- 1Antarctic Conservation and Management Program, Australian Antarctic Division, Kingston, TAS, Australia
- 2Institute for Marine and Antarctic Studies, University of Tasmania, Hobart, TAS, Australia
- 3Coastal and Marine Laboratory, Florida State University, Saint Teresa, FL, United States
The composition, spatial structure, diversity and abundance of Antarctic nematode and copepod meiobenthic communities was examined in shallow (5–25 m) marine coastal sediments at Casey Station, East Antarctica. The sampling design incorporated spatial scales ranging from 10 meters to kilometers and included testing for human impacts by comparing polluted (metal and hydrocarbon contaminated sediments adjacent to old waste disposal sites) and control areas. A total of 38 nematode genera and 20 copepod families were recorded with nematodes being dominant, comprising up to 95% of the total abundance. Variation was greatest at the largest scale (km’s) but each location had distinct assemblages. At smaller scales there were different patterns of variation for nematodes and copepods. There were significant differences between communities at control and impacted locations. Community patterns had strong correlations with concentrations of metals introduced by human activity in sediments as well as sediment grain size and total organic content. Given the strong association with environmental patterns, particularly those associated with human impacts, we provide further evidence that meiofauna are very useful indicators of anthropogenic environmental changes in Antarctica.
Introduction
Knowledge of Antarctic meiobenthic communities (here limited to metazoans, excluding foraminifera) is limited to only a few areas and shallow water (< 100 m) ecological studies are limited to the Antarctic Peninsula region, including Signy Island (Vanhove et al., 1998, 2000), King George Island (Skowronski and Corbisier, 2002; Petti et al., 2006; Pasotti et al., 2012, 2014), and Adelaide Island (Fonseca et al., 2017); and the Ross Sea (Danovaro et al., 1999; Fabiano and Danovaro, 1999). There have also been some studies of deeper water Antarctic meiobenthos: in the Weddell Sea, Ross Sea, Bransfield Strait and Drake Passage (Vanhove et al., 1995, 1999; Lee et al., 2001a; Veit-Köhler et al., 2018). Almost nothing is known of meiobenthic communities from the majority of the Antarctic coast or shelf.
The structure, composition and diversity of Antarctic meiobenthic communities are poorly understood, especially in East Antarctica. Meiobenthic studies in Antarctica, particularly the Antarctic Peninsula, have focused on their distribution (Danovaro et al., 1999; Vanhove et al., 2000; Petti et al., 2006; Pasotti et al., 2014), relationship to environmental influences and food availability (Vanhove et al., 1998; Skowronski and Corbisier, 2002; Pasotti et al., 2012), recolonization following disturbance (Peck et al., 1999; Lee et al., 2001b; Stark et al., 2017), and more recently in the context of ice-shelf disintegration and collapse (Rose et al., 2015). In most polar regions there is a pronounced dominance of nematodes, which comprise 80 to 95% of the community; the next most abundant component is usually copepods (< 1 to 27%), based on available literature. This dominance is thought to be due to the ability of nematodes to adapt, with high diversification and niche segregation between many genera and species, and generally being resilient to varied environmental conditions (Lee and Van de Velde, 1999). Worldwide, nematode genera respond to a number of environmental factors such as organic content of the sediments (i.e., food availability), grain size, physical disturbance (Ingels et al., 2009, 2011a,b; Ingels and Vanreusel, 2013) and this is also the case in the Antarctic (Vanhove et al., 1998, 2000; Lee et al., 2001a, b). Meiofauna in polar regions display large spatial variability, but the parameters controlling their distribution and community structure are unclear (Fabiano and Danovaro, 1999). One of the problems with examining meiofaunal communities is that the vast majority of species remain undescribed (Boucher and Lambshead, 1995) and this is particularly true for Antarctica (e.g., nematodes, Ingels et al., 2014). Thus most studies focus on coarser level taxonomy such as genus or family.
Coastal benthic habitats are among the most productive of marine environments (Suchanek, 1994; Borum and Sand-Jensen, 1996). They typically have high nutrient concentrations, which are influenced by both terrestrial nutrient sources and coastal phytoplankton production. In Antarctica, however, terrestrial nutrient supply is extremely limited or almost entirely absent as there are no rivers and little terrestrial primary production. In Antarctic coastal areas nutrients are relatively high throughout the year and are only briefly lowered during summer (McMinn and Hodgson, 1993; McMinn et al., 1995). Almost nothing is known of meiofaunal communities from the vast majority of the Antarctic coast, as most studies to date are from the South Shetland or South Orkney Islands.
Casey station, in the Windmill Islands in East Antarctica, is situated in a permanently ice free area of low-lying rocky peninsulas and islands. This area comprises a range of benthic habitats (Stark, 2000), from partially ice-covered, exposed coast dominated by macroalgae, to mostly ice-covered and sheltered embayments dominated by invertebrate communities including sponges, ascidians, tubeworms, echinoderms and other invertebrates (Thompson et al., 2007). Fragmented in nature, and sensitive to change owing to the adapted benthic communities, shallow water coastal habitats in Antarctica are considered to be rare and important for maintaining ecosystem function in coastal Antarctica (Clark et al., 2015). Information on the spatial distributions of benthic communities and understanding of their response to change and subsequent recovery is important given that human activities are concentrated in these types of habitat in the Antarctic (Clark et al., 2015).
The aims of this study at Casey Station were to: (1) determine the composition, diversity and spatial variability of nematode and copepod meiobenthic communities in shallow marine sediments. Spatial variation was examined at three scales: Locations (kms); Sites within locations (∼100 m); and Plots within sites (∼10 m); (2) to examine the relationship between nematode and copepod communities and local environmental influences; and (3) to investigate human impacts on nematode and copepod communities by comparing three control locations and three locations adjacent to waste disposal sites and exposed to pollution.
Materials and Methods
Sampling Design
Sampling was undertaken using a hierarchical, nested design with three spatial scales, Locations (separated by kms); within each location there were two sites (∼ 100 m apart) and at each site there were two plots (∼10m apart). Within each plot (1m diameter), two replicate cores were taken for meiofauna and two for environmental analysis, making a total of 8 meiofauna and 8 environmental cores per location, except at O’Brien Bay-5 where one meiofauna core was lost during sampling. Six locations were sampled around Casey Station. There were three control locations, two of which were within O’Brien Bay to the south of Casey [O’Brien Bay-1 (OB-1) and O’Brien Bay-5 (OB-5)]; and one within Newcomb Bay, in McGrady Cove (Figure 1). There were three locations adjacent to waste disposal sites: two locations were situated along a gradient of pollution within Brown Bay (Inner and Middle) (Stark et al., 2004; Stark, 2008); and a third location was at Wilkes, adjacent to the abandoned waste disposal site at the derelict Wilkes station (Stark et al., 2003a), all within Newcomb Bay (Figure 1). These waste disposal sites were used historically to dispose of all waste and rubbish generated on station and included used oil, building materials, electronics and batteries, food, clothing and chemicals (Snape et al., 2001; Stark et al., 2006). Both waste disposal sites are contaminated with metals and hydrocarbons above background levels (Stark et al., 2008, 2014b; Fryirs et al., 2015).
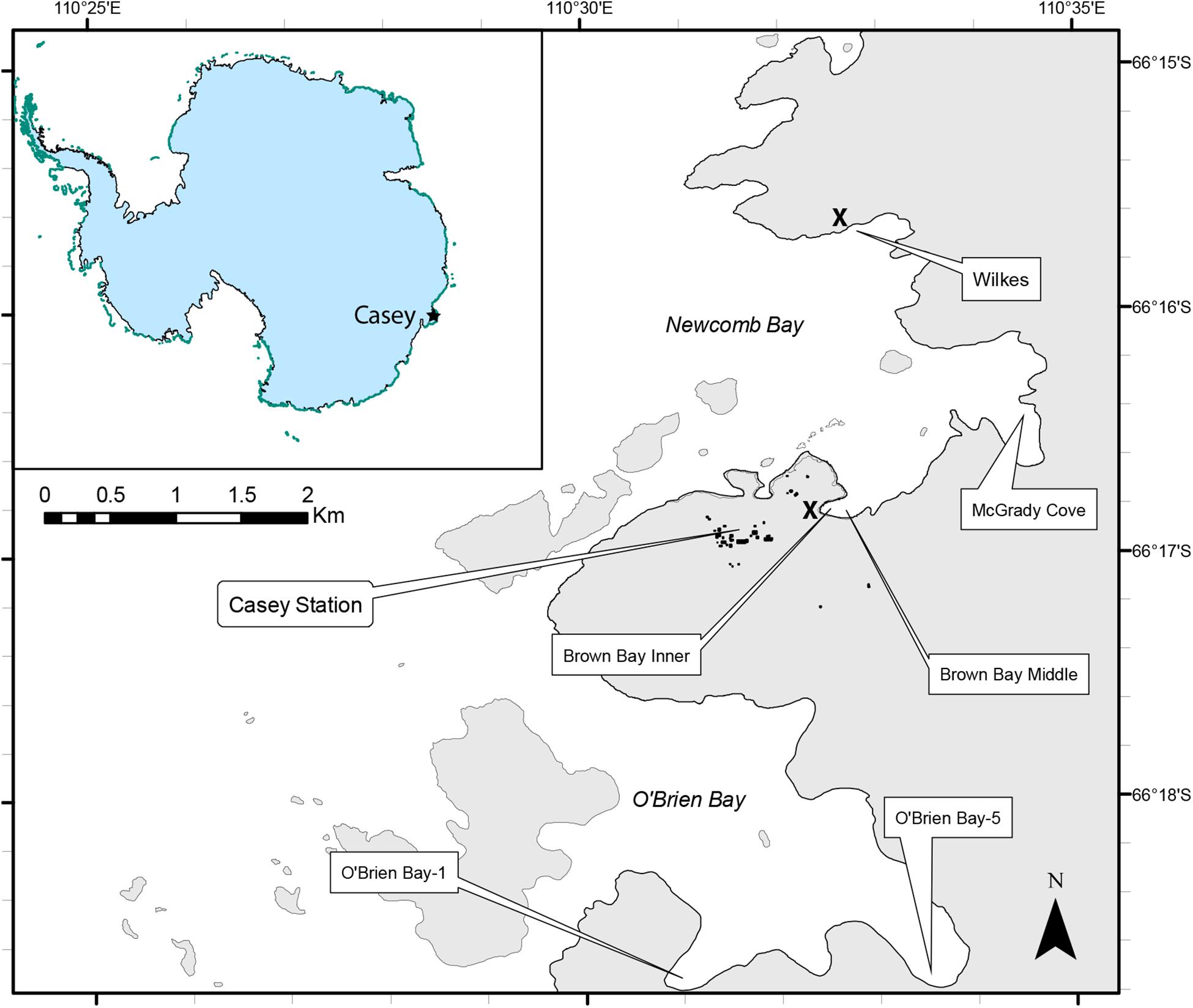
Figure 1. Map of sampling locations around Casey Station, East Antarctica. The approximate locations of the former waste disposal sites at Casey and Wilkes stations are shown by the X symbol.
Location Description
Casey Station is situated at 66°17′ S, 110°32′E on Bailey Peninsula in the Windmill Islands, East Antarctica (Figure 1). The shallow (< 50 m) benthic marine environment in the near shore region at Casey is very heterogeneous, comprising a mosaic of sediments of varying grain sizes, gravels, cobbles, boulders and bedrock (Stark et al., 2003b). All locations have relatively similar sea ice regimes, although there can be considerable local variation in timing of ice break out and duration of periods of open water. Wilkes usually has an earlier sea ice loss than the other locations by between 2 and 6 weeks. Bathymetry varies significantly in the area, but within the two large bays in the study the bathymetry is similar, with deep basins at around 90 m depth, and the small bays around the edges, where samples were taken, usually have a maximum depths of approximately 25 to 30 m. Very little is known of oceanographic patterns in the region but all the bays in this study are considered to be connected and experience similar tidal and oceanographic regimes. Sediment properties are presented in the results, but general conditions at each location are described below.
Brown Bay
Brown Bay is a small embayment at the southern end of the larger Newcomb Bay with rocky sides grading to a muddy bottom to a maximum depth of approximately 15 m. Brown Bay is typically ice-free for 1–2 months in a year, between January and March. It is adjacent to the former Casey waste disposal site, abandoned in 1986, which was on the foreshore of the bay, at the base of Thala Valley. Samples were taken from 2 locations within Brown Bay: Brown Bay Inner at a depth of 7 m was 30 m from the waste dump directly in front of the point where summer melt water from the valley enters the bay (Stark et al., 2006). Brown Bay Middle was 150 m from the waste dump and ranged from 12 to 15 m in depth. Brown Bay is polluted with metals and hydrocarbons which came from the former waste disposal site situated on the foreshore of the bay (Stark et al., 2003b, 2005).
Wilkes
Wilkes was the first research station built in the Windmill Islands area, by the United States in 1957 on Clark Peninsula on the shore of Newcomb Bay; it was abandoned in 1969. The Wilkes waste disposal site is on the foreshore of a small embayment on the northern side of Newcomb Bay. The marine benthic environment adjacent to the site consists of sandy sediments and areas of exposed rock covered with macroalgae in summer (Stark et al., 2003b). Samples were collected approximately 50 m from shore, approximately 200 m from the front of the waste dump, in 10–15 m depth. Sediment metal levels are generally similar to control locations except for cadmium, although hydrocarbons have not been measured at this location (Stark et al., 2003b).
O’Brien Bay
O’Brien Bay is a large bay several kilometers south of Casey station and is visually unaffected by human activities or contamination. The benthic environment consists of slopes and terraces of rock, boulders, cobbles and gravel, with patches of sediment dispersed among them (Stark et al., 2003a). O’Brien Bay-1 and O’Brien Bay-5 are small embayments within O’Brien Bay, with rocky sides sloping to mixed habitat of rock and sediment patches. Samples were taken at depths of 12–18 m.
McGrady Cove
McGrady Cove is a small embayment within the larger Newcomb Bay and is not directly affected by human activities or contamination. Samples were taken on the northern side of the bay which is bordered by ice cliffs which extend to the seabed at depths of 5–7 m. The benthic habitat consists of patches of rocky reef and boulders interspersed with large patches of muddy sediments. Samples were collected in 12–18 m water depth.
Sample Collection, Meiofauna Preparation, and Identification
Sediment samples were collected by divers using modified 60 ml syringes with their intake end cut off to form a small core tube (28mm internal diameter). Cores were pushed into the sediment to a depth of 10 cm, extracted, and the bottom end was capped. In a few cases samples were only taken down to 5–7 cm, where sediments were less than 10 cm deep due to underlying rock. No sediments less than 5 cm deep were sampled. Samples were collected between 25 November and 14 December 2005.
Cores were transported to Casey Station laboratories where they were emptied into sample jars and 4% formalin was added to each sample. Prior to processing, each sample was washed through a 500 μm sieve to remove the macrofauna and the coarser sediment fraction. A 32 μm sieve was used to retain the meiofauna size fraction. Meiofauna were extracted through a modified gravity gradient centrifugation technique (Heip et al., 1985; Pfannkuche et al., 1988) using a% solution of Ludox HS40 and Ludox AS in distilled water (Witthoft-Muhlmann et al., 2005). Ludox is a silica sol (a colloidal solution of Si02) which causes no plasmolysis. Samples were rinsed thoroughly over a sieve of 32 μm with tap water to prevent flocculation of Ludox. The samples were then transferred from the sieve to a large centrifuge tube. Ludox was diluted with water to specific gravity 1.18 g/ml (60% Ludox and 40% water; density = 1.18) and added to each tube until the level of the mixture was balanced for centrifuging. The sample was then centrifuged at 2800 rpm for 10 min. The supernatant was decanted and collected, and the remaining sediment pellet was resuspended. This process was repeated three times.
All supernatants were filtered on a 32 μm sieve, which was rinsed with tap water to avoid a reaction between the Ludox and formalin. After the extraction, 4% formalin and 1% of Rose Bengal (to facilitate counting) was added to preserve meiofauna before identification.
Nematodes and copepods retained on the 32 μm sieve were counted and sorted using a dissecting microscope at 25× magnification (Zeiss Stemi 2000; Zeiss Inc., Germany). Two hundred nematodes per sample were picked out at random and mounted on slides in glycerine after a slow evaporation procedure (modified after Riemann, 1988) and identified to genus level using Platt and Warwick (1983, 1988) and Warwick et al. (1998) and NeMys online identification (Steyaert et al., 2005). All copepods were picked out and mounted on slides in glycerine without evaporation for identification to family level using THAO: the Taxonomische Harpacticoida Archiv Oldenburg 2005 and Bodin (1997). The identification of nematodes and copepods was conducted on a compound microscope (1000 x magnification).
Environmental Variables
Sediment samples were taken for analysis of grain size, metals and total organic matter (TOM) using a 5 cm diameter core pushed 10 cm into the sediment. Cores were frozen at −20°C until analysis. Each core was subsampled from the top 5 cm of the frozen core, which was then homogenized by stirring and then subsampled further for separate analysis of grain size, metals and TOM. Full details of analytical methods can be found in Stark et al. (2014a) and are briefly summarized below.
Total organic matter was calculated by mass-loss on ignition at 550° for 4 h to determine ash free dry weight following Heiri et al. (2001), on a 2 g homogenized wet sub-sample, from 2 replicate cores in each plot for a total of 4 cores per location.
Grain size analysis: The outer 5 mm edge of the top 5 cm of the core was removed with a scalpel blade and dried at 45°C, then sieved through a 2mm sieve. The < 2 mm fraction and the > 2 mm fraction were weighed separately. A 5 g sample of the < 2mm fraction was analyzed using a Mastersizer 2000 Particle Size Analyzer with Hydro 2000MU accessory at the Department of Physical Geography, Macquarie University, Sydney.
Analysis of metals in sediments were done on a 3 g sub-sample of homogenized wet sediment. A 1:10 w/v 1 M HCl digest was used as recommended by Scouller et al. (2006), which gives an estimate of bioavailable elements and those more likely to have an anthropogenic source. Samples were analyzed by ICP-MS at the Central Science Laboratories (CSL), University of Tasmania for a suite of ions which included: Sr, Mo, Ag, Cd, Sn, Sb, Pb, Mg, Cr, Mn, Fe, Co, Ni, Cu, Zn, As, Al, Ba.
Statistical Methods
Abundances of nematodes were standardized to the total number per sample and the proportion of each genera was multiplied by the total number of nematodes in each sample to estimate their abundance per core. Multivariate analyses were performed on merged nematode and copepod abundances after square-root transformation as well as on separate nematode and copepod community data. Data was square root transformed to reduce the effect of dominant taxa and the Bray-Curtis resemblance measure was used. Multivariate analyses of community composition were undertaken using non-metric multidimensional scaling (nMDS), PERMANOVA, Analysis of Similarities (ANOSIM), group-average CLUSTER (including SIMPROF 5%), PERMDISP and similarity percentages (SIMPER) procedures using the PRIMER v7.0.13 statistical software package (Clarke and Gorley, 2006; Anderson et al., 2008). Spatial differences between communities were analyzed in a hierarchical 3-factor design with Location (fixed factor), Site (nested in location) and plot (nested in site). To compare control and potentially impacted locations, a series of planned contrasts were done using PERMANOVA, to further partition the variation within the Location level (Anderson et al., 2008). Brown Bay (Inner and Middle) was compared to the control locations (McGrady, O’Brien Bay-1 and -2); and Wilkes was compared to the control locations, in planned contrasts. This allows a higher level comparison of control and impacted locations without potentially confounding different impacts at Brown Bay and Wilkes by combining under the same factor of impact. The null hypotheses of no relationship among samples or variables was tested using P-values from permutation techniques [if less than 100 permutations were available Monte Carlo routine was used (Anderson and Robinson, 2001)]. The Pseudo-F ratio and p-value (from permutation) were calculated using type III sums of squares and permuting residuals under a reduced model. PERMDISP and one-way ANOSIM was performed in post hoc tests to determine which groups were different and if differences were due to multivariate location or dispersion. PERMANOVA was used to examine the relative variance component contribution of each factor to overall variance, as an estimate of magnitude of effects (Graham and Edwards, 2001).
The relationship between meiofaunal communities and environmental variables was examined using distance-based linear modeling (DSTLM) and visualized using distance-based redundancy analysis (dbRDA) to obtain an ordination of the fitted values from the selected model (Anderson et al., 2008), as well as by overlying vectors of environmental variables on community multivariate ordinations. A stepwise and a forward selection process were used in DISTLM with the AICc model selection criteria, to model the community data (Bray Curtis resemblance matrix) against the normalized environmental data which were checked for collinearity (and removed when necessary).
To further distinguish patterns in nematode genera and copepod families a coherence analysis following Somerfield and Clarke (2013) was conducted using PRIMER v7. This analysis distinguishes subsets of taxa (based on SIMPROF on species variables) in the dataset that have coherent abundance patterns across a set of samples or gradient of samples, and can be separated based on significance levels in statistical tests. In essence, the routine finds groups of taxa that have similar responses across a set of samples.
Differences in environmental conditions were explored using Principal Component analysis (PCA). Relationships among variables were visualized using Draftsman plots and skewed variables were transformed (log(x + 1) or square root) to achieve even distributions. Environmental variables with correlations > 0.95 were removed leaving a single variable to represent these groups to prevent over-parameterization. Environmental variables were normalized prior to PCA or DSTLM analysis.
For comparison of nematode and copepod abundances with other studies, data were obtained either directly from published studies or from figures in published studies using the program WebPlotDigitizer V4.11.
Results
Nematode and Copepod Abundance and Taxon Richness
Sediment meiofaunal communities at Casey were dominated by nematodes (95% of abundance), followed by copepods (5%). Other taxa were not included in this study. A total of 38 nematode genera (belonging to 16 families) and 20 harpacticoid copepod families (Table 1) were identified in the 47 samples from the six locations. Nematode abundance was highest at the two control locations in O’Brien Bay (Figure 2). Nematode diversity was greatest at Wilkes (37 genera) but the highest mean number of genera per core was found at OB-5. Copepod abundance was relatively consistent among locations ranging from a mean of 44 to 55 copepods per core (Figure 2). Mean abundance of copepods was not significantly different between locations. The number of copepod families was also relatively consistent among locations, ranging from 15 to 18 (Figure 2). Wilkes was the most taxa-rich location for both nematodes and copepods. OB-1 had the lowest number of taxa with 32 genera of nematodes and 15 families of harpacticoid copepods.
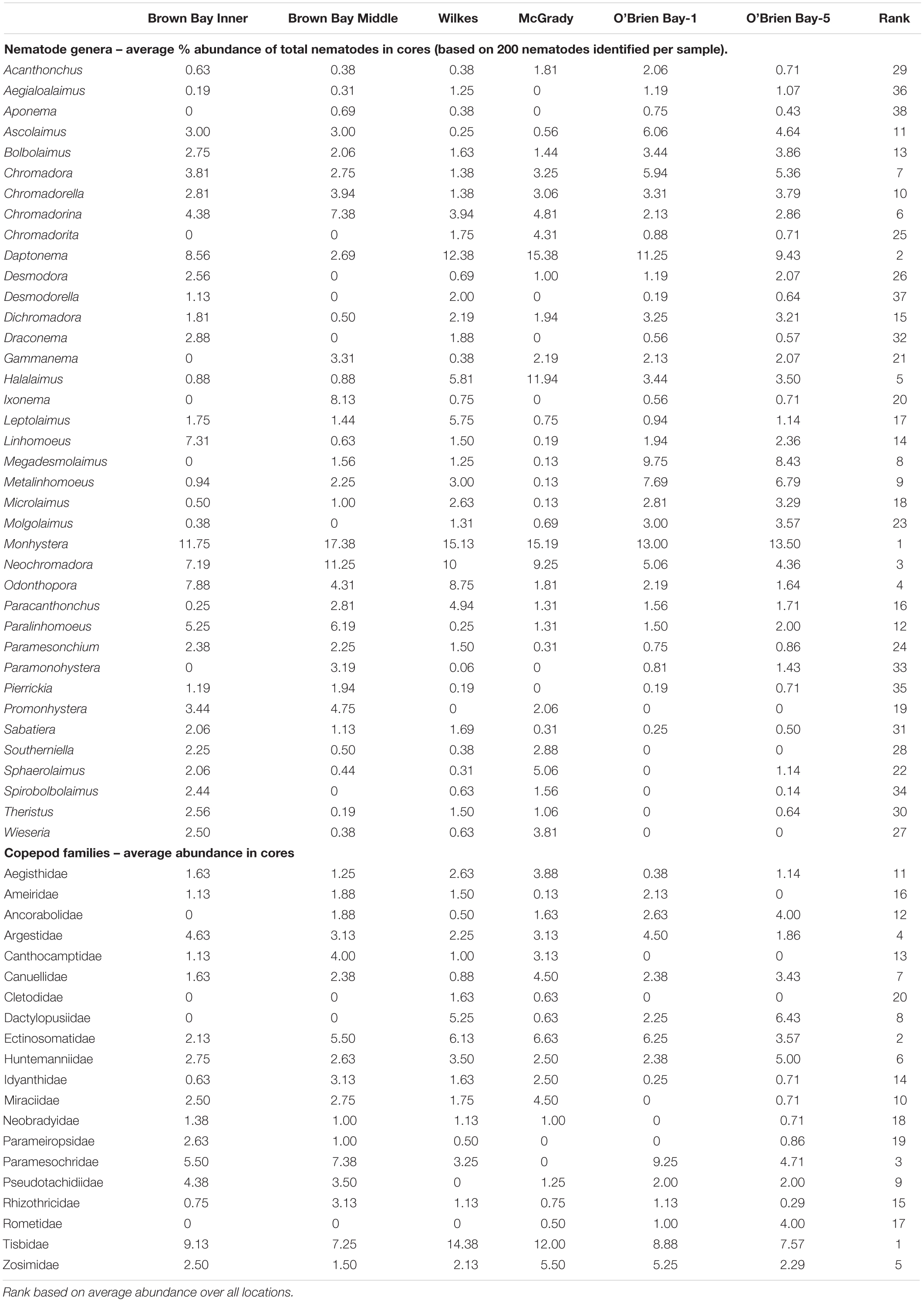
Table 1. Average abundances of nematodes and copepods in cores at six locations at Casey Station (n = 8, except at O’Brien Bay-5 n = 7).
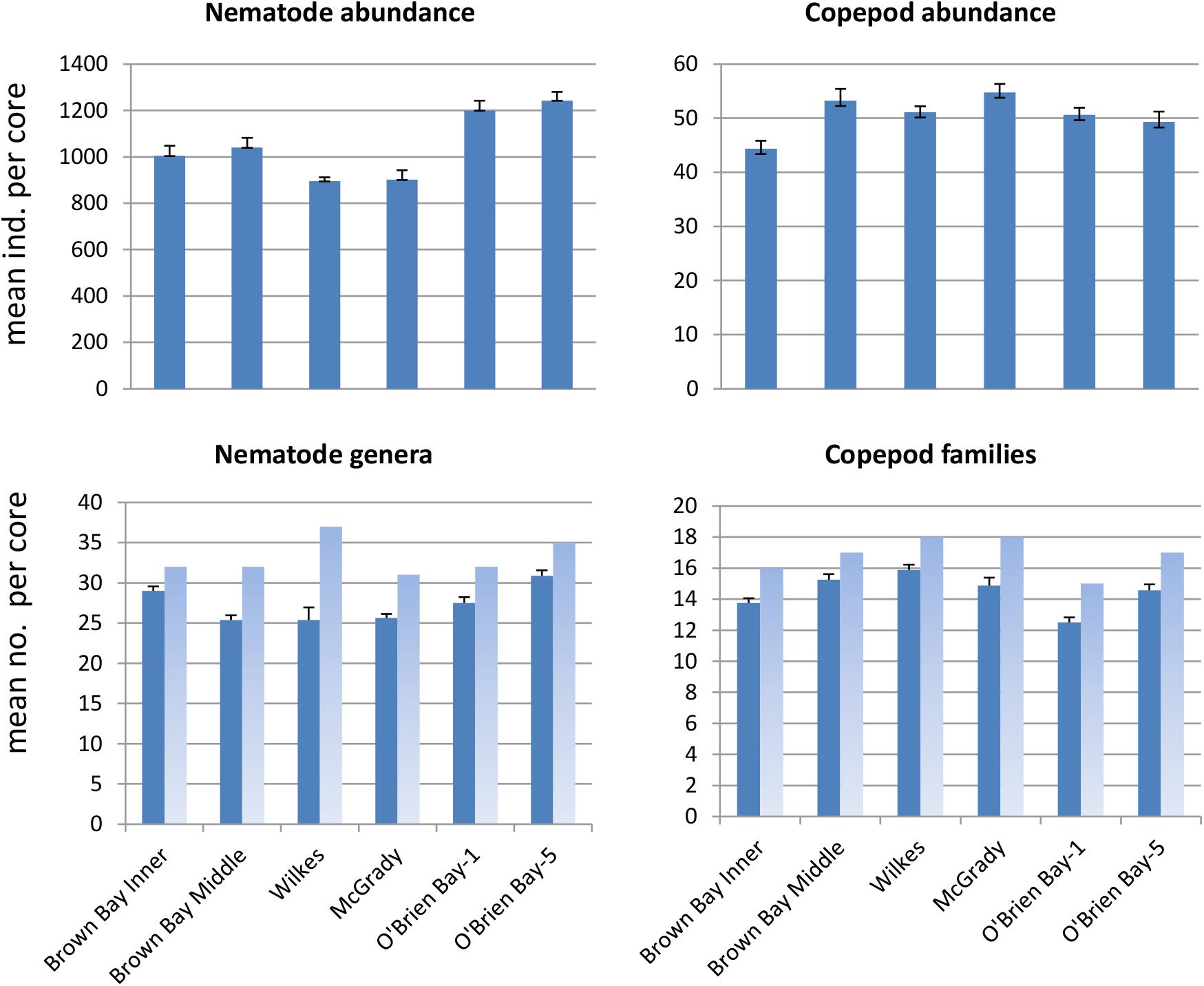
Figure 2. Mean abundance and number of taxa per core at each location (± SE). Dark bars represent mean per core (n = 8, except O’Brien Bay-5: n = 7); light bars represent total number of taxa found at each location.
Nematode abundances ranged from 1.45 to 2.01 × 106 individuals m–2. The control locations in O’Brien Bay had a significantly greater total abundance of nematodes than the impacted and control locations in Newcomb Bay (PERMANOVA p(perm.) = 0.003). Copepod abundances ranged from 0.072 to 0.089 × 106 individuals m–2, and there was no significant differences among locations (Figure 2). The most abundant nematode genera (as % of total abundance averaged across all locations) were Monhystera (13.7%), Daptonema (9.5%), Neochromadora (7.6%), Odonthopora (4.3%), Halalaimus (4.2%), and Chromadorina (4.1%) and the most abundant copepod families were Tisbidae (1%) and Ectinosomatidae (0.5%) (Table 1).
Nematode and Copepod Community Spatial Structure
Multivariate analysis of community composition revealed significant differences at the location scale for both nematode and copepod communities. Patterns for the combined nematode/copepod community data were very similar to those of the nematode community, due to the dominance of nematodes in the assemblages, and thus the nematode and copepod community data were analyzed separately. The location scale contributed most to the overall variation in communities (largest estimated component of variation, ECV) with remarkably distinct meiofaunal communities at each location, as evidenced by the distinct location groupings in the nMDS ordinations (Figure 3). All locations had significantly different copepod and nematode communities (Tables 2, 3), and form non-overlapping groups in the nMDS ordinations (Figure 3). There was also significant variation among sites (100 m scale, copepods only) within locations and among plots within sites (10 m, for nematodes). Analysis of the ECV values revealed that variation among locations accounted for about 70% of the total variation (for copepods and nematodes), with both sites and plots contributing less than the residual variation (< 18%, Figure 3 and Table 2). Variation in copepod communities increased with increasing spatial scale, with no significant variation at the 10m scale. In contrast, nematode communities varied significantly at the 10m site scale, and not at the 100m plot scale (Table 2). Further analysis of community structure was done by PERMDISP which tests for differences in multivariate dispersion, which can affect differences between groups [their multivariate location sensu Anderson (2006)]. There were significant differences in multivariate dispersion for nematodes, with Wilkes more variable than all other locations; and for copepods, with Brown Bay Inner and Middle more variable than all other locations (Table 4). However given that there is no overlap among locations in MDS plots, this difference in variation does not affect the main test of differences between locations. These PERMDISP results could be interpreted as a separate effect, with nematode communities at Wilkes and copepod communities at both Brown Bay locations being significantly more variable than other locations.
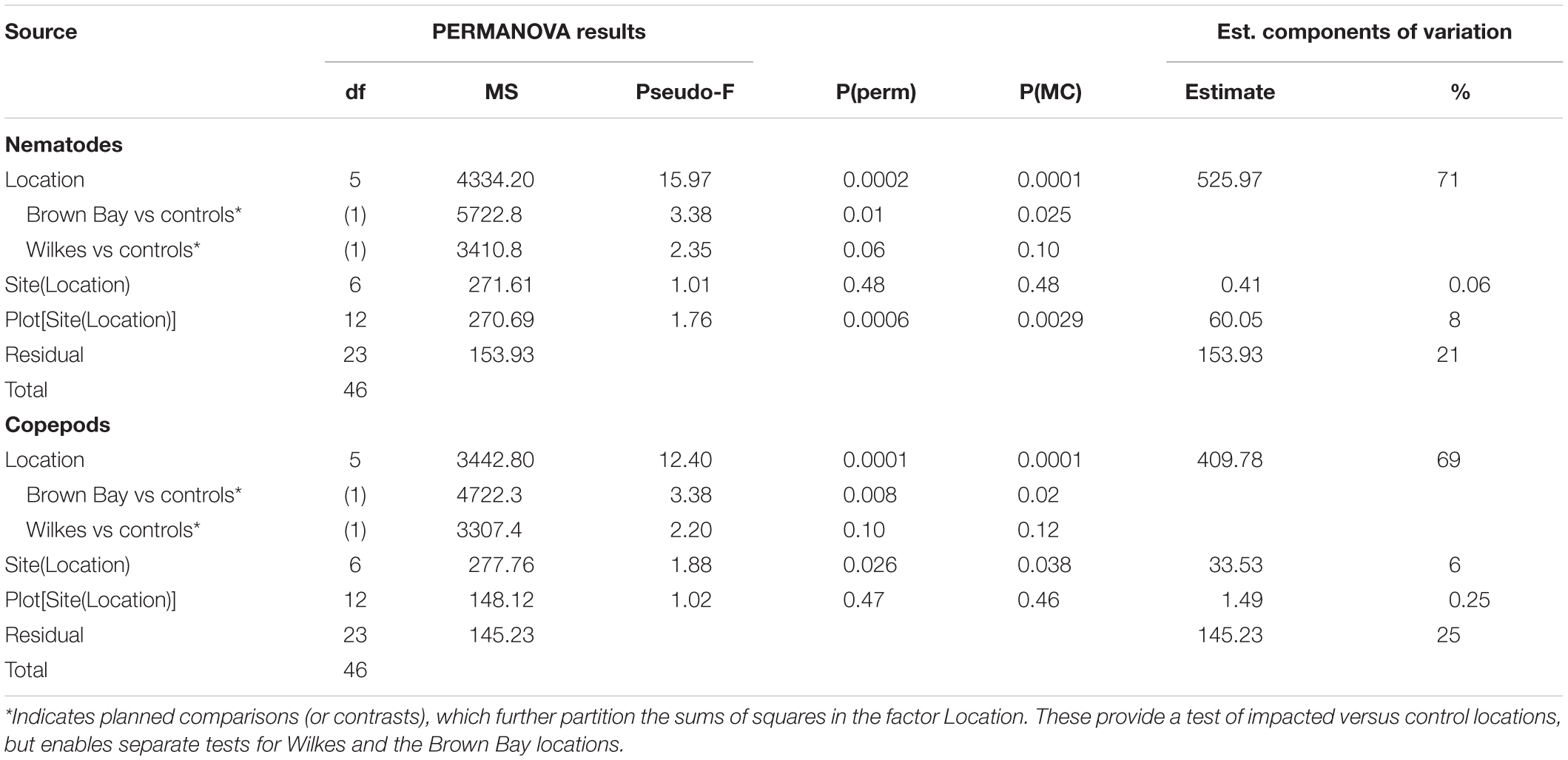
Table 2. Results of PERMANOVA analyses for nematode and copepod communities at Casey Station, with estimated components of variation (ECV) derived from PERMANOVAs.
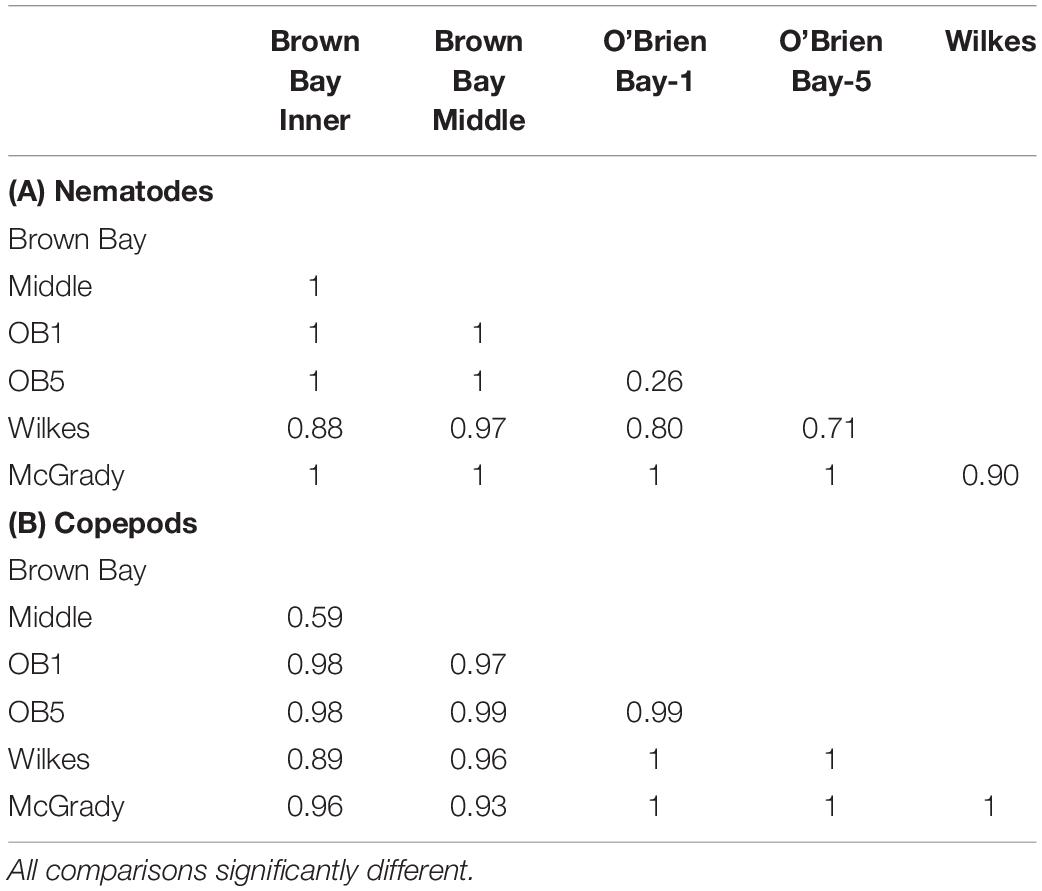
Table 3. Results from ANOSIM analysis showing R values from pairwise tests for differences in meiofauna among locations.
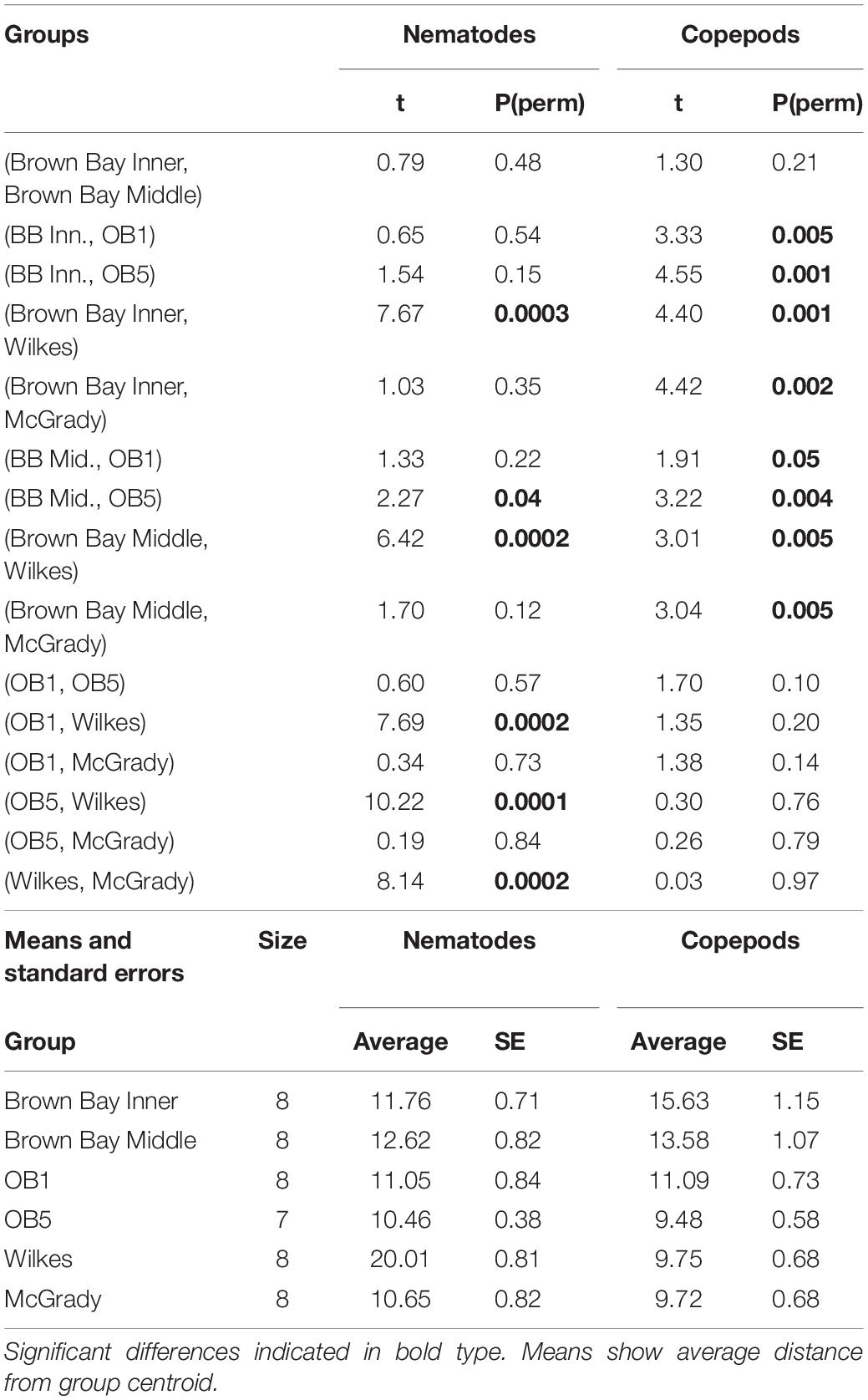
Table 4. PERMDISP analysis of multivariate dispersion in nematode and copepod communities at each location at Casey.
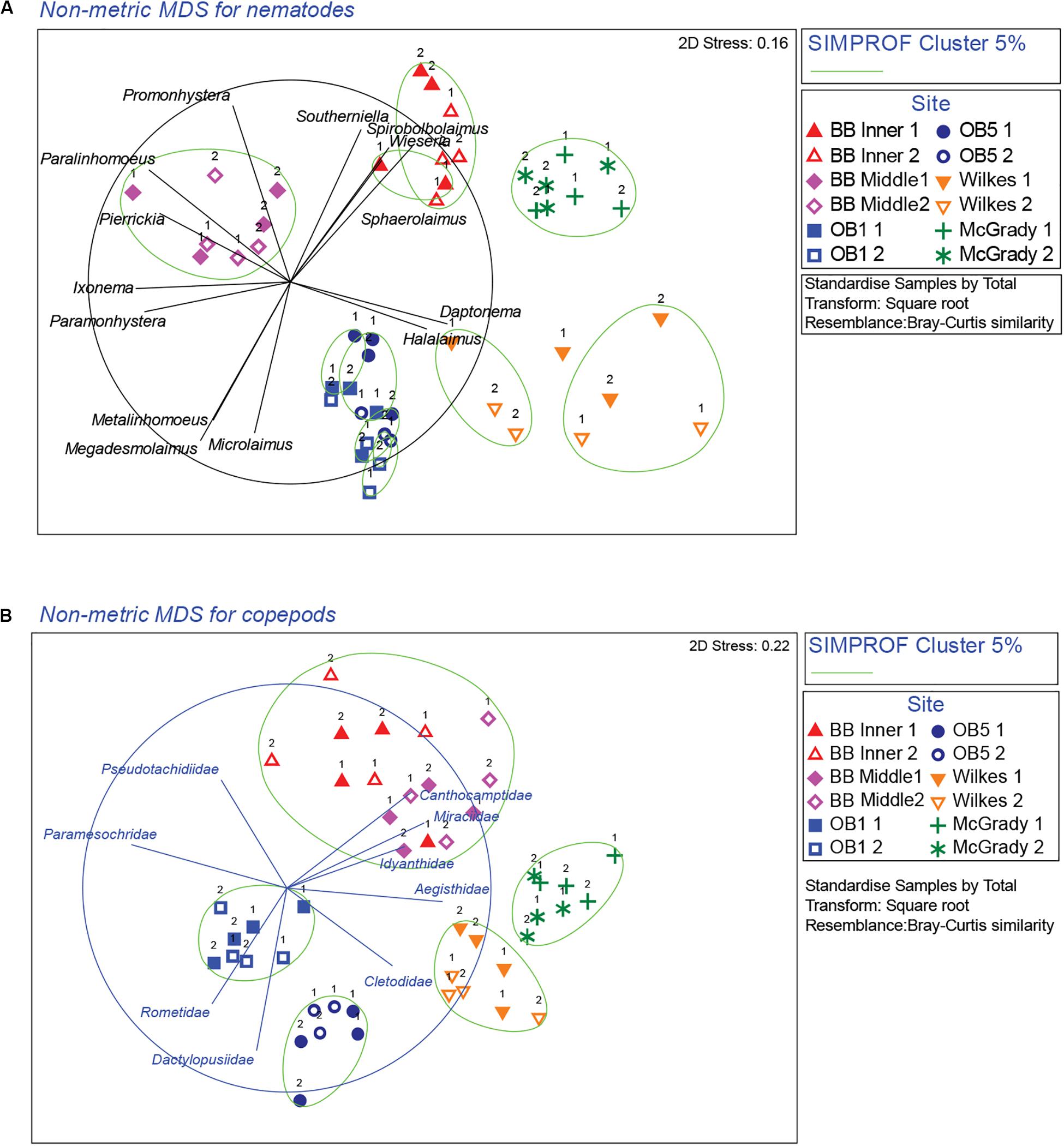
Figure 3. nMDS ordinations of nematode and copepod communities around Casey Station. Numbers next to symbols represent the different plots within each site. The taxa most responsible for contributing to differences among locations were determined using vector plots overlaid on the nMDS and similarity percentage analysis (SIMPER). (A) Nematodes, (B) copepods. Vectors are taxon correlations with nMDS axes (nematode correlation cut-off: 0.7, copepod correlation cut-off: 0.6; with circle representing correlation value of 1). Ellipses on the plot represent significant cluster groups based on SIMPROF analysis.
Human Impacts
The planned comparisons in the PERMANOVA analyses demonstrated that the Brown Bay locations were significantly different to the controls but that Wilkes was not different, for both nematode and copepod communities (Table 2).
The taxa most responsible for contributing to differences among locations were determined using vector plots and bubble plots overlaid on the nMDS and similarity percentage analysis (SIMPER).
Nematodes
Cluster analysis (group average) confirmed the patterns seen in the nMDS ordination, with cluster groups strongly aligned to each location (Figure 3A). Each location had distinct assemblages of nematodes. The species that drove differences among locations most strongly (correlation > 0.7) are shown in the vector plot (Figure 3A), with some of the strongest patterns reflected in bubble plots (Figures 4A,B), indicating strong genera selectivity between locations. In particular the genera Promonhystera, Paralinhomoeus and Pierrickia were consistently more abundant at Brown Bay than the controls, while Daptonema, Halalaimus and Microlaimus were more abundant at controls (Figure 3 and Table 1).
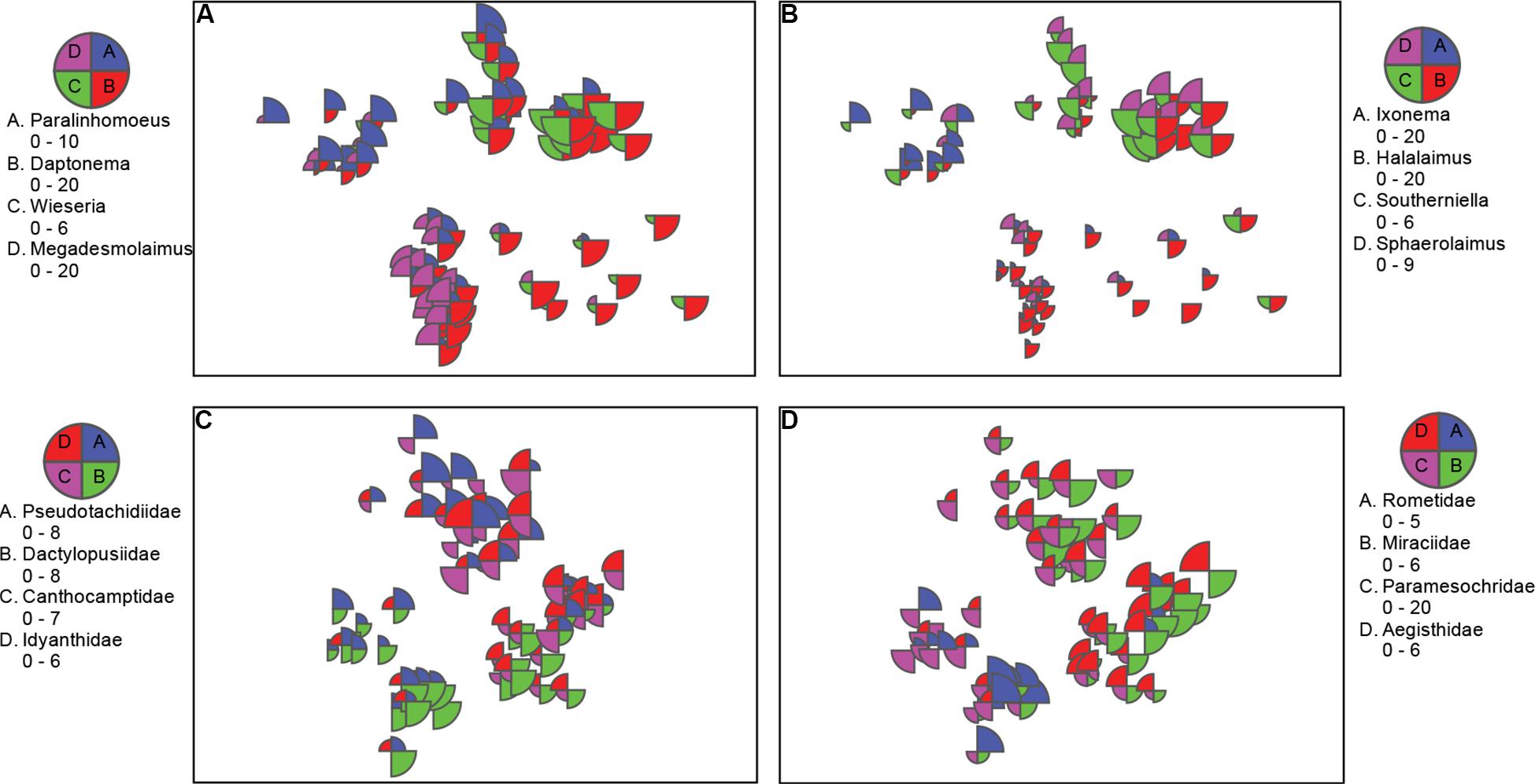
Figure 4. nMDS bubble plots with scaled abundance showing taxa with substantial contributions to location differences. (A,B) Nematode genera from nMDS in Figure 3A; and C,D) copepod families from nMDS Figure 3B. Data was standardized, square rooted, and Bray-Curtis resemblance measure was used.
Coherence analysis of nematode genera indicated five subsets of nematode genera that showed similar abundance patterns across the locations, along a pollution gradient (Figure 5): (1) there was a large group of genera with no distinguishable abundance patterns across polluted and non-polluted locations (Figure 5A); (2) a group of nematode genera that were more abundant at the two O’Brien Bay and the Wilkes locations, and that had either very low abundances or were absent at the Brown Bay locations (Figure 5B); (3) a group of nematode genera that were more abundant at the McGrady location, and had medium to low abundances at the other locations (Figure 5C); (4) nematode genera that were generally more abundant at the polluted Brown Bay Inner location, with medium or high abundance at Wilkes, and medium to low abundances at Brown Bay Middle locations (Figure 5D); and (5) a group of nematode genera that were more abundant at both Brown Bay Inner and Middle, and medium to low abundance at the other locations (Figure 5E).
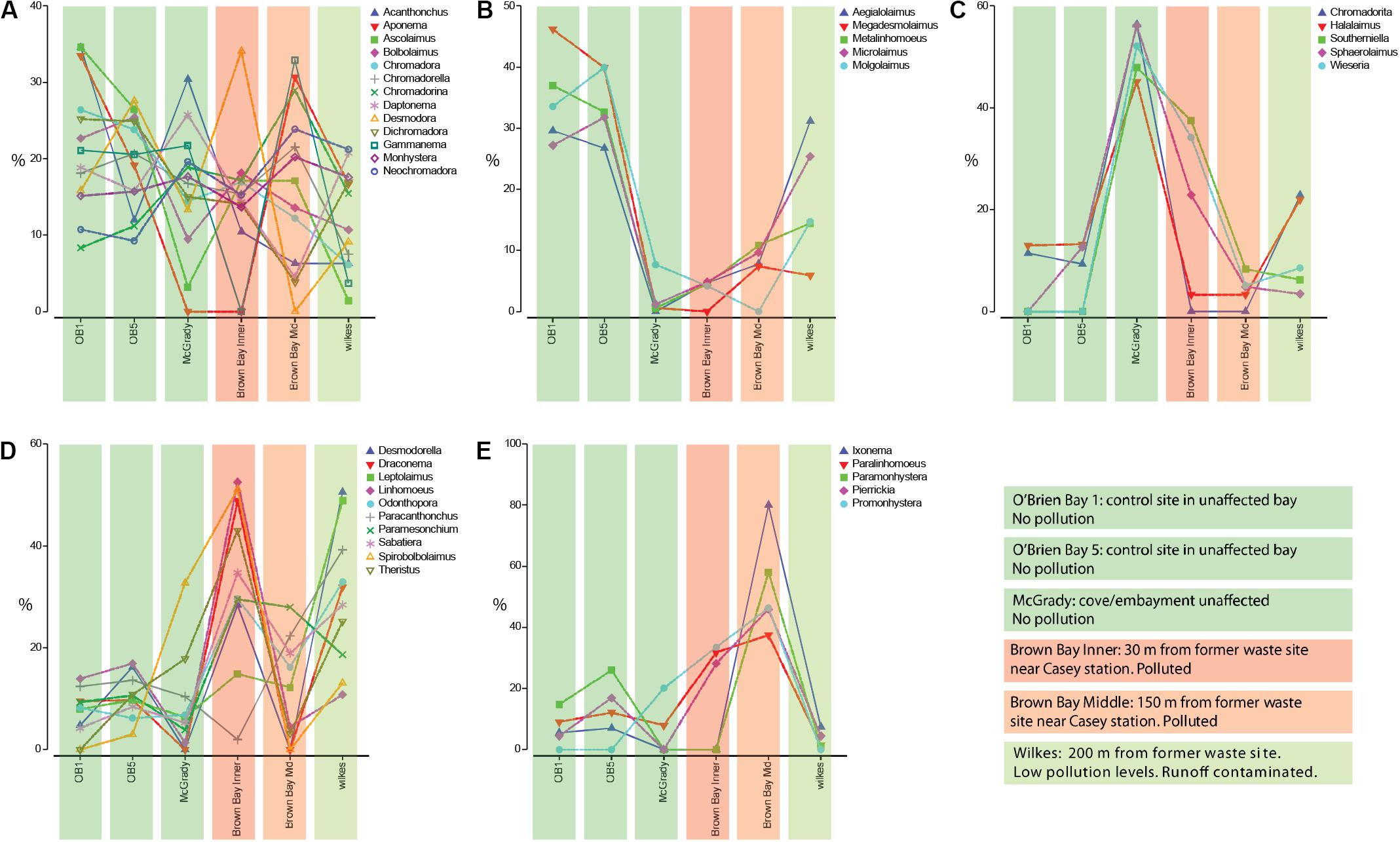
Figure 5. (A–E) Different patterns of abundance from coherance analysis of the 38 nematode genera, standardized by genus across locations, which produced five statistically different subsets of nematode genera. Colored bars are associated with each station and indicate pollution levels as explained in the color legend.
Copepods
Copepod communities were very distinct at each location, with a number of taxa contributing strongly to these differences (Figure 3B). Brown Bay Inner and Middle locations grouped together, demonstrating similarity among copepod communities within Brown Bay, although they also showed the most variation overall (Table 4). Cluster analysis (group average) confirmed the patterns seen in the nMDS ordination, with groups strongly aligned to each location (Figure 3B). Although most taxa were found at each location, there were unique presence and abundance combinations at each location, clearly distinguishing them from each other (Figure 3B, and genera bubble plots in Figures 4C,D). Pseudotachidiidae were consistently more abundant at Brown Bay locations, while Dactylopusiidae and Rometidae were more abundant at controls (Figure 3 and Table 1). In contrast to nematodes, coherence analysis of copepod families did not indicate any general patterns of abundance across locations so these are not shown.
Environmental Influences on Nematode and Copepod Communities
Sediments ranged from very fine sands to medium sands, with generally unimodal distributions, and were poorly sorted. There were significant differences in grain size distribution among locations, with most variation associated with the location scale (approx. 50% of total variance), but no difference between sites within locations, due to differences between plots within sites or residual variation between replicate cores (Table 5). Sediment grain size distributions were most different at O’Brien Bay-1, which had higher proportions of coarser sediments than the other locations (Figure 6) and the largest mean particle diameter MPD (Table 6). In contrast McGrady Cove had the highest proportion of fine sediments, the smallest MPD and high levels of organic matter (Figure 6 and Table 6). The very coarse silt fraction, 31–62.5 μm, was strongly positively correlated with organic content of sediment (r = 0.96).

Table 5. Results of PERMANOVA analyses for grain size of sediments at Casey Station, with estimated components of variation (ECV) derived from PERMANOVAs.
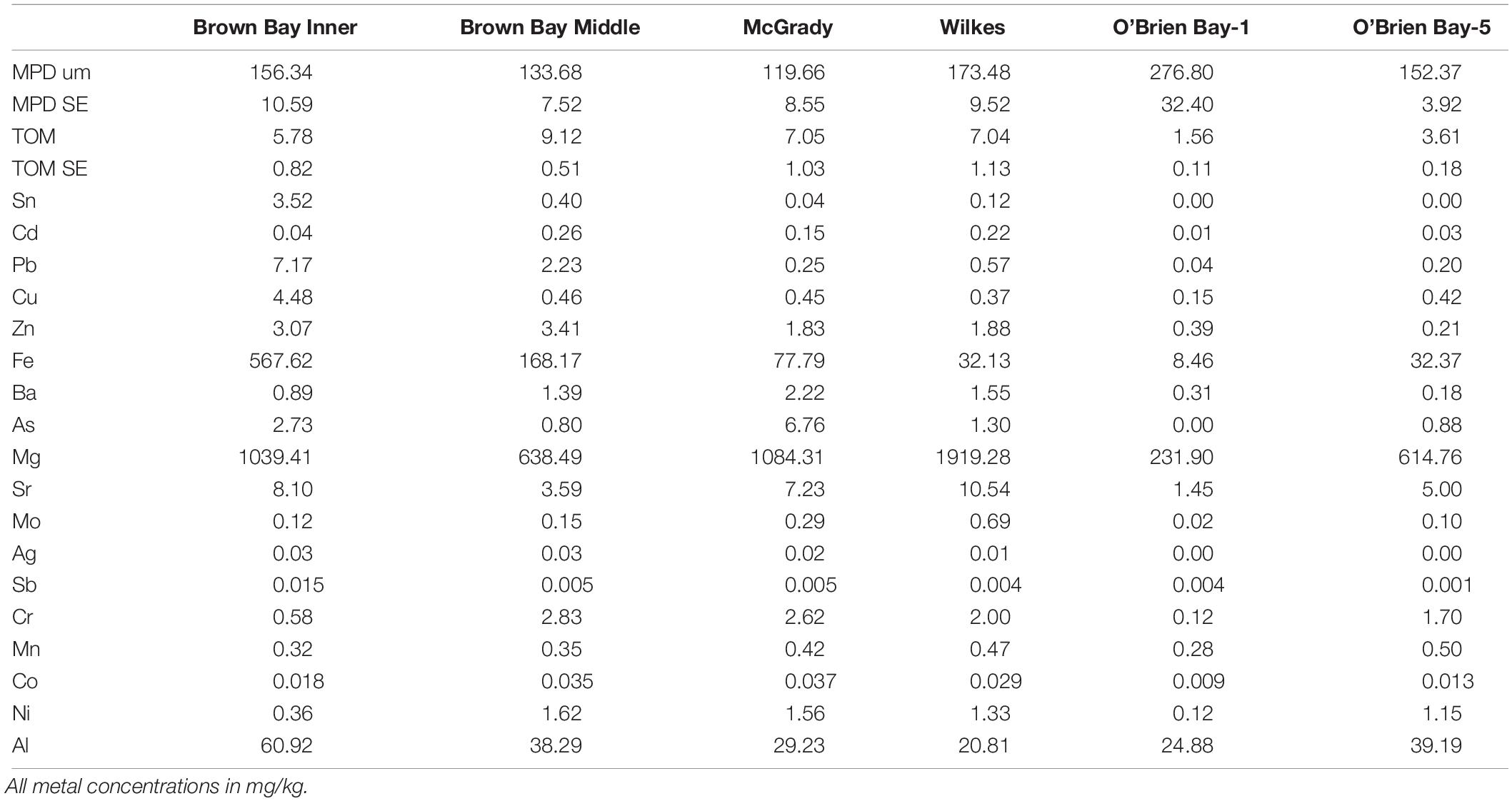
Table 6. Sediment properties at locations used in this study including: MPD = mean particle diameter; SE = standard error; TOM = total organic matter.
Multivariate analysis of sediment environmental variables including grain size, TOC and metal concentrations showed strong differences between locations (Figure 7). A PCA of all environmental data shows most locations as distinct groups, with OB-1 being most separate from all other locations (Figure 7A). Results of ANOSIM analysis (Table 7) demonstrated that all locations were significantly different. When separating grain size and metal variables these patterns changed slightly, with less differences among locations in grain size and greater differences in metals. For grain size, essentially two groups can be distinguished: OB-1 was a highly variable group on its own with the rest of the locations forming a loose grouping together (Figure 7B), yet, within the latter, the distinction of separate locations was still evident (Figure 7B). There were also strong differences between the two sites at Wilkes. For metal concentrations, both sampling locations in Brown Bay were clearly separate from the other locations in the PCA ordination (Figure 7C) with particularly high concentrations of iron, tin, copper, lead, antimony, silver and zinc. All locations had maximal differences in ANOSIM tests, baring Brown Bay Middle and McGrady (Table 7). OB-1 also formed a separate metal group but was much more similar in its metal content to OB-5. There was a gradient of metal concentration aligned with the PC2 axis, increasing toward the top of the ordination (Figure 7C).
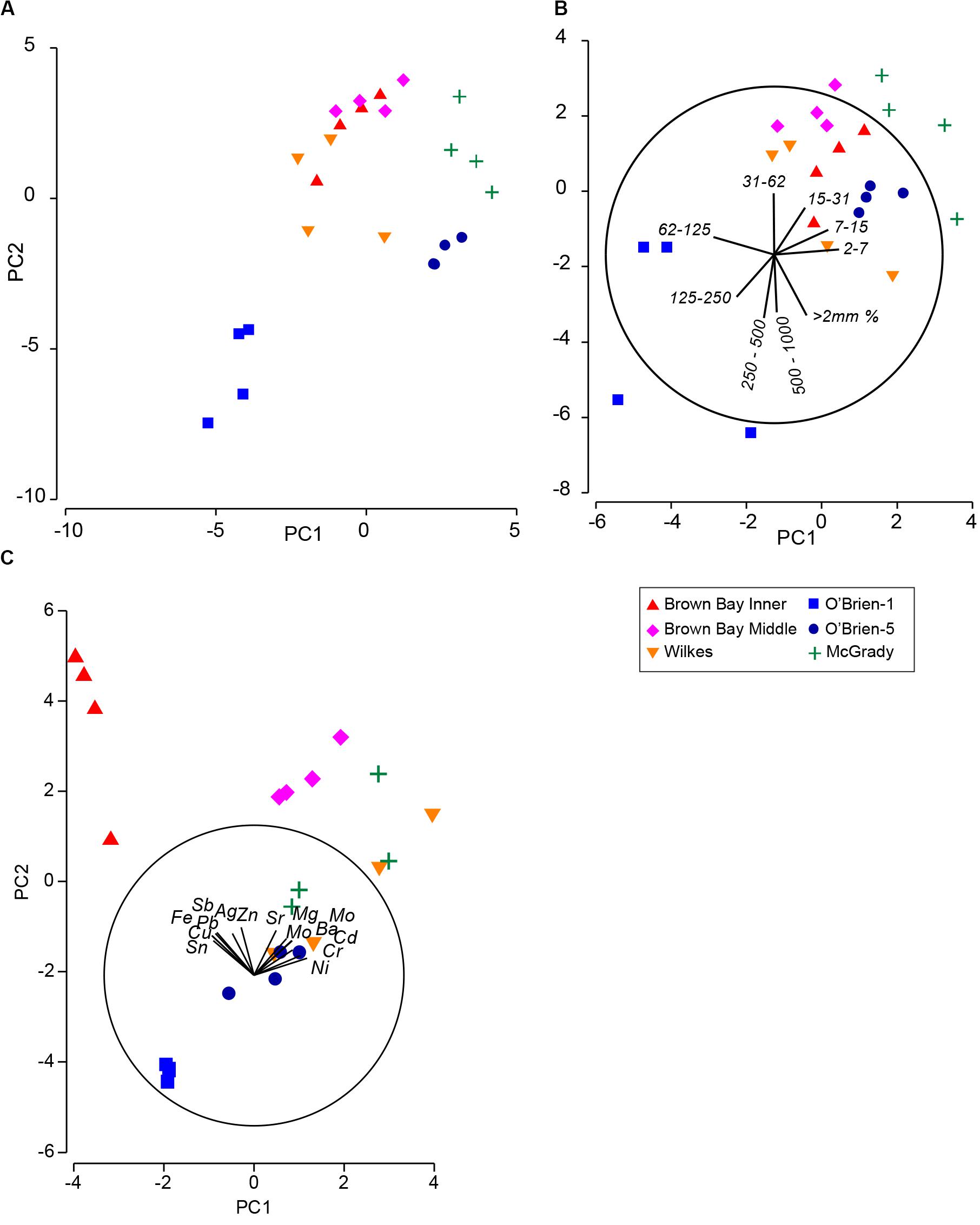
Figure 7. (A) PCA ordination of combined environmental variables; (B) PCA ordination of sediment grain size variables; (C) PCA ordination of metal concentrations in sediment. Vector plots indicate the direction and size of the correlation between PC axes and variables.
Relationships between the meiofauna communities and the environmental variables were assessed using the DISTLM modeling procedure. DISTLM results are shown in Table 8, and patterns are visualized in the PCO (copepod and nematode communities), and dbRDA plots (copepod and nematode communities modeled against environmental variables) in Figure 8. To avoid multi-collinearity (where two or more variables are highly co-linear with correlation |r| ≥ 0.95 for example), whereby variables contain the same information and are redundant for the purposes of the dbRDA analysis (Anderson et al., 2008), one or more redundant variables were dropped from the analysis. Correlations between all variables were examined and where |r| ≥ 0.95 one variable was removed to be represented by the other variable to avoid multi-collinearity and over-parameterization. These were: tin was used to represent lead and antimony; zinc was used to represent vanadium; copper was used to represent iron; nickel to represent chromium; strontium to represent magnesium, 31–62.5 μm to represent TOC; 250–500 μm to represent mean particle diameter; and 2–7.8 μm to represent 0.01–2 μm. The best DISTLM models correspond strongly with the unconstrained PCO ordination of nematode and copepod communities (Figure 8).
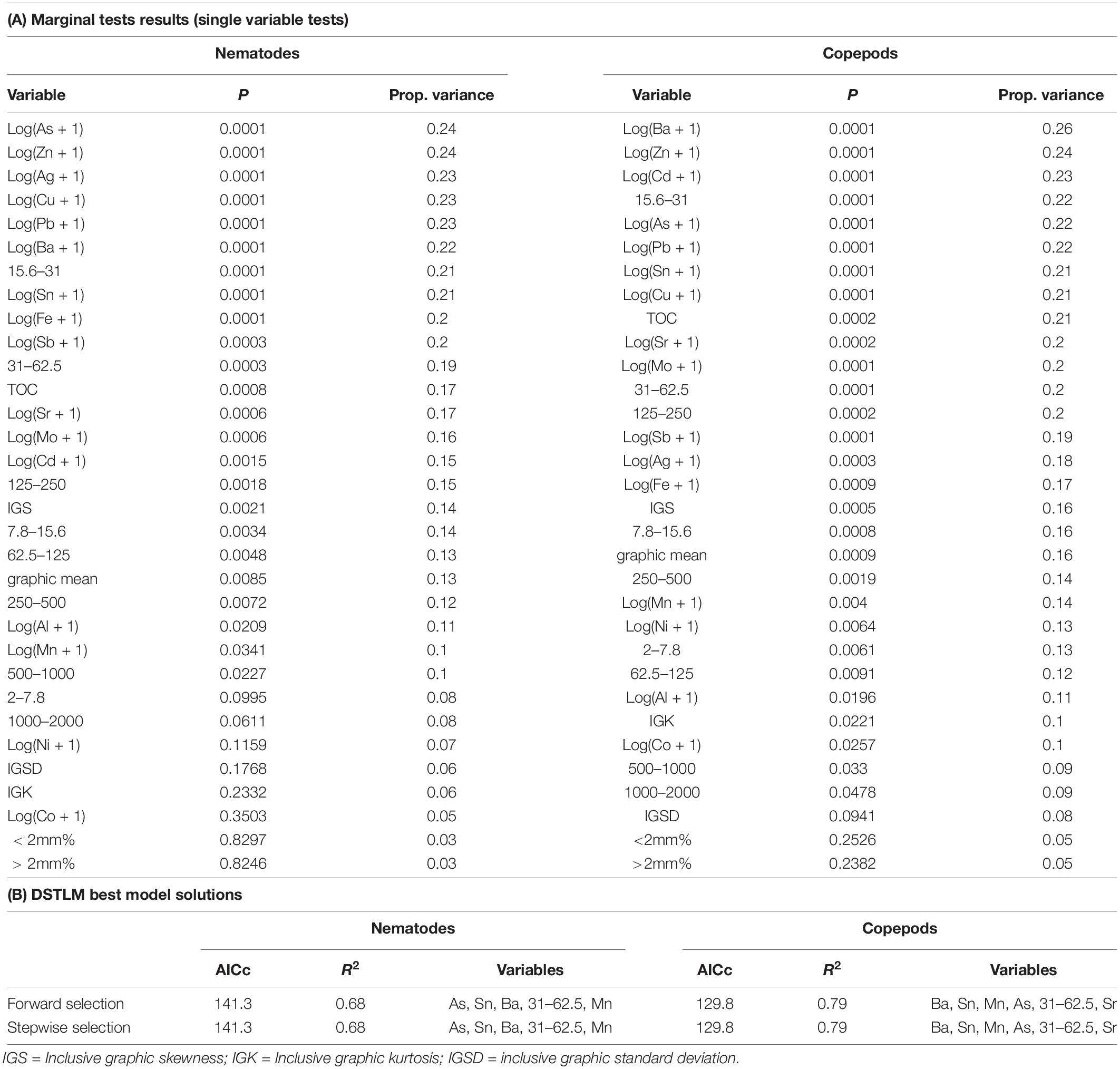
Table 8. Results of distance-based linear modeling (DISTLM) of environmental variables against nematode and copepod communities.
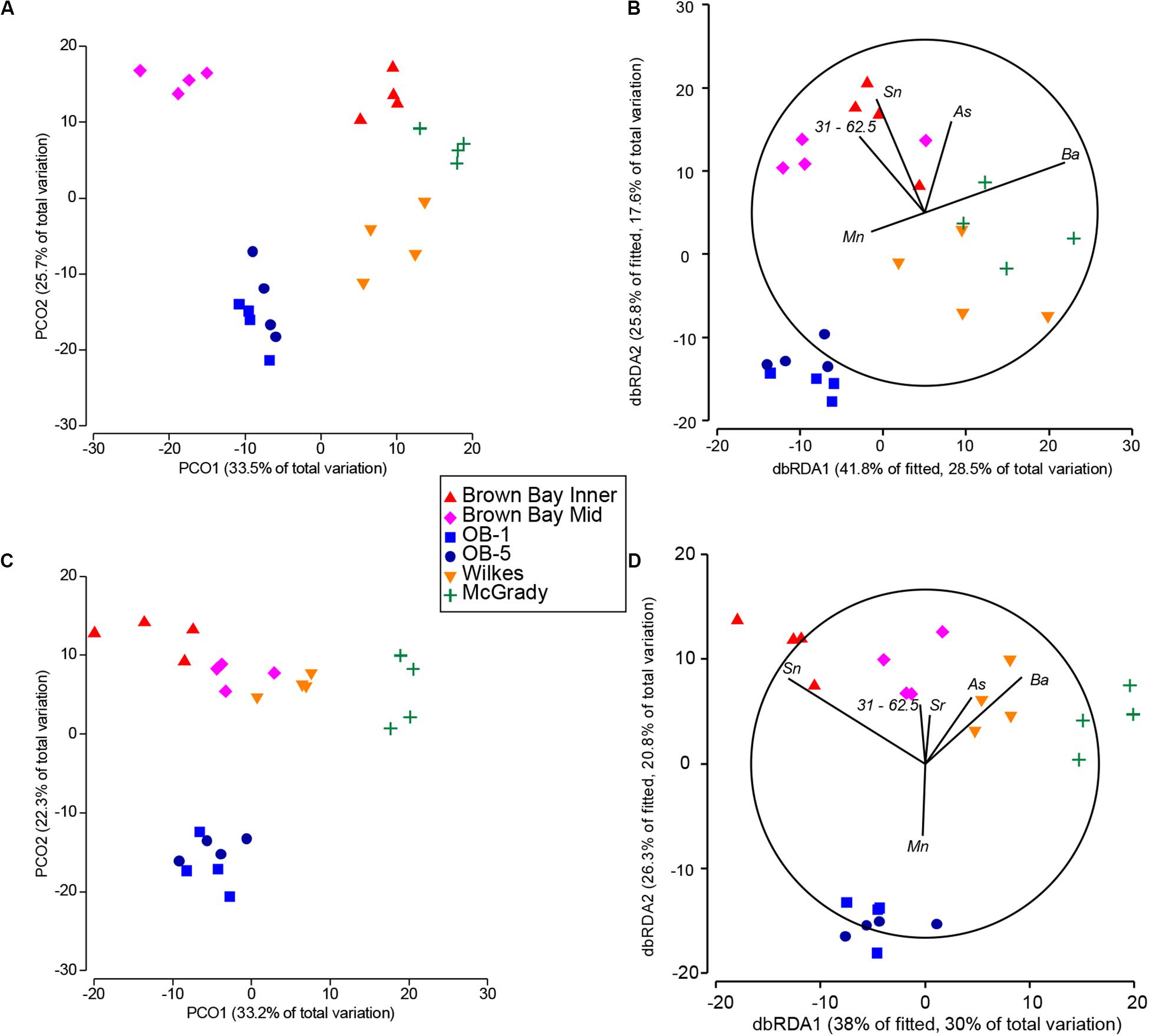
Figure 8. Comparison of fitted DSTLM environmental models with respective PCO ordinations of nematode and copepod communities. (A) PCO ordination of nematode communities; (B) dbRDA ordination of fitted best environmental model explaining nematode community patterns; (C) PCO ordination of copepod communities; (D) dbRDA ordination of fitted best environmental model explaining copepod community patterns. Sb (antimony) represents iron, lead and copper.
DISTLM/dbRDA Nematodes
The single variables with the strongest correlations identified in marginal tests for nematodes were: arsenic, zinc, silver, copper, barium, tin and sediment 15.6–31 μm (Table 8). For nematodes the best multivariate explanatory models included a group of 5 variables (from a total of 26 variables), which accounted for approximately 68% of the variation in the nematode community. These included metals likely to have an anthropogenic source: arsenic which was higher at Brown and McGrady; tin (also represents lead and antimony) which was higher at Brown Bay than all other locations; barium which was lower at the two O’Brien Bay locations; manganese; and sediment in the 31–62.5 μm very coarse silt range (also represents TOC), which was lower at the two O’Brien Bay locations. Selected models were visualized using dbRDA ordinations (Figure 8), bearing a strong resemblance to the unconstrained PCO ordination of nematode communities (Figures 8A,B), explaining almost 70% of the fitted model variation and nearly 50% of the total variation in nematode communities. The impacted locations of Brown Bay Inner and Middle have higher metal concentrations (note the Sn (represents Pb, Sb) and As vectors overlaid on Figure 8B), and finer sediments, separating these locations from the others in these ordinations. McGrady also has fine sediments but higher concentrations of barium. The two O’Brien Bay locations had lower metal concentrations as well as higher manganese (Figure 8B).
DISTLM/dbRDA Copepods
For copepods there was a slightly different set of variables with barium, zinc, cadmium, coarse silt 15.6–31 μm, arsenic, tin and copper among the highest correlations with copepod patterns (Table 8A). For copepods the best explanatory model included barium, tin (representing lead and antimony), manganese, arsenic, strontium and very coarse silt (31–63 μm), with the best dbRDA model explaining 64% of fitted and 50% of total variation (Figures 8C,D). There are two clear groups of copepod communities, those in O’Brien Bay, correlated with higher concentrations of manganese; and those in Newcomb Bay, correlated with higher concentrations of metals (tin (lead, antimony), arsenic) strongly differentiating the Brown Bay locations, and finer sediments (Figures 8C,D). Wilkes and McGrady were also differentiated from the O’Brien locations along the dbRDA 1 axis, which had a strong positive correlation with barium. Both O’Brien Bay locations were negatively correlated with fine sediments but positively correlated with manganese (Figure 8D).
Discussion
This study provides the first description of the spatial distribution, diversity and relationship to environmental influences for benthic nematode and copepod meiofaunal communities in east Antarctica. The dominance of nematodes (which comprised over 90% of the meiobenthic community) is similar to other areas of Antarctica, whether in shallow or in deep waters (Table 9).
Abundance
Abundances of copepods and nematodes in shallow waters around Casey are at the lower end of, but within, the range of abundances found to date in shallow-water Antarctic sediments (Table 9). Comparisons among studies, however, are heavily affected by sampling, preservation, extraction and sorting methods (Rudnick et al., 1985) and should be interpreted with caution. In our study, the minor deviation of core depth for a few samples below the 5 cm horizon (most samples were 10 cm, but some were only 5–7 cm) is not a major concern, as meiofauna abundance rapidly declines below the first 2 to 3 cm sediment depth. This is especially the case in coastal Antarctic sediments (as a result of high food availability at the surface) as reported by Vanhove et al. (1998) who showed a rapid decline of meiofauna abundance from 5170 ind./10cm2 in the 0–1cm layer, to 182 ind./10cm2 in the 4–5cm layer (representing only 1.38% of abundance in the top 5 cm) and at another site found that only 1.11% of total 0–5 cm meiofauna abundance was found in the 4–5cm depth layer. Pasotti et al. (2012) reported similar meiofauna declines with depth of sediment profile, with only a fraction of the total abundance in the 4–5cm layer.
Most other shallow water studies are from the Antarctic Peninsula region (including South Shetlands and South Orkneys) where densities of meiofauna have generally been found to be much higher, but which have somewhat different marine environments, with generally higher primary productivity, less sea ice (and thus more light in the water column) and warmer summer seawater temperatures (Stark et al., 2019). Such conditions may be favorable for more abundant meiobenthic communities, with abundances up to 11.5 × 106 ind. m–2 found on King George Island (Table 9). Meiofaunal abundance, however, can vary greatly over relatively small scales, for example on King George Island abundances of nematodes in shallow water vary from a mean of 0.43 × 106m–2 (Marion Cove) to 5.6 × 106 m–2 in Potter Cove (Table 9), approximately 9km apart over water. In comparison, abundances at Casey were relatively even over the areas sampled (1.5–2.0 × 106 m–2) and are more similar to abundances found in sediments on the Antarctic shelf (Table 9). As Casey is further south it may more closely resemble the shelf than the Antarctic Peninsula in environmental conditions with longer duration of sea ice leading to lower productivity and lower food availability for meiobenthos.
Diversity
While there have been several Antarctic shallow water studies of meiobenthic abundance, there have been very few which have examined diversity. We recorded 38 nematode genera and 20 copepod families at Casey but identification was not done to species level, and there is very little data on species diversity in other shallow water Antarctic meiofauna studies. In the only two shallow water Antarctic studies to examine nematode diversity, at Signy Island, Vanhove et al. (1998) found 19 nematode genera but Lee et al. (2001b) found 49 genera representing 65 species. Our study found similar diversity to the deep continental shelf in the eastern Weddell Sea, where a total of 38 nematode genera were found by Lee et al. (2001a). In contrast, nematode diversity is generally much higher in Antarctic shelf waters, for example: Vanhove et al. (1999) found 158 genera in the deeper waters of Kapp Norvegia and the Halley Bay shelf and slope (211–2080 m); Raes et al. (2010) found 80 genera in deep shelf waters of the Larsen ice-shelf area; and Hauquier et al. (2011) found 66 nematode genera in only 4 replicate cores in a supposedly impoverished Larsen Ice Shelf area which had only recently become ice free. New approaches, however, have revealed that shallow-water diversity may be much higher than described to date. Using a metabarcoding approach, the shallow water meiofaunal diversity at Adelaide Island on the Antarctic peninsula was found to be potentially as diverse as in temperate regions, with over 90 nematode operational taxonomic units (OTUs) (Fonseca et al., 2017). Such approaches have not yet been tried elsewhere specifically for meiofauna in Antarctica. There are insufficient studies to determine whether the diversity of nematodes observed in our study at Casey is generally representative of Antarctic shallow water ecosystems.
Very few studies have examined meiobenthic copepod diversity in the Antarctic region. We observed 20 copepod families in this study. The only other shallow water locality in Antarctica where copepods have been identified to family is on King George Island, where 14 copepod families have been recorded, representing 34 species (Hong et al., 2011). This suggests that the 20 families observed in our study may represent more than 20 species, which would presumably change community patterns. In a study of deeper water continental shelf and slope sediments of the subantarctic Magellan region of South America, George (2005) reported 25 families of copepods, of which 6 families comprised at least 52 genera and 122 species, 80% of which were undescribed. In the Magellan region, however, species are potentially restricted to small geographic areas and families and genera display a much wider distribution than the species they enclose, and represent a broad spectrum of ecological habitats and roles (George, 2005). Shallow water environments such as in this study are likely to be less diverse due to the narrower niche of ecological habitats compared to a broad area of shelf and slope such as the Magellan region.
Spatial Variation
Significant spatial variation in meiofaunal communities at Casey Station was observed at all scales measured, from 10’s to 1000’s of meters. The greatest variation was found at the largest scale, between locations, which accounted for approximately 70% of the variation in nematode and copepod communities. Perhaps the most striking feature of this variation was that all locations had distinctly different communities, with very little overlap of samples in multivariate ordinations. This suggests that unique conditions and adaptation to those conditions at the different locations have shaped the nematode and copepod communities over potentially a long time.
At smaller scales, nematodes and copepods had very different distribution patterns. At scales of 100 m (between sites) there was very little variation in nematode communities but significant variation (6% of overall) for copepods; while in contrast at the 10 m scale (between plots within sites) there was significant variation in nematode communities (8% of overall) and no significant variation for copepods. There was a similar amount of variation between individual replicate cores for both nematodes and copepods and this comprised the second largest source of variation (approximately 21 to 25%), indicating large variation at scales < 1 m. Potential explanations of why nematode communities may exhibit greater variability at 10 m scale and for the copepods at 100-m scale may be related to the life style and mobility range of nematodes and copepods. Nematodes are confined to the sediment as they occupy interstitial space. The different response of copepods could be related to their life style, and while copepods are also interstitial, they can also have a epibenthic-pelagic life style and may emerge and move across sediment surfaces more easily than nematodes (Rubal et al., 2011), giving them a larger range, and hence their potential responses to heterogeneity on the seafloor may be expressed at greater spatial scales compared to nematodes. The epibenthic life style of copepods may also allow them to actively avoid polluted sediments (Rubal et al., 2011). The two most abundant copepod families in our study, Tisbidae and Ectinosomatidae (Table 1), are known to actively move between the sediment to the water column, and such behavior has been described as a way to cope with pollution (Calow, 1991).
Previous studies investigating the role of drivers of meiofaunal variation at different spatial scales have shown that the smaller scales are important determinants of community composition and structure as they relate to the biogeochemical (e.g., sediment heterogeneity and micro-patchiness) and trophic environment of the meiofauna organisms in their interstitial space (Fonseca et al., 2010; Ingels and Vanreusel, 2013; Rosli et al., 2018). Small-scale environmental heterogeneity and associated trophic and biogeochemical conditions, as well as disturbance events and seasonal variability may drive the spatially contrasting settings in this area. It has been proposed that heterogeneity of habitats is an important determinant of meiofaunal biodiversity in shallow waters (Mokievsky and Azovsky, 2002; Vanreusel et al., 2010).
Similar patterns of spatial variation were observed for macrofaunal communities at the same locations (Stark et al., 2003a) suggesting that the same environmental drivers are influencing both meiofaunal and macrofaunal communities at similar scales (Stark et al., 2003b). However, meiofaunal communities displayed greater differentiation between locations than macrofauna, indicating a high degree of suitability of meiofauna for environmental monitoring purposes.
Environmental Influences on Meiofaunal Communities
Of all the environmental drivers examined in this study it was metals that were able to explain the most variation in meiofaunal community patterns, particularly those metals associated with anthropogenic sources. These had some of the strongest correlations with community patterns at the location scale. This was principally due to the large differences in metal concentrations between Brown Bay and other locations, however, this does not necessarily infer causality, as there could be other unmeasured differences in environmental conditions between Brown Bay and other locations. Cause and effect can only be established by experimental studies, either in field experiments (e.g., Thompson et al., 2007) or laboratory experiments (e.g., Stark, 1998). The effects of metals and hydrocarbons have been experimentally demonstrated on macrofaunal communities in Brown Bay (Stark et al., 2003c). Contamination in Brown Bay also includes hydrocarbons (Stark et al., 2005) and may also include persistent organic pollutants, which are known to occur around some Antarctic stations and waste disposal sites (Lenihan and Oliver, 1995; Wild et al., 2015; Stark et al., 2016).
Sediment grain-size properties and organic matter content also had strong correlations with community patterns and were included in the best explanatory models. Sediment grain size can be particularly variable in shallow marine sediments, and was found to be highly variable in this study, not only between locations but also at the plot (10 m) and replicate level, with each accounting for approximately 25% of variation in grain size. Meiofaunal diversity can be regulated by the grain size of the sediments (Steyaert et al., 1999) and the differences in grain size between locations in this study were strongly correlated with differences in meiofaunal communities. Sediment properties (grain size and food content) have been found to be important determinants of meiofaunal community patterns generally (Coull, 1999), and this has also found to apply in studies from Antarctica, such as King George Island (Skowronski and Corbisier, 2002) and Signy Island (Vanhove et al., 1998).
In our study the influence of sediment metals on meiofauna appears to be stronger than that of grain size. The differences found between nematode and copepod communities at Brown Bay and other locations were strongly correlated with higher concentrations of metals including lead, copper, iron and antimony, which were known to occur in the adjacent waste disposal site (Stark et al., 2008). Despite having very different sediment grain size properties the meiofaunal communities at the two locations in O’Brien Bay were very similar, particularly nematode communities. Meiofauna are well known to respond to metal contamination, from both laboratory and field surveys and experimental studies (Coull and Chandler, 1992; Somerfield et al., 1994; Schratzberger et al., 2000). As concentrations of all metals increases there is increased mortality of meiofauna and reduced reproductive output (in vitro) and some fauna such as copepods have been shown to be more affected by paired metal mixtures than by single metals (Coull and Chandler, 1992). Mixed pollutants, such as metals and hydrocarbons, have been shown to inhibit life-history progressions in the laboratory, and to cause synergistic reductions in the diversity and abundance of meiofauna in the field (Coull and Chandler, 1992).
Physical disturbance is another potential influence on meiobenthic communities, such as that caused by calving of ice cliffs and large snow banks that form in the lee of the ice cliffs, which can break off during summer when the sea ice breaks out. Physical disturbance caused by this would be intermittent and patchy, and not necessarily throughout the whole bay, but it may nevertheless influence the benthic communities (Schratzberger et al., 2009). The McGrady area is characterized by ice cliffs which are bigger than at Wilkes or Brown Bay, extending to the seabed at 5–7m water depth, so this may be potentially more of an influence there. The nematode communities at McGrady were more similar to Brown Bay than to the Wilkes and O’Brien locations, possibly due to physical disturbance induced by the specific glacial conditions in the bay.
There was also a difference in nematode and copepod communities between the two large bays: Newcomb Bay (which contained Brown Bay, McGrady and Wilkes) and O’Brien Bay (which contained O’Brien Bay-1 and -5). Larger scale factors such as the hydrographic characteristics of Newcomb and O’Brien Bay may also have had an effect on meiofaunal communities. Despite significant differences in sediment grain size and organic matter content between the two O’Brien Bay locations, they had very similar meiofaunal communities and together they displayed distinct differences from the locations in Newcomb Bay. Hydrographic characteristics influence food availability in benthic habitats, by influencing benthic-pelagic coupling, and can have a strong effect on meiofaunal communities (Veit-Köhler et al., 2018) and is driven by oceanographic conditions such as water temperature, salinity, sea-ice cover and pelagic primary production. Not only is food supply (quantity) very influential in structuring meiofaunal communities, but food quality can also be important (Fabiano and Danovaro, 1999), such as the concentrations of protein, carbohydrate and lipids. The distribution of deep-sea Antarctic meiofauna can be strongly related to the amount of utilizable organic matter in the sediment (Fabiano and Danovaro, 1999), which is controlled by sedimentation and organic degradation rates. Some of the differences between the two large bays and among locations observed in this study are likely to be influenced by local variation in hydrographic characteristics (currents, sea ice regime), which would also influence benthic pelagic coupling and thus sedimentation, food availability and organic degradation rates. Modeling has indicated that differences in meiofaunal communities between the two bays were also correlated with higher concentrations of organic matter (and very coarse silt) and barium in Newcomb Bay. Barium is considered to be a useful proxy for primary productivity in paleo studies (Prakash Babu et al., 2002; Liguori et al., 2016) and together with generally higher levels of organic matter in sediments may indicate generally higher levels of primary production in Newcomb Bay.
Human Impacts
Another large scale difference between the two bays could be from generally higher contamination throughout Newcomb Bay (Wilkes, McGrady, Brown Bay Inner and Middle). There is evidence for this for many metals at the locations examined in Newcomb Bay (Table 6) but there may be other contaminants present throughout the bay that have not been measured, such as hydrocarbons. Newcomb Bay has a long history of continuous human occupation, which to date has comprised three separate Antarctic research stations dating back to 1957. Prior to modern environmental management there were many practices and activities which could have led to widespread pollution, such as igniting full drums of fuel on the shoreline to farewell ships and disposing of old vehicles on the sea ice (personal communication by previous expeditioners to J. Stark). Such anecdotal evidence is not captured by official records but may have had long term consequences. A few metals are generally higher in Newcomb than O’Brien Bay including iron, arsenic and zinc, which may also explain why nematode communities at McGrady were more similar to Brown Bay than O’Brien Bay.
Meiofauna are considered to be sentinels of environmental changes making them useful bioindicators of human impacts (Somerfield et al., 1994; Kennedy and Jacoby, 1999; Semprucci and Balsamo, 2012; Alves et al., 2013; Semprucci et al., 2015; Schratzberger and Ingels, 2017). In this study there were distinctive differences in nematode and copepod communities between control and polluted locations. Activities associated with the waste disposal site in Brown Bay have resulted in increased metals, organic content (Stark, 2000; Stark et al., 2003b) and hydrocarbons (Stark et al., 2005) in sediments, which have likely contributed to the differences in meiofaunal communities between Brown Bay and control locations. Other studies have found that meiofaunal assemblages, and nematodes in particular, are indicative of differences in metal contamination and of metal gradients in sediments (Somerfield et al., 1994; Coull, 1999; Schratzberger et al., 2000).
Differences in nematode communities between the Inner and Middle locations within Brown Bay, though only several hundred meters apart, were as great as between other locations separated by many kilometers. Copepod communities at the two Brown Bay locations, however, were the most similar among all locations. Some metals have higher concentrations at Brown Bay Inner (Cu, Fe, Pb, Sn) and others are higher at Brown Bay Middle (Ag, As, Cr, Ni, Sb, Zn) (Stark et al., 2003b). It is not understood why this is the case although it may relate to the contamination history of the dump site, as large items of rubbish have been observed on the sea bed (metal components, fuel drums, treated timber) and may have contributed to localized patterns or hot spots of contamination. Previous waste management practices included using explosives to create a hole in the sea ice and bulldozing rubbish into it, although such practices have long since been discontinued (Stark et al., 2006). As discussed above, the lifestyle of copepods, with the potential for epibenthic dispersal and movement, may serve to dampen small-scale localized pollution effects, which are better reflected in nematode communities. Hydrocarbon levels are generally greater at the Inner than the Middle location (Stark et al., 2005). It is thus difficult to determine which part of Brown Bay is the more polluted but copepods appear to be responding in the same manner in both locations, while nematodes are responding differently. Warwick (1986) suggested that copepods are generally more sensitive to the effects of pollution than nematodes, however our study indicates that Antarctic nematodes may be more sensitive on small scales than copepods.
In comparison to other regions of Antarctica Brown Bay could be considered to be low to moderately polluted (Stark et al., 2014b). Contamination levels are lower than observed at the severely polluted Winter Quarters Bay at McMurdo Station, but impacts resulting from this pollution are very clear and in some respects are as large as seen at McMurdo (Stark et al., 2014b), not only in meiofauna but also in benthic diatom (Cunningham et al., 2005), macrofaunal (Stark et al., 2003a, 2004) and epifaunal communities (Stark, 2008).
Meiofaunal communities at Wilkes, however, do not appear to be impacted by the former waste disposal site at Wilkes Station. Nematode communities at Wilkes were not significantly different from controls and were most similar to the control locations in O’Brien Bay. Whereas the copepod communities at Wilkes, while also not significantly different to the controls, were more similar to the control location McGrady Cove in Newcomb Bay. Despite evidence for contamination of meltwater from sites at Wilkes flowing into the marine environment at concentrations well in excess of the Australian and New Zealand (ANZECC) guidelines (Fryirs et al., 2015), metal levels in marine sediments are generally not significantly different from the control locations, although other contaminants, such as hydrocarbons, have not been measured. The Wilkes waste dumpsite is much older than the Casey site, dating back to 1957 and was abandoned in 1969. Contaminants may not have accumulated in adjacent marine sediments to the same extent or marine communities may have recovered from pollution impacts or may not have been as strongly impacted as Brown Bay. Stark et al. (2003c) found that the macrofaunal communities at Wilkes were similar in some ways to those of the control locations (O’Brien Bay) but in other ways resembled Brown Bay, suggesting that perhaps it was impacted at some point since human occupation.
While clear patterns related to disturbance were observed at the community level, patterns for individual taxa in relation to disturbance were less clear. A few taxa were consistently more abundant at impacted locations at Brown Bay including the nematode genera Promonhystera, Paralinhomoeus and Pierrickia and the copepod family Pseudotachidiidae. A few taxa were more abundant at control locations including the nematode genera Daptonema, Halalaimus and Microlaimus and the copepod families Dactylopusiidae and Rometidae. Coherence curves for nematodes (Figure 5) provided some insights into the use of certain genera as indicators, particularly when compared to findings in previous studies. Each location exhibited a group of nematode taxa genera which were characteristic of that location (Figure 5) and in some instances may be indicative of a pollution response., e.g., Bianchelli et al. (2018). Several genera that have been shown to respond to different types of disturbances in other studies are highlighted below.
There is a subset of nematode genera with high abundance at the polluted and organic matter loaded Brown Bay Inner location (Figure 5D). Leptolaimus has been previously recognized as tolerant to high organic matter levels as well as physical disturbance (Schratzberger et al., 2009; Losi et al., 2013). Odontophora has been recognized as opportunistic in conditions with high Ni content (Balsamo et al., 2012 and references within). Sabatieria and Theristus (Figure 5D) are two genera that have often been mentioned as tolerant and opportunistic in various disturbed conditions such as physical disturbance, general pollution, hypoxia or anoxia, and in the context of metal contamination (Somerfield et al., 1994; Millward and Grant, 1995; Austen and Somerfield, 1997; Gyedu-Ababio et al., 1999; Balsamo et al., 2012). It is not surprising then that these genera are abundant at the Brown Bay Inner location as they are able to colonize disturbed or impacted sediments rapidly and persist in adverse conditions. Also striking are the abundant genera associated with the polluted Brown Bay Middle location (Figure 5E). Paramonhystera and Promonhystera are members of the monhysterid family, traditionally recognized as containing opportunistic and tolerant genera (Balsamo et al., 2012; Semprucci et al., 2015). Pierrickia has also been recognized as a genus that is tolerant to high organic matter levels and contaminated sediments (Danovaro et al., 2009; Losi et al., 2013). These genera provide some context in which to interpret the usefulness of nematode in indicating sediment disturbance and contamination. However, in this study, a number of other genera that have been postulated as either sensitive or tolerant to pollution, contamination, and disturbed conditions in other studies did not adhere to the expected abundance or presence-absence in that context. Paracanthonchus was assigned to the same subset of nematode genera in the coherence analysis, but did not generally conform to the same pattern as other taxa in the group as it had minimum abundance in Brown Bay Inner samples. Danovaro et al. (1995) and Balsamo et al. (2012) indicate that this genus is sensitive to hydrocarbon impacts and was one of the first genera to disappear following an oil spill, and hydrocarbon concentrations are generally higher at Brown Bay Inner (Stark et al., 2005). This highlights that care should be taken when interpreting individual nematode genera patterns, since communities are driven not just by individual responses, but also by a wide range of abiotic and biotic interactions. Complex succession patterns in response to disturbance and interactions with other benthic components as part of food webs or through competitive interactions may also confound clear patterns. Another example is the genus Halalaimus, which has been recognized as being sensitive to organic loads (Essink and Keidel, 1998; Mirto et al., 2002; Vezzulli et al., 2008). This is inconsistent with the observations in our study, with sediments at Brown Bay (where it was least abundant) and McGrady (where it was most abundant) both containing high levels of organic matter. This suggests that in this study it was more responsive to contaminants than organic loading of sediments. In addition, responses to pollution, disturbance or other environmental change may differ among species within the same genus.
Meiofauna as Environmental Indicators in Antarctica
While it is well documented that meiofauna are generally excellent indicators for natural or anthropogenic environmental change (Zeppilli et al., 2015), caution should be used in interpreting abundance data when little is known of their temporal or spatial distribution and heterogeneity at specific locations. For example, significant seasonal variations in meiofaunal abundance were found in shallow water at Signy Island (Vanhove et al., 2000) and at King George Island (Pasotti et al., 2014). In the present study, the specific environmental conditions related to the geographic and glacial conditions and sediment heterogeneity at the different locations likely have some influence on the nematode and copepod communities, but these varied together with the metal signatures and their impacts on the faunal communities. Temporal variability can also be related to spatial heterogeneity, particularly in the Antarctic, where glacial characteristics and influences vary seasonally with ice melt and sea ice break up.
Other benthic community components have been well studied in coastal areas of east Antarctica at Casey Station including the macrobenthos (Stark et al., 2003a, b) and microphytobenthos (Cunningham et al., 2003, 2005; Polmear et al., 2015) but the meiofauna are an important component of benthic ecosystems for which little was previously known in this region. Given the clear differences in both nematode and copepod communities at the location scale and their strong correlation with environmental patterns, particularly anthropogenic disturbance, this study adds further evidence that these taxa may be excellent indicators of environmental change in Antarctic coastal waters. While there is a clear signature of human impacts at the polluted Brown Bay locations which can be detected using nematode and copepod communities, there are also important differences among locations driven by a range of natural environmental variables. These need to be taken into account when drawing comparisons and disentangling the nature and effect of the anthropogenic and natural drivers of these communities. Further understanding of responses of Antarctic meiofauna to environmental change would be gained by examining species level responses, as well as including other less abundant elements of the meiofaunal community such as kinorhynchs, gastrotrichs, tardigrades, polychaetes and crustaceans such as amphipods.
Sampling and Field Studies
All necessary permits for sampling and observational field studies were obtained by the authors from the competent authorities. The study is compliant with CBD and Nagoya protocols.
Data Availability Statement
The datasets generated for this study are available at https://data.aad.gov.au/metadata/records/AAS_2201_Casey_Monitoring_Meiofauna.
Author Contributions
JS designed the study and contributed to the field work, collection of samples, and laboratory analysis of environmental samples. MM conducted the laboratory analysis and identification of meiofaunal communities. JS, JI, MM, and AM contributed to the statistical analysis and interpretation. JS and JI prepared the figures. All authors contributed to the writing and editing of the manuscript.
Funding
This study was funded by the Universiti Sains Malaysia, Australian Antarctic Division (AAS projects 2201 and 4180) and Institute for Marine and Antarctic Studies at the University of Tasmania.
Conflict of Interest
The authors declare that the research was conducted in the absence of any commercial or financial relationships that could be construed as a potential conflict of interest.
Acknowledgments
We are grateful to the following people for their assistance and support in this study: Sazlina Salleh, Martin Riddle, Glenn Johnstone, Scott Stark, Anne Palmer, Kate Stark, and the Casey dive team in 2005/06 season. Some of the content of this study was part of a postgraduate Ph.D. thesis of MM (Mohammad, 2011) and is the only medium it has appeared in, and is in line with the University of Tasmania policy, and can be accessed online at http://encore.lib.utas.edu.au/iii/encore/record/C__Rb1570829.
Footnotes
References
Alves, A. S., Adão, H., Ferrero, T., Marques, J., Costa, M. J., and Patrício, J. (2013). Benthic meiofauna as indicator of ecological changes in estuarine ecosystems: the use of nematodes in ecological quality assessment. Ecol. Indic. 24, 462–475. doi: 10.1016/j.ecolind.2012.07.013
Anderson, M. J. (2006). Distance-based tests for homogeneity of multivariate dispersions. Biometrics 62, 245–253. doi: 10.1111/j.1541-0420.2005.00440.x
Anderson, M. J., Gorley, R. N., and Clarke, K. R. (2008). PERMANOVA+ for PRIMER: Guide to Software and Statistical Methods. Plymouth, UK: PRIMER-E.
Anderson, M. J., and Robinson, J. (2001). Permutation tests for linear models. Aust. New Zeal. J. Stat. 43, 75–88.
Austen, M. C., and Somerfield, P. J. (1997). A community level sediment bioassay applied to an estuarine heavy metal gradient. Mar. Environ. Res. 43, 315–328. doi: 10.1016/s0141-1136(96)00094-3
Balsamo, M., Semprucci, F., Frontalini, F., and Coccioni, R. (2012). “Meiofauna as a tool for marine ecosystem biomonitoring,” in Marine Ecosystems, ed. C. Antonio (Rijeka: In Tech).
Bianchelli, S., Buschi, E., Danovaro, R., and Pusceddu, A. (2018). Nematode biodiversity and benthic trophic state are simple tools for the assessment of the environmental quality in coastal marine ecosystems. Ecol. Indicat. 95, 270–287. doi: 10.1016/j.ecolind.2018.07.032
Bodin, P. (1997). Catalogue of the new marine harpacticoid copepods. Doc. Trav. Inst. Roy. Sci. Nat. Belg. 89:304.
Borum, J., and Sand-Jensen, K. (1996). Is total primary production in shallow coastal marine waters stimulated by nitrogen loading? Oikos 76, 406–410.
Boucher, G., and Lambshead, P. J. D. (1995). Ecological biodiversity of marine nematodes in samples from temperate, tropical, and deep-sea regions. Conserv. Biol. 9, 1594–1604. doi: 10.1046/j.1523-1739.1995.09061594.x
Calow, P. (1991). Physiological costs of combating chemical toxicants: ecological implications. Comp. Biochem. Physiol. C 100, 3–6. doi: 10.1016/0742-8413(91)90110-f
Clark, G. F., Raymond, B., Riddle, M. J., Stark, J. S., and Johnston, E. L. (2015). Vulnerability of shallow Antarctic invertebrate-dominated ecosystems. Aust. Ecol. 40, 482–491. doi: 10.1111/aec.12237
Clarke, K. R., and Gorley, R. N. (2006). PRIMER v6. User Manual/Tutorial Plymouth Routine in Mulitvariate Ecological Research. Plymouth: Plymouth Marine Laboratory
Coull, B. C. (1999). Role of meiofauna in estuarine soft-bottom habitats. Aust. J. Ecol. 24, 327–343. doi: 10.1046/j.1442-9993.1999.00979.x
Coull, B. C., and Chandler, G. T. (1992). Pollution and meiofauna: field, laboratory, and mesocosm studies. Oceanogr. Mar. Biol. 30, 191–271.
Cunningham, L., Snape, I., Stark, J. S., and Riddle, M. J. (2005). Benthic diatom community response to environmental variables and metal concentrations in a contaminated bay adjacent to Casey Station. Antarctica. Mar. Pollut. Bull. 50, 264–275. doi: 10.1016/j.marpolbul.2004.10.012
Cunningham, L., Stark, J. S., Snape, I., McMinn, A., and Riddle, M. J. (2003). Effects of metal and petroleum hydrocarbon contamination on benthic diatom communities near Casey Station, Antarctica: an experimental approach. J. Phycol. 39, 490–503. doi: 10.1046/j.1529-8817.2003.01251.x
Danovaro, R., Fabiano, M., and Vincx, M. (1995). Meiofauna response to the Agip Abruzzo oil spill in subtidal sediments of the Ligurian Sea. Mar. Pollut. Bull. 30, 133–145. doi: 10.1016/0025-326x(94)00114-o
Danovaro, R., Gambi, C., Höss, S., Mirto, S., Traunspurger, W., and Zullini, A. (2009). Case Studies Using Nematode Assemblage Analysis in Aquatic Habitats. Nematodes as Enviornmental Indicators. Wallingford: CABI publishing, 146–171.
Danovaro, R., Pusceddu, A., Mirto, S., and Fabiano, M. (1999). Meiofaunal assemblages associated with scallop beds (Adamussium colbecki) in the coastal sediments of Terra Nova Bay (Ross Sea, Antarctica). Antarctic Sci. 11, 415–418. doi: 10.1017/s0954102099000528
Essink, K., and Keidel, H. (1998). Changes in estuarine nematode communities following a decrease of organic pollution. Aquatic Ecol. 32, 195–202.
Fabiano, M., and Danovaro, R. (1999). Meiofauna distribution and mesoscale variability in two sites of the Ross Sea (Antarctica) with contrasting food supply. Polar Biol. 22, 115–123. doi: 10.1007/s003000050398
Fonseca, G., Soltwedel, T., Vanreusel, A., and Lindegarth, M. (2010). Variation in nematode assemblages over multiple spatial scales and environmental conditions in Arctic deep seas. Progr. Oceanogr. 84, 174–184. doi: 10.1016/j.pocean.2009.11.001
Fonseca, V., Sinniger, F., Gaspar, J., Quince, C., Creer, S., Power, D. M., et al. (2017). Revealing higher than expected meiofaunal diversity in Antarctic sediments: a metabarcoding approach. Sci. Rep. 7:6094.
Fryirs, K. A., Hafsteinsdóttir, E. G., Stark, S. C., and Gore, D. B. (2015). Metal and petroleum hydrocarbon contamination at Wilkes Station, East Antarctica. Antarctic Sci. 27, 118–133. doi: 10.1017/s0954102014000443
George, K. H. (2005). Sublittoral and bathyal Harpacticoida (Crustacea, Copepoda) of the Magellan region. Composition, distribution and species diversity of selected major taxa. Sci. Mar. 69, 147–158. doi: 10.3989/scimar.2005.69s2147
Graham, M. H., and Edwards, M. S. (2001). Statistical significance vs. fit: estimating relative importance of individual factors in ecological analysis of variance. Oikos 93, 505–513
Gyedu-Ababio, T., Furstenberg, J., Baird, D., and Vanreusel, A. (1999). Nematodes as indicators of pollution: a case study from the Swartkops River system, South Africa. Hydrobiologia 397, 155–169.
Hauquier, F., Ingels, J., Gutt, J., Raes, M., and Vanreusel, A. (2011). Characterisation of the nematode community of a low-activity cold seep in the recently ice-shelf free Larsen B area, Eastern Antarctic Peninsula. PLoS One 6:e22240. doi: 10.1371/journal.pone.0022240
Heip, C., Vincx, M., and Vranken, G. (1985). The ecology of marine nematodes. Oceanogr. Mar. Biol. 23, 399–489.
Heiri, O., Lotter, A. F., and Lemcke, G. (2001). Loss on ignition as a method for estimating organic and carbonate content in sediments: reproducibility and comparability of results. J. Paleolimnol. 25, 101–110.
Herman, R., and Dahms, H. (1992). Meiofauna communities along a depth transect off Halley Bay (Weddell Sea-Antarctica). Polar Biol. 12, 313–320. doi: 10.1007/978-3-642-77595-6_36
Hong, J.-H., Kim, K.-C., Lee, S.-H., Back, J.-W., Lee, D.-J., and Lee, W.-C. (2011). The community structure of meiofauna in Marian cove, King George island, Antarctica. Ocean Polar Res. 33, 265–280. doi: 10.4217/opr.2011.33.3.265
Ingels, J., Billett, D. S. M., Kiriakoulakis, K., Wolff, G. A., and Vanreusel, A. (2011a). Structural and functional diversity of Nematoda in relation with environmental variables in the Setúbal and Cascais canyons, Western Iberian Margin. Deep Sea Res. Part II 58, 2354–2368. doi: 10.1016/j.dsr2.2011.04.002
Ingels, J., Tchesunov, A. V., and Vanreusel, A. (2011b). Meiofauna in the Gollum Channels and the Whittard Canyon, Celtic Margin–how local environmental conditions shape nematode structure and function. PLoS One 6:e20094. doi: 10.1371/journal.pone.0020094
Ingels, J., Kiriakoulakis, K., Wolff, G. A., and Vanreusel, A. (2009). Nematode diversity and its relation to the quantity and quality of sedimentary organic matter in the deep Nazare Canyon, Western Iberian Margin. Deep-Sea Res. Part I Oceanogr. Res. Pap. 56, 1521–1539. doi: 10.1016/j.dsr.2009.04.010
Ingels, J., and Vanreusel, A. (2013). The importance of different spatial scales in determining structural and functional characteristics of deep-sea infauna communities. Biogeosciences 10, 4547–4563. doi: 10.5194/bg-10-4547-2013
Ingels, J., Hauquier, F., Raes, M., Vanreusel, A. (2014). “Antarctic free-living marine nematodes,” in Biogeographic atlas of the Southern Ocean, eds C. De Broyer, P. Koubbi, H. Griffiths, B. Raymond, C. D. Udekem d’Acoz and A. Van de Putte (Cambridge: Scientific Committee on Antarctic Research).
Kennedy, A. D., and Jacoby, C. A. (1999). Biological indicators of marine environmental health: meiofauna – a neglected benthic component? Environ. Monit. Assess. 54, 47–68.
Lee, H. J., Gerdes, D., Vanhove, S., and Vincx, M. (2001a). Meiofauna response to iceberg disturbance on the Antarctic continental shelf at Kapp Norvegia (Weddell Sea). Polar Biol. 24, 926–933. doi: 10.1007/s003000100301
Lee, H. J., Vanhove, S., Peck, L. S., and Vincx, M. (2001b). Recolonisation of meiofauna after catastrophic iceberg scouring in shallow Antarctic sediments. Polar Biol. 24, 918–925. doi: 10.1007/s003000100300
Lee, H. J., and Van de Velde, J. (1999). “Biodiversity of Antarctic nematodes,” in Reports on Polar Research, the Expedition ANTARKTIS ZX/3 (EASIZ II), eds W. E. Aaa and J. Gaa (Bremerhaven: Boehl & Oppermann).
Lenihan, H. S., and Oliver, J. S. (1995). Anthropogenic and natural disturbances to marine benthic communities in Antarctica. Ecol. Appl. 5, 311–326.
Liguori, B. T., Almeida, M., and Rezeende, C. (2016). Barium and its Importance as an Indicator of (Paleo) Productivity. Anais Acad. Bras. Ciências 88, 2093–2103. doi: 10.1590/0001-3765201620140592
Losi, V., Ferrero, T., Moreno, M., Gaozza, L., Rovere, A., Firpo, M., et al. (2013). The use of nematodes in assessing ecological conditions in shallow waters surrounding a Mediterranean harbour facility. Estuar. Coast. Shelf Sci. 130, 209–221. doi: 10.1016/j.ecss.2013.02.017
McMinn, A., Gibson, J., and Hodgson, D. (1995). Nutrient limitation in Ellis Fjord, eastern Antarctica. Polar Biol. 15, 269–276.
McMinn, A., and Hodgson, D. (1993). Summer phytoplankton succession in Ellis Fjord, eastern Antarctica. J. Plankton Res. 15, 925–938. doi: 10.1093/plankt/15.8.925
Millward, R. N., and Grant, A. (1995). Assessing the impact of copper on nematode communities from a chronically metal-enriched estuary using pollution-induced community tolerance. Mar. Pollut. Bull. 30, 701–706. doi: 10.1016/0025-326x(95)00053-p
Mirto, S., La Rosa, T., Gambi, C., Danovaro, R., and Mazzola, A. (2002). Nematode community response to fish-farm impact in western Mediterranean. Environ. Pollut. 116, 203–214. doi: 10.1016/s0269-7491(01)00140-3
Mohammad, M. (2011). Meiofaunal communities and human impacts at Casey Station, Antarctica. PhD Thesis PhD, University of Tasmania, Hobart.
Mokievsky, V., and Azovsky, A. (2002). Re-evaluation of species diversity patterns of free-living marine nematodes. Mar. Ecol. Prog. Ser. 238, 101–108. doi: 10.3354/meps238101
Pasotti, F., Convey, P., and Vanreusel, A. (2014). Potter Cove, west Antarctic Peninsula, shallow water meiofauna: a seasonal snapshot. Antarctic Sci. 26, 554–562. doi: 10.1017/s0954102014000169
Pasotti, F., De Troch, M., Raes, M., and Vanreusel, A. (2012). Feeding ecology of shallow water meiofauna: insights from a stable isotope tracer experiment in Potter Cove, King George Island, Antarctica. Polar Biol. 35, 1629–1640. doi: 10.1007/s00300-012-1203-6
Peck, L. S., Brockington, S., Vanhove, S., and Beghyn, M. (1999). Community recovery following catastrophic iceberg impacts in a soft-sediment shallow-water site at Signy Island, Antarctica. Mar. Ecol. Prog. Ser. 186, 1–8. doi: 10.3354/meps186001
Petti, M. A. V., Nonato, E. F., Skowronski, R. S., and Corbisier, T. N. (2006). Bathymetric distribution of the meiofaunal polychaetes in the nearshore zone of Martel Inlet, King George Island, Antarctica. Antarctic Sci. 18, 163–170. doi: 10.1017/s0954102006000186
Pfannkuche, O., Thiel, H., and Samples, M. (1988). 9. Sample processing Introduction to the Study of Meiofauna. Washington DC: Smithsonian Institution Press.
Platt, H. M., and Warwick, R. M. (1983). Freeliving marine nematodes. Part 1: British enoplids. Pictorial key to World Genera and Notes for the Identification of British Species. Cambridge, UK: Cambridge University Press.
Platt, H., and Warwick, R. (1988). “Freeliving marine nematodes. Part II: British chromadorids. Pictorial key to World Genera and Notes for the Identification of British Species,” Synopses of the British fauna (new series), eds D. M. Kermack and RSK Barnes (Shrewsbury, UK: Field Studies Council (FSC)), 38:502.
Polmear, R., Stark, J. S., Roberts, D., and McMinn, A. (2015). The effects of oil pollution on Antarctic benthic diatom communities over 5 years. Mar. Pollut. Bull. 90, 33–40. doi: 10.1016/j.marpolbul.2014.11.035
Prakash Babu, C., Brumsack, H. J., Schnetger, B., and Böttcher, M. E. (2002). Barium as a productivity proxy in continental margin sediments: a study from the eastern Arabian Sea. Mar. Geol. 184, 189–206. doi: 10.1016/s0025-3227(01)00286-9
Raes, M., Rose, A., and Vanreusel, A. (2010). Response of nematode communities after large-scale ice-shelf collapse events in the Antarctic Larsen area. Glob. Change Biol. 16, 1618–1631. doi: 10.1111/j.1365-2486.2009.02137.x
Riemann, F. (1988). “Nematoda,” in Introduction to the Study of Meiofauna, eds R. Higgins and H. Thiel (Washington, DC: Smithsonian Press), 293–301.
Rose, A., Ingels, J., Raes, M., Vanreusel, A., and Arbizu, P. M. (2015). Long-term iceshelf-covered meiobenthic communities of the Antarctic continental shelf resemble those of the deep sea. Mar. Biodiv. 45, 743–762. doi: 10.1007/s12526-014-0284-6
Rosli, N., Leduc, D., Rowden, A. A., and Probert, P. K. (2018). Review of recent trends in ecological studies of deep-sea meiofauna, with focus on patterns and processes at small to regional spatial scales. Mar. Biodiv. 48, 13–34. doi: 10.1007/s12526-017-0801-5
Rubal, M., Guilhermino, L. M., and Medina, M. H. (2011). Two strategies to live in low chronic pollution estuaries: the potential role of lifestyle. Ecotoxicol. Environ. Saf. 74, 1226–1231. doi: 10.1016/j.ecoenv.2011.02.017
Rudnick, D., Elmgren, R., and Frithsen, J. (1985). Meiofaunal prominence and benthic seasonality in a coastal marine ecosystem. Oecologia 67, 157–168. doi: 10.1007/bf00384279
Schratzberger, M., Gee, J., Rees, H., Boyd, S., and Wall, C. (2000). The structure and taxonomic composition of sublittoral meiofauna assemblages as an indicator of the status of marine environments. J. Mar. Biol. Assoc. 80, 969–980. doi: 10.1017/s0025315400003039
Schratzberger, M., and Ingels, J. (2017). Meiofauna matters: the roles of meiofauna in benthic ecosystems. J. Exp. Mar. Biol. Ecol. 502, 12–25. doi: 10.1016/j.jembe.2017.01.007
Schratzberger, M., Lampadariou, N., Somerfield, P., Vandepitte, L., and Berghe, E. V. (2009). The impact of seabed disturbance on nematode communities: linking field and laboratory observations. Mar. Biol. 156, 709–724. doi: 10.1007/s00227-008-1122-9
Scouller, R. C., Snape, I., Stark, J. S., and Gore, D. B. (2006). Evaluation of geochemical methods for the discrimination of metal contamination in Antarctic marine sediments: a case study from Casey Station, East Antarctica. Chemosphere 65, 294–309. doi: 10.1016/j.chemosphere.2006.02.062
Semprucci, F., and Balsamo, M. (2012). Free-living marine nematodes as bioindicators: past, present and future perspectives. Environ. Res. J. 6, 17–35.
Semprucci, F., Frontalini, F., Sbrocca, C., Du Châtelet, E. A., Bout-Roumazeilles, V., Coccioni, R., et al. (2015). Meiobenthos and free-living nematodes as tools for biomonitoring environments affected by riverine impact. Environ. Monit. Assess. 187, 251.
Skowronski, R. S. P., and Corbisier, T. N. (2002). Meiofauna distribution in Martel Inlet, King George Island (Antarctica): sediment features versus food availability. Polar Biol. 25, 126–134. doi: 10.1007/s003000100320
Snape, I., Riddle, M. J., Stark, J. S., Cole, C. M., King, C. K., Duquesne, S., et al. (2001). Management and remediation of contaminated sites at Casey Station. Antarctica. Polar Rec. 37, 199–214. doi: 10.1017/s0032247400027236
Somerfield, P. J., and Clarke, K. R. (2013). Inverse analysis in non-parametric multivariate analyses: distinguishing groups of associated species which covary coherently across samples. J. Exp. Mar. Biol. Ecol. 449, 261–273. doi: 10.1016/j.jembe.2013.10.002
Somerfield, P. J., Gee, J. M., and Warwick, R. M. (1994). Soft sediment meiofaunal community structure in relation to a long-term heavy metal gradient in the Fal estuary system. Mar. Ecol. Prog. Ser. 105, 79–88. doi: 10.3354/meps105079
Stark, J. S. (1998). Effects of copper on macrobenthic assemblages in soft-sediments: a laboratory experimental study. Ecotoxicology 7, 161–173.
Stark, J. S. (2000). The distribution and abundance of soft-sediment macrobenthos around Casey Station. East Antarctica. Polar Biol. 23, 840–850. doi: 10.1007/s003000000162
Stark, J. S. (2008). Patterns of higher taxon colonisation and development in sessile marine benthic assemblages at Casey Station, Antarctica, and their use in environmental monitoring. Mar. Ecol. Prog. Ser. 365, 77–89. doi: 10.3354/meps07559
Stark, J. S., Bridgen, P., Dunshea, G., Galton-Fenzi, B., Hunter, J., Johnstone, G., et al. (2016). Dispersal and dilution of wastewater from an ocean outfall at Davis Station, Antarctica, and resulting environmental contamination. Chemosphere 152, 142–157. doi: 10.1016/j.chemosphere.2016.02.053
Stark, J. S., Johnstone, G., Stark, S. C., and Palmer, A. (2014a). Analytical Methods Used to Measure Chemical and Physical Properties of Davis Station Wastewater and Marine Sediments. Hobart: Australian Antarctic Data Centre, doi: 10.4225/15/5472BCFBA5E10
Stark, J. S., Kim, S. L., and Oliver, J. S. (2014b). Anthropogenic disturbance and biodiversity of marine benthic communities in antarctica: a regional comparison. PLoS One 9:e98802. doi: 10.1371/journal.pone.0098802
Stark, J. S., Mohammad, M., McMinn, A., and Ingels, J. (2017). The effects of hydrocarbons on meiofauna in marine sediments in Antarctica. J. Exp. Mar. Biol. Ecol. 496, 56–73. doi: 10.1016/j.jembe.2017.07.009
Stark, J. S., Raymond, T., Deppeler, S. L., and Morrison, A. K. (2019). “Antarctic seas,” in World Seas: an Environmental Evaluation (Second Edition), Book 3, ed. C. Sheppard (Cambridge, MA: Academic Press).
Stark, J. S., Riddle, M. J., and Simpson, R. D. (2003a). Human impacts in soft-sediment assemblages at Casey Station, East Antarctica: spatial variation, taxonomic resolution and data transformation. Aust. Ecol. 28, 287–304. doi: 10.1046/j.1442-9993.2003.01289.x
Stark, J. S., Riddle, M. J., Snape, I., and Scouller, R. C. (2003b). Human impacts in Antarctic marine soft-sediment assemblages: correlations between multivariate biological patterns and environmental variables. Estuar. Coast. Shelf Sci. 56, 717–734. doi: 10.1016/s0272-7714(02)00291-3
Stark, J. S., Snape, I., and Riddle, M. J. (2003c). The effects of hydrocarbon and heavy metal contamination of marine sediments on recruitment of Antarctic soft-sediment assemblages: a field experimental investigation. J. Exp. Mar. Biol. Ecol. 283, 21–50.
Stark, J. S., Riddle, M. J., and Smith, S. D. A. (2004). Influence of an Antarctic waste dump on recruitment to near-shore marine soft-sediment assemblages. Mar. Ecol. Prog. Ser. 276, 53–70. doi: 10.3354/meps276053
Stark, J. S., Snape, I., and Riddle, M. J. (2006). Abandoned waste disposal sites in Antarctica: monitoring remediation outcomes and limitations at Casey Station. Ecol. Manag. Restor. 7, 21–31. doi: 10.1111/j.1442-8903.2006.00243.x
Stark, J. S., Snape, I., Riddle, M. J., and Stark, S. C. (2005). Constraints on spatial variability in soft-sediment communities affected by contamination from an Antarctic waste disposal site. Mar. Pollut. Bull. 50, 276–290. doi: 10.1016/j.marpolbul.2004.10.015
Stark, S. C., Snape, I., Graham, N. J., Brennan, J. C., and Gore, D. B. (2008). Assessment of metal contamination using X-ray fluorescence spectrometry and the toxicity characteristic leaching procedure (TCLP) during remediation of a waste disposal site in Antarctica. J. Environ. Monit. 10, 60–70. doi: 10.1039/b712631j
Steyaert, M., Deprez, T., Raes, M., Bezerra, T., Demesel, I., Derycke, S., et al. (2005). Electronic key to the Free-Living Marine Nematodes. World Wide Web Electronic Publication. Available online at: http://www.marinespecies.org/aphia.php?p=idkeys_redirect&page=licence&taxon=280&keyid=13 (accessed September, 2005).
Steyaert, M., Garner, N., van Gansbeke, D., and Vincx, M. (1999). Nematode communities from the North Sea: environmental controls on species diversity and vertical distribution within the sediment. J. Mar. Biol. Assoc. 79, 253–264. doi: 10.1017/s0025315498000289
Suchanek, T. H. (1994). Temperate coastal marine communities: biodiversity and threats. Am. Zool. 34, 100–114. doi: 10.1093/icb/34.1.100
Thompson, B. A. W., Goldsworthy, P. M., Riddle, M. J., Snape, I., and Stark, J. S. (2007). Contamination effects by a conventional and a biodegradable lubricant oil on infaunal recruitment to Antarctic sediments: a field experiment. J. Exp. Mar. Biol. Ecol. 340, 213–226. doi: 10.1016/j.jembe.2006.09.010
Vanhove, S., Arntz, W., and Vincx, M. (1999). Comparative study of the nematode communities on the southeastern Weddell Sea shelf and slope (Antarctica). Mar. Ecol. Prog. Ser. 181, 237–256. doi: 10.3354/meps181237
Vanhove, S., Beghyn, M., Van Gansbeke, D., Bullough, L., and Vincx, M. (2000). A seasonally varying biotope at Signy Island, Antarctic: implications for meiofaunal structure. Mar. Ecol. Prog. Ser. 202, 13–25. doi: 10.3354/meps202013
Vanhove, S., Lee, H., Beghyn, M., Van Gansbeke, D., Brockington, S., and Vincx, M. (1998). The metazoan meiofauna in its biogeochemical environment: the case of an Antarctic coastal sediment. J. Mar. Biol. Assoc. U. K. 78, 411–434. doi: 10.1017/s0025315400041539
Vanhove, S., Wittoeck, J., Desmet, G., Van den Berghe, B., Herman, R., Bak, R., et al. (1995). Deep-sea meiofauna communities in Antarctica: structural analysis and relation with the environment. Mar. Ecol. Prog. Ser. 127, 65–76. doi: 10.3354/meps127065
Vanreusel, A., Fonseca, G., Danovaro, R., Da Silva, M. C., Esteves, A. M., Ferrero, T., et al. (2010). The contribution of deep-sea macrohabitat heterogeneity to global nematode diversity. Mar. Ecol. 31, 6–20. doi: 10.1111/j.1439-0485.2009.00352.x
Veit-Köhler, G., Durst, S., Schuckenbrock, J., Hauquier, F., Suja, L. D., Dorschel, B., et al. (2018). Oceanographic and topographic conditions structure benthic meiofauna communities in the Weddell Sea, Bransfield Strait and Drake Passage (Antarctic). Progr. Oceanogr. 162, 240–256. doi: 10.1016/j.pocean.2018.03.005
Vezzulli, L., Moreno, M., Marin, V., Pezzati, E., Bartoli, M., and Fabiano, M. (2008). Organic waste impact of capture-based Atlantic bluefin tuna aquaculture at an exposed site in the Mediterranean Sea. Estuar. Coast. Shelf Sci. 78, 369–384. doi: 10.1016/j.ecss.2008.01.002
Warwick, R. M. (1986). A new method for detecting pollution effects on marine macrobenthic communities. Mar. Biol. 92, 557–562. doi: 10.1007/BF00392515
Warwick, R. M., Platt, H. M., and Somerfield, P. J. (1998). “Freeliving marine nematodes: part III. Monhysterida,” Synopses of the British Fauna (New Series) (Shrewsbury: Field Studies Council), 53:296.
Wild, S., McLagan, D., Schlabach, M., Bossi, R., Hawker, D., Cropp, R., et al. (2015). An antarctic research station as a source of brominated and perfluorinated persistent organic pollutants to the local environment. Environ. Sci. Tech. 49, 103–112. doi: 10.1021/es5048232
Witthoft-Muhlmann, A., Traunspurger, W., and Rothhaupt, K. O. (2005). Meiobenthic response to river-borne benthic particulate matter-a microcosm experiment. Freshw. Biol. 50, 1548–1559. doi: 10.1111/j.1365-2427.2005.01425.x
Keywords: meiofauna, benthic community, marine sediments, metals, Antarctic, human impacts, nematodes, copepods
Citation: Stark JS, Mohammad M, McMinn A and Ingels J (2020) Diversity, Abundance, Spatial Variation, and Human Impacts in Marine Meiobenthic Nematode and Copepod Communities at Casey Station, East Antarctica. Front. Mar. Sci. 7:480. doi: 10.3389/fmars.2020.00480
Received: 29 November 2019; Accepted: 28 May 2020;
Published: 30 June 2020.
Edited by:
Rui Rosa, University of Lisbon, PortugalReviewed by:
Thomas Soltwedel, Alfred Wegener Institute, Helmholtz Centre for Polar and Marine Research (AWI), GermanyMarcos Rubal, University of Porto, Portugal
Copyright © 2020 Stark, Mohammad, McMinn and Ingels. This is an open-access article distributed under the terms of the Creative Commons Attribution License (CC BY). The use, distribution or reproduction in other forums is permitted, provided the original author(s) and the copyright owner(s) are credited and that the original publication in this journal is cited, in accordance with accepted academic practice. No use, distribution or reproduction is permitted which does not comply with these terms.
*Correspondence: Jonathan S. Stark, am9ubnkuc3RhcmtAYWFkLmdvdi5hdQ==; Jeroen Ingels, amluZ2Vsc0Bmc3UuZWR1
†ORCID: Jonathan S. Stark, orcid.org/0000-0002-4268-8072
‡Present address: Mahadi Mohammad, School of Biological Sciences, Universiti Sains Malaysia, George Town, Malaysia