., An, Z. S., Anderson, K. K., Baker, A. R., Bergametti, G., Brooks, N., et al. (2005). Global iron connections between desert dust, ocean biogeochemistry and climate. Science 308, 65–71. doi: 10.1126/science.1105959
Jickells, T. D., Buitenhuis, E., Altieri, K., Baker, A. R., Capone, D., Duce, R. A., et al. (2017). A re-evaluation of the magnitude and impacts of anthropogenic nitrogen inputs on the ocean. Glob. Biogeochem. Cycles 31, 289–305. doi: 10.1002/2016GB005586
Joint, I., Doney, S. C., and Karl, D. M. (2010). Will ocean acidification affect marine microbes? ISME J. 5, 1–7. doi: 10.1038/ismej.2010.79
Jumars, P. A., Penry, D. L., Baross, J. A., and Perry, M. J. (1989). Closing the microbial loop: dissolved carbon pathway to heterotrophic bacteria from incomplete ingestion, digestion and absorption in animals. Deep Sea Res. 36, 483–495. doi: 10.1016/0198-0149(89)90001-0
Jurado, E., Dachs, J., Duarte, C. M., and Simo, R. (2008). Atmospheric deposition of organic and black carbon to the global oceans. Atmos. Environ. 42, 7931–7939. doi: 10.1016/j.atmosenv.2008.07.029
Karl, D. M., Hebel, D. V., Bjorkman, K., and Letelier, R. M. (1998). The role of dissolved organic matter release in the productivity of the oligotrophic north Pacific Ocean. Limnol. Oceanogr. 43, 1270–1286. doi: 10.4319/lo.1998.43.6.1270
Kattner, G., Simon, M., and Koch, B. P. (2011). “Molecular characterization of dissolved organic matter and constraints for prokaryotic utilization,” in Microbial Carbon Pump in the Ocean, eds N. Jiao, F. Azam, and S. Sansers (Washington, DC: Science/AAAS).
Kawasaki, N., and Benner, R. (2006). Bacterial release of dissolved organic matter during cell growth and decline: molecular origin and composition. Limnol. Oceanogr. 51, 2170–2180. doi: 10.4319/lo.2006.51.5.2170
Keeling, R. E., Kortzinger, A., and Gruber, N. (2010). Ocean deoxygenation in a warming world. Annu. Rev. Mar. Sci. 2, 199–229. doi: 10.1146/annurev.marine.010908.163855
Keil, R. G., and Mayer, L. M. (2014). “Mineral matrices and organic matter,” in Treatise on Geochemistry, 2nd Edn, eds H. Holland and K. Turekian (Oxford: Elsevier), 337–359. doi: 10.1016/B978-0-08-095975-7.01024-X
Kerner, M., Hohenberg, H., Ertl, S., Reckermann, M., and Spitzy, A. (2003). Self-organization of dissolved organic matter tomicelle-like microparticles in river water. Nature 422, 150–154. doi: 10.1038/nature01469
Kieber, D. J., Keene, W. C., Frossard, A. A., Long, M. S., Maben, J. R., Russell, L. M., et al. (2016). Coupled ocean-atmosphere loss of marine refractory dissolved organic carbon. Geophys. Res. Lett. 43, 2765–2772. doi: 10.1002/2016GL068273
Kieber, R. J., Hydro, L. H., and Seaton, P. J. (1997). Photooxidation of triglycerides and fatty acids in seawater: implication toward the formation of marine humic substances. Limnol. Oceanogr. 42, 1454–1462. doi: 10.4319/lo.1997.42.6.1454
Kinney, J. C., Maslowski, W., Aksenov, Y., Cuevas, B. D., Jakacki, J., Nguyen, A., et al. (2014). On the Flow Through Bering Strait: A Synthesis of Model Results and Observations. Dordrecht, NL: Springer.
Kirtman, B., Power, S. B., Adedoyin, J. A., Boer, G. J., Bojariu, R., Camilloni, I., et al. (2013). “Near-term climate change: projections and predictability,” in Climate Change 2013: The Physical Science Basis. Contribution of Working Group I to the Fifth Assessment Report of the Intergovernmental Panel on Climate Change, eds T. F. Stocker, D. Qin, G.-K. Plattner, M. Tignor, S. K. Allen, J. Boschung, et al. (Cambridge: Cambridge University Press).
Klug, J. L. (2002). Positive and negative effects of allochthonous dissolved organic matter and inorganic nutrients on phytoplankton growth. Can. J. Fish. Aquat. Sci. 59, 85–95. doi: 10.1139/f01-194
Knutson, T. R., Mcbride, J., Chan, J., Emanuel, K. A., Holland, G., Landsea, C., et al. (2010). Tropical cyclones and climate change. Nat. Geosci. 3, 157–163. doi: 10.1038/ngeo779
Komada, T., and Reimers, C. E. (2001). Resuspension-induced partitioning of organic carbon between solid and solution phases from a river–ocean transition. Mar. Chem. 76, 155–174. doi: 10.1016/S0304-4203(01)00055-X
Krishnamurthy, A., Moore, J. K., Mahowald, N., Luo, C., and Zender, C. S. (2010). Impacts of atmospheric nutrient inputs on marine biogeochemistry. J. Geophys. Res. 115:G01006. doi: 10.1029/2009JG001115
Kroeker, K. J., Kordas, R. L., Crim, R., Hendriks, I. E., Ramajo, L., Singh, G. S., et al. (2013). Impacts of ocean acidification on marine organisms: quantifying sensitivities and interaction with warming. Glob. Change Biol. 19, 1884–1896. doi: 10.1111/gcb.12179
Kwok, R. (2018). Arctic sea ice thickness, volume, and multiyear ice coverage: losses and coupled variability (1958–2018). Environ. Res. Lett. 13:105005. doi: 10.1088/1748-9326/aae3ec
Kyselı, J., Beguería, S., Beranová, R., Gaál, L., and López-Moreno, J. I. (2012). Different patterns of climate change scenarios for short-term and multi-day precipitation extremes in the Mediterranean. Glob. Planet. Change 98, 63–72. doi: 10.1016/j.gloplacha.2012.06.010
Lago, V., and England, M. H. (2019). Projected slowdown of antarctic bottom water formation in response to amplified Meltwater contributions. J. Clim. 32, 6319–6335. doi: 10.1175/JCLI-D-18-0622.1
Lampert, W. (1978). Release of dissolved organic carbon by grazing zooplankton. Limnol. Oceanogr. 23, 831–834. doi: 10.4319/lo.1978.23.4.0831
Lang, S. Q., Butterfield, D. A., Lilley, M. D., Paul Johnson, H., and Hedges, J. I. (2006). Dissolved organic carbon in ridge-axis and ridge-flank hydrothermal systems. Geochim. Cosmochim. Acta 70, 3830–3842. doi: 10.1016/j.gca.2006.04.031
Lawson, E. C., Wadham, J. L., Tranter, M., Stibal, M., Lis, G. P., Butler, C. E. H., et al. (2014). Greenland ice sheet exports labile organic carbon to the Arctic oceans. Biogeosciences 11, 4015–4028. doi: 10.5194/bg-11-4015-2014
Lee, C. (1992). Controls on organic carbon preservation: the use of stratified water bodies to compare intrinsic rates of decomposition in oxic and anoxic systems. Geochim. Cosmochim. Acta 56, 3323–3335. doi: 10.1016/0016-7037(92)90308-6
Legendre, L., Rivkin, R. B., Weinbauer, M. G., Guidi, L., and Uitz, J. (2015). The microbial carbon pump concept: potential biogeochemical significance in the globally changing ocean. Prog. Oceanogr. 134, 432–450. doi: 10.1016/j.pocean.2015.01.008
Lehmann, J., Coumou, D., and Frieler, K. (2015). Increased record-breaking precipitation events under global warming. Clim. Change 132, 501–515. doi: 10.1007/s10584-015-1434-y
Lehner, B., Liermann, C. R., Revenga, C., Vörösmarty, C., Fekete, B., Crouzet, P., et al. (2011). High-resolution mapping of the world’s reservoirs and dams for sustainable river-flow management. Front. Ecol. Environ. 9:494–502. doi: 10.1890/100125
Letscher, R. T., Hansell, D. A., Carlson, C. A., Lumpkin, R., and Knapp, A. N. (2013). Dissolved organic nitrogen in the global surface ocean: distribution and fate. Glob. Biogeochem. Cycles 27, 141–153. doi: 10.1029/2012GB004449
Lindsay, R., and Schweiger, A. (2015). Arctic sea ice thickness loss determined using subsurface, aircraft, and satellite observations. Cryosphere 9, 269–283. doi: 10.5194/tc-9-269-2015
Loginova, A. N., Thomsen, S., and Engel, A. (2016). Chromophoric and fluorescent dissolved organic matter in and above the oxygen minimum zone off Peru. J. Geophys. Res. Oceans 121, 7973–7990. doi: 10.1002/2016JC011906
Lønborg, C., and Álvarez-Salgado, X. A. (2012). Recycling versus export of bioavailable dissolved organic matter in the coastal ocean and efficiency of the continental shelf pump. Glob. Biogeochem. Cycles 26:GB3018. doi: 10.1029/2012GB004353
Lønborg, C., Álvarez-Salgado, X. A., Davidson, K., and Miller, A. E. J. (2009). Production of bioavailable and refractory dissolved organic matter by coastal heterotrophic microbial populations. Estuar. Coast. Shelf Sci. 82, 682–688. doi: 10.1016/j.ecss.2009.02.026
Lønborg, C., Álvarez–Salgado, X. A., Letscher, R. T., and Hansell, D. A. (2018). Large stimulation of recalcitrant dissolved organic carbon degradation by increasing ocean temperatures. Front. Mar. Sci. 4:436. doi: 10.3389/fmars.2017.00436
Lønborg, C., Baltar, F., Carreira, C., and Morán, X. A. G. (2019). Dissolved organic carbon source influences tropical coastal heterotrophic bacterioplankton response to experimental warming. Front. Microbiol. 10:2807. doi: 10.3389/fmicb.2019.02807
Lønborg, C., Cuevas, L. A., Reinthaler, T., Herndl, G. J., Gasol, J. M., Morán, X. A. G., et al. (2016). Depth dependent relationships between temperature and ocean heterotrophic prokaryotic production. Front. Mar. Sci. 3:90. doi: 10.3389/fmars.2016.00090
Lønborg, C., Middelboe, M., and Brussaard, C. P. D. (2013). Viral lysis of Micromonas pusilla: impacts on dissolved organic matter production and composition. Biogeochemistry 116, 231–240. doi: 10.1007/s10533-013-9853-1
Longnecker, K., and Kujawinski, E. B. (2011). Composition of dissolved organic matter in groundwater. Geochim. Cosmochim. Acta 75, 2752–2761. doi: 10.1016/j.gca.2011.02.020
Lovecchio, E., Gruber, N., and MuüNnich, M. (2018). Mesoscale contribution to the long-range offshore transport of organic carbon from the Canary Upwelling System to the open North Atlantic. Biogeosciences 15, 5061–5091. doi: 10.5194/bg-15-5061-2018
Maavara, T., Lauerwald, R., Regnier, P., and Cappellen, P. V. (2017). Global perturbation of organic carbon cycling by river damming. Nat. Commun. 8:15347. doi: 10.1038/ncomms15347
Mahowald, N., Lindsay, K., Rothenberg, D., Doney, S. C., Moore, J. K., Thornton, P., et al. (2011). Desert dust and anthropogenic aerosol interactions in the Community Climate System Model coupled-carbon-climate model. Biogeosciences 8, 387–414. doi: 10.5194/bg-8-387-2011
Mann, C. J., and Wetzel, R. G. (1996). Loading and utilization of dissolved organic carbon from emergent macrophytes. Aquat. Bot. 53, 61–72. doi: 10.1016/0304-3770(95)01012-2
Maranger, R., and Pullin, M. J. (2003). “Elemental complexation by dissolved organic matter in lakes: implications for Fe speciation and the bioavailability of Fe and P,” in Aquatic Ecosystems: Interactivity of Dissolved Organic Matter, eds S. E. G. Findlay and R. L. Sinsabaugh (San Diego, CA: Academic Press), 185–214. doi: 10.1016/B978-012256371-3/50009-3
Maßmig, M., Lüdke, J., Krahmann, G., and Engel, A. (2019a). High bacterial organic carbon uptake in the Eastern Tropical South Pacific oxygen minimum zone. Biogeosciences 17, 215–230. doi: 10.5194/bg-17-215-2020
Maßmig, M., Piontek, J., Moigne, F. A. C. L., Cisternas-Novoa, C., and Engel, A. (2019b). Potential role of oxygen and inorganic nutrients on microbial carbon turnover in the Baltic Sea. Aquat. Microb. Ecol. 83, 95–108. doi: 10.3354/ame01902
McCarthy, M., Pratum, T., Hedges, J., and Benner, R. (1997). Chemical composition of dissolved organic nitrogen in the ocean. Nature 390, 150–154. doi: 10.1038/36535
McInnes, K. L., Erwin, T. A., and Bathols, J. M. (2011). Global Climate Model projected changes in 10 m wind speed and direction due to anthropogenic climate change. Atmos. Sci. Lett. 12, 325–333. doi: 10.1002/asl.341
Meehl, G. A., Arblaster, J. M., Fasullo, J. T., Hu, A., and Trenberth, K. E. (2011). Model-based evidence of deep-ocean heat uptake during surface-temperature hiatus periods. Nat. Clim. Change 1, 360–364. doi: 10.1038/nclimate1229
Middelburg, J. J. (2019). Marine Carbon Biogeochemistry: A Primer for Earth System Scientists. Cham: Springer Open. doi: 10.1007/978-3-030-10822-9
Middelburg, J. J., Vlug, T., Jaco, F., and van der Nat, W. A. (1993). Organic matter mineralization in marine systems. Glob. Planet. Change 8, 47–58. doi: 10.1016/0921-8181(93)90062-S
Miller, W. L., and Moran, M. A. (1997). Interaction of photochemical and microbial processes in the degradation of refractory dissolved organic matter from a coastal marine environment. Limnol. Oceanogr. 42, 1317–1324. doi: 10.4319/lo.1997.42.6.1317
Miller, W. L., Moran, M. A., Sheldon, W. M., Zepp, R. G., and Opsahl, S. (2002). Determination of apparent quantum yield spectra for the formation of biologically labile photoproducts. Limnol. Oceanogr. 47, 343–352. doi: 10.4319/lo.2002.47.2.0343
Miller, W. L., and Zepp, R. G. (1995). Photochemical production of dissolved inorganic carbon from terrestrial organic matter: significance of the oceanic organic carbon cycle. Geophys. Res. Lett. 22, 417–420. doi: 10.1029/94GL03344
Milner, A. M., Khamis, K., Battin, T. J., Brittain, J. E., Barranda, N. E., Füreder, L., et al. (2017). Glacier shrinkage driving global changes in downstream systems. Proc. Natl. Acad. Sci. U.S.A. 114, 9770–9778. doi: 10.1073/pnas.1619807114
Monteith, D. T., Stoddard, J. L., Evans, C. D., De Wit, H. A., Forsius, M., Høgåsen, T., et al. (2007). Dissolved organic carbon trends resulting from changes in atmospheric deposition chemistry. Nature 450, 537–540. doi: 10.1038/nature06316
Mopper, K., Kieber, D. J., and Stubbins, A. (2015). “Marine photochemistry of organic matter,” in Biogeochemistry of Marine Dissolved Organic Matter, eds C. A. Carlson and D. A. Hansell (Amsterdam: Elsevier), 389–450. doi: 10.1016/B978-0-12-405940-5.00008-X
Moran, M. A., and Hodson, R. E. (1994). Support of bacterioplankton production by dissolved humic substances from three marine enviroments. Mar. Ecol. Prog. Ser. 110, 241–247. doi: 10.3354/meps110241
Moran, M. A., Sheldon, W. M., and Zepp, R. G. (2000). Carbon loss and optical property changes during long-term photochemical and biological degradation of estuarine dissolved organic matter. Limnol. Oceanogr. 45, 1254–1264. doi: 10.4319/lo.2000.45.6.1254
Moran, M. A., and Zepp, R. G. (1997). Role of photoreactions in the formation of biologically labile compounds from dissolved organic matter. Limnol. Oceanogr. 42, 1307–1316. doi: 10.4319/lo.1997.42.6.1307
Morán, X. A. G., Gasol, J. M., Pernice, M. C., Mangot, J.-F., Massana, R., Lara, E., et al. (2017). Temperature regulation of marine heterotrophic prokaryotes increases latitudinally as a breach between bottom-up and top-down controls. Glob. Change Biol. 23, 3956–3964. doi: 10.1111/gcb.13730
Morán, X. A. G., Lopez-Urrutia, A., Calvo-Diaz, A., and Li, W. K. W. (2010). Increasing importance of small phytoplankton in a warmer ocean. Glob. Change Biol. 16, 1137–1144. doi: 10.1111/j.1365-2486.2009.01960.x
Morel, F. M. M., and Price, N. M. (2003). The biogeochemical cycles of trace metals in the oceans. Science 300, 944–947. doi: 10.1126/science.1083545
Mulholland, P. J. (1981). Formation of Particulate Organic Carbon in Water from a Southeastern Swamp-Stream. Limnol. Oceanogr. 26, 790–795. doi: 10.4319/lo.1981.26.4.0790
Mulholland, P. J. (2003). “Large-scale patterns in dissolved organic carbon concentration, flux, and sources,” in Aquatic Ecosystems Interactivity of Dissolved Organic Matter, eds S. E. G. Findlay and R. L. Sinsabaugh (New York, NY: Academic Press), 139–159. doi: 10.1016/B978-012256371-3/50007-X
Musilova, M., Tranter, M., Wadham, J., Telling, J., Tedstone, A., and Anesio, A. M. (2017). Microbially driven export of labile organic carbon from the Greenland ice sheet. Nat. Geosci. 10, 360–365. doi: 10.1038/ngeo2920
Myers-Pigg, A. N., Louchouarn, P., Amon, R. M. W., Prokushkin, A., Pierce, K., and Rubtsov, A. (2015). Labile pyrogenic dissolved organic carbon in major Siberian Arctic rivers: implications for wildfire-stream metabolic linkages. Geophys. Res. Lett. 42, 377–385. doi: 10.1002/2014GL062762
Neale, P., and Smyth, R. (2018). “Ares warmer waters brighter waters: an examination of the irradiance environment of lakes and oceans in a changing climate,” in Aquatic Ecosystems in a Changing Climate, ed. D.-P. Hader (Boca Raton, FL: CRC Press), 89–115. doi: 10.1201/9780429436130-6
Nelson, N. B., Siegel, D. A., and Michaels, A. F. (1998). Seasonal dynamics of colored dissolved material in the Sargasso Sea. Deep Sea Res. 45, 931–957. doi: 10.1016/S0967-0637(97)00106-4
Neubacher, E. C., Parker, R. W., and Trimmer, M. (2011). Short-term hypoxia alters the balance of the nitrogen cycling in costal sediments. Limnol. Oceanogr. 56, 651–665. doi: 10.4319/lo.2011.56.2.0651
Norman, L., Thomas, D. N., Stedmon, C. A., Granskog, M. A., Papadimitriou, S., Krapp, R. H., et al. (2011). The characteristics of dissolved organic matter (DOM) and chromophoric dissolved organic matter (CDOM) in Antarctic sea ice. Deep Sea Res. II 58, 1075–1091. doi: 10.1016/j.dsr2.2010.10.030
Norris, J. R., Allen, R. J., Evan, A. T., Zelinka, M. D., O’dell, C. W., and Klein, S. A. (2016). Evidence for climate change in the satellite cloud record. Nature 536, 72–75. doi: 10.1038/nature18273
Notz, D., and Stroeve, J. (2016). Observed Arctic sea-ice loss directly follows anthropogenic CO2 emission. Science 354, 747–750. doi: 10.1126/science.aag2345
Obernosterer, I., and Herndl, G. J. (1995). Phytoplankton extracellular release and bacterial growth: dependence on the inorganic N:P ratio. Mar. Ecol. Prog. Ser. 116, 247–257. doi: 10.3354/meps116247
Ogawa, H., Amagai, Y., Koike, I., Kaiser, K., and Benner, R. (2001). Production of refractory dissolved organic matter by bacteria. Science 292, 917–920. doi: 10.1126/science.1057627
Opsahl, S., Benner, R., and Amon, R. (1999). Major flux of terrigenous organic matter through the Arctic Ocean. Limnol. Oceanogr. 44, 2017–2023. doi: 10.4319/lo.1999.44.8.2017
Osburn, C. L., Retamal, L., and Vincent, W. F. (2009). Photoreactivity of chromophoric dissolved organic matter transported by the Mackenzie River to the Beaufort Sea. Mar. Chem. 115, 10–20. doi: 10.1016/j.marchem.2009.05.003
Osburn, C. L., Rudolph, J. C., Paerl, H. W., Hounshell, A. G., and Dam, B. R. V. (2019). Lingering carbon cycle effects of Hurricane Matthew in North Carolina’s coastal waters. Geophys. Res. Lett. 46, 2654–2661. doi: 10.1029/2019GL082014
Paerl, H. W., Hall, N. S., Hounshell, A. G. Jr., Luettich, R. A., Rossignol, K. L., et al. (2019). Recent increase in catastrophic tropical cyclone flooding in coastal North Carolina, USA: long-term observations suggest a regime shift. Sci. Rep. 9:10620. doi: 10.1038/s41598-019-46928-9
Pantoja, S., Rossel, P., Castro, R., Cuevas, L. A., Daneri, G., and Córdova, L. (2009). Microbial degradation rates of small peptides and amino acids in the oxygen minimum zones of Chilean coastal waters. Deep Sea Res. II 56, 1055–1062. doi: 10.1016/j.dsr2.2008.09.007
Pautler, B. G., Dubnick, A., Sharp, M. J., Simpson, A. J., and Simpson, M. J. (2013). Comparison of cryoconite organic matter composition from Arctic and Antarctic glaciers at the molecular-level. Geochim. Cosmochim. Acta 104, 1–18. doi: 10.1016/j.gca.2012.11.029
Peltier, W. R., Liu, Y., and Crowley, J. W. (2007). Snowball Earth prevention by dissolved organic carbon remineralization. Nature 450, 813–818. doi: 10.1038/nature06354
Penhale, P. A., and Smith, W. O. (1977). Excretion of dissolved organic carbon by eelgrass (Zostera marina) and its epiphytes. Limnol. Oceanogr. 22, 400–407. doi: 10.4319/lo.1977.22.3.0400
Piontek, J., Borchard, C., Sperling, M., Schulz, K. G., Riebesell, U., and Engel, A. (2013). Response of bacterioplankton activity in an Arctic fjord system to elevated pCO2: results from a mesocosm perturbation study. Biogeosciences 10, 297–314. doi: 10.5194/bg-10-297-2013
Piontek, J., Lunau, M., Händel, N., Borchard, C., Wurst, M., and Engel, A. (2010). Acidification increases mmicrobial polysaccharide degradation in the ocean. Biogeosciences 7, 1615–1624. doi: 10.5194/bg-7-1615-2010
Polovina, J. J., Howell, E. A., and Abecassis, M. (2008). Ocean’s least productive waters are expanding. Geophys. Res. Lett. 35:L03618. doi: 10.1029/2007GL031745
Porcal, P., Dillon, P. J., and Molot, L. A. (2015). Temperature dependence of photodegradation of dissolved organic matter to dissolved inorganic carbon and particulate organic carbon. PLoS One 10:e0128884. doi: 10.1371/journal.pone.0128884
Powell, R. T., Landing, W. M., and Bauer, J. E. (1996). Colloidal trace metals, organic carbon and nitrogen in a southeastern U.S. estuary. Mar. Chem. 55, 165–176. doi: 10.1016/S0304-4203(96)00054-0
Prăvălie, R. (2016). Drylands extent and environmental issues: a global approach. Earth Sci. Rev. 161, 259–278. doi: 10.1016/j.earscirev.2016.08.003
Pregnall, A. M. (1983). Release of dissolved organic carbon from the estuarine intertidal macroalga Enteromorpha prolifera. Mar. Biol. 73, 37–42. doi: 10.1007/BF00396283
Prein, A. F., Rasmussen, R. M., Ikeda, K., Liu, C., Clark, M. P., and Holland, G. J. (2017). The future intensification of hourly precipitation extremes. Nat. Clim. Change 7, 48–52. doi: 10.1038/nclimate3168
Qualls, R. G., and Richardson, C. J. (2003). Factors controlling concentration, export, and decomposition of dissolved organic nutrients in the Everglades of Florida. Biogeochemistry 62, 197–229. doi: 10.1023/A:1021150503664
Rabalais, N. N., Turner, R. E., Díaz, R. J., and Justić, D. (2009). Global change and eutrophication of coastal waters. ICES J. Mar. Sci. 66, 1528–1537. doi: 10.1093/icesjms/fsp047
Radić, V., and Hock, R. (2011). Regionally differentiated contribution of mountain glaciers and ice caps to future sea-level rise. Nat. Geosci. 4, 91–94. doi: 10.1038/ngeo1052
Ravichandran, M. (2004). Interactions between mercury and dissolved organic matter – A review. Chemosphere 55, 319–331. doi: 10.1016/j.chemosphere.2003.11.011
Raymond, P. A., Saiers, J. E., and Sobczak, W. V. (2016). Hydrological and biogeochemical controls on watershed dissolved organic matter transport: pulse- shunt concept. Ecology 97, 5–16. doi: 10.1890/14-1684.1
Raymond, P. A., and Spencer, R. G. M. (2015). “Riverine DOM,” in Biogeochemistry of Marine Dissolved Organic Matter, eds D. A. Hansell and C. A. Carlson (Amsterdam: Elsevier), 509–533. doi: 10.1016/B978-0-12-405940-5.00011-X
Retamal, L., Vincent, W. F., Martineau, C., and Osburn, C. L. (2007). Comparison of the optical properties of dissolved organic matter in two river-influenced coastal regions of the Canadian Arctic. Estuar. Coast. Shelf Sci. 72, 261–272. doi: 10.1016/j.ecss.2006.10.022
Rhode, S. C., Pawlowski, M., and Tollrian, R. (2001). The impact of ultraviolet radiation on the vertical distribution of zooplankton of the genus Daphnia. Nature 412, 69–72. doi: 10.1038/35083567
Robinson, C. (2019). Microbial respiration, the engine of ocean deoxygenation. Front. Mar. Sci. 5:533. doi: 10.3389/fmars.2018.00533
Romera-Castillo, C., and Jaffé, R. (2015). Free radical scavenging (antioxidant activity) of natural dissolved organic matter. Mar. Chem. 177, 668–676. doi: 10.1016/j.marchem.2015.10.008
Romera-Castillo, C., Letscher, R. T., and Hansell, D. A. (2016). New nutrients exert fundamental control on dissolved organic carbon accumulation in the surface Atlantic Ocean. Proc. Natl. Acad. Sci. U.S.A. 113, 10497–10502. doi: 10.1073/pnas.1605344113
RoyalSociety (2005). Ocean Acidification Due to Increasing Atmospheric Carbon Dioxide. London: The Royal Society.
Ruiz-Halpern, S., Sejr, M. K., Duarte, C. M., Krause-Jensen, D., Dalsgaard, T., Dachs, J., et al. (2010). Air–water exchange and vertical profiles of organic carbon in a subarctic fjord. Limnol. Oceanogr. 55, 1733–1740. doi: 10.4319/lo.2010.55.4.1733
Rykaczewski, R. R., and Dunne, J. P. (2010). Enhanced nutrient supply to the California Current Ecosystem with global warming and increased stratification in an Earth system model. Geophys. Res. Lett. 37:L21606. doi: 10.1029/2010GL045019
Rykaczewski, R. R., Dunne, J. P., Sydeman, W. J., García-Reyes, M., Black, B. A., and Bograd, S. J. (2015). Poleward displacement of coastal upwelling-favorable winds in the ocean’s eastern boundary currents through the 21st century. Geophys. Res. Lett. 42, 6424–6431. doi: 10.1002/2015GL064694
Saad, E. M., Longo, A. F., Chambers, L. R., Huang, R., Benitez-Nelson, C., Dyhrman, S. T., et al. (2016). Understanding marine dissolved organic matter production: compositional insights from axenic cultures of Thalassiosira pseudonana. Limnol. Oceanogr. 61, 2222–2233. doi: 10.1002/lno.10367
Sabine, C. L., Feely, R. A., Gruber, N., Key, R. M., Lee, K., Bullister, J. L., et al. (2004). The oceanic sink for anthropogenic CO2. Science 305, 367–371. doi: 10.1126/science.1097403
Sarmiento, J. L., Slater, R., Barber, R., Bopp, L., Doney, S. C., Hirst, A. C., et al. (2004). Response of ocean ecosystems to climate warming. Glob. Biogeochem. Cycles 18:GB3003. doi: 10.1029/2003GB002134
Schmidtko, S., Stramma, L., and Visbeck, M. (2017). Decline in global oceanic oxygen content during the past five decades. Nature 542, 335–339. doi: 10.1038/nature21399
Scholz, F., Mcmanus, J., Mix, A. C., Hensen, C., and Schneider, R. R. (2014). The impact of ocean deoxygenation on iron release from continental margin sediments. Nat. Geosci. 7, 433–437. doi: 10.1038/ngeo2162
Seitzinger, S. P., Mayorga, E., Bouwman, A. F., Kroeze, C., Beusen, A. H. W., Billen, G., et al. (2010). Global river nutrient export: a scenario analysis of past and future trends. Glob. Biogeochem. Cycles 24:GB0A08. doi: 10.1029/2009GB003587
Sexton, P. F., Norris, R. D., Wilson, P. A., Pälike, H., Westerhold, T., Röhl, U., et al. (2011). Eocene global warming events driven by ventilation of oceanic dissolved organic carbon. Nature 471, 349–353. doi: 10.1038/nature09826
Sharples, J., Middelburg, J. J., Fennel, K., and Jickells, T. D. (2016). What proportion of riverine nutrients reaches the open ocean? Glob. Biogeochem. Cycles 31, 39–58. doi: 10.1002/2016GB005483
Sholkovitz, E. R. (1976). Flocculation of dissolved organic and inorganic matter during the mixing of river water and seawater. Geochim. Cosmochim. Acta 40, 831–845. doi: 10.1016/0016-7037(76)90035-1
Sholkovitz, E. R., Boyle, E. A., and Price, N. B. (1978). The removal of dissolved humic acids and iron during estuarine mixing. Earth Planet. Sci. Lett. 40, 130–136. doi: 10.1016/0012-821X(78)90082-1
Sierra, C. A. (2011). Temperature sensitivity of organic matter decomposition in the Arrhenius equation: some theoretical considerations. Biogeochemistry 108, 1–15. doi: 10.1007/s10533-011-9596-9
Smale, D. A., Wernberg, T., Oliver, E. C. J., Thomsen, M., Harvey, B. P., Straub, S. C., et al. (2019). Marine heatwaves threaten global biodiversity and the provision of ecosystem services. Nat. Clim. Change 9, 306–312. doi: 10.1038/s41558-019-0412-1
Smith, H. J., Foster, R. A., Mcknight, D. M., Lisle, J. T., Littmann, S., Kuypers, M. M. M., et al. (2017). Microbial formation of labile organic carbon in Antarctic glacial environments. Nat. Geosci. 10, 356–359. doi: 10.1038/ngeo2925
Solomon, C. T., Jones, S. E., Weidel, B. C., Buffam, I., Fork, M. L., Karlsson, J., et al. (2015). Ecosystem consequences of changing inputs of terrestrial dissolved organic matter to lakes: current knowledge and future challenges. Ecosystems 18, 376–389. doi: 10.1007/s10021-015-9848-y
Somavilla, R., Gonzalez-Pola, C., and Fernández, J. (2017). The warmer the ocean surface, the shallower the mixed layer: How much of this is true? J. Geophys. Res. Oceans 122, 7698–7716. doi: 10.1002/2017JC013125
Sommer, U., and Lengfellner, K. (2008). Climate change and the timing, magnitude, and composition of the phytoplankton spring bloom. Glob. Change Biol. 14, 1199–1208. doi: 10.1111/j.1365-2486.2008.01571.x
Sousa, M. G., Ribeiro, A., Des, M., Gomez-Gesteira, M., De Castro, M., and Dias, J. M. (2020). NW Iberian Peninsula coastal upwelling future weakening: competition between wind intensification and surface heating. Sci. Total Environ. 703:134808. doi: 10.1016/j.scitotenv.2019.134808
Stammerjohn, S., and Maksym, T. (2017). “Gaining (and losing) Antarctic sea ice: variability, trends and mechanisms,” in Sea Ice, 3rd Edn, ed. D. Thomas (Oxford: Wiley-Blackwell), 261–289. doi: 10.1002/9781118778371.ch10
Stubbins, A., Hood, E., Raymond, P. A., Aiken, G. R., Sleighter, R. L., Hernes, P. J., et al. (2012). Anthropogenic aerosols as a source of ancient dissolved organic matter in glaciers. Nat. Geosci. 5, 198–201. doi: 10.1038/ngeo1403
Stubbins, A., Uher, G., Law, C. S., Mopper, K., Robinson, C., and Upstill-Goddard, R. C. (2006). Open-ocean carbon monoxide photoproduction. Deep Sea Res. II Top. Stud. Oceanogr. 53, 1695–1705. doi: 10.1016/j.dsr2.2006.05.011
Sulzberger, B., and Durisch-Kaiser, E. (2009). Chemical characterization of dissolved organic matter (DOM): a prerequisite for understanding UV-induced changes of DOM absorption properties and bioavailability. Aquat. Sci. 71, 104–126. doi: 10.1007/s00027-008-8082-5
Swanson-Hysell, N. L., Rose, C. V., Calmet, C. C., Halverson, G. P., Hurtgen, M. T., and Maloof, A. (2010). Cryogenian glaciation and the onset of carbon-isotope decoupling. Science 328, 608–611. doi: 10.1126/science.1184508
Sweeney, C., Hansell, D. A., Carlson, C. A., Codispoti, L. A., Gordon, L. I., Marra, J., et al. (2000). Biogeochemical regimes, net community production and carbon export in the Ross Sea, Antarctica. Deep Sea Res. II 47, 3369–3394. doi: 10.1016/S0967-0645(00)00072-2
Sydeman, W. J., Garcia-Reyes, M., Schoeman, D. S., Rykaczewski, R. R., Thompson, S. A., Black, B. A., et al. (2014). Climate change and wind intensification in coastal upwelling ecosystems. Science 345, 77–80. doi: 10.1126/science.1251635
Tagliabue, A., Bowie, A. R., Boyd, P. W., Buck, K. N., Johnson, K. S., and Saito, M. A. (2017). The integral role of iron in ocean biogeochemistry. Nature 543, 51–59. doi: 10.1038/nature21058
Tanentzap, A. J., Szkokan-Emilson, E. J., Kielstra, B. W., Arts, M. T., Yan, N. D., and Gunn, J. M. (2014). Forests fuel fish growth in freshwater deltas. Nat. Commun. 5:4077. doi: 10.1038/ncomms5077
Tarnocai, C., Canadell, J. G., Schuur, E. A. G., Kuhry, P., Mazhitova, G., and Zimov, S. (2009). Soil organic carbon pools in the northern circumpolar permafrost region. Glob. Biogeochem. Cycles 23:GB2023. doi: 10.1029/2008GB003327
Teira, E., Fernández, A., Álvarez-Salgado, X. A., García-Martín, E. E., Serret, P., and Sobrino, C. (2012). Response of two marine bacterial isolates to high CO2 concentration. Mar. Ecol. Prog. Ser. 453, 27–36. doi: 10.3354/meps09644
Terada, M., Minobe, S., and Deutsche, C. (2020). Mechanisms of future changes in equatorial upwelling: CMIP5 intermodel analysis. J. Clim. 33, 497–510. doi: 10.1175/JCLI-D-19-0128.1
Thingstad, T. F., Hagström, Å., and Rassoulzadegan, F. (1997). Accumulation of degradable DOC in surface waters: Is it caused by a malfunctioning microbial loop? Limnol. Oceanogr. 42, 398–404. doi: 10.4319/lo.1997.42.2.0398
Thingstad, T. F., Havskum, H., Kaas, H., Nielsen, T. G., Riemann, B., Lefevre, D., et al. (1999). Bacteria-protist interactions and organic matter degradation under P-limited conditions: analysis of an enclosure experiment using a simple model. Limnol. Oceanogr. 44, 62–79. doi: 10.4319/lo.1999.44.1.0062
Thornton, D. C. O. (2014). Dissolved organic matter (DOM) release by phytoplankton in the contemporary and future ocean. Eur. J. Phycol. 49, 20–46. doi: 10.1080/09670262.2013.875596
Timko, S. A., Gonsior, M., and Cooper, W. J. (2015). Influence of pH on fluorescent dissolved organic matter photo-degradation. Water Res. 85, 266–274. doi: 10.1016/j.watres.2015.08.047
Tranvik, L. J., and Sieburth, J. M. (1989). Effects of flocculated humic matter on free and attached pelagic microorganisms. Limnol. Oceanogr. 34, 688–699. doi: 10.4319/lo.1989.34.4.0688
Vahatalo, A. V., Aarnos, H., and Mantyniemi, S. (2010). Biodegradability continuum and biodegradation kinetics of natural organic matter described by the beta distribution. Biogeochemistry 100, 227–240. doi: 10.1007/s10533-010-9419-4
Vancoppenolle, M., Meiner, K. M., Michel, C., Bopp, L., Brabant, F., Carnat, G., et al. (2013). Role of sea ice in global biogeochemical cycles: emerging views and challenges. Q. Sci. Rev. 79, 207–230. doi: 10.1016/j.quascirev.2013.04.011
Varela, R., Lima, F. P., Seabra, R., Meneghesso, C., and Gómez-Gesteira, M. (2018). Coastal warming and wind-driven upwelling: a global analysis. Sci. Total Environ. 639, 1501–1511. doi: 10.1016/j.scitotenv.2018.05.273
Verdugo, P. (2012). Marine microgels. Annu. Rev. Mar. Sci. 4, 375–400. doi: 10.1146/annurev-marine-120709-142759
Volk, C., Bell, K., Ibrahim, E., Verges, D., Amy, G., and Lechevallier, M. (2000). Impact of enhanced and optimized coagulation on removal of organic matter and its biodegradable fraction in drinking water. Water Res. 34, 3247–3257. doi: 10.1016/S0043-1354(00)00033-6
Wada, S., Aoki, M. N., Tsuchiya, Y., Sato, T., Shinagawa, H., and Hama, T. (2007). Quantitative and qualitative analyses of dissolved organic matter released from Ecklonia cava Kjellman, in Oura Bay, Shimoda, Izu Peninsula, Japan. J. Exp. Mar. Biol. Ecol. 349, 344–358. doi: 10.1016/j.jembe.2007.05.024
Wang, D., Gouhier, T. C., Menge, B. A., and Ganguly, A. R. (2015). Intensification and spatial homogenization of coastal upwelling under climate change. Nature 518, 390–394. doi: 10.1038/nature14235
Wang, X.-C., Chen, R. F., and Gardner, G. B. (2004). Sources and transport of dissolved and particulate organic carbon in the Mississippi River estuary and adjacent coastal waters of the northern Gulf of Mexico. Mar. Chem. 89, 241–256. doi: 10.1016/j.marchem.2004.02.014
Ward, N. D., Keil, R. G., Medeiros, P. M., Brito, D. C., Cunha, A. C., Dittmar, T., et al. (2013). Degradation of terrestrially derived macromolecules in the Amazon River. Nat. Geosci. 6, 530–533. doi: 10.1038/ngeo1817
Watanabe, S., Sudo, K., Nagashima, T., Takemura, T., Kawase, H., and Nozawa, T. (2011). Future projections of surface UV-B in a changing climate. J. Geophys. Res. 116:D16118. doi: 10.1029/2011JD015749
Watson, A. J., Lendon, T. M., and Mills, B. J. W. (2018). Ocean deoxygenation, the global phosphorus cycle, and the possibility of human-caused large-scale ocean anoxia. Philos. Trans. A Math. Phys. Eng. Sci. 375:20160318. doi: 10.1098/rsta.2016.0318
Webb, J. R., Santos, I. R., Maher, D. T., Tait, D. R., Cyronak, T., Sadat-Noori, M., et al. (2019). Groundwater as a source of dissolved organic matter to coastal waters: insights from radon and CDOM observations in 12 shallow coastal systems. Limnol. Oceanogr. 64, 182–196. doi: 10.1002/lno.11028
Weinbauer, M. A. G. (2004). Ecology of prokaryotic viruses. FEMS Microbiol. Rev. 28, 127–181. doi: 10.1016/j.femsre.2003.08.001
Weiss, M. S., Abele, U., Weckesser, J., Welte, W., Schiltz, E., and Schulz, G. E. (1991). Molecular architecture and electrostatic properties of a bacterial porin. Science 254, 1627–1630. doi: 10.1126/science.1721242
West, L. J. A., Greenberg, B. M., and Smith, R. E. H. (1999). Ultraviolet radiation effects on a microscopic green alga and the protective effects of natural dissolved organic matter. Photochem. Photobiol. 69, 536–544. doi: 10.1111/j.1751-1097.1999.tb03324.x
Westrich, J. T., and Berner, R. A. (1984). The role of sedimentary organic matter in bacterial sulfate reduction: the G model tested. Limnol. Oceanogr. 29, 236–249. doi: 10.4319/lo.1984.29.2.0236
Wetz, M. S., and Wheeler, P. A. (2007). Release of dissolved organic matter by coastal diatoms. Limnol. Oceanogr. 52, 798–807. doi: 10.4319/lo.2007.52.2.0798
Whitney, F. A., Freeland, H. J., and Robert, M. (2007). Persistently declining oxygen levels in the interior waters of the eastern subarctic Pacific. Prog. Oceanogr. 75, 179–199. doi: 10.1016/j.pocean.2007.08.007
Willey, J. D., Kieber, R. J., Eyman, M. S. Jr., and Brooks Avery, G. (2000). Rainwater dissolved organic carbon concentrations and global flux. Glob. Biogeochem. Cycles 14, 139–148. doi: 10.1029/1999GB900036
Williamson, C. E., Overholt, E. P., Pilla, R. M., Leach, T. H., Brentrup, J. A., Knoll, L. B., et al. (2015). Ecological consequences of longterm browning in lakes. Sci. Rep. 5:18666. doi: 10.1038/srep18666
Williamson, C. E., Stemberger, R. S., Morris, D. P., Frost, T. A., and Paulsen, S. G. (1996). Ultraviolet radiation in North American lakes: attenuation estimates from DOC measurements and implications for plankton communities. Limnol. Oceanogr. 41, 1024–1034. doi: 10.4319/lo.1996.41.5.1024
Wright, J. J., Konwar, K. M., and Hallam, S. J. (2012). Microbial ecology of expanding oxygen minimum zones. Nat. Rev. Microbiol. 10, 381–394. doi: 10.1038/nrmicro2778
Wu, K., Dai, M., Chen, J., Meng, F., Li, X., Liu, Z., et al. (2015). Dissolved organic carbon in the South China Sea and its exchange with the Western Pacific Ocean. Deep Sea Res. II Top. Stud. Oceanogr. 122, 41–51. doi: 10.1016/j.dsr2.2015.06.013
Yates, C., Johnes, P., Owen, A., Brailsford, F., Glanville, H., Evans, C., et al. (2019). Variation in dissolved organic matter (DOM) stoichiometry in UK freshwaters: assessing the influence of land cover and soil C:N ratio on DOM composition. Limnol. Oceanogr. 64, 2328–2340. doi: 10.1002/lno.11186
Yool, A., Martin, A. P., Anderson, T. R., Bett, B. J., Jones, D. O., and Ruhl, H. A. (2017). Big in the benthos: future change of seafloor community biomass in a global, body size-resolved model. Glob. Change Biol. 23, 3554–3566. doi: 10.1111/gcb.13680
Yool, A., Popova, E. E., Coward, A. C., Bernie, D., and Anderson, T. R. (2013). Climate change and ocean acidification impacts on lower trophic levels and the export of organic carbon to the deep ocean. Biogeosciences 10, 5831–5854. doi: 10.5194/bg-10-5831-2013
Yoon, B., and Raymond, P. A. (2012). Dissolved organic matter export from a forested watershed during Hurricane Irene. Geophys. Res. Lett. 39:L18402. doi: 10.1029/2012GL052785
Zarfl, C., Lumsdon, A., Berlekamp, J., Tydecks, L., and Tockner, K. (2015). A global boom in hydropower dam construction. Aquat. Sci. 77, 161–170. doi: 10.1007/s00027-014-0377-0
Zark, M., Broda, N. K., Hornick, T., Grossart, H.-P., Riebesell, U., and Dittmar, T. (2017). Ocean acidification experiments in large-scale mesocosms reveal similar dynamics of dissolved organic matter production and biotransformation. Front. Mar. Sci. 4:271. doi: 10.3389/fmars.2017.00271
Zark, M., Riebesell, U., and Dittmar, T. (2015). Effects of ocean acidification on marine dissolved organic matter are not detectable over the succession of phytoplankton blooms. Sci. Adv. 1:e1500531. doi: 10.1126/sciadv.1500531
Keywords: dissolved organic carbon, ocean warming and stratification, acidification, deoxygenation, glaciers and sea ice, rivers, ocean currents, atmospheric deposition
Citation: Lønborg C, Carreira C, Jickells T and Álvarez-Salgado XA (2020) Impacts of Global Change on Ocean Dissolved Organic Carbon (DOC) Cycling. Front. Mar. Sci. 7:466. doi: 10.3389/fmars.2020.00466
Received: 23 March 2020; Accepted: 26 May 2020;
Published: 23 June 2020.
Edited by:
Jianfang Chen, Second Institute of Oceanography, Ministry of Natural Resources, ChinaReviewed by:
Liyang Yang, Fuzhou University, ChinaKai Tang, Xiamen University, China
Dennis Arthur Hansell, University of Miami, United States
Copyright © 2020 Lønborg, Carreira, Jickells and Álvarez-Salgado. This is an open-access article distributed under the terms of the Creative Commons Attribution License (CC BY). The use, distribution or reproduction in other forums is permitted, provided the original author(s) and the copyright owner(s) are credited and that the original publication in this journal is cited, in accordance with accepted academic practice. No use, distribution or reproduction is permitted which does not comply with these terms.
*Correspondence: Christian Lønborg, Y2xvbmJvcmdAZ21haWwuY29t