- 1Key Laboratory of Experimental Marine Biology, Center for Ocean Mega-Science, Institute of Oceanology, Chinese Academy of Sciences, Qingdao, China
- 2Laboratory for Marine Biology and Biotechnology, Qingdao National Laboratory for Marine Science and Technology, Qingdao, China
- 3College of Earth Science, University of Chinese Academy of Sciences, Beijing, China
- 4Department of Benthic Ecology, GEOMAR Helmholtz-Zentrum für Ozeanforschung Kiel, Kiel, Germany
- 5Excellence Center for Biodiversity of Peninsular Thailand, Faculty of Science, Prince of Songkla University, Hat Yai, Thailand
- 6Center of Marine Sciences, Faculty of Sciences and Technology, University of Algarve, Faro, Portugal
Ecological processes and intra-specific genetic diversity reciprocally affect each other. While the importance of uniting ecological variables and genetic variation to understand species’ plasticity, adaptation, and evolution is increasingly recognized, only few studies have attempted to address the intersection of population ecology and genetics using marine macrophyte as models. Representative empirical case studies on genetic diversity are reviewed that explore ecological and evolutionary processes in marine macrophytes. These include studies on environment-induced phenotypic plasticity and associated ecological adaptation; population genetic variation and structuring driven by ecological variation; and ecological consequences mediated by intraspecific and interspecific diversity. Knowledge gaps are also discussed that impede the connection of ecology and genetics in macrophytes and possible approaches to address these issues. Finally, an eco-evolutionary perspective is advocated, by incorporating structural-to-functional genomics and life cycle complexity, to increase the understanding of the adaptation and evolution of macrophytes in response to environmental heterogeneity.
Introduction
Local adaptive evolution (see explanation in Box 1, other terms are also explained in the same Box) and the evolution of phenotypic plasticity are both vital processes enabling organisms in a population to obtain maximum fitness in response to heterogeneous environments. In the marine environment, migratory connectivity via genetic exchange of adults, spores or propagules, is a salient difference compared with terrestrial ecosystem (Palumbi, 1994). Marine connectivity counteracts local adaptation on one hand (so it may decrease fitness locally), but by increasing gene flow (NeM) it allows recolonization, reduces consanguinity, and may increase effective size at the global (species or metapopulation) level (Bolnick and Nosil, 2007). Therefore, whether selection favors phenotypic plasticity or local adaptation will depend on the balance between spatial environmental heterogeneity and dispersal-mediated gene flow (van Tienderen, 1991). Classic ecological genetic studies usually combined field (e.g., reciprocal transplant) and laboratory (e.g., common garden) approaches to investigate how natural selection generated adaptive variability among populations along different ecological gradients (Schmidt et al., 2008). In recent years, the emergence of ecological/landscape genomics based on next-generation sequencing has provided more comprehensive insights into the ways in which species respond to their changing environments and into the limitations of their potential to adapt (Savolainen et al., 2013). All these types of evidence hint at how genotype, phenotype, and environment interact as a whole to ultimately influence adaptive capability and evolvability (Figure 1).
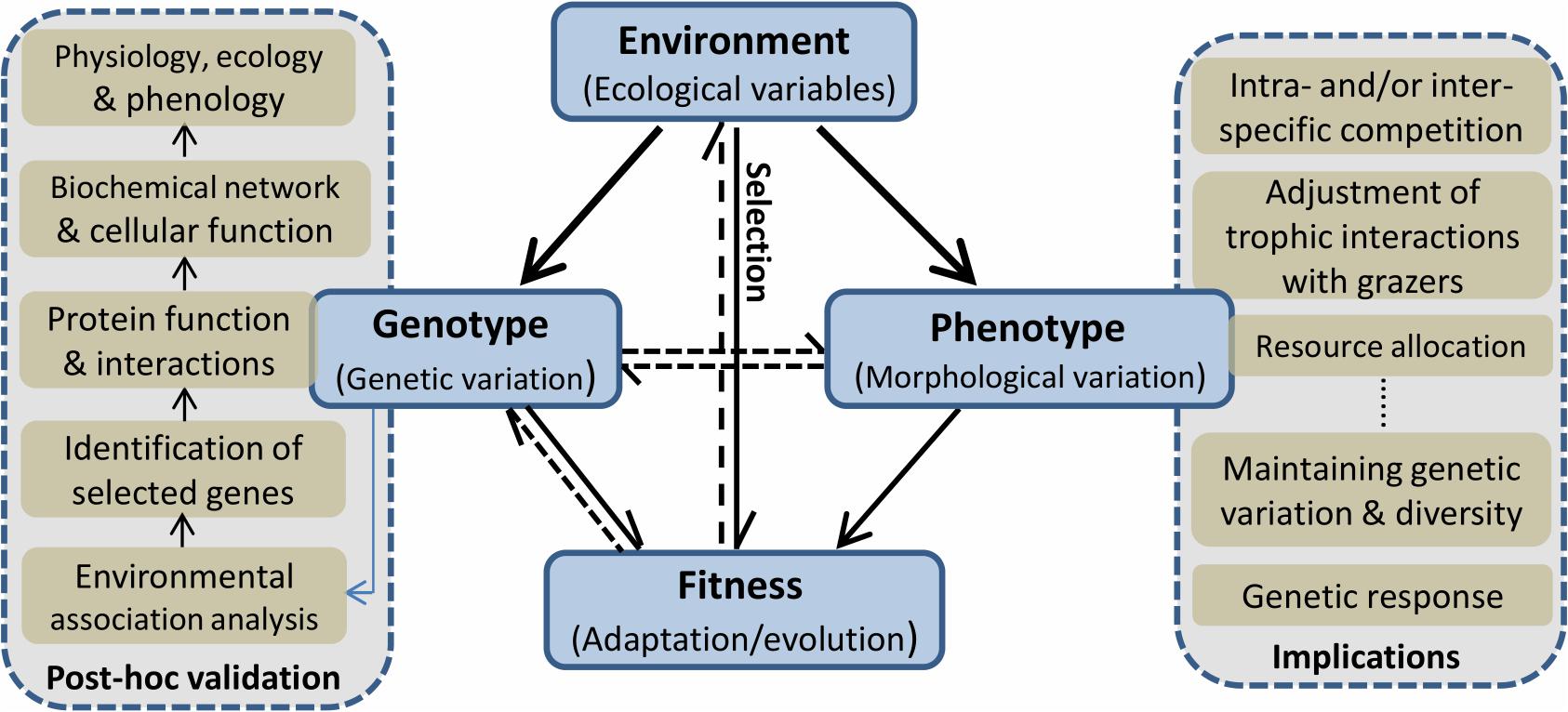
Figure 1. A simplified diagram showing the interactive relationships among environment, phenotype, and genotype, which ultimately impact fitness. The identified genotypes linked to specific adaptation can be validated in a post hoc process, including the initial association analysis with the selected environmental variables, identification of the target genes under selective pressure, and subsequently protein function and interactive network with other molecules, and finally functional identification at a tissue level. At the same time, the evolved plastic phenotypes have wide ecological implications, such as the overwhelming competition to the rival, the adjustment of community balance, and resource allocation under a harsh environment. Solid lines indicate the main research fields connecting one to another in macrophytes, and dashed lines indicate the research gaps that need to be focused on in the future.
BOX 1. Glossary of terms, defined in the context to link Ecology and Genetics in marine macrophytes.
Acclimation: A phenotypic/physiological/metabolic change during an individual’s lifetime.
Adaptation: A trans-generational fixed change at population level.
Adaptive evolution: A kind of evolutionary change that is adaptive to a particular environment, which enables an organism to cope with the environment.
Adaptive genetic variation: The variation found in the genomes that has evolved to help an organism to increase its fitness.
Clonal propagation: Producing units derived from the same original reproductive cell that have the capacity for independent life, e.g., ramets (fronds).
Co-dominant: A locus where all its alleles are expressed, and heterozygotes can therefore be discriminated from homozygotes.
Cryptic genetic variation: Unexpressed, bottled-up genetic variation that may become expressed in a new environment.
Divergent selection: The accumulation of differences between closely related populations within a species, which eventually may lead to speciation.
Fitness: The ability of a phenotype–more rarely, population or species–to survive and reproduce in an environment it inhabits.
Genetic assimilation: A process whereby environmentally induced phenotypes become genetically fixed and no longer require the original environmental stimulus.
Introgression: The transfer of genetic information from one species to another as a result of hybridization between them and repeated backcrossing.
Landscape genetics: A scientific field aiming to study how landscape features interact with microevolutionary processes (e.g., gene flow, genetic drift, and selection).
Landscape genomics: Combines genomic concepts and technologies with landscape genetics approaches.
NeM: The average number of effective migrants exchanged per generation. Ne is the product of the effective population size, and M is the migration rate.
Neutral genetic variation: A gene or locus that has no effect on fitness or may be subject to various constraints; large parts of an organism’s DNA are effectively neutral.
Outlier: An observation (or statistical measurement) that has an extreme value compared with the distribution of other FST ≅ 1/(4Nm+1) observations.
Phenotypic plasticity: The ability of one genotype to generate more than one phenotype when exposed to different environmental conditions by modifying phenotypic traits during an individual’s lifetime (Bradshaw, 1965).
Quantitative Trait Loci: Sections of the genome that correlate with a particular phenotypic trait of a population or an organism.
Seascape genetics: A scientific field aiming to study how spatially variable structural and environmental features affect genetic profiles of marine organisms.
Seaweeds (macroalgae) and seagrasses, collectively termed marine macrophytes, are ecologically important primary producers, carbon sinks, and ecosystem engineers, and highly adaptive to environmental shifts (Reusch et al., 2005; Harley et al., 2012). Ecology and genetics can each influence diversity, plasticity, adaptation, and evolution of marine macrophytes (Pereyra et al., 2009; Padilla and Savedo, 2013; Duarte et al., 2018; Wernberg et al., 2018; Figure 1). Ecological factors, such as habitat preferences, species interactions, and environmental gradients, can generate variable allelic frequencies in macrophyte populations at specific genetic loci that are associated with differentiated phenotypes (Schmidt et al., 2008), enabling them to adapt to different environments (e.g., Bergström and Kautsky, 2006; Hays, 2007). In turn, genetic variation/diversity can alter or modify the fitness and evolvability of organisms, and hence the way species metabolize, grow and survive, and ultimately interact with a changing ecosystem (e.g., Bracken et al., 2008; Hughes et al., 2008). Unraveling the link between adaptive genetic variation, ecological adaptation, and phenotypic alteration is also a major goal in evolutionary biology and conservation biogeography of macrophytes under climate change (Harley et al., 2012; Hu and Fraser, 2016).
There is a widespread conceptual recognition that ecological and genetic methods should be united to study adaptation, speciation, and morphological diversity of marine macrophytes (Serrão et al., 1996; Gabrielsen et al., 2002; Leskinen et al., 2004; Reusch et al., 2005; Bracken et al., 2008; Pereyra et al., 2009; Krueger-Hadfield et al., 2013; Zardi et al., 2013; Shimada et al., 2016). However, the respective roles of ecological processes and genetic variation are studied separately in macroalgae adapting to heterogeneous environments (reviewed in Hu et al., 2016). Only recently studies considered how the intersection of ecological, genetic, and phenotypic traits may produce and maintain divergent selection, genetic boundaries, and functional vulnerability in macroalgae (e.g., Zardi et al., 2015; Saada et al., 2016; Wernberg et al., 2018), including the identification of neutral genetic variation responding to environmental gradients (e.g., Kostamo et al., 2012; Zardi et al., 2013). Here we summarize the major findings and challenges associated either with ecological processes or genetic diversity in macrophytes responding to environmental shifts. We choose representative studies to illustrate how typical ecological factors (e.g., temperature, salinity, and water depth) contributed to genetic variation and population structure in macroalgae and seagrasses, and how the change of intra- or inter-specific diversity alter species’ responses to environments, and hence ecosystem consequences. We finally stress the necessity to select suitable models/systems and incorporate ploidy diversity (Krueger-Hadfield et al., 2019) in natural populations to accelerate the union of genetics and ecology in macrophytes. It must be stressed out that most study cases selected in this review are in theory to show signals of local adaptation and evolution in response to changing environments, because no sophisticated analysis methods and robust evidence are included to support these presumptions. However, these cases highlight a new avenue for studying the genomic basis of adaptation and evolution in macrophytes in the near future.
Phenotypic Plasticity and Adaptation in Marine Macrophytes
Phenotypic plasticity plays a fundamental role in plant adaptation, development, and function. Environment-induced phenotypic plasticity can be locally adaptive under various conditions (Pfennig et al., 2010), over a range of spatial and temporal (e.g., seasonal) scales. In some extreme cases, heterogeneous environments may not be responsible for divergent selection upon all genotypes harboring the same fitness regardless of a given environmental parameter, but the plasticity of a given trait is still expected to yield a reduced efficiency of selection in promoting adaptive processes. This can be formulated as a heuristic model of how phenotypic plasticity can affect population viability, genetic variation, and adaptive evolution (Gomez-Mestre and Jovani, 2013). Phenotypic plasticity can also be considered as a trait per se which can vary according to the benefits/constraints it brings to the adaptive process. When plasticity increases fitness, then selection favors individuals with higher plasticity. When it comes with a fitness cost (e.g., metabolic cost of the plastic response), then selection may favor specialized phenotypes with limited plasticity (Pfennig et al., 2010; Padilla and Savedo, 2013).
Macrophytes are expected to be subject to rapid selection for phenotypic plasticity, because they are sessile and many species are capable of genotypic replication through clonal propagation (e.g., ramets, Scrosati and Servière-Zaragoza, 2000). These traits are expected to promote larger phenotypic plasticity relative to motile and non-clonal taxa (Bradshaw, 1965). In macroalgae, fragments are in particular capable of independent life and therefore genetic individuals (genets) may fragment naturally into ramets that may act as independent units in selection (Tuomi and Vuorisalo, 1989). Indeed, a high degree of phenotypic plasticity was found within genetically uniform strains of the green alga Acetabularia acetabulum (L.) P.C.Silva grown under different environmental conditions (Nishimura and Mandoli, 1992), which has triggered many studies concerning the factors influencing phenotypic variation. At the same time, the great phenotypic variation in macroalgae also results from shifting environmental variables in space (e.g., light intensity, wave action, tide, temperature, salinity, nutrient concentrations, predator–prey interactions) and associated selective fitness, ultimately generating spatial variation in adaptation (Monro et al., 2007; Padilla and Savedo, 2013). This is particularly true for macroalgae with clonal propagation because it allows them to develop either intra-genet phenotypic plasticity acclimating to environmental change within short time periods or intra-population variation to ecological differences between geographic locations, leading to markedly different demographic fitness (Monro et al., 2007; Flukes et al., 2015; Duarte et al., 2018).
Plasticity may not only facilitate acclimation to environmental changes, but also mediate the interplay among gene flow and adaptation resulting from efficient divergent selection in macrophytes. Theoretical simulation has shown that phenotypic traits can evolve under directional selection and the fitness effect of increased plasticity can promote or inhibit genetic exchange between selective environments (Crispo and Chapman, 2008). Therefore, phenotypic plasticity can either inhibit adaptive genetic divergence and the effect of natural selection or promote acclimation by allowing individuals to survive in novel conditions (Crispo, 2008). Understanding such interactions involving phenotypic plasticity provides an opportunity to unravel the long-term evolutionary consequences and the ecological implications of different phenotypic patterns in the sea (Padilla and Savedo, 2013). Intra-specific trait variation can be due to heritable differences between individuals or to different environments. Suitable approaches for the distinction of plastic and heritable variation are common garden experiments and pedigree studies (Aitken and Whitlock, 2013), which are also challenging because macrophytes can be strikingly plastic in phenotypic traits.
The Correlation Between Genotype and Ecological Factors
Selkoe et al. (2016) cataloged 23 abiotic factors that were tested for correlation with spatial genetic structure in the marine environment. These ecological variables often interact, leading to a complex environment that is often highly variable in space and time. In most cases, it is therefore difficult to determine which ecological driver directly contributed to any population genetic feature. Nevertheless, there is empirical evidence that light, temperature, salinity, and water motion are the major abiotic variables affecting macroalgae (Hurd et al., 2014). This narrows the possibilities and allows us to detect genetic variation in macroalgae from the micro- to the macro-geographical level.
Most of the recent progress in studying the links between ecological processes and genetic variation is strongly dependent on breakthroughs of modern DNA-based technology (Table 1). Already before the 1990s, studies of allozyme patterns have clearly shown associations between levels of genetic variation and species-specific attributes (e.g., mating systems) (Sosa and Lindstrom, 1999). During the last 20 years, co-dominant genetic markers and DNA sequencing have been increasingly used to study genetic differentiation, population connectivity, and ecological adaptation in macrophytes (among others: Valero et al., 2001; Guillemin et al., 2016; Hu et al., 2016; Macaya et al., 2016; Wennerström et al., 2017). More recently, genome-scale restriction site-associated DNA (RAD) and genotyping-by-sequencing (GBS) allowed the assessment of the interplay of biotic and abiotic factors through the production of subtle genetic variation and evolutionary signatures during recent or incipient speciation events (Fraser et al., 2016; Kobayashi et al., 2018). Here, we present a selection of representative findings that explain how ecological factors may structure population genetic variation in macroalgae (summarized in Table 2).
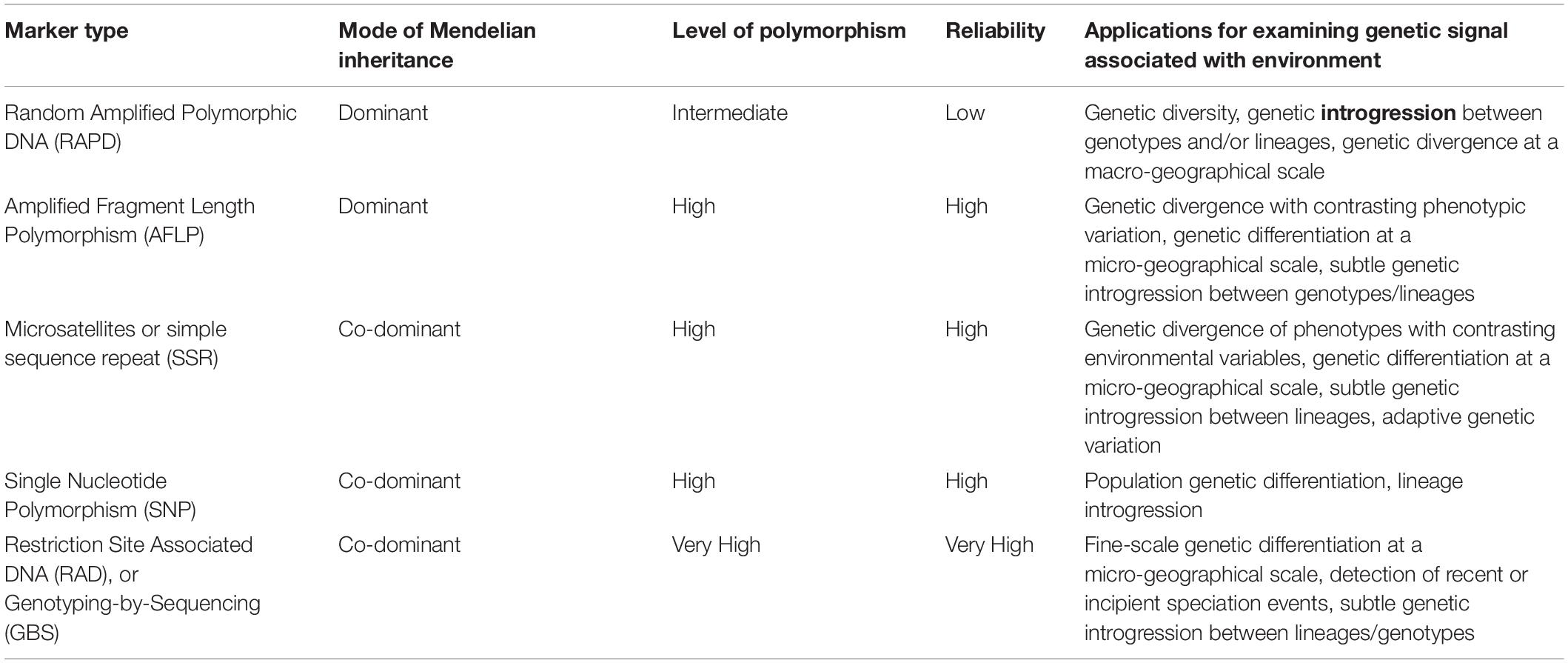
Table 1. A brief description of modern molecular techniques used to examine different levels of genetic variation in the context of the interaction of environment and genotype.
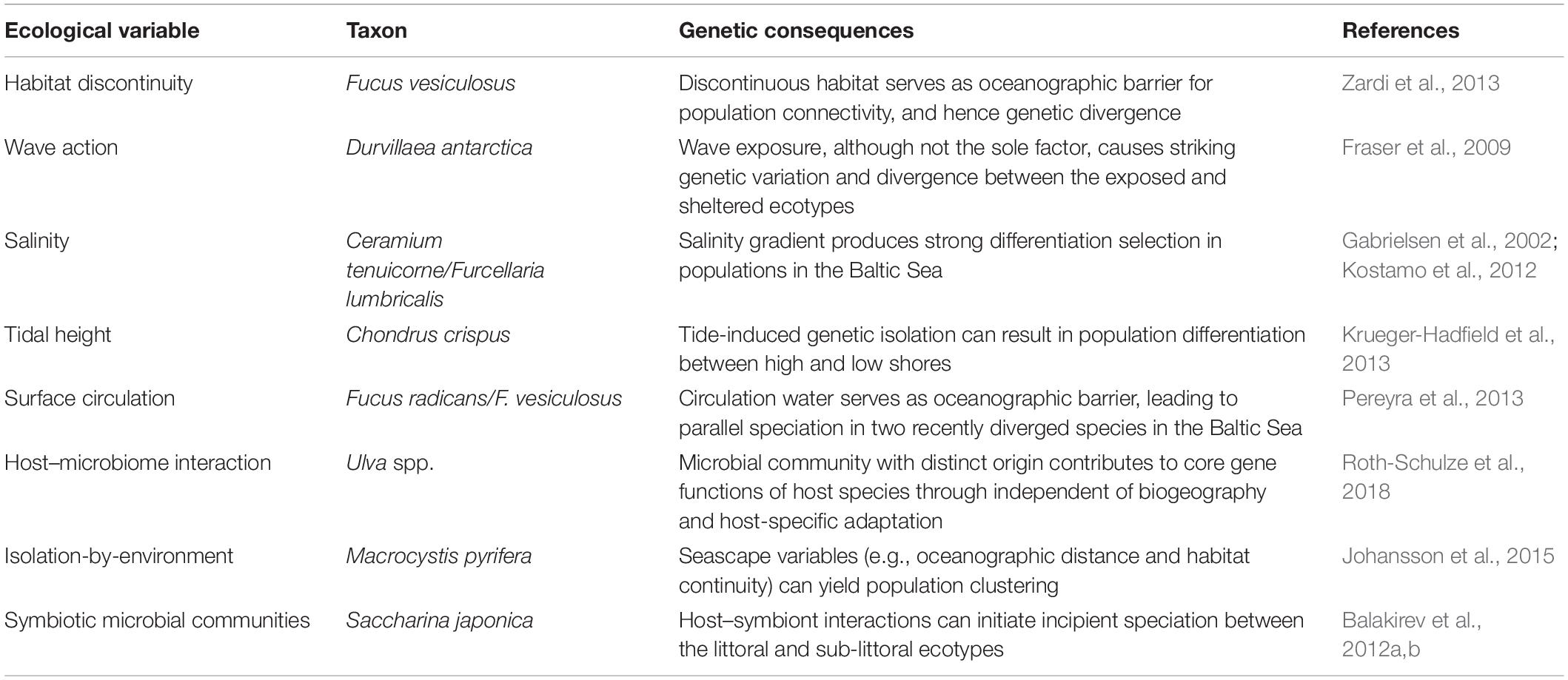
Table 2. Summary of studies illustrating the contribution of ecological variables to genetic differentiation in macroalgae.
High and low intertidal individuals experience different durations of emersion and immersion time. Such contrasting abiotic stresses can produce divergent habitat selection and hence genetic separation in macroalgae (e.g., Billard et al., 2010). Fraser et al. (2009) reported consistent genetic divergence between populations of the bull kelp Durvillaea antarctica (Chamisso) Hariot growing in sheltered and exposed habitats. In this fucoid, genetic divergence was associated with contrasting phenotypic variation along gradients of wave exposure, suggesting that the dynamic environmental variation may enable fitness to be fixed in these two phenotypes with variable eco-physiological cost and subsequently adaptive divergent selection occurs. Similarly, Krueger-Hadfield et al. (2013) found that Chondrus crispus Stackhouse populations at the high shore [mean low water (MLW) + 3.6 m] clearly diverged genetically from the low shore (MLW + 2 m) near Roscoff in Brittany, France. The isolation imposed by tidal cycles of emersion was suggested to have increased population genetic differentiation between the higher and lower stands. These studies suggest that new recruits either settle near the parents or, alternatively, there is post-settlement selection for certain genotypes/phenotypes that can yield micro-geographical genetic divergence and adaptation linked to strikingly limited gene flow.
Several examples come from algae which inhabit marine to brackish waters. Previous studies in macroalgae have shown that asexual reproduction dominates in hyposaline waters, whereas sexual reproduction is common in high salinity conditions (Tatarenkov et al., 2005; Kostamo and Mäkinen, 2006). Tatarenkov et al. (2005) explicitly connected genotypic variation in Fucus vesiculosus L. and adaptive shifts of life history caused by salinity variation, which strongly affected reproductive fitness and determined distinct salinity range boundaries among populations (Serrão et al., 1996, 1999). More recently, Kostamo et al. (2012) found high genetic differentiation in Furcellaria lumbricalis (Hudson) J.V.Lamouroux populations along a wide-ranging salinity gradient using expressed sequence tags (EST)-derived microsatellites. Two specific genetic loci reflected the strong divergent selection of different salinities. Such a differentiation between marine and brackish populations was further determined in expressed genomic regions of F. lumbricalis, illustrating that suboptimal salinity is an ecological variable that strongly affects population structure (Korpelainen, 2016). Other examples are the red alga Ceramium tenuicorne (Kützing) Waern (Gabrielsen et al., 2002) and the green alga Ulva intestinalis L. (Leskinen et al., 2004), which from the Atlantic Ocean into the brackish Baltic Sea show a continuous reduction of genotype diversity. Its phenotypes inhabiting hyposaline environments exhibited better adaptive ability to cope with very low levels of salinity. These population genetic findings, without transplantation or common garden experiments, show cases of seaweed distributions in salinity gradients which are driven by locally adapted genotypes.
Water depth is one of the five most studied drivers that shape the population genetic structure of marine organisms (Selkoe et al., 2016). Despite a close genetic affinity between distinct phenotypes of the brown alga Saccharina japonica (Areschoug) C.E. Lane, C. Mayes, Druehl, and G.W. Saunders in deep (14–25 m) vs. shallower depths (5–11 m) (Balakirev et al., 2012a), their associated microsymbiont communities were significantly different (Balakirev et al., 2012b). Such shifts of symbiotic microorganisms have caused visible phenotypic plasticity and potentially initiate some reproductive isolation between these two groups in S. japonica. Therefore, these studies are examples of how phenotypic diversification of macroalgae may enable them to acclimate or adapt to distinct bathymetric environments and, at the same time, they also highlight the important role of host–symbiont interactions as drivers of adaptation in macroalgae.
Top-down control by grazers is another well-documented factor that may shape intra-specific diversity and affect ecological and genetic dynamics in aquatic ecosystems (Jürgens and Matz, 2002; Crutsinger et al., 2008). For instance, field and experimental evidence have shown that increased grazing of planktonic bacteria may result in changing phenotypic and genotypic compositions of bacterial assemblages (Jürgens and Matz, 2002). In bacterial communities compared across three species of the green algal genus Ulva from different geographic locations, 70% of enriched functional genes were independent of host species and biogeography, demonstrating that microbial diversity with distinct phylogenetic origin contributed to host/habitat-specific gene functions (Roth-Schulze et al., 2018). These studies imply that the dynamic interactions between genetic variation and ecological traits can be driven by rapid evolution and adaptation in predator-prey systems and that ecological and genetic processes can happen on a similar time scale (Yoshida et al., 2003).
Genetic Diversity Shifts May Have Ecological Consequences
Shifts in genetic variation (i.e., changes in allele frequencies) are associated with species’ traits. These changes can influence fitness by natural selection and phenotypic variation can facilitate local adaptation of species (Endler, 1986). Genetic diversity shifts can occur at the population level (intra-specific) or at the community level (inter-specific). Studies in marine macrophytes illustrate that more intra-specific or inter-specific genetic variation can increase species’ growth, productivity, competition, and recovery after stress (Hughes and Stachowicz, 2004; Bruno et al., 2005; Reusch et al., 2005; Bracken et al., 2008). In fact, ecologically important traits [e.g., growth rate, reproductive ability (Reed et al., 1996), recruitment process, trade-off between sexual and asexual reproduction (Chu et al., 2011)] exhibit distinguishable variation that is closely associated with a species’ abundance and demographic performance. Such ecological effects imposed by genetic variation in a single species can extend to species and/or functional diversity in both community- and ecosystem-levels (Bruno et al., 2005; Hughes et al., 2008; Wernberg et al., 2018). The union of ecology and genetics can thus reveal the ecological consequences of intra-specific or inter-specific genetic variation and help us to understand mechanistic processes. There is a long history of research on how phenotypic plasticity of individuals inhabiting contrasting environments influences the adaptation of macrophytes to dynamic environments (Padilla and Savedo, 2013). In contrast, much less is known about the ecological effects of intra-specific genetic variation or diversity in macrophytes. However, ecological effects of inter-specific variation are relatively well studied in the context of the biodiversity-ecosystem functioning level.
Studies testing effects of intra-specific genotypic composition on ecological processes in marine macrophytes have mainly been conducted in seagrasses, but have also been done in macroalgae (Table 3). Field experiments with Eastern Atlantic Fucus serratus L. have shown that populations at the rear edge, characterized by low genetic diversity, had reduced fitness and lower adaptive capacity relative to more diverse populations in the distribution center (Pearson et al., 2009). Within-patch intra-specific genotypic diversity of the invasive red alga Agarophyton vermiculophyllum (Ohmi) Gurgel, J.N.Norris, and Fredericq in North America correlated with seaweed biomass and epibacterial density (Gerstenmaier et al., 2016). Field experiments outplanting monoclonal and polyclonal patches further indicated a threefold increase in net primary productivity in polyclonal patches (Gerstenmaier et al., 2016). Genetic composition can also impose effects on the community dynamics across trophic levels through rapid evolution (Pimentel, 1968). Laboratory manipulations further revealed how shifts of genetic variation affected ecological performance. Experimental field tests in transplantation experiments showed that intra-specific genetic variation of the rockweed F. vesiculosus had different effects on associated communities. By analyzing the associated periphytic algae and invertebrates as well as defense compounds and genetic profiling, the authors found that the composition of periphytic and invertebrate communities can be partially (10–18%) explained by multiple rockweed genotypes with different intra-specific variation. The authors implied that genetic variation of a foundation alga is a requisite component for structuring the function of associated communities (Jormalainen et al., 2017).
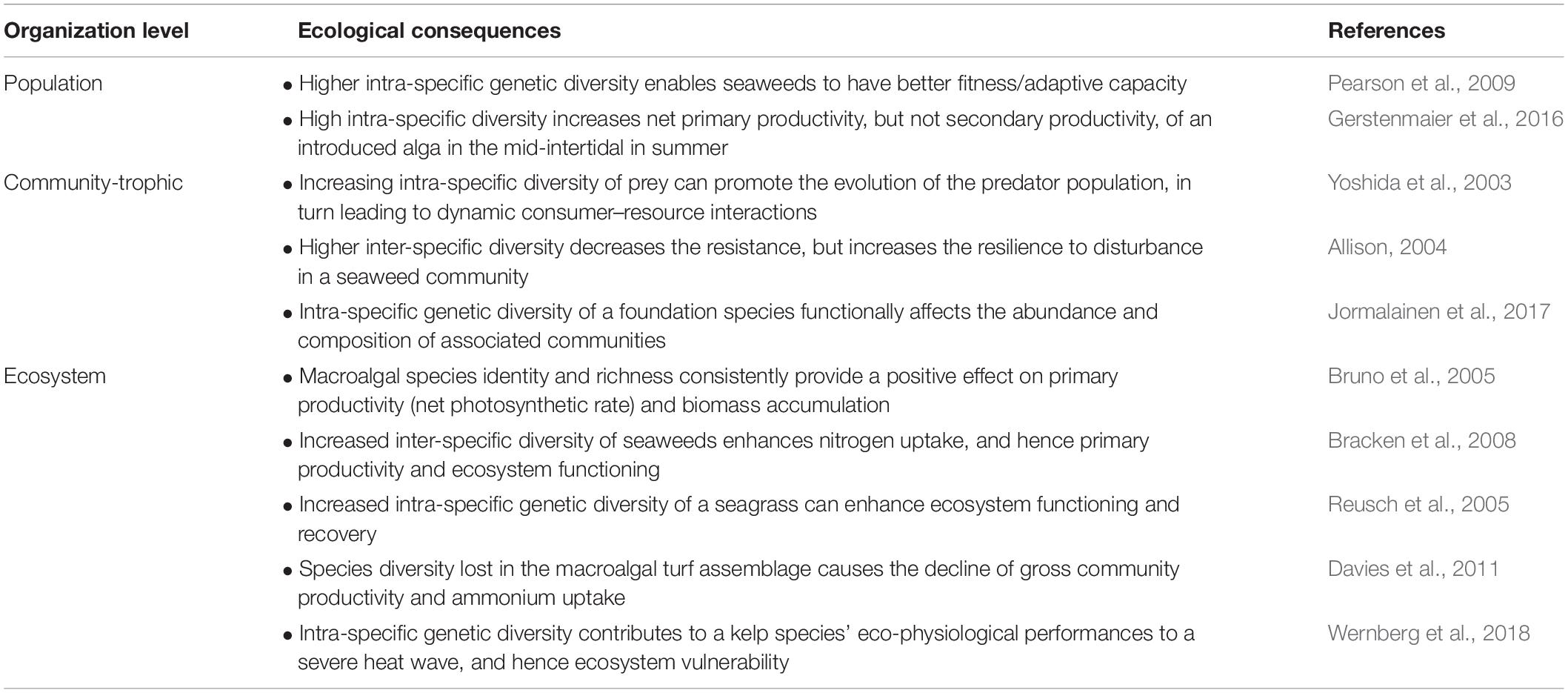
Table 3. Summary of studies illustrating the ecological consequences of intra-specific or inter-specific genetic diversity at different organization levels in marine macrophytes.
Two of the most fundamental characteristics of any ecosystem are its community (species composition) and the species interactions. Genetic variation affects the overall strength of interaction networks and single species interactions. Both competition among plants and plant–herbivore interactions can have an important effect on the relationship between biodiversity and the biomass of various trophic levels (Thébault and Loreau, 2005). Hence, studying the relationships between genetic variation and ecosystem stability has become a major focus of research, as it may facilitate to understand whether and how species diversity and genetic diversity help to maintain ecosystem processes under global change scenarios. Based on manipulative field experiments, Hughes and Stachowicz (2004) and Reusch et al. (2005) found that increasing genotypic diversity in a population of the seagrass Zostera marina L. enhanced biomass production, faunal abundance, and community resistance to disturbance by grazers. This was reflected in a decreased time requirement for recovery to levels close to pre-disturbance density. Importantly, this kind of enhanced primary production and biomass accumulation was also observed in subtidal macroalgal communities by enriched species diversity (Bruno et al., 2005). In the same way, Bracken et al. (2008) evaluated the functional consequences of realistic inter-specific diversity changes in a seaweed ecosystem. They manipulated tide pool communities to study the effects of changes in biodiversity and observed that a natural increase in species diversity enhanced rates of nitrogen uptake, whereas random changes of species diversity had no impact. Another field manipulation experiment showed that both gross community productivity and biomass declined with diversity loss in a macroalgal turf assemblage. The biodiversity–ecosystem process relationship, however, was dependent on the extinction resistance of the dominant and most resistant species (Davies et al., 2011). In a foundation kelp species [Ecklonia radiata (C.Ag.) J.Ag.], population performance and vulnerability to a marine heatwave were strongly correlated to latitudinal patterns in intra-specific genetic variation. A heat wave extirpated kelp forests with low genetic diversity (Wernberg et al., 2018), demonstrating a relationship between intra-specific genetic variation and ecological resilience. This is an empirical example of how loss of intra-specific genetic diversity could likely reduce a species’ phenotypic plasticity or adaptability, and ultimately drive widespread cascading effects on ecosystem structure and functioning. However, another field plotting experiment showed that nutrient conditions, rather than genetic heterozygosity of the strains, affected the farming productivity and physiological response of the red alga Agarophyton chilense (C.J.Bird, McLachlan, and E.C.Oliveira) Gurgel, J.N.Norris and Fredericq (as Gracilaria chilensis) in Chile (Usandizaga et al., 2018). It is thus important to study both abiotic and genetic factors to better understand acclimation and/or adaptation of marcroalgae responding to environmental change.
Gaps in the Link Between Ecology and Genetics in Macrophytes
For long, there exists a knowledge gap in the connection between ecology and genetics of macrophytes, especially in algae (Valero et al., 2001; Hughes and Stachowicz, 2004). In part this may be attributed to limited research interest and conceptual gaps in existing approaches that try to integrate population genetic tools into ecological studies, despite technical viability. Since the 1990s, eco-genetic studies have mainly been conducted on fish, gastropod, barnacle, and mussel models, with the primary aim to identify specific genetic loci that are correlated with environmental shifts and adaptation in the North Atlantic region (Schmidt et al., 2008). These studies mostly used allozymes, microsatellites, and DNA sequencing (Table 1) and found strong historical and demographic footprints in the observed clines of genetic variation, as well as secondary inter-gradation between genetically subdivided populations (Schmidt et al., 2008). However, signs of local adaptation (e.g., FST outlier analysis) may often be a result of secondary introgression and endogenous (e.g., environment-independent) genetic barriers known as tension zones (Bierne et al., 2011). Macroalgae such as F. vesiculosus and C. tenuicorne, but also U. intestinalis and Ulva compressa (Leskinen et al., 2004; Steinhagen et al., 2019a, b) are clearly subject to ecological variation (e.g., reproductive mode, resistance to freezing and desiccation, morphology) along the Baltic Sea and the North Sea coasts, that differ considerably in several environmental parameters such as salinity and tide. Therefore, they provide interesting model systems for the screening of neutral vs. non-neutral markers in populations that are subject to environmental selection (e.g., by salinity or duration of air exposure during low tide). However, a quantitative identification of genetic and phenotypic traits under selection is still largely missing.
Phenotypic plasticity, genetic assimilation, cryptic genetic variation, and widespread environmental changes can interplay with each other to generate adaptive radiations and divergence in marine species (Schneider and Meyer, 2017; Sandoval-Castillo et al., 2018). This situation leads to considerable challenges when adaptive genetic variation has to be discriminated from the standing stock variation induced by environmental conditions. It also leads to technical challenges in the application of high-throughput screening methods [e.g., single nucleotide polymorphism (SNP)] when phenotypic adaptation of macrophytes has to be linked to genetic variation. For instance, genome-wide SNP genotyping has allowed for the identification of genetic signatures of adaptive genetic variation after selection by stresses in plants and insects (Namroud et al., 2008; Chávez-Galarza et al., 2013). However, to our knowledge, SNPs have been primarily used to study the overall population genetic structure in macroalgae (Canovas et al., 2011; Olsson and Korpelainen, 2013; Provan et al., 2013; Yesson et al., 2018), including the effect of water depth and light radiation on the distribution of cryptic algal species using seascape genomics (De Jode et al., 2019). Only in a few cases SNPs have been employed to detect neutral or adaptive genetic loci linking to adaptive traits, despite quantitative trait loci (QTL) identification of SNPs associated to thermal adaptation and available plasticity (Cerutti et al., 2011; Flowers et al., 2015; Avia et al., 2017). Future studies could focus on species exhibiting clear phenotypic divergence among environments as those mentioned above and use genome-scale genotyping to link genetic variation to local adaptation and associated molecular mechanisms. These may involve disentangling plasticity from heritable variation before searching for putative loci under divergent selection among habitats. At the same time, when reference genomes become available, experimental designs and bioinformatic pipelines need to take into consideration of the haploid–diploid life cycles in macroalgae (Krueger-Hadfield et al., 2019).
Solutions: Selecting Suitable Research Models and Systems
To unite population ecology and genetics, one intuitively seeks to focus on ecological factors within a changing environment, which can differ categorically (e.g., subtidal vs. intertidal or exposed vs. sheltered, or south coast vs. north coast of an island), change gradually along a coast (e.g., salinity or temperature), or scatter more or less randomly through the entire distribution range of a species (e.g., substrate type) (Rellstab et al., 2015). For macrophytes, for example, salinity, water depth, and tidal amplitude (Kostamo et al., 2012; Krueger-Hadfield et al., 2013) are potential target factors that could come into focus if ecological adaptation under divergent natural selection is to be studied. From this point of view, seascape genetics is an ideal approach to unite ecology and genetics of macrophytes. For instance, early seascape genetics in macroalgae indicated that habitat continuity (Alberto et al., 2010), oceanographic distance (Alberto et al., 2011), and continental boundary currents (Coleman et al., 2011) can contribute to range-wide population connectivity and genetic differentiation in kelps, as well as in fucoids (Buonomo et al., 2017). Vertical gradients can cause strong structure at smaller scale, as tidal range can structure micro-geographic population genetic variation in the red alga C. crispus (Krueger-Hadfield et al., 2013). Seascape studies showed that not only cross-shore distribution range and residence depth, but also oceanographic transport, isolation-by-environment, and the availability of suitable intervening habitat can determine the genetic structure of kelp populations (Robuchon et al., 2014; Johansson et al., 2015; Durrant et al., 2018), highlighting that population genetic variation of macrophytes can be structured by interactive ecological and geographic factors over time and space.
The particular research system or model is an important determinant of the outcomes of uniting ecology and genetics in macrophyte. For instance, the semi-enclosed Baltic Sea is an evolutionarily young marine ecosystem and has established its current environmental extremes only about 8500 years ago (Andrén et al., 2011). Most of the marine species present in the Baltic Sea today are survivors of a more diverse postglacial flora and fauna established during the Littorina period, 8000–4000 BP, during which the Baltic Sea was more saline than today (Johannesson and André, 2006; Andrén et al., 2011). For example, the endemic alga Fucus radicans L.Bergström and L.Kautsky stemmed from an isolated lineage of the wide ranging F. vesiculosus (Pereyra et al., 2009). Therefore, the pronounced salinity and temperature gradients from the South West toward the North East make the Baltic Sea an ideal natural ecosystem to study neutral and/or adaptive genetic variation in macroalgae. This prediction has already been confirmed for other marine organisms in the area (Johannesson and André, 2006; Guo et al., 2016). The North Atlantic has also been proposed as a model system for marine ecological genetics (Schmidt et al., 2008), but its Eastern and Western coast lines experienced long and complicated habitat changes during the Quaternary ice ages that probably exerted considerable impact on the diversity and distribution of marine species (Schmidt et al., 2008). This means the role of historical (e.g., range shifts driven by climate fluctuation) and contemporary influences (e.g., natural selection driven by environmental gradients) needs to be disentangled cautiously when adaptive genetic variation in the field is to be detected (Epps and Keyghobardi, 2015).
Genome sequencing techniques currently revolutionize our understanding of how marine species respond and adapt to environmental change. The complete genomes have been assembled of the macroalgae Ectocarpus siliculosus (Dillwyn) Lyngbye (Cock et al., 2010), C. crispus (Collén et al., 2013), Porphyra umbilicalis Kützing (Brawley et al., 2017), S. japonica (Ye et al., 2015) and Ulva mutabilis Föyn (De Clerck et al., 2018), Pyropia yezoensis (Ueda) M.S. Hwang et H.G. Choi (Wang et al., 2020), and the seagrass Z. marina (Olsen et al., 2016). This makes these species candidate models to link eco-physiology to genetics and beyond, for example, the identification of candidate male reproductive genes and mutant generation in E. siliculosus (Peters et al., 2008; Lipinska et al., 2015), the specific adaptive responses and mechanisms to environmental extremes in C. crispus and P. umbilicalis (Knoth and Wiencke, 1984; Collén et al., 2010), or the interactive genetic and environmental impact on thallus morphogenesis in U. mutabilis (Wichard et al., 2015). These genome resources can provide unprecedented opportunities to identify potential genes or genomic loci underpinning adaptive evolution and responses to environmental selection, using outlier analysis (e.g., genotype–environment association methods) (Forester et al., 2016). It is also promising to seek for a model species that can colonize new habitats rapidly and exhibit profound adaptive capability and resilience to environmental shifts. The candidate species like E. siliculosus, P. umbilicalis, P. yezoensis, and U. mutabilis having their genomes sequenced could provide natural experimental systems to obtain a better understanding of ecological adaptation and evolution of macrophytes over ecological timescales (Colautti and Lau, 2015).
Inferences based on a single species or environmental factor can have limitations when connecting population ecology to genetics. After all, two-way interactions between the eco-genetic features of species and their surrounding environments are quite common. Recent studies have shown that some heritable traits in a single species can affect the entire community and ecosystem (Kettenring et al., 2014). In this context, community and ecosystem genetics (Whitham et al., 2006) or eco-evolutionary dynamics (Rodriguez-Verdugo et al., 2017), which place community and ecosystem within an evolutionary framework, can provide promising insights into how ecological factors have driven the evolution of phenotypic traits and genetic variation, and how ecological processes (e.g., energy flow, nutrient cycling, and habitat selection) have altered interactions between species and biotic/abiotic environments (Kettenring et al., 2014; Rodriguez-Verdugo et al., 2017). Certain heritable variation is, by definition, passed (at least partially) from one generation to the next, providing a chance to uncover the mechanistic processes of heritability (Whitham et al., 2006). Hence, population genetics and quantitative genetics of macroalgae may help us to answer some basic questions concerning the construction and maintenance of coastal marine communities and ecosystems. Some of these questions are the following: (i) What are the ecological consequences (e.g., the differentiated phenotypic plasticity and stress-resistant capability) of genetic variation shifts within a macroalga (intra-specific effects), including the associated effects on retaining or enhancing community structure and ecosystem function? (ii) What are the ecological effects of the removal of alleles with the highest frequencies in a foundation macroalga in a structured community through consumption along altered trophic cascades? (iii) Would different bioregions or macroalgal populations with unique genetic variation possess different adaptive capacities? (iv) Do species interactions and species’ population stability truly rely on only one or few genotypes in a macroalgal species, and if so (v) through which mechanisms would genetic variation of macroalgae affect the ecological properties of coastal marine ecosystems? For example, epigenetic effects have been identified to contribute to phenotypic variation in genetically depauperate invasive populations (Richards et al., 2012). One could examine whether the worldwide invader Sargassum muticum (Yendo) Fensholt with almost no intra-specific genetic diversity (Le Cam et al., 2020) was driven by ecological adaptation or by epigenetic variation (no changes in DNA sequences but heritable structural modification such as DNA methylation).
Concluding Remarks
The unraveling of the underpinning mechanisms of ecological adaptation and evolution in macrophytes is in its infancy. To unite ecology and population genetics in macrophytes remains a formidable challenge. However, the invasive nature of some macrophytes offers an opportunity to do this. The circumstance that non-native populations appear as more resistant to multiple stresses irrespective of the invaded ecological realm suggests that selection either occurred very early or multiple times during the invasion. Many macroalgae are characterized by a haplodiplontic life cycle in which meiosis and fertilization are spatiotemporally separated by long-lived haploid gametophytes and diploid sporophytes. For example, the haploid gametophytes and diploid tetrasporophytes of the invasive A. vermiculophyllum (Synonym: Gracilaria vermiculophylla) in native habitats usually form mixed stands that typically grow attached to hard substrate and which are slightly dominated by diploid specimens (Krueger-Hadfield et al., 2016). In contrast, non-native populations in most cases occur in soft bottom habitats and consist nearly exclusively of tetrasporophytes. This indicates that allocating more resources into the fitness of diploids than into the fitness of haploids might increase the chance of translocated A. vermiculophyllum to establish successfully. Therefore, taking into account ploidy diversity (i.e., the ratio of gametophytes to sporophytes in natural populations) may be promising to address the role of intra-specific genetic diversity of macroalgae in interplaying with genetic and community-level diversity (Krueger-Hadfield et al., 2019).
Taken together, as more completely sequenced genomes will become available in the near future, more opportunities will be available to develop models and to identify genes/loci involved in the diversification and adaptive evolution of macrophytes under environmental shifts. The work may include full annotation of the genome sequence and relevant functional (genome to phenome) studies, development of systems for high-throughput genotyping, identification of the causative genetic variation underlying phenotypic variation associated with ecological variables, characterization of the gene regulatory networks, pathways and mechanisms for stress tolerance, ploidy diversity, and underlying phenotypic traits essential for adapting to environmental change. In particular, epigenetic variation and mechanisms that contribute to genetic variation in gene expression and/or phenotypic plasticity have been documented in studies of adaptation to habitat in model and non-model species, including ecological and genetic processes identified using traditional analysis approaches (Richards et al., 2017; Duarte et al., 2018). In addition, statistical advances, including seascape genomics and the fusion of Geographic Information Systems, provide promising perspectives in our conceptual understanding of how the interplay between genotypes, phenotypes, and environments in macrophytes modify the survival rate.
Author Contributions
Z-MH and ES conceived the work. Z-MH and K-LZ collected relevant literatures and made tables. Z-MH and FW wrote the manuscript. D-LD, SD, and ES revised and gave critical comments to the manuscript. All authors contributed to the article and approved the submitted version.
Funding
This study was supported by the National Natural Science Foundation of China (41761144057 and 31971395), Thailand Research Fund (RDG6130002), and the Sino-German Centre for Research Promotion (GZ1357).
Conflict of Interest
The authors declare that the research was conducted in the absence of any commercial or financial relationships that could be construed as a potential conflict of interest.
References
Aitken, S. N., and Whitlock, M. C. (2013). Assisted gene flow to facilitate local adaptation to climate change. Ann. Rev. Ecol. Evol. Syst. 44, 367–388. doi: 10.1146/annurev-ecolsys-110512-135747
Alberto, F., Raimondi, P. T., Reed, D. C., Coelho, N. C., Leblois, R., Whitmer, A., et al. (2010). Habitat continuity and geographic distance predict population genetic differentiation in giant kelp. Ecology 91, 49–56. doi: 10.1890/09-0050.1
Alberto, F., Raimondi, P. T., Reed, D. C., Watson, J. R., Siegel, D. A., Mitarai, S., et al. (2011). Isolation by oceanographic distance explains genetic structure for Macrocystis pyrifera in the Santa Barbara Channel. Mol. Ecol. 20, 2543–2554. doi: 10.1111/j.1365-294x.2011.05117.x
Allison, G. (2004). The influence of species diversity and stress intensity on community resistance and resilience. Ecol. Monogr. 74, 117–134. doi: 10.1890/02-0681
Andrén, T., Björck, S., Andrén, E., Conley, D., Zillén, L., and Anjar, J. (2011). “The development of the Baltic Sea basin during the last 130 ka,” in The Baltic Sea Basin, Central and Eastern European Development Studies, ed. J. Harff (Berlin: Springer), 75–97. doi: 10.1007/978-3-642-17220-5_4
Avia, K., Coelho, S. M., Montecinos, G. J., Cormier, A., Lerck, F., and Mauger, S. (2017). High-density genetic map and identification of QTLs for responses to temperature and salinity stress in the model brown alga Ectocarpus. Sci. Rep. 7:43241.
Balakirev, E. S., Krupnova, T. N., and Ayala, F. J. (2012a). DNA variation in the phenotypically-diverse brown alga Saccharina japonica. BMC Plant Biol. 12:108. doi: 10.1186/1471-2229-12-108
Balakirev, E. S., Krupnova, T. N., and Ayala, F. J. (2012b). Symbiotic associations in the phenotypically-diverse brown alga Saccharina japonica. PLoS One 7:e39587. doi: 10.1371/journal.pone.0039587
Bergström, L., and Kautsky, L. (2006). Local adaptation of Ceramium tenuicorne (Ceramiales, Rhodophyta) within the Baltic Sea. J. Phycol. 42, 36–42. doi: 10.1111/j.1529-8817.2006.00173.x
Bierne, N., Welch, J., Loire, E., Bonhomme, F., and David, P. (2011). The coupling hypothesis: why genome scans may fail to map local adaptation genes. Mol. Ecol. 20, 2044–2072. doi: 10.1111/j.1365-294x.2011.05080.x
Billard, E., Serrão, E. A., Pearson, G. A., Destombe, C., and Valero, M. (2010). Fucus vesiculosus and spiralis species complex: a nested model of local adaptation at the shore level. Mar. Ecol. Prog. Ser. 405, 163–174. doi: 10.3354/meps08517
Bolnick, D. I., and Nosil, P. (2007). Natural selection in populations subject to a migration load. Evolution 61, 2229–2243. doi: 10.1111/j.1558-5646.2007.00179.x
Bracken, M. E. S., Friberg, S. E., Gonzalez-Dorantes, C. A., and Williams, S. L. (2008). Functional consequences of realistic biodiversity changes in a marine ecosystem. Proc. Nat. Acad. Sci. U.S.A. 105, 924–928. doi: 10.1073/pnas.0704103105
Bradshaw, A. D. (1965). Evolutionary significance of phenotypic plasticity in plants. Adv. Genet. 13, 115–155. doi: 10.1016/s0065-2660(08)60048-6
Brawley, S. H., Blouin, N. A., Ficko-Blean, E., Wheeler, G. L., Lohr, M., Goodson, H. V., et al. (2017). Insights into the red algae and eukaryotic evolution from the genome of Porphyra umbilicalis (Bangiophyceae, Rhodophyta). Proc. Nat. Acad. Sci. U.S.A. 114, E6361–E6370.
Bruno, J. F., Boyer, K. E., Duffy, J. E., Lee, S. C., and Kertesz, J. S. (2005). Effects of macroalgal species identity and richness on primary production in benthic marine communities. Ecol. Lett. 8, 1165–1174. doi: 10.1111/j.1461-0248.2005.00823.x
Buonomo, R., Assis, J., Fernandes, F., Engelen, A. H., Airoldi, L., and Serrão, E. A. (2017). Habitat continuity and stepping-stone oceanographic distances explain population genetic connectivity of the brown alga Cystoseira amentacea. Mol. Ecol. 26, 766–780. doi: 10.1111/mec.13960
Canovas, F., Mota, C., Ferreira-Costa, J., Serrão, E. A., Coyer, J., Olsen, J., et al. (2011). Development and characterization of 35 single nucleotide polymorphism markers for the brown alga Fucus vesiculosus. Eur. J. Phycol. 46, 342–351. doi: 10.1080/09670262.2011.617473
Cerutti, H., Ma, X. R., Msanne, J., and Repas, T. (2011). RNA-mediated silencing in algae: biological roles and tools for analysis of gene function. Eukaryot. Cell 10, 1164–1172. doi: 10.1128/ec.05106-11
Chávez-Galarza, J., Henriques, D., Johnston, J. S., Azevedo, J. C., Patton, J. C., Munoz, I., et al. (2013). Signatures of selection in the Iberian honey bee (Apis mellifera iberiensis) revealed by a genome scan analysis of single nucleotide polymorphisms. Mol. Ecol. 22, 5890–5907. doi: 10.1111/mec.12537
Chu, S. H., Zhang, Q. S., Liu, S. K., Zhang, S. B., Tang, Y. Z., Lu, Z. C., et al. (2011). Trade-off between vegetative regeneration and sexual reproduction of Sargassum thunbergii. Hybrodiologia 678, 127–135. doi: 10.1007/s10750-011-0835-9
Cock, J. M., Coelho, S. M., Brownlee, C., and Taylor, A. R. (2010). The Ectocarpus genome sequence: insights into brown algal biology and the evolutionary diversity of the eukaryotes. New Phytol. 188, 1–4. doi: 10.1111/j.1469-8137.2010.03454.x
Colautti, R. I., and Lau, J. A. (2015). Contemporary evolution during invasion: evidence for differentiation, natural selection, and local adaptation. Mol. Ecol. 24, 1999–2017. doi: 10.1111/mec.13162
Coleman, M. A., Roughan, M., Macdonald, H. S., Connell, S. D., Gillanders, B. M., Kelaher, B. P., et al. (2011). Variation in the strength of continental boundary currents determines continent-wide connectivity in kelp. J. Ecol. 99, 1026–1032. doi: 10.1111/j.1365-2745.2011.01822.x
Collén, J., Guisle-Marsollier, I., Léger, J. J., and Boyen, C. (2010). Response of the transcriptome of the intertidal red seaweed Chondrus crispus to controlled and natural stresses. New Phytol. 176, 45–55. doi: 10.1111/j.1469-8137.2007.02152.x
Collén, J., Porcel, B., Carré, W., Ball, S. G., Chaparro, C., Tonon, T., et al. (2013). Genome structure and metabolic features in the red seaweed Chondrus crispus shed light on evolution of the Archaeplastida. Proc. Nat. Acad. Sci. U.S.A. 110, 5247–5252.
Crispo, E. (2008). Modifying effects of phenotypic plasticity on interactions among natural selection, adaptation and gene flow. J. Evol. Biol. 21, 1460–1469. doi: 10.1111/j.1420-9101.2008.01592.x
Crispo, E., and Chapman, L. J. (2008). Population genetic structure across dissolved oxygen regimes in an African cichlid fish. Mol. Ecol. 17, 2134–2148. doi: 10.1111/j.1365-294x.2008.03729.x
Crutsinger, G. M., Collins, M. D., Fordyce, J. A., and Sanders, N. J. (2008). Temporal dynamics in non-additive responses of arthropods to host-plant genotypic diversity. Oikos 117, 255–264. doi: 10.1111/j.2007.0030-1299.16276.x
Davies, T. W., Jenkins, S. R., Kingham, R., Kenworthy, J., Hawkins, S. J., and Hiddink, J. G. (2011). Dominance, biomass and extinction resistance determine the consequences of biodiversity loss for multiple coastal ecosystem processes. PLoS One 6:e28362. doi: 10.1371/journal.pone.028362
De Clerck, O., Kao, S. M., Bogaert, K. A., Blomme, J., Foflonker, F., Kwantes, M., et al. (2018). Insights into the evolution of multicellularity from the sea lettuce genome. Curr. Biol. 28, 1–13.
De Jode, A., David, R., Haguenauer, A., Cahill, A. E., Erga, Z., Guillemain, D., et al. (2019). From seascape ecology to population genomics and back. Spatial and ecological differentiation among cryptic species of the red algae Lithophyllum stictiforme/L. cabiochiae, main bioconstructors of coralligenous habitats. Mol. Phylogenet. Evol. 137, 104–113. doi: 10.1016/j.ympev.2019.04.005
Duarte, B., Martins, I., Rosa, R., Matos, A. R., Roleda, M. Y., Reusch, T. B. H., et al. (2018). Climate change impacts on seagrass meadows and macroalgal forests: an integrative perspective on acclimation and adaptation potential. Front. Mar. Sci. 5:190. doi: 10.3389/fmars.2018.00190
Durrant, H. M. S., Barrett, N. S., Edgar, G. J., Coleman, M. A., and Burridge, C. P. (2018). Seascape habitat patchiness and hydrodynamics explain genetic structuring of kelp populations. Mar. Ecol. Prog. Ser. 587, 81–92. doi: 10.3354/meps12447
Epps, C. W., and Keyghobardi, N. (2015). Landscape genetics in a changing world: disentangling historical and contemporary influences and inferring change. Mol. Ecol. 24, 6021–6040. doi: 10.1111/mec.13454
Flowers, J. M., Hazzouri, K. M., Pham, G. M., Rosas, U., Bahmani, T., and Khraiwesh, B. (2015). Whole-genome resequencing reveals extensive natural variation in the model green alga Chlamydomonas reinhardtii. Plant Cell 27, 2353–2369. doi: 10.1105/tpc.15.00492
Flukes, E. B., Wright, J. T., and Johnson, C. R. (2015). Phenotypic plasticity and biogeographic variation in physiology of habitat-forming seaweed: response to temperature and nitrate. J. Phycol. 51, 896–909. doi: 10.1111/jpy.12330
Forester, B. A., Jones, M. R., Joost, S., Landguth, E. L., and Lasky, J. R. (2016). Detecting spatial genetic signatures of local adaptation in heterogeneous landscapes. Mol. Ecol. 25, 104–120. doi: 10.1111/mec.13476
Fraser, C. I., Hay, C. H., Spencer, H. G., and Waters, J. M. (2009). Genetic and morphological analysis of the southern bull kelp Durvillaea antarctica (Phaeophyceae: Durvillaeales) in New Zealand reveal cryptic species. J. Phycol. 45, 436–443. doi: 10.1111/j.1529-8817.2009.00658.x
Fraser, C. I., McGaughran, A., Chuah, A., and Waters, J. M. (2016). The importance of replicating genomic analyses to verify phylogenetic signal for recently evolved lineages. Mol. Ecol. 25, 3683–3695. doi: 10.1111/mec.13708
Gabrielsen, T. M., Brochmann, C., and Rueness, J. (2002). The Baltic Sea as a model system for studying postglacial colonization and ecological differentiation, exemplified by the red alga Ceramium tenuicorne. Mol. Ecol. 11, 2083–2095. doi: 10.1046/j.1365-294x.2002.01601.x
Gerstenmaier, C. E., Krueger-Hadfield, S. A., and Sotka, E. E. (2016). Genotypic diversity in a non-native ecosystem engineer has variable impacts on productivity. Mar. Ecol. Prog. Ser. 556, 79–89. doi: 10.3354/meps11809
Gomez-Mestre, I., and Jovani, R. (2013). A heuristic model on the role of plasticity in adaptive evolution: plasticity increases adaptation, population viability, and genetic variation. Proc. R. Soc. B Biol. Sci. 280:20131869. doi: 10.1098/rspb.2013.1869
Guillemin, M. L., Valero, M., Tellier, F., Macayo, E. C., Destombe, C., and Faugeron, S. (2016). “Phylogeography of macroalgae in the South East Pacific: complex evolutionary processes along a latitudinal gradient,” in Seaweed Phylogeography: Adaptation and Evolution of Macroalgae Under Environmental Change, eds Z. M. Hu and C. I. Fraser (Berlin: Springer), 251–277. doi: 10.1007/978-94-017-7534-2_10
Guo, B. C., Li, Z. T., and Merliä, J. (2016). Population genomic evidence for adaptive differentiation in the Baltic Sea herring. Mol. Ecol. 25, 2833–2852. doi: 10.1111/mec.13657
Harley, C. D., Anderson, K. M., Demes, K. W., Jorve, J. P., Kordas, R. L., Coyle, T. A., et al. (2012). Effects of climate change on global seaweed communities. J. Phycol. 48, 1064–1078.
Hays, C. G. (2007). Adaptive phenotypic differentiation across the intertidal gradient in the alga Silvetia compressa. Ecology 88, 149–157.
Hu, Z. M., Duan, D. L., and Lopez-Bautista, J. (2016). “Seaweed phylogeography from 1984 to 2014: an overview,” in Seaweed Phylogeography: Adaptation and Evolution of Macroalgae Under Environmental Change, eds Z. M. Hu and C. I. Fraser (Cham: Springer), 3–22. doi: 10.1007/978-94-017-7534-2_1
Hu, Z. M., and Fraser, C. I. (2016). Seaweed Phylogeography: Adaptation and Evolution of Macroalgae Under Environmental Change. Cham: Springer.
Hughes, A. R., Inouye, B. D., Johnson, M. T. J., Underwood, N., and Vellend, M. (2008). Ecological consequences of genetic diversity. Ecol. Lett. 11, 609–623. doi: 10.1111/j.1461-0248.2008.01179.x
Hughes, A. R., and Stachowicz, J. J. (2004). Genetic diversity enhances the resistance of a seagrass ecosystem to disturbance. Proc. Nat. Acad. Sci. U.S.A. 101, 8998–9002. doi: 10.1073/pnas.0402642101
Hurd, C. L., Harrison, P. J., Bischof, K., and Lobban, C. S. (2014). Seaweed Ecology and Physiology, 2nd Edn, Cambridge: Cambridge university press.
Johannesson, K., and André, C. (2006). Life on the margin: genetic isolation and diversity loss in a peripheral marine ecosystem, the Baltic Sea. Mol. Ecol. 15, 2013–2029. doi: 10.1111/j.1365-294x.2006.02919.x
Johansson, M. L., Alberto, F., Reed, D. C., Raimondi, P. T., Coelho, N. C., Yong, M., et al. (2015). Seascape drivers of Macrocystis pyrifera population genetic structure in the northeast Pacific. Mol. Ecol. 24, 4866–4885. doi: 10.1111/mec.13371
Jormalainen, V., Danelli, M., Gagnon, K., Hillebrand, H., Rothäusler, E., and Salminen, J. P. (2017). Genetic variation of a foundation rockweed species affects associated communities. Ecology 98, 2940–2951. doi: 10.1002/ecy.2002
Jürgens, K., and Matz, C. (2002). Predation as a shaping force for the phenotypic and genotypic composition of planktonic bacteria. Antonie Leeuwenh. Int. J. Gen. Mol. Microbiol. 81, 413–434.
Kettenring, K. M., Mercer, K. L., Adams, C. R., and Hines, J. (2014). Application of genetic diversity-ecosystem function research to ecological restoration. J. Appl. Ecol. 51, 339–348. doi: 10.1111/1365-2664.12202
Knoth, A., and Wiencke, C. (1984). Dynamic changes of protoplasmic volume and of fine structure during osmotic adaptation in the intertidal red alga Porphyra umbilicalis. Plant Cell Environ. 7, 113–119. doi: 10.1111/j.1365-3040.1984.tb01564.x
Kobayashi, H., Haino, Y., Iwasaki, T., Tezuka, A., Nagano, A., and Shimada, S. (2018). ddRAD-seq based phylogeographic study of Sargassum thunbergii (Phaeophyceae, Heterokonta) around Japanese coast. Mar. Environ. Res. 140, 104–113. doi: 10.1016/j.marenvres.2018.05.021
Korpelainen, H. (2016). “Comparative population genetgics of red alga occupying different salinity conditions,” in Seaweed Phylogeography: Adaptation and Evolution of Macroalgae Under Environmental Change, eds Z. M. Hu and C. I. Fraser (Cham: Springer), 331–344. doi: 10.1007/978-94-017-7534-2_13
Kostamo, K., Korpelainen, H., and Olsson, S. (2012). Comparative study on the population genetics of the red algae Furcellaria lumbricalis occupying different salinity conditions. Mar. Biol. 159, 561–571. doi: 10.1007/s00227-011-1835-z
Kostamo, K., and Mäkinen, A. (2006). Observations on the mode and seasonality of reproduction in Furcellaria lumbricalis (Gigatinales, Rhodophyta) populations in the northern Baltic Sea. Bot. Mar. 49, 304–309.
Krueger-Hadfield, S. A., Blakeslee, A. M. H., and Fowler, A. E. (2019). Incorporating ploidy diversity into ecological and community genetics. J. Phycol. 55, 1198–1207. doi: 10.1111/jpy.12906
Krueger-Hadfield, S. A., Kollars, N. M., Byers, J. E., Greig, T. W., Hammann, M., Murray, D. C., et al. (2016). Invasion of novel habitats uncouples haplo-diplontic life cycles. Mol. Ecol. 25, 3801–3816. doi: 10.1111/mec.13718
Krueger-Hadfield, S. A., Roze, D., Mauger, S., and Valero, M. (2013). Intergametophytic selfing and microgeographic genetic structure shape populations of the intertidal red seaweed Chondrus crispus. Mol. Ecol. 22, 3242–3260. doi: 10.1111/mec.12191
Le Cam, S., Daguin-Thiébaut, C., Bouchemousse, S., Engelen, A. H., Mieszkowska, N., and Viard, F. (2020). A genome-wide investigation of the worldwide invader Sargassum muticum shows high success albeit (almost) no genetic diversity. Evol. Appl. 13, 500–514. doi: 10.1111/eva.12837
Leskinen, E., Alström-Rapaport, C., and Pamilo, P. (2004). Phylogeographical structure, distribution and genetic variation of the green algae Ulva intestinalis and U. compressa (Chlorophyta) in the Baltic Sea area. Mol. Ecol 13, 2257–2265. doi: 10.1111/j.1365-294x.2004.02219.x
Lipinska, A., Cormier, A., Luthringer, R., Peters, A. F., Corre, E., Gachon, C. M. M., et al. (2015). Sexual dimorphism and the evolution of sex-biased gene expression in the brown alga Ectocarpus. Mol. Biol. Evol. 32, 1581–1597. doi: 10.1093/molbev/msv049
Macaya, E. C., López, B., Tala, F., Tellier, F., and Thiel, M. (2016). “Float and raft: role of buoyant macroalgae in the phylogeography and genetic structure of non-buoyant associated flora,” in Seaweed Phylogeography: Adaptation and Evolution of Macroalgae Under Environmental Change, eds Z. M. Hu and C. I. Fraser (Cham: Springer), 97–130. doi: 10.1007/978-94-017-7534-2_4
Monro, K., Poore, A. G. B., and Brooks, R. (2007). Multivariate selection shape environment-dependent variation in the clonal morphology of a red seaweed. Evol. Ecol. 21, 765–782. doi: 10.1007/s10682-006-9150-8
Namroud, M. C., Beaulieu, J., Juge, N., Laroche, J., and Bousquet, J. (2008). Scanning the genome for gene single nucleotide polymorphisms involved in adaptive population differentiation in white spruce. Mol. Ecol. 17, 3599–3613. doi: 10.1111/j.1365-294x.2008.03840.x
Nishimura, N. J., and Mandoli, D. F. (1992). Population analysis of reproductive cell structure of Acetabularia acetabulum (Chlorophyta). Phycologia 31, 351–358. doi: 10.2216/i0031-8884-31-3-4-351.1
Olsen, J. L., Rouze, P., Verhelst, B., Lin, Y. C., Bayer, T., Collen, J., et al. (2016). The genome of the seagrass Zostera marina reveals angiosperm adaptation to the sea. Nature 530, 331–335.
Olsson, S., and Korpelainen, H. (2013). Single nucleotide polymorphisms found in the red alga Furcellaria lumbricalis (Gigartinales): new markers for population and conservation genetic analyses. Aquat. Conserv. 23, 460–467. doi: 10.1002/aqc.2325
Padilla, D. K., and Savedo, M. M. (2013). A systematic review of phenotypic plasticity in marine invertebrate and plant systems. Adv. Mar. Biol. 65, 67–94. doi: 10.1016/b978-0-12-410498-3.00002-1
Palumbi, S. R. (1994). Genetic divergence, reproductive isolation, and marine speciation. Ann. Rev. Ecol. Syst. 25, 547–572. doi: 10.1146/annurev.es.25.110194.002555
Pearson, G. A., Lago-Leston, A., and Mota, C. (2009). Frayed at the edges: selective pressure and adaptive response to abiotic stressors are mismatched in low diversity edge populations. J. Ecol. 97, 450–462. doi: 10.1111/j.1365-2745.2009.01481.x
Pereyra, R. T., Bergström, L., Kastsky, L., and Johannesson, K. (2009). Rapid speciation in a newly opened postglacial marine environment, the Baltic Sea. BMC Evol. Biol. 9:70. doi: 10.1186/1471-2229-12-070
Pereyra, R. T., Huenchunir, C., Johansson, D., Forslund, H., Kautsky, L., Jonsson, P. R., et al. (2013). Parallell speciation or long-distance dispersal? Lessons from seaweeds (Fucus) in the Baltic Sea. J. Evol. Ecol. 26, 1727–1737. doi: 10.1111/jeb.12170
Peters, A. F., Scornet, D., Ratin, M., Charrier, B., Monnier, A., Merrien, Y., et al. (2008). Life-cycle-generation-specific development processes are modified in the immediate upright mutant of the brown alga Ectocarpus siliculosus. Development 135, 1503–1512. doi: 10.1242/dev.016303
Pfennig, D. W., Wund, M. A., Snell-Rood, E. C., Cruickshank, T., Schlichting, C. D., and Moczek, P. (2010). Phenotypic plasticity’s impacts on diversification and speciation. Trends Ecol. Evol. 25, 459–467.
Pimentel, D. (1968). Population regulation and genetic feedback. Science 159, 1432–1437. doi: 10.1126/science.159.3822.1432
Provan, J., Glendinning, K., Kelly, R., and Maggs, C. A. (2013). Levels and patterns of population genetic diversity in the red seaweed Chondrus crispus (Florideophyceae): a direct comparison of single nucleotide polymorphisms and microsatellites. Biol. J. Linn. Soc. 108, 251–262. doi: 10.1111/j.1095-8312.2012.02010.x
Reed, D. C., Ebeling, A. W., Anderson, T. W., and Anghera, M. (1996). Different reproductive responses to fluctuating resources in two macroalgae with different reproductive strategies. Ecology 77, 300–316. doi: 10.2307/2265679
Rellstab, C., Gugerli, F., Eckert, A. J., Hancock, A. M., and Holderegger, R. (2015). A practical guide to environmental association analysis in landscape genomics. Mol. Ecol. 24, 4348–4370. doi: 10.1111/mec.13322
Reusch, T. B. H., Ehlers, A., Haemmerli, A., and Worm, B. (2005). Ecosystem recovery after climatic extremes enhanced by genotypic diversity. Proc. Nat. Acad. Sci. U.S.A. 102, 2826–2831. doi: 10.1073/pnas.0500008102
Richards, C. L., Alonso, C., Becker, C., Bossdorf, O., Bucher, E., Colomé-Tatché, M., et al. (2017). Ecological plant epigenetics: evidence from model and non-model species, and the way forward. Ecol. Lett. 20, 1576–1590. doi: 10.1111/ele.12858
Richards, C. L., Schrey, A. W., and Pigliucci, M. (2012). Invasion of diverse habitats by few Japanese knotweed genotype is correlated with epigenetic differentiation. Ecol. Lett. 15, 1016–1025. doi: 10.1111/j.1461-0248.2012.01824.x
Robuchon, M., Le Gall, L., Mauger, S., and Valero, M. (2014). Contrasting genetic diversity patterns in two sister kelp species co-distributed along the coast of Brittany, France. Mol. Ecol. 23, 2669–2685. doi: 10.1111/mec.12774
Rodriguez-Verdugo, A., Buckley, J., and Stapley, J. (2017). The genomic basis of eco-evolutionary dynamics. Mol. Ecol. 26, 1456–1464. doi: 10.1111/mec.14045
Roth-Schulze, A. J., Pintado, J., Zozaya-Valdés, E., Cremades, J., Ruiz, P., Kjelleberg, S., et al. (2018). Functional biogeography and host specificity of bacterial communities associated with the marine green alga Ulva spp. Mol. Ecol. 27, 1952–1965. doi: 10.1111/mec.14529
Saada, G., Nicastro, K. R., Jacinto, R., and McQuaid, C. D. (2016). Taking the heat: distinct vulnerability to thermal stress of central and threatened peripheral lineages of a marine macroalga. Diver. Distr. 22, 1060–1068. doi: 10.1111/ddi.12474
Sandoval-Castillo, J., Robinson, N. A., Hart, A. M., Strain, L. W. S., and Beheregaray, L. B. (2018). Seascape genomics reveals adaptive divergence in a connected and commercially important mollusk, the green lip abalone (Haliotis laevigata), along a longitudinal environmental gradient. Mol. Ecol. 27, 1603–1620. doi: 10.1111/mec.14526
Savolainen, O., Lascoux, M., and Merilä, J. (2013). Ecological genomics of local adaptation. Nat. Rev. Genet. 14, 807–820. doi: 10.1038/nrg3522
Schmidt, P. S., Serrão, E. A., Pearson, G. A., Riginos, C., Rawson, P. D., Hilbish, T. J., et al. (2008). Ecological genetics in the North Atlantic: environmental gradients and adaptation at specific loci. Ecology 89, S91–S107.
Schneider, R. F., and Meyer, A. (2017). How plasticity, genetic assimilation and cryptic genetic variation may contribute to adaptive radiations. Mol. Ecol. 26, 330–350. doi: 10.1111/mec.13880
Scrosati, R., and Servière-Zaragoza, E. (2000). Ramet dynamics for the clonal seaweed Pterocladiella capillacea (Rhodophyta): a comparison with Chondrus crispus and with Mazzaella cornucopiae (Gigartinales). J. Phycol. 36, 1061–1068. doi: 10.1046/j.1529-8817.2000.00041.x
Selkoe, K. A., D’Aloia, C. C., Crandall, E. D., Iacchei, M., Liggins, L., Britz, J. B., et al. (2016). A decade of seascape genetics: contributions to basic and applied marine connectivity. Mar. Ecol. Prog. Ser. 554, 1–19. doi: 10.3354/meps11792
Serrão, E. A., Brawley, S. H., Hedman, J., Kautsky, L., and Samuelsson, G. (1999). Reproductive success in Fucus vesiculosus in the Baltic Sea. J. Phycol. 35, 254–269. doi: 10.1046/j.1529-8817.1999.3520254.x
Serrão, E. A., Kautsky, L., and Brawley, S. H. (1996). Distributional success of the marine seaweed Fucus vesiculosus L. in the brackish Baltic Sea correlates with osmotic capabilities of gametes. Oecologia 107, 1–12. doi: 10.1007/bf00582229
Shimada, S., Ichihara, K., Masakiyo, Y., Kawaguchi, R., and Kikuchi, N. (2016). “Phylogeography of macroalgal species distributed in brackish water: Ulva prolifera (Ulvophyceae) and Pyropia tenera (Bangiophyceae),” in Seaweed Phylogeography: Adaptation and Evolution of Macroalgae Under Environmental Change, eds Z. M. Hu and C. I. Fraser (Cham: Springer), 345–360. doi: 10.1007/978-94-017-7534-2_14
Sosa, P., and Lindstrom, S. (1999). Isozymes in macroalgae (macroalgae): genetic differentiation, genetic variability and applications in systematics. Eur. J. Phycol. 34, 427–442. doi: 10.1080/09541449910001718791
Steinhagen, S., Karez, R., and Weinberger, F. (2019a). Molecular analysis of Ulva compressa (Chlorophyta, Ulvales) reveals its morphological plasticity, distribution and potential invasiveness on German North Sea and Baltic Sea coasts. Eur. J. Phycol. 54, 102–114. doi: 10.1080/09670262.2018.1513167
Steinhagen, S., Karez, R., and Weinberger, F. (2019b). Surveying macroalgae from the Ulvales and Fucales in the world’s most frequently used artificial waterway, the Kiel Canal. Bot. Mar. 62, 51–61. doi: 10.1515/bot-2018-0020
Tatarenkov, A., Bergström, L., Jönsson, R., Serrão, E. A., Kautsky, L., and Johannesson, K. (2005). Intriguing asexual life in the brown seaweed Fucus vesiculosus. Mol. Ecol. 14, 647–651. doi: 10.1111/j.1365-294x.2005.02425.x
Thébault, E., and Loreau, M. (2005). Trophic interactions and the relationships between species diversity and ecosystem stability. Am. Nat. 166, E95–E114.
Tuomi, J., and Vuorisalo, T. (1989). Hierarchical selection in modular organisms. Trends Ecol. Evol. 4, 209–213. doi: 10.1016/0169-5347(89)90075-x
Usandizaga, Z., Camus, C., Kappes, J. L., Guillemin, M. L., and Buschmann, A. H. (2018). Nutrient, but not genetic diversity, affect Gracilaria chilensis (Rhodophyta) farming productivity and physiological responses. J. Phycol. 54, 860–869. doi: 10.1111/jpy.12785
Valero, M., Engel, C., Billot, C., Kloareg, B., and Destombe, C. (2001). Concepts and issues of population genetics in macroalgae. Cahiers De Biol. Mar. 42, 53–62.
van Tienderen, P. H. (1991). Evolution of generalists and specialists in spatially heterogeneous environments. Evolution 45, 1317–1331. doi: 10.1111/j.1558-5646.1991.tb02638.x
Wang, D. M., Yu, X. Z., Xu, K. P., Bi, G. Q., Cao, M., Zelzion, E., et al. (2020). Pyropia yezoensis genome reveals diverse mechanisms of carbon acquisition in the intertidal environment. Nat. Commun. 11:4028.
Wennerström, L., Jansson, E., and Laikre, L. (2017). Baltic Sea genetic diversity: current knowledge relating to conservation management. Aquat. Conserv. Mar. Fresh. Ecosyst. 27, 1069–1090. doi: 10.1002/aqc.2771
Wernberg, T., Coleman, M. A., Bennett, S., Thomsen, M. S., Tuya, F., and Kelaher, B. P. (2018). Genetic diversity and kelp forest vulnerability to climate stress. Sci. Rep. 8:1851.
Whitham, T. G., Bailey, J. K., Schweitzer, J. A., Shuster, S. M., Bangert, R. K., LeRoy, C. J., et al. (2006). A framework for community and ecosystem genetics: from genes to ecosystems. Nat. Rev. Genet. 7, 510–523. doi: 10.1038/nrg1877
Wichard, T., Charrier, B., Mineur, F., Bothwell, J. H., De Clerck, O., and Coates, J. C. (2015). The green seaweed Ulva: a model system to study morphogenesis. Front. Plant Sci. 6:72. doi: 10.3389/fmars.2018.00072
Ye, N. H., Zhang, X. W., Miao, M., Fan, X., Zheng, Y., Xu, D., et al. (2015). Saccharina genomes provide novel insight into kelp biology. Nat. Commun. 6:6986.
Yesson, C., Jackson, A., Russell, S., Williamson, C. J., and Brodie, J. (2018). SNPs reveal geographical population structure of Corallina officinalis (Corallinaceae, Rhodophyta). Eur. J. Phycol. 53, 180–188. doi: 10.1080/09670262.2017.1402373
Yoshida, T., Jones, L. E., Ellner, S. P., Fussman, G. F., and Hairston, N. G. Jr. (2003). Rapid evolution drives ecological dynamics in a predator-prey system. Nature 424, 303–306. doi: 10.1038/nature01767
Zardi, G. I., Nicastro, K. R., Costa, J. F., Serrão, E. A., and Pearson, G. A. (2013). Broad scale agreement between intertidal habitats and adaptive traits on a basis of contrasting population genetic structure. Estuar. Coast. Shelf Sci. 131, 140–148. doi: 10.1016/j.ecss.2013.08.016
Keywords: adaptive genetic variation, divergent selection, ecological processes, phenotypic plasticity, ploidy diversity, genetic consequences
Citation: Hu Z-M, Zhong K-L, Weinberger F, Duan D-L, Draisma SGA and Serrão EA (2020) Linking Ecology to Genetics to Better Understand Adaptation and Evolution: A Review in Marine Macrophytes. Front. Mar. Sci. 7:545102. doi: 10.3389/fmars.2020.545102
Received: 23 April 2020; Accepted: 21 October 2020;
Published: 13 November 2020.
Edited by:
Christos Dimitrios Arvanitidis, Hellenic Centre for Marine Research (HCMR), GreeceReviewed by:
Anne Chenuil, Centre National de la Recherche Scientifique (CNRS), FranceFrancisco Arenas, University of Porto, Portugal
Copyright © 2020 Hu, Zhong, Weinberger, Duan, Draisma and Serrão. This is an open-access article distributed under the terms of the Creative Commons Attribution License (CC BY). The use, distribution or reproduction in other forums is permitted, provided the original author(s) and the copyright owner(s) are credited and that the original publication in this journal is cited, in accordance with accepted academic practice. No use, distribution or reproduction is permitted which does not comply with these terms.
*Correspondence: Zi-Min Hu, aHV6aW1pbjk3MTJAMTYzLmNvbQ==