- 1UCIBIO – Applied Biomolecular Sciences Unit, Chemistry Department, Blue Biotechnology & Biomedicine Lab, School of Science and Technology, NOVA University Lisbon, Caparica, Portugal
- 2UCIBIO – Applied Biomolecular Sciences Unit, Chemistry Department, BIOENG Lab, School of Science and Technology, NOVA University Lisbon, Caparica, Portugal
- 3LAQV– REQUIMTE, Chemistry Department, School of Science and Technology, NOVA University Lisbon, Caparica, Portugal
- 4Marine Biology Station Piran, National Institute of Biology, Piran, Slovenia
- 5I3N – CENIMAT, Materials Science Department, School of Science and Technology, NOVA University Lisbon, Caparica, Portugal
- 6Physics Department, Instituto Superior de Engenharia de Lisboa, Instituto Politécnico de Lisboa, Lisbon, Portugal
Plastics are very useful materials and present numerous advantages in the daily life of individuals and society. However, plastics are accumulating in the environment and due to their low biodegradability rate, this problem will persist for centuries. Until recently, oceans were treated as places to dispose of litter, thus the persistent substances are causing serious pollution issues. Plastic and microplastic waste has a negative environmental, social, and economic impact, e.g., causing injury/death to marine organisms and entering the food chain, which leads to health problems. The development of solutions and methods to mitigate marine (micro)plastic pollution is in high demand. There is a knowledge gap in this field, reason why research on this thematic is increasing. Recent studies reported the biodegradation of some types of polymers using different bacteria, biofilm forming bacteria, bacterial consortia, and fungi. Biodegradation is influenced by several factors, from the type of microorganism to the type of polymers, their physicochemical properties, and the environment conditions (e.g., temperature, pH, UV radiation). Currently, green environmentally friendly alternatives to plastic made from renewable feedstocks are starting to enter the market. This review covers the period from 1964 to April 2020 and comprehensively gathers investigation on marine plastic and microplastic pollution, negative consequences of plastic use, and bioplastic production. It lists the most useful methods for plastic degradation and recycling valorization, including degradation mediated by microorganisms (biodegradation) and the methods used to detect and analyze the biodegradation.
Introduction
One of the main consequences of the models and patterns of production and consumption adopted by today’s society is the generation of waste, a major environmental problem that is increasingly demanding attention to the search for solutions, especially with regard to marine pollution (Savelli et al., 2017; Walker, 2018; Wysocki and Billon, 2019). Marine pollution caused by solid waste is a growing problem on a global scale and generates intergenerational impacts (Li et al., 2019; Lestari and Trihadiningrum, 2019). Despite decades of efforts to prevent and reduce marine litter in many countries, there is evidence that the problem is persistent and continues to increase, as human populations and single-use consumption patterns continue to rise. Billions of tons of garbage are thrown (intentionally or unintentionally) into the oceans each year, and approximately 80% of these wastes come from land-based sources (Oelofse et al., 2004; Andrady, 2011). Due to the low rate of degradation, plastics remain in the marine ecosystem for long periods (Hopewell et al., 2009). These residues tend to accumulate in certain locations, due to oceans waves, currents, and winds, and can be found even in remote areas (GESAMP, 2015; Sebille et al., 2020). In coastal areas, where human activities are concentrated, the problem of increasing plastic pollution becomes even more apparent (Barnes et al., 2009; Ho and Not, 2019).
By definition, marine litter is considered to be any type of solid waste produced by humans, generated on land or at sea that has been introduced into the marine environment, including the transport of these materials through rivers, drains, water systems, sewage, or wind, excluding organic materials, such as food and vegetable scraps (Cheshire et al., 2009). One of the main current concerns of the academic community, non-governmental organizations, governments, and the general population is related to the abundance and fate of solid waste, mainly plastics, in the marine and coastal environments (Gregory, 2009).
Plastics are produced and used on a large scale for a broad range of applications, due to their notable thermo-elastic and mechanical properties, high resistance, stability and durability, chemical inertness, malleability, low water permeability, light weight, and low cost (Thompson et al., 2009; Huerta et al., 2018; Raddadi and Fava, 2019). The usage of plastic materials allowed the development of great advances and benefits to society. However, it also caused several environmental issues associated with the high incidence of plastics as waste (Hopewell et al., 2009; Ügdüler et al., 2020).
Environmental scientists, such as ecologists and biologists, have pointed out for decades that plastic materials discarded in the sea represent one of the greatest threats to the environment. About 90% of the solid waste found in the oceans is plastic and from all the manufactured plastic around 10% enter the hydrosphere (Williams, 1999; Sheavly and Register, 2007; Scalenghe, 2018). As there is a lack of inexpensive alternatives to plastics, it is difficult for individuals and industries to ban plastic in their everyday life. Nevertheless, as plastics have low environmental biodegradability rate, there are growing concerns about massive accumulation in the environment that can persist for centuries and we are witnessing a high demand for solutions to mitigate this issue (Raddadi and Fava, 2019).
Plastics are polymers, that is, they are organic macromolecules formed by hundreds or thousands of segments linked together, forming a chain. In industrial environments, the term “plastics” is defined as a class of synthetic organic polymers that pass through the plastic state, a moldable state between liquid and solid, in conditions above room temperature. Today, plastic is an integral part of our life, being present in an immeasurable number of objects, such as packaging, garbage bags, domestic utilities, soft drink bottles, and a multitude of objects. Plastics also play an important role in the general improvement of human health, as disposable medical equipment is made with this material (Tokiwa et al., 2009; Correia et al., 2020). Plastic use and production worldwide have extensively increased in recent years in numerous industrial sectors and activities, due to its versatility and physicochemical properties (Figure 1). Plastic has replaced, with advantages, wood, metals and metal alloys, glass, paper, vegetable, and animals’ fibers, as many of them are already scarce in nature or have much higher production costs.
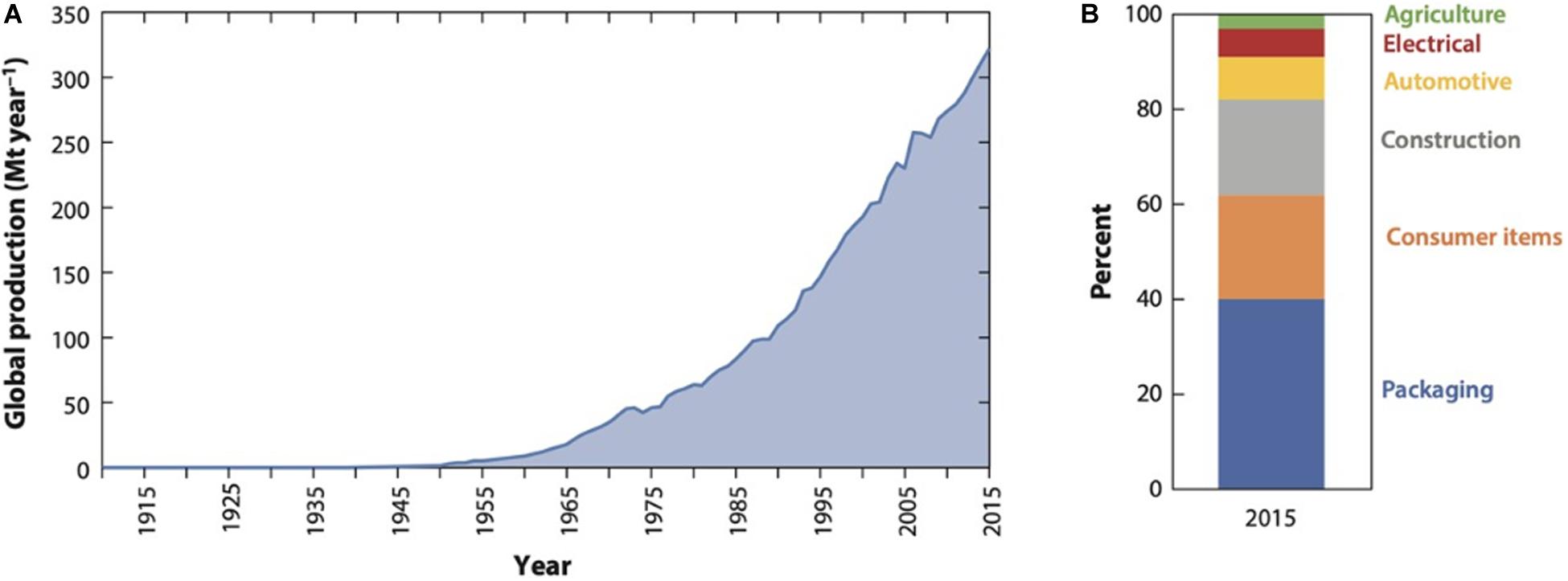
Figure 1. Evolution in total plastic production worldwide. (A) World production in million metric tons per year (Mt⋅year–1). (B) Plastic utilization distribution of plastic in Worm et al. (2017). Reproduced with permission from the Annual Review of Environment and Resources, Volume 42 © 2017 by Annual Reviews, http://www.annualreviews.org. Copyright 2017, Annual Review of Environment and Resources.
According to the statistics, the quantity of global plastic waste between 1950 and 2015 was estimated to be 6.3 billion tons (Zhang et al., 2019); in 2017 and 2018, reached 350 and 359 million tons worldwide, respectively (Raddadi and Fava, 2019). The lack of proper waste management has led to the accumulation of over 250 thousand tons of plastic pieces floating in the ocean (Eriksen et al., 2014). Only 9% of this waste has been recycled, 12% is incinerated, and 79% still ends up in landfills or in the environment (Figure 2; Geyer et al., 2017). Plastics can be recycled mechanically, chemically, and by composting/biodegrading. However, their overall low recycling rate is mostly due to the costs of collecting, sorting, reprocessing, and the low market value of recycled plastics (OECD, 2018; Scalenghe, 2018).
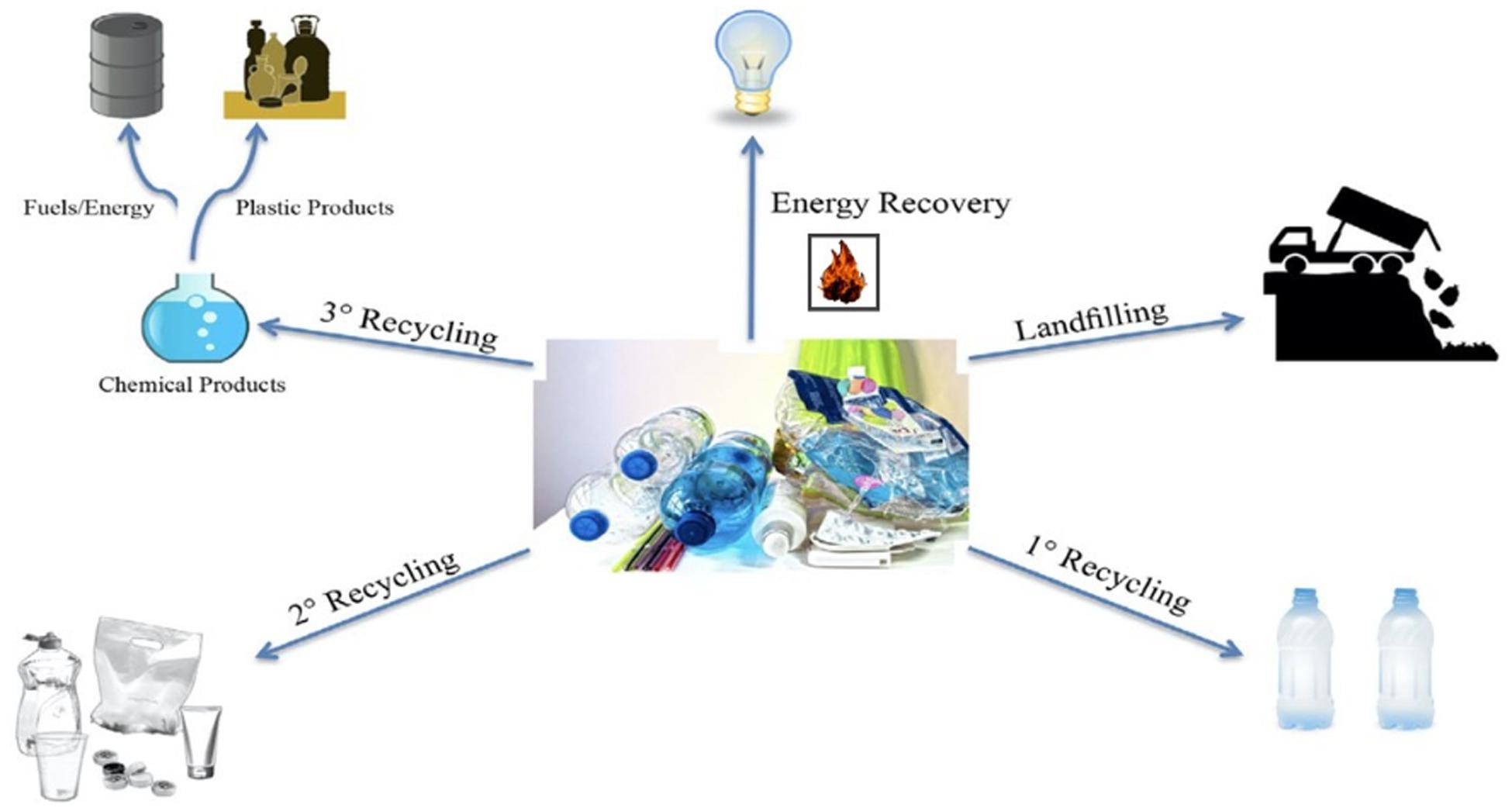
Figure 2. Usual destination for current plastic waste. Recycling classifications: 1° Recycling—primary—turning uncontaminated discarded plastics into the same new product, without the loss of properties. 2° Recycling—secondary—mechanical recycling, where the polymer is physically reprocessed and generally used for a different purpose than its original use. 3° Recycling—tertiary—chemical recycling, where chemical processes are used to break down the polymer into added-value products, and energy recovery—polymers are incinerated and energy is recovered in the form of heat (Thiounn and Smith, 2020). Reproduced with permission. Copyright 2020, John Wiley and Sons.
Marine plastic pollution has a negative ecological, social, and economic impact. Plastic pieces floating in the ocean can be ingested by marine organisms, such as fish, turtles, birds, and mammals, creating digesting and malnutrition problems. Besides, these organisms can be entangled in synthetic ropes and lines, drift nets, and plastic debris, causing lethal wounds and respiratory impairment. In addition, plastics contain chemical contaminants that leach to the surrounding environment, causing toxicity issues. Moreover, plastics have been detected in all levels of the food chain (Figure 3), also leading to human health problems. In addition, the mismanaged plastic entering the environment can provide a breeding ground for mosquitoes or block water drainage, which can cause floods and the spread of diseases, interfering with the regular ecosystem’s functions (Derraik, 2002; GESAMP, 2015; Revel et al., 2018; UNEP, 2018).
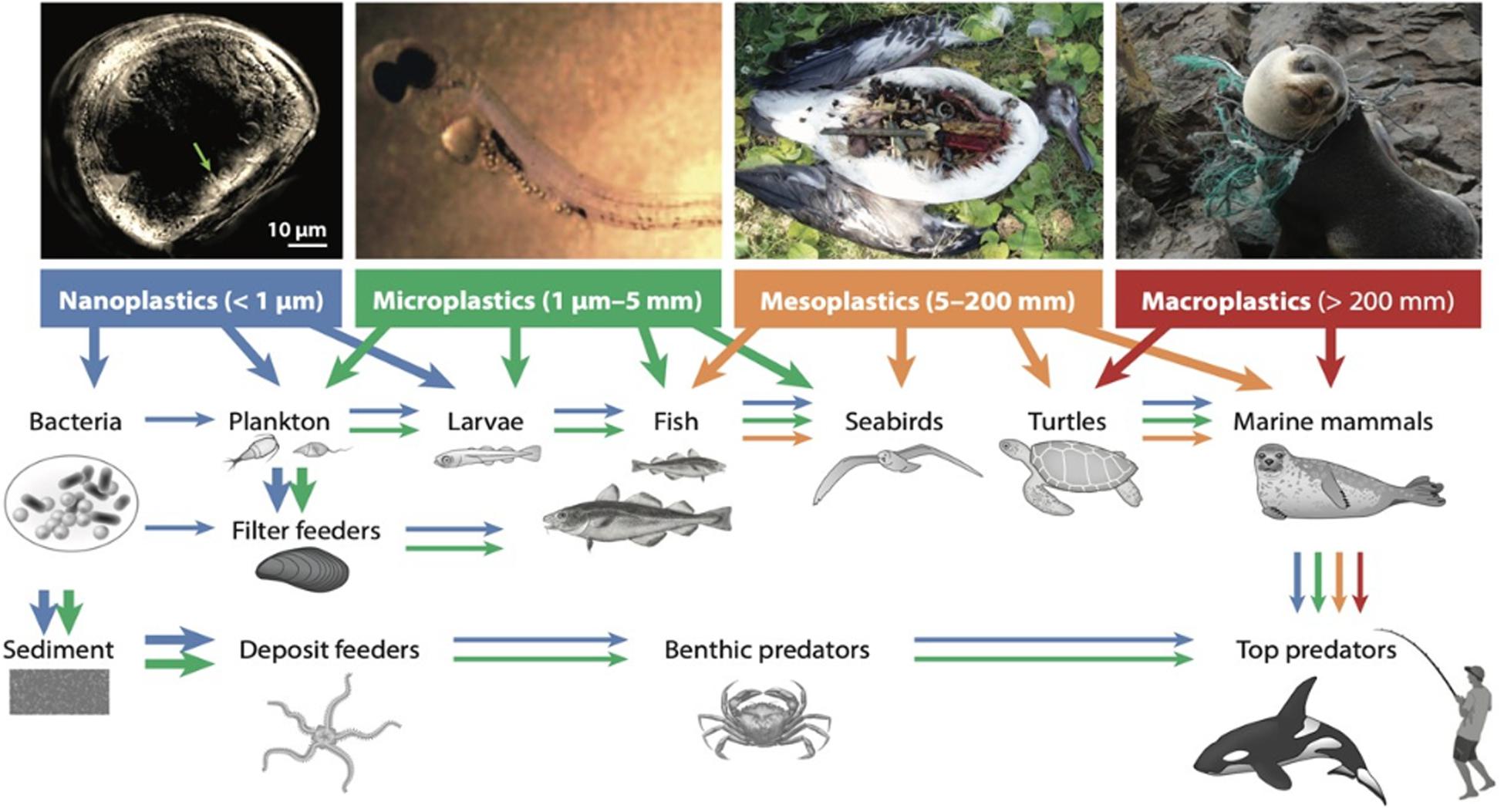
Figure 3. Uptake and trophic transfer of plastic pollution in marine food chain. Plastic debris of different size categories have been shown to affect species directly by ingestion or entanglement (thick arrows) or indirectly via uptake of food sources (thin arrows). Fauna of different sizes and trophic positions will be exposed to particles of s sizes (blue to red) with some degree of bioaccumulation expected, for both particles themselves and associated chemical pollutants. Photographs depict (left to right) nanoplastic particles taken up by oyster larvae, microplastic beads ingested by European perch, dead albatross chick with micro- and mesoplastic debris in the stomach, and sea lion entangled in macroplastic fishing gear (Worm et al., 2017). Reproduced with permission from the Annual Review of Environment and Resources, Volume 42 © 2017 by Annual Reviews, http://www.annualreviews.org. Copyright 2017, Annual Review of Environment and Resources.
It is of utmost importance to discover and develop new methods and solutions for the problem of persistent pollution by (micro)plastics. A new marine biotechnology research field is starting, focusing on marine debris pollution such as plastic degradation by microorganisms, which will be addressed herein. In detail, this review covers aspects of the degradation, biodegradation, and recycling valorization of plastics and microplastics, types of most common existing polymers and their uses, bioplastic production, and degradation mediated by microorganisms, including the methods used to detect and analyze the biodegradation under laboratory conditions, based on selected literature from 1964 to April 2020, in a total of 266 papers. Moreover, several microorganisms that are capable of degrading plastics/microplastics were enumerated, as well as their efficacy to biodegrade different types of polymers. Our objective was also to summarize the most useful methods for plastic degradation and valorization. This review compiles the data spread across disciplines and will fill the existing knowledge gaps in this area, helping researchers to set experimental methodologies in their search for solutions to mitigate the problem caused by plastic pollution.
Plastic Types
Most currently used polymers, such as plastics, rubbers, and fibers, are synthesized from chemical monomers derived from petroleum, in the presence of a catalyst and an energy source, typically heat. Monomers are processed in the form of a fluid or a solution or emulsion, through two processes: condensation (step growth) and addition/polymerization (chain growth) (Jefferson, 2019). The conventional plastics that dominate the world market are polypropylene (PP) 21.1%, polyethylene (PE): low-density polyethylene (LDPE) 19.9%, high-density polyethylene (HDPE) 16.1%, polyvinyl chloride (PVC) 11.8%, polyethylene terephthalate (PET) 10.2%, polyurethane (PU) 8.4%, and polystyrene (PS) 7.8% (Geyer et al., 2017). The abovementioned plastic types have different applications; for example, PE is usually used in packaging products that have a short shelf life, like plastic bags, bottles, cups, and storage containers. PS is usually used in utensils, tableware, cafeteria trays, and containers. PET is typically used in bottles and cups. PP is used in auto parts, food containers, and industrial fibers. PVC is commonly used in building construction, as it has much longer lifetime. PU is used in medical devices, packaging, coating, and building construction (Tokiwa et al., 2009; GESAMP, 2015). Table 1 summarizes the main applications of the most used abovementioned plastics.
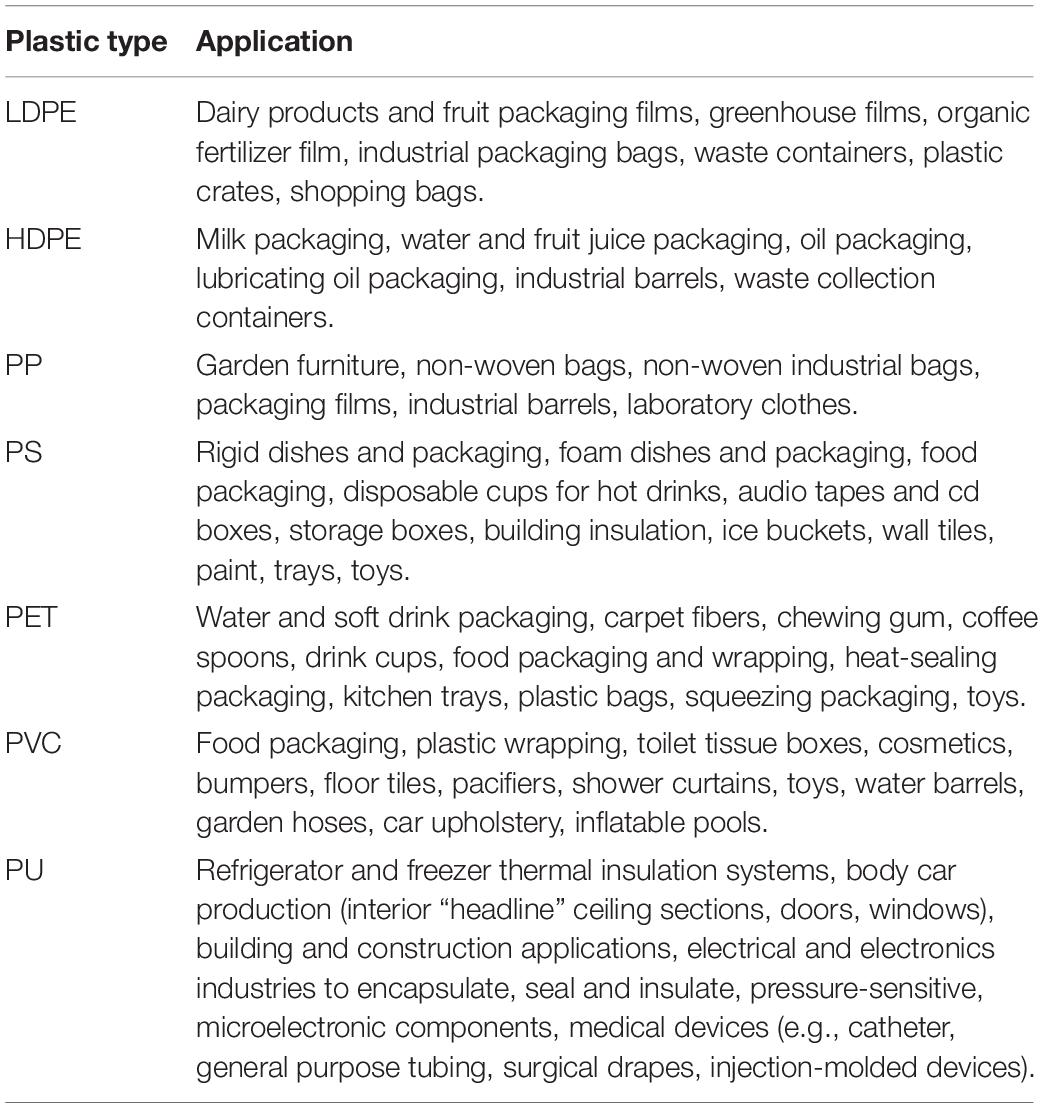
Table 1. Plastics main applications (Gondal and Siddiqui, 2007).
Plastic materials can be classified in different ways; however, within the scope of this review it is important to distinguish two categories: thermosetting and thermoplastics (Lithner et al., 2011).
Thermosets are plastics that harden during their manufacturing and hot molding processes. They solidify forming a solid and stable body, which prevents their subsequent reuse. Therefore, they cannot be transformed again by softening and molding. Examples of thermosetting products include “bakelite,” urea-formaldehyde resin, epoxy resins, vulcanized rubber, and some polyurethanes. They are particularly used in mechanics and specifically in the automobile industry.
Thermoplastics are very high molecular weight polymers, rigid or flexible at room temperature, but soft and elastic at high temperatures. Thus, they can be molded plastically as many times as necessary, returning to the solid state after cooling. As this process can be repeated several times, these plastics are recyclable and can be reused. The recycled thermoplastic cannot be used in food packaging to avoid contamination from paints and toxic products and can return in the form of buckets, hoses, garbage bags, and other modalities. This category includes the main six families of plastics: low-density polyethylene (LDPE), high-density polyethylene (HDPE), polypropylene (PP), polystyrene (PS), polyethylene terephthalate (PET), and polyvinyl chloride (PVC).
Polyethylene (PE)
Polyethylene is considered the simplest basic structure of any polymer and constitutes the most commercialized group of alkanes. PE is a linear hydrocarbon polymer consisting of long chains of ethylene monomers (C2H4)n (Huerta et al., 2018; Figure 4A). Basically, it is formed by opening the double chain of ethylene molecules and joining them in straight or branched chains. This structure gives PE a hydrophobic nature and a dimension too large to pass through microbial cell membranes (Arutchelvi et al., 2008).
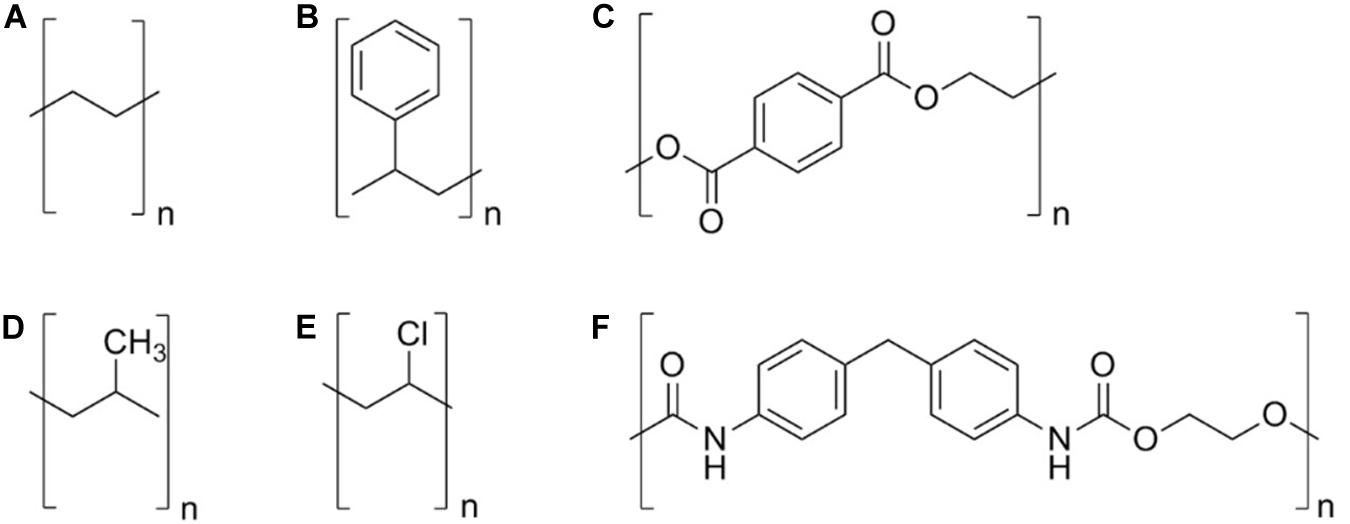
Figure 4. Molecular structure of plastic polymers. (A) Polyethylene, (B) polystyrene, (C) poly(ethylene terephthalate), (D) polypropylene, (E) polyvinyl chloride, and (F) polyurethane.
Polyethylene is made from petrochemical feedstock through an efficient catalytic polymerization process and it can be easily processed to various products (Arutchelvi et al., 2008).
Polyethylene reached a widespread use in food and beverages, in the 1950s (Bardají et al., 2020). PE has several characteristics that made it attractive in trade, like low cost, ease of manufacture, chemical resistance, processability, flexibility, versatility, toughness, and excellent electrical insulation (Ronca, 2017; Bardají et al., 2020). Hence, PE has a large range of applications, such as food packaging, textiles, lab equipment, and automotive components (Arutchelvi et al., 2008). Polyethylene can be classified in low-density polyethylene (LDPE) and high-density polyethylene (HDPE), because of differences in toughness, resistance to chemicals, flexibility, and clarity. HDPE is characterized by its large density to strength ratio, which means that its intermolecular forces are strong and are resistant to several solvents. On the other hand, LDPE is resistant to acids, alcohols, bases, and esters but is unstable in the presence of strong oxidizing agents and aliphatic and aromatic hydrocarbons (Scalenghe, 2018). HDPE has mostly linear chains, while LDPE has a high degree of branching. PE is considered a recalcitrant material, which contributed to the prevalence and overaccumulation of PE in the environment. PE has good properties for use in packaging, is a good barrier to moisture and water vapor, and is heat stable. These polymers are not the most efficient barrier to oils, fats, and gases such as oxygen and carbon dioxide, compared to other plastics, although the barrier properties can be improved by increasing density. Heat resistance is also lower than other plastics used in packaging, having a melting point of 120°C. They are very likely to generate static charges and therefore need an anti-static agent. Despite this, PE biodegradation studies by microorganisms have had success in the past decade (Soni et al., 2008).
Polystyrene (PS)
Polystyrene is a homopolymer resulting from the polymerization of styrene monomers (Figure 4B). In the pure solid state, it is colorless and hard and has limited flexibility (Mckeen, 2014). Polystyrene is the most durable thermoplastic polymer, existing in three forms: crystalline, high impact, and expanded. This plastic type is used in a wide range of products, as a result of low cost, ease of production, high transparency/easy coloring, high resistance, including to biodegradation, stiffness, light weight, shiny surface, and low barrier to water vapor and common gases, making it suitable for packaging products that need air flow (Mor and Sivan, 2008; Abhijith et al., 2018).
The increasing use of PS began when it was combined with rubber reinforcement, allowing to obtain a dull plastic and to be used in the packaging industry (Cai et al., 2017).
Poly(Ethylene Terephthalate) (PET)
Poly(ethylene terephthalate) is formed through polycondensation of terephthalic acid (TPA) and ethylene glycol (EG) or by transesterification of dimethyl terephthalate and EG (Figure 4C; Hiraga et al., 2019). Poly(ethylene terephthalate) is a crystalline polyester, colorless, and chemically stable, often used in the manufacture of food and drink containers and packaging, as well as in electronic components. PET has high resistance to heat, alcohols, and solvents and, when oriented, has very high mechanical resistance, such as to wear and tear. It also represents a medium barrier to oxygen. Its melting point occurs at high temperature, 260°C, making it suitable for high-temperature applications such as steam sterilization and microwave cooking (Crawford and Quinn, 2017; Raheem et al., 2019).
Polypropylene (PP)
Polypropylene is a thermoplastic made from polymerization of propene (or propylene) monomers (Figure 4D). The polymerization process is similar to that carried out in the production of polyethylene. PP is the lightest polymer and may undergo a wide range of manufacturing processes, such as injection molding, extrusion, and expansion molding. Polypropylene exists in three different forms: crystalline, semi-crystalline, and amorphous, but commercial propylene is typically a mixture of 75% isotactic (semi-crystalline) and 25% atactic (amorphous) (Crawford and Quinn, 2017). This polymer is one of the most versatile plastics, used in packaging for cookies, snacks, and dry food. Polypropylene is stronger and denser than PE and more transparent in its natural form. Compared to polymers other than PE, it has the lowest density and is relatively low cost. It also has other suitable properties, such as being a barrier to water vapor and being resistant to oils and fats. Its high melting point makes it suitable for applications such as microwave packaging. PP has low surface tension and requires additional treatment to be suitable for printing, coatings and lamination (Spoerk et al., 2020). This polymer is used in filling, reinforcing, and blending and when combined with natural fibers is one of the most promising sources for creating natural-synthetic polymer composites (Shubhra, 2013).
Polyvinyl Chloride (PVC)
Polyvinyl chloride is a product of polymerization of the vinyl chloride monomer (VCM) using a process similar to the production of PE, PP, and PS (Figure 4E). PVC is a thermoplastic and amorphous polymer, and it is produced in two varieties, flexible as films and rigid for more structural applications (Crawford and Quinn, 2017). It is a chemically inert polymer, resistant to acids and inorganic chemicals, but sensitive to ultraviolet radiation. The presence of chloride in the polymer provides protection against oxidation and fire retarding properties, having an ignition temperature of 455°C (Crawford and Quinn, 2017). Without plasticization, PVC is a hard and brittle material that needs to be modified. PVC has excellent resistance to fats and oils. Its permeability to water vapor and gases depends on the amount of plasticizer used in the manufacture (Close et al., 1977). This polymer is used in building and construction industry.
Polyurethane (PU)
Polyurethane is a block copolymer, with altering polymeric segments by repeating unit “A” or “B.” These materials are designed so that one of the segments is crystalline or glassy at the use temperature (hard segment), while the other segment is designed to be malleable/rubbery (soft segment). The PU synthesis has two steps and employs three precursor molecules: diisocyanates, diols, and chain extenders. The diisocyanates and chain extenders form the hard segment, while the diols form the soft segment, in the final polymer molecule (Figure 4F). This type of block copolymer structure has unique and useful properties, making PU a very versatile polymer. Its excellent mechanical properties, biocompatibility, and stability cause PU to be widely used in different fields, for example, medical devices (e.g., pacemakers, blood contacting applications), building and construction, and coatings and in automotive applications (Heath and Cooper, 2013; Akindoyo et al., 2016).
Microplastics
Plastics can be harmful, especially when they become microplastics, thus representing a big environmental concern (Scalenghe, 2018). Marine litter can be divided into two large groups: macroplastics (fragments larger than 5 mm) and microplastics (smaller than 5 mm) (Hidalgo-Ruz et al., 2012). Microplastics are plastic particles between 1 μm and 5 mm in size, originating from different types of polymers that, due to characteristics, such as small size, low density, composition, and persistence in the environment, contribute to their global dispersion through sea currents (Desforges et al., 2014). Microplastic particles also consist of manufactured plastic material of microscopic size, such as abrasives used in industrial and household cleaning products (Derraik, 2002), industrial raw materials used for the production of plastics (Andrady, 2011), and plastic fragments and fibers derived from the breakdown of larger plastic products (Moore, 2007, 2008). These particles are present in many environments, including marine, freshwater, and terrestrial ecosystems. They have high molecular weight and hydrophobicity and are highly recalcitrant against biodegradation. Because of this, microplastics are considered emerging pollutants (Zhang et al., 2019; Park and Kim, 2019).
Microplastics originate from several sources and are categorized into primary and secondary microplastics. When the plastic particles are manufactured to have less than 5 mm size, they are primary microplastics, and when resulting from the breakdown of macroplastics, they are secondary. The primary microplastics include plastic powders used in molding, microbeads used in cosmetic formulations, and plastic nanoparticles used in a variety of industrial processes. Secondary microplastics result from the use of products like textiles, tires, and paints or can accumulate when the items that are released into the environment by incorrect discards, start to degrade through physical, chemical, and biological environmental factors. The thermo and photo oxidative processes in nature weaken the plastic integrity leading to the production of particles < 5 mm (GESAMP, 2015; Huerta et al., 2018; Correia et al., 2020). Microplastics of different polymers and physical characteristics are found in the marine environment in varying concentrations along the water column (Chubarenko et al., 2016). Patches of microplastics in the water column with concentration ranging from 257.9 to 1,215 particles per m3 have already been reported on the coast of South Africa (Nel and Froneman, 2015) and 0.15 particles per m3 in the waters of the Mediterranean Sea (de Lucia et al., 2014). The predominance of the type of microplastic (fragments, pellets, styrofoam, or fibers) also has a great variation in surface waters. The deposition areas, such as sandy beaches, are the main final destinations for microplastics in the marine environment (Turra et al., 2014). After being deposited on the sand beach, microplastics can pose ecological risks, due to changes in heat transfer, with slower heating and lower sediment maximum temperatures than sediments without microplastics, which can interfere with the eggs’ incubation/hatching time and temperature-dependent sex determination of several animals (Derraik, 2002; Carson et al., 2011). Moreover, microplastics have a severe negative impact in marine ecosystems and human health, either by ingestion (entering the food chain) or by leaching of toxic chemicals or by accumulation of organic pollutants (Figure 5). In addition, microplastics can be colonized by microorganisms and algae, creating a habitat and new microbial activities. The new communities are significantly different from those of the surrounding environments (Zhang et al., 2019). Microplastics can be associated with severe environmental contamination, since they can be found even in remote locations and far from their anthropogenic sources of emissions (Park and Kim, 2019).
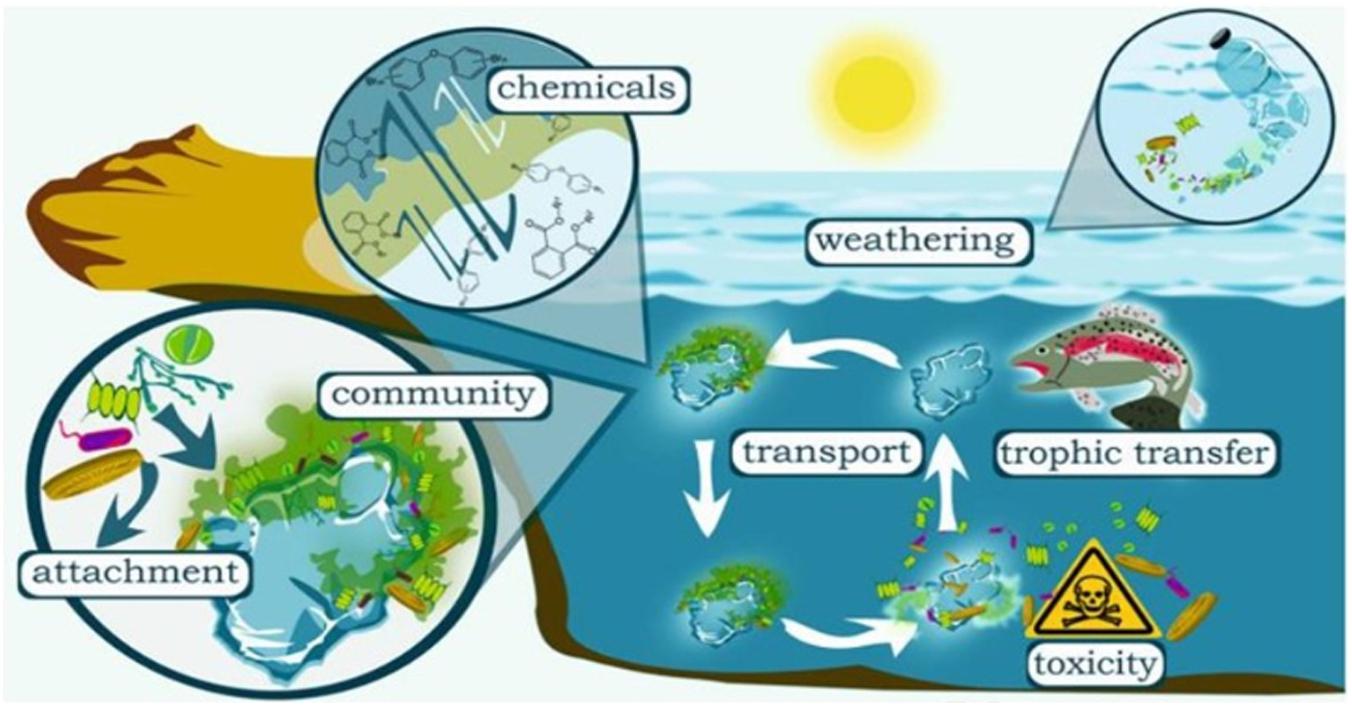
Figure 5. Abiotic and biotic plastic transformation into microplastics and leaching (Rummel et al., 2017). Reproduced with permission from Environmental Science and Technology Letters 2017, 4, 7, 258–267, available at https://pubs.acs.org/doi/10.1021/acs.estlett.7b00164. Copyright 2017, ACS Chemistry for Life.
Although microplastics still represent a small proportion of the total mass of plastics in the ocean, their concentration in seawater may reach 102,000 particles per m3 (Correia et al., 2020). This is an increasingly worrying issue as macroplastic waste in the sea undergoes continuous degradation and will prevail for many years, even after plastic discharges stop (GESAMP, 2015).
Microplastics Health Impact on Marine Organisms and Humans
Microplastics have a huge impact on health, since they can be transport vectors of persistent organic pollutants and heavy metals as well as a source of chemical pollutants themselves (Lee et al., 2014; Enders et al., 2015). Moreover, the small microplastic particles have the size range of zooplankton food, allowing their entrance in the food chain by marine animals, including accidental ingestion (Gregory, 2009). Microplastics can compete with nutritious food, which causes the loss of energetic resources that lead to sub-lethal effects in reproductive output (Enders et al., 2015). Large amounts of ingested plastic can cause intestinal blockage or stomach ulcers, reducing the absorption of nutrients (Connors and Smith, 1982; van Franeker, 1985). It can cause a false feeling of satiety (Gregory, 2009) in addition to hormonal changes harmful to the animals’ reproduction (Derraik, 2002). Changes like these can result in decreases in energy reserves, decreasing the ability to survive in adverse environmental conditions, with a consequent reduction in growth rates (Colabuono et al., 2009) and increased risk of death due to starvation, that is, weakening by absence or deficiency in food assimilation (Bugoni et al., 2001). The number of studies that point to the various negative ecological impacts caused by the entry of these microplastics into the marine environment is growing (Hidalgo-Ruz et al., 2012; Wright et al., 2013).
The consequences of microplastic exposure for humans are not fully understood, since this material is present in many consumer products leading to human exposure to these particles. Microplastics have been reported in a wide range of food items, like mussels, commercial fish, and table salt, among others (Neves et al., 2015; Li et al., 2016). There are different hypotheses for the paths of exposure and toxicity mechanisms associated with microplastics (Correia et al., 2020). The three main routes of exposure are through ingestion of food containing microplastics, through inhalation of microplastics in the air, and by dermal contact with these particles (Revel et al., 2018).
According to studies based on the consumption of food, intake of 39,000 to 52,000 microplastic particles per person each year is estimated (Cox et al., 2019). When these particles reach the gastrointestinal system, they may lead to inflammatory response and changes in gut microbe composition and metabolism (Salim et al., 2014). Contaminated food is not the only problem, since packaging and plastic containers can also act as contamination sources (Rist et al., 2018).
Microplastics can also be inhaled, since these particles are released to the air by numerous sources (synthetic textiles, resuspension of microplastics in surfaces, and abrasion of materials, like car tires). It was estimated that individual inhalation per day is between 26 and 130 airborne microplastics (Prata, 2018). The outdoor microplastic concentrations were determined to be between 0.3 and 1.5 particles per m3 and the indoor concentration 0.4 to 56.5 particles per cubic meter (Mandin et al., 2017). The microplastic particles’ size and density influence their deposition on respiratory system. The less dense and smaller particles settle deepest in lungs. When microplastic particles deposit, the large surface area of these particles may induce intense release of chemotactic factors, leading to chronic inflammation (Correia et al., 2020).
Furthermore, dermal contact by dust, synthetic fibers, and microbeads in cosmetics is considered a route of exposure, but less significant. It has been speculated that nanoparticles smaller than 100 nm could transverse the dermal barrier (Revel et al., 2018). Evidence is still needed, but if proven, nanoparticles can cause toxicity after crossing the dermal barrier, inducing low inflammatory reactions and foreign body reactions, causing fibrous encapsulation (Correia et al., 2020).
Microplastics have a high surface area which may lead to oxidative stress and cytotoxicity. Due to their persistent nature, their removal from the organism is difficult. This can cause chronic inflammation, which increases the risk of cancer (Kirstein et al., 2016; Revel et al., 2018).
Degradation of (Micro)Plastics
A current approach to the development of new polymeric materials is related to their life cycle and considers environmental impact, from transformation until when becoming useless (Al-Rasheed et al., 2019; Abbas-Abadi, 2020). When discarded inadequately or in uncontrolled conditions, polymeric materials threaten natural environments and the planet’s life quality (Fernandes et al., 2002). Although few products are designed considering their final destination (disposal or recycling), easy-to-dispose plastics are being widely criticized, due to the visual pollution they cause, the difficulty of removing them in the environment, and their high resistance to degradation. Degradation is a chemical reaction that leads to the fission of polymer chains, which can be caused by different types of physical and chemical agents and can irreversibly modify the properties of polymeric materials (Santos et al., 2002). One of the challenges of this century is evaluating how long a material resists, before degrading or biodegrading, so that its final disposal occurs in ecologically acceptable conditions.
Studies indicate that after disposal, thermoplastics degrade by various mechanisms, which can occur gradually or rapidly, depending on the conditions to which they are subjected to, as well as their chemical nature. Saturated polymeric chains do not favor degradation by microorganisms, unlike biodegradable polymers that might include heteroatoms in the molecular chain and thus, when subjected to favorable conditions, rapidly degrade (Raghavan, 1995).
Biodegradable polymers, such as chitosan or cellulose, have been studied as an environmentally friendly alternative to gradually degradable or non-biodegradable polymers. However, it is known that the biodegradable polymers available today have unsatisfactory mechanical or surface properties for certain applications; some have high cost, which restricts their use in certain applications (Fernandes et al., 2002; Shah and Vasava, 2019).
When plastics enter the marine environment, it is the material density, compared with seawater density, that mostly influences the behavior of this material, determining whether the plastic material floats or sinks.
The degradation process of a polymer depends on its nature and the conditions that it is subjected to, ranging from abiotic factors (sun, heat, humidity) to assimilation by microorganisms (bacteria and fungi) (Karamanlioglu et al., 2017). Therefore, the polymer degradation can be classified as abiotic or biotic (Figure 5). The abiotic degradation is classified as deterioration caused by environmental factors, like temperature, UV irradiation, wind, and waves. On the other hand, the biotic degradation is classified as the biodegradation caused by the action of microorganisms that modify and consume the polymer, changing its properties. Usually, in nature, both degradation types act simultaneously (Restrepo-Flórez et al., 2014).
Abiotic Degradation
Plastics are generally not biodegradable, so they can persist for long periods of time. UV radiation is the central cause of plastic degradation in the environment because the plastic debris on soils or beaches or floating in the water is exposed to solar radiation, facilitating oxidative degradation of polymers. Through advanced stages of degradation, plastic develops surface features, becoming weak and starting to lose mechanical integrity. For this reason, any mechanical forces (e.g., waves, wind, etc.) can break the degraded plastic into progressively smaller particles (GESAMP, 2015; Scalenghe, 2018). The abiotic degradation reactions of polymers can be classified as thermal, mechanical, and chemical degradation (photodegradation, thermo-oxidation, photo-oxidation) (Sazanov et al., 1979; Scott, 1995; Kamo et al., 2004; Li et al., 2004; Chen et al., 2018).
Thermal degradation refers to the reaction of degradation at temperatures higher than those supported by the polymeric structures, which can induce chemical changes in polymers. Most organic polymers are sensitive to temperature, changing their stability by the action of heat, and these characteristics vary with the type of chemical structure of the polymer (Sazanov et al., 1979; Kamo et al., 2004).
Thermo-oxidation is the degradation of polymers by the action of light, heat, chemical attack, or shear, causing the loss of properties, by reaction with oxygen, and the formation of oxidation products and carbon dioxide (CO2). This process can occur according to a mechanism that comprises four stages: initiation, propagation, branching, and termination (Hawkins, 1964; Neiman, 1964; Drozdov, 2007).
Normally, the degradation processes occur through chain reactions, via free radicals, when there is a gradual deterioration of the polymer’s properties, with polymeric chain and polymeric chain branch disruptions. The initiation of the oxidation process, with the generation of free radicals in the presence of oxygen can be promoted or accelerated by the action of UV light, called photo-oxidation (Rabek and Ranby, 1974a,b; Rabek et al., 1975, 1976, 1977; Ranby, 1978; Jian et al., 1985).
Photodegradation is responsible for modifications in materials decomposed by solar radiation. This process changes the surface appearance of the materials; some of the most relevant modifications are discoloration (yellowing), brittle surfaces, surface hardening, and decreased values of mechanical and other properties (Andrady et al., 1998; Martínez et al., 2004).
The products generated in plastic degradation (polyolefin chain) by thermal energy are similar to the ones generated by UV radiation, with a difference in the amount of acids generated, which is higher in the photo-oxidation process (Pickett and Moore, 1993). Unlike thermal oxidation that occurs at the plastic surface and inner layers, photo-oxidation is a process limited to the surface (Pospísil et al., 2006). Heat-initiated peroxidation yields more stable ketone products, when compared to the UV radiation process (Wiles and Scott, 2006).
Biodegradation
Biotic degradation takes place by the action of microbial enzymes. Biodegradation converts organic compounds into simpler organic compounds, mineralized, and redistributed in elementary cycles, such as carbon, nitrogen, and sulfur. As sub-products of this process, carbon dioxide, methane, and microbial cellular components are generated, among others (Chandra and Rustgi, 1998; Banker et al., 2016).
Two types of microorganisms, bacteria and fungi, are of particular interest in the biodegradation of natural and synthetic polymers (Rujnić-Sokele and Pilipović, 2017). Biodegradation depends on the environment as the biodiversity and occurrence of microorganisms vary locally. There are two levels of factors that affect plastics biodegradability, the microbial level and the polymer characteristic level. Microbial characteristics comprise the microorganism type, distribution, growth conditions (temperature, pH, oxygen concentration, nutrients, etc.), and enzyme types (intracellular and/or extracellular enzymes, leading to exo or endo polymer cleavage). The chemical and physical properties of polymer characteristics include surface conditions (surface area, hydrophilic and hydrophobic properties), first-order structures (chemical structure, molecular weight, and molecular distribution), and higher-order structures (glass transition temperature, melting temperature, modulus of elasticity, crystallinity, and crystal structure) (Tokiwa et al., 2009).
The principal mechanism for biodegradation is the adherence of microorganisms to the polymer surface, followed by colonization of the exposed surface. After colonization, the polymer enzymatic degradation occurs by hydrolysis; first the enzyme binds to the polymer substrate and then catalyzes the hydrolytic cleavage. Through the biodegradation process, polymers become low molecular weight oligomers, dimers, and monomers, until final mineralization to CO2 and H2O (Tokiwa et al., 2009).
The extent of colonization on the polymer can be quantified by the surface characterization, where hydrophilic surfaces are more easily colonized by microorganisms. This is a limitation, because the polymer high hydrophobic surface contrasts with the microorganisms hydrophilic surface (Restrepo-Flórez et al., 2014).
Crystallinity also influences the biodegradation rate; the more crystalline a material is, the harder the degradation by microorganisms will be. As a consequence, the amorphous regions are consumed first, because the polymer monomers are loosely packed in these zones (Restrepo-Flórez et al., 2014).
The molecular weight is also a biodegradability factor, because polymers with side chains are less assimilated than polymers without side chains; hence, the increasing of molecular weight decreases the biodegradability of the polymer (Tokiwa et al., 2009).
Recently, the investigations on microbial degradation of plastic material have been focused on the isolation of microorganisms from natural environments (terrestrial and marine) (Park and Kim, 2019). Microorganisms living in environments polluted with microplastics are more likely to already have an adapted metabolism, so their polymer degrading potential is higher.
Microorganisms Capable of Degrading (Micro)Plastics
In nature, (micro)plastic degradation is an integrated process of physicochemical and microbial degradation factors. Microorganisms can adapt to almost every environment and have the potential to degrade several compounds, including (micro)plastics (Brooks and Beer, 2012; Krueger et al., 2015). Therefore, microorganisms can be used in bioremediation, without causing adverse effects. However, there is still lack of knowledge about the interactions between microorganisms and (micro)plastics, which prevents a wider adoption of plastic biodegradation approaches (Shah et al., 2008a; Alshehrei, 2017).
In several reported studies, bacterial biofilms, pure bacterial cultures, bacterial consortia, and pure fungal cultures were used for plastic degradation. These studies are presented in Tables 2–4.
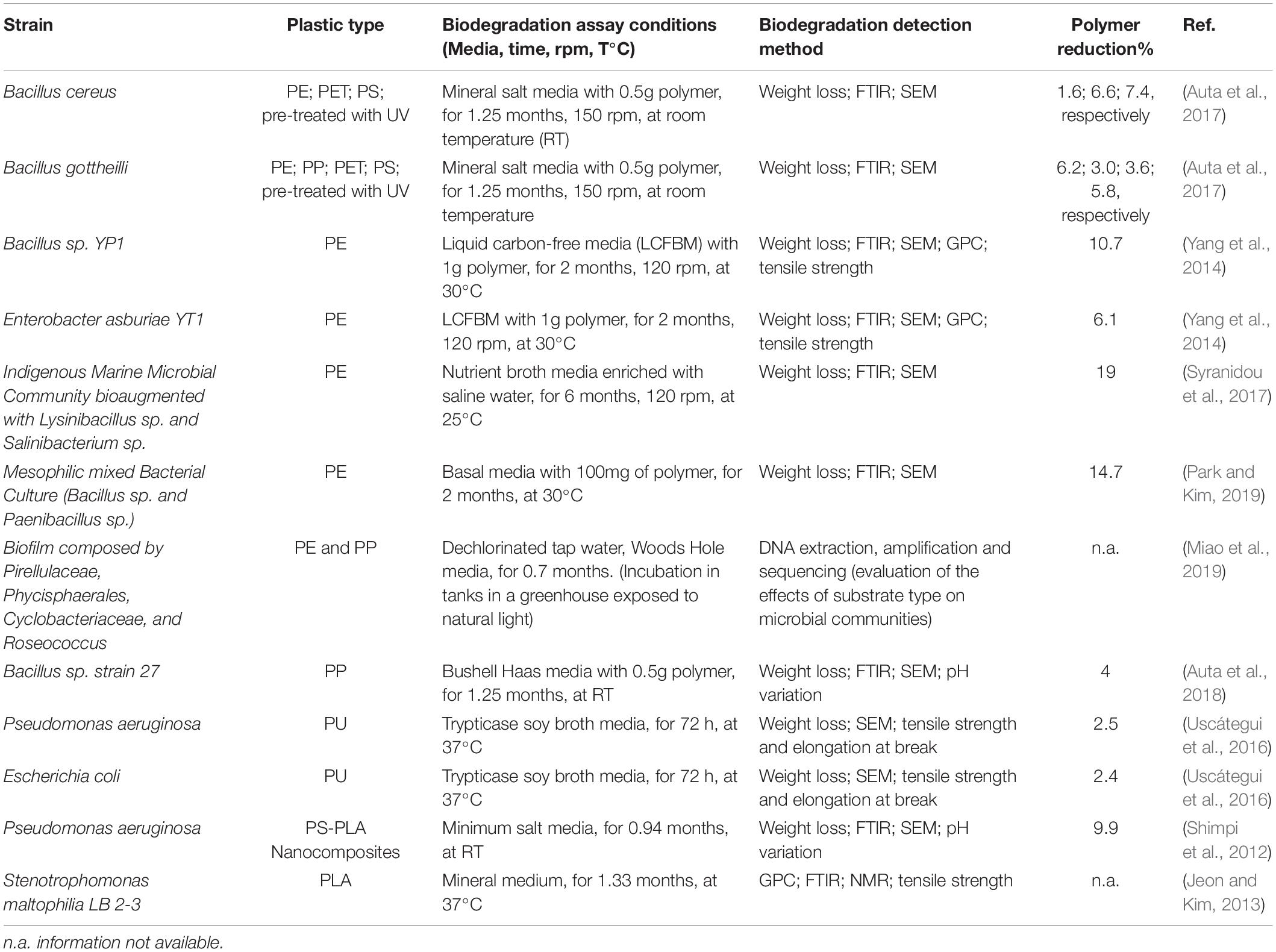
Table 2. Plastics degraded by microorganisms, biodegradation assay conditions, and used detection methods.
In the environment, (micro)plastics are in contact with microorganisms, organic matter, and inorganic particles. This promotes their attachment to the surface of (micro)plastics, which serve as substrate, creating a favorable environment for the development of biofilms by different types of microorganisms, such as bacteria, fungi, algae, protists, and viruses (Yuan et al., 2020). This leads to the (micro)plastic structural damage and loss of properties as a result of microbial enzymatic reactions (Miao et al., 2019). Microplastic and plastic fragments can offer support for colonization and growth of microorganisms, while serving as a carbon source (Yuan et al., 2020). The biofilm formation and mediated degradation of microplastics has four stages: (1) microorganisms adhere to the surface of (micro)plastics and alter their surface properties, (2) biodeterioration, where secreted enzymes increase degradation, leading to the release of additives and monomers out of the (micro)plastics, (3) biofragmentation—the plastic material starts losing its mechanical stability and becomes fragile, due to the microbial attack, and (4) assimilation—during the fourth stage microbial filaments and water start to penetrate the polymer, which results in decomposition and utilization of (micro)plastics by microorganisms (Yuan et al., 2020).
Bacteria
Bacterial strains catalyze metabolic reactions that contribute to (micro)plastic adsorption, desorption, and breakdown (Auta et al., 2017, 2018). These microorganisms use polymer materials as the sole carbon source in minimal nutrient media, reducing the dry weight, average molecular weight, and molecular distribution of polymers and induce changes in morphological structure and chemical structure. This implies that in practice, these microorganisms can be used for the reduction of plastic and microplastic pollution in the environment. The use of pure strains allows the discovery of metabolic pathways to evaluate the effect of different environmental conditions on (micro)plastic degradation (Janssen et al., 2002). Bacterial consortia, i.e., two or more bacteria living symbiotically, were also used to study (micro)plastic degradation. The use of bacterial consortia offers a stable microbial community, eliminating the effects of toxic metabolites produced by some strains present in the consortia (Yuan et al., 2020). Usually, toxic metabolites produced by one strain can be used as a substrate by another strain (Singh and Wahid, 2015). This may be the cause for the better biodegradation efficiency, since these consortia show degradation levels above the average obtained for pure strains.
Table 2 integrates the knowledge on the type of microorganisms, assay conditions, detection methods, and polymer reduction percentage obtained in various studies. The biodegradation results are different depending on the used strain and on the incubation conditions.
In the case of Bacillus cereus and Bacillus gottheilii, microplastics were pretreated with UV radiation. The pretreatment could improve the degradation by the Bacillus strains, by increasing the susceptibility of the microplastics, since during the radiation process C=O and O–H groups are introduced. Furthermore, UV radiation decreased the hydrophobicity of microplastics and this promoted and improved the compatibility with the microorganisms (Arutchelvi et al., 2008). Both strains were able to change the surface of the microplastics, where cracks and grooves appeared and altered structural functional groups and other properties. Moreover, the two strains showed different responses to the different microplastics. B. cereus had greater response to PS, causing a higher weight lost percentage, but B. gottheilii showed higher capacity to degrade a wide variety of microplastics and can be considered a potential multiple degrader (Auta et al., 2017).
Bacillus sp. strain 27 colonized, modified, and used PP as sole carbon source, confirmed by the formation of several pores and irregularities on the surface of the microplastics (Auta et al., 2018). Bacillus sp. YP1 degraded about 10.7% of initial PE weight, in only 2 months, creating damage in the microplastic surface, including holes and pits, and introducing carbonyl groups during the biodegradation assays, thus revealing high degrading capacity. This strain formed a biofilm on PE, and this enabled the microorganisms to utilize the non-soluble substrate efficiently (Yang et al., 2014). The combination of microplastics with a bioplastic, like poly(lactic acid) (PLA), can enhance the polymer biodegradability. Lactic acid is a fermented product from microbial renewable sources and is a naturally occurring nontoxic material. PLA decomposition is a rapid process occurring in a typical compost environment, forming CO2, water, and biomass. When this strategy was used with Pseudomonas aeruginosa, 9.9% biodegradation of the PS and PLA nanocomposites was obtained. The maximum biodegradation rate by P. aeruginosa was obtained by using about 10% of PLA in nanocomposites (Shimpi et al., 2012).
Actinobacteria
Nature often provides the solution for our problems, which can be an inspiration and a source of diverse products (Grassle and Maciolek, 2013). Actinobacteria, commonly named actinomycetes, are Gram-positive bacteria. These bacteria are present in various ecological habitats, like soils and freshwater and marine environments (Arasu et al., 2012). Actinomycetes have numerous distinct metabolisms and can have different functions in the environment. Besides the production of antibiotics, anticancer drugs, fungicides, and other bioactive secondary metabolites, some can digest resistant carbohydrates (for example cellulose and chitin); others are used in bioremediation due to their ability to degrade toxic materials or play a role in the recycling of organic carbon and, recently discovered, in the degradation of complex polymers (Sharma et al., 2014). Several studies reported the degradation of plastics/microplastics by actinomycetes and are presented in Table 3.
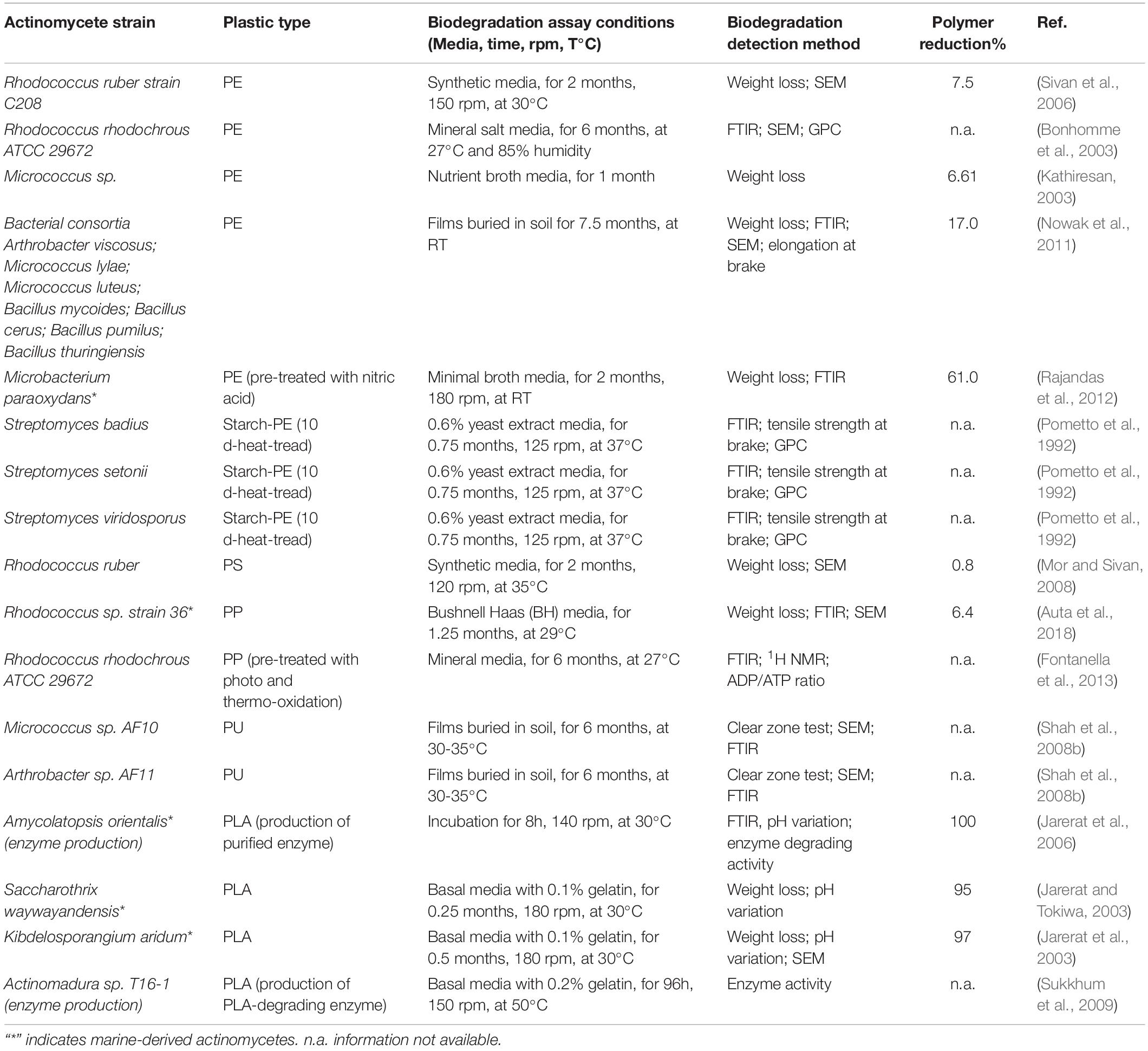
Table 3. Plastics degraded by actinomycetes, biodegradation assay conditions, and used detection methods.
Actinomycetes have relatively good degradation capacities of several microplastics types (Table 3). When using Rhodococcus ruber strain C208, the initial signs of degradation appeared after only 16 days. This species could form a biofilm in PE surface, and microcolonies started to be organized and differentiated after 12–15 h incubation, increasing their size, shape, and density, leading to the formation of three-dimensional multicellular structures. After 1 day of incubation, the biofilm structures reached their final size and a small portion of bacteria formed a scarce single-layer biofilm, surrounding the complex biofilm structure. In only 2 months, R. ruber degraded 7.5% of the initial weight of the microplastic and could use PE as a sole carbon source (Sivan et al., 2006). Rhodococcus ruber can be considered a model species in polymer degradation. Another Rhodococcus strain, R. rhodococcus ATCC 29672, showed convincing biodegradation results. In this case, the polymer was pretreated, subject to photo aging, and a sharp decrease of the weight and molecular distribution of the polymer was observed (Fontanella et al., 2013). This UV pretreatment method simulates the natural environment, that is, the plastic entry into the environment and its abiotic degradation (caused by environmental factors), followed by the biotic degradation (polymer consumption). So, this type of pretreatment mimics the real-environment conditions, thus enhancing biodegradability.
Bacterial consortia with actinomycetes and different Bacillus species presented high capacity of biodegradation. After incubation, besides the weight loss indicated in Table 3, the microplastic underwent structural, physical and chemical changes, such as the appearance of holes and narrow gaps (Nowak et al., 2011). This consortium, composed by actinomycetes and Bacillus, may have contributed to the increased polymer reduction, since the genus Bacillus has good degradative capacities (section “Bacteria”).
Pretreatment with nitric acid represents an advantage for the degradation by microorganisms. Nitric acid reduces native bonds in polymers and breaks them into smaller chains, allowing the incorporation of carbonyl groups in the backbone of the polymers, which enhanced the degradation rate by Microbacterium paraoxydans, since carbonyl groups are biodegradation triggers (Rajandas et al., 2012). Pretreatments in polymers were used to obtain materials that can be emulsified, soluble in water or in low boiling solvents. Hence, pretreatment is advantageous for enhancing biodegradation. Polymers in their original form take long time to degrade, but the use of nitric acid facilitates biodegradation, to values of 61% of polymer reduction, making it the highest reported reduction of polymers from non-renewable sources (Rajandas et al., 2012).
The members of the phylum Actinobacteria demonstrate an efficient degradation capacity of polylactic acid (PLA). This has a positive impact, since commercially available PLA is one of the most exploited bioplastics as a biodegradable polymer alternative. PLA-degrading actinomycetes can degrade this biopolymer either under field trials or laboratory conditions (Butbunchu and Pathom-aree, 2019). In Table 3, Amycolatopsis orientalis produced extracellular PLA-degrading enzyme with potent degrading activity, meaning that the PLA powder was completely degraded within 8 h. Kibdelosporangium aridum strain also showed high biodegradation ability, reducing about 97% of the initial polymer. Furthermore, this strain created many small pits over the PLA surface, and with the progress of degradation, most of the surfaces were attacked and the pits became deeper (Jarerat et al., 2003). Strains from Amycolatopsis and Actinomadura genera were reported as having promising PLA degradation capacity. These should be further investigated as the market demand for PLA plastics is increasing (Butbunchu and Pathom-aree, 2019).
Within these studies, PU, a polymer not widely used in biodegradation assays under laboratory conditions, proved to be susceptible for microbial degradation. Also, the addition of gelatin (0.1–0.2%), in some studies, revealed to be the best organic nitrogen source, as well as an inducer, increasing the biodegradation rate and polymer reduction by microorganisms. The polymer percentage reduction can be due to microbial activity and indicates not only weight loss, but also changes in the chemical and physical structures of the polymers.
Marine-derived actinomycetes are highlighted with “∗” in Table 3. These revealed high polymer degradation as well as polymer structural and chemical changes. Therefore, marine actinomycete counterparts appear to be quite effective in using polymers as a sole carbon source, especially for PLA degradation.
Fungi
Due to their vast metabolic potential, including the extracellular multienzyme complexes (Matavulj and Molitoris, 2009; Kettner et al., 2017), fungi are natural candidates for the research of their plastic biodegradability capabilities. As with bacterial species, (micro)plastic biofilms are a promising resource of fungal species that can degrade plastic fragments. Importantly, biofilms represent a hotspot for microbial activity (including fungal growth) as the composition fungal communities on biofilms significantly differs from surrounding environments (Kettner et al., 2017). On one hand, this might represent a source of pathogenic species and thus an environmental and health threat; on the other hand, these communities represent an opportunity for identification of novel plastic degrading metabolic pathways and species. Importantly, the studies involving the potential of marine fungal species for plastic degradation have often been overlooked in comparison with the bacterial ones, hence there is potential for knowledge creation and provision of new solutions using fungal species.
Fungi can efficiently degrade several types of plastics, as described in Table 4. In fact, fungi revealed higher degradation rates and capacity for degrading several polymer types than actinomycetes and other bacteria. Several strains of the Aspergillus, Penicillium, and Trichoderma genera, namely, Aspergillus niger, A. versicolor, Penicillium pinophilum, P. frequentan, P. oxalicum, and P. chrysogenum, were capable of degrading polyethylene (Table 4; Restrepo-Flórez et al., 2014; Pathak and Navneet, 2017; Munir et al., 2018; Luz et al., 2019). Moreover, Fusarium redolens, Phanerochaete chrysosporium, Acremonium kiliense, Verticillium lecanii, and Glioclodium virens were also able to degrade PE (Restrepo-Flórez et al., 2014; Pathak and Navneet, 2017). All abovementioned fungi were capable of either colonizing or performing PE biodegradation, or both.
Zalerion maritimum, a marine fungus, exhibited the highest PE degradative capacity (43%), in minimum growth media, using it as a sole carbon source. It caused severe damage to the PE films, decreasing their mass and size, with increase of its biomass (Paço et al., 2017).
Fungal degradation can be enhanced using plastic pretreatment with UV radiation before the biodegradation assay. UV light is an initiator of PE oxidation; it produces carbonyl groups that are the main factors for the beginning of biodegradation, promoting the microorganisms’ attack on the shorter segments of PE molecular chains. One example is strain Trichoderma harzianum that efficiently degraded PE treated with UV radiation, causing the formation of cavities and erosion on the plastic surface, as well as the formation of new chemical groups, detected by FTIR and NMR (Sowmya et al., 2014). On the other hand, exposing films to the sunlight as pretreatment is not enough to promote biodegradation, but this method is important to increase strains growth on the polymers, as the case of Pleurotus ostreatus. Thus, the combination of abiotic and biotic processes is more efficient for microplastic degradation (Luz et al., 2019).
Polyurethane biodegradation by fungi can occur under suboptimal laboratory conditions and under a variety of landfill conditions. However, the temperature, humidity, and carbon source availability in the soil and other environmental conditions are important for PU biodegradation (Cosgrove et al., 2007; Khan et al., 2017). cladosporioides cladosporioide, Phoma sp., and Aspergillus tubingensis were capable of degrading PU by 90–95% (Table 4), causing damage to the films, such as erosion, cracking the surface, pore formation, and loss of tensile strength (Cosgrove et al., 2007; Khan et al., 2017; Brunner et al., 2018).
Polystyrene is not recyclable nor biodegradable (Chaudhary and Vijayakumar, 2020), which can explain the low degradation rates presented by Cephalosporium sp. and Mucor sp. However, morphological changes in the PS surface, which led to the appearance of cracks, holes, and erosion, were observed (Chaudhary and Vijayakumar, 2020). When polystyrene is used in biodegradation assays in the form of styrofoam, the biodegradation rate is much higher. In this case, a malt extract liquid medium was used, a rich source of carbon and nitrogen that promoted the microbial growth and supported the production of enzymes. Media optimization plays an important role in biodegradation efficiency (Yanto et al., 2019).
For PLA, temperature is a key parameter when considering fungal degradation (Qi et al., 2017). When PLA is near its temperature of glass transition (50–62°C), the biodegradation increases substantially, as in this state the hydrolysis of the ester linkages and microbial attachment are favored by the increasing of water absorption. Thermomyces lanuginosus strongly colonized the PLA surface and the polymer lost tensile strength (Karamanlioglu et al., 2014). The Tritirachium album medium for biodegradation assay was supplied by gelatin, which improved PLA biodegradation, leading to fragmentation, edge disintegration, the appearance of holes, and surface roughness (Jarerat and Tokiwa, 2001). Another strategy to increase biodegradation is blending the polymers with organic compounds or mixing them with other synthetic polymers, thus creating different properties on the polymers. For example, Trichoderma viride promoted the biodegradation of PLA plasticized with USE (epoxidized soybean oil). This USE addition increased the biodegradation rate of PLA, and polymer chemical structural modification was observed. Using a plasticizer can be a good option to improve biodegradation rates (Lipsa et al., 2016).
Fungal strains are also able to degrade PVC. Phanerochaete chrysosporium could adhere and grow on the surface of PVC films using this polymer as carbon source, which indicates the capacity to degrade this polymer (Ali et al., 2009).
Knowledge about the enzymes that induce plastic biodegradation is of utmost importance for future industrial application, as it may mimic microbial biodegradation without the use of the original microorganism.
Methods to Detect the Biodegradation of (Micro)Plastics
Several techniques can be applied to detect and quantify biodegradation activity by microorganisms (Figure 6). We have listed them to help the reader choose a method for performing a plastic biodegradation assay.
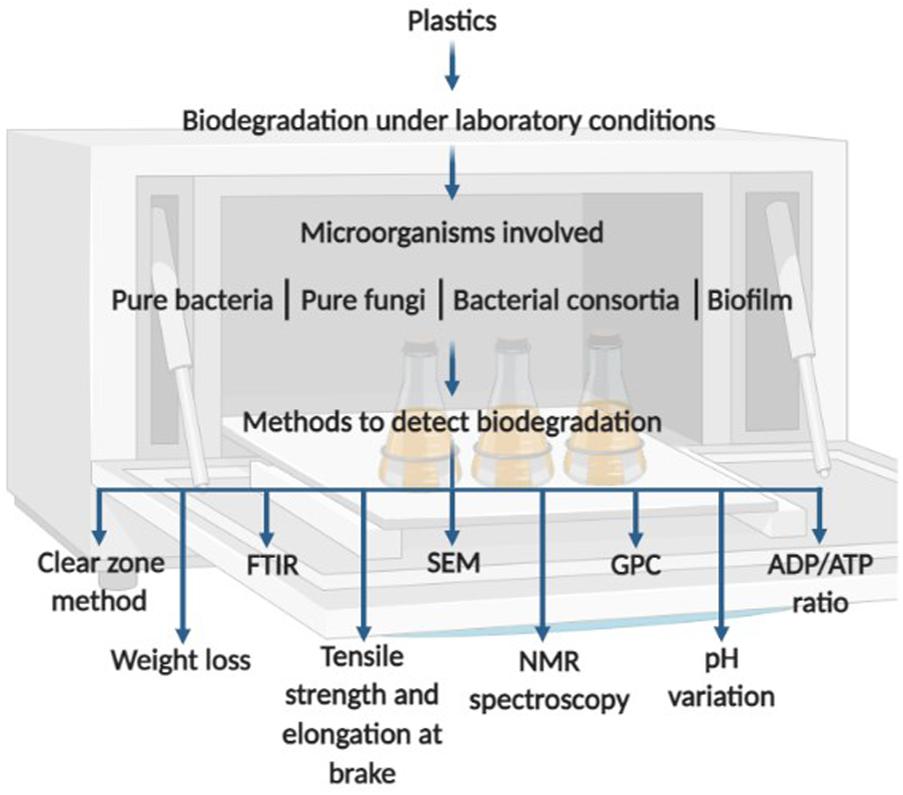
Figure 6. Methods used to detect plastics biodegradation under laboratory conditions. FTIR, Fourier Transform Infrared Spectroscopy; SEM, Scanning Electron Microscopy; NMR, Nuclear Magnetic Resonance spectroscopy; GPC, Gel Permeation Chromatography; ADP/ATP, adenosine diphosphate/adenosine triphosphate ratio.
Clear Zone Method
First, it is important to perform a previous screening of available microorganisms to discover which microorganisms have the capacity to degrade polymers and which polymers can be degraded. For this screening, the clear zone method with agar plates is the best approach, through the microorganism’s inoculation into agar plates containing emulsified polymers. This assay provides information on the degradation potential of microorganisms for a selected polymer. The microorganisms capable of degrading polymers excrete extracellular enzymes which diffuse through the agar and degrade the polymer into water-soluble materials (Mergaert and Swings, 1996). The main advantages of this method are the simplicity, relatively low cost, rapid response, and effectiveness. The solid media containing suspended polymer particles allow the isolation of potent degraders. When a microorganism grows in this media and consumes the polymer, a clear zone can be seen on a turbid plate, confirming that polymer degradation occurred. It does not necessarily indicate the consumption of all the compounds but reflects the breakdown of the polymer chains (Augusta et al., 1993). To perform the screening, it is required to incorporate the polymer in the medium. This incorporation can be done through emulsion, ground powder, or suspension. As this process can sometimes be complex, the use of chemical techniques to help in the incorporation process is advised. A chemical pretreatment of the polymers can be advantageous to obtain materials soluble in water or in low-boiling solvents. Pretreatment suggestions can be found in Section “Chemical Methods for Plastic Recycling Valorization.”
Gravimetric Determination of Weight Loss
This technique is widely used in polymer degradation assays for the evaluation of the gravimetric weight loss. This method should be used and interpreted carefully, since the weight loss can result from chemical hydrolysis and disintegration (fragmentation) of plastics, especially in the case of powder polymers. The weight loss results are highly inaccurate, since the biodegradation extent is limited and relatively slow (Raddadi and Fava, 2019). The weight loss depends on the incubation time and on the assay conditions, in addition to the used microorganisms and polymers (Nowak et al., 2011). This method must be associated with other techniques to prove that biodegradation occurred.
Fourier Transform Infrared Spectroscopy (FTIR)
Fourier transform infrared spectroscopy can be used to study and monitor chemical modifications in the polymer film structure. FTIR spectra give information about the polymer chemical functional groups, detecting chemical changes either by their consumption or by production due to the microbial activity (Hadad et al., 2005; Singh and Sharma, 2008). These changes occur in functional groups of the polymer’s surface, including hydrogen bonding, end-group detection, degradation reactions, molecular cross-linking, and copolymer composition in liquid or solid form, elucidating about modifications in the chemical and physical structure. FTR (Fourier transform Raman) spectroscopy is a fast and highly selective technique, identifying even the mineral fillers present in plastics (Azapagic et al., 2003). The efficiencies of FTIR and FTR spectroscopy allow the detection of most polymers. However, some limitations have been verified in FTIR spectroscopy, including a high sensitivity to the state of the polymer’s surface (Azapagic et al., 2003).
Scanning Electron Microscopy (SEM)
Scanning electron microscopy (SEM) allows the detection of microbial colonization and consequential surface degradation by changes in the physical aspect of the polymer surface. The evaluation of polymer biodegradation is seen by changes in the physical properties, by the formation of holes and cracks or by the formation of biofilms on polymer surfaces (Raddadi and Fava, 2019). The disadvantage of SEM is that it is costly and requires advanced training.
Gel Permeation Chromatography (GPC)
This technique is based on the average molecular weight (MW%) and the molecular weight distribution of the polymer. Usually, this analysis is performed on the bulk polymer and may not allow the detection of the initial steps of biodegradation, since biodegradation firstly occurs on the polymer surface. Therefore, GPC is not a highly sensitive technique but can be used combined with others, as the decrease in the MW of the polymer can be proof of a chain scission, which indicates microbial attack (Raddadi and Fava, 2019).
Tensile Strength (Rm) and Elongation at Brake (εr) Test
Modifications at tensile strength and elongation at brake are signs of biodegradation, i.e., microbial attack on polymers. Once the polymers are constituted with different monomers and the chains are oriented differently, they have distinct values for tensile strength and elongation at brake. This means that the force required to deform and break a polymer will be different. When microbial degradation takes place, significant changes in the mechanical properties occur, caused by biochemical modifications of the polymers. These modifications can result from the formation of cross-linking bonds between monomer chains or the film disintegration, shortening the polymer chains and serving as a source of carbon and energy. Depending on the microorganism, the polymer, and the incubation time, Rm and εr values differ (Nowak et al., 2011).
Nuclear Magnetic Resonance (NMR) Spectroscopy
Nuclear magnetic resonance is a technique that explores the magnetic properties of certain atomic nuclei (e.g., 1H nuclei) to determine physical or chemical properties of atoms or molecules that compose the polymers. This technique gives detailed information about structure, dynamics, reaction state, and chemical environment of the molecules. Most molecules are composed of hydrogen atoms, and this nucleus has a strong resonance, providing 1H NMR with broad applications. Protons in different chemical groups have different magnetic shielding; thus, 1H nuclei in different surroundings give rise to different chemical shifts (Gerothanassis et al., 2002). NMR spectroscopy allows the analysis of the molecular changes that occur during the biodegradation process. Changes in intensity of the peaks, integration values, and chemical shifting in the NMR spectra can be attributed to polymer biodegradation by microorganisms (Satti et al., 2020). With NMR, it is possible to analyze the molecular structure of the degraded products or their intermediates. The NMR analysis of the photo- and thermo-oxidized polymers can reveal the presence of degraded products and intermediates during biodegradation assays (Arutchelvi et al., 2008). This technique allows visualizing the soluble substrates obtained from the oxidized polymers that can be used as source of carbon and energy by the microbial cells (Fontanella et al., 2013).
pH Variation
pH is a very important factor for the survival and enzymatic activity of microorganisms, so it influences the overall biodegradation rate. pH variations are observed when biodegradation occurs, since intermediates and degraded products can affect the media pH. These pH variations can be advantageous for the growth and development of microorganisms (Auta et al., 2018).
ADP/ATP Ratio
Adenosine triphosphate (ATP) is a key molecule in all energetic metabolisms, and it reflects the levels of metabolic activity of a culture. In this assay, the concentrations of ATP and adenosine diphosphate (ADP) are measured, determining if microorganisms can maintain their energetic status over biodegradation time. So, the ADP/ATP ratio is extremely sensitive and quantitative, showing the energetic status of cells in the presence of different polymers (Fontanella et al., 2013).
Bioplastics as Alternatives to Petroleum-Based Plastics
It is a challenge to avoid plastics in our everyday life, as they are present in clothes, appliances, cosmetics, or food packaging, among others. As previously mentioned, plastics present disadvantages and associated environmental risks; an active field of research was established to provide alternative eco-friendly solutions. Nowadays, we may find sustainable takeaway containers from various natural and organic sources. For example, areca palm sheaths can replace plastics in products like bags, cups, plates, and wrapping. In addition, sal leaves, pineapple leaves, cactus leaves, Bauhinia vahlii leaves, coconut fibers/shells, and banana fibers can be used as food packaging and take-away containers, as these are waterproof. The soft fibers of the banana plants may be used as an alternative to plastic-based clothes (Muralidharan et al., 2017). Waterproof cork has been increasingly used for clothes, bags, umbrellas, and shoes, among others (Gil, 2014).
Bioplastics are biorelated polymers that have been considered suitable for replacing traditional fossil-based plastic materials and include two distinct concepts: biobased and biodegradable (Figure 7). Biobased plastics are bioplastics that contain organic carbon of renewable origin. On the other hand, biodegradable plastics are bioplastics that can be converted by microorganisms into natural substances such as water and carbon dioxide (Vert et al., 2014; Lambert and Wagner, 2017).
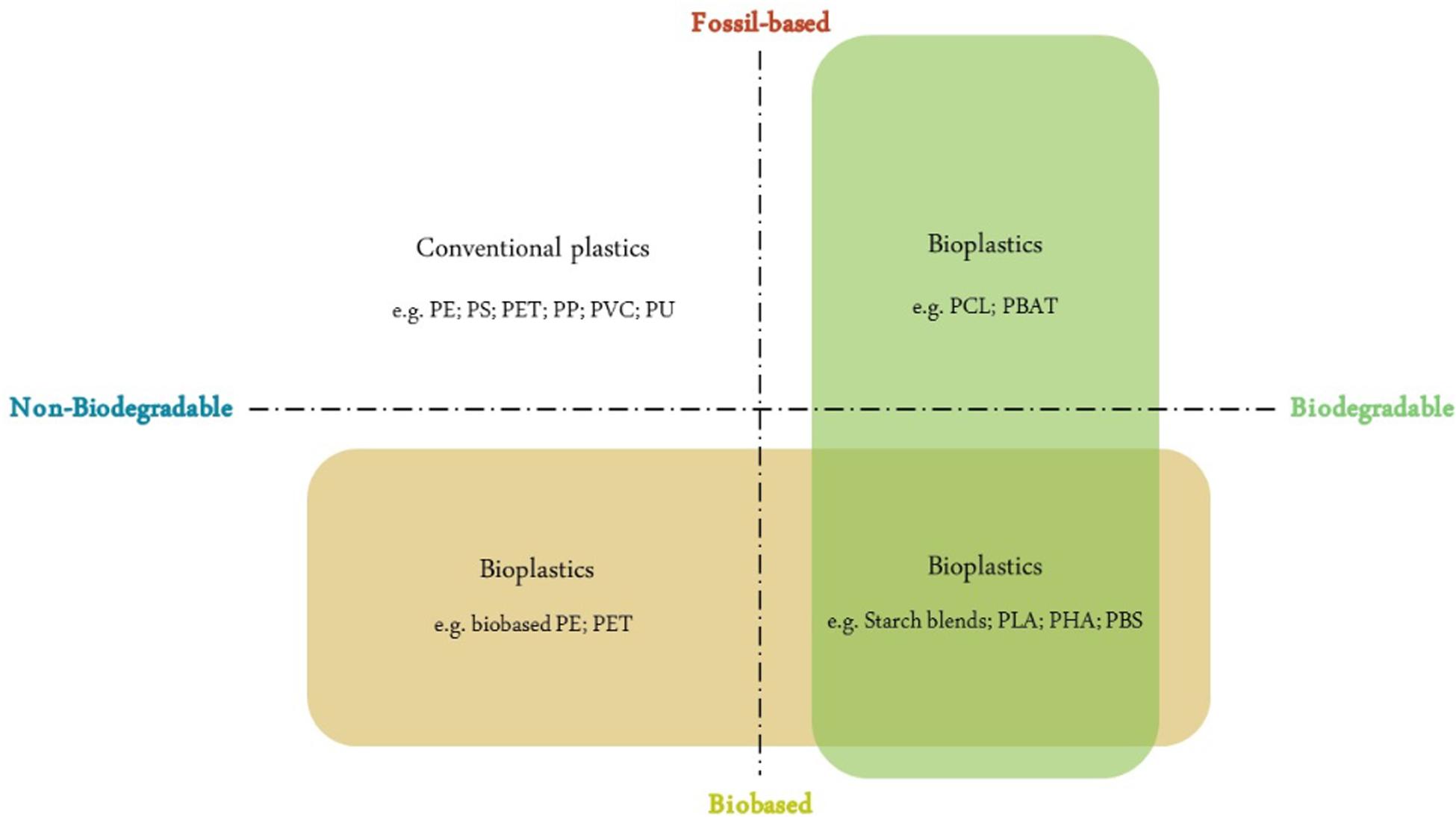
Figure 7. Degradable and non-degradable relations of fossil-based and biobased plastics types. PE, polyethylene; PS, polystyrene; PET, polyethylene terephthalate; PP, polypropylene; PVC, polyvinyl chloride; PU, polyurethane; PCL, polycaprolactone; PBAT, polybutyrate adipate terephthalate; PLA, polylactic acid; PHA, polyhydroxyalkanoates; PBS, polybutylene succinate.
Biodegradation is influenced by the plastic chemical structure, and it is not related to the origin of the plastic material. Thus, biobased plastics are not necessarily biodegradable, and some fossil-based plastics might be susceptible to biodegradation (available at https://www.european-bioplastics.org/bioplastics/, accessed at 29/05/2020). For instance, the molecular structures of biobased PE and biobased PET are similar to those of their fuel-based counterparts, but their production involves a smaller carbon footprint, since these polymers derive from renewable feedstocks. Yet, they are still non-biodegradable (Lambert and Wagner, 2017). On the other hand, polybutylene succinate (PBS) and polybutylene adipate-co-terephthalate (PBAT) are petroleum-based plastics with molecular structures that make them susceptible to biodegradation (available at http://en.europeanbioplastics.org/bioplastics/, accessed at 29/05/2020).
Bioplastics must have similar properties to the fuel-based plastics to be considered as suitable alternatives for traditional plastics. These include mechanical, thermal, and optical characteristics, and also water and/or gas barrier features, depending on the specific applications (Cooper, 2013). Moreover, the bioplastics with the highest potential for becoming sustainable alternatives to conventional plastics are both biobased and biodegradable. Their production contributes to reducing the dependency on limited fossil resources and the greenhouse gas (GHG) emissions but also contributes to increasing resource efficiency through a closed resource cycle, in a circular economy system. The polymers included in this group that are currently considered leading options to mitigate the environmental impact of petroleum-based plastics are starch, polylactic acid (PLA) and polyhydroxyalkanoates (PHA).
Starch
Starch is a highly abundant and readily available polysaccharide that can be easily extracted from tapioca, potato, and corn (Ferreira et al., 2016). Native starch polymers are generally difficult to process since they are brittle and absorb moisture. Thus, they are normally used with the addition of plasticizers or blended with other polymers, which can compromise their biodegradability (Sudesh and Iwata, 2008; Jabeen et al., 2015; Lambert and Wagner, 2017). Nonetheless, the resulting thermoplastic materials have been successfully used in some industries, namely in food, pharmaceutical and medical applications (Ferreira et al., 2016).
Polylactic Acid (PLA)
Polylactic acid (PLA) is a aliphatic polyester that can be produced by fermentative biotechnological processes using agricultural products as feedstock, namely, corn, potato, wheat, and sugarcane (Qi et al., 2017). The biobased lactic acid monomers resulting from the fermentation process are chemically polymerized either by direct polycondensation or ring-opening polymerization (Prathipa et al., 2018).
Polylactic acid presents mechanical, optical, and barrier properties comparable to those of PP, PET, and PS and has potential applications varying from packaging to medical applications, like surgical sutures, because it is hydrolyzable into soluble oligomers in the human body (Jarerat et al., 2003). Despite not being readily biodegradable, PLA is recyclable and is biodegradable under industrial composting conditions (mixed microbial community in aerobic conditions, with a temperature higher than 55°C and a relative humidity higher than 65%). However, the full potential of this polymer will only be attained with the development of adequate infrastructures for sorting, recycling, and composting PLA plastics (Tokiwa et al., 2009; Castro-Aguirre et al., 2016; Qi et al., 2017).
Polyhydroxyalkanoates (PHA)
Polyhydroxyalkanotes (PHA) are chemically diverse biobased, biodegradable, and biocompatible aliphatic polyesters of microbial origin with high potential for replacing synthetic plastics in applications ranging from packaging to pharmaceutical and medical products. Although over 150 hydroxyalkanoate monomers and 300 PHA-producing bacterial species have been identified (Reis et al., 2011), the most relevant polymers of the PHA family are poly(3-hydroxybutyrate), poly(3-hydroxybutyrate-co-3-valerate), and poly(3-hydroxybutyrate-co-3-hydroxyhexanoate) and they are already commercially available (Cooper, 2013). The monomeric composition and consequent characteristics of PHA polymers depend on the microorganisms involved in their production and on the type of carbon sources available.
When compared to PLA and starch-based plastics, PHA exhibit a wider variability in material properties due to the range of possible monomeric compositions, structures, and molecular weight distributions (Keshavarz and Roy, 2010). In addition, while PLA is only biodegradable under specific conditions of industrial composting, PHA has the advantage of being industrially and home compostable, anaerobically digestible, and biodegradable in soil and marine environments. In fact, PHA are easily biodegradable by several bacteria that produce extracellular PHA depolymerases (Cooper, 2013; Lambert and Wagner, 2017) and there is enough evidence that PHA are prone to complete and fast biodegradation in natural environments (Meereboer et al., 2020). Under the appropriate sample morphology and environmental conditions (temperature, moisture, microorganisms, nutrients, salinity, and others), PHA can be totally degraded within 28 days in fresh and seawater environments, namely rivers, lakes, or oceans (Meereboer et al., 2020).
Polyhydroxyalkanoates are produced as internal carbon storage and energy reserve by several bacterial strains. Current commercial PHA production processes are based on expensive organic sources and pure microbial cultures (PMC) under sterile conditions and fed batch feeding strategy. The culture is supplied with growth media and when the appropriate cell density is reached, growth-limiting conditions are imposed to induce PHA storage (Keshavarz and Roy, 2010; Reis et al., 2011). This strategy results in high cell densities and productivities but entails high costs at industrial scale (Rodriguez-Perez et al., 2018).
Over the last two decades, efforts have been made in the development of alternative processes with reduced costs. Using inexpensive waste organic carbon as feedstock for PHA production by mixed microbial cultures (MMC) in non-sterilized conditions is a potential cost-efficient method compared to PMC systems, reducing substrate and operational costs and producing copolymers with multiple applications (Reis et al., 2011; Mannina et al., 2020). In MMC systems operated in non-sterile conditions, a selective pressure must be applied to favor the microorganisms with high PHA storage capacity. These processes are normally operated in three-stages (Figure 8). The first stage corresponds to the acidogenic fermentation of the waste organic carbon to produce volatile fatty acids (VFA) that are used for PHA synthesis and is normally carried out in a continuous upflow anaerobic sludge blanket (UASB) reactor. The second stage is the selection of the MMC, where the microbial culture is enriched in PHA-accumulating bacteria. The selection normally takes place in a sequencing batch reactor (SBR) fed with the VFA-rich stream produced in the first stage and operated under aerobic dynamic feeding (ADF), i.e., with short periods of substrate abundance (feast) followed by long periods of substrate absence (famine). Under these conditions, PHA-accumulating organisms have a competitive advantage since they can use internal carbon reserves and external nitrogen for growth and maintenance during the famine phase. The last stage is PHA accumulation where the enriched MMC of the selection stage is fed with the VFA-rich stream from the first stage in the absence of nutrients so that the carbon is mainly used for PHA storage rather than for growth (Albuquerque et al., 2007). This stage is commonly operated in fed batch mode with pulse-wise substrate feeding whenever the carbon source from the previous pulse is depleted. In general, when compared to PMC, the overall PHA productivities using MMC are still significantly lower, but the production costs are reduced and the use of waste streams as substrate significantly contributes to the reduction of their polluting load (Reis et al., 2011).
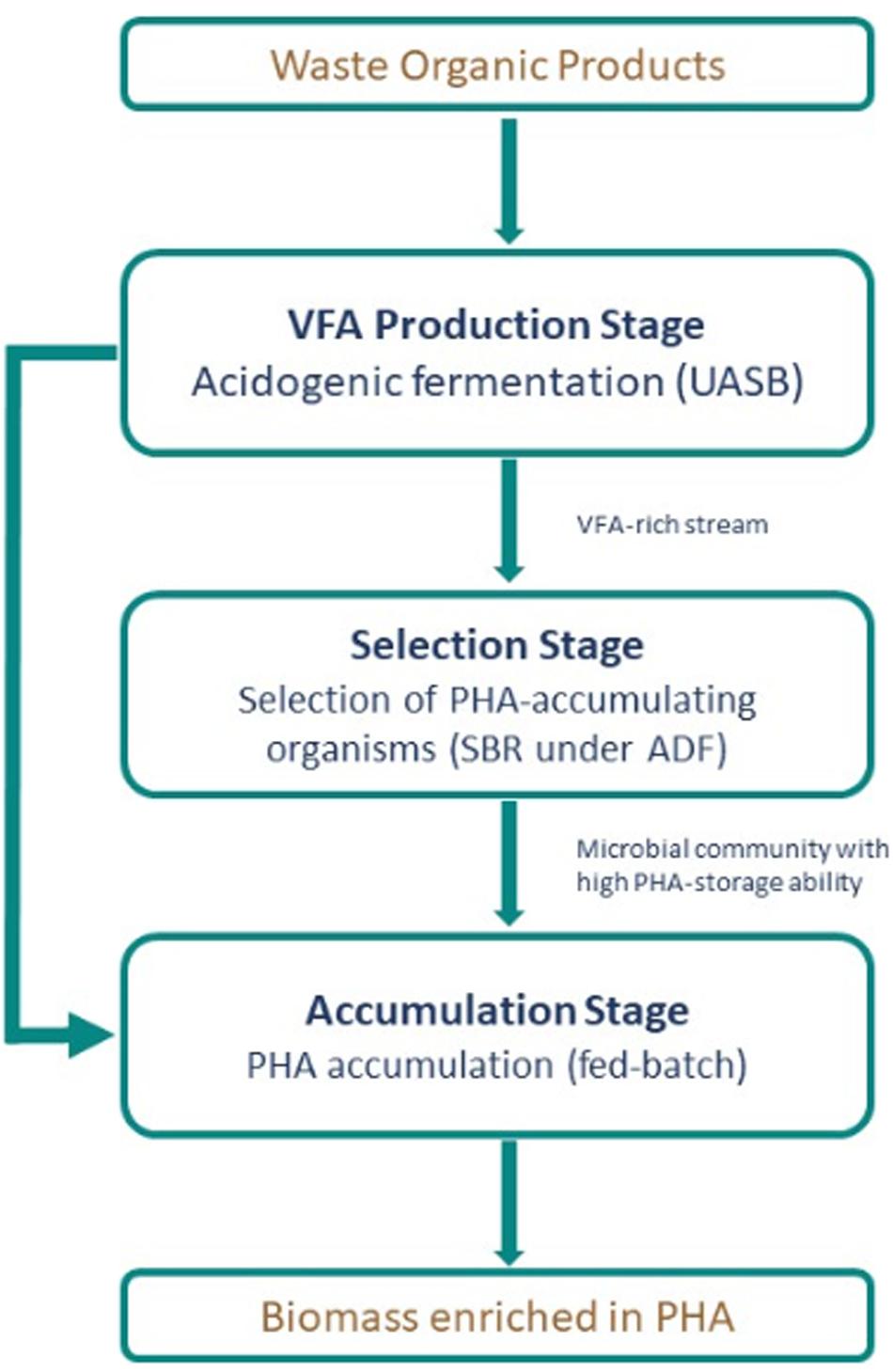
Figure 8. Schematic representation of the PHA production process by mixed microbial cultures, using waste organic carbon as feedstock. VFA, volatile fatty acids; UASB, upflow anaerobic sludge blanket; SBR, sequencing batch reactor; ADF, aerobic dynamic feeding.
Both MMC and PMC for PHA production have advantages and drawbacks, and the choice depends on several factors, including specificity/versatility in the use of substrates, type of PHA produced, and final application. Moreover, to become a competitive alternative to synthetic plastics (Reis et al., 2011; Yadav et al., 2020), PHA production from PMC or MMC still requires optimization and cost reduction, including the downstream processing (recovery and purification), which can have a significant impact on the cost and ecological footprint of the entire process (Yadav et al., 2020).
Bioplastics Market
The world plastic production has been rising considerably in the past 30 years, reaching almost 360 million tons in 2018, where European plastic industry accounts for 62 million tons with a turnover of 360 billion euros (Guzik et al., 2014). Currently, bioplastics represent less than 1% of the annual plastic production, but the market is continuously growing and the global bioplastic production capacity is expected to increase by approximately 15% in 5 years, from around 2.11 million tons in 2019 to 2.43 million tons in 2024 (available at http://en.europeanbioplastics.org/bioplastics/, accessed at 29/05/2020). From the 2.11 million tons produced in 2019, the biggest fraction of biobased polymers corresponded to bio-PE (11.8%) and the biggest fraction of biodegradable polymers corresponded to starch blends (21.3%) (Figure 9). Biobased, non-biodegradable plastics altogether currently represent over 44% (almost 1 million tons) of the global bioplastics production capacities. Biodegradable plastics, including PLA, PHA, starch blends, and others, account for over 55.5% (over 1 million tons) of the global bioplastics production capacities (available at http://en.europeanbioplastics.org/bioplastics/, accessed at 29/05/2020).
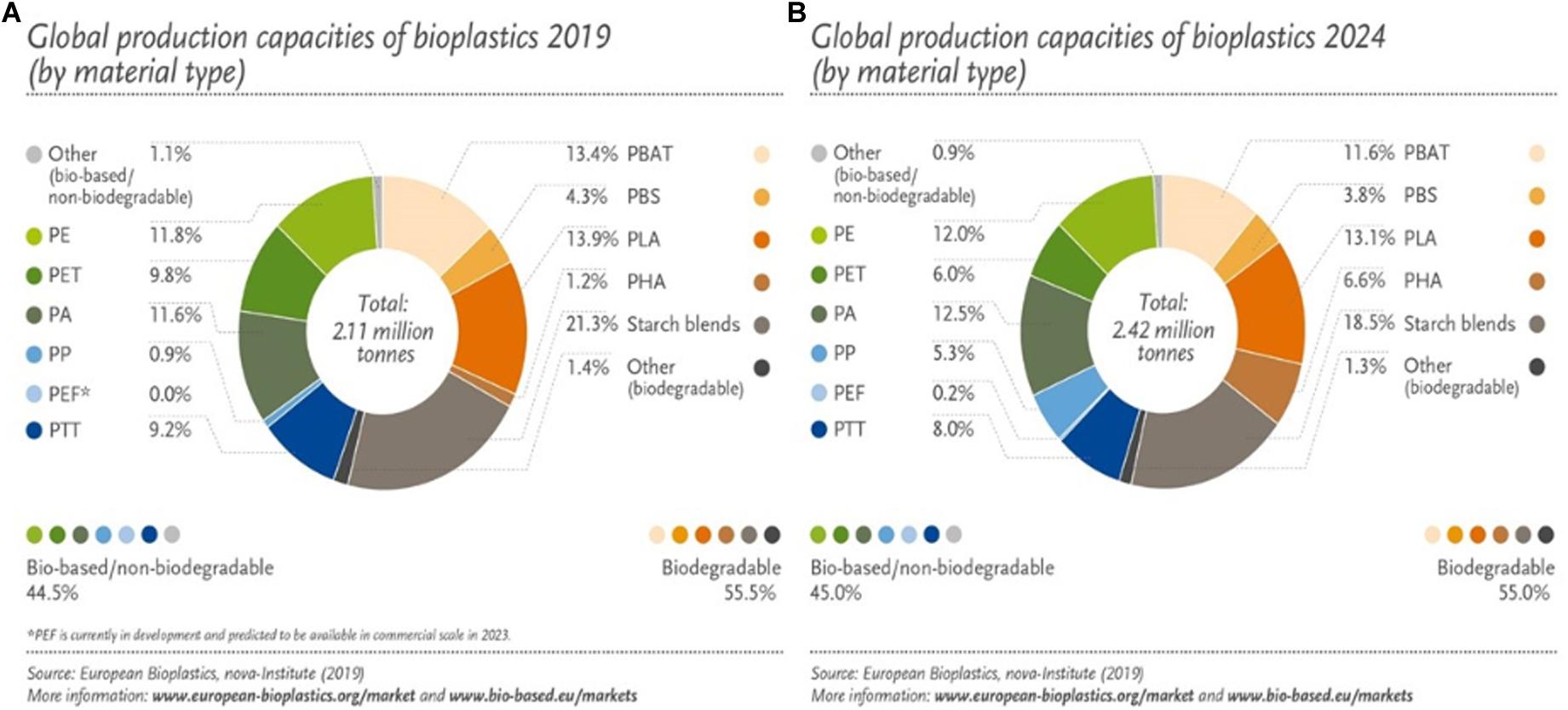
Figure 9. Global production of bioplastics. (A) In 2019, the higher fraction of biobased polymers and biodegradable polymers corresponds to bio-PE and starch blends, respectively. (B) In 2024, the production of biodegradable polymers is expected to increase, mainly due to the significant growth rates of PHA production. PA, polyamide; PEF, polyethylene 2,5-furandicarboxylate; PTT, polytrimethylene terephthalate. Reproduced with permission. Copyright 2017, European Bioplastics, available at https://www.european-bioplastics.org/market/.
PHA still represent a minor share of the global bioplastics production capacity; however, their production capacities are expected to grow from 1.2% in 2019 to 6.6% in 2024 (Figure 9). The production of biodegradable plastics is expected to reach 1.33 million tons in 2024, mainly due to the significant growth rates of PHA production.
Having circular economy in mind, the discovery of plastic-degrading bacteria that simultaneously produce PLA or PHA would constitute a great advance in this field, as we could use microplastic in their feedstock as carbon source for the production of bioplastics, not only creating added valuable for microplastics but also mitigating the plastic pollution challenge. Marine-derived bacteria can play an important role in this achievement because these bacteria grow in seawater media and produce different enzymes than their terrestrial counterparts.
Chemical Methods for Plastic Recycling Valorization
In general, the main methods for plastic waste reduction/elimination are thermal and chemical degradation, photodegradation, oxidative degradation, and biodegradation (Haider et al., 2019; Anju et al., 2020). Mechanical recycling and energy recycling (recovery) are often employed. Yet, these are not efficient enough to deal with the plastic pollution problem; alternatively, chemical decomposition can be applied to depolymerize and repolymerize plastic material in a sustainable number of cycles (Jehanno et al., 2019). Several recent reviews on this topic have been published, and they focus on characteristic plastic transformation routes, such as thermochemical routes (Lopez et al., 2017; Uzoejinwa et al., 2018), carbonization route (Chen et al., 2020), petrochemical routes and hydrocracking (Thybaut and Marin, 2016; Hassan et al., 2019), production of hydrogen and nanotubes (Sharma and Batra, 2020), bioconversion by immobilized enzymes (Bilal and Iqbal, 2019), plastic gasification (Lopez et al., 2018), and catalytic pyrolysis (Qiu et al., 2018). In addition to these reviews, the aim of this section is to evaluate recent literature focusing on chemical methods complementary to biodegradation and applied (or applicable) to some of the major plastic pollutants. We decided to limit on the polymers in higher demand: polyethylene (PE), polyethylene terephthalate (PET), polystyrene (PS), and polylactic acid (PLA). The methods for chemically degrading these polymers are described below and are summarized in Table 5, highlighting their experimental conditions, reaction yields, and products.
Polyethylene (PE) Degradation
Polyethylene is inert to many chemicals, and it is available in multiple types (HDPE, LDPE), which complicates the recycling processes. In fact, PE is the most challenging polymer for recycling and therefore is a waste stream (Bäckström et al., 2017). Its backbone is extremely stable, connected by strong single C–C and C–H bonds containing mainly secondary and primary carbons, both robust to oxidation by exposure to heat or ultraviolet radiation (Jia et al., 2016). Most studies on PE recycling are oxidative degradation and pyrolysis (thermal or catalytic) (Table 5).
Commercial polymers such as PE and PP are susceptible to photo- and thermo-oxidation and undergo chain scission with reduction in their molar mass (Anju et al., 2020). Thus, the main used methods for the degradation of PE are the oxidative methods. Oxidation occurs in the presence of abiotic factors, such as UV radiation, oxygen, temperature and chemical oxidants (Wei and Zimmermann, 2017).
The behavior of polyethylene has been studied by photo-oxidation with λ ≥ 300 nm light and by thermo-oxidation at a temperature of 100°C (Gardette et al., 2013). Photo-oxidation, similarly to thermo-oxidation, involves a chain of reactions and is generally divided into three steps: initiation, propagation, and termination (Figure 10A; Singh and Sharma, 2008). In the initiation step, chemical bonds in the main polymer chain are broken, and oxygen-mediated disintegration of polymers liberates free radicals (Gewert et al., 2015; Anju et al., 2020). In the propagation phase, the polymer radical reacts with oxygen and forms a peroxy radical and this leads to the scission of long polymer chains into shorter chains (Singh and Sharma, 2008; Gewert et al., 2015). The autoxidation occurs by complex radical reactions, random chain scission, and cross-linking and leads to the formation of oxygenated fragments of low molecular weight oxygenated fragments, such as aliphatic carboxylic acids, alcohols, aldehydes, and ketones (Singh and Sharma, 2008; Lyu and Untereker, 2009; Anju et al., 2020). The termination of the radical reaction occurs when free radicals react with themselves leading to the decrease of free radicals and to the formation of inert products (Lyu and Untereker, 2009; Gewert et al., 2015). Olefins, carboxylic acids, aldehydes, and ketones are the products expected at the end of radical reactions (Figure 10A).
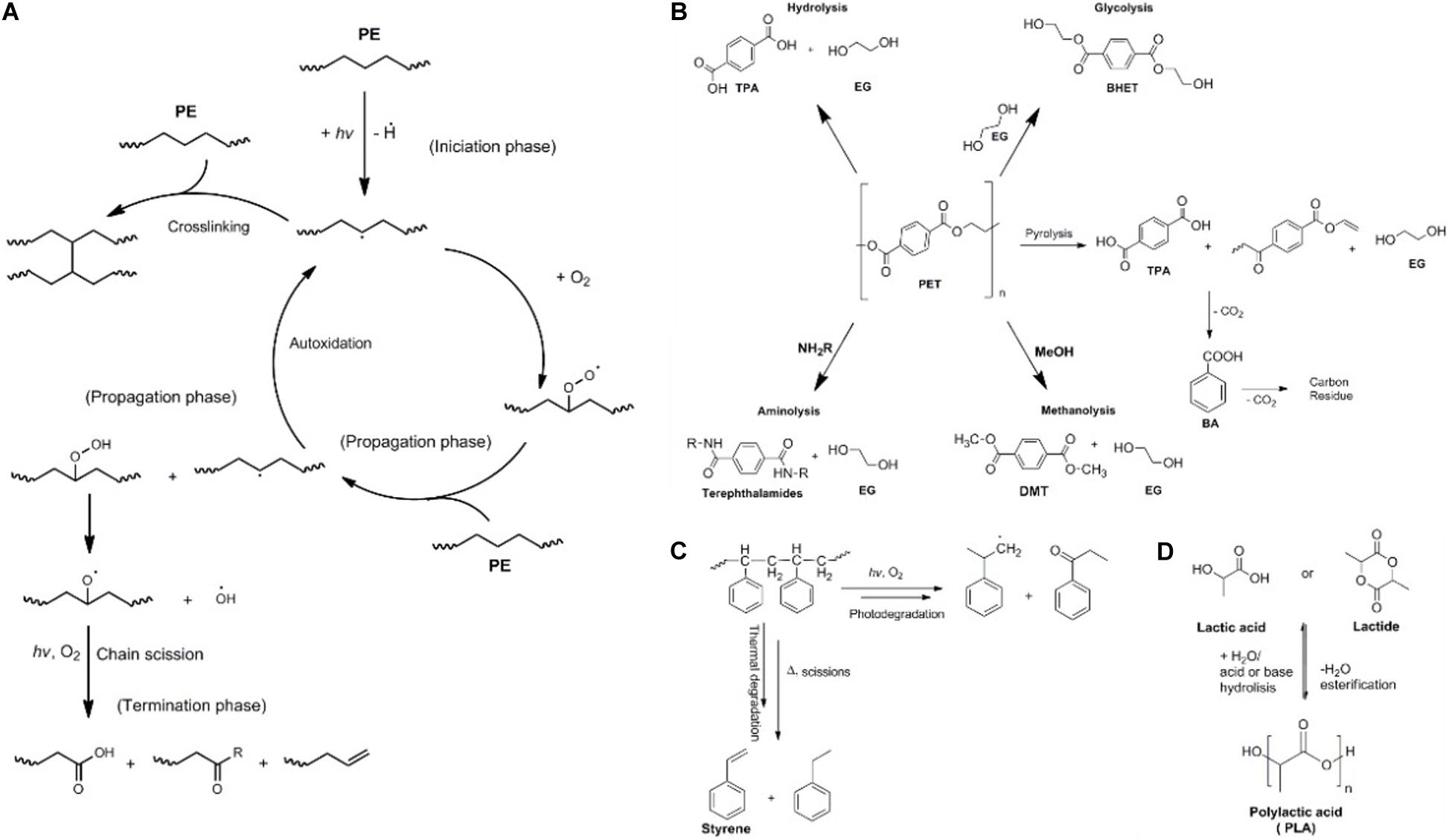
Figure 10. Chemical recycling degradation reactions. (A) Photo-oxidation degradation of polyethylene. (B) Degradation of polyethylene terephthalate by pyrolysis and other methods. (C) Photo and thermal degradation of polystyrene. (D) Hydrolytic polylactic acid degradation.
In addition to degradative oxidation, other methods such as thermal (T > 400°C) and catalytic pyrolysis have also been applied to PE degradation (Serrano et al., 2012).
The other alternative is to use high-pressure pyrolysis conditions that can change the product component distribution, producing aromatic compounds, isoparaffins, and more cycloalkanes and fewer olefins in the liquid product (Cheng et al., 2020). Another alternative for PE degradation is chemical depolymerization (Jehanno et al., 2019). However, due to the stable C–C backbone bonds of PE, severe conditions are necessary to allow and control the depolymerization reaction.
In an attempt to depolymerize polyethylene (LDPE) by microwave-assisted and oxidative process (1 h of microwave irradiation at 180°C in nitric acid solution 0.1 g/mL), the LDPE was totally degraded. Different kinds of products were obtained, such as succinic, glutaric, and adipic acids, as well as longer dicarboxylic acids, acetic acid, and propionic acid (Bäckström et al., 2017; Jehanno et al., 2019). In addition to the mentioned alternatives, some studies have explored the use of PE as a precursor in the production of carbon fiber through carbonization (Laycock et al., 2020).
The reactions for degradation of PE are listed in Table 5. It is important to note the broad diversity of reaction conditions and formed products. Overall, reaction time varies from 1 h to 400 h and the reaction yield from 71 to 95.5%. If the goal is to transform PE into fuel, catalytic pyrolysis meets the best advantages. If elimination of PE as a pollutant is the goal, then oxidation or carbonization can provide a much better solution.
The limitation of thermal and oxidative degradation and pyrolysis methods is the lack of product control, resulting in the formation of complex product compositions, including hydrocarbon gas, oil, wax, and char (Jia et al., 2016). Of these, catalytic pyrolysis is advantageous, as it uses a catalyst to reduce the temperature and reaction time, increasing the selectivity and economic viability. High-pressure pyrolysis has the advantage of generating products with characteristics that are closer to the standard fuel, such as isoparaffins, as well as higher amount of cycloalkanes and fewer olefins in the liquid product. On the other hand, this method requires severe conditions.
Microwave irradiation methods accelerate the chemical reactions, thus shortening the reaction times and decreasing the temperature, attaining high chemo-selectivity and product yields.
Polyethylene Terephthalate (PET) Degradation Methods
The most commonly used degradation methods for PET are thermal or oxidative degradation (Venkatachalam et al., 2012) and chemical degradation, such as hydrolysis, methanolysis, glycolysis, and aminolysis (Table 5). Among the chemical degradation processes, glycolysis is the preferred method for recycling PET (Khoonkari et al., 2015; Zanela et al., 2018).
Thermal Degradation/Pyrolysis of PET
Pyrolysis (catalytic and thermal) is a low-cost process that cleaves various chemical bonds in polymers and additives using high temperatures in the absence of oxygen, avoiding the use of solvents. The PET pyrolysis results in terephthalic acid (TPA), benzoic acid (BA), and carbon residues (Kumagai and Yoshioka, 2016; Figure 10B). While thermal pyrolysis is not very selective, catalytic pyrolysis is used to increase selectivity (Almeida and De Fátima Marques, 2016). Catalysts, such as zeolites which are microporous crystalline aluminosilicates (Almeida and De Fátima Marques, 2016), sulfated zirconia (Diaz-Silvarrey et al., 2018), or CaO/steam (Kumagai and Yoshioka, 2016), are employed to break the PET structure and increase the cracking selectivity to obtain other products or purer products. Generally, the level of catalytic activity increases by increasing the number of acidic sites of the catalysts (Brønsted or Lewis acids). They facilitate the thermal decomposition by proton donation (Brønsted) or electron acceptance (Lewis) creating active chemical species that further crack into smaller molecules. The formation of purer compounds is thus facilitated via carbocations of intermediate compounds (Almeida and De Fátima Marques, 2016). Other ways have been studied to improve pyrolysis, for example, controlling the end temperature and heating rate, typically known as “slow pyrolysis” (Dhahak et al., 2019). In methanolic pyrolysis, adding methanol as solvent under microwave irradiation reduces the overall reaction time and provides a high degree of depolymerization (Siddiqui et al., 2012).
Pyrolysis is rarely used to depolymerize PET at an industrial scale, as it produces gaseous and liquid side-products, which reduces the efficiency of the process and implies costly separation steps. However, this process has flexibility, as it is possible to vary the liquid/gas ratios of the desired end products, by operating temperature and pressure parameters. The pyrolysis major output is a combination of liquid and gaseous fuels (Table 5); whereas liquid fuel has various applications and great economic value (e.g., immediate use in turbines, furnaces, among others), the gaseous fuel can provide energy input for the overall process, to compensate the consumption of energy.
As an advantage, it is possible to treat different mixed plastics types, mitigating the contaminants negative impacts caused by several organic, inorganic, or biological residues in organic waste.
Pyrolysis presents a contrasting advantage over high-temperature incineration, since the last one generates and emits toxic and greenhouse gases. Moreover, it forms a closed recycling loop because of the production of usable side products. However, it still needs a high-energy input (Raheem et al., 2019; Lee and Liew, 2020).
Thermo-Oxidative Degradation
The thermo oxidative protocol resembles pyrolysis with the simple difference that pyrolysis is done in the absence of oxygen, while thermo-oxidative protocol occurs in the presence of oxygen (Botelho et al., 2001). Initially, hydrogen peroxide is formed on the methylene groups, resulting in the formation of carbon and oxygen radicals along with vinyl ester end groups (Botelho et al., 2001; Romão et al., 2009; Kruse and Wagner, 2016). Formation of colored PET during thermo-oxidative degradation occurs due to the hydroxylation of the terephthalic ring and oxidation of the phenolic hydroxyl groups to quinonoid species. This process occurs especially in the presence of metallic catalysts used in the PET synthesis (Yang et al., 2010). Overall, the reaction time for this process ranges from 2 h to 670 h (Table 5). Despite the obtained added-value products, this method is not quantitative, as the product yields have not been determined.
Thermo-oxidative degradation disadvantage is the lack of selectivity, resulting in the formation of complex product mixtures that lead to separation associated costs.
Hydrolytic Degradation
Hydrolytic degradation is a process that uses high temperature and pressure and only water as a solvent. It is a low-cost degradation process resulting in terephthalic acid (TPA) and ethylene glycol (EG) (Sinha et al., 2010; Valh et al., 2019), as shown in Figure 10B.
There are three hydrolytic processes: (1) acid hydrolysis, digestion of PET waste with water in acidic conditions for 96 h (Table 5), using strong acids (e.g., H2SO4) to depolymerize PET with 80% yield. This method has the advantage of not requiring high temperature (25–100°C) and pressure (∼ 0.101 MPa) and is a fast process. However, it presents a major disadvantage, and the amount of acid needed to industrialize this method poses economic, process, and environmental problems. (2) Alkaline hydrolysis occurs in the presence of esterification catalysts, alkaline (NaOH), at a temperature range of 200–250°C, under pressure (1.4–2 MPa) for 3–5 h with 93% yield (Table 5), to produce sodium salt of TPA, from which TPA is precipitated by acidification (H2SO4) (Sinha et al., 2010; Zanela et al., 2018). Alkaline hydrolysis can treat PET contaminated with other materials but requires catalyst waste management as the used acid and base are environmental threats. (3) Neutral hydrolysis uses water or steam as reagents, and the reaction occurs at temperature range 200–256°C and pressure range 1–4 MPa, for 2 h with 92% yield (Table 5). Neutral hydrolysis requires an extensive TPA purification, since the contaminants present in PET waste are carried over the product, which lowers the monomer’s purity. This hydrolysis does not produce inorganic salts and is eco-friendly. However, the major disadvantages of this process are the severe operation conditions (high temperature and pressure).
Biocatalysis is another successful alternative. The cutinases (polyester hydrolases) are more eco-friendly and less expensive with remarkable capability to hydrolyze PET (95%), but slower (48 h) (Table 5; Shirke et al., 2018).
Glycolysis
Glycolysis is the most favorable PET recycling method using depolymerization and re-polymerization. It is sustainable since the product of glycolysis—bis(2-hydroxyethyl)terephthalate (BHET)—can be polymerized to high-quality PET (Figure 10B; Jehanno et al., 2019). In addition, glycolysis is a simple process using EG as a solvent and reagent at high temperatures (180–240°C) (Khoonkari et al., 2015). Therefore, methods to improve glycolysis are being studied to provide a less costly process at lower temperatures. The glycolysis reactions for PET degradation are listed in Table 5.
A wide range of metal salts and Lewis acids were used as catalysts to carry out the PET glycolysis, in various conditions. They react with polymer carbonyl groups, facilitating PET degradation (Esquer and García, 2019). The best catalytic system in terms of activity and BHET yield was the use of CoCl2⋅6H2O as a cheap catalyst, compared with the already reported catalytic systems based on zinc acetate, since zinc proves to be a great catalyst to form BHET. However, it has some major problems in product separation and purification (Shukla and Kulkarni, 2002; Xi et al., 2005; Imran et al., 2013; Lopez et al., 2017). Noteworthily, the addition of BEt3 improved the BHET yield and reaction time by up to 84% yield in 3 h. Additionally, the use of an ancillary ligand can help to increase the activity, but a careful selection must be considered (Troev et al., 2003).
Organocatalysts, such as ionic liquids, deep eutectic solvents, and nitrogen bases, as well as organometallic catalysts, such as acetates and alkoxides, can also be successfully applied for the preparation of BHET (Yue et al., 2011; Jehanno et al., 2019). Therefore, imidazolium-based ionic liquids (based on 1-butyl-3-methylimidazolium cation [BMIM]) were first studied in PET glycolysis and hydrolysis, but it turned out that they mostly needed an additional (Lewis acid) catalyst. An exception was Lewis-based ionic liquid [BMIM][OAc] that provided BHT as a single reaction product in 58% yield, in 3 h at 190°C (Al-Sabagh et al., 2014). Urea-based ionic liquids (mixture of urea and choline chloride) also provided BHET in 190 min at 190°C, in 73.8% yield. The more basic tetramethylammonium alaninate [N1111][Ala] gave a similar monomer yield (74.3%), only faster, 50 min at 170°C (Wang et al., 2012). Choline phosphate[cholin]3[PO4] ionic liquid can be used for high loading of PET (10 wt %) at relatively low temperatures (120°C, 3 h) and under water-rich conditions. Further exploration showed that in the presence of EG, the [choline]3[PO4] catalyzed glycolysis of PET with approximately 100% conversion of PET and approximately 60.6% yield of BHET (Sun et al., 2018). Glycolysis appeared to be more efficient (i.e., faster with lower undesirable oligomers content) when strong bases, such as 1,5,7-triazabicyclo[4.4.0]dec-5-ene (TBD), 1,8-diazabicyclo[5.4.0]undec-7-ene (DBU), or 1,5-diazabicyclo[4.3.0]non-5-ene (DBN), were used compared to bases with a lower pKa, such as 1-methylimidazole (NMI) or dimethylaniline (DMA) (Fukushima et al., 2013a). However, an equimolar quantity of triazabicyclodecene (TBD) and methanesulfonic acid (MSA) (TBD-MSA) seems to be an even better system and completely depolymerizes PET in less than 2 h, producing 91% of highly pure BHET (Jehanno et al., 2018). This novel catalyst showed very stable protic properties (up to 400°C) and can be also successfully used for BHET polymerization to PET at 270°C and recycled at least 5 times to depolymerize more PET waste. Clearly the transesterification mechanism in organocatalysis can be more complex than the above-described mechanism (acid and base) and beneficial for overall PET degradation due to the bifunctional nature of TBD involving the formation of hydrogen-bonding interactions between the reactants and key hydrogen-bond donor and acceptor sites on the organocatalyst that facilitate nucleophilic attack and ester formation (Horn et al., 2012). Microwave heating became a famous process offering a minute gap of heating, leaving the material untouched with high specificity (Hoang et al., 2019).
Glycolysis is the most advanced PET chemical recycling method in terms of technology readiness, as it is already into polymer production lines. A challenge of this method is the immiscibility of PET with polyols and, consequently, the need for a purification step of the end products.
Methanolysis
The methanolysis in the process of PET degradation adds some advantages as methanol is employed both as reagent and as solvent at high temperature and pressure, resulting in dimethyl terephthalate (DMT) and EG (Figure 10B). Both DMT and EG are very useful products as they can be polymerized into PET again through glycolysis (Sinha et al., 2010; Siddiqui et al., 2012).
Methanolysis conversion rates can reach 99.8% in 2 h (Table 5), with purification of DMT (alternative feedstock) by distillation. The DMT is produced as a product with identical quality to the virgin DMT. In addition, EG and methanol can be recovered and recycled.
This process presents several disadvantages, such as high temperature and pressure conditions, being difficult to inject waste material into a pressurized reactor. Moreover, purification of the final products is necessary (crude products contain alcohols and phthalate derivates), increasing the energy consumption and costs (Lee and Liew, 2020). Nevertheless, product separation can be improved by other processes (Sinha et al., 2010) such as working under supercritical conditions (300–350°C and 20 MPa) (Goto et al., 2002; Yang et al., 2002; Liu et al., 2015), using vapor methanolysis for easier separation of methanol vapor and products (Kim et al., 2008; Pudack et al., 2020), applying catalysts like zinc acetate or manganese salts (Kurokawa et al., 2003; Mishra and Goje, 2003; Siddiqui et al., 2012), or shortening the reaction time under microwave conditions (Siddiqui et al., 2012).
Aminolysis and Ammonolysis
Aminolysis is a new industrial process using temperatures between 20 and 100°C, under atmospheric pressure, and several amines, resulting in terephthalamides in very pure forms and EG in high yields 85–95%, in 0.5 to 3 h (Table 5; George and Kurian, 2016; Musale and Shukla, 2016). This method is more thermodynamically favorable than methanolysis (Figure 10B; Fukushima et al., 2013b; Musale and Shukla, 2016). The most employed amines are methylamine, ethylamine, and butylamine (Sinha et al., 2010). However, triethylamine provided the highest products yield of TPA and EG.
The products of PET aminolysis are used to produce, for example, polyurethanes (PU) (Shamsi et al., 2009; Sadeghi et al., 2011) or other polyesters (Musale and Shukla, 2016), employing catalysts, such as organic catalysts, amides, sodium acetate, or potassium sulfate. This variety of end products widens the potential use range for aminolysis (Sinha et al., 2010; George and Kurian, 2016; Kárpáti et al., 2019).
The products of aminolysis are added-value products, due to their potential to undergo further reactions. However, this process is rarely used since amines are often toxic and expensive.
In comparison to other chemical recycling methods, ammonolysis has been less explored. The degradation process in ammonolysis is carried out by the action of ammonia to PET, using a suitable catalyst (e.g., zinc acetate), at a temperature range of 70–180°C and pressure of 2 MPa, for 1–7 h, yielding terephthaldiamide (TPA di-amide) and EG, in 87 to 90% yield (Table 5). TPA di-amide serves as an intermediate product that can be converted to p-xylylenediamine or 1,4-bis(amino-methyl)cyclohexane. Ammonolysis is much slower and expensive than aminolysis, as it requires higher temperature and pressure conditions and the use of a catalyst to increase the rate of degradation (Gupta and Bhandari, 2019).
Polystyrene Chemical Degradation (PS)
Thermal and catalytic degradation methods are mainly used for PS degradation; the most studied mechanisms are pyrolysis, catalytic cracking, and hydrocracking (Fuentes-Ordóñez et al., 2016; Nisar et al., 2019). Thus, polystyrene degradation can occur by breaking the C–C from the backbone generating free radicals as seen in pyrolysis (Ali et al., 2020), or through cracking, due to PS high selectivity to produce aromatic hydrocarbons, which can be employed in fuels (Fuentes-Ordóñez et al., 2016). The processes for degradation of PS are listed in Table 5, including their reaction conditions, with overall time ranging from 1.1 to 480 h and yielding from 82 to 93.5%.
The thermal photodegradation of PS undergoes a chain scission mechanism consisting of initiation, propagation, and termination processes for reduction in their molar mass (Fuentes-Ordóñez et al., 2016; Anju et al., 2020). This process is similar to that of PE (Figure 10C), where in the initiation reactions, the polymeric chain is broken into shorter chains (caused by breakage of weak bonds), followed by C–C bond breakage which generates free radicals. During propagation, hydrogen abstraction occurs between radical intermediate polymer chains, enabling degradation into smaller polymer chains. The termination step normally occurs with the recombination of free radicals and formation of styrene (Fuentes-Ordóñez et al., 2016; Ali et al., 2020).
In addition to the thermal degradation, PS can also undergo photo-oxidation based on the radical process for oxidation induced by UV light from the aromatic rings (Figure 10C; Xiao et al., 2020). The conversion of PS into styrene can be considered one of the most effective chemical recycling techniques (Uttaravalli et al., 2020).
The advantages of catalytic degradation (catalytic cracking and hydrocracking) over the thermal process are the lower-temperature reaction conditions and higher selectivity of the desired reactions (Uttaravalli et al., 2020). When considering expended polystyrene (EPS), this method offers a higher conversion rate and styrene yield over thermal degradation. Hydrocracking is the most advantageous of PS degradation processes, as it is simpler, unifying in a single step cracking and hydro-treatment methods, and provides higher product quality. This process allows for the saturation of olefins, transformation of paraffins into isoparaffins, promotion of ring opening, and aromatic hydrogenation, reactions that are important for fuel production (Hesse and White, 2004; Fuentes-Ordóñez et al., 2016).
Polylactic Acid Degradation (PLA)
Polylactic acid (PLA) is synthetically polymerized aliphatic polyester that can be decomposed to lactic acid by the cleavage of ester bonds (Figure 10D). These polymers are industrially biodegradable and can undergo chemical degradation (hydrolytic under high temperature and humidity), being easily susceptible to biological degradation (Cho et al., 2000; Delamarche et al., 2020). However, this limits their durability and long-term performance (Muthuraj et al., 2015). In abiotic hydrolysis the polymer matrix is converted into oligomers. In this step, the polymeric chain scission depends on the presence of water, which diffuses into the polymer which cleaves ester bonds, thus forming carboxyl and hydroxyl groups (Rodriguez et al., 2016). The methods for degradation of PLA are listed in Table 5, having overall time ranging from 10 to 60 days. However, the yields have not been reported.
Noteworthily, although PLA degradation can also occur under anaerobic conditions, this only occurs at temperatures higher than those normally found in the natural environment (≥230°C) (Kopinke et al., 1996; Chamas et al., 2020). Under anaerobic biological conditions typical of a landfill, the PLA does not undergo direct biological degradation and the hydrolysis of the PLA is slow; it is likely that the degradation of PLA in a landfill requires a chemical hydrolysis step before any biodegradation can occur (Kolstad et al., 2012). In addition, the hydrolysis of PLA is highly dependent on the temperature and pH of the media. Thus, the hydrolysis process is strongly accelerated under alkaline conditions and high temperatures (55–60°C) (Schliecker et al., 2003; Su et al., 2019; Delamarche et al., 2020).
Polylactic acid is an interesting alternative to petrochemical-based polyesters due to the significant rates of degradation under industrial composting conditions (>60°C, in the presence of O2 and moisture) (Musioł et al., 2016; Chamas et al., 2020).
Finally, although degradation by hydrolysis is the most used method for the degradation of PLA, some works explore photo- and thermo-oxidative processes (Lu and Xu, 2009; Harris and Lee, 2013; Muthuraj et al., 2015; Siracusa et al., 2015) to evaluate the durability behaviors of possible plastic packaging prepared from these polymers.
Depolymerization of PLA to produce lactic acid is less energy consuming than producing lactic acid by glucose fermentation. PLA chemical recycling represents a solution for the end-life products since the acid lactic monomers can be used as raw materials.
Polylactic acid thermal depolymerization is a complex and expensive process, as it requires severe operating conditions (e.g., high temperature) and purification steps of the final products. Recycling PLA by hydrolysis is a simpler, more economic, and environment-friendly solution. Depolymerization by this method does not need the use of catalysts (hydrolysis is an autocatalytic reaction) or severe operating conditions. Moreover, it leads to the production of high-quality lactic acid, with the same properties of the virgin material, maintaining its utility and value (Piemonte et al., 2013; McKeown and Jones, 2020).
Polylactic acid chemical recycling generates a higher environmental footprint than PLA mechanical recycling. However, PLA resulting from mechanical recycling leads to products with highly inferior properties than the original product (Piemonte et al., 2013).
Plastics Recycling and Biodegradation Advantages and Disadvantages
Typically, plastics found in the ocean are made by different polymer types and not a single polymer. In the ocean, plastic bottles and disposable diapers, mainly composed by PET and PP, respectively, can take up to 450 years to degrade. Plastic beverage holders (PET and HDPE), styrofoam cups (PS), and plastic grocery bags (PE) can last up to 400, 50, and 20 years, respectively (Chamas et al., 2020). In general, plastic recycling saves energy, burns fewer fossil fuels, reduces CO2 in the atmosphere, and decreases other GHG emissions, which consequently reduces global warming.
One ton of recycled plastic saves 5,774 Kwh of energy, 16.3 barrels of oil, and 23 cubic meters of landfill space (available at https://www.epa.gov/warm, accessed at 13/10/2020). The energy to produce virgin HPDE, PP, PET, PS, and PVC varies from 63 to 105 MJ kg–1, and their price ranges from 1.4 to 2.1 $ kg–1, while the energy to recycle these polymers varies from 40 to 64 MJ kg–1 and the price of the recycled polymers ranges from 0.75 to 1.2 $ kg–1 (Figure 11; Shenoy et al., 1983; Shenoy and Saini, 1996; Rasel and Sarkar, 2019).
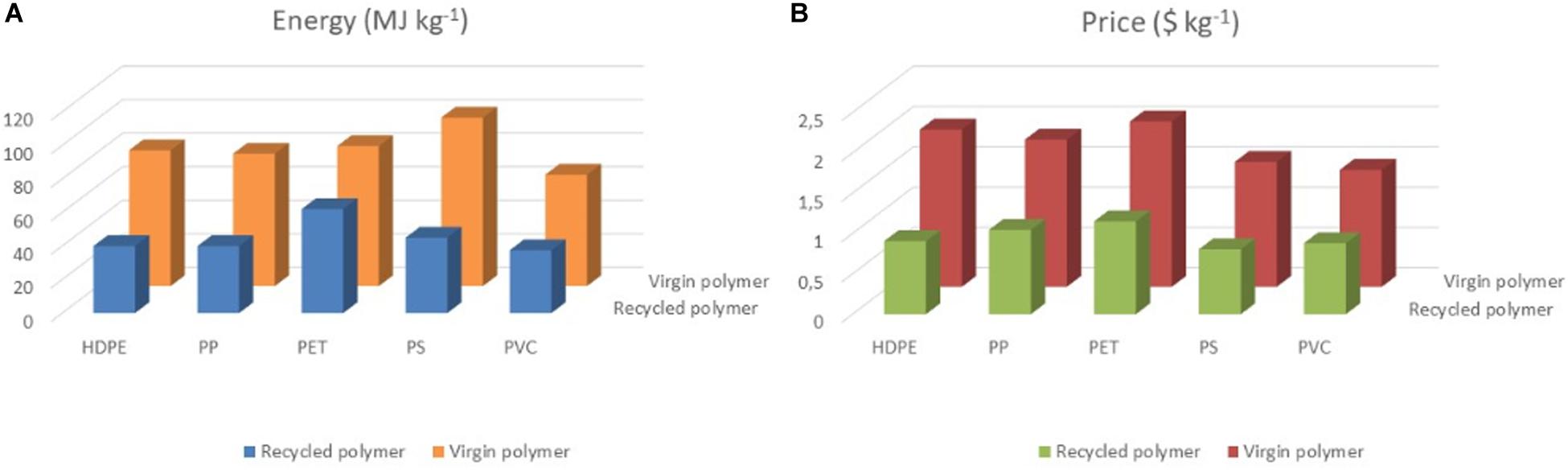
Figure 11. (A) Energies and (B) prices of virgin and recycled polymers. HDPE, high-density polyethylene; PP, polypropylene; PET, polyethylene terephthalate; PS, polystyrene; PVC, polyvinyl chloride.
In highly developed countries, the predominant method of dealing with polymer material waste is energy recycling, i.e., plastic combustion with energy recovery (Mierzwa-Hersztek et al., 2019). This method has serious drawbacks as many toxic and harmful substances, beside carbon dioxide, are created, such as hazardous dioxins, carbon monoxide, hydrogen cyanide, hydrogen chloride, phosgene, phosphine, nitric oxide and sulfur dioxide, phenol, and formaldehyde, depending also on the type of material. Therefore, the applicable regulations enforce the use of afterburner chambers to completely remove (burn out) these products. The use of afterburning gases reduces the energy efficiency of the process and significantly increases the cost (Mierzwa-Hersztek et al., 2019).
Mechanical recycling, i.e., processing plastic waste without changing the molecular framework to physically transform it for a different function, is another currently used recycling method, which is hindered by a high variety in plastic waste streams, decontamination costs, and production of low-value products (Thiounn and Smith, 2020).
Chemical recycling is the most advantageous of the recycling methods, as depolymerization can break polymers into their raw materials for reconversion into added-value polymers, thus contributing to the circular economy. For example, pyrolysis can turn mixed plastic waste into naphtha that can be cracked into petrochemicals (fuel, fine chemicals) and plastics. Fossil resources for chemical production can be replaced with recycled material from plastic waste, providing an environmentally friendly alternative. Pyrolysis of mixed plastic waste emits 50% less CO2 than incineration of the same waste (Miandad et al., 2019; Thiounn and Smith, 2020). Furthermore, chemical recycling reduces the amount of plastic waste which ends up in landfills or is incinerated, enabling the recycling of a wider range of plastic materials and the use of plastic waste mixtures, when compared with the traditional mechanical recycling. The major challenge of chemical recycling however is its cost, as it requires building infrastructures/plants, aggregates of large quantities of plastic waste, and requires high amounts of external heat and/or pressure and chemical agents. To make chemical recycling profitable it is essential to deploy/implement it at a large scale and worldwide. Chemical recycling also frequently produces toxic waste products and by-products. To mitigate this issue, we suggest a combination of two processes, e.g., chemical recycling and biodegradation, to improve the total outcome and eliminate or at least reduce some of the non-commercial waste products.
The natural process of degradation of plastics is affected by uncontrollable and unpredictable environmental factors, including abiotic and biotic conditions, and depends on the molecular weight, the polymer structure, and its physical properties. This slow process can be further slowed down when additives are added to the plastic polymers (Min et al., 2020). However, as plastic fragments might serve as new colonizing habitats for marine species with a metabolism that is adapted for an efficient plastic degradation, the degradation process can somewhat be accelerated. In marine environments, abiotic degradation and biodegradation occur simultaneously with a slow rate of polymer weight loss between 0.39 and 1.02% per month (Welden and Cowie, 2017). When the biodegradation process is optimized in laboratory conditions (either by using selected microbial cultures or by abiotic pretreatment), the degradation time and yield can be optimized. Overall, biodegradation time, under laboratory conditions, ranges from 0.25 to 7.5 months and the polymer biodegradation yield ranges from 0.8% to 100%, with PS being the harder to degrade and PLA the most biodegradable (Tables 2–4). The discovery of microorganisms and their metabolic pathways that can speed up the biodegradation process of plastics is an area of active research, but the technologies of the biodegradation solutions are not yet market-ready. Nevertheless, with increased financing resources, such as the Green Deal1 or Horizon Europe2, the technology readiness could increase from the current developmental stage to demonstration and implementation stages.
Bioplastic production is another field under active development. Biodegradable bioplastics have high environmental benefit as they totally degrade in short time. Nowadays, the development of biobased solutions for biodegradable bioplastic production enables the transition from the linear to circular economy (European Bioplastics, 2016). However, for the effective practical implementation of biobased plastic production, orchestrated efforts will have to be conducted from the scientific community (providing the process optimization), industry (investment and scaling-up), administrative authorities (providing legal background for production of these materials), and the general public (providing joint waste collection).
We provide a comparative overview of the current plastic degradation solutions in terms of their cost-effectiveness, degradation time, energy consumption, toxic emissions, and environmental benefit (Figure 12). Overall, biodegradable bioplastic production scored the highest in our comparison, making this technology the most promising and worth investing. Biodegradation also has environmental benefit, low toxic waste production and GHG emissions, and lower energy consumption in comparison with all the recycling methods. In sum, the biodegradation of plastic is a greener but slower process than chemical recycling, which takes only 1 to 670 h (Table 5), even for biodegradable bioplastic materials (Chinaglia et al., 2018; Chamas et al., 2020). Like chemical recycling, biodegradation and degradable bioplastic production methodologies have low technology readiness. Once these technologies are advanced, their cost-effectiveness will significantly improve.
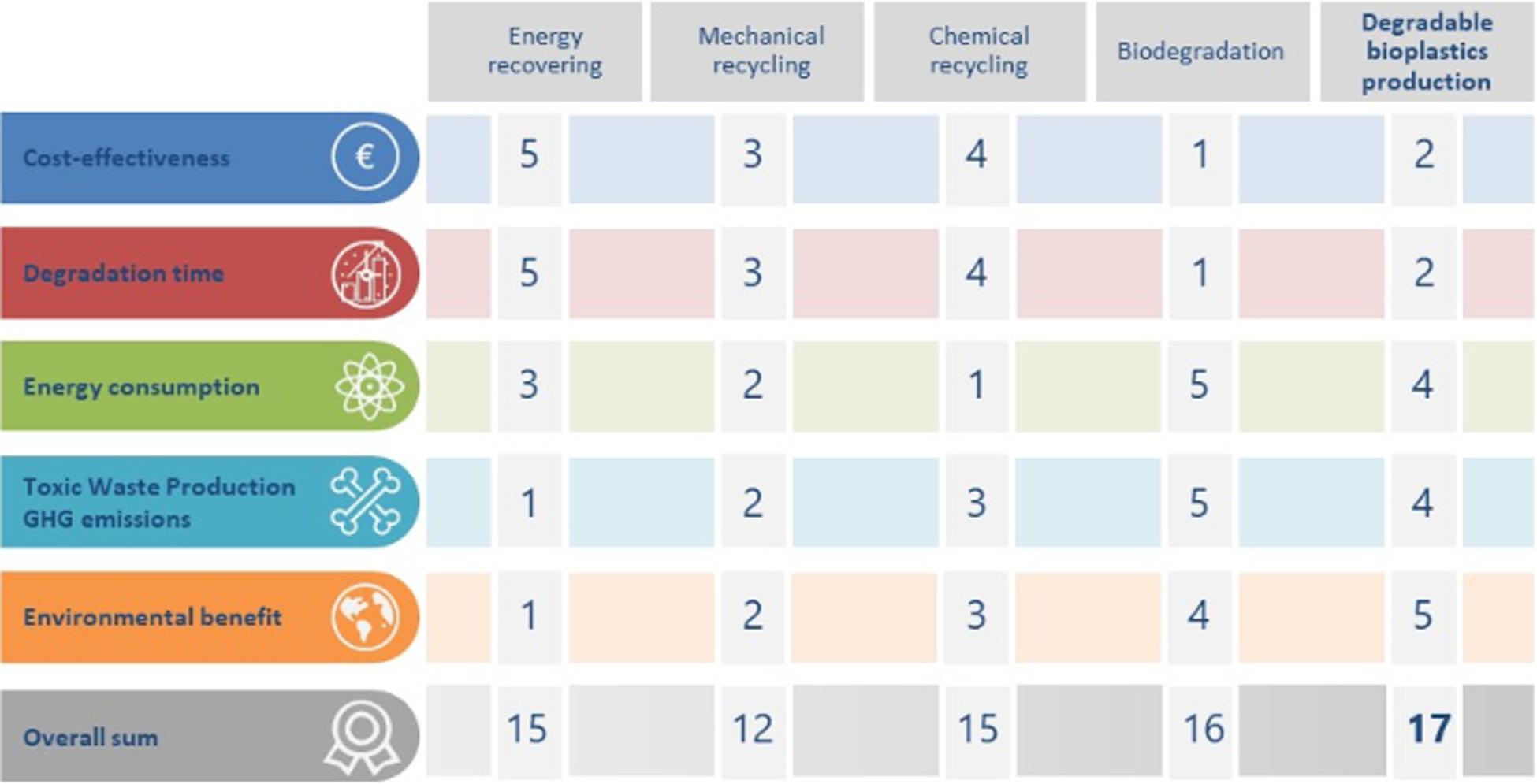
Figure 12. A comparative overview of the plastic recycling and biodegradation alternatives (columns). Each plastic reduction category (rows in the figure) was scored from 1 (the least efficient) to 5 (the most efficient). The last row sums the scores for each alternative, where the highest score obtained represents the best alternative. Note: this comparative overview takes into consideration the alternatives being used as standalone options. When two alternatives are combined (e.g., degradable bioplastic production and energy recovery), the overall score can be further increased. Moreover, when all the alternatives attain the same technology readiness, the overall score for chemical recycling, biodegradation and degradable bioplastic production can substantially increase.
The described biodegradation and chemical recycling valorization processes are not feasible for direct application into the ocean, as the use of microorganisms could create serious ecological imbalances and the use of chemical reagents and high temperatures is unviable. Industrial approaches using these methods would imply, as any other recycling method, the collection of plastic waste from both land and the ocean.
Biodegradable bioplastics are game changers. Of all the polymers described in the review, PHA are the only polymers that are fully degraded in the ocean in short time (∼1 month). Although biopolymer production costs are 3–5 times higher than chemical counterparts, research efforts on the discovery of 100% biodegradable biopolymers are our best hope to win the plastic pollution challenge.
Conclusion
The marine environmental pollution caused by plastics has major negative impacts on environment, society, health, and economy. Solutions and products for the mitigation of this problem are in high demand. Microorganisms are capable of degrading plastics/microplastics as well as producing bioplastics. Bacteria (including actinomycetes) and fungi are present in various ecological habitats, including marine environment, and have several distinct metabolisms, thus providing great prospects for biodegradation.
Plastics and microplastics can be efficiently incorporated in culture media as a sole carbon source. Pretreatment of microplastics with nitric acid and UV radiation proved to enhance their biodegradation. It is also possible to effectively improve the biodegradability of polymers by blending them with natural biodegradable polymers, like PLA, or by mixing them with pro-oxidants. There are microbial species more adapted and capable of using polymers as carbon source than others; for example, Bacillus genera can easily adapt to different microplastic types, reaching satisfactory levels of biodegradation. Biofilm formation is a crucial step to starting biodegradation, since microorganisms colonize the polymer surface, use it as substrate, and create complex three-dimensional structures. In the case of PLA, these biopolymers are more rapidly decomposed, mainly by actinomycetes, which corroborates PLA’s biocompatibility and makes it a good alternative to traditional plastics.
FTIR spectroscopy, SEM analyses, and weight loss are the most used biodegradation detection methods. FTIR and SEM are the most informative, while the weight loss method needs to be combined with other techniques to prove that biodegradation occurred.
The first eco-friendly alternatives to plastics produced from non-renewable resources are starting to arise. These are bioplastics from vegetal renewable feedstocks and from microorganisms. Different plant species can be used to produce takeaway containers, clothes, and shoes. Microbial PLA is starting to be used as a replacement of traditional plastic in some everyday use objects and in several biotechnological areas, including medical. PHA are a great promise to replace conventional plastics. However, the associated production costs still need to be reduced.
Despite all the positive scientific advances, it is still necessary to search for new sustainable, time-efficient, and cost-effective methods and improving existing ones to achieve optimal (micro)plastic degradation. For this ambitious goal, new microorganisms especially from marine ecosystems can be effectively studied, improving the targeted isolation of microorganisms, specific for plastic biodegradation and bioplastic production and inventing new cost-effective degradation methods that are faster than currently studied alternatives or biodegradation in nature. We foresee an increase of research efforts to find solutions and sustainable methods to decrease the pollution caused by plastics in the years to come.
Author Contributions
SG designed and edited the article concept. All authors drafted the manuscript and read, commented, improved, and approved the final version.
Funding
This work was supported by the Applied Molecular Biosciences Unit-UCIBIO (UID/Multi/04378/2020), which is financed by national funds from FCT/MCTES, and I3N CENIMAT (UID/CTM/50025/2020), which is also financed by national funds from FCT/MCTES. The publication is part of a project that has received funding from the European Union Horizon 2020 Research and Innovation Programme under grant agreement no. 774499 – GoJelly project. This research was funded by the Slovenian Research Agency (research core funding P1-0245).
Conflict of Interest
The authors declare that the research was conducted in the absence of any commercial or financial relationships that could be construed as a potential conflict of interest.
Acknowledgments
This publication is based upon work from COST Action CA18238 (Ocean4Biotech, https://www.ocean4biotech.eu/), supported by COST (European Cooperation in Science and Technology) program. SG thanks financial support provided by Fundação para a Ciência e a Tecnologia, MCTES, through grant IF/00700/2014. ZP thanks FCT/MCTES for the Norma transitória DL 57/2016 Program Contract. VdS thanks Coordenação de Aperfeiçoamento de Pessoal de Nível Superior - Brasil (CAPES) FinanceCode001.
Footnotes
- ^ https://ec.europa.eu/info/strategy/priorities-2019-2024/european-green-deal_en
- ^ https://ec.europa.eu/info/horizon-europe-next-research-and-innovation-framework-programme_en
References
Abbas-Abadi, M. S. (2020). The effect of process and structural parameters on the stability, thermo-mechanical and thermal degradation of polymers with hydrocarbon skeleton containing PE, PP, PS, PVC, NR, PBR and SBR. J. Therm. Anal. Calorim. doi: 10.1007/s10973-020-09344-0
Abhijith, R., Ashok, A., and Rejeesh, C. R. (2018). Sustainable packaging applications from mycelium to substitute polystyrene: a review. Mater. Today Proc. 5, 2139–2145. doi: 10.1016/j.matpr.2017.09.211
Ajibola, A. A., Omoleye, J. A., and Efeovbokhan, V. E. (2018). Catalytic cracking of polyethylene plastic waste using synthesised zeolite Y from Nigerian kaolin deposit. Appl. Petrochem. Res. 8, 211–2177. doi: 10.1007/s13203-018-0216-7
Akindoyo, J. O., Beg, M. D. H., Ghazali, S., Islam, M. R., Jeyaratnam, N., and Yuvaraj, A. R. (2016). Polyurethane types, synthesis and applications - A review. RSC Adv. R. Soc. Chem. 6, 114453–114482. doi: 10.1039/c6ra14525f
Albuquerque, M. G. E., Eiroa, M., Torres, C., Nunes, B. R., and Reis, M. A. M. (2007). Strategies for the development of a side stream process for polyhydroxyalkanoate (PHA) production from sugar cane molasses. J. Biotechnol. 130, 411–421. doi: 10.1016/j.jbiotec.2007.05.011
Ali, G., Nisar, J., Iqbal, M., Shah, A., Abbas, M., Shah, M., et al. (2020). Thermo-catalytic decomposition of polystyrene waste: comparative analysis using different kinetic models. Waste Manage. Res. 38, 202–212. doi: 10.1177/0734242X19865339
Ali, M. I., Perveen, Q., Ahmad, B., Javed, I., Razi-Ul-Hussnain, R., Andleeb, S., et al. (2009). Studies on biodegradation of cellulose blended polyvinyl chloride films. Int. J. Agric. Biol. 11, 577–580.
Almeida, D., and De Fátima Marques, M. F. (2016). Thermal and catalytic pyrolysis of plastic waste. Polimeros 26, 44–51. doi: 10.1590/0104-1428.2100
Al-Rasheed, H. H., Mohammady, S. Z., Dahlous, K., Siddiqui, M. R. H., and El-Faham, A. (2019). Synthesis, characterization, thermal stability and kinetics of thermal degradation of novel polymers based-s-triazine Schiff base. J. Polym. Res. 27:10.
Al-Sabagh, A. M., Yehia, F. Z., Eissa, A. M. F., Moustafa, M. E., Eshaq, G., Rabie, A. M., et al. (2014). Glycolysis of Poly(ethylene terephthalate) catalyzed by the lewis base ionic liquid [Bmim][OAc]. Ind. Eng. Chem. Res. 53, 18443–18451. doi: 10.1021/ie503677w
Alshehrei, F. (2017). Biodegradation of synthetic and natural plastic by microorganisms. J. Appl. Environ. Microbiol. 5, 8–19.
Andrady, A. L. (2011). Microplastics in the marine environment. Mar. Pollut. Bull. 62, 1596–1605. doi: 10.1016/j.marpolbul.2011.05.030
Andrady, A. L., Hamid, S. H., Hu, X., and Torikai, A. (1998). Effects of increased solar ultraviolet radiation on terrestrial ecosystems. J. Photochem. Photobiol. B Biol. 46, 96–103.
Anju, S., Prajitha, N., Sukanya, V. S., and Mohanan, P. V. (2020). Complicity of degradable polymers in health-care applications. Mater. Today Chem. 16, 100236. doi: 10.1016/j.mtchem.2019.100236
Arasu, M. V., Asha, K. R. T., Duraipandiyan, V., Ignacimuthu, S., and Agastian, P. (2012). Characterization and phylogenetic analysis of novel polyene type antimicrobial metabolite producing actinomycetes from marine sediments: bay of Bengal India. Asian Pac. J. Trop. Biomed. 2, 803–810. doi: 10.1016/s2221-1691(12)60233-0
Arutchelvi, J., Sudhakar, M., Arkatkar, A., Doble, M., Bhaduri, S., and Uppara, P. V. (2008). Biodegradation of polyethylene and polypropylene. Indian J. Biotechnol. 7, 9–22. doi: 10.1016/0306-3747(95)90387-9
Augusta, J., Miiller, R., and Widdecke, H. (1993). A rapid evaluation plate-test for the biodegradability of plastics. Appl. Microbiol. Biotechnol. 39, 673–678. doi: 10.1007/bf00205073
Auta, H. S., Emenike, C. U., and Fauziah, S. H. (2017). Screening of Bacillus strains isolated from mangrove ecosystems in Peninsular Malaysia for microplastic degradation. Environ. Pollut. 231, 1552–1559. doi: 10.1016/j.envpol.2017.09.043
Auta, H. S., Emenike, C. U., Jayanthi, B., and Fauziah, S. H. (2018). Growth kinetics and biodeterioration of polypropylene microplastics by Bacillus sp. and Rhodococcus sp. isolated from mangrove sediment. Mar. Pollut. Bull. 127, 15–21. doi: 10.1016/j.marpolbul.2017.11.036
Azapagic, A., Emsley, A., and Hamerton, I. (2003). Polymers: The Environment and Sustainable Development, Choice Reviews Online. Hoboken, NJ: Wiley.
Bäckström, E., Odelius, K., and Hakkarainen, M. (2017). Trash to treasure: microwave-assisted conversion of polyethylene to functional chemicals. Ind. Eng. Chem. Res. 56, 14814–14821. doi: 10.1021/acs.iecr.7b04091
Banker, D., Ahuja, A., Sanadhya, H., Purohit, C., Chandra, S., Sethi, R., et al. (2016). Clinical performance of biodegradable polymer coated sirolimus eluting stents in unselected “real-world” population with Coronary Artery Disease - Results from Multi-centre CORE Registry. Minerva Cardioangiol. 64, 9–14.
Bardají, D. K. R., Moretto, J. A. S., Furlan, J. P. R., and Stehling, E. G. (2020). A mini-review: current advances in polyethylene biodegradation. World J. Microbiol. Biotechnol. 36:32.
Barnes, D. K. A., Galgani, F., Thompson, R. C., and Barlaz, M. (2009). Accumulation and fragmentation of plastic debris in global environments. Philos. Trans. R. Soc. 364, 1985–1998. doi: 10.1098/rstb.2008.0205
Bilal, M., and Iqbal, H. M. N. (2019). Sustainable bioconversion of food waste into high-value products by immobilized enzymes to meet bio-economy challenges and opportunities – A review. Food Res. Int. 123, 226–240. doi: 10.1016/j.foodres.2019.04.066
Bonhomme, S., Cuer, A., Delort, A., Lemaire, J., Sancelme, M., and Scott, G. (2003). Environmental biodegradation of polyethylene. Polym. Degrad. Stab. 81, 441–452.
Botelho, G., Queirós, A., Liberal, S., and Gijsman, P. (2001). Studies on thermal and thermo-oxidative degradation of poly(ethylene terephthalate) and poly(butylene terephthalate). Polym. Degrad. Stab. 74, 39–48. doi: 10.1016/S0141-3910(01)00088-X
Brooks, A. N., and Beer, K. D. (2012). Adaptation of cells to new environments. Wiley Interdiscip. Rev. Syst. Biol. Med. 3, 544–561. doi: 10.1002/wsbm.136
Brunner, I., Fischer, M., Rüthi, J., Stierli, B., and Frey, B. (2018). Ability of fungi isolated from plastic debris floating in the shoreline of a lake to degrade plastics. PLoS One 13:e0202047. doi: 10.1371/journal.pone.0202047
Bugoni, L., Krause, L., and Petry, M. V. (2001). Marine debris and human impacts on sea turtles in Southern Brazil. Mar. Pollut. Bull. 42, 1330–1334. doi: 10.1016/s0025-326x(01)00147-3
Butbunchu, N., and Pathom-aree, W. (2019). Actinobacteria as promising candidate for polylactic acid type bioplastic degradation. Front. Microbiol. 10:2834. doi: 10.3389/fmicb.2019.02834
Cai, S., Zhang, B., and Cremaschi, L. (2017). Review of moisture behavior and thermal performance of polystyrene insulation in building applications. Build. Environ. 123, 50–65. doi: 10.1016/j.buildenv.2017.06.034
Carson, H. S., Colbert, S. L., Kaylor, M. J., and McDermid, K. J. (2011). Small plastic debris changes water movement and heat transfer through beach sediments. Mar. Pollut. Bull. 62, 1708–1713. doi: 10.1016/j.marpolbul.2011.05.032
Castro-Aguirre, E., Iñiguez-Franco, F., Samsudin, H., Fang, X., and Auras, R. (2016). Poly(lactic acid)—Mass production, processing, industrial applications, and end of life. Adv. Drug Deliv. Rev. 107, 333–366. doi: 10.1016/j.addr.2016.03.010
Chamas, A., Moon, H., Zheng, J., Qiu, Y., Tabassum, T., Jang, J. H., et al. (2020). Degradation rates of plastics in the environment. ACS Sustain. Chem. Eng. 8, 3494–3511. doi: 10.1021/acssuschemeng.9b06635
Chaudhary, A. K., and Vijayakumar, R. P. (2020). Studies on biological degradation of polystyrene by pure fungal cultures. Environ. Dev. Sustain. 22, 4495–4508. doi: 10.1007/s10668-019-00394-5
Chen, J., Nie, X. A., Jiang, J. C., and Zhou, Y. H. (2018). Thermal degradation and plasticizing mechanism of poly(vinyl chloride) plasticized with a novel cardanol derived plasticizer. IOP Conf. Ser. Mater. Sci. Eng. 292:012008. doi: 10.1088/1757-899X/292/1/012008
Chen, S., Liu, Z., Jiang, S., and Hou, H. (2020). Carbonization: a feasible route for reutilization of plastic wastes. Sci. Total Environ. 710:136250. doi: 10.1016/j.scitotenv.2019.136250
Cheng, L., Gu, J., Wang, Y., Zhang, J., Yuan, H., and Chen, Y. (2020). Polyethylene high-pressure pyrolysis: better product distribution and process mechanism analysis. Chem. Eng. J. 385:123866. doi: 10.1016/j.cej.2019.123866
Cheshire, A., Adler, E., Barbière, J., Cohen, Y., Evans, S., Jarayabhand, S., et al. (2009). UNEP/IOC Guidelines on Survey and Monitoring of Marine Litter, Regional S, p. IOC Technical Series No. 83. (Nairobi: UNEP), 120.
Chinaglia, S., Tosin, M., and Degli-Innocenti, F. (2018). Biodegradation rate of biodegradable plastics at molecular level. Polym. Degrad. Stab. 147, 237–244. doi: 10.1016/j.polymdegradstab.2017.12.011
Cho, K., Lee, J., and Kwon, K. (2000). Hydrolytic degradation behavior of poly(butylene succinate)s with different crystalline morphologies. J. Appl. Polym. Sci. 79, 1025–1033. doi: 10.1002/1097-4628(20010207)79:6<1025::aid-app50>3.0.co;2-7
Chubarenko, I., Bagaev, A., Zobkov, M., and Esiukova, E. (2016). On some physical and dynamical properties of microplastic particles in marine environment. Mar. Pollut. Bull. 108, 105–112. doi: 10.1016/j.marpolbul.2016.04.048
Close, L. G., Gilbert, R. D., and Fornes, R. E. (1977). Poly(vinyl chloride) Degradation — A review. Polym. Plast. Technol. Eng. 8, 177–198. doi: 10.1080/03602557708545035
Colabuono, F. I., Barquete, V., Domingues, B. S., and Montone, R. C. (2009). Plastic ingestion by Procellariiformes in Southern Brazil. Mar. Pollut. Bull. 58, 93–96. doi: 10.1016/j.marpolbul.2008.08.020
Connors, P. G., and Smith, K. G. (1982). Oceanic plastic particle pollution: suspected effect on fat deposition in red phalaropes. Mar. Pollut. Bull. 13, 18–20. doi: 10.1016/0025-326x(82)90490-8
Cooper, T. A. (2013). Developments in Bioplastic Materials for Packaging Food, Beverages and Other Fast-Moving Consumer Goods. Sawston: Woodhead Publising Limited, 108–152. doi: 10.1533/9780857098979.108
Correia, J., João, P., Lopes, I., Duarte, A. C., and Rocha-Santos, T. (2020). Environmental exposure to microplastics: an overview on possible human health effects. Sci. Total Environ. 702:134455. doi: 10.1016/j.scitotenv.2019.134455
Cosgrove, L., McGeechan, P. L., Robson, G. D., and Handley, P. S. (2007). Fungal communities associated with degradation of polyester polyurethane in soil. Appl. Environ. Microbiol. 73, 5817–5824. doi: 10.1128/aem.01083-07
Cox, K. D., Covernton, G. A., Davies, H. L., Dower, J. F., Juanes, F., and Dudas, S. E. (2019). Human consumption of microplastics. Environ. Sci. Technol. 53, 7068–7074. doi: 10.1021/acs.est.9b01517
Crawford, C. B., and Quinn, B. (2017). “Physiochemical properties and degradation,” in Microplastic Pollutants eds C. B. Crawford and B. Quinn (Amsterdam: Elsevier), 57–100. doi: 10.1016/b978-0-12-809406-8.00004-9
de Lucia, G. A., Caliani, I., Marra, S., Camedda, A., Coppa, S., Alcaro, L., et al. (2014). Amount and distribution of neustonic micro-plastic off the western Sardinian coast (Central-Western Mediterranean Sea). Mar. Environ. Res. 100, 10–16. doi: 10.1016/j.marenvres.2014.03.017
Delamarche, E., Mattlet, A., Livi, S., Gérard, J., Bayard, R., and Massardier, V. (2020). Tailoring biodegradability of Poly(Butylene Succinate)/Poly(Lactic Acid) blends with a deep eutectic solvent. Front. Mater. 7:7. doi: 10.3389/fmats.2020.00007
Derraik, J. G. B. (2002). The pollution of the marine environment by plastic debris: a review. Mar. Pollut. Bull. 44, 842–852. doi: 10.1016/s0025-326x(02)00220-5
Desforges, J. P. W., Galbraith, M., Dangerfield, N., and Ross, P. S. (2014). Widespread distribution of microplastics in subsurface seawater in the NE Pacific Ocean. Mar. Pollut. Bull. 79, 94–99. doi: 10.1016/j.marpolbul.2013.12.035
Devi, R. S., Kannan, V. R., Nivas, D., Kannan, K., Chandru, S., and Antony, A. R. (2015). Biodegradation of HDPE by Aspergillus spp. from marine ecosystem of Gulf of Mannar India. Mar. Pollut. Bull. 96, 32–40. doi: 10.1016/j.marpolbul.2015.05.050
Dhahak, A., Hild, G., Rouaud, M., Mauviel, G., and Burkle-Vitzthum, V. (2019). Slow pyrolysis of polyethylene terephthalate: Online monitoring of gas production and quantitative analysis of waxy products. J. Anal. Appl. Pyrolysis 142:104664. doi: 10.1016/j.jaap.2019.104664
Diaz-Silvarrey, L. S., McMahon, A., and Phan, A. N. (2018). Benzoic acid recovery via waste poly(ethylene terephthalate) (PET) catalytic pyrolysis using sulphated zirconia catalyst. J. Anal. Appl. Pyrolysis Elsevier 134, 621–631. doi: 10.1016/j.jaap.2018.08.014
Drozdov, A. D. (2007). The effect of thermal oxidative degradation of polymers on their viscoelastic response. Int. J. Eng. Sci. 45, 882–904. doi: 10.1016/j.ijengsci.2007.07.004
Enders, K., Lenz, R., Stedmon, C. A., and Nielsen, T. G. (2015). Abundance, size and polymer composition of marine microplastics ≥ 10 μ m in the Atlantic Ocean and their modelled vertical distribution. Mar. Pollut. Bull. 100, 70–81. doi: 10.1016/j.marpolbul.2015.09.027
Eriksen, M. M., Lebreton, L. C., Carson, H. S., Thiel, M., Moore, C. J., Borerro, J. C., et al. (2014). Plastic pollution in the world ’ s oceans: more than 5 trillion plastic pieces weighing over 250,000 Tons Afloat at Sea. PLoS One 9:e111913. doi: 10.1371/journal.pone.0111913
Esquer, R., and García, J. J. (2019). Metal-catalysed Poly(Ethylene) terephthalate and polyurethane degradations by glycolysis. J. Organomet. Chem. 902, 120972. doi: 10.1016/j.jorganchem.2019.120972
European Bioplastics (2016). Bioplastics and the Circular Economy. Available at: https://docs.european-bioplastics.org/publications/pp/EUBP_PP_Circular_economy_package.pdf (accessed May 27, 2020).
Fernandes, E. G., Kenawy, E. R., Miertus, S., and Chiellini, E. (2002). Environmentally degradable plastics: thermal behavior of polymer blends based on waste gelatin. Polimery 47, 500–508. doi: 10.14314/polimery.2002.500
Ferreira, A. R. V., Alves, V. D., and Coelhoso, I. M. (2016). Polysaccharide-based membranes in food packaging applications. Membranes 6:22. doi: 10.3390/membranes6020022
Fontanella, S., Bonhomme, S., Brusson, J., Pitteri, S., Samuel, G., Pichon, G., et al. (2013). Comparison of biodegradability of various polypropylene films containing pro-oxidant additives based on Mn, Mn/Fe or Co. Polym. Degrad. Stab. 98, 875–884. doi: 10.1016/j.polymdegradstab.2013.01.002
Fuentes-Ordóñez, E. G., Salbidegoitia, J. A., González-Marcos, M. P., and González-Velasco, J. R. (2016). Mechanism and kinetics in catalytic hydrocracking of polystyrene in solution. Polym. Degrad. Stab. 124, 51–59. doi: 10.1016/j.polymdegradstab.2015.12.009
Fukushima, K., Coady, D. J., Jones, G. O., Almegren, H. A., Alabdulrahman, A. M., Alsewailem, F. D., et al. (2013a). Advanced chemical recycling of poly(ethylene terephthalate) through organocatalytic aminolysis. Polym. Chem. 4, 1610–1616. doi: 10.1039/c2py20793a
Fukushima, K., Lecuyer, J. M., Wei, D. S., Horn, H. W., Jones, G. O., Al-Megren, H. A., et al. (2013b). Unexpected efficiency of cyclic amidine catalysts in depolymerizing poly(ethylene terephthalate). J. Polym. Sci. Part A Polym. Chem. 51, 1606–1611. doi: 10.1002/pola.26530
Gardette, M., Perthue, A., Gardette, J., Janecska, T., Földes, E., Pukánszky, B., et al. (2013). Photo- and thermal-oxidation of polyethylene: comparison of mechanisms and influence of unsaturation content. Polym. Degrad. Stab. 98, 2383–2390. doi: 10.1016/j.polymdegradstab.2013.07.017
Genta, M., Iwaya, T., Sasaki, M., Goto, M., and Hirose, T. (2005). Depolymerization -mechanism of Poly(ethylene terephthalate) in Supercritical Methanol. Ind. Eng. Chem. Res. 44, 3894–3900. doi: 10.1021/ie0488187
George, N., and Kurian, T. (2016). Sodium carbonate catalyzed aminolytic degradation of PET. Prog. Rubber Plast. Recycl. Technol. 32, 153–168. doi: 10.1177/147776061603200304
Gerothanassis, I. P., Troganis, A., Exarchou, V., and Barbarossou, K. (2002). Nuclear Magnetic Resonance (NMR) spectroscopy: basic principles and phenomena, and their applications to chemistry, biology and medicine. Chem. Educ. Res. Pract. Europe 3, 229–252. doi: 10.1039/b2rp90018a
GESAMP (2015). “Sources, fate and effects of microplastics in the marine environment: a global assessment,” in IMO/FAO/UNESCO-IOC/UNIDO/WMO/IAEA/UN/UNEP/UNDP Joint Group of Experts on the Scientific Aspects of Marine Environmental Protection, ed. P. J. Kershaw (London: GESAMP).
Gewert, B., Plassmann, M. M., and Macleod, M. (2015). Pathways for degradation of plastic polymers floating in the marine environment. Environ. Sci. Process. Impacts R. Soc. Chem. 17, 1513–1521. doi: 10.1039/c5em00207a
Geyer, R., Jambeck, J. R., and Law, K. L. (2017). Production, use, and fate of all plastics ever made. Sci. Adv. 3:e1700782. doi: 10.1126/sciadv.1700782
Gondal, M. A., and Siddiqui, M. N. (2007). Identification of different kinds of plastics using laser-induced breakdown spectroscopy for waste management. J. Environ. Sci. Health Part A 42, 1989–1997. doi: 10.1080/10934520701628973
Goto, M., Koyamoto, H., Kodama, A., Hirose, T., Nagaoka, S., and McCoy, B. J. (2002). Degradation kinetics of polyethylene terephthalate in supercritical methanol. AIChE J. 48, 136–144. doi: 10.1002/aic.690480114
Grassle, J. F., and Maciolek, N. J. (2013). Deep-sea species richness: regional and local diversity estimates from quantitative bottom samples. Am. Nat. 139, 313–341. doi: 10.1086/285329
Gregory, M. R. (2009). Environmental implications of plastic debris in marine settings — entanglement, ingestion, and alien invasions. Philos. Trans. R. Soc. 364, 2013–2025. doi: 10.1098/rstb.2008.0265
Gupta, P., and Bhandari, S. (2019). “Chemical depolymerization of PET Bottles via Ammonolysis and Aminolysis,” in Recycling of Polyethylene Terephthalate Bottles eds S. Thomas, A. Rane, K. Kanny, V. K. Abitha, and M. G. Thomas (Amsterdam: Elsevier), 109–134. doi: 10.1016/b978-0-12-811361-5.00006-7
Guzik, M. W., Kenny, S. T., Duane, G. F., Casey, E., Woods, T., Babu, R. P., et al. (2014). Conversion of post consumer polyethylene to the biodegradable polymer polyhydroxyalkanoate. Appl. Microbiol. Biotechnol. 98, 4223–4232. doi: 10.1007/s00253-013-5489-2
Hadad, D., Geresh, S., and Sivan, A. (2005). Biodegradation of polyethylene by the thermophilic bacterium Brevibacillus borstelensis. J. Appl. Microbiol. 98, 1093–1100. doi: 10.1111/j.1365-2672.2005.02553.x
Haider, T. P., Völker, C., Kramm, J., Landfester, K., and Wurm, F. R. (2019). Plastics of the Future? The impact of biodegradable polymers on the environment and on society. Angew. Chem. Int. Ed. 58, 50–62. doi: 10.1002/anie.201805766
Harris, A. M., and Lee, E. C. (2013). Durability of polylactide-based polymer blends for injection-molded applications. J. Appl. Polym. Sci. 128, 2136–2144. doi: 10.1002/app.38407
Hassan, A. A., Abbas, A., Rasheed, T., Bilal, M., Iqbal, H. M. N., and Wang, S. (2019). Development, influencing parameters and interactions of bioplasticizers: an environmentally friendlier alternative to petro industry-based sources. Sci. Total Environ. 682, 394–404. doi: 10.1016/j.scitotenv.2019.05.140
Hawkins, W. L. (1964). Thermal and oxidative degradation of polymers. Polym. Eng. Sci. 4, 187–192. doi: 10.1002/pen.760040309
Heath, D. E., and Cooper, S. L. (2013). “A - Polyurethanes,” in Biomaterials Science - An Introduction to Materials in Medicine, 3rd Edn, eds B. Ratner, A. Hoffman, F. Schoen, and J. Lemons (Amsterdam: Elsevier), 79–82.
Hesse, N. D., and White, R. L. (2004). Polyethylene Catalytic Hydrocracking by PtHZSM-5, PtHY, and PtHMCM-41. J. Appl. Polym. Sci. 92, 1293–1301. doi: 10.1002/app.20083
Hidalgo-Ruz, V., Gutow, L., Thompson, R. C., and Thiel, M. (2012). Microplastics in the marine environment: a review of the methods used for identification and quantification. Environ. Sci. Technol. 46, 3060–3075. doi: 10.1021/es2031505
Hiraga, K., Taniguchi, I., Yoshida, S., Kimura, Y., and Oda, K. (2019). A sustainable solution for dealing with plastic pollution. EMBO Rep. 20:e49365. doi: 10.15252/embr.201949365
Ho, N. H. E., and Not, C. (2019). Selective accumulation of plastic debris at the breaking wave area of coastal waters. Environ. Pollut. 245, 702–710. doi: 10.1016/j.envpol.2018.11.041
Hoang, C. N., Le, T. T. N., and Hoang, Q. D. (2019). Glycolysis of poly(ethylene terephthalate) waste with diethyleneglycol under microwave irradiation and ZnSO4 ⋅7H2O catalyst. Polym. Bull. 76, 23–34. doi: 10.1007/s00289-018-2369-z
Hopewell, J., Dvorak, R., and Kosior, E. (2009). Plastics recycling: challenges and opportunities. Philos. Trans. R. Soc. 364, 2115–2126. doi: 10.1098/rstb.2008.0311
Horn, H. W., Jones, G. O., Wei, D. S., Fukushima, K. M., Lecuyer, J., Coady, D. J., et al. (2012). Mechanisms of organocatalytic amidation and trans-esterification of aromatic esters as a model for the depolymerization of poly(ethylene) terephthalate. J. Phys. Chem. A 116, 12389–12398. doi: 10.1021/jp304212y
Huerta, E., Thapa, B., Yang, X., Gertsen, H., Salánki, T., Geissen, V., et al. (2018). Decay of low-density polyethylene by bacteria extracted from earthworm’s guts: a potential for soil restoration. Sci. Total Environ. 624, 753–757. doi: 10.1016/j.scitotenv.2017.12.144
Imran, M., Kim, D. H., Al-Masry, W. A., Mahmood, A., Hassan, A., Haider, S., et al. (2013). Manganese-, cobalt-, and zinc-based mixed-oxide spinels as novel catalysts for the chemical recycling of poly(ethylene terephthalate) via glycolysis. Polym. Degrad. Stab. 98, 904–915. doi: 10.1016/j.polymdegradstab.2013.01.007
Jabeen, N., Majid, I., and Nayik, G. A. (2015). Bioplastics and food packaging: a review. Cogent Food Agric. 1:1117749. doi: 10.1080/23311932.2015.1117749
Janssen, P. H., Yates, P. S., Grinton, B. E., Taylor, P. M., and Sait, M. (2002). Improved culturability of soil bacteria and isolation in pure culture of novel members of the divisions Acidobacteria, Actinobacteria, Proteobacteria, and Verrucomicrobia. Appl. Environ. Microbiol. 68, 2391–2396. doi: 10.1128/AEM.68.5.2391
Jarerat, A., and Tokiwa, Y. (2001). Degradation of Poly(L-lactide) by a Fungus. Macromol. Biosci. 1, 136–140.
Jarerat, A., and Tokiwa, Y. (2003). Poly(L -lactide) degradation by Saccharothrix waywayandensis. Biotechnol. Lett. 1012, 401–404.
Jarerat, A., Tokiwa, Y., and Tanaka, H. (2003). Poly(L-lactide) degradation by Kibdelosporangium aridum. Biotechnol. Lett. 25, 2035–2038. doi: 10.1023/b:bile.0000004398.38799.29
Jarerat, A., Tokiwa, Y., and Tanaka, H. (2006). Production of poly(L-lactide) -degrading enzyme by Amycolatopsis orientalis for biological recycling of poly(L-lactide). Appl. Microbiol. Biotechnol. 72, 726–731. doi: 10.1007/s00253-006-0343-4
Jefferson, M. (2019). Whither Plastics?— Petrochemicals, plastics and sustainability in a garbage- riddled world. Energy Res. Soc. Sci. 56:101229. doi: 10.1016/j.erss.2019.101229
Jehanno, C., Flores, I., Dove, A. P., Müller, A. J., Ruipérez, F., and Sardon, H. (2018). Organocatalysed depolymerisation of PET in a fully sustainable cycle using thermally stable protic ionic salt. Green Chem. 20, 1205–1212. doi: 10.1039/C7GC03396F
Jehanno, C., Pérez-Madrigal, M. M., Demarteau, J., Sardon, H., and Dove, A. P. (2019). Organocatalysis for depolymerisation. Polym. Chem. R. Soc. Chem. 172–186. doi: 10.1039/c8py01284a
Jeon, H. J., and Kim, M. N. (2013). Biodegradation of poly (L-lactide) (PLA) exposed to UV irradiation by a mesophilic bacterium. Int. Biodeterior. Biodegrad. 85, 289–293. doi: 10.1016/j.ibiod.2013.08.013
Jia, X., Qin, C., Friedberger, T., Guan, Z., and Huang, Z. (2016). Efficient and selective degradation of polyethylenes into liquid fuels and waxes under mild conditions. Sci. Adv. 2:e1501591. doi: 10.1126/sciadv.1501591
Jian, S. Z., Lucki, J., Rabek, J. F., and Ranby, B. (1985). Photooxidation and photostabilization of unsaturated cross-linked polyesters. ACS Symp. Ser. 280, 353–358. doi: 10.1021/bk-1985-0280.ch024
Kamo, T., Kodera, Y., Sato, Y., and Kushiyama, S. (2004). Effects of pressure on the degradation of poly(vinyl chloride). Polym. Degrad. Stab. 84, 79–85. doi: 10.1016/j.polymdegradstab.2003.09.014
Karamanlioglu, M., Houlden, A., and Robson, G. D. (2014). Isolation and characterisation of fungal communities associated with degradation and growth on the surface of poly(lactic) acid (PLA) in soil and compost. Int. Biodeterior. Biodegrad. 95, 301–310. doi: 10.1016/j.ibiod.2014.09.006
Karamanlioglu, M., Preziosi, R., and Robson, G. D. (2017). Abiotic and biotic environmental degradation of the bioplastic polymer poly(lactic acid): a review. Polym. Degrad. Stab. 137, 122–130. doi: 10.1016/j.polymdegradstab.2017.01.009
Kárpáti, L., Ganyecz, Á., Nagy, T., Hamar, G., Banka, E., Kállay, M., et al. (2019). Synthesis and characterization of isophorondiamine-based oligoamides: catalytic effect of amides during the curing of epoxy resins. Polym. Bull. 77, 4655–4678. doi: 10.1007/s00289-019-02987-6
Kathiresan, K. (2003). Polythene and Plastics-degrading microbes from the mangrove soil. Rev. Biol. Trop. 51, 629–634.
Keshavarz, T., and Roy, I. (2010). Polyhydroxyalkanoates: bioplastics with a green agenda. Curr. Opin. Microbiol. 13, 321–326. doi: 10.1016/j.mib.2010.02.006
Kettner, M. T., Rojas-Jimenez, K., Oberbeckmann, S., Labrenz, M., and Grossart, H. P. (2017). Microplastics alter composition of fungal communities in aquatic ecosystems. Environ. Microbiol. 19, 4447–4459. doi: 10.1111/1462-2920.13891
Khan, S., Nadir, S., Shah, Z. U., Shah, A. A., Karunarathna, S. C., Xu, J., et al. (2017). Biodegradation of polyester polyurethane by Aspergillus tubingensis. Environ. Pollut. 225, 469–480. doi: 10.1016/j.envpol.2017.03.012
Khoonkari, M., Haghighi, A. H., Sefidbakht, Y., Shekoohi, K., and Ghaderian, A. (2015). Chemical recycling of PET wastes with different catalysts. Int. J. Polym. Sci. 2015, 124524. doi: 10.1155/2015/124524
Kim, B. K., Kim, D., Cho, Y., and Han, M. (2008). Chemical recycling of poly(ethylene terephthalate) using a new hybrid process. J. Chem. Eng. Jpn. 41, 923–928. doi: 10.1252/jcej.07WE304
Kirstein, I. V., Kirmizi, S., Wichels, A., Garin-fernandez, A., Erler, R., and Martin, L. (2016). Dangerous hitchhikers? Evidence for potentially pathogenic Vibrio spp. on microplastic particles. Mar. Environ. Res 120, 1–8. doi: 10.1016/j.marenvres.2016.07.004
Kolstad, J. J., Vink, E. T. H., De Wilde, B., and Debeer, L. (2012). Assessment of anaerobic degradation of IngeoTM polylactides under accelerated landfill conditions. Polym. Degrad. Stab. 97, 1131–1141. doi: 10.1016/j.polymdegradstab.2012.04.003
Kopinke, F.-D., Remmler, M., Mackenzie, K., Möder, M., and Wachsen, O. (1996). Thermal decomposition of biodegradable polyesters—II. Poly(lactic acid). Polym. Degrad. Stab. 53, 329–342. doi: 10.1016/0141-3910(96)00102-4
Krueger, M. C., Harms, H., and Schlosser, D. (2015). Prospects for microbiological solutions to environmental pollution with plastics. 8857–8874. doi: 10.1007/s00253-015-6879-4
Kruse, M., and Wagner, M. H. (2016). Time-resolved rheometry of poly(ethylene terephthalate) during thermal and thermo-oxidative degradation. Rheol. Acta 55, 789–800. doi: 10.1007/s00397-016-0955-2
Kumagai, S., and Yoshioka, T. (2016). Feedstock recycling via waste plastic pyrolysis. J. Jpn. Petrol. Inst. 59, 243–253. doi: 10.1627/jpi.59.243
Kurokawa, H., Ohshima, M. A., Sugiyama, K., and Miura, H. (2003). Methanolysis of polyethylene terephthalate (PET) in the presence of aluminium tiisopropoxide catalyst to form dimethyl terephthalate and ethylene glycol. Polym. Degrad. Stab. 79, 529–533. doi: 10.1016/s0141-3910(02)00370-1
Lambert, S., and Wagner, M. (2017). Environmental performance of bio-based and biodegradable plastics: the road ahead, Chemical Society Reviews. R. Soc. Chem. 46, 6855–6871. doi: 10.1039/c7cs00149e
Laycock, B., Wang, X., Liu, R., Annamalai, P. K., Cork, J., Derstine, C., et al. (2020). Pyrolysis of brominated polyethylene as an alternative carbon fibre precursor. Polym. Degrad. Stab. 172:109057. doi: 10.1016/j.polymdegradstab.2019.109057
Lee, A., and Liew, M. S. (2020). Tertiary recycling of plastics waste: an analysis of feedstock, chemical and biological degradation methods. J. Mater. Cycles Waste Manage. doi: 10.1007/s10163-020-01106-2
Lee, H., Joon, W., and Kwon, J. (2014). Sorption capacity of plastic debris for hydrophobic organic chemicals. Sci. Total Environ. 470-471, 1545–1552. doi: 10.1016/j.scitotenv.2013.08.023
Lestari, P., and Trihadiningrum, Y. (2019). The impact of improper solid waste management to plastic pollution in Indonesian coast and marine environment. Mar. Pollut. Bull. 149:110505. doi: 10.1016/j.marpolbul.2019.110505
Li, J., Qu, X., Su, L., Zhang, W., Yang, D., and Kolandhasamy, P. (2016). Microplastics in mussels along the coastal waters of China. Environ. Pollut. 214, 177–184. doi: 10.1016/j.envpol.2016.04.012
Li, L., Guan, C., Zhang, A., Chen, D., and Qing, Z. (2004). Thermal stabilities and the thermal degradation kinetics of polyimides. Polym. Degrad. Stab. 84, 369–373. doi: 10.1016/j.polymdegradstab.2003.11.007
Li, X., Tian, Y., Xu, C., and Cheng, B. (2019). The impact of marine pollution control on the output value of marine fisheries based on the spatial econometric model. J. Coast. Res. 98, 381–384. doi: 10.2112/SI98-088.1
Lipsa, R., Tudorachi, N., Darie-Nita, R. N., Opricã, L., Vasile, C., and Chiriac, A. (2016). Biodegradation of poly(lactic acid) and some of its based systems with Trichoderma viride. Int. J. Biol. Macromol. 88, 515–526. doi: 10.1016/j.ijbiomac.2016.04.017
Lithner, D., Larsson, A., and Dave, G. (2011). Environmental and health hazard ranking and assessment of plastic polymers based on chemical composition. Sci. Total Environ. 409, 3309–3324. doi: 10.1016/j.scitotenv.2011.04.038
Liu, Q., Li, R., and Fang, T. (2015). Investigating and modeling PET methanolysis under supercritical conditions by response surface methodology approach. Chem. Eng. J. 270, 535–541. doi: 10.1016/j.cej.2015.02.039
Lopez, G., Artetxe, M., Amutio, M., Alvarez, J., Bilbao, J., and Olazar, M. (2018). Recent advances in the gasification of waste plastics. A critical overview. Renew. Sustain. Energy Rev. 82, 576–596. doi: 10.1016/j.rser.2017.09.032
Lopez, G., Artetxe, M., Amutio, M., Bilbao, J., and Olazar, M. (2017). Thermochemical routes for the valorization of waste polyolefinic plastics to produce fuels and chemicals. A review. Renew. Sustain. Energy Rev 73, 346–368. doi: 10.1016/j.rser.2017.01.142
Lu, L., and Xu, F. (2009). Effect of light-barrier property of packaging film on the photo-oxidation and shelf life of cookies based on accelerated tests. Packag. Technol. Sci. 22, 107–113. doi: 10.1002/pts.838
Luz, J. M. R., Silva, L. C. S., Santos, L. F., and Kasuya, M. C. M. (2019). Plastics Polymers Degradation by Fungi. London: IntechOpen, doi: 10.5772/intechopen.88608
Lyu, S., and Untereker, D. (2009). Degradability of polymers for implantable biomedical devices. Int. J. Mol. Sci. 10, 4033–4065. doi: 10.3390/ijms10094033
Mancini, S. D., and Zanin, M. (2007). Post consumer PET depolymerization by acid hydrolysis. Polym. Plast. Technol. Eng. 46, 135–144. doi: 10.1080/03602550601152945
Mandin, C., Dris, R., Gasperi, J., and Guerrouache, M. (2017). A first overview of textile fibers, including microplastics, in indoor and outdoor environments. Environ. Pollut. 221, 453–458. doi: 10.1016/j.envpol.2016.12.013
Mannina, G., Presti, D., Montiel-Jarillo, G., Carrera, J., and Suárez-Ojeda, M. E. (2020). Recovery of polyhydroxyalkanoates (PHAs) from wastewater: a review. Bioresour. Technol. 297, 122478. doi: 10.1016/j.biortech.2019.122478
Martínez, J. G., Benavides, R., Guerrero, C., and Reyes, B. E. (2004). UV sensitisation of polyethylenes for grafting of maleic anhydride. Polym. Degrad. Stab. 86, 129–134. doi: 10.1016/j.polymdegradstab.2004.02.016
Matavulj, M., and Molitoris, H. (2009). Marine fungi: degraders of poly-3-hydroxyalkanoate based plastic materials. Proc. Nat. Sci Matica Srpska Novi Sad 116, 253–265. doi: 10.2298/zmspn0916253m
Mckeen, L. W. (2014). “Handbook of polymer applications in medicine and medical devices,” in Handbook of Polymer Applications in Medicine and Medical Devices, eds K. Modjarrad and S. Ebnesajjad (Amsterdam: Elsevier Inc), 21–54. doi: 10.1016/b978-0-323-22805-3.00003-7
McKeown, P., and Jones, M. D. (2020). The chemical recycling of PLA: a review. Sustain. Chem. 1, 1–22. doi: 10.3390/suschem1010001
Meereboer, K. W., Misra, M., and Mohanty, A. K. (2020). Review of recent advances in the biodegradability of polyhydroxyalkanoate (PHA) bioplastics and their composites. Green Chem. 22, 5519–5558. doi: 10.1039/d0gc01647k
Mergaert, J., and Swings, J. (1996). Biodiversity of microorganisms that degrade bacterial and synthetic polyesters. J. Ind. Microbiol. 17, 463–469. doi: 10.1007/bf01574777
Miandad, R., Rehan, M., Barakat, M. A., Aburiazaiza, A. S., Khan, H., Ismail, I. M. I., et al. (2019). Catalytic pyrolysis of plastic waste: moving toward pyrolysis based biorefineries. Front. Energy Res. 7:27. doi: 10.3389/fenrg.2019.00027
Miao, L., Wang, P., Hou, J., Yao, Y., Liu, Z., Liu, S., et al. (2019). Distinct community structure and microbial functions of bio films colonizing microplastics. Sci. Total Environ. 650, 2395–2402. doi: 10.1016/j.scitotenv.2018.09.378
Mierzwa-Hersztek, M., Gondek, K., and Kopeć, M. (2019). Degradation of polyethylene and biocomponent-derived polymer materials: an overview. J. Polym. Environ. 27, 600–611. doi: 10.1007/s10924-019-01368-4
Min, K., Cuiffi, J. D., and Mathers, R. T. (2020). Ranking environmental degradation trends of plastic marine debris based on physical properties and molecular structure. Nat. Commun. 11:727. doi: 10.1038/s41467-020-14538-z
Mishra, S., and Goje, A. S. (2003). Kinetic and thermodynamic study of methanolysis of poly(ethylene terephthalate) waste powder. Polym. Int. 52, 337–342. doi: 10.1002/pi.1147
Moore, C. (2007). “Synthetic polymers in the marine environment: What We Know. What We Need to Know. What Can be Done?,” in Proceedings of the International Seminar on Nuclear War and Planetary Emergencies 36th Session Vol. 30, (Singapore: World Scientific), 2221.
Moore, C. J. (2008). Synthetic polymers in the marine environment: a rapidly increasing, long-term threat. Environ. Res. 108, 131–139. doi: 10.1016/j.envres.2008.07.025
Mor, R., and Sivan, A. (2008). Biofilm formation and partial biodegradation of polystyrene by the actinomycete Rhodococcus ruber: biodegradation of polystyrene. Biodegradation. 19, 851–858. doi: 10.1007/s10532-008-9188-0
Muhonja, C. N., Makonde, H., Magoma, G., and Imbuga, M. (2018). Biodegradability of polyethylene by bacteria and fungi from Dandora dumpsite Nairobi-Kenya. PLoS One 13:e0198446. doi: 10.1371/journal.pone.0198446
Munir, E., Harefa, R. S. M., Priyani, N., and Suryanto, D. (2018). Plastic degrading fungi Trichoderma viride and Aspergillus nomius isolated from local landfill soil in Medan. IOP Conf. Ser. Earth Environ. Sci. 126, 012145. doi: 10.1088/1755-1315/126/1/012145
Muralidharan, A., Pina, A., Ferrão, P., Fournier, J., Lacarrière, B., and Corre, O. L. (2017). District alternatives Heating and Cooling Study, design and analysis of sustainable to plastic takeaway cutlery and crockery Assessing the feasibility of using the heat demand-outdoor temperature function for a long-term demand forecast. Energy Procedia 136, 507–512. doi: 10.1016/j.egypro.2017.10.273
Musale, R. M., and Shukla, S. R. (2016). Deep eutectic solvent as effective catalyst for aminolysis of polyethylene terephthalate (PET) waste. Int. J. Plast. Technol. 20, 106–120. doi: 10.1007/s12588-016-9134-7
Musioł, M., Sikorska, W., Adamus, G., Janeczek, H., Richert, J., Malinowski, R., et al. (2016). Forensic engineering of advanced polymeric materials. Part III - Biodegradation of thermoformed rigid PLA packaging under industrial composting conditions. Waste Manage. 52, 69–76. doi: 10.1016/j.wasman.2016.04.016
Muthuraj, R., Misra, M., and Mohanty, A. K. (2015). Hydrolytic degradation of biodegradable polyesters under simulated environmental conditions. J. Appl. Polym. Sci. 132, 1–13. doi: 10.1002/app.42189
Neiman, M. B. (1964). Mechanism of the oxidative thermal degradation and of the stabilisation of polymers. Russ. Chem. Rev. 33, 13–27.
Nel, H. A., and Froneman, P. W. (2015). A quantitative analysis of microplastic pollution along the south-eastern coastline of South Africa. Mar. Pollut. Bull. 101, 274–279. doi: 10.1016/j.marpolbul.2015.09.043
Neves, D., Sobral, P., Lia, J., and Pereira, T. (2015). Ingestion of microplastics by commercial fish off the Portuguese coast. MPB 101, 119–126. doi: 10.1016/j.marpolbul.2015.11.008
Nisar, J., Ali, G., Shah, A., Shah, M. R., Iqbal, M., Ashiq, M. N., et al. (2019). Pyrolysis of expanded waste polystyrene: influence of nickel-doped copper oxide on kinetics, thermodynamics, and product distribution. Energy Fuels 33, 12666–12678. doi: 10.1021/acs.energyfuels.9b03004
Nowak, B., Pajak, J., Drozd-bratkowicz, M., and Rymarz, G. (2011). Microorganisms participating in the biodegradation of modified polyethylene films in different soils under laboratory conditions. Int. Biodeterior. Biodegrad. 65, 757–767. doi: 10.1016/j.ibiod.2011.04.007
OECD (2018). Improving Markets for Recycled Plastics: Trends, Prospects and Policy Responses. Paris: OECD Publishing.
Oelofse, S. H. H., Viljoen, P., Taljaard, S., and Botes, W. A. M. (2004). Discharge of water containing waste emanating from land to the marine environment: a water quality management perspective. Water S.A 330, 56–60.
Paço, A., Duarte, K., da Costa, J. P., Santos, P. S. M., Pereira, R., Pereira, M. E., et al. (2017). Biodegradation of polyethylene microplastics by the marine fungus Zalerion maritimum. Sci. Total Environ. 586, 10–15. doi: 10.1016/j.scitotenv.2017.02.017
Park, S. Y., and Kim, C. G. (2019). Biodegradation of micro-polyethylene particles by bacterial colonization of a mixed microbial consortium isolated from a landfill site. Chemosphere. 222, 527–533. doi: 10.1016/j.chemosphere.2019.01.159
Pathak, V. M., and Navneet. (2017). Review on the current status of polymer degradation: a microbial approach. Bioresour. Bioprocess. 4, 1–31.
Pickett, J. E., and Moore, J. E. (1993). Photodegradation of UV screeners. Polym. Degrad. Stab. 42, 231–244. doi: 10.1016/0141-3910(93)90219-9
Piemonte, V., Sabatini, S., and Gironi, F. (2013). Chemical Recycling of PLA: A Great Opportunity Towards the Sustainable Development? J. Polym. Environ 21, 640–647. doi: 10.1007/s10924-013-0608-9
Pometto, A. L. III, Lee, B., and Johnson, K. E. (1992). Production of an extracellular polyethylene-degrading enzyme(s) by Streptomyces species. Appl. Environ. Microbiol. 58, 731–733. doi: 10.1128/aem.58.2.731-733.1992
Pospísil, J., Pilar, J., Billingham, N. C., Marek, A., Horák, Z., and Nespůrek, S. (2006). Factors affecting accelerated testing of polymer photostability. Polym. Degrad. Stab. 91, 417–422. doi: 10.1016/j.polymdegradstab.2005.01.049
Prata, J. C. (2018). Airborne microplastics: Consequences to human health? Environ. Pollut. 234, 115–126. doi: 10.1016/j.envpol.2017.11.043
Prathipa, R., Sivakumar, C., and Shanmugasundaram, B. (2018). Biodegradable polymers for sustainable packaging applications. Int. J. Mech. Eng. Technol. 9, 293–303.
Pudack, C., Stepanski, M., and Fässler, P. (2020). PET Recycling – Contributions of crystallization to sustainability. Chem. Ing. Tech. 92, 452–458. doi: 10.1002/cite.201900085
Qi, X., Ren, Y., and Wang, X. (2017). New advances in the biodegradation of Poly(lactic) acid. Int. Biodeterior. Biodegrad. 117, 215–223. doi: 10.1016/j.ibiod.2017.01.010
Qiu, B., Deng, N., Zhang, Y., and Wan, H. (2018). Application of industrial solid wastes in catalytic pyrolysis. Asia Pac. J. Chem. Eng. 13:e2150. doi: 10.1002/apj.2150
Rabek, J. F., Canback, G., Lucky, J., and Ramby, B. (1976). Studies on the Photooxidation Mechanism of Polymers. 4. Effect of ultraviolet light (2537 A) on Solid PVC Particles Suspended in Different Liquids. J. Polym. Sci. 14, 1447–1462. doi: 10.1002/pol.1976.170140612
Rabek, J. F., Canback, G., and Ranby, B. (1977). Studies on the Photo-oxidation Mechanism of Polymers. 6. the role of commercial thermostabilizers in the photostability of Poly(viny1 Chloride). J. Polym. Sci. 21, 2211–2223. doi: 10.1002/app.1977.070210818
Rabek, J. F., and Ranby, B. (1974a). Studies on the photooxidation mechanism of polymers - 1. Photolysis and photooxidation of polystyrene. J. Polym. Sci. 12, 273–294. doi: 10.1002/pol.1974.170120204
Rabek, J. F., and Ranby, B. (1974b). Studies on the photooxidation mechanism of polymers - 2. the role of quinones as sensitizers in the photooxidative degradation of polystyrene. J. Polym. Sci. 12, 295–306. doi: 10.1002/pol.1974.170120204
Rabek, J. F., Shurh, Y. J., and Ranby, B. (1975). Studies of the Photooxidation Mechanism of Polymers - 3. Role of Tetrahydrofuran in the Photooxidative Degradation of Poly(Vinyl Chloride). J. Polym. Sci. 13, 1285–1295. doi: 10.1002/pol.1975.170130602
Raddadi, N., and Fava, F. (2019). Biodegradation of oil-based plastics in the environment: existing knowledge and needs of research and innovation. Sci. Total Environ. 679, 148–158. doi: 10.1016/j.scitotenv.2019.04.419
Raghavan, D. (1995). Characterization of biodegradable plastics. Polym. Plast. Technol. Eng. 34, 41–63. doi: 10.1080/03602559508017212
Raheem, A. B., Noor, Z. Z., Hassan, A., Hamid, M. K. A., Samsudin, S. A., and Sabeen, A. H. (2019). Current developments in chemical recycling of post-consumer polyethylene terephthalate wastes for new materials production: a review. J. Clean. Prod. 225, 1052–1064. doi: 10.1016/j.jclepro.2019.04.019
Rajandas, H., Parimannan, S., Sathasivam, K., Ravichandran, M., and Yin, L. S. (2012). A novel FTIR-ATR spectroscopy based technique for the estimation of low-density polyethylene biodegradation. Polym. Test. 31, 1094–1099. doi: 10.1016/j.polymertesting.2012.07.015
Ranby, B. (1978). Effects of photooxidation, singlet oxygen and biodegradation of polymers. Abstr. Pap. Am. Chem. Soc. 176, 48–48.
Rasel, S., and Sarkar, J. (2019). Manufacturing of Fabric by RECYCLING PLASTIC BOTTLES: AN ECOLOGICAL APPROACH Part 2: Manufacturing Process. Available at: http://textilefocus.com/manufacturing-fabric-recycling-plastic-bottles-ecological-approach-part-2-manufacturing-process/ (accessed November 13, 2020).
Reis, M., Albuquerque, M., Villano, M., and Majone, M. (2011). “Mixed culture processes for polyhydroxyalkanoate production from agro-industrial surplus/wastes as feedstocks,” in Comprehensive Biotechnology, eds M. Moo-Young, F. Fava, and S. Agathos (Burlington, VT: Academic Press), 669–683. doi: 10.1016/b978-0-08-088504-9.00464-5
Restrepo-Flórez, J., Bassi, A., and Thompson, M. R. (2014). Microbial degradation and deterioration of polyethylene - A review. Int. Biodeterior. Biodegrad. 88, 83–90. doi: 10.1016/j.ibiod.2013.12.014
Revel, M., Châtel, A., and Mouneyrac, C. (2018). Micro(nano) plastics: A threat to human health? Curr. Opin. Environ. Sci. Health. 1, 17–23. doi: 10.1016/j.coesh.2017.10.003
Rist, S., Carney, B., Hartmann, N. B., and Karlsson, T. M. (2018). A critical perspective on early communications concerning human health aspects of microplastics. Sci. Total Environ. 626, 720–726. doi: 10.1016/j.scitotenv.2018.01.092
Rodriguez, E. J., Marcos, B., and Huneault, M. A. (2016). Hydrolysis of polylactide in aqueous media. J. Appl. Polym. Sci. 133, 1–11. doi: 10.1002/app.44152
Rodriguez-Perez, S., Serrano, A., Pantión, A. A., and Alonso-Fariñas, B. (2018). Challenges of scaling-up PHA production from waste streams. A review. J. Environ. Manage. 205, 215–230. doi: 10.1016/j.jenvman.2017.09.083
Romão, W., Franco, M. F., Corilo, Y. E., Eberlin, M. N., Spinacé, M. A. S., and De Paoli, M. A. (2009). Poly (ethylene terephthalate) thermo-mechanical and thermo-oxidative degradation mechanisms. Polym. Degrad. Stab. 94, 1849–1859. doi: 10.1016/j.polymdegradstab.2009.05.017
Ronca, S. (2017). Chapter 10 - Polyethylene in Brydson’s Plastics Materials, 8th Edn. (Amsterdam: Elsevier), 247–278.
Rujnić-Sokele, M., and Pilipović, A. (2017). Challenges and opportunities of biodegradable plastics: a mini review. Waste Manage. Res. 35, 132–140. doi: 10.1177/0734242X16683272
Rummel, C. D., Jahnke, A., Gorokhova, E., Kühnel, D., and Schmitt-Jansen, M. (2017). Impacts of biofilm formation on the fate and potential effects of microplastic in the aquatic environment. Environ. Sci. Technol. Lett. 4, 258–267. doi: 10.1021/acs.estlett.7b00164
Sadeghi, G. M. M., Shamsi, R., and Sayaf, M. (2011). From Aminolysis Product of PET Waste to Novel Biodegradable Polyurethanes. J. Polym. Environ. 19, 522–534. doi: 10.1007/s10924-011-0283-7
Salim, S. Y., Kaplan, G. G., and Madsen, K. L. (2014). Air pollution effects on the gut microbiota: a link between exposure and inflammatory disease. Gut Microbes 5, 215–219. doi: 10.4161/gmic.27251
Santos, A. S. F., Agnelli, J. A. M., Trevisan, D. W., and Manrich, S. (2002). Degradation and stabilization of polyolefins from municipal plastic waste during multiple extrusions under different reprocessing conditions. Polym. Degrad. Stab. 77, 441–447. doi: 10.1016/s0141-3910(02)00101-5
Satti, S. M., Shah, Z., Luqman, A., Hasan, F., Osman, M., and Shah, A. A. (2020). Biodegradation of Poly(3-hydroxybutyrate) and Poly(3-hydroxybutyrate-co-3-hydroxyvalerate) by newly isolated Penicillium oxalicum SS2 in soil microcosms and partial characterization of extracellular depolymerase. Curr. Microbiol. 77, 1622–1636. doi: 10.1007/s00284-020-01968-7
Savelli, H., Beunen, R., Kalz, M., Ragas, A., and Belleghem, F. V. (2017). Solutions for global marine litter pollution. Curr. Opin. Environ. Sustain. 28, 90–99. doi: 10.1016/j.cosust.2017.08.009
Sazanov, Y. N., Florinsky, F. S., and Koton, M. M. (1979). Investigation of thermal and thermooxidative degradation of some polyimides containing oxyphenylene groups in the main chain. Eur. Polym. J. 15, 781–786. doi: 10.1016/0014-3057(79)90032-6
Scalenghe, R. (2018). Resource or waste? A perspective of plastics degradation in soil with a focus on end-of-life options. Heliyon 4, e00941. doi: 10.1016/j.heliyon.2018.e00941
Schliecker, G., Schmidt, C., Fuchs, S., and Kissel, T. (2003). Characterization of a homologous series of d, l -lactic acid oligomers; a mechanistic study on the degradation kinetics in vitro. Biomaterials 24, 3835–3844. doi: 10.1016/s0142-9612(03)00243-6
Scott, G. (1995). Initiation processes in polymer degradation. Polym. Degrad. Stab. 48, 315–324. doi: 10.1016/0141-3910(95)00090-9
Sebille, E., Aliani, S., Law, K. L., Maximenko, N., Alsina, J. M., Bagaev, A., et al. (2020). The physical oceanography of the transport of floating marine debris. Environ. Res. Lett. 15:2.
Serrano, D. P., Aguado, J., and Escola, J. M. (2012). Developing advanced catalysts for the conversion of polyolefinic waste plastics into fuels and chemicals. ACS Catal. 2, 1924–1941. doi: 10.1021/cs3003403
Shah, A. A., Hasan, F., Hameed, A., and Ahmed, S. (2008a). Biological degradation of plastics: a comprehensive review. Biotechnol. Adv. 26, 246–265. doi: 10.1016/j.biotechadv.2007.12.005
Shah, A. A., Hasan, F., Akhter, J. I., Hameed, A., and Ahmed, S. (2008b). Degradation of polyurethane by novel bacterial consortium isolated from soil. Ann. Microbiol. 58, 381–386. doi: 10.1007/BF03175532
Shah, T. V., and Vasava, D. V. (2019). A glimpse of biodegradable polymers and biomedical applications. E Polymers 19, 385–410. doi: 10.1515/epoly-2019-0041
Shamsi, R., Abdouss, M., Sadeghi, G. M. M., and Taromi, F. A. (2009). Synthesis and characterization of novel polyurethanes based on aminolysis of poly(ethylene terephthalate) wastes, and evaluation of their thermal and mechanical properties. Polym. Int. 58, 22–30. doi: 10.1002/pi.2488
Sharma, M., Dangi, P., and Choudhary, M. (2014). Actinomycetes: source, identification, and their applications. Int. J. Curr. Microbiol. Appl. Sci. 3, 801–832.
Sharma, S. S., and Batra, V. S. (2020). Production of hydrogen and carbon nanotubes via catalytic thermo–chemical conversion of plastic waste: review. J. Chem. Technol. Biotechnol. 95, 11–19. doi: 10.1002/jctb.6193
Sheavly, S. B., and Register, K. M. (2007). Marine debris & plastics: environmental concerns, sources, impacts and solutions. J. Polym. Environ. 15, 301–305.
Shenoy, A. V., and Saini, D. R. (1996). Thermoplastic Melt Rheology and Processing. New York, NY: CRC Press.
Shenoy, A. V., Saini, D. R., and Nadkarni, V. M. (1983). Estimation of the melt rheology of polymer waste from melt flow index. Polymer 24, 722–728. doi: 10.1016/0032-3861(83)90010-1
Shimpi, N., Borane, M., Mishra, S., and Kadam, M. (2012). Biodegradation of Polystyrene (PS)-Poly (lactic acid) (PLA) Nanocomposites using Pseudomonas aeruginosa. Macromol. Res. 20, 181–187. doi: 10.1007/s13233-012-0026-1
Shirke, A. N., White, C., Englaender, J. A., Zwarycz, A., Butterfoss, G. L., Linhardt, R. J., et al. (2018). Stabilizing leaf and branch compost cutinase (LCC) with glycosylation: mechanism and effect on PET hydrolysis. Biochemistry 57, 1190–1200. doi: 10.1021/acs.biochem.7b01189
Shubhra, Q. T. H. (2013). Mechanical properties of polypropylene composites a review mechanical properties of polypropylene composites: a review. J. Thermoplast. Compos. Mater. 26, 362–391. doi: 10.1177/0892705711428659
Shukla, S. R., and Kulkarni, K. S. (2002). Depolymerization of poly(ethylene terephthalate) waste. J. Appl. Polym. Sci. 85, 1765–1770. doi: 10.1002/app.10714
Siddiqui, M. N., Redhwi, H. H., and Achilias, D. S. (2012). Recycling of poly(ethylene terephthalate) waste through methanolic pyrolysis in a microwave reactor. J. Anal. Appl. Pyrolysis 98, 214–220. doi: 10.1016/j.jaap.2012.09.007
Singh, B., and Sharma, N. (2008). Mechanistic implications of plastic degradation. Polym. Degrad. Stab. 93, 561–584. doi: 10.1016/j.polymdegradstab.2007.11.008
Singh, L., and Wahid, Z. A. (2015). Methods for enhancing bio-hydrogen production from biological process: a review. J. Ind. Eng. Chem. 21, 70–80. doi: 10.1016/j.jiec.2014.05.035
Sinha, V., Patel, M. R., and Patel, J. V. (2010). PET waste management by chemical recycling: a review. J. Polym. Environ. 18, 8–25. doi: 10.1007/s10924-008-0106-7
Siracusa, V., Lotti, N., Munari, A., and Rosa, M. D. (2015). Poly(butylene succinate) and poly(butylene succinate-co-adipate) for food packaging applications: gas barrier properties after stressed treatments. Polym. Degrad. Stab. 119, 35–45. doi: 10.1016/j.polymdegradstab.2015.04.026
Sivan, A., Szanto, M., and Pavlov, V. (2006). Biofilm development of the polyethylene-degrading bacterium Rhodococcus ruber. Appl. Microb. Cell Physiol. 72, 346–352. doi: 10.1007/s00253-005-0259-4
Soni, R., Zaidi, M., Shouche, Y., and Goel, R. (2008). Comparative biodegradation of HDPE and LDPE using an indigenously developed microbial consortium. J. Microbiol. Biotechnol. 18, 477–482.
Sowmya, H. V., Ramalingappa, Krishnappa, M., and Thippeswamy, B. (2014). Degradation of polyethylene by Trichoderma harzianum—SEM, FTIR, and NMR analyses. Environ. Monit. Assess. 186, 6577–6586. doi: 10.1007/s10661-014-3875-6
Spoerk, M., Holzer, C., and Gonzalez-Gutierrez, J. (2020). Material extrusion-based additive manufacturing of polypropylene: a review on how to improve dimensional inaccuracy and warpage. J. Appl. Polym. Sci. 137, 1–16. doi: 10.1002/app.48545
Su, S., Kopitzky, R., Tolga, S., and Kabasci, S. (2019). Polylactide (PLA) and its blends with poly(butylene succinate) (PBS): a brief review. Polymers 11:1193. doi: 10.3390/polym11071193
Sudesh, K., and Iwata, T. (2008). Sustainability of biobased and biodegradable plastics. Clean 36, 433–442. doi: 10.1002/clen.200700183
Sukkhum, S., Tokuyama, S., and Kitpreechavanich, V. (2009). Development of fermentation process for PLA-degrading enzyme production by a new thermophilic Actinomadura sp. T16-1. Biotechnol. Bioprocess Eng. 14, 302–306. doi: 10.1007/s12257-008-0207-0
Sun, J., Liu, D., Young, R. P., Cruz, A. G., Isern, N. G., Schuerg, T., et al. (2018). Solubilization and upgrading of high polyethylene terephthalate loadings in a low-costing bifunctional ionic liquid. ChemSusChem 11, 781–792. doi: 10.1002/cssc.201701798
Syranidou, E., Karkanorachaki, K., Amorotti, F., Repouskou, E., Kroll, K., Kolvenbach, B., et al. (2017). Development of tailored indigenous marine consortia for the degradation of naturally weathered polyethylene films. PLoS One 12:e0183984. doi: 10.1371/journal.pone.0183984
Thiounn, T., and Smith, R. C. (2020). Advances and approaches for chemical recycling of plastic waste. J. Polym. Sci. 58, 1327–1364. doi: 10.1002/pol.20190261
Thompson, R. C., Swan, S. H., Moore, C. J., and Saal, F. S. V. (2009). Our plastic age. Philos. Trans. R. Soc. Lond. B Biol. 364, 1973–1976. doi: 10.1098/rstb.2009.0054
Thybaut, J. W., and Marin, G. B. (2016). chapter two - multiscale aspects in hydrocracking: from reaction mechanism over catalysts to kinetics and industrial application. Adv. Catal. 59, 109–238. doi: 10.1016/bs.acat.2016.10.001
Tokiwa, Y., Calabia, B. P., Ugwu, C. U., and Aiba, S. (2009). Biodegradability of plastics. Int. J. Mol. Sci. 10, 3722–3742. doi: 10.3390/ijms10093722
Troev, K., Grancharov, G., Tsevi, R., and Gitsov, I. (2003). A novel catalyst for the glycolysis of poly(ethylene terephthalate). J. Appl. Polym. Sci. 90, 1148–1152. doi: 10.1002/app.12711
Turra, A., Manzano, A. B., Dias, R. J. S., Mahiques, M. M., Barbosa, L., Balthazar-Silva, D., et al. (2014). Three-dimensional distribution of plastic pellets in sandy beaches: Shifting paradigms. Sci. Rep. 4:4435. doi: 10.1038/srep04435
Ügdüler, S., Geem, K. M. V., Roosen, M., Delbeke, E. I. P., and Meester, S. D. (2020). Challenges and opportunities of solvent-based additive extraction methods for plastic recycling. Waste Manage. 104, 148–182. doi: 10.1016/j.wasman.2020.01.003
Uscátegui, Y. L., Arévalo, F. R., Díaz, L. E., Cobo, M. I., and Valero, M. F. (2016). Microbial degradation, cytotoxicity and antibacterial activity of polyurethanes based on modified castor oil and polycaprolactone. J. Biomater. Sci. Polym. Ed. 27, 1860–1879. doi: 10.1080/09205063.2016.1239948
Uttaravalli, A. N., Dinda, S., and Gidla, B. R. (2020). Scientific and engineering aspects of potential applications of post-consumer (waste) expanded polystyrene: a review. Process Saf. Environ. Prot. 137, 140–148. doi: 10.1016/j.psep.2020.02.023
Uzoejinwa, B. B., He, X., Wang, S., Abomohra, A. E., Hu, Y., and Wang, Q. (2018). Co-pyrolysis of biomass and waste plastics as a thermochemical conversion technology for high-grade biofuel production: recent progress and future directions elsewhere worldwide. Energy Convers. Manage. 163, 468–492. doi: 10.1016/j.enconman.2018.02.004
Valh, J. V., Vončina, B., Lobnik, A., Zemljič, L. F., Škodič, L., and Vajnhandl, S. (2019). Conversion of polyethylene terephthalate to high-quality terephthalic acid by hydrothermal hydrolysis: the study of process parameters. Text. Res. J. 90, 1446–1461. doi: 10.1177/0040517519893714
van Franeker, J. A. (1985). Plastic ingestion in the North Atlantic fulmar. Mar. Pollut. Bull. 16, 367–369. doi: 10.1016/0025-326x(85)90090-6
Venkatachalam, S., Shilpa, G., Jayprakash, V., Prashant, R., Krishna, R., and Anil, K. (2012). “Degradation and Recyclability of Poly (Ethylene Terephthalate),” in Polyester, ed. H. E.-D. Saleh (London: InTechOpen). doi: 10.5772/48612
Vert, M., Doi, Y., Hellwich, K., Hess, M., Hodge, P., Kubisa, P., et al. (2014). Terminology for biorelated polymers and applications. Chem. Int. 33:25. doi: 10.1515/ci.2011.33.2.25c
Walker, T. R. (2018). Drowning in debris: solutions for a global pervasive marine pollution problem. Mar. Pollut. Bull. 126:338. doi: 10.1016/j.marpolbul.2017.11.039
Wang, Q., Yao, X., Tang, S., Lu, X., Zhang, X., and Zhang, S. (2012). Urea as an efficient and reusable catalyst for the glycolysis of poly(ethylene terephthalate) wastes and the role of hydrogen bond in this process. Green Chem. 14, 2559–2566. doi: 10.1039/c2gc35696a
Wei, R., and Zimmermann, W. (2017). Microbial enzymes for the recycling of recalcitrant petroleum–based plastics: how far are we? Microb. Biotechnol. 10, 1308–1322. doi: 10.1111/1751-7915.12710
Welden, N. A., and Cowie, P. R. (2017). Degradation of common polymer ropes in a sublittoral marine environment. Mar. Pollut. Bull. 118, 248–253. doi: 10.1016/j.marpolbul.2017.02.072
Wiles, D. M., and Scott, G. (2006). Polyolefins with controlled environmental degradability. Polym. Degrad. Stab. 91, 1581–1592. doi: 10.1016/j.polymdegradstab.2005.09.010
Williams, A. T. (1999). ‘Marine debris: sources, impacts, and solutions’. Geogr. J. 233–233. doi: 10.2307/3060422
Worm, B., Lotze, H. K., Jubinville, I., Wilcox, C., and Jambeck, J. (2017). Plastic as a persistent marine pollutant. Annu. Rev. Environ. Resour. 42, 1–26. doi: 10.1146/annurev-environ-102016-060700
Wright, S. L., Thompson, R. C., and Galloway, T. S. (2013). The physical impacts of microplastics on marine organisms: a review. Environ. Pollut. 178, 483–492. doi: 10.1016/j.envpol.2013.02.031
Wysocki, I. T., and Billon, P. L. (2019). Plastics at sea: treaty design for a global solution to marine plastic pollution. Environ. Sci. Policy 100, 94–104. doi: 10.1016/j.envsci.2019.06.005
Xi, G., Lu, M., and Sun, C. (2005). ‘Study on depolymerization of waste polyethylene terephthalate into monomer of bis(2-hydroxyethyl terephthalate). Polym. Degrad. Stab. 87, 117–120. doi: 10.1016/j.polymdegradstab.2004.07.017
Xiao, L., Zhao, Y., Jin, B., Zhang, Q., Chai, Z., and Peng, R. (2020). Synthesis of novel ultraviolet stabilizers based on [60]fullerene and their effects on photo-oxidative degradation of polystyrene. Fullerenes Nanotubes Carbon Nanostruct. 28, 465–473. doi: 10.1080/1536383X.2019.1703695
Yadav, B., Pandey, A., Kumar, L. R., and Tyagi, R. D. (2020). Bioconversion of waste (water)/residues to bioplastics- A circular bioeconomy approach. Bioresour. Technol. 298, 122584. doi: 10.1016/j.biortech.2019.122584
Yamada-Onodera, K., Mukumoto, H., and Katsuyaya, Y. (2001). Degradation of polyethylene by a fungus, Penicillium simplicissimum YK. Polym. Degrad. Stab. 72, 323–327. doi: 10.1016/s0141-3910(01)00027-1
Yang, J., Xia, Z., Kong, F., and Ma, X. (2010). The effect of metal catalyst on the discoloration of poly(ethylene terephthalate) in thermo-oxidative degradation. Polym. Degrad. Stab. 95, 53–58. doi: 10.1016/j.polymdegradstab.2009.10.009
Yang, J., Yang, Y., Wu, W., Zhao, J., and Jiang, L. (2014). Evidence of polyethylene biodegradation by bacterial strains from the guts of plastic-eating Waxworms. Environ. Sci. Technol. 48, 13776–13784. doi: 10.1021/es504038a
Yang, Y., Lu, Y., Xiang, H., Xu, Y., and Li, Y. (2002). Study on methanolytic depolymerization of PET with supercritical methanol for chemical recycling. Polym. Degrad. Stab. 75, 185–191. doi: 10.1016/s0141-3910(01)00217-8
Yanto, D. H. Y., Krishanti, N. P. R. A., Ardiati, F. C., Anita, S. H., Nugraha, I. K., Sari, F. P., et al. (2019). Biodegradation of styrofoam waste by ligninolytic fungi and bacteria. Earth Environ. Sci. 308, 012001. doi: 10.1088/1755-1315/308/1/012001
Yuan, J., Ma, J., Sun, Y., Zhou, T., Zhao, Y., and Yu, F. (2020). Microbial degradation and other environmental aspects of microplastics/plastics. Sci. Total Environ. 715, 136968. doi: 10.1016/j.scitotenv.2020.136968
Yue, Q. F., Wang, C. X., Zhang, L. N., Ni, Y., and Jin, Y. X. (2011). Glycolysis of poly(ethylene terephthalate) (PET) using basic ionic liquids as catalysts. Polym. Degrad. Stab. 96, 399–403. doi: 10.1016/j.polymdegradstab.2010.12.020
Zanela, T. M. P., Muniz, E. C., and Almeida, C. A. P. (2018). Chemical recycling of poly(Ethylene terephthalate) (PET) by alkaline hydrolysis and catalyzed glycolysis. Orbital 10, 226–233. doi: 10.17807/orbital.v10i3.1104
Keywords: plastic and microplastic pollution, biodegradation, marine debris, actinobacteria, chemical recycling, bioplastic production
Citation: Oliveira J, Belchior A, da Silva VD, Rotter A, Petrovski Ž, Almeida PL, Lourenço ND and Gaudêncio SP (2020) Marine Environmental Plastic Pollution: Mitigation by Microorganism Degradation and Recycling Valorization. Front. Mar. Sci. 7:567126. doi: 10.3389/fmars.2020.567126
Received: 29 May 2020; Accepted: 30 October 2020;
Published: 17 December 2020.
Edited by:
Conxita Avila, University of Barcelona, SpainReviewed by:
Sílvia C. Gonçalves, Polytechnic Institute of Leiria, PortugalElisenda Balleste, University of Barcelona, Spain
Copyright © 2020 Oliveira, Belchior, da Silva, Rotter, Petrovski, Almeida, Lourenço and Gaudêncio. This is an open-access article distributed under the terms of the Creative Commons Attribution License (CC BY). The use, distribution or reproduction in other forums is permitted, provided the original author(s) and the copyright owner(s) are credited and that the original publication in this journal is cited, in accordance with accepted academic practice. No use, distribution or reproduction is permitted which does not comply with these terms.
*Correspondence: Susana P. Gaudêncio, cy5nYXVkZW5jaW9AZmN0LnVubC5wdA==; Juliana Oliveira, anBjLm9saXZlaXJhQGNhbXB1cy5mY3QudW5sLnB0