- 1National Institute of Water and Atmospheric Research Ltd. (NIWA), Wellington, New Zealand
- 2School of Earth and Environmental Sciences (SEES), University of Queensland, Brisbane, QLD, Australia
- 3Victoria University of Wellington (VUW), Wellington, New Zealand
- 4The Research School of Earth Sciences, The Australian National University (ANU), Canberra, ACT, Australia
Deep-sea corals are a highly diverse group of marine organisms, several of which are characterised by slow growth and extreme longevity up to thousands of years. Due to their fragile forms, skeletal composition, and location, they are vulnerable to various anthropogenic threats, with some groups expected to have little to no ability to recover. Within the New Zealand region an understanding of age and growth parameters and recovery potential for some deep-sea coral fauna is being developed, but a limited understanding remains for some of the key Antipatharian black coral fauna. Ten individual colonies of the black coral species Bathypathes patula were sourced from the National Institute of Water and Atmospheric Research Invertebrate Collection for the purposes of this age estimation study. Corals were selected based on their size, completeness of the colony (whole colony from base to tip), and the regional water mass within which they grew. Coral samples from the Chatham Rise, east of New Zealand, and the Bay of Plenty, north-eastern New Zealand, were selected as the water masses for these two regions are reasonably well understood. Thin section preparations of the main stem of the 10 specimens of B. patula were observed with compound microscopes. Two interpretation protocols were used to describe the zone structures observed, both the coarse wide zones and the thin fine zones, and counts were then made of these structures. Four of the specimens were also sampled for radiocarbon assay. The radiocarbon isotope (14C) age data results were used to independently verify if either of the developed zone counting protocols reflected annual periodicity. Neither method was verified, indicating zone counting protocols could not be used to generate reliable age estimates for B. patula. Twenty radiocarbon assays from four specimens were used to derive the age and growth rate estimates presented here. The radiocarbon results from this work show B. patula to be a long-lived species, attaining ages in excess of 385 years, with linear growth rates of 5.2–9.6 mm/yr, and radial growth rates ranging from 11.1 to 35.7 μm/yr. The delicate nature of these organisms along with their longevity and slow growth rates means a low resilience and low recoverability from anthropogenic activities such as fishing and mining.
Introduction
Deep-sea corals are a highly diverse group of marine organisms, and the New Zealand region is a hotspot for deep-sea corals (Sánchez and Rowden, 2006; Cairns et al., 2009). Several studies have investigated the effects of bottom trawling on deep-sea macro-invertebrate assemblages (including coral) in the South Pacific region (Althaus et al., 2009; Clark and Rowden, 2009; Clark et al., 2010, 2019; Williams et al., 2010; Anderson et al., 2014). These have demonstrated appreciable impacts from bottom trawling on slow growing and fragile deep-sea coral colonies and communities with little to no ability to recover. Environmental impacts predicted from climate change, such as ocean warming and ocean acidification, are also expected to threaten the health of deep-sea corals with some habitats becoming too warm for them to thrive in Anthony and Marshall (2009), or reduced in pH and carbonate ions, which will likely impact deep-sea corals with carbonate based skeletons (Gammon et al., 2018). To better inform future risk assessments and marine resource management, an understanding of deep-sea coral age and growth is key to determining coral regeneration times and recoverability following anthropogenic disturbances or other natural damage.
A considerable amount of work has been undertaken in the New Zealand region on the taxonomy of deep-sea corals, and the distribution of different species and their environmental preferences, as well as the importance of the deep-sea coral to the benthic ecosystems. A review of our understanding and knowledge of deep water corals in the New Zealand region has recently been published (Tracey and Hjorvarsdottir, 2019). This review also outlined major gaps in our understanding of deep-sea corals, including limited age and growth data for many genera and species. In this study we assess the age and growth rate of the protected deep-sea black coral Antipatharian Bathypathes patula. The age and growth rate for this coral have not been previously studied in this region. The selection of this species was based on the risk assessment priority list (Clark et al., 2014) and the literature review (Tracey et al., 2018), coupled with availability of samples (location and total numbers), and other complementary black coral research being undertaken in New Zealand (Hitt et al., 2020).
Tracey et al. (2018) outlined the current methods that are being utilised to age deep-sea coral species. The advantages and disadvantages of each method were discussed. The main methods that have been successfully applied to measure age and growth of deep-sea corals are (1) direct observation, such as in situ measurements or in-aquaria experiments of linear growth or surface extensions; polyp addition rate; estimation of calcification rates (e.g., using the buoyant weight technique), (2) enumeration of skeletal growth zones or bands (sclerochronology), and (3) radiometric analyses.
Direct observations and in situ or in-aquaria measurements are very difficult to undertake for deep-sea corals as it is hard to obtain living viable specimens and keep them alive as they take a long time to grow (Gammon et al., 2018; Tracey et al., 2018). Enumeration of growth zones, Sclerochronology, is ideal for deep-sea coral species that have a high contrast between the zone-like growth patterns observed in skeletal cross sections. It is essential, however, that an independent validation method is used to test the chronological validity of counts of observed growth zones (Andrews et al., 2002). The method to obtain visible growth zone counts in the skeletal structure has been successfully applied to determine age and growth rate data of gorgonian octocorals including 400 year old bamboo corals Keratoisis sp., 100 year old seafan Primnoa spp., and 60 year old bamboo coral Lepidisis sp. (Thresher et al., 2004, 2007; Mortensen and Buhl-Mortensen, 2005; Sherwood et al., 2005; Tracey et al., 2007; Sherwood and Edinger, 2009). Black corals have also been successfully aged using growth zone or counts, with ages of 150 years old to 480 years for Antipathes dendrochristos and Leiopathes glaberrima, respectively (Love et al., 2007; Williams et al., 2007). In a study of the black coral Stauropathes arctica, zone counts of 55–58 were obtained (Sherwood and Edinger, 2009), with the authors noting that radiocarbon dating constrained these ages to 55 and 82 years. Growth rates of black corals via growth zone enumeration reveal low radial growth from 8 to 140 μm/yr (Love et al., 2007; Prouty et al., 2011).
There are potential limitations to the enumeration of growth zone method (see summary in Tracey et al., 2018). Many corals do not display clear regular zone structure throughout their skeletons, or the clear zone structure may only be apparent in portions of the skeleton. Even when present the zone structure may be variable or display differing interpretation possibilities. For example, basal and tip counts of bamboo octocoral species Keratoisis sp. and Lepidisis sp. were higher than the ages estimated by lead 210 (210Pb) radiometric method (Tracey et al., 2007). It was hypothesised that zones observed by light microscope have a bi-annual periodicity and that SEM-observed zones at the nodal juncture may represent an environmental event, such as lunar periodicity. Lunar cycles can be associated with strong tidal events, that could enhance food availability to sessile filter feeding organisms resulting in pulses of somatic growth. Gorgonian bubblegum corals (e.g., Paragorgia spp.) and red precious corals (e.g., Corallium sp.) have not been aged successfully using zone counts due to inherently complex or poorly defined banding patterns (Griffin and Druffel, 1989; Andrews et al., 2005).
The most common radiometric method used in deep-sea corals is dating with the radiocarbon (14C) isotope (Druffel et al., 1995; Sherwood et al., 2005; Tracey et al., 2018). This method relies on the fact that a very small amount of natural carbon in the atmosphere is radioactive in the form of 14C and is incorporated into the coral skeleton when it forms its calcium carbonate or protein and chitin (Adkins et al., 2002; Tracey et al., 2003; Consalvey et al., 2006). Because the half-life of radiocarbon is known to be 5,730 years, this method, albeit expensive, can be used to reliably age specimens of less than 50,000 years ago; beyond that, the activity of 14C becomes too low to detect (Coleman, 1991). During the 1950s–60s, there was rapid increase of atmospheric 14C resulting from the testing of nuclear devices; this “bomb carbon” can also be used as a tool to calibrate ages, providing a reference point for more recent samples (Coleman, 1991; Druffel et al., 1995; Tracey et al., 2003; Roark et al., 2009; Sherwood and Edinger, 2009).
Radiocarbon dating has been successfully used on several coral species, including stony corals, black corals and gorgonian octocorals (Druffel et al., 1990; Roark et al., 2006; Carreiro-Silva et al., 2013; Prouty et al., 2016; Hitt et al., 2020). However, there are also limitations with this method. As a result of ocean circulation, the radiocarbon age of the water in the oceans is significantly older than the atmosphere, the so called “marine reservoir age” (Tracey et al., 2003; Consalvey et al., 2006; Roark et al., 2006; Noé et al., 2008). Errors in the reservoir age can have significant effects on coral age estimates. Correcting for reservoir ages requires knowledge of surrounding waters and assumes constant ratios of radiocarbon in the water; however, these levels may be altered by mixing within the ocean, localised upwelling events and productivity blooms, all of which can alter carbon cycle transformations and coral skeletal composition of 14C (Tracey et al., 2018).
Based on the Tracey et al. (2018) review we chose to use two ageing methods for B. patula; counts of skeletal growth bands and the radiometric method of radiocarbon dating (14C). The application of these methods enables comparisons with other previous coral ageing studies both in New Zealand and globally. Sample selection, micro-milling of material and sectioning methods, and the interpretation of the zone counts and radiocarbon dating are presented. Age and growth characteristics of the black coral B. patula and one colony of Bathypathes cf. conferta colony (initially assumed to be B. patula) are provided.
Materials and Methods
Sample Selection
The samples used in this study were sourced from preserved specimens lodged in the New Zealand National Institute of Water and Atmospheric Research (NIWA), National Invertebrate Collection (NIC).
Samples were selected from two regions; the Bay of Plenty (north-east of the North Island of New Zealand) and Chatham Rise (east of New Zealand), to compare results and assess variability in growth rates between different areas, and also with other deep-sea black coral genera, specifically Antipathella, Leiopathes, and Antipathes, from the same regions (Hitt et al., 2020).
Oceanographic currents influence deep-sea corals by providing a steady flow of food and dispersal of larvae. Consequently, these currents also influence the availability of prey and distribution of deep-sea corals. While deep-sea corals are most affected by the bottom water currents that flow over them and influence the distribution of sediments, they are also affected by the surface water currents which influence primary productivity and thus the food supply that rains down to the deep-sea coral on the seafloor. The New Zealand landmass sits at the cross-roads between warm, nutrient-poor, subtropical surface waters flowing in from the north, and cool, nutrient-rich, subantarctic surface waters flowing in from the south. The present understanding of the water masses and ocean currents in the region at the surface, thermocline, intermediate waters, and deep waters, was recently reviewed by Chiswell et al. (2015). The oceanographic setting for the corals from this study are as follows.
The Bay of Plenty is bathed by warm, low nutrient, subtropical surface waters that are sourced from the East Australian Current and flow in from the west and form the East Auckland Current. The Chatham Rise is the meeting point of the warm, low nutrient, Subtropical waters (STW) and cool, high nutrient, Subantarctic waters (SAW) sourced from the Southern Ocean is the Subtropical Front (STF). The mixing of STW and SAW water masses at the STF results in the Chatham Rise being a zone of very high primary productivity providing a significant food source and hence carbon supply for these corals, chlorophyll a concentrations range from 0.3 and 0.48 mg/m3 in the Chatham Rise and Bay of Plenty, compared to 0.03–0.3 mg/m3 in the central Pacific (Pinkerton, 2016). At depths of 500–1500 m the cool (3.5–10°C), low salinity (34.4–34.6) and high oxygen (200–250 μmol/kg) and high nutrient (nitrate 15–36 μmol/kg) Antarctic intermediate waters (AAIW) flow into this region from the north and south (Chiswell et al., 2015).
Corals from the Chatham Rise were chosen as there are modern 14C reservoir age data from this area (Sikes et al., 2008). Knowing the local 14C reservoir age enables a more precise radiocarbon age estimate from corals.
The samples were selected from existing specimens collected by Fisheries New Zealand (FNZ), Ministry for Primary Industries (MPI) Observers and NIWA researchers and are held in the (NIC). A plot of the distribution data for B. patula aided the decision on colony site selection and sample numbers (Figure 1). Two specimens from the Bay of Plenty (NIWA24190 and NIWA85940) were selected for radiocarbon dating and thin sections for zone counts. The rest of the specimens were selected from the Chatham Rise.
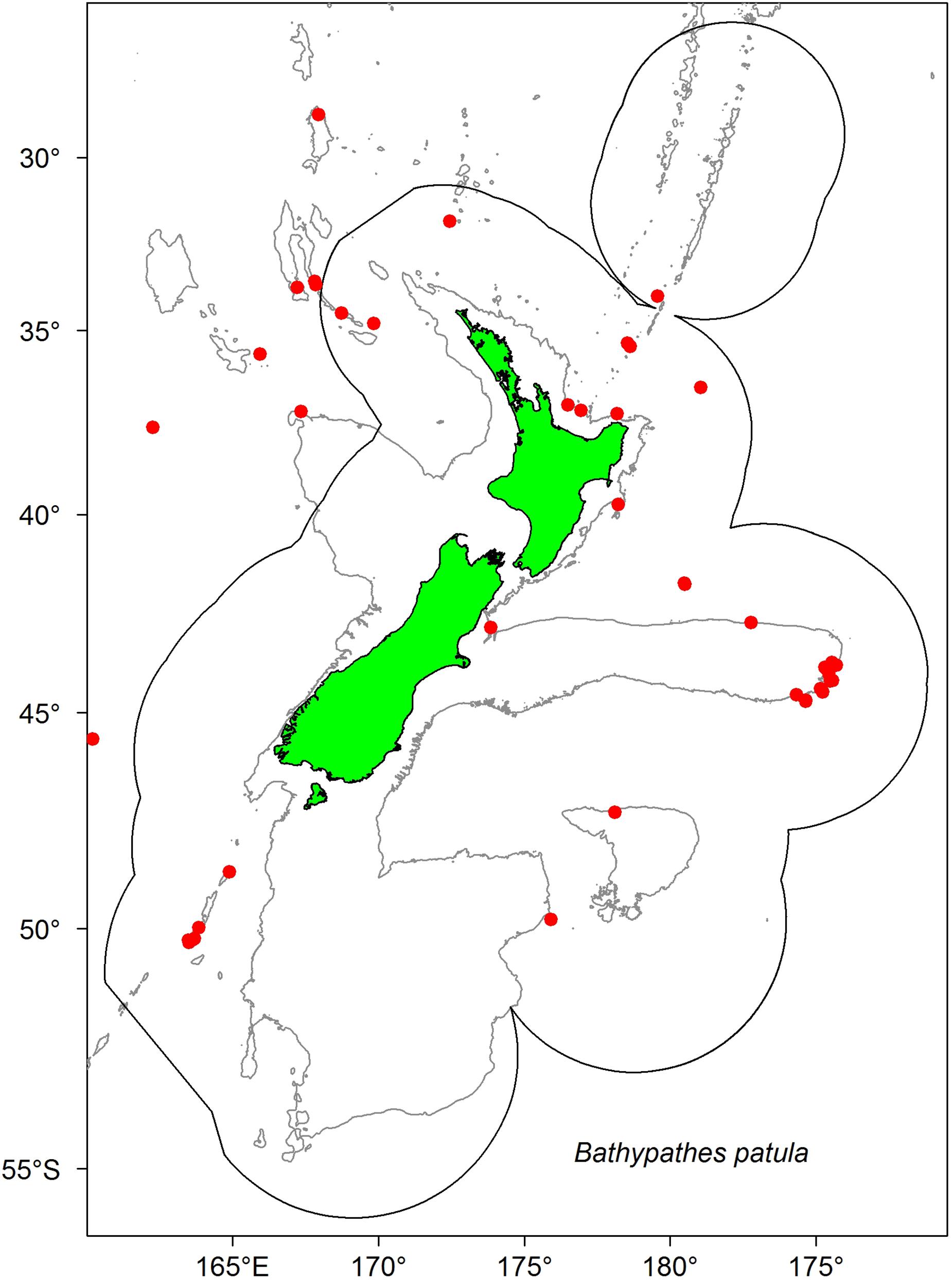
Figure 1. Locations where Bathypathes patula has been sampled in the New Zealand region. Red dots denote individual coral sample source locations for B. patula specimens held in the National Invertebrate Collection of New Zealand (NIC). Gray lines denote 200 m water depth contour. Black line denotes the extent of the New Zealand Exclusive Economic Zone (EEZ).
In late 2018, a visiting specialist in black coral taxonomy (Jeremy Horowitz, James Cook University) reviewed the identifications of several samples in the NIC. During this process one of the black coral colonies selected for this age estimation research and assumed to be Bathypathes patula, was given a revised identification. Black coral NIWA24190 has now been identified as being Bathypathes cf. conferta. The sample has been referred to as Bathypathes cf. conferta throughout this paper (Figure 2), the most likely correct identification. Further taxonomic review of this specimen is ongoing (Opresko pers comm). The distribution map (Figure 1) may include additional examples of B. cf. conferta.
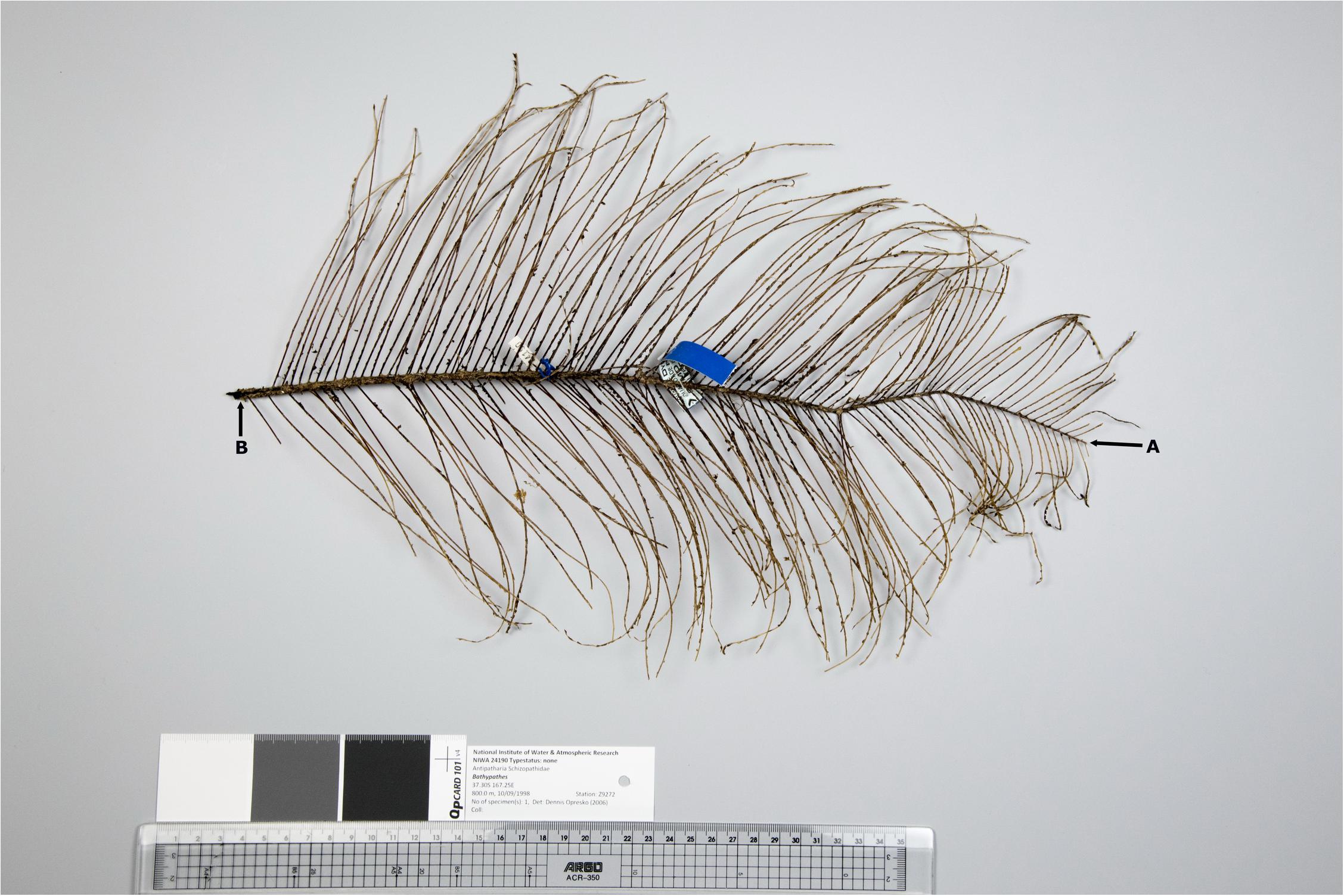
Figure 2. Bathypathes cf. conferta. NIWA24190. Specimen showing where samples were extracted for analysis. (A) is the growing tip, (B) the basal section. Specimen from Bay of Plenty, New Zealand. Scale bar is a 35 cm ruler.
Preparation of Material
Once suitable samples had been identified, small (less than 10 mm sections) were excised from the growing tips and basal portion of the corals’ main stem. As many of the samples had been broken during the collection process, additional sections were taken along the main stem so that reliable estimates of linear growth rate could be generated (Figures 2–5). The samples from the growing tips were then split into two fragments, one for radiocarbon dating the other for thin section preparation to obtain zone counts.
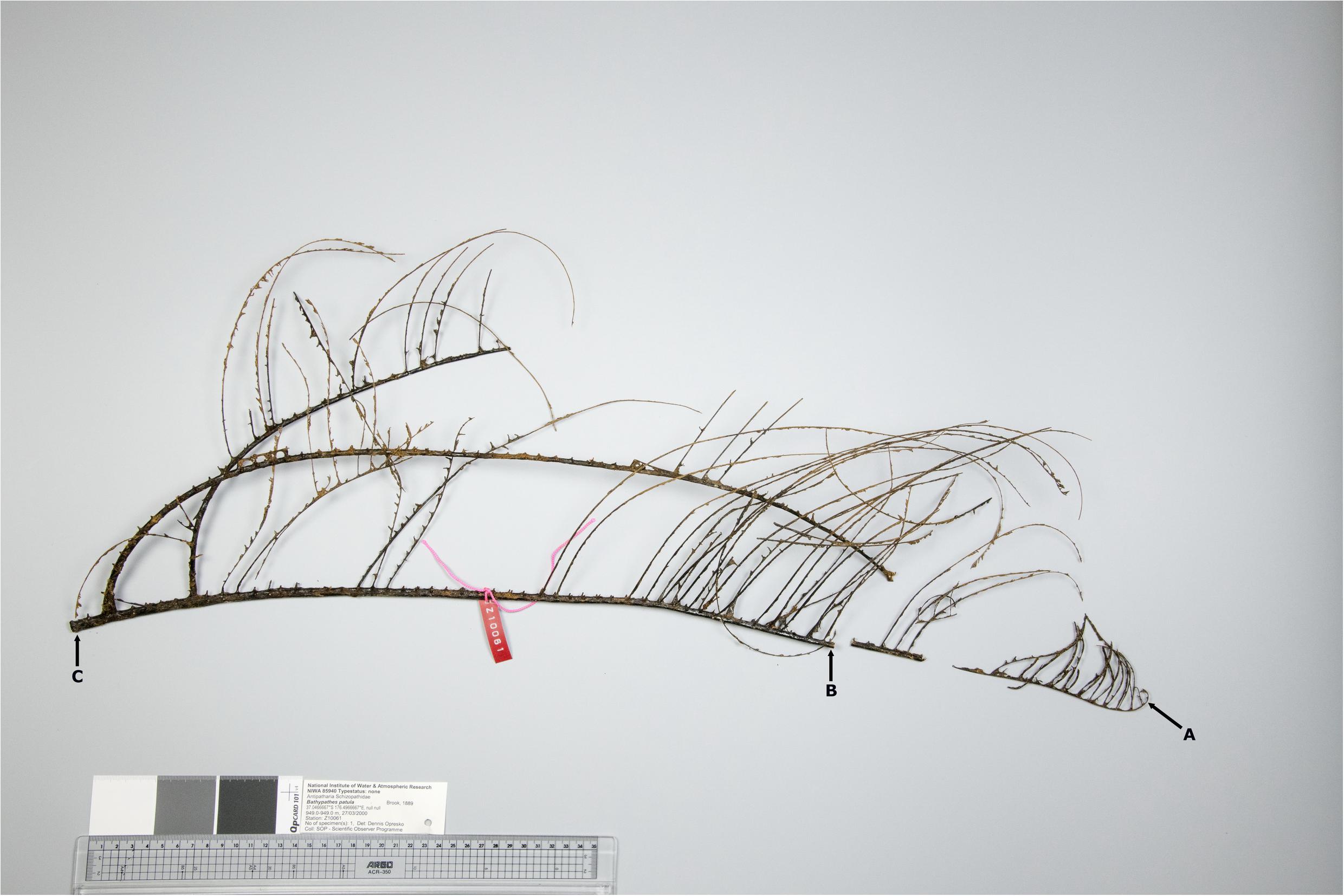
Figure 3. Bathypathes patula NIWA85940. Specimen showing where samples were extracted for analysis. (A) is the growing tip, (C) the basal section, and (B) is an intermediate sample sites as the specimen was broken on collection and unknown lengths of the main branch stem may be missing above this site. Specimen from Bay of Plenty, New Zealand. Scale bar is a 35 cm ruler.
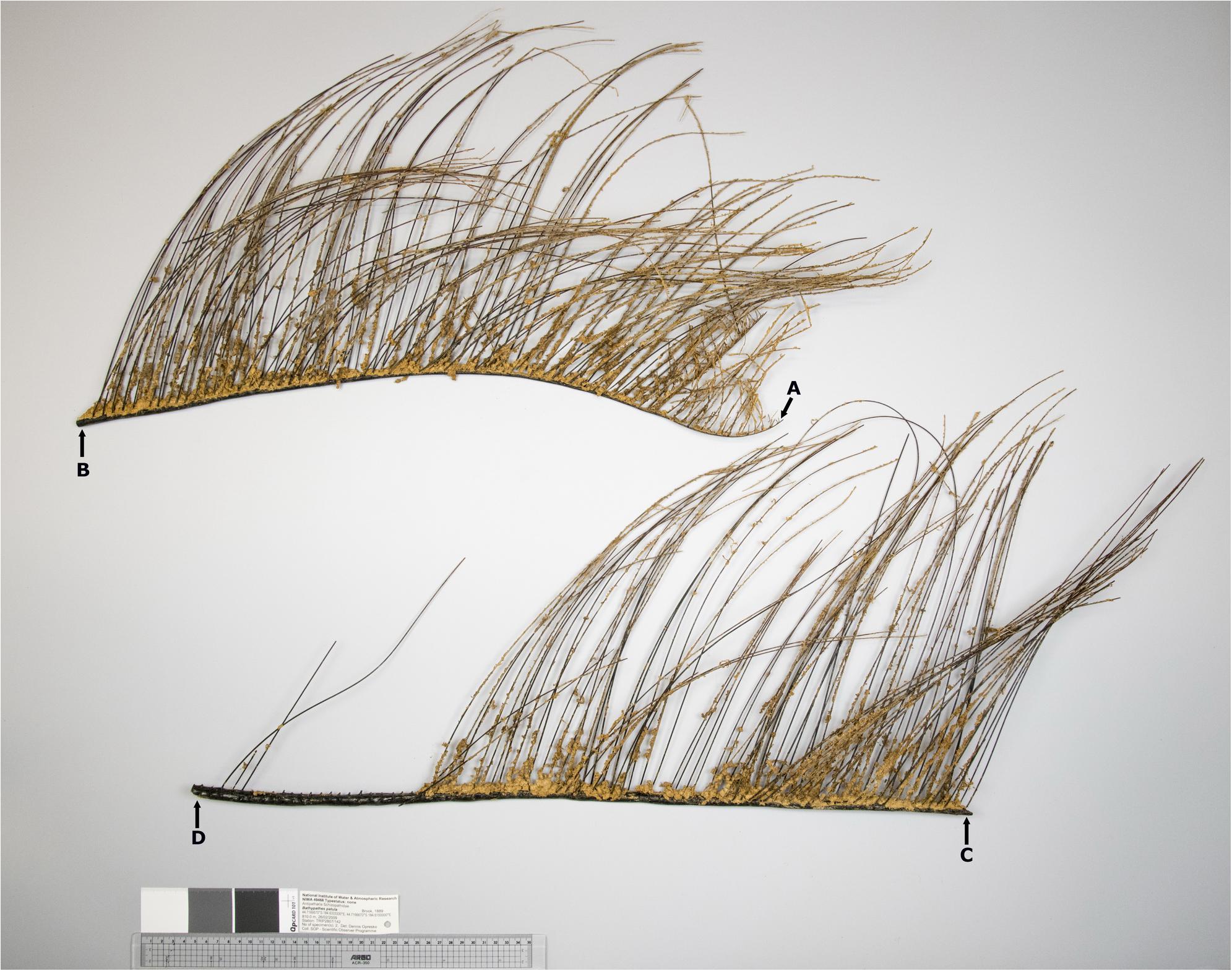
Figure 4. Bathypathes patula NIWA49468. Specimen showing where samples were extracted for analysis. (A) is the growing tip, (D) the basal section, and (B,C) are intermediate sample sites as the specimen was broken on collection and an unknown length of the main branch stem may be missing from between these two sites. Specimen from Chatham Rise, New Zealand. Scale bar is a 35 cm ruler.
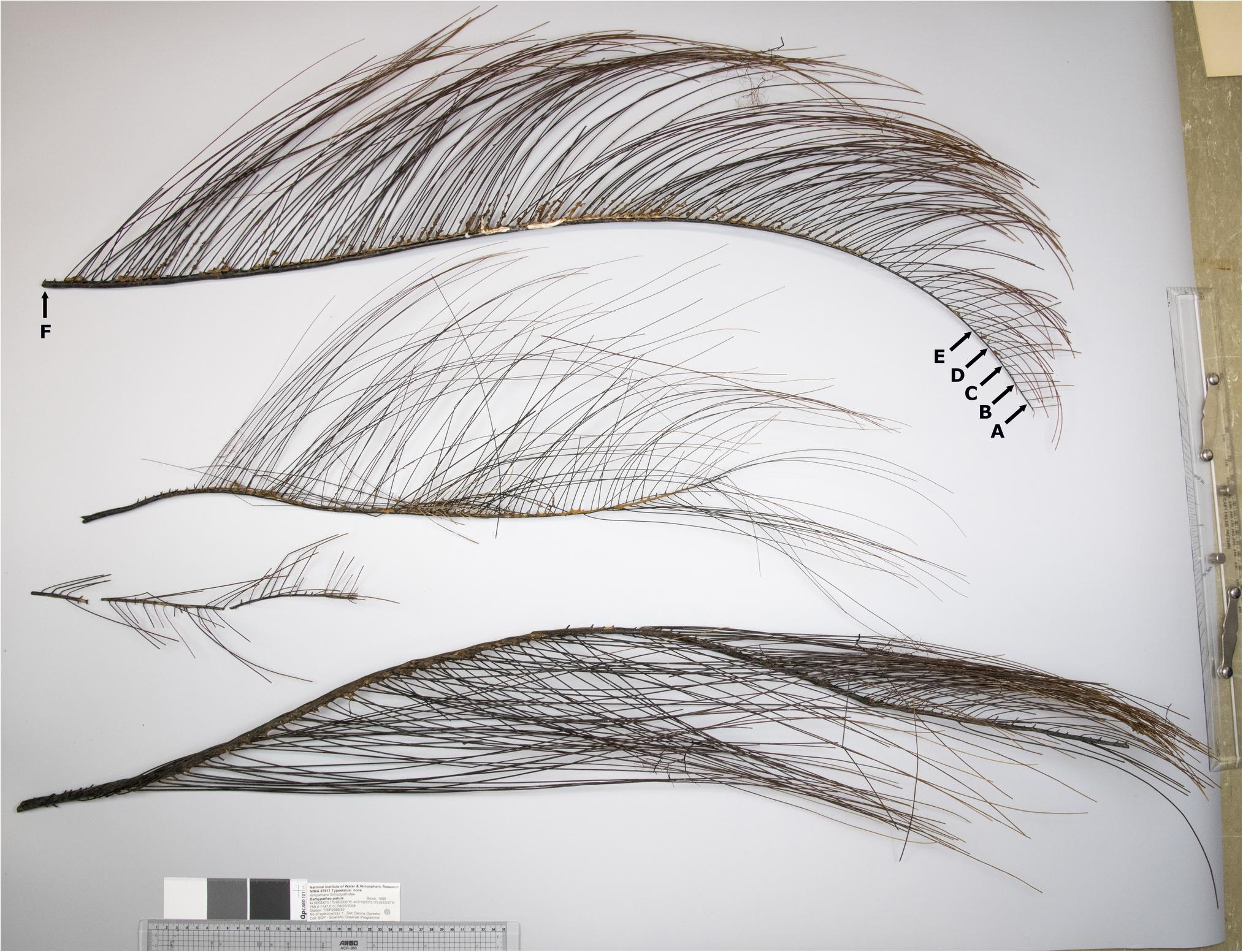
Figure 5. Bathypathes patula NIWA47911. Specimen showing where samples were extracted for analysis. (A) is the growing tip, (F) the basal section and, (B–E) are intermediate sample sites taken to look for the radiocarbon bomb signal. Specimen from Chatham Rise, New Zealand. Scale bar is a 35 cm ruler.
Micro-Milling of Material
From previous work it had been noted that black coral sections are prone to drawing resin up through their porous matrix via capillary action and so the sections in this study could not be bonded with resin to a baseplate for milling. Instead a chuck was manufactured to hold the section during the milling process, thus eliminating the risk of resin contaminating the radiocarbon samples.
Powdered radiocarbon samples were extracted from the edge and core of basal, middle and tip sections using a New WaveTM micromill with a 0.5 mm Brassler H2.11.006 milling burr at NIWA. The edge samples comprised material from the outer 250 microns of the section, the core samples were obtained from material within a 750 micron radius of the primordium. We attempted to extract at least 1.5 mg of material per sample. The samples were then weighed and analysed for radiocarbon at Australian National University (ANU) Radiocarbon Laboratory.
Thin Sectioning Method and Zone Counting Protocols
Coral stem sections were embedded in clear two-part epoxy resin and sectioned with a diamond-wafering saw at NIWA. The sections were polished on one side and mounted polished side down on a glass microscopy slide, then ground and polished until they were an optimum thickness for viewing the growth zone structure using transmitted light. The optimum thickness for sections of this species was about 250–300 μm and follows standard procedure for thin section preparation (Andrews et al., 2002; Mortensen and Buhl-Mortensen, 2005; Tracey et al., 2007). Counting the periodic growth bands or increments, assumed to be formed annually, took place after thin section preparation at NIWA.
To generate zone counts, thin section preparations of the main branch sections were viewed under a compound microscope with transmitted light. As an aid to zone interpretation the sections were also viewed using ultra-violet light following the methodology of Sherwood and Edinger (2009). Here zone counts were made using transmitted bright field lighting and ultra-violet illumination was a useful aid in helping to further define the observed zone structure.
Radiocarbon Dating Method
Prior to analyses, the samples were cleaned (e.g., acid leached) of black crusts and endolithic activity to remove any potential younger contaminant 14C which may alter results (Adkins et al., 2002). Powdered coral samples were treated with <1 ml of 0.1 M HCL. The acid was then pipetted off and samples were then washed three times with milliQ water. The sample was then frozen with dry ice and lyophilised. Approximately 1mg of cleaned coral sample was weighed into a 6 mm OD quartz tube, ∼60 mg CuO and a silver cup was added. The tubes were evacuated to <3e-3 torr (<0.4 Pa) and sealed with a torch. Tubes were then baked at 900°C for 6 h to generate CO2. The resulting CO2 was purified and converted to graphite in the presence of hydrogen with powered Fe as a catalyst. The graphite was then measured on the ANU single stage accelerator mass spectrometer (Fallon et al., 2010). All data were corrected using on-line AMS δ13C, normalised to Oxalic Acid I and background subtracted using 14C free coal treated in the same manner as the coral samples. Data are presented according to the recommendations of Stuiver and Polach (1977).
Results
Thin Sections for Zone Counts
The zone structural patterns observed on the skeletal sections for these species is very complex and can be interpreted in many ways. For the radial growth counts the prepared sections were carefully observed and the axis that best exhibited a clear zone structure from the primordium to the outer edge was then used to obtain zone counts. Radial growth of the branches in B. patula was reasonably regular, there was no marked bias in the zone deposition forming thicker zones on any particular side of the branch, which has previously been observed in other black coral species. The radii used for the growth rate calculations was a mean of the minimum and maximum radii observed in the prepared sections, meaning reported radial growth rates will be a mean radial growth rate for the coral.
On the coral skeleton there is an initial coarse banding pattern largely defined by alternating darker and lighter zones (Figure 6) when observed under lower power (10–20×). On closer examination under higher power (100–200×), there is also a reasonably regular fine scale banding pattern apparent (Figure 7). Here we applied two reading protocols – counting both the coarsely formed wide zones as well as the thin finely formed zones (Table 1). Zone counts presented here were all made by a single reader (Marriott). During the process of developing the zone interpretation protocols, many readings were made applying the various iterations of the protocols being developed. The zone counts from the two protocols presented here were each derived from multiple counts across the section.
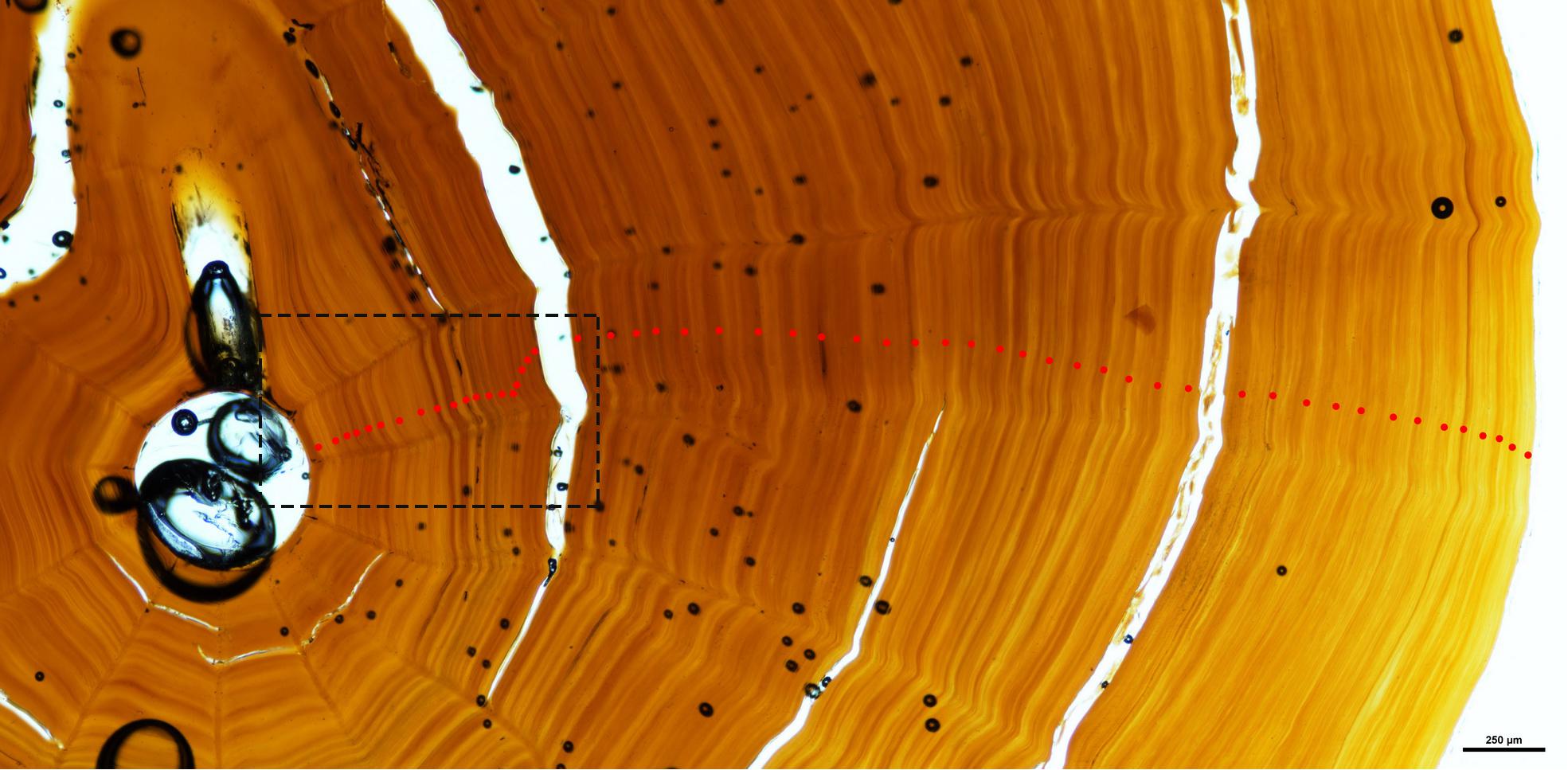
Figure 6. Basal section of coral NIWA47911 showing the coarse coloured banding interpretation of the observed zone structure. The specimen shows 54 zones marked with red dots. Inset dashed rectangle shows the central portion of the basal section imaged in Figure 7.
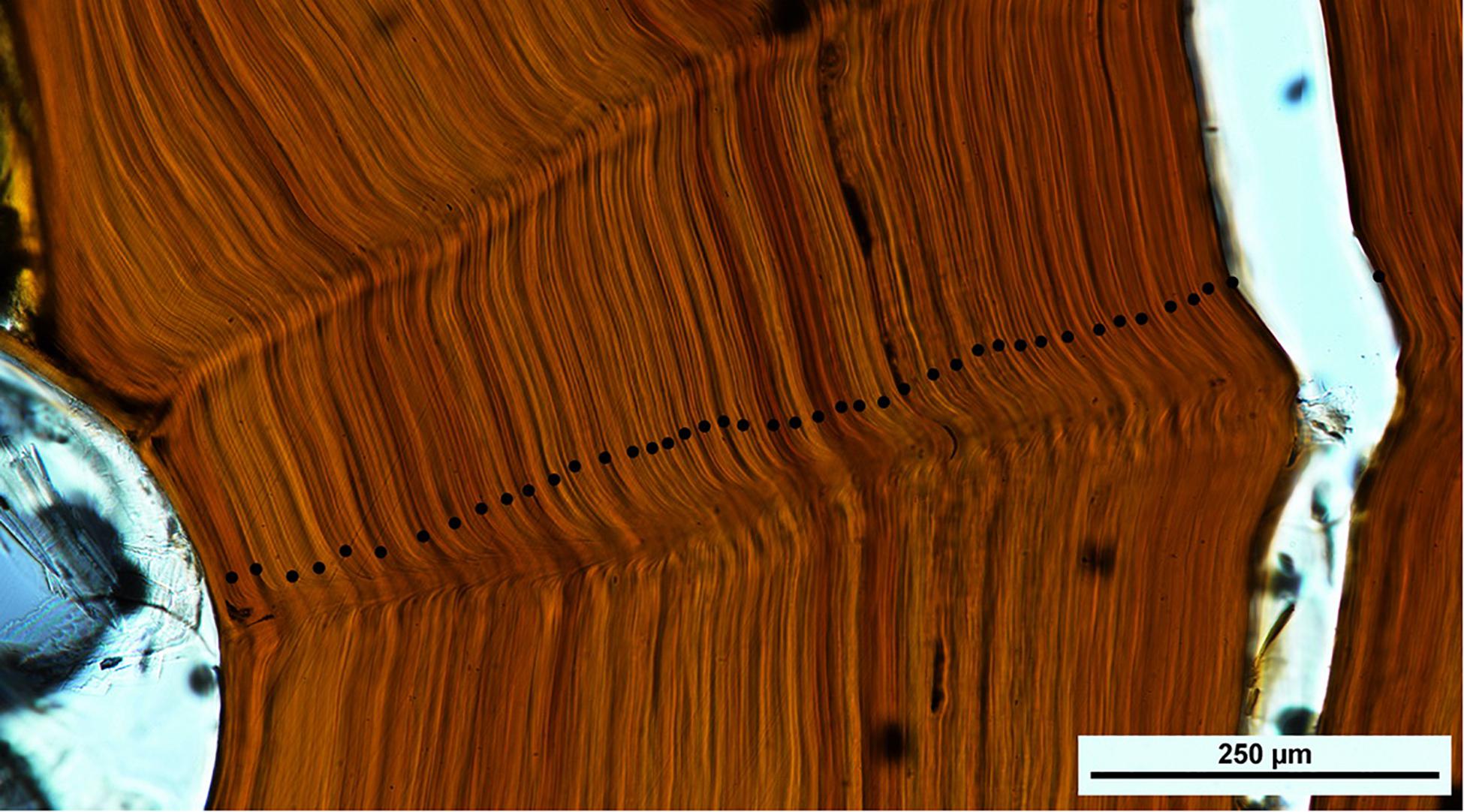
Figure 7. Central portion of the basal section of coral NIWA47911 showing the fine scale interpretation protocol of the observed zone structure. The specimen shows 42 zones marked with black dots across this inner region of the section; the whole section showed 211 zones.
For the youngest part of the study specimens, the tip, or near tip region, counts ranged from 4 to 7 (coarse zone counts) and 10 to 19 (fine zone counts). In the mid-region of the main branch, counts ranged from 55 to 60 (coarse zone counts) and 370 to 374 (fine zone counts). For the colony base region counts ranged from 37 to 193 (coarse zone counts) and from 78 to 481 (fine zone counts). The complexity of the zonation patterns highlighted the need for validation.
Radiocarbon Analysis
The milled samples listed in Table 2 were sent to ANU for radiocarbon dating. The results from the 17 coral samples are shown in the radiocarbon results (Table 3). Three samples (Samples 47911-F2; 24190-B1; 85940-B1) failed in the laboratory, with insufficient material remaining to attempt a duplicate. The radiocarbon results from the remaining corals were calibrated to a calendar age using OxCal4.3 (Bronk Ramsey, 2001), the Marine13 curve and a New Zealand local deltaR (radiocarbon reservoir offset) of −18 ± 36 (Reimer and Reimer, 2001). The age range distributions for pre-bomb coral samples are shown in Table 3. All coral specimens used in this study were live-at-capture, so any age estimates given are the age at capture not the lifespan of the specimen. Projected lifespans for this species will be in excess of the age estimates presented here. Coral 85940 was approximately 380 ± 50 years old, coral 49468 approximately 310 ± 70 years old, and coral 47911 approximately 120 ± 50 years old (Table 3). All of the coral outer edge and tip samples contained radiocarbon values F14C >1 (Table 3), except for 85940-B2 and 85940-C2, which indicates these corals were alive and incorporating surface water carbon at the time of collection. Corals 85940-B2 and 85940-C2 had outer edge radiocarbon values F14C <1 (Table 3) indicating that part of the skeleton was not actively adding material. This has been observed previously where the basal part of the coral colony stops growing, however, the upper section continues to grow (Komugabe-Dixson et al., 2016).
Growth Rate Estimates
Growth rate estimates were based on the radiocarbon results as zone counts were problematic and proved to be unvalidated so could not be used to generate reliable growth rate estimates (see section “Bathypathes patula Age and Growth Estimates” for a further discussion on zone counting). Growth rates were derived from the physical distances between sampling points and the calibrated radiocarbon ages at the relevant sample site. Due to some samples failing during the radiocarbon analysis, only a limited set of growth rate estimates could be generated. Radial growth rates, the rate at which the colony branch thickens radially over time, were estimated by dividing the calibrated calendar years of growth between the core and the edge of the sample site by the mean radius of the branch segment. Radial growth ranged from 11.1 to 35.7 μm/yr. Linear growth rates, the rate at which the colony branch lengthens longitudinally over time, were estimated by dividing the difference between the calibrated calendar ages of core samples by the linear distance between two sample sites. Two linear growth rates were obtained, 5.2 and 9.6 mm/yr (Table 4).
Discussion
Bathypathes patula Age and Growth Estimates
The radiocarbon results from this work show B. patula to be a long-lived species, with ages in excess of 385 years. Slow linear (5.2–9.6 mm/yr) and radial (11.1–35.7 μm/yr) growth rates were obtained from the radiocarbon age data.
Neither of the zone count protocols reliably correlated with the independent radiocarbon age estimates. The fine scale zone counting protocol showed the closest correlation with the 14C age data providing some overall support for longevity when comparing the zone counts with 14C dates. Age estimates between the radiocarbon age data and the fine scale zone count data routinely showed a disparity of more than one hundred years in branch sections with calibrated radiocarbon ages of 112–385 years (Tables 1, 3). Differences in estimated ages from comparable samples using the two methods were negative and positive; there was no under- or over-ageing using zone counts by a proportional amount (Table 1). This means the observed zone structure does not display annual, nor any other regular chronological pattern of periodicity, and our zone counting protocols cannot be used to reliably generate age estimates for B. patula.
The edge radiocarbon results from specimen NIWA-85940 suggest that the basal section (C2) of the main stem stopped depositing skeletal material on its outer surface 293 years pre-harvest, and the mid-section (B2) stopped depositing skeletal material 88 years pre-harvest. This is despite the observation that these sections of branch stem were immediately adjacent to lateral pinnules with living polyps. The optical appearance of these sections gave no indication that zone deposition had ceased on their outer margin. The surface of the corals branch still appeared to be relatively pristine, it did not show any evidence of superficial erosion or discolouring, as might be expected in a coral branch that was no longer accreting new skeletal material to its outer surface. This suggests if branch stems can stop accreting skeletal layers, while the branch ends continue to grow, then zone counting method protocols cannot be reliably utilised for the generation of age estimates from basal sections for this species of coral.
In specimen NIWA47911 five samples were extracted from the growing tip of the main axial branch towards the coral base. These samples were spaced 25 mm apart. This was an attempt to locate the appearance of bomb radiocarbon in the corals’ skeleton. This would mean the linear growth rate could be accurately assessed for the more recent growing tips of the colony’s branches. If the rise of bomb radiocarbon could be accurately placed, then it would also give an accurate tie point for setting the 14C correction to apply to the pre-bomb radiocarbon samples for this colony and to other colonies from the same water mass. Unfortunately, all these samples proved to be post-bomb so this process could not be completed. Future sampling further back down this branch stem would be useful to establish this tie point and contemporary linear growth rate. Our work measured overall linear growth rates of 5.2–9.6 mm/yr, assuming the fastest growth rate you would need to sample 700 mm back down the main branch stem from the growing tip to be confident of observing the rise of bomb radiocarbon in the skeleton.
Research by Love et al. (2007) on the black coral species Antipathes dendrochristos also reported two interpretations of the zone structure similar to the coarse and fine protocols described herein. However, these authors found that the coarser coloured banding structure correlated with their radiocarbon and 210Pb results indicating annual banding (Love et al., 2007).
The radiocarbon measurements from the current work exhibited F14C >1 in samples from the growing branch tips and main-branch surface, indicating these samples showed modern post-bomb levels of radiocarbon. The presence of post-bomb carbon below the mixed layer of the ocean, indicates that the B. patula black corals are using recently exported surface water POC. At these depths POC is the only carbon source tagged with bomb 14C. The dissolved inorganic carbon (DIC) of the ambient water that the corals are living in has much lower 14C values (Matsumoto, 2007; Alves et al., 2018). Detecting bomb 14C in corals which grow in waters that have not experienced bomb 14C (i.e., through ocean ventilation) reinforces that black corals derive their carbon from surface derived POC (Roark et al., 2009; Sherwood and Edinger, 2009; Williams and Grottoli, 2010; Prouty et al., 2011). This also means that we do not need to model for a deep-water reservoir effect, as is the case of the stony corals, which derive their skeletal carbon from DIC in the intermediate waters that bathe them.
Comparisons With Recent New Zealand and Global Black Coral Age Data
The radiocarbon derived age estimates and growth rates from this project are similar to the results of a number of other age and growth studies that have recently been undertaken for key deep-sea black corals (Antipatharia) in the New Zealand region (Table 5). These age and growth estimates for B. patula are similar to other coral genera, such as Leiopathes, Antipathes, and Antipathella colonies, which live up to 2600 years and grow on the order of 1–143 μm/yr (Hitt et al., 2020). Our results provide further support that New Zealand black corals are some of the slowest growing marine animals in the region, with some species showing extreme longevity.
The results from B. patula and other black corals that have been aged in New Zealand do not show clear trends in growth rates or longevity with respect to the water masses and depths that these corals reside. Samples from the Chatham Rise and Bay of Plenty mostly came from reasonably similar water depths, from between 750 and <1269 m. The deeper sourced Chatham Rise B. patula specimen came from somewhere between 1120 and 1269 m (Tables 1, 5). The radial growth rate of our Chatham Rise samples (NIWA-49468 and NIWA47911) at 20–36 μm/yr was higher than the Bay of Plenty specimen (NIWA-85940) at 11 μm/yr. As only three growth rates were successfully obtained for the B. patula samples from these two regions, no assumptions about the regional differences in growth rates can be made. The spectrum of growth rate data for Chatham Rise black corals show an Antipathes sp. sample had the fastest observed growth rate to date, 91–143 μm/yr, as well as one of the slowest, 5–15 μm/yr for a Leiopathes secunda specimen. Bay of Plenty black coral specimens Bathypathes patula, Leiopathes secunda, and Leiopathes sp. exhibit middling to low radial growth rates, 5–40 μm/yr. In contrast, shallow water Fiordland black coral Antipathella fiordensis have a relatively high radial growth rate (50–57 μm/yr), but it is unclear whether this is a function of species or the fiord environmental conditions, or a mixture of both.
This research also agrees with global studies that have shown black corals to be some of the slowest-growing deep-sea corals. Sherwood and Edinger (2009) give age estimates for the black coral Stauropathes arctica up to 82 calendar years. Prouty et al. (2011) used radiocarbon to generate age estimates of live at capture Leiopathes sp. colonies to 2100 years. Carreiro-Silva et al. (2013) aged an Azores colony of Leiopathes sp. at 2320 years old. A specimen of Leiopathes annosahas from Hawaii exhibited the potential to reach a lifespan in excess of 4000 years (Roark et al., 2009).
These new age and growth rates for the delicate deep-sea black coral B. patula highlight that this is a long-lived species, shown in this study to attain ages in excess of 385 years, and exhibits slow linear and radial growth rates. The results indicate a likely low resilience and slow recovery time following disturbance/removal. This research will be used to inform future risk assessments and management strategies in the New Zealand framework for the management of corals (Freeman and Cryer, 2019). The slow growth and extreme longevity further highlights that these corals will be highly vulnerable to submarine disturbances, such as fishing and mining, and may take centuries to recover from anthropogenic impacts (Hitt et al., 2020).
Conclusion
The age and growth rate of deep-sea black coral B. patula samples from two regions of New Zealand, Bay of Plenty and Chatham Rise were determined using two different methods; growth ring counting (sclerochronology) and radiocarbon dating. Two different protocols were used to count the growth rings, using dark and light growth layers. These provided varying results, which were also inconsistent with the radiocarbon ages on several of these corals. The radiocarbon ages from B. patula indicate that this coral species lives for a long time, with ages of over 385 years, with slow linear (5.2–9.6 mm/yr) and radial (11.1–35.7 μm/yr) growth rates. These results are comparable with the ages and growth rates of other black coral genera from the same regions of New Zealand and globally. Age and growth rates for deep-sea species are critical to determine the potential risk of these organisms to anthropogenic disturbances. These results will be used to inform future risk assessments and management strategies in the New Zealand framework for the management of corals.
Data Availability Statement
All datasets generated for this study are included in the article/supplementary material.
Author Contributions
PM, DT, HB, and NH contributed to the conception and design of the study. PM performed all the sample preparation, sectioning image analysis, thin section protocol development and zone counting, and the micromilling of radiocarbon samples. NH assayed the radiocarbon samples. SF produced corrected age estimates from the raw radiocarbon data and wrote sections of the manuscript. PM and DT wrote the first draft of the manuscript. All authors contributed to the manuscript revision.
Funding
This research was funded from Year Two of the Conservation Services Programme (CSP), Department of Conservation (DOC) Project POP2017-07 Objective to “Develop a methodology to determine the age and growth characteristics of key high-risk New Zealand deep-sea (cold-water) coral species.” Funding for the publication fee for this journal was provided by NIWA, New Zealand.
Conflict of Interest
The authors declare that the research was conducted in the absence of any commercial or financial relationships that could be construed as a potential conflict of interest.
Acknowledgments
We thank the Conservation Services Programme Group, Department of Conservation New Zealand, particularly Ian Angus, Kris Ramm, and also Freydis Hjorvarsdottir (previously of CSP now Ministry of Primary Industries New Zealand) for their support of the work. Thanks to Helen Neil (NIWA) and Dan Sinclair (VUW), for supporting the provision of their preliminary data. We acknowledge NIWA staff Sadie Mills and Diana Macpherson for their input into sample selection, Owen Anderson for the production of Figure 1, Peter Horn for providing a thorough internal review, and Rosemary Hurst for helpful comments and advice on the manuscript. We thank Jeremy Horowitz (James Cook University, Australia) for sample identification, and Dennis Opresko (Emeritus Smithsonian Institute) for comments on black coral taxonomy revisions. Finally, we thank the three reviewers, this document greatly benefitted from their detailed and constructive assessment.
References
Adkins, J. F., Griffin, S., Kashgarian, M., Cheng, H., Druffel, E. R. M., Boyle, E. A., et al. (2002). Radiocarbon dating of deep-sea corals. Radiocarbon 44, 567–580. doi: 10.1017/s0033822200031921
Althaus, F., Williams, A., Schlacher, T. A., Kloser, R. J., Green, M. A., Barker, B. A., et al. (2009). Impacts of bottom trawling on deep-coral ecosystems of seamounts are long-lasting. Mar. Ecol. Prog. Ser. 397, 279–294. doi: 10.3354/meps08248
Alves, E. Q., Macario, K., Ascough, P., and Bronk Ramsey, C. (2018). The worldwide marine radiocarbon reservoir effect: definitions, mechanisms, and prospects. Rev. Geophys. 56, 278–305. doi: 10.1002/2017RG000588
Anderson, O., Tracey, D., Bostock, H., Williams, M., and Clark, M. (2014). Refined Habitat Suitability Modelling for Protected Coral Species in the New Zealand EEZ. NIWA Client Report prepared for Department of Conservation. WLG2014-69No. 46. Auckland: NIWA.
Andrews, A. H., Cailliet, G. M., Kerr, L. A., Coale, K. H., Lundstrom, C., and DeVogelaere, A. P. (2005). “Investigations of age and growth for three deep-sea corals from the Davidson Seamount off central California,” in Cold-Water Corals and Ecosystems, eds A. Freiwald and J. M. Roberts (Berlin: Springer-Verlag), 1021–1038. doi: 10.1007/3-540-27673-4_51
Andrews, A. H., Cordes, E. E., Mahoney, M. M., Munk, K., Coale, K. H., Cailliet, G. M., et al. (2002). Age, growth and radiometric age validation of a deep-sea, habitat-forming gorgonian (Primnoa resedaeformis) from the Gulf of Alaska. Hydrobiologia 471, 101–110.
Anthony, K. R. N., and Marshall, P. A. (2009). Coral Reefs and Climate Change. Marine Climate Change Impacts and Adaptation Report Card for Australia 2009. Gland: IUCN.
Bronk Ramsey, C. (2001). Development of the radiocarbon calibration program OxCal. Radiocarbon 43, 355–363. doi: 10.1017/s0033822200038212
Cairns, S., Gershwin, L., Brook, F., Pugh, P., Dawson, E., Ocanþa, O., et al. (2009). “Phylum cnidaria: corals, medusae, hydroids myxozo-ans,” in New Zealand Inventory of Biodiversity, Volume 1: Kingdom Animalia, ed. D. P. Gordon (New Zealand: Canterbury University Press), 59–101.
Carreiro-Silva, M., Andrews, A. H., Braga-Henriques, A., de Matos, V., Porteiro, F. M., and Santos, R. S. (2013). Variability in growth rates of long-loved black coral Leiopathes sp. from the Azores. Mar. Ecol. Progr. Ser. 473, 189–199. doi: 10.3354/meps10052
Chiswell, S. M., Bostock, H. C., Sutton, P. J. H., and Williams, M. J. M. (2015). Physical oceanography of the deep seas around New Zealand: a review, New Zealand. J. Mar. Freshw. Res. 49, 1–32. doi: 10.1080/00288330.2014.992918
Clark, M., Bowden, D., Baird, S., and Stewart, R. (2010). Effects of fishing on the benthic biodiversity of seamounts of the “Graveyard” complex, northern Chatham Rise. New Zeal. Aqu. Environ. Biodivers. Rep. 46:40.
Clark, M., and Rowden, A. (2009). Effects of deepwater trawling on the macro-invertebrate assemblages of seamounts on the Chatham Rise, New Zealand. Deep Sea Res. I 56, 1540–1554. doi: 10.1016/j.dsr.2009.04.015
Clark, M., Tracey, D., Anderson, O., and Parker, S. (2014). Pilot Ecological Risk Assessment for Protected Corals. Wellington: National Institute of Water and Atmospheric Research, 32.
Clark, M. R., Bowden, D. A., Rowden, A. A., and Stewart, R. (2019). Little evidence of benthic community resilience to bottom trawling on seamounts after 15 years. Front. Mar. Sci. 6:63. doi: 10.3389/fmars.2019.00063
Consalvey, M., MacKay, K., and Tracey, D. (2006). Information review for protected deep-sea coral species in the New Zealand region. NIWA Client Report. WLG2006-85. 60. Auckland: NIWA.
Druffel, E. R. M., Griffin, S., Witter, A., Nelson, E., Southon, J., Kashgarian, M., et al. (1995). Gerardia: bristlecone Pine of the deep-sea? Geochim. Cosmochim. Acta 59, 5031–5036. doi: 10.1016/0016-7037(95)00373-8
Druffel, E. R. M., King, L. L., Belastock, R. A., and Buesseller, K. O. (1990). Growth rate of a deep-sea coral using 210Pb and other isotopes. Geochim. Cosmochim. Acta 54, 1493–1500.
Fallon, S. J., Fifield, L. K., and Chappell, J. M. (2010). The next chapter in radiocarbon dating at the Australian National University: status report on the single stage AMS. Nuclear Instr. Methods Phys. Res. Sec. B 268, 898–901. doi: 10.1016/j.nimb.2009.10.059
Freeman, D., and Cryer, M. (2019). “Current management measures and threats,” in The State of Knowledge of Deep-Sea Corals in the New Zealand Region, Chap. 9, eds D. M. Tracey and F. Hjorvarsdottir (Auckland: NIWA Science and Technology Series).
Gammon, M. J., Tracey, D. M., Marriott, P. M., Cummings, V. J., and Davy, S. K. (2018). The physiological response of the deep-sea coral Solenosmilia variabilis to ocean acidification. PEERJ 6:e5236. doi: 10.7717/peerj.5236
Griffin, S., and Druffel, E. M. (1989). Sources of carbon to deep-sea corals. Radiocarbon 31, 533–543. doi: 10.1017/s0033822200012121
Hitt, N. T., Sinclair, D. J., Fallon, S. J., Neil, H. L., Tracey, D. M., Komugabe-Dixson, A., et al. (2020). Growth and longevity of New Zealand black corals. Deep Sea Res. I 2020:103298. doi: 10.1016/j.dsr.2020.103298
Komugabe, A. F., Fallon, S. J., Thresher, R. E., and Eggins, S. M. (2014). Modern Tasman Sea surface reservoir ages from deep-sea black corals. Deep-Sea Res. 99, 207–212. doi: 10.1016/j.dsr2.2013.05.024
Komugabe-Dixson, A. F., Fallon, S. J., Eggins, S. M., and Thresher, R. E. (2016). Radiocarbon evidence for mid-late Holocene changes in southwest Pacific Ocean circulation. Paleoceanography 31, 971–985. doi: 10.1002/2016PA002929
Love, M. S., Yoklavich, M. M., Black, B. A., and Andrews, A. H. (2007). Age of black coral (Antipathes dendrochristos) colonies, with notes on associated invertebrate species. Bull. Mar. Sci. 80, 391–400.
Matsumoto, K. (2007). Radiocarbon-based circulation age of the world oceans. J. Geophys. Res. 112:C09004. doi: 10.1029/2007JC004095
Mortensen, P. B., and Buhl-Mortensen, L. (2005). Morphology and growth of the deep-water gorgonians Primnoa resedaeformis and Paragorgia arborea. Mar. Biol. 147, 775–788. doi: 10.1007/s00227-005-1604-y
Noé, S. U., Lembke-Jene, L., and Chr Dullo, W. (2008). Varying growth rates in bamboo corals: sclerochronology and radiocarbon dating of a mid-Holocene deep-water gorgonian skeleton (Keratosis sp.: Octocorallia) from Chatham Rise (New Zealand). Facies 54, 151–166. doi: 10.1007/s10347-007-0129-x
Pinkerton, M. H. (2016). Ocean Colour Satellite Observations of Phytoplankton in the New Zealand EEZ, 1997-2016. Ministry for the Environment. Wellington: NIWA.
Prouty, N. G., Fisher, C. R., Demopoulous, A. W. J., and Druffel, E. R. M. (2016). Growth rates and ages of dee-sea corals impacted by the Deepwater Horizon oil spill. Deep-Sea Res. II 129, 196–212. doi: 10.1016/j.dsr2.2014.10.021
Prouty, N. G., Roark, E. B., Buster, N. A., and Ross, S. W. (2011). Growth rate and age distribution of deep-sea black corals in the Gulf of Mexico. Mar. Ecol. Prog. Ser. 423, 101–115. doi: 10.3354/meps08953
Reimer, P. J., Bard, E., Bayliss, A., Beck, J. W., Blackwell, P. G., Bronk Ramsey, C., et al. (2013). IntCal13 and Marine13 radiocarbon age calibration curves 0–50,000 years cal BP. Radiocarbon 55, 1869–1887. doi: 10.2458/azu_js_rc.55.16947
Reimer, P. J., and Reimer, R. W. (2001). A marine reservoir correction database and on-line interface. Radiocarbon 43, 461–463. doi: 10.1017/s0033822200038339
Roark, E. B., Guilderson, T. P., Dunbar, R. B., Fallon, S. J., and Mucclarone, D. A. (2009). Extreme longevity in proteinaceous deep-sea corals. Proc. Natl. Acad. Sci. U.S.A. 106, 5204–5208. doi: 10.1073/pnas.0810875106
Roark, E. B., Guilderson, T. P., Dunbar, R. B., and Ingram, B. L. (2006). Radiocarbon based ages and growth rates: Hawaiian deepsea corals. Mar. Ecol. Progr. Ser. 327, 1–37.
Sánchez, J., and Rowden, A. (2006). “Octocoral diversity on New Zealand Seamounts,” in Proceedings of the Presented at the 10th International Coral Reef Symposium, Okinawa.
Sherwood, O. A., and Edinger, E. N. (2009). Ages and growth rates of some deep-sea gorgonian and antipatharian corals of Newfoundland and Labrador. Can. J. Fish. Aquatic Sci. 66, 142–152. doi: 10.1139/f08-195
Sherwood, O. A., Scott, D. B., Risk, M. J., and Guilderson, T. P. (2005). Radiocarbon evidence for annual growth rings in the deep-sea octocoral Primnoa resedaeformis. Mar. Ecol. Prog. Ser. 301, 129–134. doi: 10.3354/meps301129
Sikes, E., Burgess, S., Grandpre, R., and Guilderson, T. (2008). Assessing modern deep-water ages in the New Zealand region using deep-water corals. Deep Sea Res. I 55, 38–49. doi: 10.1016/j.dsr.2007.10.004
Stuiver, M., and Polach, H. A. (1977). Discussion reporting of 14C data. Radiocarbon 19, 355–363. doi: 10.1017/S0033822200003672
Thresher, R., Rintoul, S. R., Koslow, J. A., Weidman, C., Adkins, J., and Proctor, C. (2004). Oceanic evidence of climate change in southern Australia over the last three centuries. Geophys. Res. Lett. 31:l07212.
Thresher, R. E., MacRae, C. M., Wilson, N. C., and Gurney, R. (2007). Environmental effects on the skeletal composition of deep-water gorgonians (Keratoisis spp.; Isididae). Bull. Mar. Sci. 8, 409–422.
Tracey, D., Bostock, H., and Shaffer, M. (2018). Ageing Methods for Protected Deep-Sea Corals: A Review and Recommendation for an Ageing Study. DOC Contract 4527 GMC - Age and Growth of coral (POP2017-07). NIWA Client Report WN2018035. Auckland: NIWA, 40.
Tracey, D., Neil, H., Gordon, D., and O’Shea, S. (2003). Chronicles of the deep: ageing deep-sea corals in New Zealand waters. NIWA Water Atmosph. 11, 22–24.
Tracey, D. M., and Hjorvarsdottir, F. (eds) (2019). “The state of knowledge of deep-sea corals in the new zealand region,” in NIWA Science and Technology Series Number 84, (Auckland: NIWA),140.
Tracey, D. M., Neil, H., Marriott, P., Andrews, A. H., Cailliet, G. M., and Sanchez, J. A. (2007). Age and growth of two genera of deep-sea bamboo corals (family Isididae) in New Zealand waters. Bull. Mar. Sci. 81, 393–408.
Williams, A., Schlacher, T. A., Rowden, A. A., Althaus, F., Clark, M. R., Bowden, D. A., et al. (2010). Seamount megabenthic assemblages fail to recover from trawling impacts. Mar. Ecol. 31, 183–199. doi: 10.1111/j.1439-0485.2010.00385.x
Williams, B., and Grottoli, A. G. (2010). Stable nitrogen and carbon isotope (δ15N and δ13C) variability in shallow tropical Pacific soft coral and black coral taxa and implications for paleoceanographic reconstructions. Geochim. Cosmochim. Acta 74, 5280–5288. doi: 10.1016/j.gca.2010.06.026
Keywords: Bathypathes patula, black coral, age, growth, zone count, radiocarbon, New Zealand
Citation: Marriott P, Tracey DM, Bostock H, Hitt N and Fallon SJ (2020) Ageing Deep-Sea Black Coral Bathypathes patula. Front. Mar. Sci. 7:479. doi: 10.3389/fmars.2020.00479
Received: 08 January 2020; Accepted: 28 May 2020;
Published: 23 June 2020.
Edited by:
Santiago Herrera, Lehigh University, United StatesReviewed by:
Mackenzie E. Gerringer, SUNY Geneseo, United StatesNancy Grumet Prouty, United States Geological Survey (USGS), United States
Copyright © 2020 Marriott, Tracey, Bostock, Hitt and Fallon. This is an open-access article distributed under the terms of the Creative Commons Attribution License (CC BY). The use, distribution or reproduction in other forums is permitted, provided the original author(s) and the copyright owner(s) are credited and that the original publication in this journal is cited, in accordance with accepted academic practice. No use, distribution or reproduction is permitted which does not comply with these terms.
*Correspondence: Peter Marriott, cGV0ZXIubWFycmlvdHRAbml3YS5jby5ueg==