- 1College of Marine Science, University of South Florida, St. Petersburg, FL, United States
- 2Shell Health – Americas, Shell Oil Company, Houston, TX, United States
- 3Nature Coast Biological Station, University of Florida, Cedar Key, FL, United States
- 4Gulf States Marine Fisheries Commission, Ocean Springs, MS, United States
- 5Division of Coastal Sciences, The University of Southern Mississippi, Ocean Springs, MS, United States
- 6Florida Fish and Wildlife Research Institute, St. Petersburg, FL, United States
- 7Alabama Department of Conservation and Natural Resources, Dauphin Island, AL, United States
- 8Department of Biology, University of Louisiana at Lafayette, Lafayette, LA, United States
The 2010 Deepwater Horizon (DWH) oil blowout in the Gulf of Mexico began on April 20, originating in the deep sea 66 km off the Louisiana coast. By early June, DWH oil had spread to coastal Louisiana, Mississippi, Alabama and western Florida. An estimated 2,113 km of shoreline were oiled, making DWH the largest marine oil spill in global history by length of affected shoreline. Additionally, a series of oil spill response measures were deployed, including diversions of Mississippi River discharge to forestall oil coming ashore, and the establishment of large-scale fishery closures, with both affecting coastal resources to varying degrees. Here, we review published studies and describe additional analyses evaluating long-term impacts of DWH on coastal/nearshore biological resources. We assembled time-series data collected by state, federal and academic partners on population abundance and environmental conditions to evaluate species and community change. Our study focused on plankton, invertebrates, fishes and dolphins, and 13 “key species” were selected to conduct semi-quantitative vulnerability-resilience (V-R) analyses. At one extreme, early life stages of Gulf Menhaden (Brevoortia patronus) were not affected due to seasonal spawning and larval development preceding the spill. In contrast, demographically independent populations of the common Bottlenose Dolphin, (Tursiops truncatus) suffered a variety of severe and ongoing health effects owing to oil exposure. Virtually all of the heavily oiled salt marsh habitat was in Louisiana, with the majority occurring in Barataria Bay. Multispecies trawl survey abundances declined post-DWH throughout eastern coastal Louisiana but remained stable elsewhere. A regime shift in composition of Barataria Bay trawl survey catches occurred during and following the spill, the persistence of which was associated with long-term reductions in average salinity and increases in water clarity. In some cases, fishery closures were associated with measurable but ephemeral increases in abundance of some targeted and bycatch species. Freshwater flooding of marshes was ineffective in preventing coastal oiling and severely affected benthic euryhaline resources including Eastern Oyster (Crassostrea virginica) and Marsh Periwinkle (Littoraria irrorata). The flooding response measure experiment also indicates the directionality of impacts that further planned water diversions may have on ecological communities of lower Mississippi River basins.
Introduction
The Deepwater Horizon (DWH) oil spill began on April 20, 2010, and released a total of ∼750 million liters of oil over an 87-day period, which formed a surface expression encompassing over 149 thousand km2 in the northern Gulf of Mexico (GoM; Figure 1; MacDonald et al., 2015). By April 30, the first coastal landfall of the oil had occurred in the Mississippi River Delta of Louisiana, and by early June, had spread to the coasts of Mississippi, Alabama and the Florida Panhandle (Figure 1; Deepwater Horizon Natural Resource Damage Assessment Trustees, 2016; Turner et al., 2019a). Once ashore, DWH oil was found on an estimated 2,113 km of shoreline, including beaches and in wetlands, making DWH the largest marine oil spill in global history by length of shoreline oiled (Nixon et al., 2016; Figure 1). Virtually all of the heavily oiled wetlands habitat (>99%) was in Louisiana, with the majority of heavily oiled shoreline occurring in and around Barataria Bay (Nixon et al., 2016; Figure 1B). Coastal margins, and particularly salt marshes, are critical nursery and feeding areas for a wide variety of estuarine and marine organisms. Hence there was concern that oiling of these areas would have both acute and potentially chronic impacts on many species of economic and environmental importance and degrade the broader array of ecosystem services they provide (Grabowski et al., 2012; National Research Council, 2013; Deepwater Horizon Natural Resource Damage Assessment Trustees, 2016; Powers et al., 2017a; Turner et al., 2019a; Figure 1).
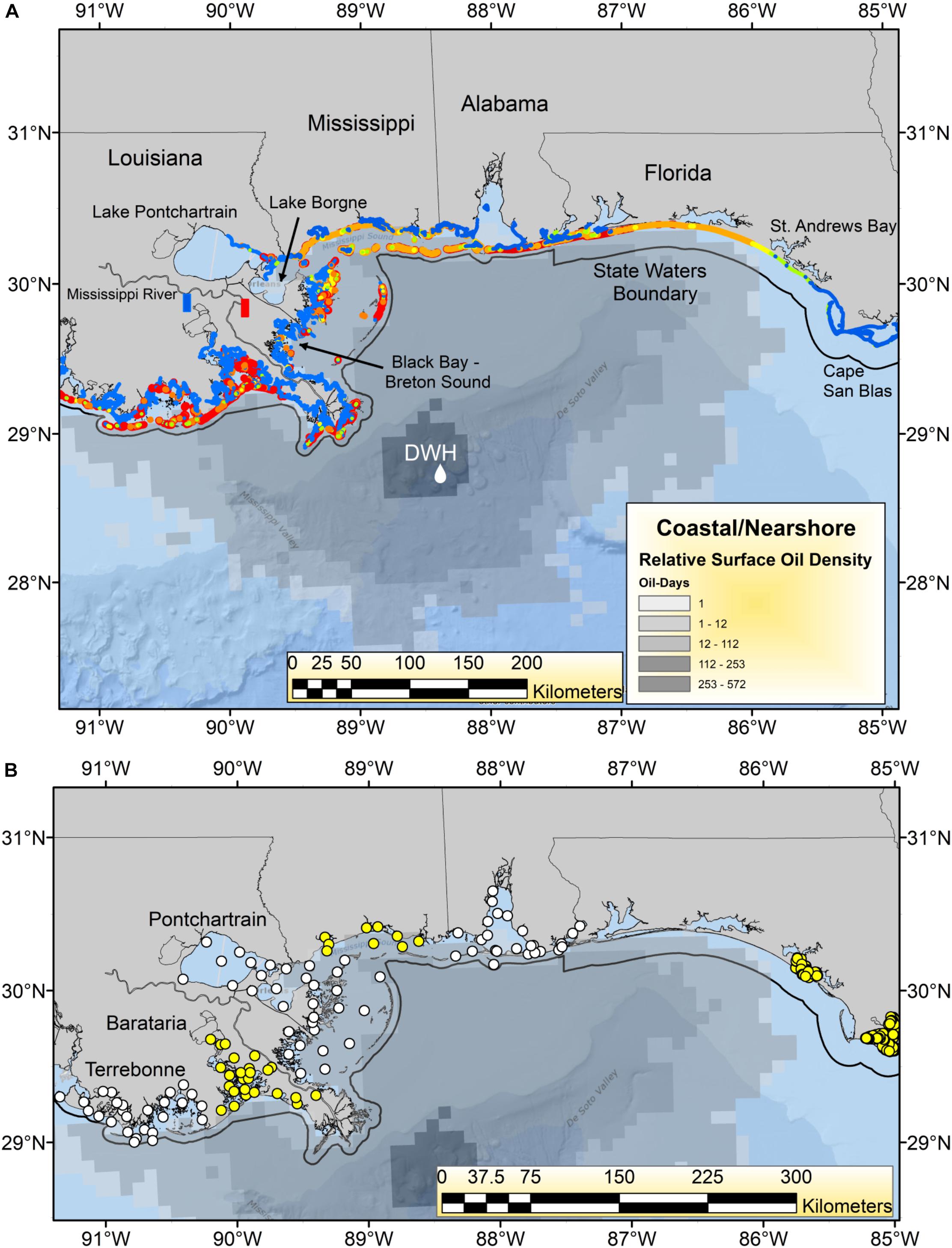
Figure 1. (A) Geographic footprint of surface oil expression [number of “oil days” i.e., days oil was present at each pixel of the oil surface multiplied by the relative oil thickness (Murawski et al., 2014)] and shoreline oiling relative concentrations for the Deepwater Horizon (DWH) oil spill in 2010. Shoreline oiling colors indicate maximum levels observed from the National Oceanic and Atmospheric Administration’s SCAT (Shoreline Cleanup Assessment Technique) results: (https://catalog.data.gov/dataset/deepwater-horizon-mc252-response-data-from-the-environmental-resource-management-application-er9c019). Color codings are: blue = no oiling, yellow = light, orange = medium, red = heavy oiling. The boundaries of state territorial waters are plotted as a black line. The Mississippi River diversions at Davis Pond (blue rectangle) and Caernarvon (red rectangle) are identified. (B) Locations of most recent trawl survey stations for each state and region. Alternating yellow and white circles indicate identity of adjacent regions or states. Three estuarine systems in coastal Louisiana (Pontchartrain, Barataria, and Terrebonne) are identified.
To mitigate the direct effects of oil on living resources, several oil spill response measures were deployed during the spill to remove oil from the marine environment and to impede its progression into coastal habitats. These measures included mechanical oil recovery by skimming in offshore and nearshore waters, and along shoreline habitats, burning of corralled oil at the surface [generally 5 km (3 miles) offshore and greater], and the use of chemical dispersants, both at the sea surface in offshore waters and in novel subsea dispersant injection (SSDI) at the wellhead (Deepwater Horizon Natural Resource Damage Assessment Trustees, 2016; National Academies of Science, Engineering, and Medicine [NASEM], 2020; Figure 2). Other response measures were also deployed to prevent or obstruct oil from reaching environmentally sensitive and economically significant shorelines, including wetlands. These included the deployment of sorbent booms, the construction of protective sand berms (Martínez et al., 2012; Suir et al., 2016), and increasing the discharge of freshwater from the mainstem Mississippi River into marshlands through freshwater diversion gates at Davis Pond (leading to Barataria Bay) and Caernarvon (leading to Black Bay, Louisiana; Figure 1; de Mutsert and Cowan, 2012; O’Connor et al., 2016; White et al., 2018; Turner et al., 2019b), though the latter two response measures are not considered standard practice. Emergency managers also instituted large-scale fishery closures of state and federal waters to commercial and recreational fishing (Gohlke et al., 2011; Ylitalo et al., 2012; Cockrell et al., 2019), at their maximum extending 217,000 km2 in federal waters and including all state waters from affected areas.
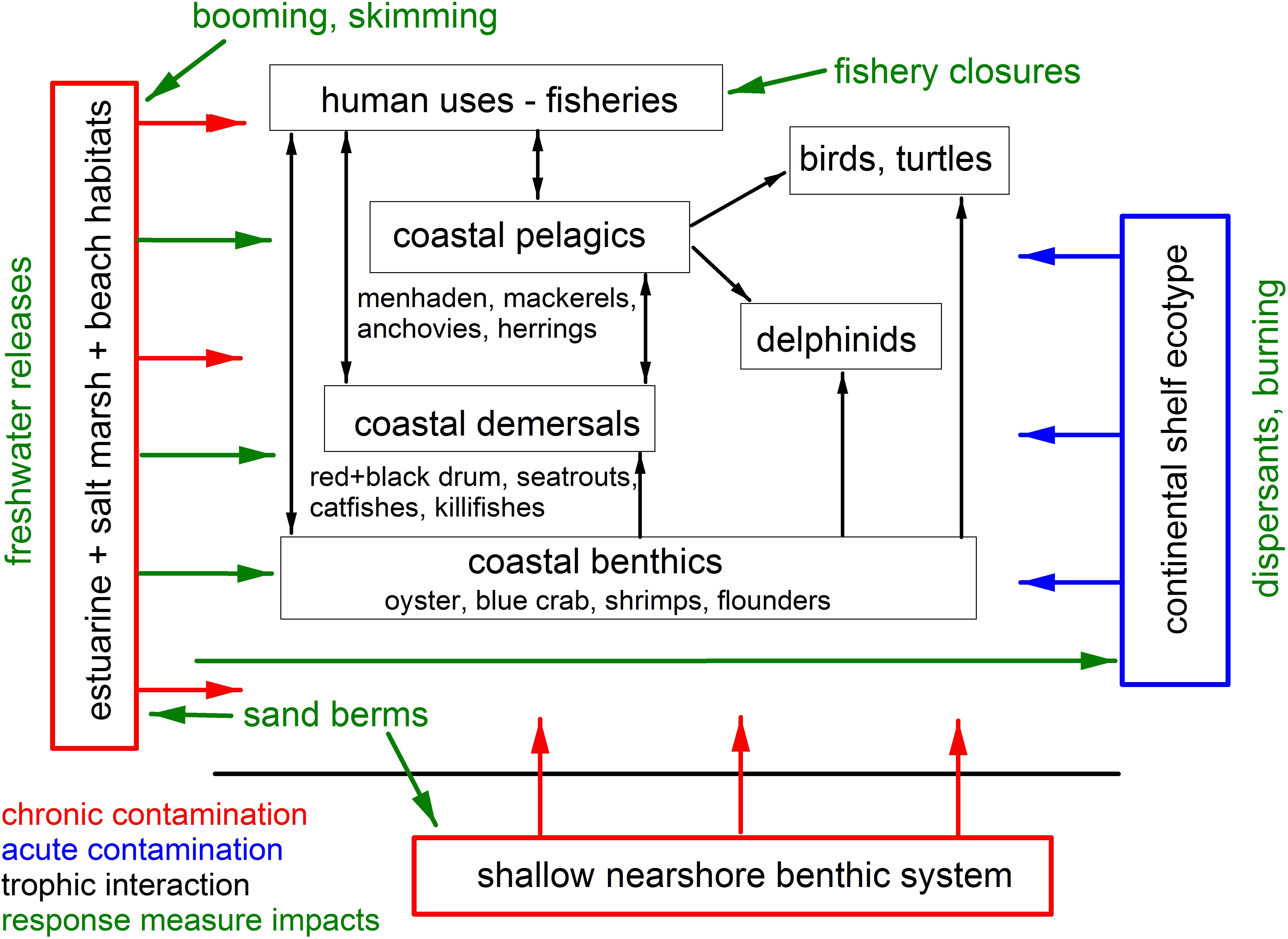
Figure 2. Schematic of the source (in blue), pollutant reservoirs (in red) and impacted species groups, and their interrelationships (black arrows) for the Deepwater Horizon oil spill affecting coastal and nearshore resources in the northern GoM. Green arrows indicate the directionality of potential impacts of oil spill response measures on resource species.
The combination of the occurrence and concentration of oil in coastal habitats and the use of some spill response measures resulted in a series of complex interactions affecting biota (Figure 2) but their full impacts remain unresolved. For example, while common Bottlenose Dolphins (Tursiops truncatus) tolerate exposures to low salinities for short periods of time, prolonged exposures caused by freshwater releases can result in adverse biological and physiological responses. In Barataria Bay, dolphins use areas with salinities greater than 11 ppt but avoid areas with salinities below about 5 ppt (Hornsby et al., 2017; White et al., 2018). Additionally, despite the use of response measures and extensive shoreline cleanup efforts, significant reservoirs of DWH oil remained in some coastal areas, with oil concentrations an order of magnitude higher than pre-spill baselines in Louisiana marshes even 8 years after the spill (Duan et al., 2017; Turner et al., 2019a). Initial distributions of oil in affected marshlands became “smeared” through storms, tidal flushing and periodic flooding, resulting in an expanding area of influence. Because some DWH oil is now sequestered in anoxic sediments, levels will likely remain significantly above background for decades (Reddy et al., 2002; Turner et al., 2019a).
Numerous previous investigations have evaluated the direct effects of DWH oiling and indirect effects of response measures on various coastal and nearshore biota including microbial communities (Kostka et al., 2011), marsh vegetation (Hester et al., 2016), marsh invertebrates (e.g., McCall and Pennings, 2012; Mishra et al., 2012; Silliman et al., 2012; Brunner et al., 2013; Pennings et al., 2014, 2016; Rozas et al., 2014; Fleeger et al., 2015, 2018; Deis et al., 2017; Powers et al., 2017a; Zengel et al., 2017), nearshore decapods and fishes (Fodrie and Heck, 2011; Moody et al., 2013; Fodrie et al., 2014; van der Ham and de Mutsert, 2014; Able et al., 2015; Zengel et al., 2016; Martin, 2017), terrestrial and coastal bird populations (Haney et al., 2014; Walter et al., 2014; Bonisoli-Alquati et al., 2016; Deepwater Horizon Natural Resource Damage Assessment Trustees, 2016), and marine turtles and mammals (Lane et al., 2015; Venn-Watson et al., 2015a; Aichinger-Dias et al., 2017; Kellar et al., 2017; Smith et al., 2017; Wallace et al., 2017; Frasier et al., 2020). Syntheses of ecological outcomes of DWH were initiated within 1 year of the spill, and have been published intermittently hence (Fodrie and Heck, 2011; Fodrie et al., 2014; Beyer et al., 2016; Deepwater Horizon Natural Resource Damage Assessment Trustees, 2016; Murawski et al., 2016; Powers and Scyphers, 2016; Rabalais and Turner, 2016; Baker et al., 2017; Powers et al., 2017b,c; Rouhani et al., 2017). Often in the cases of marine oil spills, the full measure of environmental impacts is not apparent in the immediate years following the spill, and particularly when oil persists in the environment or long-lived animal populations have been affected (Peterson et al., 2003, 2012; Esler et al., 2018). It is thus appropriate – on the decadal anniversary of DWH – to yet again synthesize and interpret information regarding longer-term impacts of the spill on coastal living marine resources, and to recognize that this will not be the last synthesis as research on the effects of this spill are likely to continue for many years.
The specific goals of our study, which are generally consistent with the three other “ecotype” summaries published in this Frontiers in Marine Science Research Topic on Vulnerability and Resilience of Marine Ecosystems Affected by the Deepwater Horizon Oil Spill, were to:
(a) identify relevant information from previous studies and long-term data sets to more fully analyze and interpret DWH impacts,
(b) summarize pre- and post-oil spill patterns in the abundance, composition and dynamics of species for which these data are available,
(c) construct a conceptual model of species interactions and how DWH-caused oiling and spill response measures, specifically freshwater diversions affected species and communities,
(d) identify several northern GoM coastal and nearshore “key” species and evaluate their vulnerability of those species to effects of DWH and their respective resiliency and recovery potentials,
(e) provide commentary on the utility of existing ecological monitoring and modeling programs, particularly in relation to background variability and co-stressors, and,
(f) recommend additional research to detect oil spill effects and evaluate the impacts of oil spill response measures (e.g., freshwater diversions and berm construction) in the event of future DWH-scale oil spills.
Synthesis Approach
Our synthesis relied heavily on the extensive record of published literature concerning DWH effects on nearshore biota and, as well, our re-analyses of long-term data sets. While not all biota in the coastal and nearshore areas of the northern GoM are evaluated in long-term continuous or intermittent monitoring programs, there are ongoing programs to assess the status of fishes and some invertebrate species for fisheries management. Each of the affected states maintains a variety of fishery-independent sampling programs including bottom trawling surveys for groundfish and shrimp, gill net sampling for large fishes and, in most cases, quadrat-based surveys of oyster populations (Table 1). Even within a gear type, the methods were not consistent across states. For this reason, we did not conduct analyses of pooled data across jurisdictions (although this has previously been done, see O’Connell et al., 2019). The time series’ lengths and availability of data to independent investigators varies by state varies as well (Table 1). Importantly, the most relevant surveys spanned the time previous to and after the DWH spill and thus provide information required to assess ecosystem status coincident with the spill due to their continuity and establishment of baseline characteristics. In most cases a suite of environmental measurements (temperature, salinity, water clarity, etc.) was obtained concurrent with biological sampling and thus provided information with which to evaluate various environmental factors that may be driving biological responses. While fishery-independent surveys are primarily directed to economically or ecologically significant species, sampling gears, and particularly trawls, catch a wide variety of species (in the case of the Louisiana trawl survey >300 species, Supplementary Table 1, and similar species lists for Mississippi and Alabama, O’Connell et al., 2019). Thus, these surveys not only serve as important sources of information for individual species; but also allow for community-level analyses describing changes in species composition and abundance, (beta-diversity). We undertook both relation to the DWH spill.
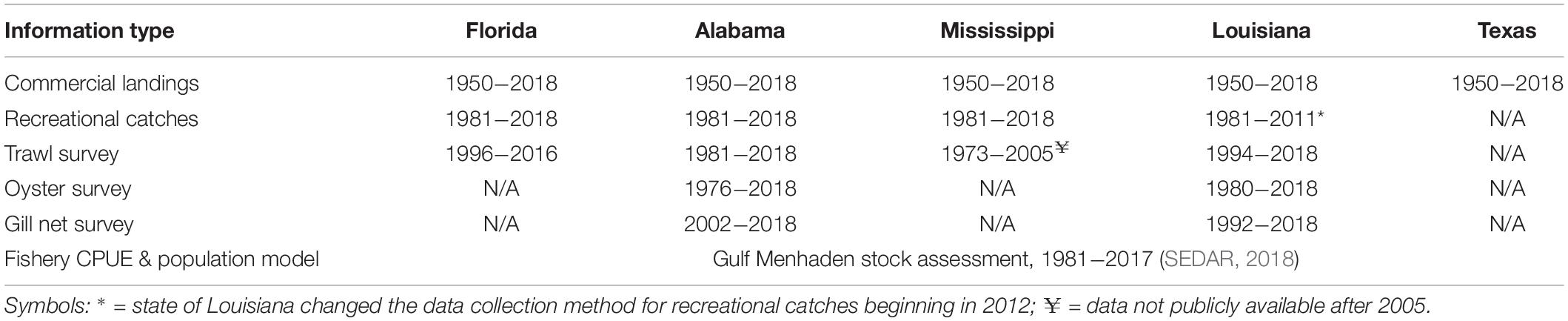
Table 1. Data sources used to evaluate the status of living resources in coastal and nearshore areas in the northern GoM affected by the Deepwater Horizon oil spill.
Data Sets
Primary data sets used in these analyses include fishery landings information (e.g., from commercial fisheries) and catch data from recreational fishing surveys (Table 1 and Supplementary Figures 1–7). Additionally, fishery-independent survey data collected from ongoing trawl, gill net and oyster sampling programs were evaluated (Table 1). For fishery-independent surveys conducted in Florida, we limited our data selection to trawl survey stations west of 85°W longitude to encompass areas potentially impacted by the DWH spill (Figure 1). Some Florida trawl data were collected intermittently from Pensacola Bay, near the Florida-Alabama border, but the time series information analyzed were primarily from St. Andrews Bay (Panama City, FL) and the area west of 85° W. longitude, near Cape San Blas (Figure 1).
To evaluate a wider set of species impacts of the spill and response measures (i.e., freshwater diversions), we also summarized results of studies using data collected from various sources, including:
• Marine mammal stranding information,
• Marine mammal aerial and vessel surveys,
• Natural Resource Damage Assessment (NRDA)-supported post-DWH surveys (Deepwater Horizon Natural Resource Damage Assessment Trustees, 2016),
• Post-DWH supported surveys of various biota supported by GoMRI and other research programs.
Fishery-independent sampling surveys supported by the states are conducted during multiple sampling intervals within the year. Because the operational details of these programs varied between states, we chose to evaluate an overall annual abundance signal by combining station and set information across sampling intervals within years. The relative abundance of various species was standardized to catches (numbers) of animals encountered per gear deployment (e.g., per trawl haul, gill net set, etc.). For gill net data, the various state surveys use graduated mesh-sizes of the panels comprising an individual net. We combined the panel-by-panel data into an overall catch per set index. To account for the variance of fish catch data from surveys we transformed data by either applying an inverse-variance weighting technique to mean catches per set, or by applying a natural log transformation (ln + 1) to numbers set–1.
Because of the large number of individual species occurring in coastal waters affected by the spill and the variety of species sampled (e.g., Supplementary Table 1) we chose two strategies to evaluate and summarize both the impacts of the spill, its associated response measures, and the recovery potential of affected species and ecosystems. First, we conducted in-depth analyses of a series of “key species” based on their economic or ecological significance in coastal areas affected by the spill. Second, we developed multispecies/multivariate analyses of the communities observed in multispecies sampling (e.g., trawling surveys) to evaluate the potential for systematic change or regime shifts in composition and abundance coincident in time with the DWH spill and its aftermath.
While there is no single accepted methodology to select “key species” we chose 13 species or taxonomic groups for in-depth evaluation due to their importance to commercial and recreational fisheries, representativeness of trophic levels from benthic invertebrates to marine mammals, or designation as species of particular importance to ecosystem function (Deepwater Horizon Natural Resource Damage Assessment Trustees, 2016; McCann et al., 2017). A similar approach was undertaken by de Mutsert and Cowan (2012) in evaluating the effects of river diversions into the Pontchartrain basin of Louisiana, and who selected four of the same species as we did to evaluate in detail as “focus species.” Our selection of key species also spans the range of interacting species groups and processes depicted in our schematic of coastal and nearshore effects of DWH (Figure 2). The 13 key species/groups chosen were: Eastern Oyster Crassostrea virginica, Blue Crab Callinectes sapidus, White Shrimp Litopenaeus setiferus, Brown Shrimp Farfantepenaeus aztecus, Gulf Menhaden Brevoortia patronus, Spotted Seatrout Cynoscion nebulosus, Red Drum Sciaenops ocellatus, phytoplankton, mesozooplankton, gelatinous plankton, ichthyoplankton, benthic infauna, and Bottlenose Dolphin.
We evaluated time series of abundance information available from standardized surveys and in several cases, the history of commercial and recreational fisheries catches (which extend for several decades) and demographic and animal health information bearing on the evaluation of impacts. Additionally, we conducted a semi-quantitative vulnerability-resilience (V-R) analysis to evaluate, on a relative basis, the circumstances of the DWH spill focusing on how they affected species outcomes, and the various life-history aspects of the species that make them more or less resilient to large-scale pollution events such as oil spills.
Vulnerability-Resilience Analysis
The concept of a two-way evaluation of vulnerability and resilience of populations to stressors is well founded in existing literature (e.g., Stobutzki et al., 2001; Field et al., 2010; Patrick et al., 2010; Hobday et al., 2011) and reviewed within the context of the DWH spill.
Briefly, our evaluation of the vulnerability of individual populations to the specifics of the DWH spill is based on a series of 13 attributes related to the spatial and temporal overlap of oil contamination with the population in question, the duration frequency of acute/chronic exposure to oil, the ability of the species to detect and avoid such exposures, and other factors including the impacts of spill response measures (Supplementary Table 2). For example, spat and adult Eastern Oyster have no capacity to avoid either toxic concentrations of oil or fresh waters resulting from marsh flooding because they are sedentary (Powers et al., 2017a). For each of the 13 attributes, vulnerability was evaluated using a categorical scoring system as low, medium or high for each of the key species or groups. Evaluators independently scored each species or functional group on the 13 attributes. Based on subsequent group discussion we provided a single vulnerability categorization integrating attribute assignments of the 13 key species we evaluated.
Similar to the vulnerability axis, we defined 11 attributes contributing to resilience potential of key taxa (Supplementary Table 2). The directionality of resilience scores for each attribute are generally obvious. For example, highly fecund species are likely to be more resilient to perturbations than less fecund ones, as are ones that are highly networked with adjacent populations unaffected by the perturbation (e.g., through immigration). Again, each selected species was evaluated by each co-author categorizing the 11 attributes, and an overall resilience determination was assigned as noted for vulnerability. Polidoro et al. (2020) developed a similar set of attributes describing aspects of combined vulnerability and resilience to hypothetical petrochemical contaminant exposures.
Community-Level Analyses
In addition to the population-level summaries for key species, we undertook community-level analyses of species compositions and abundances to evaluate DWH effects on overall resource productivity and to explore the possibility of post-DWH regime shifts in ecosystems’ beta-diversity. Using the long time series of trawl survey observations available for three basins in eastern coastal Louisiana (Figure 3) and the time series in Mississippi (partial time series), Alabama and Florida (Table 1 and Figure 4) we computed overall abundance (numbers trawl haul–1) and, in the case of the Louisiana and Alabama time series, divided the catches into three habitat and taxonomic groups: (1) freshwater species, (2) invertebrate species, and (3) all finfishes (Figure 3).
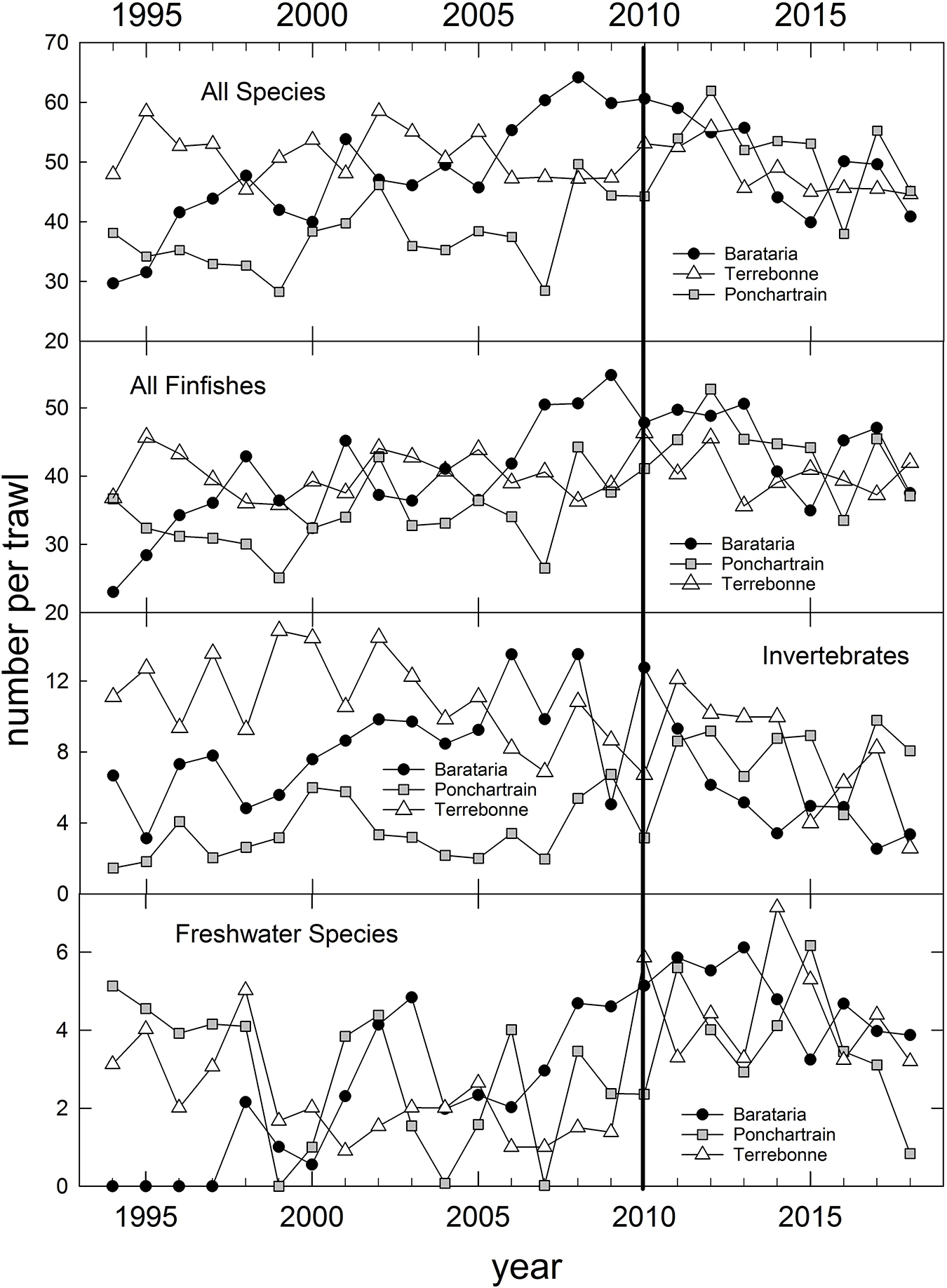
Figure 3. Annual relative abundance of all species catches and three species groups (Supplementary Table 1) caught in fishery-independent trawl sampling by the Louisiana Department of Wildlife and Fisheries, in three Louisiana coastal basins, 1994–2018. Data are inverse-variance weighted mean catches trawl– 1.
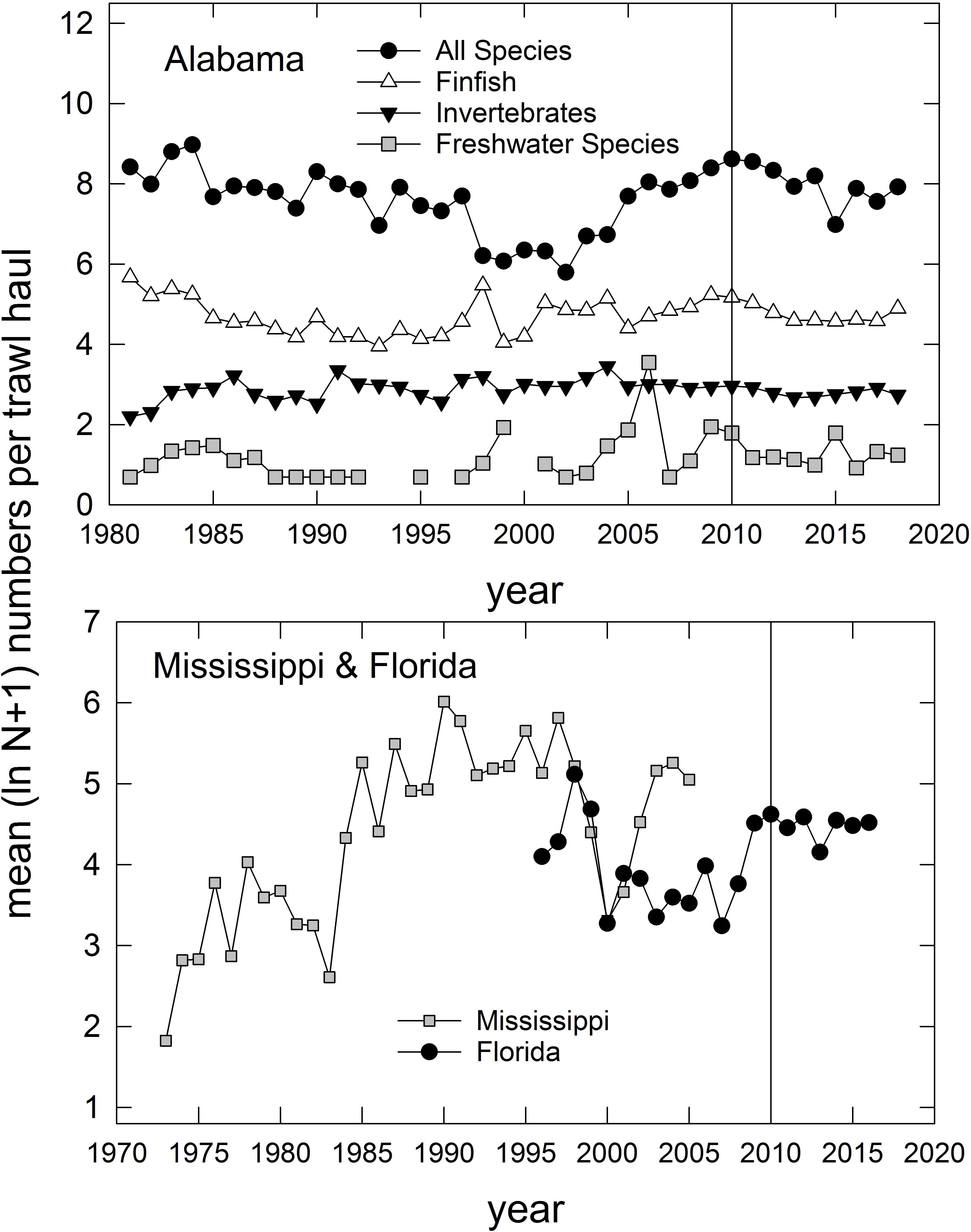
Figure 4. Annual relative abundance (mean ln [N + 1]) of species caught in trawl surveys in Alabama (top panel), Florida (west of 85° W longitude), and Mississippi (partial time series; bottom panel). Vertical line represents the year that the Deepwater Horizon accident occurred. For Alabama trawl catches, species are divided into several groups, consistent with categories indicated in Supplementary Table 1. Data are from the Alabama Marine Resources Department, the Louisiana Department of Wildlife and Fisheries, and the Florida Fish and Wildlife Research Institute. Data for Mississippi were collected by Mississippi Department of Marine Resources, and are served via the National Centers for Environmental Information (NCEI) at: https://data.noaa.gov/dataset/dataset/fisheries-independent- biological-and-environmental-trawl-data-from-the-mississippi-department-o.
Because virtually all of the heavily oiled wetlands sites were in coastal Louisiana (Figure 1A; Nixon et al., 2016) we undertook a series of multivariate analyses with data from that state to test for community-level shifts in species dominance both as a means of efficiently evaluating changes in over the 300 + species indexed by trawl surveys (Supplementary Table 1) and to detect beta-diversity regime shifts that may be associated in time with the DWH event. Since the highest concentration of heavily oiled sites was within Barataria Bay (Figure 1; Nixon et al., 2016), this synthesis focused on the analyses of the trawl data set from that basin (illustrated in Figures 5–8), although some community-level results from the other two Louisiana basins considered are described in the Supplementary Material (Supplementary Figures 8, 9).
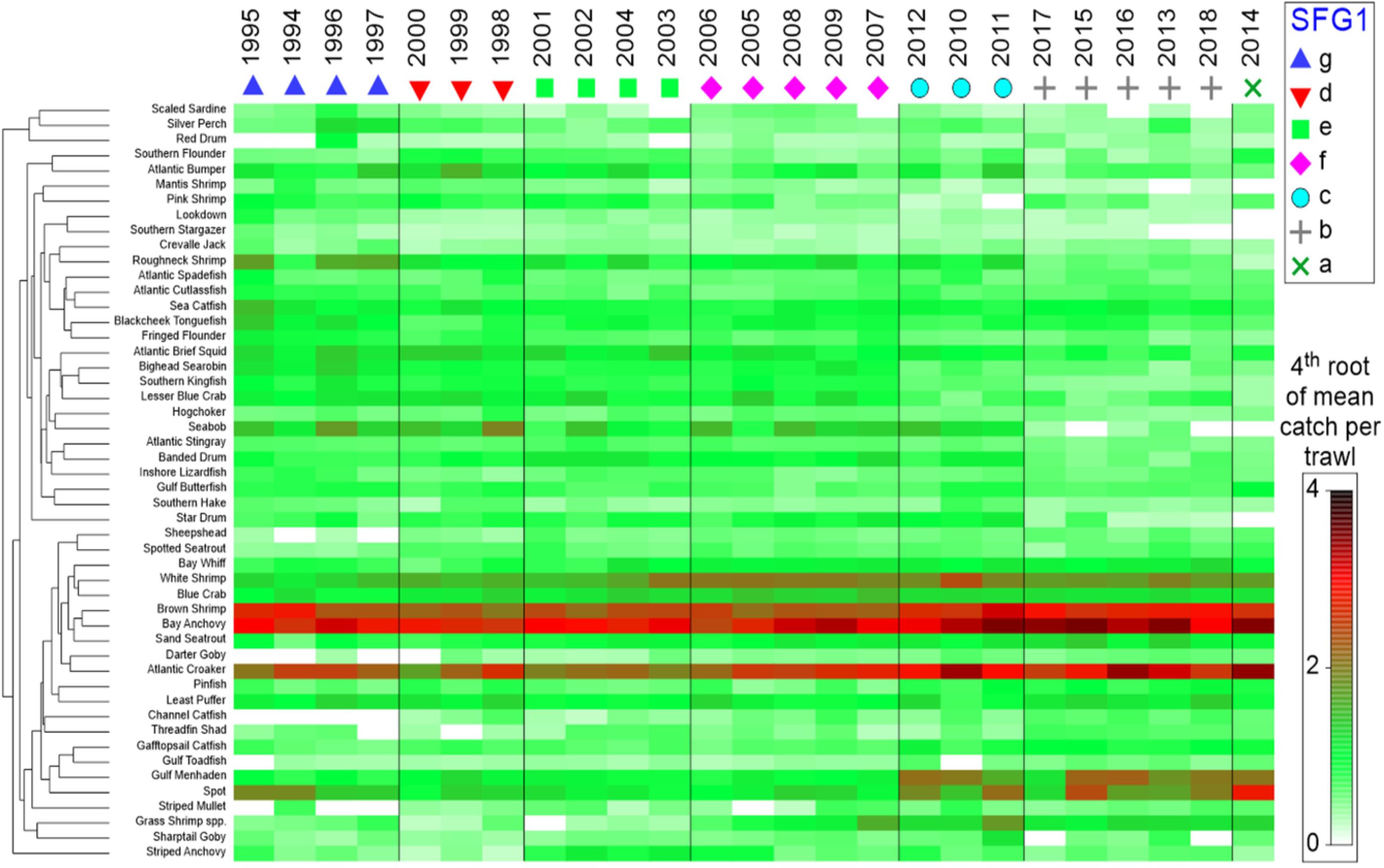
Figure 5. Seriated heatmap of annual abundance data for species captured in trawl surveys conducted by the Louisiana Department of Wildlife and Fisheries, 1994–2018. Similarity Profile Analysis (SIMPROF) was applied to Bray-Curtis hierarchical year clusters (group average method), with significant SIMPROF groups (SFG1) arranged in columns. Shading is the 4th root of the relative abundance indices (mean numbers per trawl tow) by species. Species that were absent in five or more years were excluded.
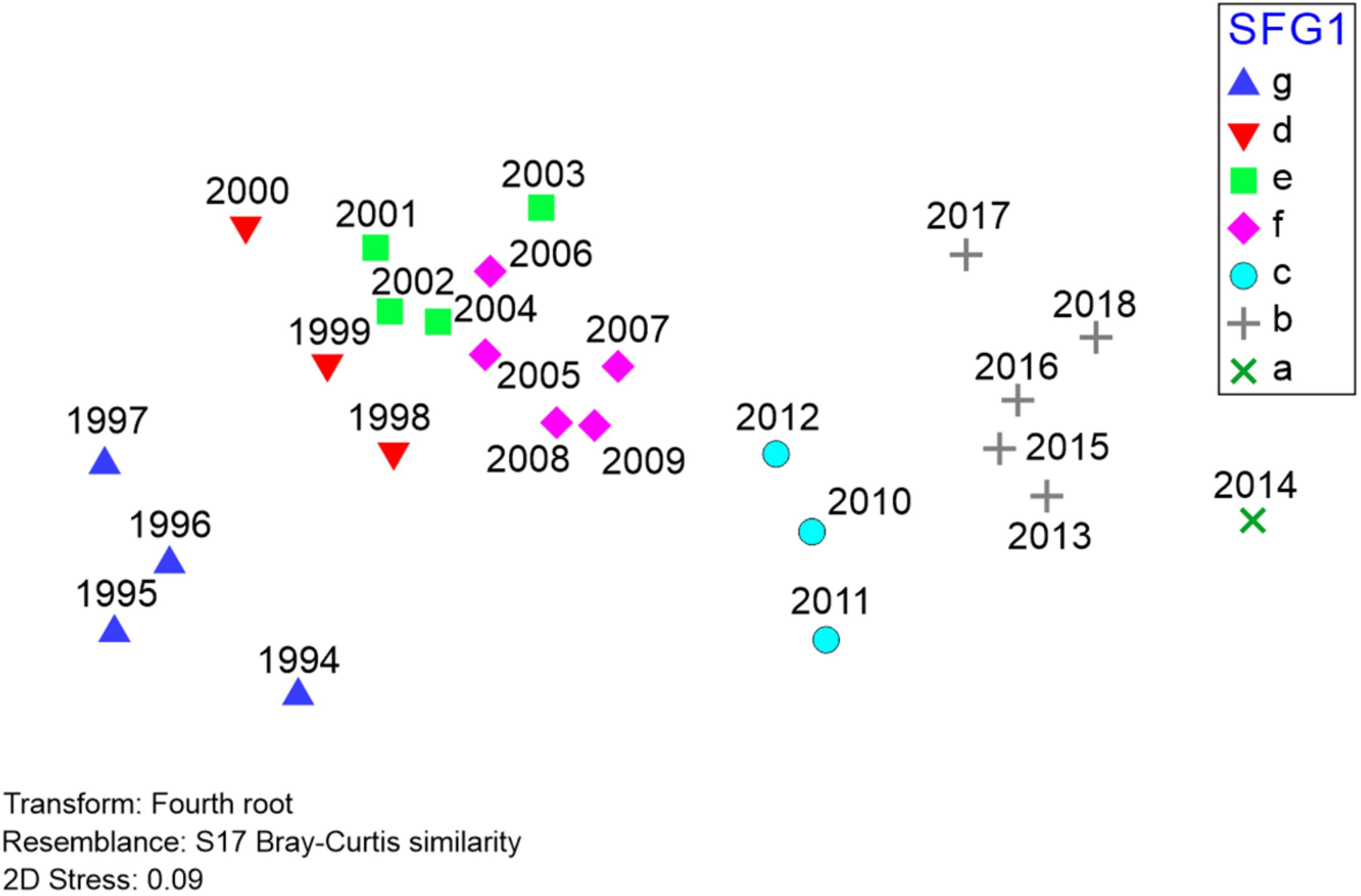
Figure 6. Results of non-metric multidimensional scaling (nMDS) of Barataria Bay trawl survey catches, 1994–2018 (data and symbols as in Figure 5). Relative positions of different years are determined by Bray-Curtis similarities between all possible pairings of years, with 2D stress reflecting the extent of distortion (low distortion, in this case) created by representing these Bray-Curtis similarities in a two-dimensional Euclidean space.
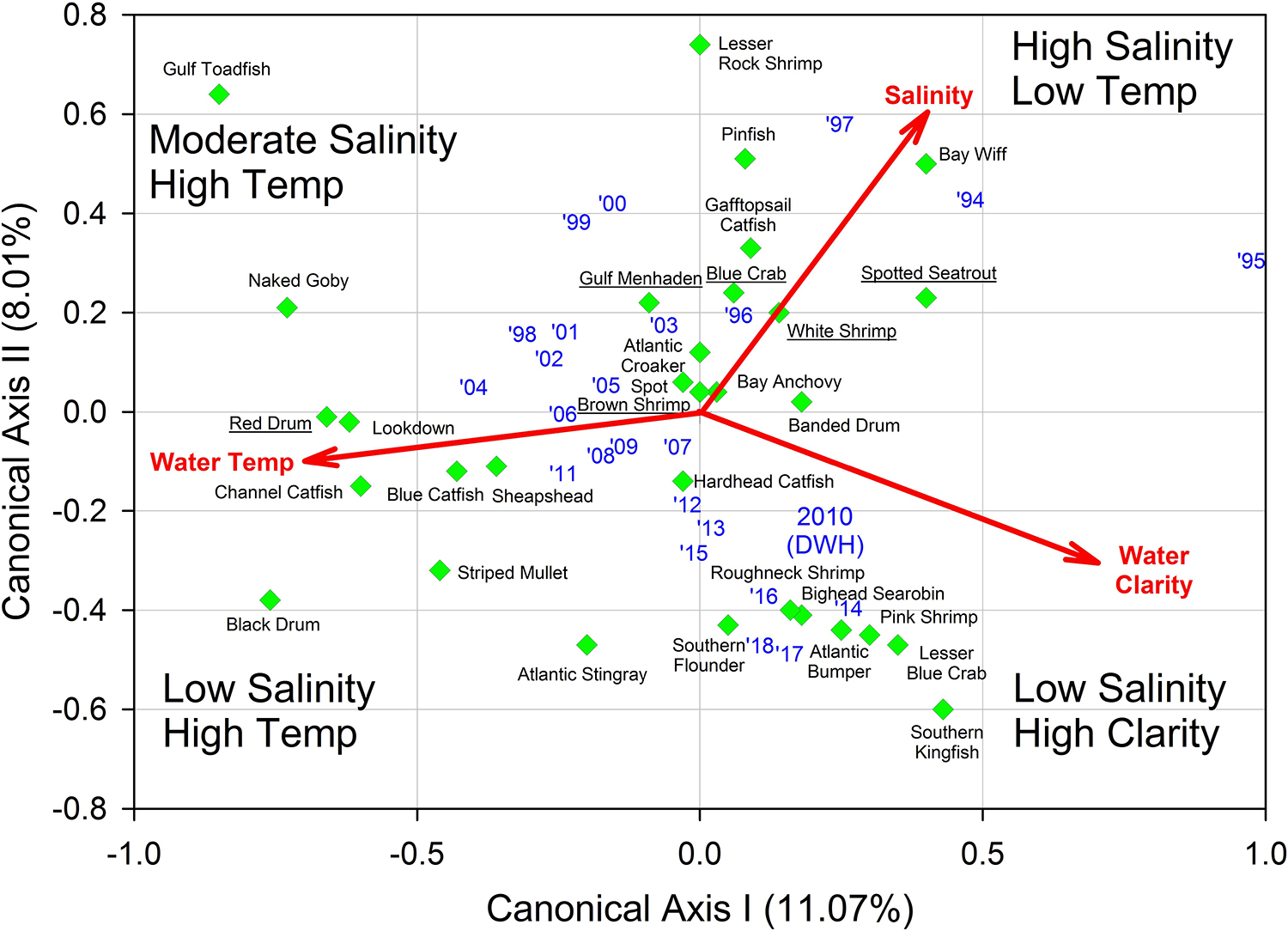
Figure 7. Results of multivariate redundancy analysis (RDA) relating three important environmental variables (salinity, water temperature, water clarity) to relative fish and invertebrate abundance from Barataria Bay trawl surveys, 1994–2018. Blue texts are individual years. Common names of the “key species” are underlined. Data were analyzed from the Louisiana Department of Wildlife and Fisheries trawl surveys.
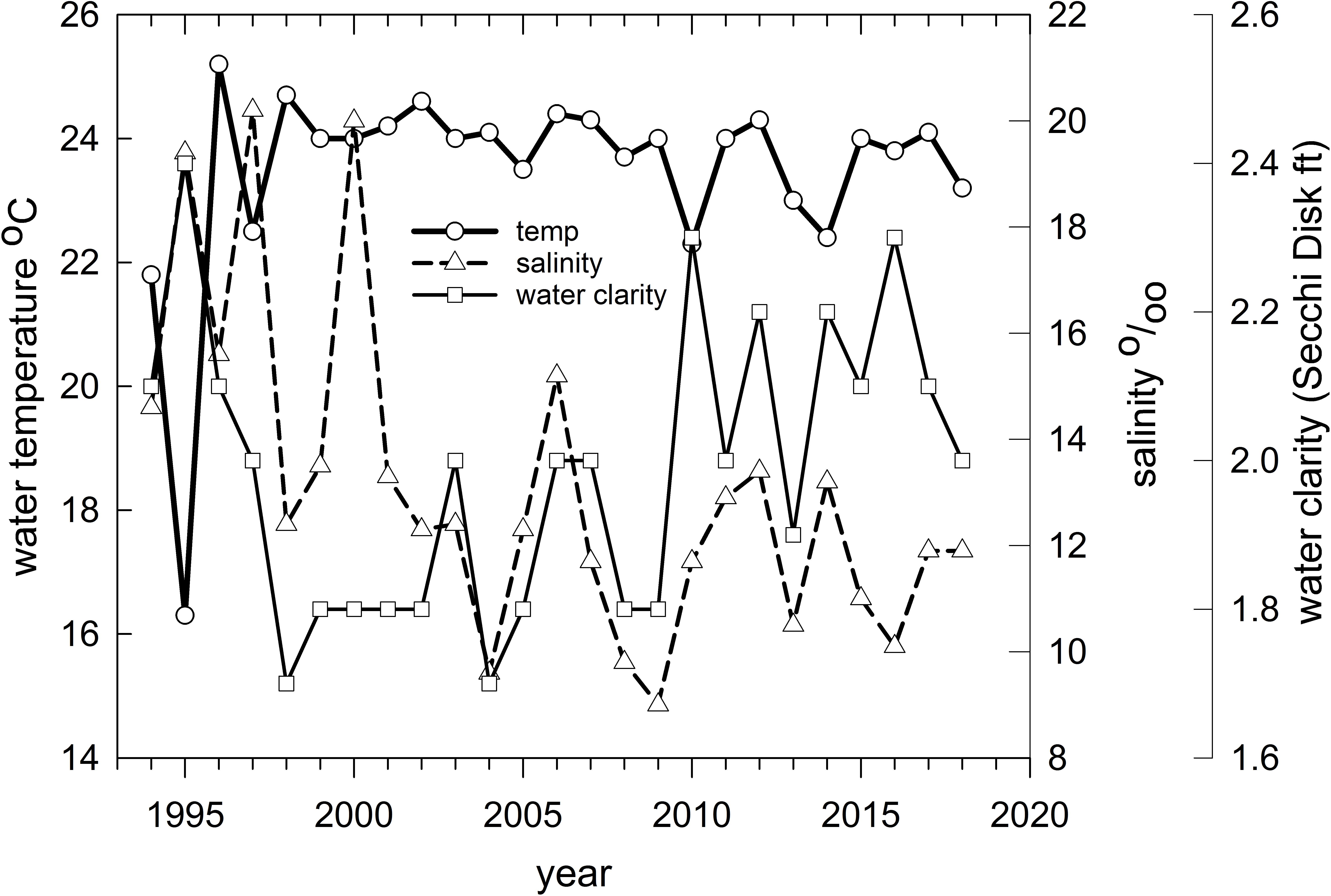
Figure 8. Mean water temperature (°C), clarity (Secchi disk reading in feet) and salinity (parts per thousand) for Barataria Bay trawl stations sampled by the Louisiana Department of Wildlife and Fisheries, 1994–2018.
Temporal differences in beta-diversity across the Barataria Bay trawl-survey time series were quantified using Bray-Curtis similarity for pairwise yearly comparisons; the similarities were clustered using the unweighted pair-group method with arithmetic mean (UPGMA), and the statistical significance of the resulting clusters of similar years was tested using similarity profile analysis (SIMPROF) with the PRIMER-E + software system (Clarke et al., 2008; Clarke and Gorley, 2015; Kilborn et al., 2017). The null hypothesis was that the observed similarity profile across time was random and did not have consistent temporal clustering of species communities (Clarke et al., 2008). Additionally, a second Bray-Curtis, UPGMA-clustering procedure grouped species with similar temporal trends in their average annual basin-wide relative abundance (CPUE = numbers per standardized trawl haul; vertical axis in Figure 5). A fourth-root transformation of the data was used to minimize the effect of aberrant large catches.
To further represent the degree of association between yearly species compositions, we also applied non-metric multidimensional scaling (nMDS) to the Barataria Bay trawl survey time series (Figure 6). In our context the nMDS procedure utilizes a matrix of ranked dissimilarities of abundance data by species (hence “non-metric” data) comparing year-to-year trawl catch compositions. The procedure (Borcard et al., 2018) is conceptually similar to principal coordinates analysis (PCoA) and collapses the comparisons among a set of independent variables into m dimensions (typically two) to depict associations among observations, and particularly related to changes in beta-diversity. A test statistic (the “stress” level, ranging from 0 indicating perfect fit, to 1, no fit) evaluates the distortion resulting from representing all non-Eucliden pairwise comparisons (i.e., Bray-Curtis) in only two Euclidean dimensions. A stress statistic <∼0.1 indicates relatively low distortion, providing a useful representation of the ecosystem’s underlying interrelationships.
Finally, we applied redundancy analysis (RDA) to evaluate the associations between Hellinger-transformed species catches and a series of contemporaneously measured environmental variables (after z-score translation). The RDA procedure first applies a multivariate multiple linear regression model associating environmental data and response variables (species abundances) followed by a PCA of the resultant fitted values (Borcard et al., 2018). The RDA ordination diagram (Figure 7) provides the annual depiction of the ecosystem’s state, with respect to its community response, and the capacity of any associated environmental drivers to account for the observed variability in that response. We used the Barataria Bay trawl series and associated measurements of water temperature, clarity (measured via Secchi disk), and salinity as environmental correlates (see Figure 8 for the time series). This analysis was particularly important in helping to deconvolve long-term oil spill effects from variation and trends in other potentially determinative environmental attributes.
Results
Species-Level Summaries
Eastern Oyster – Oyster populations have been the subject of a variety of post-DWH analyses given their commercial and cultural importance in the region (Deepwater Horizon Natural Resource Damage Assessment Trustees, 2016; Powers et al., 2017a; Supplementary Figure 1). The overall abundance of natural oyster populations has been in long-term decline and particularly since the mid-1990s throughout the region (VanderKooy, 2012; Tunnell, 2017), as reflected both in the relative abundance surveys (Figure 9) and in fishery landings (Supplementary Figure 1). Several factors affect inter- and sub-tidal oyster populations. A series of natural disasters and especially hurricanes (e.g., Katrina in 2005) as well as flooding events (and especially in 2019) have negatively affected local populations (Moore et al., 2020). Oysters are filter feeding invertebrates, and as such have limited capacity to metabolize organic pollutants such as polycyclic aromatic hydrocarbons (PAHs) resulting in their bioaccumulation within tissues. Abundance surveys in Louisiana (three eastern basin systems, Figure 1) and Alabama documented longer-term declines and short-term impacts coincident with DWH (Figure 9). The relative abundance of oysters declined between 2009 and 2010 in all estuarine systems (Figure 9), and subsequently increased in 2011 (2012 for Pontchatrain), although interannual variability was often large. Relative abundance in the Louisiana basins remained elevated for several years following the spill, but abundance in all estuaries subsequently fell, and by 2018 relative abundance of oyster populations was is in line with long-term resource trends (Figure 9). Oyster catches off Texas and western Louisiana remained near decadal averages. In contrast, landings decreased severely in 2010 in Alabama, Mississippi and Louisiana, but increased in areas not subject to fishery closures in 2010 (e.g., Texas and parts of Florida; Supplementary Figure 1). Landings in Alabama and especially Mississippi were among the time series lows in 2018, with the latter likely affected by freshwater flooding of Mississippi Sound from heavy rains and the opening of the Bonnet Carré Spillway. For Florida, most landings occurred east of the area affected by the DWH spill, and long-term declines there are likely related to hydrological changes (Moore et al., 2020).
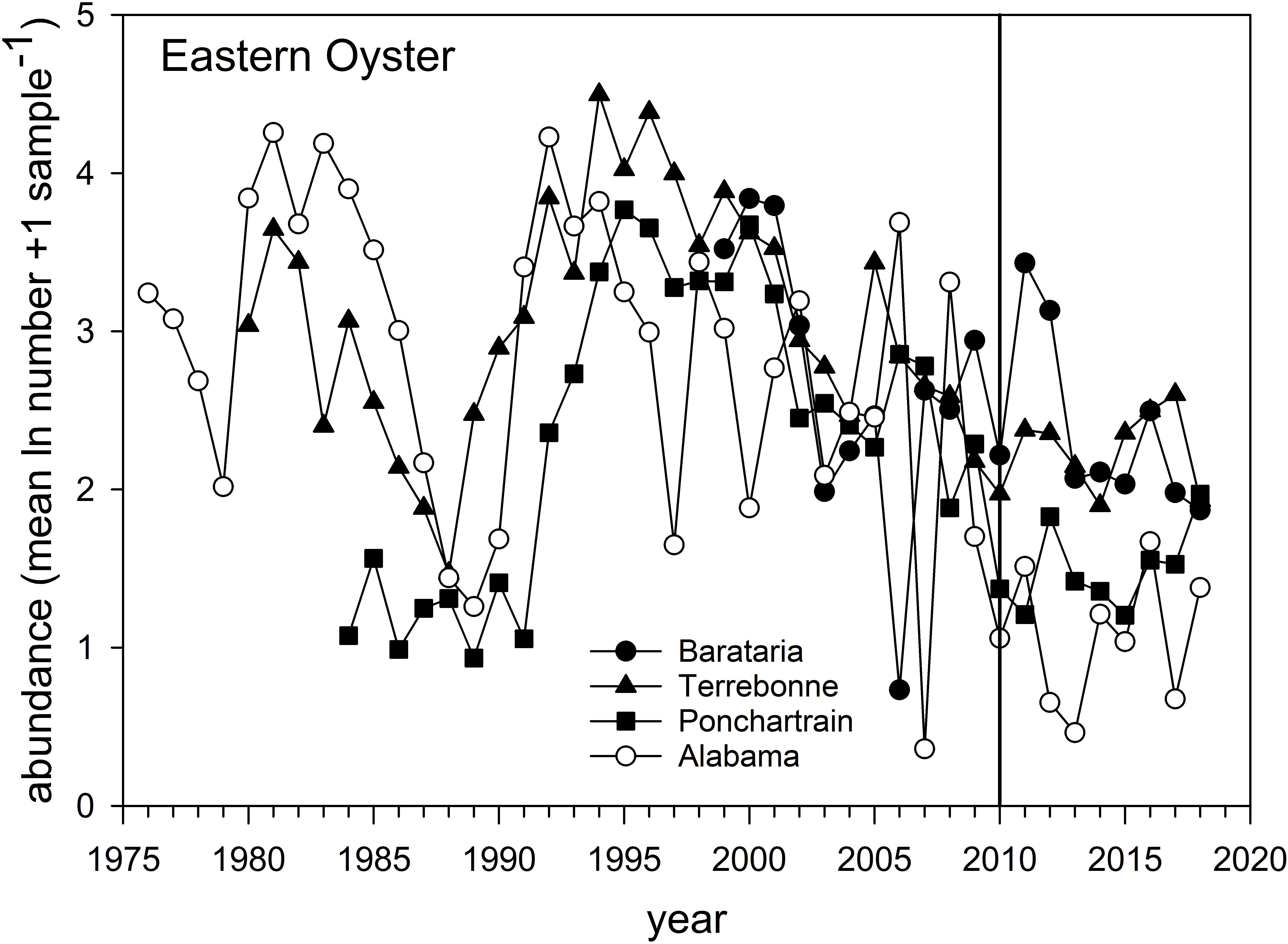
Figure 9. Relative abundance of Eastern Oyster sampled in three Louisiana basins, 1980–2020, and off Alabama from 1976 to 2018. Data are the mean (ln + 1) abundance (numbers) caught in standardized sampling quadrats. Data are from the Alabama Marine Resources Department and the Louisiana Department of Wildlife and Fisheries oyster sampling quadrat programs.
Considerable research has documented the decline of oyster populations in the Louisiana basins, particularly Barataria and Pontchartrain, associated with DWH (Deepwater Horizon Natural Resource Damage Assessment Trustees, 2016; Powers et al., 2017a,b,c; Figure 1). In particular, releases of freshwater from the mainstem Mississippi River via the Davis Pond freshwater diversion, which leads to the upper Barataria Bay (White et al., 2018), and the Caernarvon freshwater diversion, which leads to Black Bay, considerably freshened the usually brackish waters of the middle and lower bays (Figure 1A; O’Connor et al., 2016; Powers et al., 2017a). While oysters have substantialtolerance for varying salinity and temperature regimes (Sehlinger et al., 2019), prolonged exposure to low salinities of <5 ppt will eventually result in death (Powers et al., 2017a). Schrandt et al. (2018) in fact demonstrated that in laboratory conditions, short-term episodes of low salinity water may mitigate effects of oil/dispersant exposures. However, long-term fresh water conditions persisted in Breton Sound/Black Bay and Barataria Bay in much of the spring and summer of 2010, likely resulting in excess mortalities of 1.16–3.29 billion market sized oysters due to low salinity stress (Powers et al., 2017a). This is consistent with reduced population sizes in the Pontchartrain basin and Barataria Bay, as observed in standardized surveys (Figure 9). The effects of elevated levels of oil contamination in these two systems (e.g., Turner et al., 2019a) on oyster mortalities of various life stages are unknown but intertidal oyster cover declined >99% at oiled sites as opposed to unoiled ones (Grabowski et al., 2017; Powers et al., 2017c), an effect that persisted for several years after the DWH event.
The vulnerability of Eastern Oyster populations to the effects of DWH and associated response measures (i.e., freshwater diversions) was heightened by the sedentary existence of spat and adult animals but mitigated in part by their reproductive strategy. As noted above, oyster reefs occurred less frequently at heavily oiled areas than along non-oiled marsh edges, and prolonged low salinity exposure as a result of freshwater diversions (in Barataria Bay and Breton Sound) resulted in considerable excess mortality. However, oysters have an extended bimodal spawning period with peaks in May-June and again in September-October (Tunnell, 2017). Because of the non-uniform oiling of oyster populations even in areas that were characterized as heavily oiled, there was residual spawning potential residing in these basins to support sustained reproduction. In considering the criteria for characterizing vulnerability to DWH, we evaluated oyster populations of Barataria Bay to be of medium vulnerability, and likely medium to lower vulnerability elsewhere where acute oil effects and freshwater discharges were less intense.
Oysters are highly fecund, capable of reproducing in 30 days or less following settlement from the planktonic stage and they can be relatively long lived in the absence of other stressors including fishing (Tunnell, 2017). They are amenable to rebuilding strategies that include planting of oyster shell cultch, and there may be some potential for planktonic stages to mingle with adjacent populations. In summary, we consider oysters (in this case the Barataria Bay population) to be of medium resiliency potential. Summary Evaluation: Vulnerability – Medium, Resilience – Medium.
Blue Crab – Populations in the U.S. GoM have been separated into two population units (east and west of Cape San Blas; Figure 1) for the purposes of stock assessment and management (VanderKooy, 2013). However, recent analysis based on microsatellite data reveal high levels of gene flow throughout the northern GoM, potentially indicating a single panmictic population (Macedo et al., 2019). Like the Eastern Oyster, Blue Crabs in the GoM have an extended spawning period and complex early life history. Spawning (release of fertilized eggs) occurs from March through December, with Spring (April–May) and late Summer (August–September) peaks (Tunnell, 2017, his Figure 8.4). Females mate once in their lifetime in the upper estuary, then migrate to coastal waters where they may spawn repeatedly throughout their lives. Mating occurs when estuarine temperatures exceed about 22°C (e.g., in spring) in the upper estuaries but fertilized eggs are released into coastal waters, thus subjecting the various larval stages to potentially wide dispersal during their 31–49 day larval development period, which provides justification to explain the lack of genetic population differentiation (Macedo et al., 2019). Overall commercial landings of Blue Crab in the U.S. GoM have remained relatively stable since about 1990, however, in some states (Alabama, Florida and Texas) landings have declined since the late 1990s (Supplementary Figure 2). Modest declines in Blue Crab landings occurred in states affected by fishery closures, but in all cases, landings were higher in 2011 than in 2010 (Supplementary Figure 2). Crab abundance, as indexed in trawl surveys, declined steadily and significantly in Alabama and Mississippi waters since the 1980s (Figure 10; O’Connell et al., 2019) likely due to long-term reductions in habitat or water quality. In Louisiana waters, crab densities in Barataria Bay have declined since the 1990s, but remained relatively high in the Pontchartrain and Terrebonne basins (Figure 10). There was no pronounced change in crab abundance associated with the DWH event in the Louisiana basins, although recent (2018) abundances are generally lower than historic averages (Figure 10). Crab abundance in the Florida Panhandle has been highly variable throughout (Figure 10).
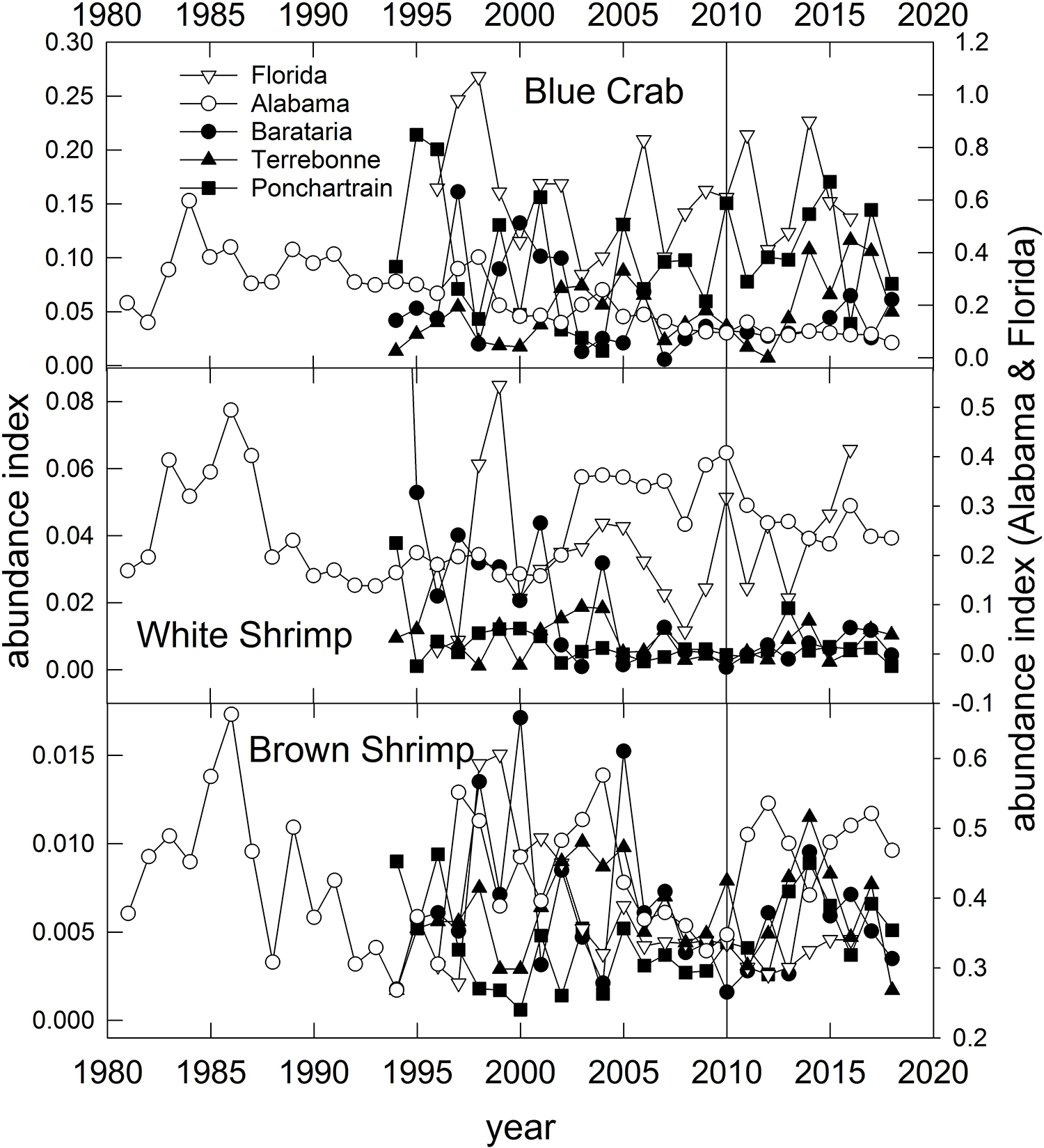
Figure 10. Annual relative abundance indices for Blue Crab, White Shrimp and Brown Shrimp sampled from fisheries-independent trawl surveys conducted in three Louisiana basins, off Alabama and the Florida Panhandle (Figure 1). Data are from the Alabama Marine Resources Department, the Louisiana Department of Wildlife and Fisheries and the Florida Fish and Wildlife Research Institute’s trawl survey programs.
We considered Blue Crab to be highly vulnerable to the effects both of the oiling scenario from DWH and the ensuing response measures (i.e., freshwater diversions). Because mating females occurred in the upper estuaries during the months when oil entered coastal marshes (particularly in Louisiana), and their reliance on benthic prey in areas where oil was sedimented, a high degree of overlap between crabs and DWH oil in sediments and the water column likely exists. Also, transport of larval stages developing from spring spawning locations in the GoM into the estuaries occurred when oil was present in coastal waters (June–July). However, we considered the Blue Crab populations to be moderately resilient to the effects of the DWH event, and similar pollution-related events, due to the extended spawning (double peak) duration, long larval period in oceanic waters and the high potential for larval supplements from adjacent estuaries. Summary Evaluation: Vulnerability – High, Resilience – Medium.
Penaeid Shrimps -- White Shrimp and Brown Shrimp support the most valuable U.S. fishery in the GoM, collectively worth an average $375 million per year to U.S. fishers between 2000 and 2018, and $393 million per year since 20111. Landings of White Shrimp (Supplementary Figure 3) declined somewhat in all states except Texas and Florida in 2010, but quickly rebounded in 2011 after the fishery closures were rescinded. Landings of White Shrimp varied without trend in all states following 2011 (Supplementary Figure 3). Brown Shrimp landings were more variable, declining sharply in all states in 2010, followed by rapid rebounds in Mississippi, Alabama and Louisiana a year or two after the spill (Supplementary Figure 4). Except for Florida (a minor participant in the fishery), Brown Shrimp landings across the Gulf have varied without trend or increased slightly since the DWH spill.
Extensive research on the environmental impacts of the DWH spill on Penaeid shrimps has been conducted. van der Ham and de Mutsert (2014) analyzed trawl survey abundance data available from the Louisiana Department of Wildlife and Fisheries for the pre-spill, during-spill (2010), and just post-spill (2011 and 2012) time periods. They concluded that the abundances of both Brown and White Shrimps were significantly higher just after the spill but that the effect was ephemeral, and by 2012 the effect was negligible. They hypothesized that increased abundance in the basins most affected by the spill was either due to the effects of short-term shrimp fishery closures (thus reducing fishing mortality), or that the oil spill affected growth rates negatively and restricted emigration of shrimp out of the estuary and into adjacent coastal waters. Evidence to support each hypothesis exists. Schaefer et al. (2016) documented a statistically significant increase in the abundance of fish species traditionally caught as bycatch in directed shrimp fisheries off Mississippi, and this trend reversed once DWH-related fishery closures ended. Likewise, Rozas et al. (2014) documented reduced White and Brown Shrimp growth rates in mesocosms exposed to crude oil in areas adjacent to oil-polluted marsh edges (Powers and Scyphers, 2016).
The relative abundance of White and particularly Brown Shrimps increased in most assessed regions in the few years following DWH, but for the most part declined to pre-spill abundance levels by the late 2010s (Figure 10). Because of long-term and significant reductions in shrimp fishing effort (Caillouet et al., 2008; Tunnell, 2017), shrimp (and particularly Brown Shrimp) abundance has generally increased, and notably since the mid-2000s (Figure 10; Tunnell, 2017). While the general life histories of the two species are similar, they differ in details of spawning and migration of adults and larvae along with their environmental requirements (Turner and Brody, 1983; Tunnell, 2017). Both species copulate and the females carry fertilized eggs until they are released offshore. Also, both species spawn offshore in relatively deep waters, although Brown Shrimp release fertilized eggs deeper (46–109 m) than White Shrimp (7–31 m; Turner and Brody, 1983). Two spawning periods for Brown Shrimp have been observed off Texas and Louisiana, with peaks in April–May and again in September–November, although spawning can occur in most months with the exception of June-August. White Shrimp generally have one spawning peak (June–July) and a narrower range of timing (March–October). Postlarvae, which develop offshore, are recruited back into the estuaries where juvenile-to-adult development occurs in both species. The postlarval immigration from the ocean to estuaries occurs in all months with a peak in February–April for Brown Shrimp and more narrow windows of immigration peaking in June and September for White Shrimp (Tunnell, 2017). Generally White Shrimp postlarvae migrate farther up estuaries (into more fresh water) than Brown Shrimp (Tunnell, 2017).
It is likely that there was little interaction between the spawning cycle of Brown Shrimp and oil in the water column given the timing of spawning and immigration (of larvae from the ocean to the estuaries) or emigration (of adults from estuaries to the ocean to spawn) of the two species. The second spawning of cohorts (April–May) occurred prior to the majority of DWH oil coming inshore, and the DWH well was capped in July prior to the first spawning of the successive cohort (September–November). In contrast, the peak of White Shrimp spawning activity typically is in June-July when DWH oil had entered coastal waters (Figure 11). A large-scale fishery closure, usually from May 15 to July (variable end-dates), has been in place since 1960 to improve fishery yields and shrimp sizes in Brown Shrimp catches (Purcell et al., 2017), and this closure coincides with the emigration of adults from estuaries. Additionally, fishery closures due to DWH resulted in decreases in fishing mortality on adults in the northern GoM, with a concomitant increase in fishing effort for shrimp off Texas when the annual closure there expired (Carroll et al., 2016; Cockrell et al., 2019). The timing of the DWH oil migration to the coast in relation to the differential distributions of Brown and White Shrimp adults and larval stages may explain why Brown Shrimp appeared to show a more positive response during and just after the DWH episode (Figure 10). Based on the timing of overlap of critical life stages with the presence of DWH oil and the mitigative effects of DWH-related fishery closures we consider these species to have been of moderate vulnerability to the spill. Because of the large population size, extended and often multiple spawning periods for the species, short life cycles (maximum age of 18 months) and the likelihood of metapopulation dynamics among adjoining estuaries we consider these species to be of high resilience potential to similar events. Summary Evaluation: Vulnerability – Medium, Resilience – High.
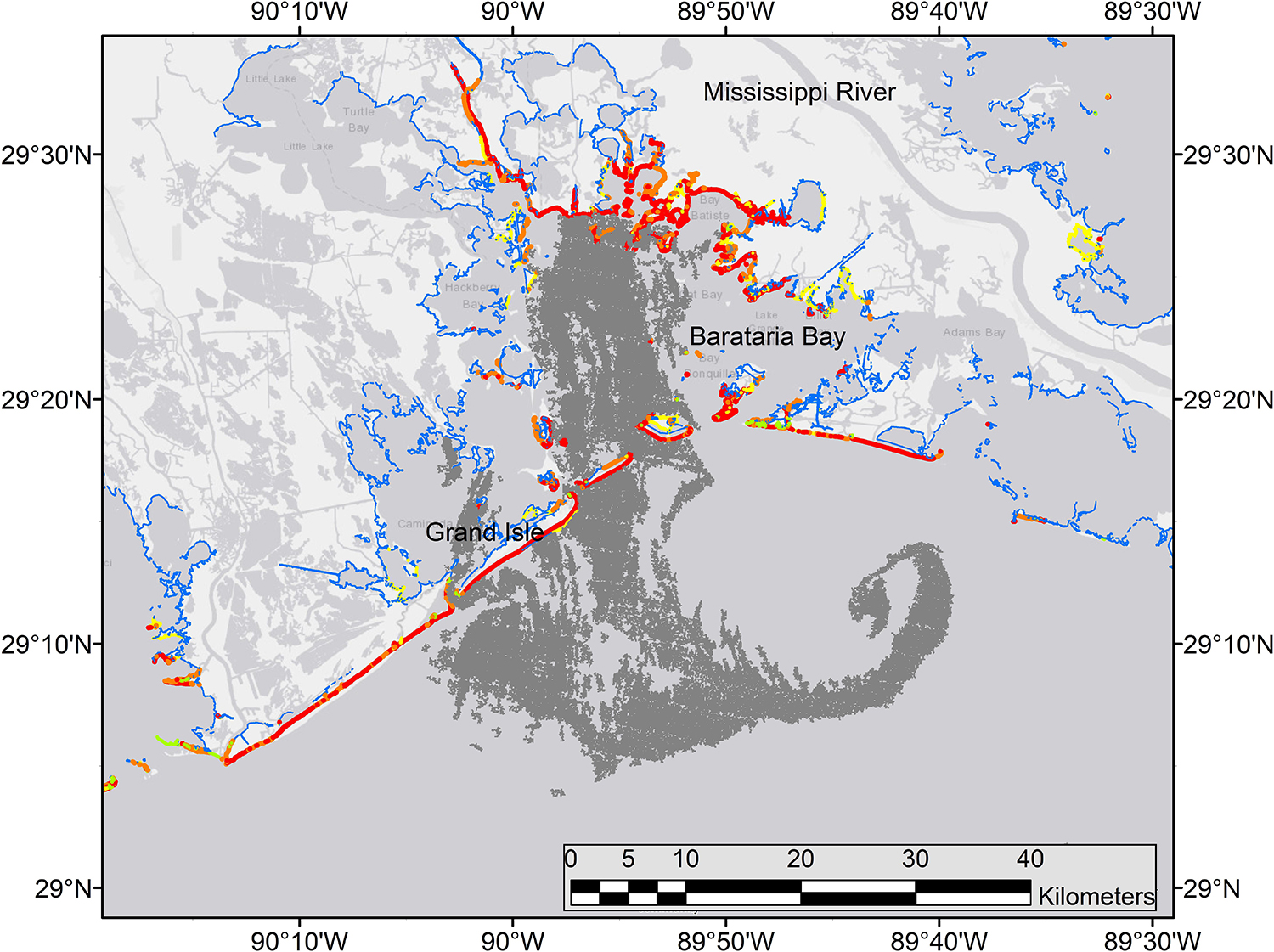
Figure 11. Synthetic aperature radar (SAR) image of oil at the sea surface (dark gray) across the mouth of Barataria Bay, Louisiana, June 4, 2010. Details of instruments and processing methods are given here: https://erma.noaa.gov/admin/layer/32308. Colors represent shoreline oiling categories as in Figure 1.
Gulf Menhaden – Menhaden support the largest fishery, by volume, of any species in the U.S. GoM. Fishery landings approached 1 million mt yr–1 in several years in the 1980s (Figure 12 and Supplementary Figure 5), and prior to the development of pollock fisheries in Alaska, supported the largest commercial fishery (by landings volume) in America. In recent years Gulf Menhaden catches averaged about 500 million mt yr–1 (Figure 12), with the majority of landings being from Louisiana and Mississippi (Supplementary Figure 5). Because of concerns of overfishing this short-lived species, landings were scaled back from their peak levels in the 1980s with resultant increases in relative abundance (as measured in commercial purse seine catch set–1) and in the total population fecundity of potentially spawned eggs (SEDAR, 2018; Figure 12). Following the fishery closures in 2010, landings, abundance (CPUE) and cumulative population fecundity increased sharply in 2011, likely due to the anomalously large 2010 year-class (Short et al., 2017; SEDAR, 2018; Figure 12). Several hypotheses have been advanced regarding the drivers of Gulf Menhaden year class strength, including reductions in predation in 2010 (Short et al., 2017) and long-term correlations with Mississippi River discharge (Govoni, 1997). In more recent years catches have declined to pre-2010 levels, concomitant with increases in population fecundity and CPUE, likely resulting from decreased fishing mortality (Figure 12; SEDAR, 2018).
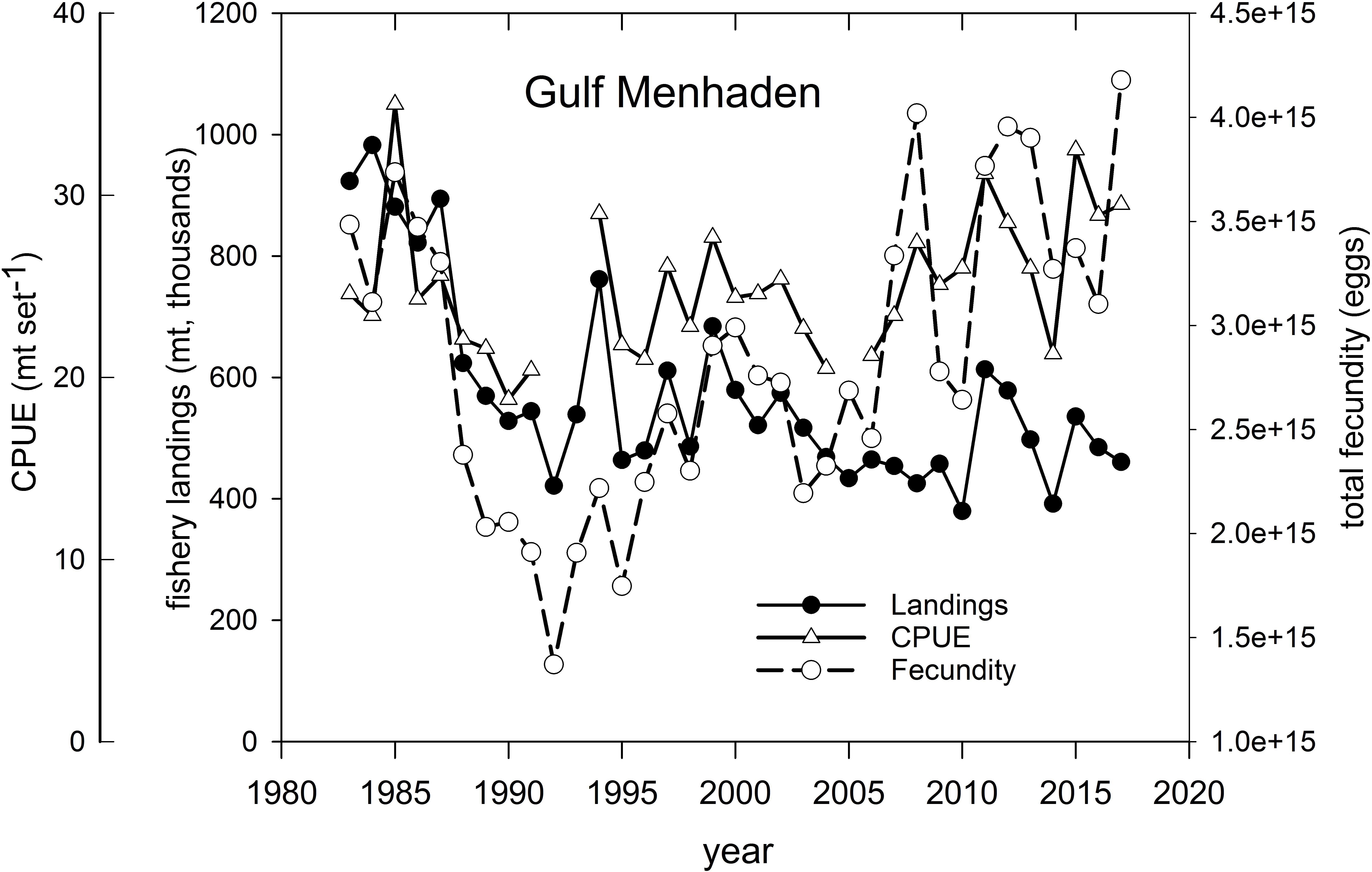
Figure 12. Annual fishery landings (thousands of metric tons), relative abundance [measured as mean standardized catch (mt) per purse seine set) and estimated total population fecundity of Gulf Menhaden, 1983–2017. Graph was developed from information provided in SEDAR (2018).
The life history and spawning dynamics of Gulf Menhaden resulted in minimum spatial overlap with oil coming ashore from DWH and the distribution of sensitive early life stages of the species (Figures 13, 11). Menhaden have a short life cycle with 1- and 2-year-old individuals comprising the bulk of the adult population (SEDAR, 2018). They spend their first year of life within the upper reaches of estuaries and are thus prey for a wide variety of bird, fish and invertebrate predators. Adult Gulf Menhaden emigrate from the estuaries to the coastal ocean to spawn from October through March, with a spawning peak in December to February (Figure 13; Lewis and Roithmayr, 1981; SEDAR, 2018).
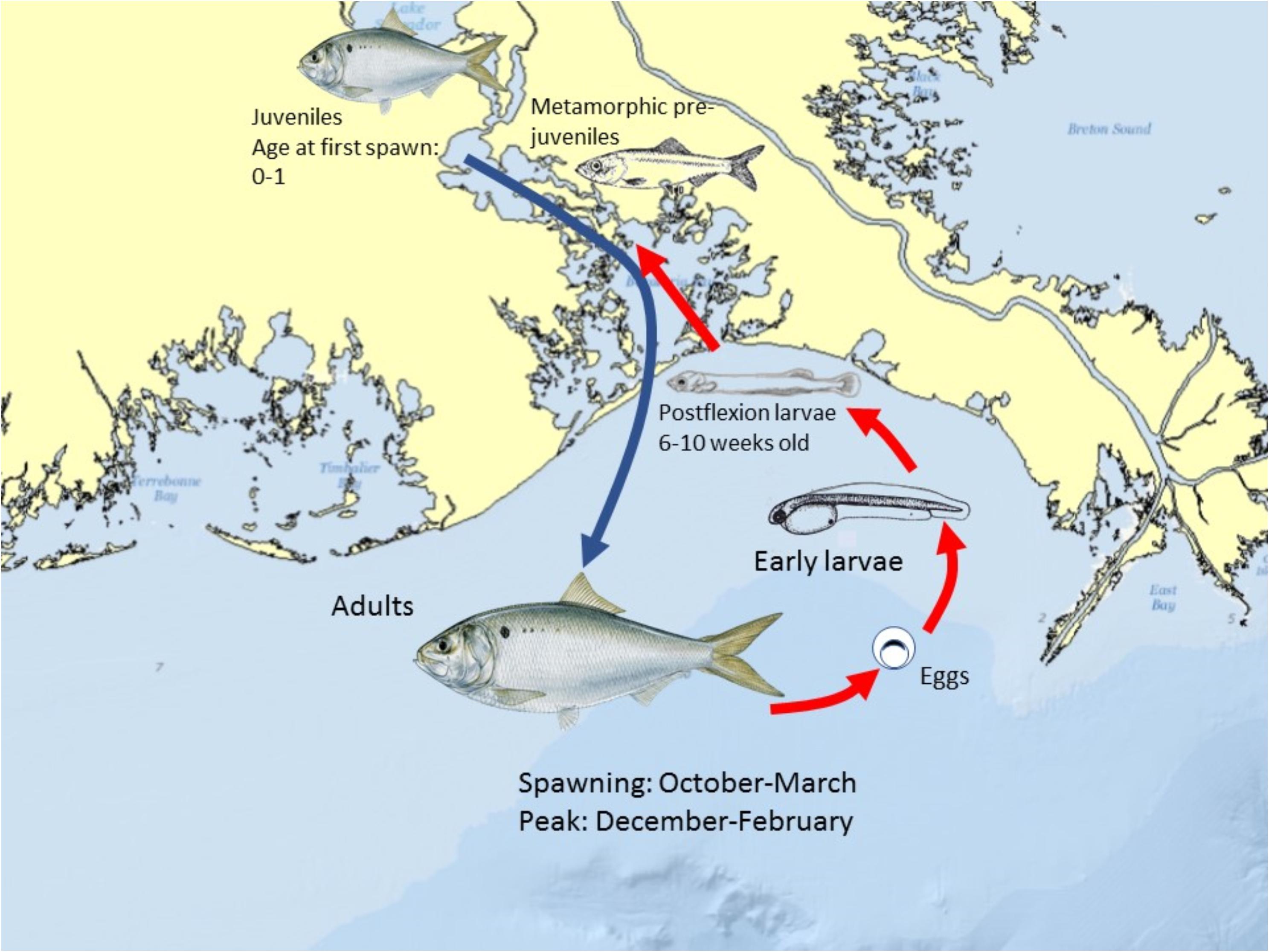
Figure 13. Schematic of the spawning and early life history of Gulf Menhaden in the northern GoM. Peak spawning occurs between December and February, with growth to the advanced larval largely completed by early-mid May, prior to the earliest dates Deepwater Horizon oil came ashore in coastal Louisiana. Menhaden color graphics with permission of the artist Diane Rome Peebles®.
Spawning occurs in relatively shallow coastal waters, as Shaw et al. (1985) found the highest densities of eggs in 10–23 m water depths. Fertilized eggs hatch rapidly, followed by yolk-sac and advanced larval development in 6–10 weeks, when postlarvae migrate into the upper parts of estuaries, and where they finally metamorphose into juveniles. Furthermore, while in the upper estuary, juveniles are highly tolerant of relatively low salinity conditions (Figure 13; Shaw et al., 1988; Deegan, 1990; SEDAR, 2018). It is likely that the bulk of Gulf Menhaden postlarvae migrated from coastal waters and into juvenile rearing areas in the upper estuary by the time DWH entered the lower estuaries (Figure 13). If the peak of offshore spawning ended in February, a 6–10-week development period to postlarval stage occurred by late April to early May. Since appreciable quantities of oil did not appear in coastal areas of Louisiana until late May and early June (Figures 11, 13; Deepwater Horizon Natural Resource Damage Assessment Trustees, 2016; Turner et al., 2019a), the bulk of Gulf Menhaden larvae, postlarvae, and early juveniles would not have been exposed to DWH oil. While large juvenile and adult Gulf Menhaden were initially exposed to relatively high levels of PAHs presumably from DWH oil (Olson et al., 2015), by 2013 the PAH levels were diminished, probably because these later sampled fish were spawned after the DWH event. Given the timing of spawning and lack of spatiotemporal overlap of larvae with DWH oil, along with the fact that Gulf Menhaden are considered to be a single panmictic population, we concluded their vulnerability to the DWH spill to be low. Because of their large population size comprising a unit stock, high rate of intrinsic population growth, and high population fecundity, we consider Gulf Menhaden to be highly resilient. Summary Evaluation: Vulnerability – Low, Resilience – High.
Sciaenid Fishes – Spotted Seatrout and Red Drum are, arguably, the dominant target species of recreational anglers fishing in estuarine waters of the northern GoM. Spotted Seatrout usually account for the highest recreational harvest annually in the northern GoM, and Red Drum (aka Redfish) are highly prized by anglers (Perret et al., 1980; VanderKooy, 2001; Supplementary Figures 6, 7). State fishing regulations and degree of management control vary widely for the two species, which contributes to their variable population status as managed entities (Murphy and Crabtree, 2001; Bortone, 2003; Herdter et al., 2019). Although both species are members of the family Sciaenidae (i.e., drums and croakers), Spotted Seatrout and Red Drum differ in the essentials of their life history and exhibit some degree of estuarine habitat partitioning (Wakeman and Ramsey, 1985; Moulton et al., 2017). Spotted Seatrout can live up to ages 8–9, but the majority of animals in catches are age 4 and younger (Nieland et al., 2002; DeVries et al., 2003; Herdter et al., 2019), whereas Red Drum are capable of living in excess of 30 years (Wilson and Nieland, 1994). Sexual maturity of females is essentially complete by age 1 in Spotted Seatrout (Nieland et al., 2002; Herdter et al., 2019) but not until age 6 or greater in Red Drum (Wilson and Nieland, 1994). Spawning seasons also differ between the species, with Spotted Seatrout primarily spawning from May to August (Helser et al., 1993) with peaks varying across the GoM (Brown-Peterson et al., 2002). In Louisiana, there are two peaks in Spotted Seatrout female GSI (gonadosomatic indices): in April-May and again in August (Brown-Peterson et al., 2002). Red Drum have a spawning peak in mid-August to early September in the northern GoM (Wilson and Nieland, 1994) and September-October off the west Coast of Florida (Lowerre-Barbieri et al., 2019). Tagging data for the species indicate highly localized populations of Spotted Seatrout, with excursions beyond natal estuaries being infrequent (VanderKooy, 2001; Zarada et al., 2019). While Red Drum spawn in the ocean more seaward than Spotted Seatrout (Saucier and Baltz, 1993; Lowerre-Barbieri et al., 2016, 2019; Moulton et al., 2017; Zarada et al., 2019), straying of spawning Red Drum among estuarine populations is also infrequent (Lowerre-Barbieri et al., 2019). Post-spawning, Red Drum adults may extend their oceanic distributions over ∼150 km alongshore and up to 90 km offshore (Lowerre-Barbieri et al., 2019), whereas Spotted Seatrout rarely occur in oceanic waters beyond passes and channels (Saucier and Baltz, 1993). Neither species was collected during winter nor in the spring larval sampling of Sciaenids in ocean waters off Louisiana (Cowan and Shaw, 1988), which is reflective of their strong affinity for estuarine nurseries.
The abundance of Spotted Seatrout and Red Drum has been tracked in gill net-based fishery-independent sampling for several decades (Figure 14). Spotted Seatrout have been in long-term decline throughout the northern GoM (Figure 14; Herdter et al., 2019), likely due to intensive fishing and habitat degradation. Red Drum were subject to a commercial moratorium beginning in 1988 in EEZ waters (i.e., during their late summer-autumn spawning season and post-spawning dispersal) and abundance off Louisiana and Alabama has increased since about 2005 (Figure 14). The abundance of Red Drum increased in all monitored estuaries we evaluated between 2009 and 2010 (Figure 14) and peaked in Barataria Bay and off Alabama prior to 2015. In contrast, Spotted Seatrout abundance decreased everywhere between 2009 and 2010, and increased only in Barataria Bay post-2011 (Figure 14).
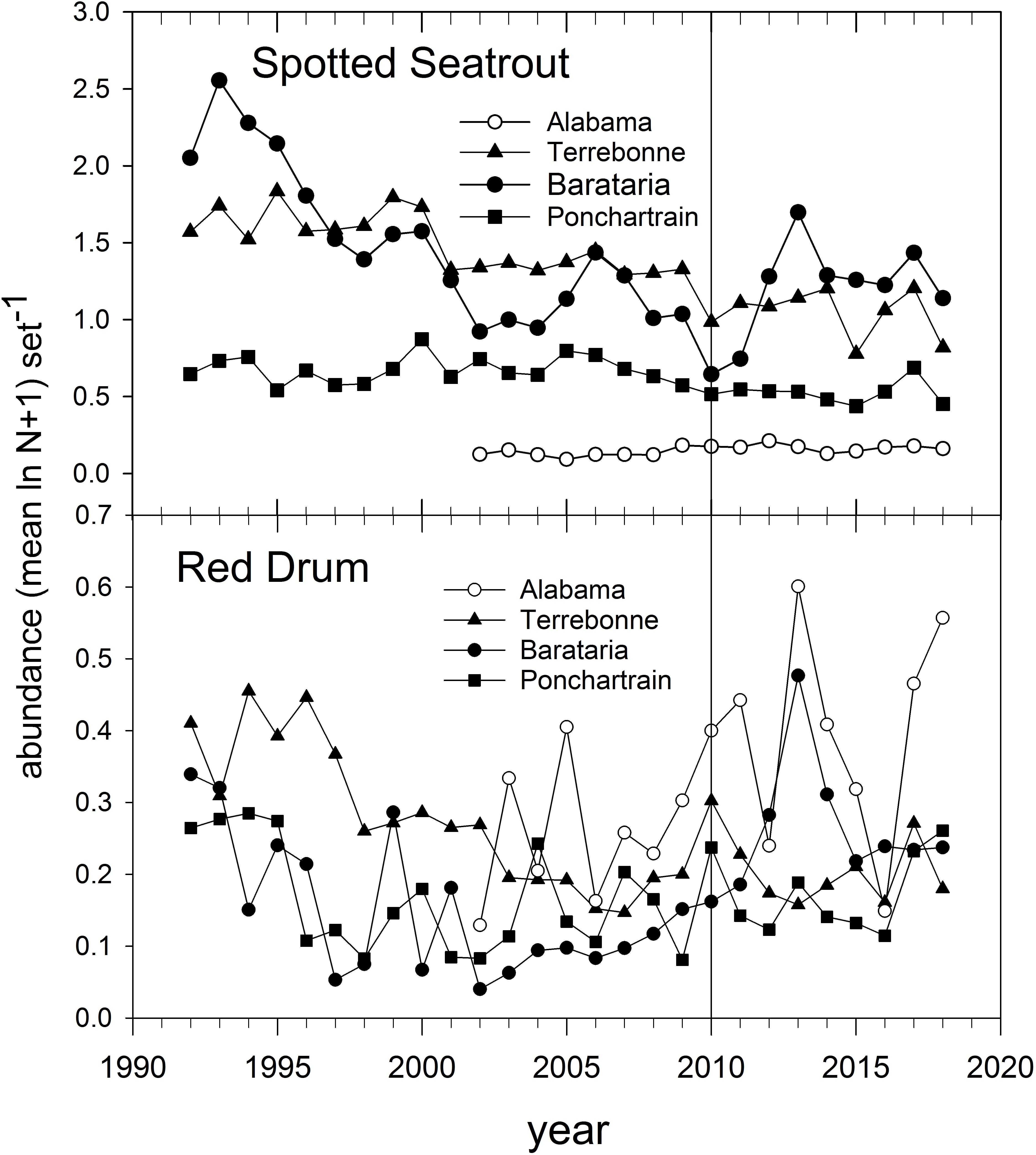
Figure 14. Annual relative abundance of Spotted Seatrout and Red Drum in fishery-independent gill net surveys off Alabama and in three Louisiana basins. Data are from the Alabama Marine Resources Department and the Louisiana Department of Wildlife and Fisheries gill net sampling programs.
Because of the estuarine dependence of juveniles and adults of both species, they were subject to exposure in marsh areas contaminated with oil. Pulster et al. (2020) documented high concentrations of PAH metabolites in bile (indicative of recent exposure) of large juvenile and adult Red Drum sampled in Barataria and Terrebonne Bays in summer 2011. Likewise, Powers and Scyphers (2016) documented significantly depressed juvenile Red Drum growth in mesocosms deployed adjacent to oiled marsh edges, as compared to control sites. The differential population trajectories of Spotted Seatrout and Red Drum post-DWH (Figure 14) may be indicative of the relative tolerance to pollution-related effects (Figure 14), but because both species spend so much of their life cycles in areas potentially impacted by DWH oil, we considered both to be of moderate vulnerability to DWH effects. It is unclear if there were differential impacts of freshwater discharges on the species, although Spotted Seatrout generally can be found in deeper and more saline areas of estuaries than Red Drum (Moulton et al., 2017; Livernois et al., 2020). We judged both species to have moderate resilience potential but for different reasons. In the case of Spotted Seatrout, spawning near the mouths of estuaries occurs over an extended time period with two apparent spawning peaks (Brown-Peterson et al., 2002), the second of which (August) would have occurred after fresh DWH oil was absent from the water column. Most Spotted Seatrout are capable of spawning at the end of their first year of life, and their reproductive strategy appears to be r-selected. While Red Drum have an extended juvenile stanza of up to 6 years, their longevity and spawning peak (late summer-autumn), after DWH oil had transited coastal ocean spawning locations, potentially mitigate DWH impacts on Red Drum populations. Summary Evaluation: Vulnerability – Medium, Resilience – Medium.
Plankton – Plankton is a broad term used to describe aquatic and marine species that cannot swim against water currents. Plankton are cosmopolitan and taxonomically and functionally diverse. Taxa are often subdivided by taxa according to their size and how they assimilate energy. For the vulnerability-resilience analysis, we focused on three planktonic systems critical to estuarine and coastal food webs and ecosystem functioning: phytoplankton, mesozooplankton (200–2,000 μm), and gelatinous plankton (>2 mm).
Northern GoM phytoplankton abundance in coastal and nearshore waters is primary influenced by river discharge, dissolved nitrogen and phosphorous concentrations, photosynthetically active radiation (i.e., light availability), and water temperature. Phytoplankton concentrations vary seasonally with these factors, typically peaking in summer and at minimum in winter (Redalje et al., 1994). While there are numerous, temporally focused and spatially discrete studies on phytoplankton in northern GoM estuarine and coastal waters (e.g., Hu et al., 2011; Chakraborty and Lohrenz, 2015; Gomez et al., 2018), there are few time-series that bookend the DWHOS in these ecosystems (but see Parsons et al., 2015). One reason is that interpretation of remotely sensed chlorophyll-α (an established method for estimating ocean primary productivity) in coastal and nearshore water requires caution due to complexity introduced by colored dissolved organic matter and suspended solids (Gilerson et al., 2007; McKee et al., 2007; Blondeau-Patissier et al., 2014). The best proxies are estimates from the adjacent shelf that include edges of coastal waters; though, these too have some uncertainty (D’Sa and Miller, 2003; Hu et al., 2003).
These time-series reveal variable responses by northern GOM phytoplankton in space and time to DWH oiling. Weekly estimates using coupled satellite and numerical modeling indicated anomalously high chlorophyll-α concentrations along Louisiana’s central coast 3 weeks after the oil well was capped (Hu et al., 2011). Inter-annual studies show primary productivity in impacted waters southeast of the Louisiana Delta were below-average in 2011–2013 even after accounting for non-DWH environmental factors including river discharge (Li et al., 2019). This finding corroborates the 85% reduction of in situ chlorophyll May–October 2010 from monthly data off the central Louisiana coast relative to a 20-year baseline (Parsons et al., 2015).
Reduced phytoplankton production may be linked to crude oil toxicity. Exposure to crude oil’s chemical constituents can cause specific physiological harm to phytoplankton, including damage to photosynthetic processes and nuclear mechanisms regulating growth (see Ozhan et al., 2014 and references therein). However, the contrasting observations of increases (or decreases) in phytoplankton at different spatiotemporal scales strongly suggests not all phytoplankton taxa respond the same to oiling. Some groups like diatoms and cyanobacteria tolerate exposure to oil and dispersants better than ciliates and phytoflagellates. Even within a group (like diatoms), toxicity sensitivity is heterogeneous, possibly influenced by factors like cell size (González et al., 2009).
Overall, phytoplankton are considered highly vulnerable to crude oil exposure based on their inability to move away from oiled areas, their direct acute and chronic exposure, their overlap in space and time with oiled waters, and their demonstrated sensitivity to crude oil’s chemical constituents. A key takeaway from the numerous studies on crude oil exposure on phytoplankton is that taxa responses are dissimilar. These differences, combined with their life history and ecological traits, suggested that phytoplankton, as a whole group, are highly resilient to oil spills. Phytoplankton are numerous (r-selected), have short generation times (i.e., hours-days), high dispersal rates and connectivity, and are somewhat resilient to co-varying stressors such as freshwater inputs. These V-R scores are conditional as we lack a baseline, in situ phytoplankton time-series to measure post-DWH data against in northern GoM coastal and nearshore waters. Summary Evaluation: Vulnerability – High, Resilience – High.
Mesozooplankton are heterotrophic grazers and carnivores. Assemblages in northern GoM nearshore and coastal waters include the larvae of six of our 13 key species (i.e., sciaenid fishes, Menhaden, Oysters, Brown and White shrimp, and Blue Crab). Copepods numerically dominate—accounting for over 80% of total abundance (Carassou et al., 2014; Selle, 2020). Mesozooplankton are the primary trophic energy transfer pathway between phytoplankton and plankton predators like larval fishes and Gulf Menhaden. Seasonal production typically tracks with phytoplankton, with maxima in summer and minima in winter. The most robust mesozooplankton time-series on the inner shelf is the Fisheries Oceanography of Coastal Alabama (FOCAL) data set, which includes near-monthly (and depth-discrete) plankton collections along a transect extending from the mouth of Mobile Bay to the 35 m contour (Hernandez et al., 2010a; Carassou et al., 2012). Using a portion of the FOCAL time series, Carassou et al. (2014) found that mesozooplankton assemblage during the early part of the oil spill (May and June 2010) was different relative to baseline conditions (May and June 2005–2009); however, recovery was rapid, and the baseline assemblage structure was observed by July and August 2010. Changes in mesozooplankton abundance during the oil spill (May–August 2010) relative to the pre-oil spill period (May–August 2005–2009) varied by taxa, with numerous taxa (e.g., calanoid and cyclopoid copepods, ostracods, bivalve larvae and cladocerans) having higher densities during the oil spill. Overall, their results suggest that the mesozooplankton community was relatively resilient to oil spill impacts.
In contrast to the resilience at the mesozooplankton community-level, numerous laboratory studies have demonstrated that chemical constituents in crude oil and dispersants have lethal and sub-lethal effects on individual taxa, calanoid copepods in particular. Exposure to polycyclic aromatic hydrocarbons (PAHs) can cause narcosis (Barata et al., 2005), changes in feeding (Saiz et al., 2009), and reproduction (Suderman and Marcus, 2002; Bellas and Thor, 2007; Calbet et al., 2007; Seuront, 2011), and development (Bejarano et al., 2006) in copepods. Acartia tonsa (a common estuarine and coastal copepod) exposed to PAH compounds fluoranthene, phenanthrene and pyrene reduced egg production and recruitment rates by 60–88% (Bellas and Thor, 2007). Pyrene toxicity on egg production was amplified with exposure to ultraviolet (UV) light, decreasing recruitment and survival of females by 3–16 fold. The photoinduced toxicity of PAHs is key as oiling occurred during boreal summer when UV radiation in the northern GoM is at its seasonal peak. Lethal effects of crude oil were also found when field collected mesozooplankton were exposed to increasing oil concentrations and dispersant in mesocosms (Almeda et al., 2013). The combination of crude oil and dispersant was even more lethal to mesozooplankton. At the 1:20 ratio of dispersant to oil (a common ratio used in the treatment of oil spills), and based on loading concentrations, dispersant and dispersant-treated oil were 2–3 times more toxic than oil alone. Exposure to UV light exacerbated these effects by increasing mesozooplankton mortality by 35% on top of the effect found for crude oil and dispersant. Despite the adverse effects observed under laboratory conditions, direct extrapolations to effects under field conditions are challenging due to differences in both exposure conditions and concentrations.
Despite the individual physiological sensitivities to crude oil and dispersant components, mesozooplankton at the community level were seemingly resilient. Three hypotheses may explain this disconnect. First, fisheries closures resulted in higher predation rates on small, planktivorous fishes like gulf menhaden and bay anchovies, releasing mesozooplankton populations (Carassou et al., 2014). Second, increases abundance of oil-degrading microbes strengthened microbial-zooplankton trophic connections (Graham et al., 2010; Chanton et al., 2012), providing a competitive edge to zooplankton taxa able to access that resource pool. Third, mesozooplankton may have adapted to crude oil exposure. Wild populations of the calanoid copepod Eurytemora affinis seemingly increased their evolutionary tolerance to crude oil after 8 generations after the DWH spill (Lee et al., 2017). Post-spill populations showed increased survival and rapid development in the presence of crude oil relative to pre-spill populations. Using a 9- to 10-day generation time (Lee et al., 2017), this timescale suggests E. affinis populations were more tolerant to oil exposure 2.5 months post-DWHOS. This timeframe coincides with the July 2010 recovery of mesozooplankton communities (Carassou et al., 2014). Nonetheless, identifying which hypothesis (if any) may explain resilience contrasts across mesozooplankton individuals, populations, and communities may be difficult since it will require disentangling varying species-specific sensitivity to crude oil toxicity, predatory and competitive interactions, and inherent patchiness in time and space (Walsh, 1978).
Mesozooplankton are considered highly vulnerable to crude oil exposure based their overlap in space and time with oiled waters, their inability to avoid these waters, their direct acute and chronic exposure, and the lethal and sub-lethal effects of crude oil exposure on feeding and reproduction rates. Like phytoplankton, mesozooplankton responses were taxa-specific and highly variable, with some showing increased abundances post-spill. These contrasts, combined with their life history traits, indicate that mesozooplankton overall are highly resilient to oil spills. They are numerous (r-selected), have short generation times (i.e., days-weeks), high dispersal rates and connectivity, and recover quickly from co-varying stressors such as freshwater inputs. Summary Evaluation: Vulnerability – High, Resilience – High.
Gelatinous plankton (“jellies”) are often overlooked in northern GoM coastal ecosystems; yet, the region supports a diverse array of taxa who provide numerous ecosystem services (Graham et al., 2003), including being prey for apex predators such as tuna, billfish, sunfish, and sea turtles (Arai, 2005; Houghton et al., 2006; Cardona et al., 2012; Robinson et al., 2015). Two gelatinous functional groups were considered for the V-R analysis. The first were large, gelatinous plankton predators like scyphomedusae (common coastal taxa include Aurelia spp. and Chrysaora spp.) and ctenophores that are voracious consumers of mesozooplankton and fish eggs and larvae (Cowan and Houde, 1993; Purcell, 1997; Shiplett, 2011). The second group were pelagic tunicates (i.e., salps, doliolds, pyrosomes, and larvaceans), that play key roles in biogeochemical cycling. These taxa filter and consume microbes and phyto-detritus particles as small as 0.1μm (Sutherland et al., 2010), producing mucus-rich aggregates (i.e., marine snow) and fecal pellets that are metabolized by a suite of organisms as they sink (Alldredge, 1976).
Both groups potentially affected the fate of Macondo crude oil. Dolioletta gegenbarui, an abundant Gulf of Mexico thaliacean, consumed and released oil droplets in their fecal pellets at rates that could potentially export 200 g of oil m–3 d–1 to the benthos (Lee et al., 2012). Gemmell et al. (2016) found Aurelia sp. scyphomedusae swimming through an emulsified suspension of crude oil droplets extruded copious amounts of mucus rich in carbon, nitrogen, and phosphorus as a stress response and chemical defense (Ducklow and Mitchell, 1979; Shanks and Graham, 1988; Pitt et al., 2009). This prodigious amount of mucus contained significantly higher densities of oil droplets relative to the surrounding waters and hydrocarbon degrading bacteria relative to control treatments. This outcome strongly suggests scyphozoan medusa may accelerate microbial degradation of crude oil by relieving nutrient limitation through mucus production.
Gelatinous plankton are tolerant overall of crude oil crude oil and PAH compared to zooplankton like copepods, larval fish, and other invertebrates (see Almeda et al., 2013 and references therein). Toxicity tests of Macondo crude oil and Corexit 9500 dispersant on Aurelia spp. ephyra showed crude oil alone did not cause acute toxicity, but the combination of oil and dispersant resulted in marked mortality and physical and behavioral abnormalities (Echols et al., 2016). Almeda et al. (2013) found adult Aurelia sp. survived in crude oil concentrations ≤25 μL L–1 after 6 days of exposure; though, exposure did cause tissue damage and abnormal swimming. Larval stages were more vulnerable. Aurelia spp. ephyra and the larvae of the lobate ctenophore Mnemisopsis leidyi decreased with increased exposure duration and oil concentration, with 100% mortality at 25 μL L–1. Altered swimming behavior (e.g., low mobility, slow swimming speed) was also observed in larval stages.
Limited evidence suggests the negative effects of DWH oiling on individual gelatinous plankters did not translate into population effects. Coastal doliolid densities were reduced significantly relative to historical baselines in coastal Alabama throughout the summer after the spill but recovered August 2010 (Carassou et al., 2014). However, we are not aware of other data to corroborate or contrast this finding. The relatively quick recovery further evidences the challenges in extrapolating DWH effects on plankton individuals under controlled laboratory conditions and populations exposed in the field.
Gelatinous plankton life-history traits may partially explain population recoveries. Gelatinous plankton taxa can quickly dominant plankton biomass at local scales through rapid rates of sexual or asexual reproduction. Time to first reproduction varies from 2 days (in the case of larval ctenophore reproduction; Martindale, 1987) to 1–3 months for scyphozoan polyps to produce ephryae (Purcell et al., 1999; Purcell, 2007). Scyphozoans with benthic polyp stages are highly fecund; an individual polyp can produce 40 ephyrae per strobilation event (Lucas, 2001). This rate can translate to 10 billion ephyrae produced annually for a single port (Duarte et al., 2012). Moreover, polyps can strobilate repeatedly, are perennial, and produce for years (Arai, 1997). The individual life span of gelatinous plankter can vary from weeks (ctenophore) to 1 year (scyphomedusae) in subtropical waters. This reproductive strategy can potentially yield millions to billions of jellyfish per year (Robinson and Graham, 2013). Overall, these life-history traits allow some gelatinous plankton taxa like the scyphozoans to tolerate and succeed in otherwise environmentally stressful conditions. Summary Evaluation: Vulnerability - High, Resilience – Medium.
Ichthyoplankton Nearly all marine fishes in the GoM have pelagic egg and larval stages (ichthyoplankton) which are part of the plankton for durations ranging from days to months, depending on the species. Under “natural” conditions ichthyoplankton mortality rates approach 100%, primarily due to predation (Fuiman, 2002; Houde, 2008), therefore a major concern during the DWH event was the impact of additional mortality on these vulnerable life stages (Hernandez et al., 2016). The temporal and spatial patterns of ichthyoplankton distribution and abundance are largely determined by adult fish spawning behaviors (e.g., when and where spawning occurs), as well as oceanographic processes that transport (or locally retain) eggs and larvae, and larval fish behaviors (e.g., vertical migrations; Hernandez et al., 2010a). The phenology of larval fish occurrence in the coastal and nearshore waters of the northern GoM has been described by numerous surveys and synthesis works (e.g., Ditty, 1986; Ditty et al., 1988; Hernandez and Shaw, 2003; Hernandez et al., 2003, 2010a,b); therefore we have a reasonable idea of which species were present and at risk to oil and dispersant exposure during DWH.
Most laboratory studies examining the impact of oil exposure on the development and survival of fish eggs and larvae have reported sublethal or lethal effects (Fodrie et al., 2014), and collectively suggest a wide range of developmental impacts. For example, numerous sublethal effects were observed in recently hatched Red Drum (Sciaenops ocellatus) larvae after eggs were exposed to weathered slick oil, including reductions in brain and eye size, and abnormal cardiac and nervous system development (Khursigara et al., 2017; Xu et al., 2017). Magnuson et al. (2018) also reported impaired ocular development in Red Drum and Sheepshead Minnow (Cyprinodon variegatus) larvae, which resulted in observable reductions in behavioral (optomotor) responses to external stimuli. In addition, numerous studies have reported significant impacts at the genomic level after oil exposure, resulting in alterations of gene expression, organ development, cellular pathways, and immune responses (e.g., Whitehead et al., 2012; Dubansky et al., 2013), as well as the combined effects of oil exposure with variable environmental factors, such as dissolved oxygen, temperature, salinity and light exposure (e.g., Alloy et al., 2017; Serafin et al., 2019; Rodgers et al., 2020). Collectively, these sublethal effects suggest that fish larvae exposed to DWH oil in situ would have had impaired survival abilities needed to evade predators and locate prey. If these laboratory results are indicative of the actual exposures experienced by coastal and nearshore ichthyoplankton during DWH, then it is reasonable to assume that additional mortality would have resulted.
In spite of the laboratory results, field-based evidence for detrimental impacts to ichthyoplankton during the DWH oil spill has been equivocal. Several studies using plankton survey data have estimated the degree of overlap between DWH surface oil and larval fishes, but these analyses were largely limited to offshore pelagic taxa (Muhling et al., 2012; Rooker et al., 2013). Using SEAMAP plankton survey data, Chancellor (2015) estimated the proportions of fish larvae potentially exposed to DWH oil for a suite of taxa. Overall, the exposure probabilities were relatively low for most taxa with larval stages common in nearshore waters, such as Atlantic Bumper (Chloroscombrus chrysurus, 6.15%), Scaled Sardine (Harengula jaguana, 7.3%), Sand Seatrout (Cynoscion arenarius, 12.84%), Red Drum (0.02%), Spanish Mackerel (Scomberomorus maculatus, 10.59), and Harvestfish (Peprilus paru, 4.37%), among others. Interestingly, the highest estimated proportion of exposure among all taxa examined was for the larvae of Spotted Seatrout (26.27%), a common coastal resident and recreational fisheries target. Overall, the limited availability of nearshore SEAMAP sampling stations, however, may have resulted in an underestimation of exposure for many taxa.
Other field-based studies have examined pre- and post-DWH abundances of fish larvae, as well as potential DWH effects on larval feeding and growth. Hernandez et al. (2016), for example, reported the abundances of larval Red Snapper (Lutjanus campechanus) collected relatively near shore (20 m isobath) were highly variable, but did not differ between surveys conducted before (2007–2009), during (2010) and after (2011, 2013) the DWH oil spill. However, the body condition (proxy for growth) of larval Red Snapper collected in 2010, 2011, and 2013 was relatively poor compared to pre-DWH larvae. In contrast, Ransom et al. (2016) found that body condition of larval Spanish Mackerel was relatively higher during the DWH event than years before (2007-2009) and after (2011), and reported no significant changes in larval abundances or diet (based on stable isotopes of C and N). The contrasting results in body condition observed during DWH for larval Red Snapper and Spanish Mackerel suggest that impacts are taxon-specific, and therefore caution should be used when categorizing impacts on “ichthyoplankton” collectively.
Like mesozooplankton, ichthyoplankton are considered highly vulnerable to crude oil exposure based their overlap in space and time with oiled waters, their inability to avoid these waters, their direct acute and chronic exposure, and the lethal and sub-lethal effects of crude oil exposure on larval development. Ichthyoplankton overlap with oil was taxon-specific and highly variable in time and space, as were abundances and responses (e.g., body condition) during DWH relative to baseline conditions. Overall, ichthyoplankton were highly resilient to the DWH oil spill. Most of the nearshore and coastal fish species that may have been impacted by the oil spill are highly fecund, have extended spawning periods (several months), and are wide-ranging in their distribution across the northern GoM. As such, high dispersal rates and connectivity would allow for relatively quick recovery. Summary Evaluation: Vulnerability – High, Resilience – High.
Benthic Infauna Considerable research on impacts to nearshore benthic communities has been published in the wake of DWH focused primarily on beach sand habitats (e.g., Kostka et al., 2011; Bik et al., 2012; Bejarano and Michel, 2016; Michel et al., 2017; Huettel et al., 2018; Bociu et al., 2019) and marsh invertebrate infauna (Brunner et al., 2013; Michel and Rutherford, 2013; Fleeger et al., 2015; Murawski et al., 2016; Rabalais and Turner, 2016; Zengel et al., 2016; Deis et al., 2017; Fleeger et al., 2018). Additionally, Schwing et al. (2020) evaluated impacts and recovery potential for offshore benthic environments.
Beach environments are unique in that effective and efficient oil recovery is possible and emphasized in oil spill response because many are considered “amenity” recreation areas of high public value and use. Impacts to beaches occur both from the initial and chronic oiling, but also through escalating levels of “recovery impacts” (Michel et al., 2017), including manual pick up of surface oil, augering, beach grooming/tilling with mechanical devices, submerged oil mat removal, and excavation of sub-surface oil deposited and thereafter covered. The intensity of these activities combined with the degree of initial oiling can retard recovery of beach fauna by months to years (Bejarano and Michel, 2016; Michel et al., 2017). Indeed, sediment-oil agglomerates buried in aerobic sediments can take decades to degrade (Bociu et al., 2019). Microbial composition of sand beaches experiencing oiling was dramatically different in short-term contrasts between control and treatment locations (Kostka et al., 2011; Bik et al., 2012), although microbial populations returned to pre-spill profiles within a year of the event (Huettel et al., 2018). Based on a meta-analysis of beach oiling from historical oil spills, Bejarano and Michel (2016) concluded that recovery of beach benthic communities is highly variable and depends primarily upon site-specific conditions (degree of exposure, sediment grain size, etc.) as well as oil depth penetration, level of response intervention and species-specific life history traits. Depending on the combination of such factors, recovery may take from months to years.
Unlike beach habitats, salt marsh benthic communities (particularly in the heavily oiled Louisiana marshes) are not amenable to active oil response measures and natural attenuation is the recommended method of oil removal. Michel and Rutherford (2013) ranked a number of response measures specifically for marsh habitats, concluding that natural attenuation and the use of sorbents were the least detrimental, and mechanical oil removal, sediment reworking/tilling and vegetation cutting had the most adverse habitat impacts. Because of the transgression of large quantities of oil into the marshes during the DWH event (Figures 1, 11; Nixon et al., 2016; Turner et al., 2019a) high levels of PAHs and other oil components penetrated into soft bottom marsh habitats (Turner et al., 2019a). Even 8 years after the DWH event, some locations exhibited PAH concentrations an order of magnitude or greater than background (Turner et al., 2019a). At the salt marsh edge, extensive oiling killed above- and below-ground plant biomass resulting in collapse of the marsh edge. Fleeger et al. (2015, 2019) noted the importance of these “foundation” plant species (e.g., Spartina), soil quality and benthic algae in promoting the recovery of meiobenthos and burrowing epi-benthos (Zengel et al., 2016). Heavily oiled areas exhibited significant reductions in most infaunal taxa, including nematodes, polychaetes, copepods, amphipods, annelids, bivalves and other species (Fleeger et al., 2019). Whereas nematodes and some polychaetes rebounded rather quickly in moderately and heavily oiled sites, kinorhynchs, other polychaetes, ostracods, and juvenile gastropods recovered more slowly, and were not fully recovered even 6.5 years after initial oiling (Fleeger et al., 2019). Poor soil quality was associated with the slow recovery at these sites. Overall, the effects of oiling on benthic infauna were highly variable, dependent on the degree of oiling, effects of recovery impacts, and influenced by the weathering of oil in sediments. Summary Evaluation: Vulnerability – High, Resilience – Medium to High (depending on life history, local conditions and type of habitat).
Bottlenose Dolphin – The common Bottlenose Dolphin is found across the globe, including within the nearshore and coastal waters of the DWH surface oiling footprint (Deepwater Horizon Natural Resource Damage Assessment Trustees, 2016; Figure 1). Within this footprint, the National Oceanic and Atmospheric Administration (NOAA) manages this protected species as 11 “biologically meaningful stocks”: nine resident stocks within each of the bay, sound, and estuarine (BSE) bodies of water along the coastline (from Terrebonne and Timbalier Bays in Louisiana to St. Andrews Bay, FL), and two coastal stocks that typically range from the coastline to the 20 m-isobath (the GoM Northern Coastal Stock and the GoM Western Coastal Stock; Deepwater Horizon Natural Resource Damage Assessment Trustees, 2016). During the spill, BSE and coastal dolphins (in addition to continental shelf and oceanic stocks/species) were observed swimming in DWH oil slicks (Aichinger-Dias et al., 2017), putting them at risk of inhaling and aspirating toxic airborne and waterborne oil constituents (Takeshita et al., 2017). In the years during and following the spill, over 1,000 cetaceans (mostly common Bottlenose Dolphins) stranded dead along the northern GoM coastline, resulting in the largest unusual mortality event on record in the northern GoM (Litz et al., 2014; Venn-Watson et al., 2015b). The dead dolphins had a high prevalence of lung and adrenal disease, as well as high prevalence of late-term pregnancy loss (Venn-Watson et al., 2015a; Colegrove et al., 2016). In the years following the spill, researchers studying live dolphins in Louisiana and Mississippi found a variety of oil-related adverse health effects that were consistent with the observed pathologies from stranded dolphins. The effects documented in live dolphins included lung disease, poor reproductive success, abnormal stress response, and immune system dysfunction (Schwacke et al., 2014; Lane et al., 2015; De Guise et al., 2017; Kellar et al., 2017; Smith et al., 2017).
Their respiratory physiology and behavior make dolphins (and cetaceans in general) especially vulnerable to surface oil spills like DWH because of their deep breaths at the air-water interface, where the highest concentrations of volatile organic compounds, and aerosolized and surface oil can be found. Inhaled toxicants are not filtered through turbinates (as in other mammals) before absorption into the large blood supply in dolphins’ lungs, which gets pumped directly to the heart. After DWH, it is also clear that dolphins did not swim away from or avoid surface oil (Aichinger-Dias et al., 2017). The BSE dolphins, especially, appear to have maintained their relatively small usage areas despite DWH oil contamination (Wells et al., 2017). As compared with Bottlenose Dolphins comprising coastal stocks, dolphins in Barataria Bay exhibit a high degree of site fidelity, are confined to small home ranges and exhibit year-round residency, all of which made them particularly vulnerable to the presence of DWH oil (Wells et al., 2017).
Relative to other marine wildlife, marine mammals have a low maximum rate of population increase (rmax), and therefore, have slow recoveries after facing reductions in their populations (e.g., Davidson et al., 2012). As keystone predators in the northern GoM marine ecosystem, common Bottlenose Dolphins are at especially high risk from perturbations in their habitats. Compared to lower trophic level species, they have low productivity due to a high body mass at weaning and a low number of births per year. In combination with their small usage areas (BSE dolphins) and/or narrow habitat ranges (coastal dolphins), their k-selected life histories mean that northern GoM Bottlenose Dolphins are less resilient against large-scale threats/risks such as DWH. Models of the GoM bottlenose dolphin stocks within the DWH surface oil spill footprint projected that most stocks lost over 50% of their population, and that each stock will require three to five decades to recover to their pre-spill abundance levels (see Table 4.9-10 in Deepwater Horizon Natural Resource Damage Assessment Trustees, 2016). These compromised stocks are likely even more vulnerable and less resilient against any additional stressors that they may face in the near future. Summary Evaluation: Vulnerability – Medium to High (depending on the degree of site fidelity and home range size), Resilience – Medium to Low (depending on the range of distribution across stocks).
Community – Level Analyses
We conducted two types of community-level analyses, (1) time-series summaries of mixed-species catches in fishery-independent trawl surveys by estuary (Figures 3, 4), and (2) multivariate analyses exploring species associations and evaluating the strength of coincidentally collected environmental information affecting species abundance and distribution patterns (Figures 5–7 and Supplementary Figures 8, 9). These analyses provided complementary information on the responses to variability in environmental conditions affecting abundance and distribution, as well information on pre- and post-DWH time frames to evaluate whether changes in species composition were associated in time with the DWH oil spill.
For the Louisiana and Alabama information, we evaluated overall abundance (numbers standardized trawl haul–1) for all species and for three subsets of species, including all finfishes, invertebrate species, and those typically considered freshwater-associated (Figure 3 and Supplementary Table 1). The overall abundance of trawl-caught species was relatively high during 2010 in all three Louisiana basins (Figure 3) but in all cases had declined modestly post-DWH. These changes were primarily driven by lower overall catches of finfishes and especially invertebrates (Figure 3). Invertebrate abundance (primarily shrimps and crabs) increased in 2010 in Barataria Bay but declined thereafter to recent time series lows (Figure 3). Invertebrate abundance in Terrebonne and Pontchartrain basins peaked in 2011 and 2012, respectively, and either fluctuated without trend (Pontchartrain) or declined to time-series lows (Terrebonne). The abundance of freshwater species, particularly in Barataria and Terrebonne Bay trawl catches has increased since about 2000 and peaked in the mid-2010s after DWH (Figure 3). The increase in abundance of freshwater species was inversely correlated with the decline in average annual salinity (Figure 8) in Barataria Bay (r = −0.69, p = 0.001).
Alabama trawl survey catches peaked at near time-series high in 2010 and had declined somewhat since then (Figure 4). Likewise, finfish, invertebrate and freshwater species abundance has remained relatively stable since 2010, similar to the findings of Moody et al. (2013). Freshwater species abundance has fluctuated annually with no obvious trend, and the peak in Alabama in 2005–2006 (Figure 4) coincides with hurricane Katrina in 2005 and coastal flooding associated with its aftermath.
The overall species catches in Florida estuaries (Figure 4) confirmed the general post-DWH trend of catch stability as seen in Alabama. For those years when Mississippi abundance data were publicly available (1973–2005) there was similarity between the Mississippi and Alabama trends, which has been confirmed in past analyses of trawl survey data, e.g., O’Connell et al. (2019) found more similarity in trawl survey catches between coastal Alabama and Mississippi than with Louisiana (Lake Borgne) catches. Data reviewed in Schaefer et al. (2016) confirmed the relative stability or short-term increases in fish abundance in coastal Mississippi pre- and post-DWH. The overall decline in catches in Alabama, Mississippi and Florida between the late 1990s and about 2005 reflects a pattern similar among all three states and is possibly indicative of regionally coherent environmental drivers.
Results of community-level patterns assessed in the SIMPROF analyses of Barataria Bay trawl catches revealed coherent changes in species assemblages and sub-structures of species groupings over the time series (Figure 5). The SIMPROF clustering of years with similar species assemblages revealed a few distinct stanzas since the mid-1990s. There was a notable progression in beta-diversity over time, with 2010 (the DWH year) through 2012 being relatively distinct from the years before and after this three-year period (Figure 6). The patterns were so distinct between pre- and post-DWH Barataria Bay trawl catches that they may be considered to constitute a regime shift (e.g., Lees et al., 2006; deYoung et al., 2008; Levin and Möllmann, 2015). The change in community structure post-DWH was most prominent in declining abundances of the species constituting the upper right section of the seriated heatmap figure (e.g., species from Southern Flounder to Star Drum; Figure 5), and which contribute to the declining trend in overall abundance for the region observed since 2010 (Figure 3). The species-clustering dendrogram (Figure 5) also identified a large group of species (Sheepshead to Sharptail Goby) that have generally increased in abundance over time, and especially post-DWH.
Results of the redundancy analyses (Figure 7 and Supplementary Figures 8, 9) revealed the importance of environmental variables and how various species align in multidimensional ecological space. There was a clear pattern of environmental change, as illustrated by the ordination of years along the two canonical axes and the relative associations of the abiotic factors to those axes visualized as vectors (Figure 7). In the case of the three environmental variables investigated (salinity, water temperature, and water clarity), they were generally opposed to each other. For example, conditions in the mid-1990s indicated the highest relative salinities and lower temperatures than the late-1990s through 2010s, and the post-DWH conditions were characterized by the highest relative water clarity, similar temperatures to the mid-1990s, and moderate-to-low salinities. Predominantly freshwater species such as Blue and Channel Catfishes occur in relatively warm, low-salinity conditions of moderate water clarity. Spotted Seatrout are consistently associated with more saline, relatively cooler waters within Barataria Bay than are Red Drum. Gulf Menhaden, Blue Crab, and White Shrimp appear somewhat associated with conditions influenced by ocean-water intrusions into the bay, whereas Brown Shrimp, Spot, Atlantic Croaker, and Bay Anchovy occupy neutral space along these environmental gradients (Figure 7). The time series of mean annual environmental measurements of temperature, salinity, and water clarity for Barataria Bay (Figure 8) confirmed a long-term decline in average salinity, especially after 2001. Water temperatures were initially relatively low but have fluctuated with little trend since the late 1990s. Water clarity declined from the time-series high in the mid-1990s and remained relatively low throughout the 2000s, and has increased since 2010 to conditions similar to those in the mid- to late-1990s (Figure 8).
The RDA diagram for the Pontchartrain basin illustrated the dominance of water clarity and also emphasized that water temperature and salinity were important in structuring the trawl catch communities (Supplementary Figure 8). Unlike Barataria Bay, however, there was no consistent environmental changes over time or in system-wide organization; for example, 1999 and 2018 had similar environmental and species assemblage conditions (Supplementary Figure 8). In the Terrebonne basin, water clarity and salinity were the dominant co-variates determining species distribution, with water temperature being a far weaker covariate than either water clarity or salinity (Supplementary Figure 9). In Terrebonne Bay, the years since the 2010 DWH oil spill generally exhibited higher water clarity and salinity than in previous years (Supplementary Figure 8).
Discussion
While the DWH oil spill did not originate in the coastal/nearshore region, within six weeks large quantities of weathered oil had reached the coasts of affected states, impacting beaches, entering estuaries and resulting in a patchwork of oiling ranging from no-oil observed to heavy (Deepwater Horizon Natural Resource Damage Assessment Trustees, 2016; Turner et al., 2019a; Figures 1, 2, 11). The oil transport scenario and the ensuing use of protective fishery closures and oil spill response measures, enacted ostensibly to slow the progression and lessen the severity of impacts from DWH, resulted in a complex set of interacting effects on coastal and nearshore biota (Figure 2). Disentangling population and community-level effects of oil contamination and associated response measures from other factors such as fishing, climate change, habitat loss and increasingly managed hydrology represents a difficult challenge in synthesizing oil spill impacts. This challenge was exacerbated by the dearth of comprehensive, synoptic baselines of oil contamination and related species life history data pre-spill, as has been noted by many previous authors.
A number of previous studies have conducted short-term, BACI-type (Before-After-Control-Impact) analyses of DWH effects (e.g., Moody et al., 2013; van der Ham and de Mutsert, 2014; Schaefer et al., 2016) using pre- and relatively short post-DWH time series from abundance surveys, but these studies were not designed to specifically index oil-spill effects. Such analyses may not fully capture the complexity of coincident drivers affecting the organization of species or the inherent variability of animal abundance and recruitment. Short-term analyses may be particularly problematic for longer-lived species (e.g., Red Drum, Bottlenose Dolphin, etc.), and longer-term and extended post-event monitoring can provide broader perspectives of how such events may have interacted with or interrupted normal variability and trends, particularly where significant human control of ecosystems occurs (as in the northern GoM). For example, ongoing analyses of the effects of the Exxon Valdez oil spill, even three decades hence continue to allow re-evaluation of the impacts and threats posed by large scale spills and the proximal drivers of population and ecosystem change (Peterson et al., 2003; Bodkin et al., 2014; Esler et al., 2018). In this regard, our syntheses of DWH-associated effects should be viewed not as definitive, but as contingent upon the collection of yet longer time-series data over varying spatial scales to provide more meaningful temporal context (e.g., short-term fluctuations vs. longer term trends) for the observations we are summarizing.
The key species we analyzed exhibited a wide range in vulnerability to the effects of DWH and potential population resiliency. As we note above, differences among species are determined by a number of factors (Supplementary Table 2). Vulnerability of populations to spill effects is in large part due to the spatiotemporal overlap of particularly sensitive life stages (eggs, larvae and postlarvae) with toxic concentrations of oil in the water column and in sediments (Deepwater Horizon Natural Resource Damage Assessment Trustees, 2016). Most of the species we characterized either had sensitive life stages spawned and developed prior to oil overlapping their distributions (e.g., Gulf Menhaden, Red Drum) or extended spawning intervals resulting in significant fractions of annual larval production outside the temporal overlap with the spill (e.g., shrimps, Spotted Seatrout, Eastern Oyster). For several species there are complex interactions between the use of the coastal ocean for spawning and recruitment back into estuaries (e.g., shrimps, Gulf Menhaden, Blue Crab and Red Drum) which may translate to increased potential for limited exposure of vulnerable life stages and enhanced connectivity among adjacent estuaries thereby buffering spill impacts on individual populations. While there was high vulnerability of plankton (phyto- and zooplankton) to oil in the water column, the affected population fractions were small and recruitment from outside the spill area combined with rapid population turnover limited extensive negative impacts. Where overlap of toxic concentrations of oil with significant portions of populations did occur, the impacts were more profound (Bottlenose Dolphin BSE). The combination of high vulnerability to DWH and low resilience potential of affected Dolphin populations resulted in BSE Bottlenose Dolphins being the most negatively affected by DWH of the key species we evaluated in the coastal/nearshore area. An important contribution of the present synthesis is the further recognition that taxonomically closely related species (e.g., Brown and White Shrimp; and Spotted Seatrout and Red Drum) and even demographically independent populations of the same species (e.g., Barataria Bay BSE and coastal stocks) can have differing vulnerabilities resulting from life-history traits, habitat preferences and behavioral adaptations (e.g., high site fidelity). Thus, extrapolating impacts from a small subset of closely related species should be done cautiously.
Because of the large number of species occurring in northern GoM coastal areas and the diversity of their life histories, it is important not only to consider differential impacts of DWH on a small number of key species but in community-level analyses as well. Trawling surveys in coastal estuaries of the northern GoM typically catch several hundred species or species groups (e.g., Louisiana Department of Fisheries and Wildlife trawl surveys documented 308 species or groups in the three basins we evaluated; Supplementary Table 1). The high biodiversity in coastal regions is reflective of the transition zones that estuaries play between freshwater and oceanic conditions. As well, these regions serve as productive nursery areas for species that, as adults, may spend the majority of their lives in the ocean, and especially those supporting the most productive fisheries in the GoM (Cowan et al., 2008). Changing environmental conditions due either to disruptive events or long-term environmental trends will necessarily result in changing distributions of animals relative to the habitats in which they have evolved.
Long-term trawl survey data documented both declining trends in multispecies abundance (Barataria and Terrebonne Bays, Pontchartrain basin; Figure 3) and relative stability (Alabama, Florida, Figure 4) post-DWH. In Barataria Bay (the estuary with the highest concentration of heavily oiled marshlands, Figure 1), SIMPROF analyses and associated nMDS plots documented significant changes in species composition coherent in time with DWH and driven by a number of species that we did not consider to be key species because of their direct economic importance (Figures 5, 6). The changes in the relative abundance of species we document are similar to those observed by Chesney et al. (2000) after the initiation of operations of the Caernarvon freshwater diversion in Black Bay in addition to continuing freshwater inputs from the Bohemia Spillway south of Caernarvon, and emptying into Breton Sound. Differences in the composition of the finfish and epi-benthic invertebrate communities, as revealed in trawl survey catches (Figure 5), pre- and post-DWH were so pronounced that the changes fit criteria generally associated with ecological regime shifts. In 2008, deYoung et al. (2008) noted that “Regime shifts are abrupt changes between contrasting, persistent states of any complex system.” Clustering of species compositions sampled post-DWH abruptly shift beginning in 2010 (Figure 6) and data collected through 2018 do not show the system reverting to pre-DWH abundance or compositional characteristics (Figure 6). The question remains as to what is driving the apparent regime shift in Barataria Bay – the effects of DWH and its aftermath or longer-term changes in extant environmental conditions (or both)? Additionally, there are also apparent long-term shifts in productivity and organization at the GoM large marine ecosystem (LME) scale likely caused by ocean basin environmental forcing and changes in fisheries management policies (Karnauskas et al., 2015; Kilborn et al., 2018).
With the opening of the Davis Pond freshwater diversion channel in 2002 (Cowan et al., 2008; Das et al., 2012; de Mutsert and Cowan, 2012; Rose et al., 2014; Coastal Protection and Restoration Authority of Louisiana, 2017; de Mutsert et al., 2017; White et al., 2018; Turner et al., 2019b), the hydrology of Barataria Bay has changed, as reflected in the yearly average salinity and water clarity sampled coincident with trawl survey stations (Figure 8). The shunting of water and sediment through the Davis Pond diversion during 2010 was exceptional in volume and duration (O’Connor et al., 2016) leading to seaward shift of the salinity gradient and large-scale mortalities of species such as Eastern Oyster and Marsh Periwinkle, along with others incapable of movement out of low salinity areas (Powers et al., 2017a; Zengel et al., 2017). Our RDA investigation for Barataria Bay documents the importance of salinity, water clarity and temperature in structuring the estuarine fish and invertebrate communities as sampled by trawls (Figure 6). Modeling of the effects of the Davis Pond freshwater diversion on structure and beta-diversity of fish and invertebrate communities predicted some of the changes we document here, including increases in the abundance of freshwater species as well as declines in Spotted Seatrout and other species dependent upon higher salinities (de Mutsert and Cowan, 2012; Rose et al., 2014). The lack of recovery of the species assemblage to pre-DWH conditions, perhaps, indicates that the freshwater surge in 2010 combined with the direct effects of oiling was sufficient to flip the system into a new productivity regime from which it has yet to return. As additional sediment diversions are planned both for Barataria Bay and the adjoining Breton Sound/Black Bay complex (Coastal Protection and Restoration Authority of Louisiana, 2017; White et al., 2018) the regime change initiated in 2010 may be a permanent feature of the system and in fact additional seaward shifts in salinity-dependent species and changes in productivity may ensue. As Cowan et al. (2008) mused “Will the Louisiana coastal ecosystem and its related fish production respond to restoration efforts as if the region has experienced a regime shift or a shift in the ecological baseline?” Our analyses indicate that one such shift was initiated coincident with the DWH event in Barataria Bay. species and changes in productivity may Our RDA analysis of community composition in the Pontchartrain basin did not show a similar pattern of directional change in the time series of environmental conditions (Supplementary Figure 8), as with Barataria Bay (Figure 7). In the Pontchartrain basin, water clarity and temperature were more closely associated with species composition than salinity. However, the Pontchartrain basin (Figure 1) is comprised of a number of relatively distinct sub-basins (e.g., Breton Sound/Black Bay along the eastern side of the Mississippi River, Lake Borgne and Lake Pontchartrain) that have unique hydrologies and are subject to differing impacts of flooding and water management scenarios. For example, like Barataria Bay, the Caernarvon freshwater diversion channel at the head of Breton Sound was opened during 2010 in a similar effort to forestall oil dispersion into salt marshes (O’Connor et al., 2016). The Caernarvon diversion and additional planned diversions for the eastern side of the Mississippi River are key elements of the restoration plan aimed at diverting sediments to stem land loss in Louisiana coastal marshes (Coastal Protection and Restoration Authority of Louisiana, 2017; Turner et al., 2019b). Rose et al. (2014) modeled movement potential of salinity-sensitive species, projecting down estuary movements of 15–35 km in response to increased water volumes from diversions into Breton Sound, and they noted the importance of salinity in structuring that community. Additional research subdividing the Pontchartrain basin into several component systems is needed to identify systematic changes in species composition that may be associated in time with DWH and other alterations to the hydrologic regime (as for Barataria Bay).
The RDA for Terrebonne Bay (Supplementary Figure 9) differed from both the Barataria and Pontchartrain basins in that water clarity and salinity were the major environmental parameters accounting for the structure of fish and invertebrate communities. Although not as clear as for Barataria Bay, there was a pattern of increasing water clarity over time, with clearer, lower-salinity waters occurring post-DWH (Supplementary Figure 9). No freshwater/sediment diversions are currently operating or planned in the Terrebonne basin (Coastal Protection and Restoration Authority of Louisiana, 2017), and thus large-scale changes in the salinity gradient will likely not occur.
In our analyses of species abundance and associated environmental conditions, we computed annual averages from multiple seasonal surveys to depict long-term change. Within these regions there is strong seasonal forcing (e.g., spring floods, hurricanes, etc.). Thus, more detailed seasonally based analyses are feasible to evaluate a range of questions, especially with respect to timing of life history events in relation to environmental fluxes (e.g., O’Connell et al., 2019).
The analyses described above illustrate the difficulty of attributing species and ecosystem change to the direct effects of the DWH oil spill and the ensuing response measures, including fishery closures. Some impacts, however, were severe and obvious. For sedentary species including Eastern Oyster the combination of the toxicity of oil and the effects of freshening the marshes not only resulted in mass oyster mortalities (Powers et al., 2017a), but oiling of the marshes was also was associated with collapse of the marsh edges and the loss of oyster bars, which in turn resulted in increased soil erosion and other negative consequences for marsh ecosystems (Grabowski et al., 2012; Powers et al., 2017c; Hughes et al., 2018). Likewise, Bottlenose Dolphins’ exposure to toxic oil concentrations presented a variety of negative health effects including reduced reproduction and associated morbidities and mortalities (Schwacke et al., 2014, 2017). The association between changes in the salinity gradients in Barataria Bay and Breton Sound, the distributions of dolphin populations therein, and their exposures to oil remain conjectural (White et al., 2018), but nonetheless they are important to resolve.
The use of freshwater diversions and the construction of sand berms to forestall oil progression into sensitive estuarine habitats may have at best been ineffectual (Figure 11; Martínez et al., 2012; Suir et al., 2016) but more likely, as in the case of freshwater diversions, they exacerbated the negative impacts of oil exposure. For example, despite the high freshwater flow rates through the Davis Pond and Caernarvon diversions during the DWH spill, large quantities of oil readily progressed into the marshes of Barataria Bay and Breton Sound, resulting in oil contamination several orders of magnitude in excess of background concentrations (Turner et al., 2019a). The transition of oil through the channels near Grand Isle, LA and into lower Barataria Bay is clearly seen in Figure 11. Other management measures associated with the DWH spill, including inshore and offshore fishery closures (Cockrell et al., 2019), likely had more positive impacts on a variety of estuarine species, especially including short-life cycle species such as shrimps, Gulf Menhaden, and Blue Crab. However, fishery closure effects were ephemeral and the balance of impacts from the combination of response measures and fishery closures remains uncertain and highly species-specific.
In their review of the environmental consequences of the Exxon Valdez oil spill, Peterson et al. (2003) noted the importance of an evolving understanding of “…delayed, chronic and indirect effects of petroleum contamination.” In this regard, early reviews of the effects of the DWH oil spill on nearshore communities of organisms may have been too sanguine (e.g., Fodrie and Heck, 2011; Fodrie et al., 2014; Schaefer et al., 2016). For example, while oil contamination in marsh sediments has declined from the initially 100–1,000 times background, levels have remained an order of magnitude above pre-spill levels 8 years post-DWH, and will likely remain so for decades (Turner et al., 2019a). How this reservoir of highly weathered and biodegraded oil contamination will interact with estuarine biota depends on how rapidly it becomes biologically unavailable. This was particularly the case for benthic infauna. Likewise, the extended mean generation times of a number of coastal species impacted by the spill, including Bottlenose Dolphin, Red Drum, Black Drum, and some bird species, means that the consequences of spill impacts on population demography are yet to be fully realized.
The Peterson et al. (2003) review also emphasized two important aspects of the oil spill scenario that, until then, had been underappreciated in oil-spill research. These include understanding that oil-spill response measures (e.g., freshwater diversions) can be as deleterious as oil contamination itself, and evolving perspectives regarding how spills can affect the structure and functioning of interacting species, communities and ecosystems. As noted above, response measures such as freshwater flooding and creation of sand berms were probably ineffective, and in the former case enhanced mortality of sedentary species. In the case of Exxon Valdez, response measures such as steam-washing beach cobbles may have resulted in driving oil deeper into sediments and volatizing toxic compounds. In both the DWH and Exxon Valdez spills, the problematic response measures were not standard practice2, further suggesting that their future use be carefully considered. Furthermore, the potentially negative implications of these and related response measures should be clearly acknowledged before they are suggested in the politically charged heat of battle.
Peterson et al. (2003) also noted that perspectives on oil spill impacts had evolved from primarily being a focus on contamination of a few key species based upon extrapolation from laboratory studies, to a broader consideration of impacts on habitats and species associations within affected communities. Our work illustrates the complementary perspectives that can be gained from both looking at population consequences for key species and the broader multispecies community effects that may occur (e.g., Figures 5–7). Our work indicates that that population trajectories varied substantially for a number of the key species with obvious DWH-related impacts to some. The community-wide perspectives, as illustrated for Barataria Bay, show a complex series of post-DWH effects including declining overall abundance (Figure 3) and a regime shift in species dominance that would not have otherwise been detected through more traditional approaches to oil-spill impacts monitoring. Attribution of these effects solely to DWH oil contamination and associated response measures remains elusive, however, because of the changing environmental conditions and evolving natural resource management schemes at play in the northern GoM coastal environment. Nevertheless, with respect to large-scale oil spills, an evolving ecosystem-scale approach to understanding complex effects and interactions provides important new perspectives and approaches for assessing the cumulative impacts of human activities (e.g., Baker et al., 2017).
Priorities for Management and Research
With respect to cumulative impacts from large-scale stressors, one might consider how to increase the resiliency of ecosystems and their component species to better withstand future shocks to the system from oil spills, hurricanes, harmful agal blooms, and other serious threats. Because there are other management levers that can be manipulated, one might consider, for example, strategies to improve the volume and quality of reproduction of species, emphasizing the build-up of multiple age classes of animals contributing to the age structure. There is precedent for such strategies in the northern GoM. Due to reductions in fishery catches by about half, the cumulative spawning output and population density of Gulf Menhaden were relatively high when DWH occurred and have increased still to near time-series record values (Figure 12). One might also consider similar management tactics for other species of economic or ecosystem importance. Management tactics for Spotted Seatrout vary widely among GoM states. For example, in the Panhandle of Florida, 2020 sport fishing regulations for Spotted Seatrout include a bag limit of three fish with a slot size-limit from 15 to 19 inches, and one fish allowed to be >19 inches per vessel (Florida Wildlife Commission, 2020). By contrast, regulations in eastern Louisiana allow a bag limit of 25 fish ≥12 inches with no more than 2 fish >25 inches (Louisiana Department of Wildlife and Fisheries, 2020). The difference in management tactics could easily be translated into the relative spawning escapement and thus resilience potential for various management strategies, given information on fishing effort, bycatch mortality and a few other parameters (e.g., eggs/recruit analysis).
The dendrogram on the Barataria Bay trawl catch heatmap (Figure 5) divides the species into two main sub-groups. The group from Southern Flounder to Star Drum is comprised of species preferring more saline, less eutrophic waters (and is becoming less abundant over the decades). Conversely, the species group from Sheepshead to Sharptail Goby is generally found in less saline, more eutrophic waters (and is becoming more abundant). The overall patterns also support the thesis that nutrient loading/retention in Barataria Bay has increased on a decadal scale, and that eutrophication is contributing to the regime shift, as a number of eutrophication-sensitive species have changed in abundance. Management strategies that more effectively control nutrient enrichment may thus be important in managing for estuarine community structure.
Other strategies to increase the resiliency of the northern GoM coastal/nearshore system to future perturbations through reductions in co-stressors might include greater use of marine and coastal protected areas and/or restoration of critical nursery habitats, as is the objective of billions of dollars in habitat restoration funding resulting from the fines imposed on the parties responsible for DWH (Coastal Protection and Restoration Authority of Louisiana, 2017; Wallace et al., 2019; Boesch, 2020).
Our research has focused on a re-examination of multiple long-term time series of data that describe the state of the northern GoM coastal/estuarine systems for decades prior to, and after the DWH event. These data present a nuanced and variable picture of DWH impacts on species, populations, communities and ecosystems within the region. Because none of the series pre-dating the spill were collected with the specific intent of creating oil spill baseline information, the individual surveys have limited power to detect change, particularly over short time intervals. The increased length of the time series of many of these surveys post-DWH and general coherence across surveys now reveals important patterns that have emerged from the noise of the data. As a result, we propose a number of research recommendations to collect information necessary to be more predictive of large-scale oil spill impacts. These include:
Evaluating Connectivity Among Populations at Increasing Spatial Scales in Coastal/Estuarine Regions of the GoM
While the broad patterns of population demography for coastal and nearshore populations and their interactions are generally understood, much remains unknown regarding the degree of meta-population connectivity among adjacent groups. Because the degree of oiling of coastal marshes and beaches was so sporadic (e.g., Figures 1, 11), it is likely that rather than presenting uniform population-level effects, DWH resulted in a mosaic of impacts ranging from low to substantial, even within a single estuary (Figure 11). In order to evaluate the degree to which population connectivity ameliorates population or community effects of oil spills (and other such events) and promotes resiliency, more focused research on these issues is of great practical importance. Using modern genomic tools, advanced tagging and movement data and hydrodynamic modeling would allow an evaluation of the applicable scales of population connectivity and thus the degree to which contaminant effects may transfer across landscapes ranging from local to large marine ecosystems.
New Tools to Evaluate Cumulative Effects of Environmental Co-stressors
As Peterson et al. (2003) and others have emphasized, it is of increasing importance to evaluate the effects of large oil spills—such as Exxon Valdez and DWH – in the context of the full range of stressors affecting marine and coastal resources. In particular, GoM living marine resources are subject to long-term, pervasive stress from drivers such as intensive fishing and chronic inputs of pollutants from point and non-point sources including oil-related compounds, pathogens, pesticides, heavy metals and a series of industrially generated toxins such as PCBs and PFAS compounds. Additionally, aperiodic events such as hurricanes, harmful agal blooms, disease outbreaks, and chemical releases as well as increasingly managed hydrologic regimes result in a complex set of interacting factors affecting the demographics and health of species and communities. Thus, it is difficult to isolate the effects of a particular spill on resources, and in particular, to identify tipping points when the cumulative impacts of chronic and acute events undermine adaptive capacities. Rather than simply chronicling the list of such stressors, new experimental, monitoring and modeling paradigms (e.g., Ainsworth et al., 2015, 2018; Chagaris et al., 2020) are necessary to understand the totality of cumulative effects across space and time scales relevant to GoM living resources and their sustainable management.
Longer-Term Monitoring of DWH-Affected Populations, Species and Communities
We have noted that while a decadal assessment of DWH effects provides important perspectives on the direct and indirect impacts of the spill, continued monitoring of particularly vulnerable species (especially the long-lived ones) is required in order to fully evaluate the consequences to species and ecosystems. Such was the case for the Exxon Valdez, and we expect that with ongoing, targeted monitoring and evaluation, yet new insights into the delayed, chronic and cascading impacts of DWH will be revealed over time. The GoMRI-funded research program (that existed from 2010–2020) provided a large fraction of research investments directed at understanding ecosystem impacts of the spill during the past decade. While ongoing ecosystem restoration activities will have some monitoring component, it is imperative that important surveillance elements of the long-term research established in the aftermath of DWH be sustained in order to evaluate and interpret not only the ongoing impacts of the spill, but also how the cumulative impacts of multiple restoration projects affect the related resources. Furthermore, collection of additional chemical pollutant baselines (in biota, water and sediments) will facilitate more rapid and precise evaluation of effects of future spills and other catastrophic events.
Monitoring and Modeling of the Effects of Water Diversions and Other Habitat Restoration Activities
Billions of dollars in settlement moneys from the DWH accident are now being invested in large- and small-scale projects to change primarily coastal and nearshore habitats. Some of these projects address the specific injuries to ecosystems accruing from DWH, while others (e.g., Coastal Protection and Restoration Authority of Louisiana, 2017) address long-term environmental degradation from the historical development of the oil and gas industry, climate change (specifically sea level rise), wetlands loss and other impacts. As we have noted, apart from individual species impacts of DWH, which were variable, there was an apparent regime shift in community composition in one of the major estuaries most impacted by the DWH spill. The degree to which ongoing changes to the hydrology regime and salinity manipulation are contributing to this shift are not fully resolved but are likely an important factor. It is imperative that longer-term and more focused research on the ecological impacts of these investments be made in order to understand, predict and monitor how these ecosystems will actually change and the cumulative benefits and impacts that may accrue from such an enormous investment.
Summary
We provide an evaluation of population and community change in coastal and nearshore environments of the northern GoM following the DWH oil spill of 2010. The coastal areas of the northern GoM had been monitored for decades prior to DWH through a series of state- and federally sponsored research programs that primarily support fisheries management. These surveys were augmented by a wide variety of research programs initiated during and after the DWH oil spill, that provide important species and site-specific information. Our evaluation highlights impact to 13 “key” species of economic and ecological significance to the GoM, as well as community-level analyses of multispecies impacts. We examined each of the “key” species/populations using a series of predefined criteria and classified their relative degree of vulnerability to the specifics of the DWH spill, and the likely resilience potential of populations to future disturbance events.
Of the 13 species/groups evaluated, a few failed to show effects that could be distinguished from the background variability exhibited by these populations. Others, including Bottlenose Dolphin and Eastern Oyster were heavily impacted due to a combination of direct oil exposure and the effects of oil spill response measures. Furthermore, recovery rates vary significantly depending on species life histories. In particular, Bottlenose Dolphin (BSE stocks) may take decades to recover given their long-life cycle and reduced reproductive and health status even a decade after the DWH spill. Some short-lived species, including Brown and White Shrimps, exhibited ephemeral population increases probably due to the effects of large-scale but short-term fishery closures.
The degree of oiling and impacts of spill response measures varied substantially across coastal areas impacted by oil transiting from the offshore spill into coastal waters. In particular, areas of coastal Louisiana and specifically Barataria Bay were subjected to the highest prevalence of heavily oiled shoreline. As compared to coastal areas in Alabama and Florida, the impacts to Louisiana coastal resources were more severe. We document post-DWH declines in multispecies trawl catches in three Louisiana coastal basins as well as what appears to be a regime shift in species composition in trawl survey catches in Barataria Bay. This regime shift appears to be related in time to the direct effects of DWH and freshwater diversions intended to forestall oil entry to the estuary, although some trends in community composition and environmental conditions and regime-state changes were evident prior to the spill (Figures 5–8). Post-DWH changes in the hydrology, eutrophication and salinity of Barataria Bay may be maintaining the shift in community composition.
Water diversions into marshes and the creation of sand berms used in an attempt to ameliorate oil spill impacts to coastal areas, were at best ineffectual and in the case of water diversions exacerbated negative population outcomes for sedentary species. Their use for future oil spill response is highly discouraged.
Because of lessons learned following the DWH oil spill, future efforts outlined in the preceding section should focus on better evaluating population connectivity across the many landscape scales of coastal regions, as well as development of new tools for evaluating co-stressors, longer-term monitoring of affected regions and species, and ongoing evaluation and modeling of the impacts of habitat restoration projects.
Data Availability Statement
The datasets analyzed for this study can be found in the Gulf of Mexico Research Initiative Information and Data Cooperative (GRIIDC) repository at: https://data.gulfresearchinitiative.org/, and in the databases of state agencies contributing data to these studies.
Ethics Statement
The animal studies conducted by the C-IMAGE consortium were reviewed and approved by University of South Florida IACUC Committee.
Author Contributions
All authors collaborated in the design, analysis, evaluation, and publication writing and editing leading to this contribution. This publication is based on two workshops conducted by the authors and others in July (St. Petersburg, FL, United States) and October (Washington, DC, United States) 2019. SM led the workshops, conducted analysis of species and community data and drafted sections of the manuscript. JK and EP participated in the workshops and conducted community-level analyses of trawl survey data. JK also contributed to time series analyses of fishery-independent/dependent monitoring data. AB participated in the workshops and provided expertise in toxicology. DC participated in the workshops and drafted sections related to ecosystem modeling. DD participated in the workshops and interpretation of state-level data. TM and CN provided state level analyses and data. KR and FH developed the information and write-ups for plankton.
Funding
Compilation of this document was supported with grants from the Gulf of Mexico Research Initiative. Funding for the project was primarily provided by the Gulf of Mexico Research Initiative through the Center for Integrated Modeling and Analysis of Gulf Ecosystems (C-IMAGE, Grant Number SA 18-16).
Conflict of Interest
AB was employed by Shell Health – Americas, Shell Oil Company.
The remaining authors declare that the research was conducted in the absence of any commercial or financial relationships that could be construed as a potential conflict of interest.
Acknowledgments
In particular, we thank the Gulf of Mexico Research Initiative (GoMRI) Core Area 3 “motivators” (Rick Shaw, Margaret Leinen, Dick Dodge, Bill Hogarth, Bob Shipp, John Farrington, and Ken Halanych) for their help and encouragement. As well, the staffs of the GoMRI Research Board and AIBS provided travel and logistical support, including Chuck Wilson, Michael Feldman, Callan Yanoff, and Jennifer Pettit. Lisa DiPinto, of NOAA’s Office of Response and Restoration, provided critical input to the process and comments on the document. Access to fishery-independent survey data was generously provided by the Louisiana Department of Wildlife and Fisheries (LDWF), the Alabama Marine Resources Department and the Florida Fish and Wildlife Research Institute. Ryan Takeshita and Lori Schwacke of the National Marine Mammal Foundation and Jenny Litz of the National Marine Fisheries Service contributed to the Bottlenose Dolphin V-R analysis and write-up, and commented on the manuscript. We thank Diane Rome Peebles for permission to use the Gulf Menhaden pictures in Figure 13. Finally, we appreciate the support of the editors and reviewers of this contribution to the Frontiers in Marine Science’s Research Topic on Vulnerability and Resilience of Species to DWH.
Supplementary Material
The Supplementary Material for this article can be found online at: https://www.frontiersin.org/articles/10.3389/fmars.2020.594862/full#supplementary-material
Footnotes
- ^ https://www.fisheries.noaa.gov/national/sustainable-fisheries/commercial-fisherieslandings
- ^ Response measures that are considered standard practice include physical recovery, dispersant applications, in situ burning and monitoring natural recovery on open water, and manual oil collection, flushing, cleaning agents, pressure washing, bioremediation and monitoring natural recovery on shorelines. Each response measure has advantages and disadvantages, and their values depend on the situation. Typically, an analysis is undertaken in order to select the best response strategy that mitigates oil impacts.
References
Able, K. W., Lopez-Duarte, P. C., Fodrie, F. J., Jensen, O. P., Martin, C. W., Roberts, B. J., et al. (2015). Fish assemblages in Louisiana salt marshes: effects of the Macondo oil spill. Estuar. Coast. 38, 1385–1398. doi: 10.1007/s12237-014-9890-6
Aichinger-Dias, L. A., Litz, J., Garrison, L., Martinez, A., Barry, K., and Speakman, T. (2017). Exposure of cetaceans to petroleum products following the Deepwater Horizon oil spill in the Gulf of Mexico. Endang. Spec. Res. 33, 119–125. doi: 10.3354/esr00770
Ainsworth, C. H., Paris, C. B., Perlin, N., Dornberger, L. N., Patterson, W. F. III, Chancellor, E., et al. (2018). Impacts of the Deepwater Horizon oil spill evaluated using an end-to-end ecosystem model. PLoS One 13:e0190840. doi: 10.1371/journal.pone.0190840
Ainsworth, C. H., Schirripa, M. J., and Morzaria-Luna, H. (eds) (2015). An Atlantis Ecosystem Model for the Gulf of Mexico Supporting Integrated Ecosystem Assessment. US Dept. Comm. NOAA Tech Memo NMFSSEFSC-676. Washington, DC: NOAA, 149.
Alldredge, A. L. (1976). Discarded appendicularian houses as sources of food, surface habitats, and particulate organic matter in plankton environments. Limno. Oceanogr. 21, 14–23. doi: 10.4319/lo.1976.21.1.0014
Alloy, M., Garner, T. R., Bridges, K., Mansfield, C., Carney, M., Forth, H., et al. (2017). Co-exposure to sunlight enhances the toxicity of naturally weathered Deepwater Horizon oil to early lifestage red drum (Sciaenops ocellatus) and speckled seatrout (Cynoscion nebulosus). Environ. Tox. Chem. 36, 780–785. doi: 10.1002/etc.3640
Almeda, R., Wambaugh, Z., Wang, Z., Hyatt, C., Liu, Z., and Buskey, E. J. (2013). Interactions between zooplankton and crude oil: toxic effects and bioaccumulation of polycyclic aromatic hydrocarbons. PLoS One 8:e67212. doi: 10.1371/journal.pone.0067212
Baker, M. C., Steinhoff, M. A., and Fricano, G. F. (2017). Integrated effects of the Deepwater Horizon oil spill on nearshore ecosystems. Mar. Ecol. Prog. Ser. 576, 219–234. doi: 10.3354/meps11920
Barata, C., Calbet, A., Saiz, E., Ortiz, L., and Bayona, J. M. (2005). Predicting single and mixture toxicity of petrogenic polycyclic aromatic hydrocarbons to the copepod Oithona davisae. Environ. Toxicol. Chem. 24, 2992–2999. doi: 10.1897/05-189r.1
Bejarano, A. C., Chandler, G. T., He, L., and Coull, B. C. (2006). Individual to population level effects of South Louisiana crude oil water accommodated fraction (WAF) on a marine meiobenthic copepod. J. Exp. Mar. Biol. Ecol. 332, 49–59. doi: 10.1016/j.jembe.2005.11.006
Bejarano, A. C., and Michel, J. (2016). Oil spills and their impacts on sand beach invertebrate communities: a literature review. Environ. Pollut. 218, 709–722. doi: 10.1016/j.envpol.2016.07.065
Bellas, J., and Thor, P. (2007). Effects of selected PAHs on reproduction and survival of the calanoid copepod Acartia tonsa. Ecotoxicology 16, 465–674. doi: 10.1007/s10646-007-0152-2
Beyer, J., Trannum, H. C., Bakke, T., Hodson, P. V., and Collier, T. K. (2016). Environmental effects of the Deepwater Horizon oil spill: a review. Mar. Poll. Bull. 110, 28–51. doi: 10.1016/j.marpolbul.2016.027
Bik, H. M., Halanych, K. M., Sharma, J., and Thomas, W. K. (2012). Dramatic shifts in benthic microbial eukaryote communities following the Deepwater Horizon oil spill. PLoS One 7:e38550. doi: 10.1371/journal.pone.0038550
Blondeau-Patissier, D., Gower, J. F. R., Dekker, A. G., Phinn, S. R., and Brando, V. E. (2014). A review of ocean color remote sensing methods and statistical techniques for the detection, mapping and analysis of phytoplankton blooms in coastal and open oceans. Prog. Oceanogr. 123, 123–144. doi: 10.1016/j.pocean.2013.12.008
Bociu, I., Shin, B., Wells, W. B., Kostka, J. E., Konstantinidis, K. T., and Huettel, M. (2019). Decomposition of sediment-oil agglomerates in a Gulf of Mexico sandy beach. Sci. Rep. 9:10071. doi: 10.1038/s41598-019-46301-w
Bodkin, J. L., Esler, D., Rice, S. D., Matkin, C. O., and Ballachey, B. E. (2014). “The effects of spilled oil on coastal ecosystems: lessons from the Exxon Valdez spill,” in Coastal Conservation, eds B. Maslo and J. L. Lockwood (New York, NY: Cambridge University Press), 311–346. doi: 10.1017/cbo9781139137089.013
Boesch, D. F. (2020). Envisioning the Future of the Louisiana Gulf Coast. Washington, DC: Walton Family Foundation, 86.
Bonisoli-Alquati, A., Stouffer, P. C., Turner, R. E., Woltmann, S., and Taylor, S. S. (2016). Incorporation of Deepwater Horizon oil in a terrestrial bird. Environ. Res. Lett. 11:114023. doi: 10.1088/1748-9326/11/11/114023
Borcard, D., Gillet, F., and Legendre, P. (2018). Numerical Ecology with R, 2nd Edn, Berlin: Springer, 435.
S. A. Bortone (ed.) (2003). Biology of the Spotted Seatrout. CRC Marine Biology Series. Boca Raton: CRC Press, 328.
Brown-Peterson, N. J., Peterson, M. S., Nieland, D. L., Murphy, M. D., Taylor, R. G., and Warren, J. R. (2002). Reproductive biology of female spotted seatrout, Cynoscion nebulosus, in the Gulf of Mexico: differences among estuaries? Environ. Biol. Fish. 63, 405–415. doi: 10.1023/A:1014925421111
Brunner, C. A., Yeager, K. M., Hatch, R., Simpson, S., Keim, J., Briggs, K. B., et al. (2013). Effects of oil from the 2010 Macondo well blowout on marsh foraminifera of Mississippi and Louisiana, USA. Environ. Sci. Tech. 47, 9115–9123. doi: 10.1021/es401943y
Caillouet, C. W. Jr., Hart, R. A., and Nance, J. M. (2008). Growth overfishing in the brown shrimp fishery of Texas, Louisiana, and adjoining Gulf of Mexico EEZ. Fish. Res. 92, 289–302. doi: 10.1016/j.fishres.2008.01.009
Calbet, A., Saiz, E., and Barata, C. (2007). Letal and sublethal effects of naphthalene and 1,2 dimethylnaphthalene on the marine copepod Paracartia grani. Mar. Biol. 151, 195–204. doi: 10.1007/s00227-006-0468-0
Carassou, L., Hernandez, F. J., and Graham, W. H. (2014). Change and recovery of coastal mesozooplankton community structure during the Deepwater Horizon oil spill. Environ. Res. Lett. 9:124003. doi: 10.1088/1748-9326/9/12/124003
Carassou, L., Hernandez, F. J., Powers, S. P., and Graham, W. M. (2012). Cross-shore, seasonal, and depth-related structure of ichthyoplankton assemblages in coastal Alabama. Trans. Am. Fish. Soc. 141, 1137–1150. doi: 10.1080/00028487.2012.675920
Cardona, L., Álvarez de Quevedo, I., Borrell, A., and Aguilar, A. (2012). Massive consumption of gelatinous plankton by Mediterranean apex predators. PLoS One 7:e31329. doi: 10.1371/journal.pone.0031329
Carroll, M., Gentner, B., Larkin, S., Quigley, K., Perlot, N., Dehner, L., et al. (2016). An Analysis of the Impacts of the Deepwater Horizon Oil Spill on the Gulf of Mexico Seafood Industry. New Orleans, LA: U.S. Dept. of the Interior, Bureau of Ocean Energy Management.
Chagaris, D. D., Patterson, W. F. III, and Allen, M. S. (2020). Relative effects of multiple stressors on reef food webs in the northern Gulf of Mexico revealed via ecosystem modeling. Front. Mar. Sci. 7:513. doi: 10.3389/fmars.2020.00513
Chakraborty, S., and Lohrenz, S. E. (2015). Phytoplankton community structure in the river-influenced continental margin of the northern Gulf of Mexico. Mar. Ecol. Prog. Ser. 521, 31–47. doi: 10.3354/meps11107
Chancellor, E. (2015). Vulnerability of Larval Fish Populations to Oil Well Blowouts in the Northern Gulf of Mexico. M. Sc. Thesis, University of South Florida, Tampa, FL, 97.
Chanton, J. P., Cherrier, J., Wilson, R. M., Sarkodee-Adoo, J., Bosman, S., Mickle, A., et al. (2012). Radiocarbon evidence that carbon from the Deepwater Horizon spill entered the planktonic food web of the Gulf of Mexico. Environ. Res. Lett. 7:045303. doi: 10.1088/1748-9326/7/4/045303
Chesney, E. J., Baltz, D. M., and Thomas, R. G. (2000). Louisiana estuarine and coastal fisheries and habitats: perspectives from a fish’s eye view. Ecol. Appl. 10, 350–366. doi: 10.1890/1051-0761(2000)010[0350:leacfa]2.0.co;2
Clarke, K. R., and Gorley, R. N. (2015). PRIMER Version 7: User Manual/Tutorial. Plymouth: PRIMER-E.
Clarke, K. R., Somerfield, P. J., and Gorley, R. N. (2008). Testing of null hypotheses in exploratory community analyses: similarity profiles and biota-environment linkage. J. Exp. Mar. Biol. Ecol. 366, 56–69. doi: 10.1016/j.jembe.2008.07.009
Coastal Protection and Restoration Authority of Louisiana (2017). Louisiana’s Comprehensive Master Plan for a Sustainable Coast, Effective June 2, 2017. Baton Rouge, LA: Coastal Protection and Restoration Authority of Louisiana.
Cockrell, M. L., O’Farrell, S., Sanchirico, J., Murawski, S. A., Perruso, L., and Strelcheck, A. (2019). Resilience of a commercial fishing fleet following emergency closures in the Gulf of Mexico. Fish. Res. 218, 69–82. doi: 10.1016/j.fishres.2019.04.017
Colegrove, K. M., Venn-Watson, S., Litz, J., Kinsel, M. J., Terio, K. A., Fougeres, E., et al. (2016). Fetal distress and in utero pneumonia in perinatal dolphins during the Northern Gulf of Mexico unusual mortality event. Dis. Aquat. Organ. 119, 1–16. doi: 10.3354/dao02969
Cowan, J. H. Jr., Grimes, C. B., and Shaw, R. F. (2008). Life history, history hysteresis and habitat changes in Louisiana’s coastal ecosystem. Bull Mar. Sci. 83, 197–215.
Cowan, J. H. Jr., and Shaw, R. F. (1988). The distribution, abundance, and transport of larval sciaenids collected during winter and early spring from the continental shelf waters off west Louisiana. Fish. Bull., U.S. 86, 129–142.
Cowan, J. H., and Houde, E. D. (1993). Relative predation potentials of scyphomedusae, ctenophores and planktivorous fish on ichthyoplankton in Chesapeake Bay. Mar. Ecol. Prog. Ser. 95, 55–65. doi: 10.3354/meps095055
Das, D. J., Inoue, M., Hoda, A., Huang, H., and Park, D. (2012). Impacts of Mississippi River diversions on salinity gradients in a deltaic Louisiana estuary: ecological and management implications. Estuar. Coast. Shelf Sci. 111, 17–26. doi: 10.1016/j.ecss.2012.06.005
Davidson, A. D., Boyer, A. G., Kim, H., Pompa-Mansilla, S., Hamilton, M. J., Costa, D. P., et al. (2012). Drivers and hotspots of extinction risk in marine mammals. Proc. Nat. Acad. Sci. U.S.A. 109, 3395–3400. doi: 10.1073/pnas.1121469109
De Guise, S., Levin, M., Gebhard, E., Jasperse, L., Saliki, J. T., Burdett Hart, L., et al. (2017). Changes in immune functions in bottlenose dolphins in the northern Gulf of Mexico associated with the Deepwater Horizon oil spill. Endang. Spec. Res. 33, 291–303. doi: 10.3354/esr00814
de Mutsert, K., and Cowan, J. H. Jr. (2012). A Before-After-Control-Impact analysis of the effects of a Mississippi River freshwater diversion on nekton in Louisiana, USA. Estuar. Coasts 35, 1237–1248. doi: 10.1007/s12237-012-9522-y
de Mutsert, K., Lewis, K., Milroy, S., Buszowski, J., and Steenbeek, J. (2017). Using ecosystem modeling to evaluate trade-offs in coastal management: effects of large-scale river diversions on fish and fisheries. Ecol. Model. 360, 14–26. doi: 10.1016/j.ecolmodel.2017.06.029
Deegan, L. A. (1990). Effects of estuarine environmental conditions on population dynamics of young-of-the-year gulf menhaden. Mar. Ecol. Prog. Ser. 68, 195–205. doi: 10.3354/meps068195
Deepwater Horizon Natural Resource Damage Assessment Trustees (2016). Deepwater Horizon Oil Spill: Final Programmatic Damage Assessment and Restoration Plan and Final Programmatic Environmental Impact Statement. Available online at: http://www.gulfspillrestoration.noaa.gov/restoration-planning/gulf-plan (accessed July 17, 2019).
Deis, D. R., Fleeger, J. W., Bourgoin, S. M., Mendelssohn, I. A., Lin, Q., and Hou, A. (2017). Shoreline oiling effects and recovery of Saltmarsh macroinvertebrates after the Deepwater Horizon oil spill. PeerJ 5:e3680. doi: 10.7717/peerj.3680
DeVries, D., Bedee, C. D., Palmer, C., and Bortone, S. (2003). “The demographics and reproductive biology of Spotted Seatrout, Cynoscion nebulosus,” in Biology of the Spotted Seatrout, ed. S. Bortone (Boca Raton, EL: CRC Press), 79–98. doi: 10.1201/9781420040791.ch7
deYoung, B., Barange, M., Beaugrand, G., Harris, R., Perry, R. I., Scheffer, M., et al. (2008). Regime shifts in marine ecosystems: detection, prediction and management. Trends Ecol. Evol. 23, 402–409. doi: 10.1016/j.tree.2008.03.008
Ditty, J. G. (1986). Ichthyoplankton in neritic waters of the northern Gulf of Mexico off Louisiana: composition, relative abundance, and seasonality. Fish. Bull. 84, 935–946.
Ditty, J. G., Zieske, G. G., and Shaw, R. F. (1988). Seasonality and depth distribution of larval fishes in the northern Gulf of Mexico above latitude 26 degree 00’N. Fish. Bull. 86, 811–823.
D’Sa, E. J., and Miller, R. L. (2003). Bio-optical properties in waters influenced by the Mississippi river during low flow conditions. Remote Sens. Environ. 84, 538–549. doi: 10.1016/s0034-4257(02)00163-3
Duan, J., Liu, W., Zhao, X., Han, Y., O’Reilly, S. E., and Zhao, D. (2017). Study of residual oil in Bay Jimmy sediment 5 years after the Deepwater Horizon oil spill: persistence of sediment retained oil hydrocarbons and effect of dispersants on desorption. Sci. Tot. Environ. 618, 1244–1253. doi: 10.1016/j.scitotenv.2017.09.234
Duarte, C. M., Pitt, K. A., Lucas, C. H., Purcell, J. E., Uye, S.-I., Robinson, K., et al. (2012). Is global ocean sprawl a cause of jellyfish blooms? Front. Ecol. Environ. 11, 91–97. doi: 10.1890/110246
Dubansky, B., Whitehead, A., Miller, J. T., Rice, C. D., and Galvez, F. (2013). Multitissue molecular, genomic, and developmental effects of the Deepwater Horizon oil spill on resident Gulf killifish (Fundulus grandis). Environ. Sci. Technol. 47, 5074–5082. doi: 10.1021/es400458p
Ducklow, H. W., and Mitchell, R. (1979). Composition of mucus released by coral reef coelenterates. Limno. Oceanogr. 24, 706–714. doi: 10.4319/lo.1979.24.4.0706
Echols, B. S., Smith, A. J., Gardinali, P. R., and Rand, G. M. (2016). The use of ephyrae of a scyphozoan jellyfish, Aurelia aurita, in the aquatic toxicological assessment of Macondo oils from Deepwater Horizon incident. Chemosphere 144, 1893–1900. doi: 10.1016/j.chemosphere.2015.10.082
Esler, D., Ballachey, B. E., Matkin, C., Cushing, D., Kaler, R., Bodkin, J., et al. (2018). Timelines and mechanisms of wildlife population recovery following the Exxon Valdez oil spill. Deep Sea Res. Pt II 147, 36–42. doi: 10.1016/j.dsr2.2017.04.007
Field, J., Cope, J., and Key, M. (2010). A descriptive example of applying vulnerability evaluation criteria to California nearshore finfish species. Manag. Data Poor Fish. 1, 235–246.
Fleeger, J. W., Carman, K. R., Riggio, M. R., Mendelssohn, I. A., Lin, Q., Hou, A., et al. (2015). Recovery of saltmarsh benthic microalgae and meiofauna following the Deepwater Horizon oil spill linked to recovery of Spartina alterniflora. Mar. Ecol. Prog. Ser. 536, 39–54. doi: 10.3354/meps11451
Fleeger, J. W., Riggio, M. R., Mendelssohn, I. A., Lin, Q., Deis, D. R., Johnson, D. S., et al. (2019). What promotes the recovery of salt marsh infauna after oil spills? Estuar. Coasts 42, 204–217. doi: 10.1007/s12237-018-0443-2
Fleeger, J. W., Riggio, M. R., Mendelssohn, I. A., Lin, Q., Hou, A., and Deis, D. R. (2018). Recovery of saltmarsh meiofauna six years after the Deepwater Horizon oil spill. J. Exper. Mar. Biol. Ecol. 502, 182–190. doi: 10.1016/j.jembe.2017.03.001
Florida Wildlife Commission (2020). Florida Saltwater Recreational Fishing Regulations. Available online at: http://www.eregulations.com/wp-content/uploads/2020/07/20FLSW_JULY-LR.pdf (accessed July 5, 2020).
Fodrie, F. J., and Heck, K. L. (2011). Response of coastal fishes to the Gulf of Mexico oil disaster. PLoS One 6:e21609. doi: 10.1371/journal.pone.0021609
Fodrie, J., Able, K. W., Galvez, F., Heck, K. L. Jr., and Jensen, O. P. (2014). Integrating organismal and population responses of estuarine fishes in Macondo Spill research. Bioscience 64, 778–788. doi: 10.1093/biosci/biu123
Frasier, K. E., Solsona-Berga, A., Stokes, L., and Hildebrand, J. A. (2020). “Impacts of the deepwater horizon oil spill on marine mammals and sea turtles,” in Deep Oil Spills, eds S. A. Murawski, C. H. Ainsworth, S. Gilbert, D. J. Hollander, C. B. Paris, M. Schlüter, et al. (Berlin: Springer), 431–462. doi: 10.1007/978-3-030-11605-7
Fuiman, L. A. (2002). “Special considerations of fish eggs and larvae,” in Fishery Science: The Unique Contributions of Early Life Stages, eds L. A. Fuiman and R. G. Werner (Hoboken, NJ: Blackwell Science), 1–32.
Gemmell, B. J., Bacosa, H. P., Liu, Z., and Buskey, E. J. (2016). Can gelatinous zooplankton influence the fate of crude oil in marine environments? Mar. Poll. Bull. 113, 483–487. doi: 10.1016/j.marpolbul.2016.08.065
Gilerson, A., Zhou, J., Hlaing, S., Ioannou, I. J., Schalles, J., and Gross, B. (2007). Fluorescence component in the reflectance spectra from coastal waters. Dependence on water composition. Opt. Express 15, 15702–15721. doi: 10.1364/oe.15.015702
Gohlke, J. M., Doke, D., Tipre, M., Leader, M., and Fitzgerald, T. (2011). A review of seafood safety after the Deepwater Horizon blowout. Environ. Health Perspt. 119, 1062–1069. doi: 10.1289/ehp.1103507
Gomez, F. A., Lee, S.-K., Liu, Y., Hernandez, F. J. Jr., and Muller-Karger, F. E. (2018). Seasonal patterns in phytoplankton biomass across the northern and deep Gulf of Mexico: a numerical model study. Biogeoscience 15, 3561–3576. doi: 10.5194/bg-15-3561-2018
González, J., Figueiras, F., Aranguren-Gassis, M., Crespo, B., Fernández, E., Morán, X. A. G., et al. (2009). Effect of a simulated oil spill on natural assemblages of marine phytoplankton enclosed in microcosms. Estuar. Coast. Shelf Sci. 83, 265–276. doi: 10.1016/j.ecss.2009.04.001
Govoni, J. J. (1997). The association of the population recruitment of gulf menhaden, Brevoortia patronus, with Mississippi River discharge. J. Mar. Syst. 12, 101–108. doi: 10.1016/s0924-7963(96)00091-7
Grabowski, J. H., Brumbaugh, R. D., Conrad, R. F., Keeler, A. G., Opaluch, J. J., Peterson, C. H., et al. (2012). Economic valuation of ecosystem services provided by oyster reefs. Bioscience 62, 900–909. doi: 10.1525/bio.2012.62.10.10
Grabowski, J. H., Powers, S. P., Roman, H., and Rouhani, S. (2017). Potential impacts of the 2010 Deepwater Horizon oil spill on subtidal oysters in the Gulf of Mexico. Mar. Ecol. Prog. Ser. 576, 163–174. doi: 10.3354/meps12208
Graham, W. H., Martin, D. L., Felder, D. L., Asper, V. L., and Perry, H. M. (2003). Ecological and economic implications of a tropical jellyfish invader in the Gulf of Mexico. Biol. Invas. 5, 53–69. doi: 10.1007/978-94-010-0169-4_6
Graham, W. M., Condon, R. H., Carmichael, R. H., D’Ambra, I., Patterson, H. K., Linn, L. J., et al. (2010). Oil carbon entered the coastal planktonic food web during the Deepwater Horizon oil spill Environ. Res. Lett. 5:045301. doi: 10.1088/1748-9326/5/4/045301
Haney, J. C., Geiger, H. J., and Short, J. W. (2014). Bird mortality from the Deepwater Horizon oil spill. II. Carcass sampling and exposure probability in the coastal Gulf of Mexico. Mar. Ecol. Prog. Ser. 513, 239–252. doi: 10.3354/meps10839
Helser, T. E., Condrey, R. E., and Geaghan, J. P. (1993). Spotted Seatrout distribution in four coastal Louisiana estuaries. Trans. Am. Fish Soc. 122, 99–111. doi: 10.1577/1548-8659(1993)122<0099:ssdifc>2.3.co;2
Herdter, E., Mahmoudi, B., Peebles, E., and Murawski, S. A. (2019). Spatial variability in size structure, growth, and recruitment of Spotted Seatrout among six Florida estuaries. Mar. Coast. Fish. 11, 97–111. doi: 10.1002/mcf2.10063
Hernandez, F. J. Jr., Powers, S. P., and Graham, W. M. (2010a). Detailed examination of ichthyoplankton seasonality from a high-resolution time series in the northern Gulf of Mexico during 2004-2006. Trans. Am. Fish. Soc. 139, 1511–1525. doi: 10.1577/T10-001.1
Hernandez, F. J. Jr., Powers, S. P., and Graham, W. M. (2010b). Seasonal variability in ichthyoplankton abundance and assemblage composition in the northern Gulf of Mexico off Alabama. Fish. Bull. 108, 193–207.
Hernandez, F. J. Jr., and Shaw, R. F. (2003). Comparison of plankton net and light-trap methodologies for sampling larval and juvenile fishes at offshore petroleum platforms and a coastal jetty off Louisiana. Am. Fish. Soc. Symp. 36, 15–38.
Hernandez, F. J. Jr., Shaw, R. F., Cope, J. S., Ditty, J. G., and Farooqi, T. (2003). The across-shelf larval, postlarval, and juvenile fish community associated with offshore oil and gas platforms and a coastal rock jetty west of the Mississippi River Delta. Am. Fish. Soc. Symp. 36, 39–72.
Hernandez, F. J., Filbrun, J. E., Fang, J., and Ransom, J. T. (2016). Condition of larval Red Snapper (Lutjanus campechanus) relative to environmental variability and the Deepwater Horizon oil spill. Environ. Res. Lett. 11:094019. doi: 10.1088/1748-9326/11/9/094019
Hester, M. W., Willis, J. M., Rouhani, S., Steinhoff, M. A., and Baker, M. C. (2016). Impacts of the Deepwater Horizon oil spill on the salt marsh vegetation of Louisiana. Environ. Pollut. 216, 361–370. doi: 10.1016/j.envpol.2016.05.065
Hobday, A. J., Smith, A. D. M., Stobutzki, I. C., Bulman, C., Daley, R., Dambacher, J. M., et al. (2011). Ecological risk assessment for the effects of fishing. Fish. Res. 108, 372–384. doi: 10.1016/j.fishres.2011.01.013
Hornsby, F., McDonald, T., Balmer, B., Speakman, T., Mullin, K., Rosel, P., et al. (2017). Using salinity to identify common bottlenose dolphin habitat in Barataria Bay, Louisiana, USA. Endang. Spec. Res. 33, 181–192. doi: 10.3354/esr00807
Houde, E. D. (2008). Emerging from Hjort’s shadow. J. Northwest Atl. Fish. Sci. 41, 53–70. doi: 10.2960/J.v41.m634
Houghton, J. D. R., Doyle, T. K., Davenport, J., and Hays, G. C. (2006). The ocean sunfish Mola mola: insights into distribution, abundance and behaviour in the Irish and Celtic Seas. J. Mar. Biol. Assoc. 86, 1237–1243. doi: 10.1017/S002531540601424X
Hu, C., Muller-Karger, F. E., Biggs, D. C., Carder, K. L., Nababan, B., Nadeau, D., et al. (2003). Comparison of ship and satellite bio-optical measurements on the continental margin of the NE Gulf of Mexico. Int. J. Remote Sens. 24, 2597–2612. doi: 10.1080/0143116031000067007
Hu, C., Weisberg, R. H., Liu, Y., Zheng, L., and Daly, K. L. (2011). Did the northeastern Gulf of Mexico become greener after the Deepwater Horizon oil spill? Geophys. Res. Lett. 38:L09601. doi: 10.1029/2011GL047184
Huettel, M., Overholt, W. A., Kostka, J. E., Hagan, C., Kaba, J., Wells, W. B., et al. (2018). Degradation of Deepwater Horizon oil buried in a Florida beach influenced by tidal pumping. Mar. Pollu. Bull. 126, 488–500. doi: 10.1016/j.marpolbul.2017.10.061
Hughes, A. R., Cebrian, J., Heck, K., Goff, J., Hanley, T. C., Scheffel, W., et al. (2018). Effects of oil exposure, plant species composition, and plant genotypic diversity on salt marsh and mangrove assemblages. Ecosphere 9:e02207. doi: 10.1002/ecs2.2207
Karnauskas, M., Schirripa, M. J., Craig, J. K., Cook, G. S., Kelble, C. R., Agar, J. J., et al. (2015). Evidence of climate-driven ecosystem reorganization in the Gulf of Mexico. Glo. Chang. Biol. 15, 12894. doi: 10.1111/gcb.12894
Kellar, N. M., Speakman, T. R., Smith, C. R., Lane, S. M., Balmer, B. C., Trego, M. L., et al. (2017). Low reproductive success rates of common bottlenose dolphins Tursiops truncatus in the northern Gulf of Mexico following the Deepwater Horizon disaster (2010-2015). Endang. Spec. Res. 33, 143–158. doi: 10.3354/esr00775
Khursigara, A. J., Perrichon, P., Bautista, N. M., Burggren, W. W., and Esbaugh, A. J. (2017). Cardiac function and survival are affected by crude oil in larval red drum, Sciaenops ocellatus. Sci. Total Environ. 579, 797–804. doi: 10.1016/j.scitotenv.2016.11.026
Kilborn, J. P., Drexler, M., and Jones, D. L. (2018). Fluctuating fishing intensities and climate dynamics reorganize the Gulf of Mexico’s fisheries resources. Ecosphere 9:e02487. doi: 10.1002/ecs2.2487
Kilborn, J. P., Jones, D. L., Peebles, E. B., and Naar, D. F. (2017). Resemblance profiles as clustering decision criteria: estimating statistical power, error, and correspondence for a hypothesis test for multivariate structure. Ecol. Evol. 7, 2039–2057. doi: 10.1002/ece3.2760
Kostka, J. E., Prakash, O., Overholt, W. A., Green, S. J., Freyer, G., Canion, A., et al. (2011). Hydrocarbon-degrading bacteria and the bacterial community response in Gulf of Mexico beach sands impacted by the Deepwater Horizon oil spill. Appl. Environ. Microb. 77, 962–967. doi: 10.1128/AEM.05402-5411
Lane, S. M., Smith, C. R., Mitchell, J., Balmer, B. C., Barry, K. P., McDonald, T., et al. (2015). Reproductive outcome and survival of common bottlenose dolphins sampled in Barataria Bay, Louisiana, USA, following the Deepwater Horizon oil spill. Proc. R. Soc. B Biol. Sci. 282:20151944. doi: 10.1098/rspb.2015
Lee, C. E., Remfert, J. L., Opgenorth, T., Lee, K. M., Stanford, E., Connolly, J. W., et al. (2017). Evolutionary responses to crude oil from the Deepwater Horizon oil spill by the copepod Eurytemora affinis. Evol. Appl. 10, 813–828. doi: 10.1111/eva.12502
Lee, R. F., Köster, M., and Paffenhöfer, G.-A. (2012). Ingestion and defecation of dispersed oil droplets by pelagic tunicates. J. Plank. Res. 34, 1058–1063. doi: 10.1093/plankt/fbs065
Lees, K., Pitois, S., Scott, C., Frid, C., and Mackinson, S. (2006). Characterizing regime shifts in the marine environment. Fish Fish. 7, 104–127. doi: 10.1111/j.1467-2979.2006.00215.x
Levin, P. S., and Möllmann, C. (2015). Marine ecosystem regime shifts: challenges and opportunities for ecosystem-based management. Philos. Trans. R. Soc. B 370:20130275. doi: 10.1098/rstb.2013.0275
Lewis, R. M., and Roithmayr, C. M. (1981). Spawning and sexual maturity of gulf menhaden, Brevoortia patronus. Fish. Bull. 78, 947–951.
Li, Y., Hu, C., Quigg, A., and Gao, H. (2019). Potential influence of the Deepwater Horizon oil spill on phytoplankton primary productivity in the northern Gulf of Mexico. Environ. Res. Lett. 14:094018. doi: 10.1088/1748-9326/ab3735
Litz, J. A., Baran, M. A., Bowen-Stevens, S. R., Carmichael, R. H., Colegrove, K. M., Garrison, L. P., et al. (2014). Review of historical unusual mortality events (UMEs) in the Gulf of Mexico (1990-2009): providing context for the multi-year northern Gulf of Mexico cetacean UME declared in 2010. Dis. Aquat. Organ. 112, 161–175. doi: 10.3354/dao02807
Livernois, M. C., Powers, S. P., Albins, M. A., and Mareska, J. F. (2020). Habitat associations and co-occurrence patterns of two estuarine-dependent predatory fishes. Mar. Coast. Fish. 12, 64–77. doi: 10.1002/mcf2.10104
Louisiana Department of Wildlife and Fisheries (2020). Louisiana 2020 Fishing Regulations. Available online at: http://www.eregulations.com/wp-content/uploads/2019/12/20LAFW_LR.pdf (accessed July 5, 2020).
Lowerre-Barbieri, S. K., Tringali, M. D., Shea, C. P., Waters, B. S., Bickford, J., Murphy, M., et al. (2019). Assessing red drum spawning aggregations and abundance in the eastern Gulf of Mexico: a multidisciplinary approach. ICES J. Mar. Sci. 76, 516–529. doi: 10.1093/icesjms/fsy173
Lowerre-Barbieri, S. K., Waters, B. S. L., and Bickford, J. W. (2016). Assessing reproductive behavior important to fisheries management: a case study with red drum, Sciaenops ocellatus. Ecol. Appl. 26, 979–995. doi: 10.1890/15-0497
Lucas, C. H. (2001). Reproduction and life history strategies of the common jellyfish, Aurelia aurita, in relation to its ambient environment. Hydrobiologia 451, 229–246. doi: 10.1007/978-94-010-0722-1_19
MacDonald, I. R., Garcia-Pineda, O., Beet, A., Daneshgar Asl, S., Feng, L., Graettinger, G., et al. (2015). Natural and unnatural oil slicks in the Gulf of Mexico. J. Geophys. Res. Ocean 120, 8364–8380. doi: 10.1002/2015JC011062
Macedo, D., Caballero, I., Mateos, M., Leblois, R., McCay, S., and Hurtado, L. A. (2019). Population genetics and historical demographic inferences of the blue crab Callinectes sapidus in the US based on microsatellites. PeerJ 7:e7780. doi: 10.7717/peerj.7780
Magnuson, J. T., Khursigara, A. J., Allmon, E. B., Esbaugh, A. J., and Roberts, A. P. (2018). Effects of Deepwater Horizon crude oil on ocular development in two estuarine fish species, red drum (Sciaenops ocellatus) and sheepshead minnow (Cyprinodon variegatus). Ecotoxicol. Environ. Saf. 166, 186–191. doi: 10.1016/j.ecoenv.2018.09.087
Martin, C. W. (2017). Avoidance of oil contaminated sediments by estuarine fishes. Mar. Ecol. Prog. Ser. 576, 125–134. doi: 10.3354/meps12084
Martindale, M. Q. (1987). Larval reproduction in the ctenophore Mnemiopsis mccradyi (order Lobata). Mar. Biol. 94, 409–414. doi: 10.1007/BF00428247
Martínez, M. L., Feagin, R. A., Yeager, K. M., Day, J., Costanza, R., Harris, J. A., et al. (2012). Artificial modifications of the coast in response to the Deepwater Horizon oil spill: quick solutions or longterm liabilities? Front. Ecol. Environ. 10, 44–49. doi: 10.1890/100151
McCall, B. D., and Pennings, S. C. (2012). Disturbance and recovery of salt marsh arthropod communities following BP Deepwater Horizon oil spill. PLoS One 7:e32735. doi: 10.1371/journal.pone.0032735
McCann, M. J., Able, K. W., Christian, R. R., Fodrie, F. J., Jensen, O., Johnson, J. J., et al. (2017). Key taxa in food web responses to stressors: the Deepwater Horizon oil spill. Front. Ecol. Environ. 15, 142–149. doi: 10.1002/fee.1474
McKee, D., Cunningham, A., Wright, D., and Hay, L. (2007). Potential impacts of nonalgal materials on water-leaving Sun induced chlorophyll fluorescence signals in coastal waters. Appl. Optics 46, 7720–7729. doi: 10.1364/AO.46.007720
Michel, J., Fegley, S. R., Dahlin, J. A., and Wood, C. (2017). Oil spill response-related injuries on sand beaches: when shoreline treatment extends the impacts beyond the oil. Mar. Ecol. Prog. Ser. 576, 203–218. doi: 10.3354/meps11917
Michel, J., and Rutherford, N. (2013). Oil Spills in Marshes: Planning and Response Considerations. Available online at: https://response.restoration.noaa.gov/sites/default/files/Oil_Spills_in_Marshes.pdf (accessed November 23, 2020).
Mishra, D. R., Cho, H. J., Ghosh, S., Fox, A., Downs, C., Merani, P. B. T., et al. (2012). Post-spill state of the marsh: remote estimation of the ecological impact of the Gulf of Mexico oil spill on Louisiana salt marshes. Remote Sens. Environ. 118, 176–185. doi: 10.1016/j.rse.2011.11.007
Moody, R. M., Cebrian, J., and Heck, K. L. (2013). Interannual recruitment dynamics for resident and transient marsh species: evidence for a lack of impact by the Macondo oil spill. PLoS One 8:e58376. doi: 10.1371/journal.pone.0058376
Moore, J. F., Pine, W. E. III, Frederick, P. C., Beck, S., and Moreno, M. (2020). Trends in oyster populations in the northeastern Gulf of Mexico: an assessment of river discharge and fishing effects over time and space. Mar. Coast. Fish. 12, 191–204. doi: 10.1002/mcf2.10117
Moulton, D. L., Dance, M. A., Williams, J. A., Sluis, M. Z., Stunz, G. W., and Rooker, J. R. (2017). Habitat partitioning and seasonal movement of Red Drum and Spotted Seatrout. Estuar. Coast. 40, 905–916. doi: 10.1007/s12237-016-0189-7
Muhling, B. A., Roffer, M. A., Lamkin, J. T., Ingram, G. W. Jr., Upton, M. A., Gawlikowski, G., et al. (2012). Overlap between Atlantic bluefin tuna spawning grounds and observed Deepwater Horizon surface oil in the northern Gulf of Mexico. Mar. Poll. Bull. 64, 679–687. doi: 10.1016/j.marpolbul.2012.01.034
Murawski, S. A., Fleeger, J. W., Patterson, W. F. III, Hu, C., Daly, K. I., Romero, I., et al. (2016). How did the Deepwater Horizon oil spill affect coastal and continental shelf ecosystems of the Gulf of Mexico? Oceanography 29, 160–173. doi: 10.5670/oceanog.2016.80
Murawski, S. A., Hogarth, W. T., Peebles, E. B., and Barbieri, L. (2014). Prevalence of external skin lesions and polycyclic aromatic hydrocarbon concentrations in Gulf of Mexico fishes, post-Deepwater Horizon. Trans. Am. Fish. Soc. 143, 1084–1097. doi: 10.1080/00028487.2014.911205
Murphy, M. D., and Crabtree, R. E. (2001). Changes in the age structure of nearshore adult Red Drum off West-Central Florida related to recruitment and fishing mortality. North. Am. J. Fish. Mgt. 21, 671–678. doi: 10.1577/1548-8675(2001)021<0671:citaso>2.0.co;2
National Academies of Science, Engineering, and Medicine [NASEM] (2020). The Use of Dispersants in Marine Oil Spill Response. Washington, DC: The National Academies Press.
National Research Council (2013). Report of the Committee on the Effects of the Deepwater Horizon Mississippi Canyon-252 Oil Spill on Ecosystem Services in the Gulf of Mexico. Washington, DC: The National Academies Press.
Nieland, D. L., Thomas, R. G., and Wilson, C. A. (2002). Age, growth, and reproduction of spotted seatrout in Barataria Bay, Louisiana. Trans. Am. Fish. Soc. 131, 245–259. doi: 10.1577/1548-8659(2002)131<0245:agaros>2.0.co;2
Nixon, Z., Zengel, S., Baker, M., Steinhoff, M., Fricano, G., Rouhani, S., et al. (2016). Shoreline oiling from the Deepwater Horizon oil spill. Mar. Poll. Bull. 107, 170–178. doi: 10.1016/j.marpolbul.2016.04.003
O’Connell, M. T., Peterson, M. S., Powers, S. P., Uzee-O’Connell, A. M., Anderson, E. J., and Hendon, J. R. (2019). Assessing nearshore nekton abundance, substrate, and environmental conditions in the northern Gulf of Mexico: are there differences among three adjacent coastal areas and have there been changes over three decades? Estuar. Coasts 42:6769263. doi: 10.1007/s12237-019-00632-z
O’Connor, B. S., Muller-Karger, F. E., Nero, R. W., Hu, C., and Peebles, E. B. (2016). The role of Mississippi River discharge in offshore phytoplankton blooming in the northeastern Gulf of Mexico during August 2010. Rem. Sens. Environ. 173, 133–144. doi: 10.1016/j.rse.2015.11.004
Olson, G. M., Meyer, B. M., and Portier, R. J. (2015). Assessment of the toxic potential of polycyclic aromatic hydrocarbons (PAHs) affecting Gulf menhaden (Brevoortia patronus) harvested from waters impacted by the BP Deepwater Horizon Spill. Chemosphere 145, 322–328. doi: 10.1016/j.chemosphere.2015.11.087
Ozhan, K., Parsons, M. L., and Bargu, S. (2014). How were phytoplankton affected by the Deepwater Horizon oil spill? Bioscience 64, 829–836. doi: 10.1093/biosci/biu117
Parsons, M., Morrison, W., Rabalais, N., Turner, R., and Tyre, K. (2015). Phytoplankton and the Macondo oil spill: a comparison of the 2010 phytoplankton assemblage to baseline conditions on the Louisiana shelf. Environ. Pollut. 207, 152–160. doi: 10.1016/j.envpol.2015.09.019
Patrick, W. S., Spencer, P., Link, J., Cope, J., Field, J., Kobayashi, D., et al. (2010). Using productivity and susceptibility indices to assess the vulnerability of United States fish stocks to overfishing. Fish Bull. 108, 305–322.
Pennings, S. C., McCall, B. D., and Hooper-Bui, L. (2014). Effects of oil spills on terrestrial arthropods in coastal wetlands. Bioscience 64, 789–795. doi: 10.1093/biosci/biu118
Pennings, S. C., Zengel, S., Oehrig, J., Alber, M., Bishop, T. D., Deis, D. R., et al. (2016). Marine ecoregion and Deepwater Horizon oil spill affect recruitment and population structure of a salt marsh snail. Ecosphere 7:e01588. doi: 10.1002/ecs2.1588
Perret, W. S., Weaver, J. E., Williams, R. O., Johansen, P. L., Mcilwain, T. D., Raulerson, R. C., et al. (1980). Fishery Profiles of Red Drum and Spotted Seatrout. Gulf States Marine Fisheries Commission, Report No. 6. Ocean Springs, MI: Gulf States Marine Fisheries Commission.
Peterson, C. H., Anderson, S. S., Cherr, G. N., Ambrose, R. F., Bay, S., and Blum, M. (2012). A tale of two spills: novel science and policy implications of an emerging new oil spill model. Bioscience 62, 461–469. doi: 10.1525/bio.2012.62.5.7
Peterson, C. H., Rice, S. D., Short, J. W., Esler, D., Bodkin, J. L., Ballachey, B. E., et al. (2003). Long-term ecosystem response to the exxon valdez oil spill. Science 302, 2082–2086. doi: 10.1126/science.1084282
Pitt, K. A., Welsh, D. T., and Condon, R. H. (2009). Influence of jellyfish blooms on carbon, nitrogen and phosphorus cycling and plankton production. Hydrobiologia 616, 133–149. doi: 10.1007/s10750-008-9584-9
Polidoro, B., Matson, C. W., Ottinger, M. A., Renegar, D. A., Romero, I. C., Schlenk, D., et al. (2020). A multi-taxonomic framework for assessing relative petrochemical vulnerability of marine biodiversity in the Gulf of Mexico. Sci. Tot. Environ. (in press). doi: 10.1016/j.scitotenv.2020.142986
Powers, S. P., Grabowski, J. H., Roman, H., Geggel, A., Rouhani, S., Oehrig, J., et al. (2017a). Consequences of large-scale salinity alteration during the Deepwater Horizon oil spill on subtidal oyster populations. Mar. Ecol. Prog. Ser. 576, 175–187. doi: 10.3354/meps12147
Powers, S. P., Peterson, C. H., Cebrian, J., and Heck, K. L. Jr. (2017b). Response of nearshore ecosystems to the Deepwater Horizon spill. Mar. Ecol. Prog. Ser. 576, 107–110. doi: 10.3354/meps12254
Powers, S. P., Rouhani, S., Baker, M. C., Roman, H., Grabowski, J. H., Scyphers, S. B., et al. (2017c). Ecosystem services are lost when facilitation between two ecosystem engineers is compromised by oil. Mar. Ecol. Prog. Ser. 576, 189–202. doi: 10.3354/meps12201
Powers, S. P., and Scyphers, S. B. (2016). Estimating Injury to Nearshore Fauna Resulting from the Deepwater Horizon Oil Spill. Technical Report. Available online at: https://www.fws.gov/doiddata/dwh-ardocuments/913/DWH-AR0301453.pdf (accessed June 25, 2020).
Pulster, E. L., Gracia, A., Armenteros, M., Toro-Farmer, G., Snyder, S. M., Carr, B. E., et al. (2020). A first comprehensive baseline of hydrocarbon pollution in Gulf of Mexico fishes. Sci. Rep. 10:6437. doi: 10.1038/s41598-020-62944-6
Purcell, J. E. (1997). Pelagic cnidarians and ctenophores as predators: selective predation, feeding rates, and effects on prey populations. Annu. l’Instit. Oceanogr. 73, 125–137.
Purcell, J. E. (2007). Environmental effects on asexual reproduction rates of the scyphozoan Aurelia labiate. Mar. Ecol. Prog. Ser. 348, 183–196. doi: 10.3354/meps07056
Purcell, J. E., White, J. R., Nemazie, D. A., and Wright, D. A. (1999). Temperature, salinity and food effects on asexual reproduction and abundance of the scyphozoan Chrysaora quinquecirrha. Mar. Ecol. Prog. Ser. 180, 187–196. doi: 10.3354/meps180187
Purcell, K. M., Craig, J. K., Nance, J. M., Smith, M. D., and Bennear, L. S. (2017). Fleet behavior is responsive to a large-scale environmental disturbance: hypoxia effects on the spatial dynamics of the northern Gulf of Mexico shrimp fishery. PLoS One 12:e0183032. doi: 10.1371/journal.pone.0183032
Rabalais, N. N., and Turner, R. E. (2016). Effects of the Deepwater Horizon oil spill on coastal marshes and associated organisms. Oceanography 29, 150–159. doi: 10.5670/oceanog.2016.79
Ransom, J. T., Filbrun, J. E., and Hernandez, F. J. (2016). Condition of larval Spanish mackerel Scomberomorus maculatus in relation to the Deepwater Horizon oil spill. Mar. Ecol. Prog. Ser. 558, 143–152. doi: 10.3354/meps11880
Redalje, D. G., Lohrenz, S. E., and Fahnenstiel, G. L. (1994). The relationship between primary production and the vertical export of particulate organic matter in a river-impacted coastal ecosystem. Estuaries 17, 829–838. doi: 10.2307/1352751
Reddy, C. M., Eglinton, T. I., Hounshell, A., White, H. K., Xu, L., Gaines, R. B., et al. (2002). The West Falmouth oil spill: the persistence of petroleum hydrocarbons in marsh sediments. Environ. Sci. Tech. 36, 4754–4760. doi: 10.1021/es020656n
Robinson, K. L., and Graham, W. M. (2013). Long-term change in the abundances of northern Gulf of Mexico scyphomedusae Chrysaora spp. and Aurelia spp. with links to climate variability. Limnol. Oceanogr. 58, 235–253. doi: 10.4319/lo.2013.58.1.0235
Robinson, K. L., Ruzicka, J. J., Hernandez, F. J., Graham, W. H., Decker, M. B., Brodeur, R. D., et al. (2015). Evaluating energy flows through jellyfish and gulf menhaden (Brevoortia patronus) and the effects of fishing on the northern Gulf of Mexico ecosystem. ICES J. Mar. Sci. 72, 2301–2312. doi: 10.1093/icesjms/fsv088
Rodgers, M. L., Simning, D., Sepúlveda, M. S., De Guise, S., Bosker, T., and Griffitt, R. J. (2020). Exposure to oil and hypoxia results in alterations of immune transcriptional patterns in developing Sheepshead Minnows (Cyprinodon variegatus). Sci. Rep. 10, 1–9. doi: 10.1038/s41598-020-58171-8
Rooker, J. R., Kitchens, L. L., Dance, M. A., Wells, R. D., Falterman, B., and Cornic, M. (2013). Spatial, temporal, and habitat-related variation in abundance of pelagic fishes in the Gulf of Mexico: potential implications of the Deepwater Horizon oil spill. PLoS One 8:e76080. doi: 10.1371/journal.pone.0076080
Rose, K. A., Huang, H., Justic, D., and de Mutsert, K. (2014). Simulating fish movement responses to and potential salinity stress from large-scale river diversions. Mar. Coast. Fish. 6, 43–61. doi: 10.1080/19425120.2013.866999
Rouhani, S., Baker, M. C., Steinhoff, M., Zhang, M., Oehrig, J., Zelo, I. J., et al. (2017). Nearshore exposure to Deepwater Horizon oil. Mar. Ecol. Prog. Ser. 576, 111–124. doi: 10.3354/meps11811
Rozas, L. P., Minello, T. J., and Miles, M. S. (2014). Effect of Deepwater Horizon oil on growth rates of juvenile penaeid shrimps. Estuar. Coasts. 37, 1403–1414. doi: 10.1007/s12237-013-9766-1
Saiz, E., Movilla, J., Yebra, L., Barata, C., and Calbet, A. (2009). Lethal and sublethal effects of naphthalene and 1,2-dimethylnaphthalene on naupliar and adult stages of the marine cyclopoid copepod Oithona davisae. Environ. Poll. 157, 1219–1226. doi: 10.1016/j.envpol.2008.12.011
Saucier, M. H., and Baltz, D. M. (1993). Spawning site selection by spotted seatrout, Cynoscion nebulosus, and black drum, Pogonias cromis, in Louisiana. Environ. Biol. Fish. 36, 257–272. doi: 10.1007/bf00001722
Schaefer, J., Frazier, N., and Barr, J. (2016). Dynamics of near-coastal fish assemblages following the Deepwater Horizon oil spill in the northern Gulf of Mexico. Trans. Am. Fish. Soc. 145, 108–119. doi: 10.1080/00028487.2015.1111253
Schrandt, M. C., Powers, S. P., Rikard, S., Thongda, W., and Peatman, E. (2018). Short-term low salinity mitigates effects of oil and dispersant on juvenile eastern oysters: a laboratory experiment with implications for oil spill response activities. PLoS One 13:e0203485. doi: 10.1371/journal.pone.0203485
Schwacke, L. H., Smith, C. R., Townsend, F. I., Wells, R. S., Hart, L. B., Balmer, B. C., et al. (2014). Health of common bottlenose dolphins (Tursiops truncatus) in Barataria Bay, Louisiana, following the Deepwater Horizon oil spill. Environ. Sci. Technol. 48, 93–103. doi: 10.1021/es403610f
Schwacke, L. H., Thomas, L., Wells, R. S., Mcfee, W. E., Hohn, A. A., Mullin, K. D., et al. (2017). Quantifying injury to common bottlenose dolphins from the Deepwater Horizon oil spill using an age-, sex-and class-structured population model. Endang. Spec. Res. 33, 265–279. doi: 10.3354/esr00777
Schwing, P. T., Montagna, P. A., Joye, S. B., Paris, C. B., Cordes, E. E., McClain, C. R., et al. (2020). A synthesis of deep benthic faunal impacts and resilience following the Deepwater Horizon oil spill. Front. Mar. Sci. 7:560012. doi: 10.3389/fmars.2020.56001
Sehlinger, T., Lowe, M. R., La Peyre, M. K., and Soniat, T. M. (2019). Differential effects of temperature and salinity on growth and mortality of oysters (Crassostrea virginica) in Barataria Bay and Breton Sound, Louisiana. J. Shellfish Res. 38, 317–326. doi: 10.2983/035.038.0212
Selle, C. (2020). Linking Spatiotemporal Variability Among Phytoplankton, Microzooplankton and Mesozooplankton in a Shallow, Microtidal Estuary. M. Sc. Thesis, University of Louisiana at Lafayette, Lafayette, 59.
Serafin, J., Guffey, S. C., Bosker, T., Griffitt, R. J., De Guise, S., Perkins, C., et al. (2019). Combined effects of salinity, temperature, hypoxia, and Deepwater Horizon oil on Fundulus grandis larvae. Ecotoxicol. Environ. Saf. 181, 106–113. doi: 10.1016/j.ecoenv.2019.05.059
Seuront, L. (2011). Hydrocarbon contamination decreases mating success in a marine planktonic copepod. PLoS One 6:e026283. doi: 10.1371/journal.pone.0026283
Shanks, A. L., and Graham, W. M. (1988). Chemical defense in a scyphomedusa. Mar. Ecol. Prog. Ser. 45, 81–86. doi: 10.3354/meps045081
Shaw, R. F., Cowan, J. A. Jr., and Tilman, T. L. (1985). Distribution and density of Brevoortia patronus (gulf menhaden) eggs and larvae in the continental shelf waters of Western Louisiana. Bull. Mar. Sci. 36, 96–103.
Shaw, R. F., Rogers, B. D., Cowan, J. A. Jr., and Herke, W. H. (1988). Ocean-estuary coupling of ichthyoplankton and nekton in the northern Gulf of Mexico. Am. Fish. Soc. Symp. 3, 7–89.
Shiplett, R. M. (2011). Selective Feeding and Ichthyoplankton Predation by Scyphomedusae in the Northern Gulf of Mexico. M. Sc. thesis, University of South Alabama, Mobile, AL, 110.
Short, J. W., Geiger, H. J., Haney, C., Voss, C. M., Vozzo, M. L., Guillory, V., et al. (2017). Anomalously high recruitment of the 2010 Gulf Menhaden (Brevoortia patronus) year class: evidence of indirect effects from the Deepwater Horizon blowout in the Gulf of Mexico. Arch. Environ. Contam. Toxicol. 73, 76–92. doi: 10.1007/s00244-017-0374-0
Silliman, B. R., Koppel, J. V. D., McCoy, M. W., Diller, J., Kasozi, G. N., Earl, K., et al. (2012). Degradation and resilience in Louisiana salt marshes after the BP-Deepwater Horizon oil spill. Proc. Nat. Acad. Sci. U.S.A. 109, 11234–11239. doi: 10.1073/pnas.1204922109
Smith, C. R., Rowles, T. K., Hart, L. B., Townsend, F. I., Wells, R. S., Zolman, E. S., et al. (2017). Slow recovery of Barataria Bay dolphin health following the Deepwater Horizon oil spill (2013-2014), with evidence of persistent lung disease and impaired stress response. Endang. Spec. Res. 33, 127–142. doi: 10.3354/esr00778
Stobutzki, I., Miller, M., and Brewer, D. (2001). Sustainability of fishery bycatch: a process for assessing highly diverse and numerous bycatch. Environ. Conserv. 28, 167–181. doi: 10.1017/S0376892901000170
Suderman, B. L., and Marcus, N. H. (2002). The effects of Orimulsion and Fuel Oil #6 on the hatching success of copepod resting eggs in the seabed of Tampa Bay, Florida. Environ. Pollut. 120, 787–795.
Suir, G. M., Jones, W. R., Garber, A. L., and Gailani, J. Z. (2016). Landscape Evolution of the Oil Spill Mitigation Sand Berm in the Chandeleur Islands, Louisiana. U.S. Army Corps of Engineers, ERDC TR-16-15. Washington, DC: U.S. Army Corps of Engineers.
Sutherland, K. R., Madin, L. P., and Stocker, R. (2010). Filtration of submicrometer particles by pelagic tunicates. Proc. Natl. Acad. Sci. U.S.A. 107, 15129–15134. doi: 10.1073/pnas.1003599107
Takeshita, R., Sullivan, L., Smith, C., Collier, T., Hall, A., Brosnan, T., et al. (2017). The Deepwater Horizon oil spill marine mammal injury assessment. Endang. Spec. Res. 33, 95–106. doi: 10.3354/esr00808
Tunnell, J. W. Jr. (2017). “Shellfish of the Gulf of Mexico,” in Habitats and Biota of the Gulf of Mexico: Before the Deepwater Horizon Oil Spill, ed. C. H. Ward (Cham: Springer), 769–839. doi: 10.1007/978-1-4939-3447-8_8
Turner, R. E., and Brody, M. S. (1983). Habitat Suitability Index Models: Northern Gul f of Mexico Brown Shrimp and White Shrimp. Washington, DC: U.S. Fish and Wildlife Service.
Turner, R. E., Layne, M., Mo, Y., and Swensen, E. M. (2019b). Net land gain or loss for two Mississippi river diversions: Caernarvon and Davis Pond. Restor. Ecol. 27, 1231–1240. doi: 10.1111/rec.13024
Turner, R. E., Rabalais, N. N., Overton, E. B., Meyer, B. M., McClenachan, G., Swenson, E. M., et al. (2019a). Oiling of the continental shelf and coastal marshes over eight years after the 2010 Deepwater Horizon oil spill. Environ. Pollut. 252, 1367–1376. doi: 10.1016/j.envpol.2019.05.134
van der Ham, J. L., and de Mutsert, K. (2014). Abundance and size of Gulf shrimp in Louisiana’s coastal estuaries following the Deepwater Horizon oil spill. PLoS One 9:e108884. doi: 10.1371/journal.pone.0108884
S. VanderKooy (ed.) (2013). GDAR01 Stock Assessment Report Gulf of Mexico Blue Crab. Gulf States Marine Fisheries Commission No. 2015. Available online at: https://www.gsmfc.org/publications/GSMFC%20Number%20215.pdf (accessed June 23, 2020).
S. J. VanderKooy (ed.) (2001). The Spotted Seatrout Fishery of the Gulf of Mexico, United States: A Regional Management Plan. Gulf States Marine Fisheries Commission Report 87. Available online at: https://www.gsmfc.org/publications/GSMFC%20Number%20087.pdf (accessed June 26, 2020).
S. J. VanderKooy (ed.) (2012). The Oyster Fishery of the Gulf of Mexico, United States: A fisheries Management Plan 2012 Revision by the Oyster Technical Task Force. Gulf States Marine Fisheries Commission Report 202. Available online at: https://www.gsmfc.org/publications/GSMFC%20Number%20202.pdf (accessed June 24, 2020).
Venn-Watson, S., Colegrove, K. M., Litz, J., Kinsel, M., Terio, K., Saliki, J., et al. (2015a). Adrenal gland and lung lesions in Gulf of Mexico common bottlenose dolphins (Tursiops truncatus) found dead following the Deepwater Horizon oil spill. PLoS One 10:e0126538. doi: 10.1371/journal.pone.0126538
Venn-Watson, S., Garrison, L., Litz, J., Fougeres, E., Mase, B., Rappucci, G., et al. (2015b). Demographic clusters identified within the northern Gulf of Mexico common bottlenose dolphin (Tursiops truncatus) unusual mortality event: January 2010-June 2013. PLoS One 10:e0117248. doi: 10.1371/journal.pone.0117248
Wakeman, J. M., and Ramsey, P. R. (1985). A survey of population characteristics for Red Drum and Spotted Seatrout in Louisiana. Gulf Res. Rep. 8, 1–8. doi: 10.18785/grr.0801.01
Wallace, B. P., Stacy, B. A., Rissing, M., Cacela, D., Garrison, L. P., Graettinger, G. D., et al. (2017). Estimating sea turtle exposures to Deepwater Horizon oil. Endang. Spec. Res. 33, 51–67. doi: 10.3354/esr00728
Wallace, R. L., Gilbert, S., and Reynolds, J. E. III (2019). Improving the integration of restoration and conservation in marine and coastal ecosystems: Lessons from the Deepwater Horizon disaster. Bioscience 69, 920–927. doi: 10.1093/biosci/biz103
Walsh, G. E. (1978). “Toxic effects of pollutants on plankton,” in Principles of Ecotoxicology, ed. G. C. Butler (New York, NY: Wiley), 257–274.
Walter, S. T., Carloss, M. R., Hess, T. J., and Leberg, P. L. (2014). Demographic trends of Brown Pelicans in Louisiana before and after the Deepwater Horizon oil spill. J. Field Ornithol. 85, 421–429. doi: 10.1111/jofo.12081
Wells, R. S., Schwacke, L. H., Rowles, T. K., Balmer, B. C., Zolman, E., Speakman, T., et al. (2017). Ranging patterns of common bottlenose dolphins Tursiops truncatus in Barataria Bay, Louisiana, following the Deepwater Horizon oil spill. Endang. Spec. Res. 33, 159–180. doi: 10.3354/esr00732
White, E. D., Messina, F., Moss, L., and Meselhe, E. (2018). Salinity and marine mammal dynamics in Barataria Basin: historic patterns and modeled diversion scenarios. Water 10:1015. doi: 10.3390/w10081015
Whitehead, A., Dubansky, B., Bodinier, C., Garcia, T. I., Miles, S., Pilley, L., et al. (2012). Genomic and physiological footprint of the Deepwater Horizon oil spill on resident marsh fishes. Proc. Natl. Acad. Sci. U.S.A. 109, 20298–20302. doi: 10.1073/pnas.1109545108
Wilson, C. A., and Nieland, D. L. (1994). Reproductive biology of red drum, Sciaenaps ocellatus, from the neritic waters of the northern Gulf of Mexico. Fish. Bull. 92, 841–850.
Xu, E. G., Khursigara, A. J., Magnuson, J., Hazard, E. S., Hardiman, G., Esbaugh, A. J., et al. (2017). Larval red drum (Sciaenops ocellatus) sublethal exposure to weathered Deepwater Horizon crude oil: developmental and transcriptomic consequences. Environ. Sci. Technol. 51, 10162–10172. doi: 10.1021/acs.est.7b02037
Ylitalo, G. M., Krahn, M. M., Dickhoff, W. W., Stein, J. E., Walker, C. C., Lassitter, C. L., et al. (2012). Federal seafood safety response to the Deepwater Horizon oil spill (2012). Proc. Natl. Acad. Sci. U.S.A. 109, 20274–20279. doi: 10.1073/pnas.1108886109
Zarada, K., Walters Burnsed, S., Bickford, J., Ducharme-Barth, N., Ahrens, R. N. M., and Lowerre-Barbieri, S. (2019). Estimating site-specific spawning parameters for a spawning aggregation: an example with spotted seatrout. Mar. Ecol. Prog. Ser. 624, 117–129. doi: 10.3354/meps13016
Zengel, S., Pennings, S. C., Silliman, B., Montague, C., Weaver, J., Deis, D. R., et al. (2016). Deepwater Horizon oil spill impacts on salt marsh fiddler crabs (Uca spp.). Estuar. Coasts 39, 1154–1163. doi: 10.1007/s12237-016-0072-6
Keywords: coastal impacts, ecosystem resilience, marine oil spills, Deepwater Horizon, Gulf of Mexico
Citation: Murawski SA, Kilborn JP, Bejarano AC, Chagaris D, Donaldson D, Hernandez FJ Jr, MacDonald TC, Newton C, Peebles E and Robinson KL (2021) A Synthesis of Deepwater Horizon Impacts on Coastal and Nearshore Living Marine Resources. Front. Mar. Sci. 7:594862. doi: 10.3389/fmars.2020.594862
Received: 14 August 2020; Accepted: 16 December 2020;
Published: 01 February 2021.
Edited by:
Just Cebrian, Mississippi State University, United StatesReviewed by:
Thadickal V. Joydas, King Fahd University of Petroleum and Minerals, Saudi ArabiaSean Powers, University of South Alabama, United States
Copyright © 2021 Murawski, Kilborn, Bejarano, Chagaris, Donaldson, Hernandez, MacDonald, Newton, Peebles and Robinson. This is an open-access article distributed under the terms of the Creative Commons Attribution License (CC BY). The use, distribution or reproduction in other forums is permitted, provided the original author(s) and the copyright owner(s) are credited and that the original publication in this journal is cited, in accordance with accepted academic practice. No use, distribution or reproduction is permitted which does not comply with these terms.
*Correspondence: Steven A. Murawski, c211cmF3c2tpQHVzZi5lZHU=