- 1School of Natural and Environmental Sciences, Newcastle University, Newcastle upon Tyne, United Kingdom
- 2British Antarctic Survey, Cambridge, United Kingdom
- 3Alfred Wegener Institute, Bremerhaven, Germany
- 4Museums Victoria, Melbourne, VIC, Australia
Habitat heterogeneity is important for maintaining high levels of benthic biodiversity. The Prince Gustav Channel, on the Eastern Antarctic Peninsula, is characterized by an array of habitat types, ranging from flat, mud-dominated sheltered bays to steep and rocky exposed slopes. The channel has undergone dramatic environmental changes in recent decades, with the southern end of the channel permanently covered by the Prince Gustav Ice Shelf until it completely collapsed in 1995. Until now the marine benthic fauna of the Prince Gustav Channel has remained unstudied. A shallow underwater camera system and Agassiz trawl were deployed at different locations across the channel to collect information on habitat type and heterogeneity, benthic community composition and macrofaunal biomass. The texture of the seafloor was found to have a significant influence on the benthos, with hard substrates supporting higher abundances and diversity. Suspension and filter feeding organisms, including porifera, crinoids, and anthozoans, were strongly associated with hard substrates, with the same being true for deposit feeders, such as holothurians, and soft sediments. Habitat heterogeneity was high across the Prince Gustav Channel, particularly on a local scale, and this was significant in determining patterns of benthic composition and abundance. Other physical variables including depth and seafloor gradient played significant, interactive roles in determining composition potentially mediated through other processes. Sites that were once covered by the Prince Gustav Ice Shelf held distinct and unique communities, suggesting that the legacy of the ice shelf collapse may still be reflected in the benthos. Biomass estimations suggest that critical thresholds of vulnerable marine ecosystem indicator taxa, as defined by the Commission for the Conservation of Antarctic Marine Living Resources, have been met at multiple locations within the Prince Gustav Channel, which has implications for the future establishment of no take zones and marine protected areas within the region.
Introduction
The Antarctic continental shelf and slope is characterized by a highly diverse benthos that displays high levels of endemism and spatial variability (Convey et al., 2014). All main types of macrobenthic communities, in particular suspension and mobile or deposit feeders, can occur all around the Southern Ocean, which suggests that their distribution on a regional and local scale is likely unpredictable and assumed to be shaped by complex biological and physical interactions (Gutt et al., 2013). Measuring species and biological diversity on the Antarctic shelf is notoriously difficult as a result of high community patchiness and complex hierarchical scales of spatial variation (Thrush et al., 2006). Additionally, sampling gaps limit our understanding of the processes at work (Griffiths, 2010). Current biodiversity estimates come either from regions which vary from small, well-sampled locations such as King George Island or the South Orkney Islands to areas like the Amundsen Sea, which spans a large area but where no fauna had been collected prior to 2008 (Kaiser et al., 2009). The development of statistical and modeling methods using the to-date knowledge on all known or described Antarctic species are also useful in the development of biodiversity estimates (Gutt et al., 2004). Constraining interactions between physical and biological variables is vital for understanding drivers of Antarctic biodiversity and enabling development of predictive ecological models (Convey et al., 2014). Physical datasets are available across larger scales than biological observations, with broad-scale bathymetric, geomorphological, temperature, sea ice patterns, and more available from observations and satellite data (Post et al., 2014). Thus, physical datasets provide an opportunity to build predictive models of species distributions and diversity if significant relationships can be established. Such models can be significant for guiding Southern Ocean ecosystem management, including the selection and monitoring of Marine Protected Areas (MPAs).
Habitat heterogeneity and substrate characteristics have been found to be important determinants of benthic community composition in the South Orkney Islands, Ross Sea, and East Antarctica (Cummings et al., 2006; Post et al., 2017; Brasier et al., 2018), while in other regions the relationship between the benthos and substrate is less consistent (Gutt et al., 2016). These patterns vary between regions, and there is little if any clear evidence relationships between biodiversity and depth, latitude or environmental parameters that can be applied to benthos across the whole Southern Ocean (Brandt et al., 2007). In addition to developing broad-scale biodiversity models, it is important to consider the significance of fine-scale variability and its impact on biological communities. The complex interplay of different biological and physical factors creates habitat patchiness at a range of scales that significantly enhance biological diversity on the Antarctic shelf on both a local and regional scale (Gutt and Piepenburg, 2003). The impacts of variations in physical determiners are mediated by the biological characteristics and life history traits of the local fauna, including mode of dispersal, growth rate, and functional role (Gutt and Koltun, 1995).
The Commission for the Conservation of Antarctic Marine Living Resources (CCAMLR) is responsible for the management of fisheries and conservation of marine ecosystems throughout the Southern Ocean. Part of CCAMLR's remit is the identification and protection of Vulnerable Marine Ecosystems (VMEs) to protect benthic habitats from the adverse impacts of bottom fishing activities (CCAMLR, 2009a; Parker and Bowden, 2010). To facilitate this, CCAMLR developed a classification of VME indicator organisms (CCAMLR, 2009b), taxa deemed potentially vulnerable to the impacts of bottom fishing. Conservation Measures dictate that when specific thresholds of VME taxa biomass are met, specifically 10 kg of biomass per 1200 m longline, these areas will be defined as “VME Risk Area” and are then to be closed to fishing activities until management decisions can be determined by the Commission (CCAMLR, 2009a). Several previous studies based on the Antarctic shelf and slope have provided data on VME biomass that have been useful for the notification of VME Risk Areas, as week as the development of protected areas within the region (Parker and Bowden, 2010; Lockhart and Hocevar, 2018).
The Prince Gustav Channel (PGC) is located at the north-eastern tip of the Antarctic Peninsula, defined by the Peninsula itself to the west and James Ross Island to the east. The channel extends to the Antarctic Sound and Andersson Island to the north, including Eagle Island and the associated Duse Bay, and reaches Cape Obelisk at the very south. The south region of the channel was covered by the Prince Gustav Ice Shelf, which was more than 15 nautical miles across and included Ross Bay and part of James Ross Island (Cook and Vaughan, 2010). The ice shelf began to collapse in the mid-19th century, and by 1995 the PGC was completely open throughout its length during the austral summer (Cooper, 1997). By these same estimates, the ice shelf still permanently covered the sea at Cape Obelisk as recently as 1989, making this a relatively newly uncovered area of seafloor. The southern part of the channel was included in a proposed Weddell Sea MPA presented to CCAMLR in 2016 (Teschke et al., 2016). CCAMLR has not yet reached an agreement on the proposal.
The goal of the present research was to investigate the benthic community of the PGC and how these relate to aspects of habitat complexity and heterogeneity. Specifically, the aims were to: (1) describe the physical habitat characteristics and overall structure of the benthos at sites across the PGC; (2) investigate how these communities vary across different regions and depths; (3) establish the physical variables that are associated with differences in community structure (4) investigate the abundance and distribution associated with VME taxa. This study represents the first assessment of the benthic community of the PGC. It provides an opportunity to assess the implications for conservation and management for this section of the Weddell Sea and establish a baseline for assessing the impacts of future biological or environmental changes that occur in the region.
Materials and Methods
Data Collection
In 2018, the RSS James Clark Ross expedition JR17003a sampled four sites within the PGC and one site north of the PGC in the vicinity of Andersson Island between 63°30′S and 64°8′S (Figure 1; Table 1). Before deployment of the Shallow Underwater Camera System (SUCS) and Agassiz Trawl (AGT), multibeam bathymetry data were collected to ascertain seafloor topography, slope gradient, and suitability for deployment of these sampling gears. The sampling covered depths between 200 and 1200 m, although not all depths were sampled at each location because of either ice cover or unsuitable seafloor topography (Table 1). The SUCS is a tethered drop-camera system with an HD camera and live feed back to the ship. The system allows the capture of high-resolution images (2448 × 2050 pixels) covering 0.51 m2 of seafloor. It was deployed 12 times across the five locations (Table 1). Each deployment was at a unique depth within that location and consisted of three transects of 10 photos. Each photo within a transect was taken 10 m apart. At the end of each transect the SUCS was moved 100 m to begin the next. The direction of the transects was determined by the wind direction and topography. In some cases, not all the transects could be completed because of icebergs in close vicinity to the vessel or problems with the gear. The AGT was deployed six times across three locations, all of which were also sampled using the SUCS (Table 1). The AGT had a mouth width of 2 m and a mesh size of 1 cm. It was trawled at 1 knot for 2–10 min. These provided reference specimens to help identify species in the SUCS images. Five trawls were identified to the lowest possible taxonomic level and sorted during the cruise while the final trawl was fixed in bulk in 99.8% absolute ethanol and sorted at a later date. The wet weight (biomass) of all different taxa was assessed on board using calibrated scales. Topography and presence of pack-ice meant that not all locations where SUCS sampling was undertaken had a corresponding AGT sample.
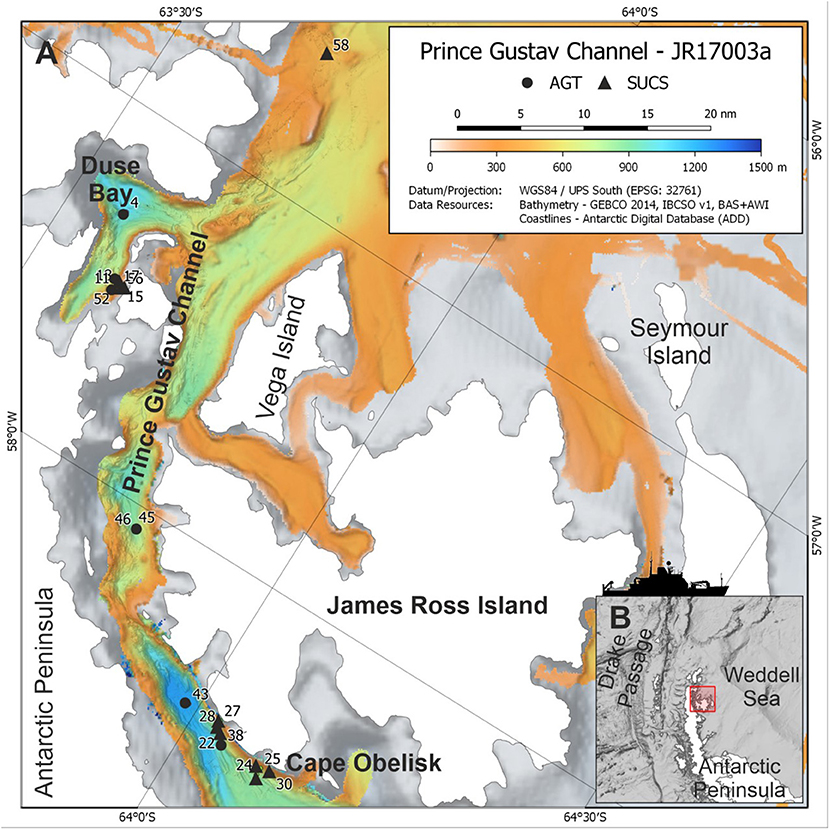
Figure 1. Map of study area. (A) Station locations of SUCS and AGT deployments during JR17003a, including event deployment numbers. (B) Position of the Prince Gustav Channel on the Antarctic Peninsula in the Southern Ocean. Bathymetry data archived from Dreutter et al. (2020).
Image Analysis
All organisms in each SUCS image were identified to the lowest possible taxonomic level, or to morphospecies, dependent on resolution of the images and cross-checked where possible with taxa identified from the AGT trawls at the corresponding location and depth. Some biological material was unidentifiable to phyla but distinguishable as VME species, for example branched or budding fragments which were identifiable as cnidarian or bryozoan species. These individuals were classed as “VME unidentifiable.” Length-weight relationships were used to estimate VME biomass present in the SUCS imagery, derived from measurements of specimens collected from the South Orkney Islands (Brasier et al., 2018). SUCS images were used to investigate the relationships between habitat type and community structure. The percentage cover of different substrate types in each image was recorded. Substrate was classified as either mud, sand, gravel, pebbles, cobbles, boulders (which included dropstones) or bedrock, based on size classifications from Sedimentary Petrology: An Introduction to the Origin of Sedimentary Rocks (Tucker, 1991). Substrate size classifications were adjusted to align with the image size on screen using the known SUCS field of view (0.51 m2). Each image was assigned a texture, based on the dominating substrate type in each image, with mud and sand classified as “Soft” and all others as “Hard.” Biogenic substrate was also determined as percentage cover of each photo. Biogenic substrate refers to any ecosystem engineering organisms that create a three-dimensional structure that contributes to the given habitat. This included organisms such as Porifera, tube forming polychaeta and Ascidians, Cnidaria and Bryozoa.
Data Analysis
Data analysis was performed in RStudio, version 1.3.1056. All organisms observed in the SUCS images and AGT were totalled and converted into proportion of total catch to allow for comparison of proportional catch between gear type. As the sample size was relatively low, no significant statistical analysis was carried out on AGT data. The observable taxa in each image of the SUCS were counted and an ANOVA was used to test the difference among sample sites within the PGC. Diversity measures (Simpson's index and Shannon-Weiner index) were calculated for each photo using the total number of individuals and the total number of different taxa present in any given photo using the Vegan package for R (Oksanen et al., 2011). These measures were chosen as each can be used to infer different information about the community, and both can be viewed as appropriate representations in marine systems (Washington, 1984). While Shannon-Weiner represents a combination of both species richness and equitability in distribution among a sample, Simpson's is weighted toward more abundant species in a sample, thereby giving an impression of species dominance within a system. Estimated VME biomass over 1200 m2 (the area used by CCAMLR to define a VME Risk Area) (CCAMLR, 2009a) was calculated using the known area of the SUCS field of view (0.51 m2), where wet weight over 1200 m2 = (average weight × 1.961) × 1200.
Beta-diversity and the degree to which benthic habitat characteristics were associated with the benthos were analyzed using a combination of correspondence analysis (CA) and canonical correspondence analysis (CCA) using the Vegan package for R. The CA site scores from the ordination represent the similarity between sites. The result is that two sites with similar CA scores have a very similar composition of benthic fauna whereas those with widely different CA scores contain a very different collection of benthic fauna. Any major trend in the first axis represents the degree to which the benthic community turns over along an environmental gradient. The CA scores are used as a measure of beta-diversity. The beta-diversity estimates will be based on the images from the SUCS so will represent the epibenthic fauna that are visible. A structural equational model (SEM) was used to determine the direct and indirect effects of physical variables on beta-diversity. SEMs are a regression-based approach to evaluating causal links between multiple variables in a multivariate system (Lefcheck, 2016). This approach allows variables to function as both predictors and responses within a single model allowing the identification of indirect effects and the testing of specific hypotheses on the interactions between these variables. The SEM was constructed using the R-package piecewiseSEM (Lefcheck, 2016), which allows for the inclusion of non-normally distributed response variables and random effects that account for non-independence in the data. CA1 and CA2 axis scores were used as proxies to represent beta-diversity. The SEM had two indirect pathways, which flow from depth and slope angle to substrate cover and on to CA1 and CA2 scores. Depth and slope angle also have direct pathways to CA1 and CA2, which allow evaluation of whether any depth or slope related change are due to changes in the indirect pathways or due to a different unmeasured variable. A second SEM was used to investigate the indirect and direct effects of slope, depth, and substrate cover on predicted VME biomass. Indirect pathways flowing from depth and slope gradient to biomass were evaluated, as were the corresponding direct pathways. Regression coefficients were standardized using scaling by standard deviation, accomplished using the formula
where β is the coefficient, x is the predictor variable and y is the response variable. Non-significant pathways were removed, and the model Akaike information criterion (AIC) were checked, with the model with the lowest AIC being selected. The model fit of the SEMs were tested by the test of direct separation (Lefcheck, 2016). If the p-value is >0.05, then the model adequately fits the hypothesized causal network with no important paths missing.
Results
Physical Characteristics of the Seafloor
Substrate type and habitat complexity varied across all 12 SUCS deployments sites (Figure 2). Soft, muddy sediments dominated the seafloor at all depths at Duse Bay (95.6%), Cape Obelisk (91.4%), and Andersson Island (96.2%) (Table 2), making the habitat at these sites highly homogenous. An increase in hard substrates was observed at the PGC Mid and PGC South sites where mud only covered an average of 13% of the seafloor. These “hard” sites were instead dominated by a wider variety of harder and coarser sediments, predominantly gravel (38.7%), pebbles (21.8%), and bedrock (11.6%), making these regions more heterogenous in nature. Boulders could be found sporadically across all depths within PGC South, PGC Mid and Cape Obelisk, but were absent from most of Duse Bay and the Andersson Island site. PGC South 200 m had the highest occurrence of boulders, which covered an average of 19.3% of the seafloor. Boulders were also found in small amounts (0.4%) at Duse Bay 200 m. Biogenic substrate could be found at all 12 deployment sites. PGC South 200 m had the highest proportions of biogenic cover (16.7%), while Duse Bay 400 m had the lowest (0.6%). All other sites had biogenic cover within this range. Depth ranges within each transect were generally small (<30 m). Slope gradient remained below 10° at all transects in Duse Bay, PGC Mid, Cape Obelisk, and Andersson Island, with the majority of these transects carried out on a slope <5°. PGC South had much higher slope gradients, peaking at 22° on a transect at 800 m.
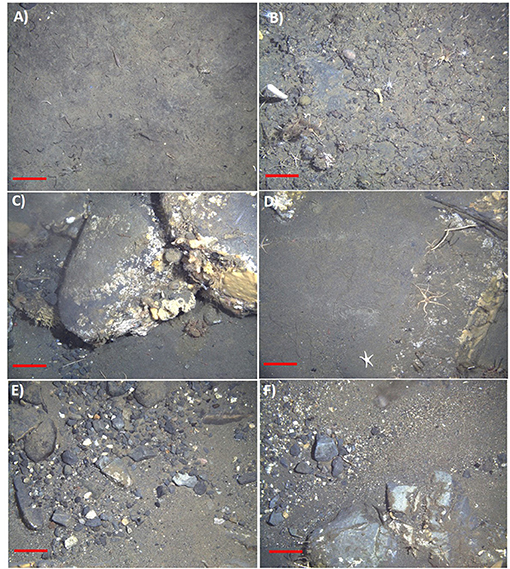
Figure 2. Examples of in-situ SUCS images showing different substrate classifications. (A) Mud dominated, (B) gravel and small pebbles above exposed bedrock, (C) boulders/dropstones, (D) sandy bottom with some exposed boulders, (E) gravel and small pebbles, and (F) mixture of sand, gravel, pebbles, and cobbles. Scale bars represent 10 cm.
Benthic Assemblage
A total of 11,396 individuals from eight known phyla were identified from the SUCS imagery (Table 3). Ophiuroids were the most common taxa by abundance, representing 40.63% of the total number of individuals recorded. Pycnogonids were the next most abundant taxon, representing 15.51% of the total number. The AGTs sampled a total of 5351 individuals, of which 34.57% were ophiuroids and 17.83% were pycnogonids. Relative proportions of taxa between the sampling gears were mostly consistent, although VME taxa such as bryozoans, soft corals, hydrozoans, and poriferans were underrepresented in the AGT catch, while bivalves, holothurians, echinoids, and hemichordates were underrepresented in the SUCS. In total 11 VME taxonomic categories were observed from SUCS imagery and 13 from the AGT. Several VME taxa were absent from the SUCS imagery, including brachiopods, chemosynthetic species (e.g., decapods, bivalves, and tubeworms), acorn barnacles, stalked crinoids, basket stars, and the scallop Adamussium colbecki. Brachiopods and acorn barnacles were present, but rare, in the AGT sample. VME abundance varied significantly between sites (Table 4). The highest abundances of VME taxa were found at PGC South and Mid, with its peak at PGC South 200 m (33.17 ± 12.18), while the lowest abundances were at Duse Bay 400 and 500 m (1.47 ± 1.59 and 1.93 ± 3.86, respectively). VME biomass was also greatest at PGC South, with a combined total of 30.07 kg across the three sampled depths. VME biomass was dominated by Porifera in both the AGT and SUCS samples, accounting for 73.64 and 42.52% of biomass, respectively. For 10 out of the 12 sampled sites, estimated VME biomass over 1200 m2 exceeded the 10 kg threshold set out by CCAMLR to define a VME Risk Area (Table 5).
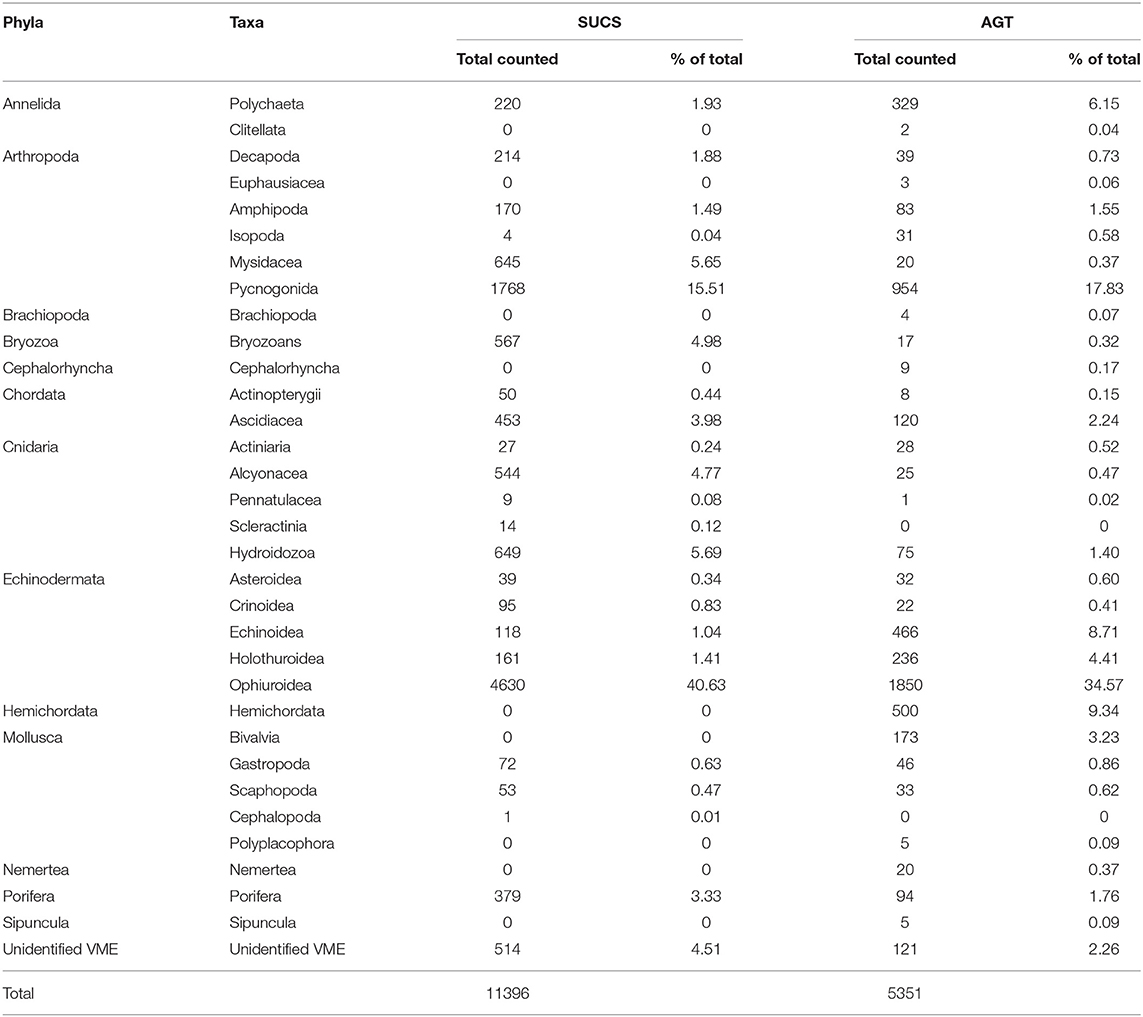
Table 3. All taxa observed and identified from SUCS imagery and AGT, including relative proportion of total observation/catch.
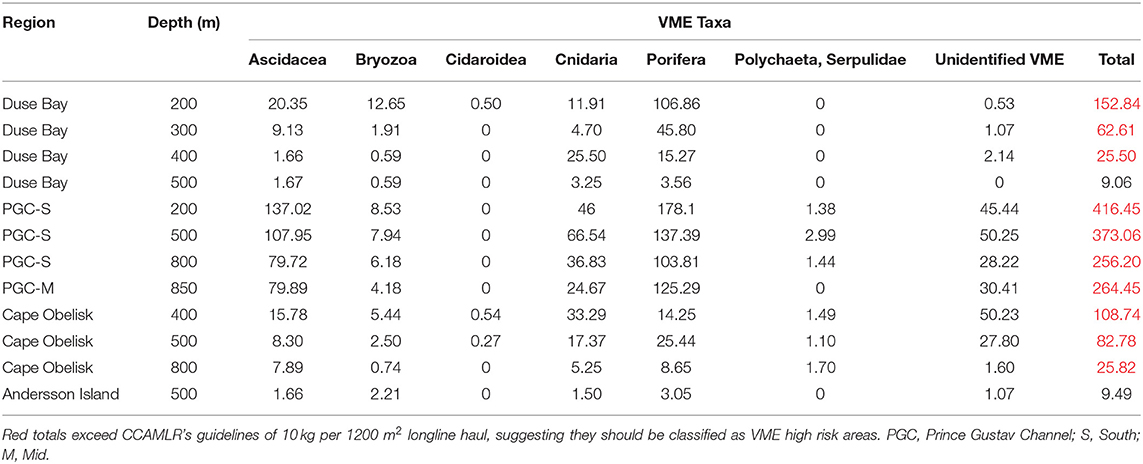
Table 5. Estimated VME taxa wet weight over 1200 m2 (kg) using mean weights determined for each taxa from AGT data per SUCS deployment site.
From the SUCS imagery alone, benthic community structure varied greatly between regions (Figure 3), and there were significant differences in the numbers of organisms observed between deployment sites (Table 4). PGC South had the highest mean numbers of individuals per photo at its three sampled depths (65.32 ± 19.22, 58.97 ± 17.39, and 56.38 ± 17.39 at 800, 500, and 200 m, respectively). The PGC Mid 850 m site contained an average of 39.93 ± 9.66 individuals per photo, while all other regions had <30. The lowest average abundances were found at Duse Bay 400 m (13.93 ± 4.67) and Andersson Island 500 m (18.53 ± 5.52). On average hard substrates were inhabited by nearly double the number of taxa (11.31 ± 3.78 compared to 6.38 ± 2.8) and more than double the number of individuals (53.63 ± 24.77 compared to 21.03 ± 12.55) as soft substrates. Diversity as measured by Shannon-Weiner index was significantly greater on hard substrata, while there was no significance in Simpson's diversity between textures (Figure 4). This suggests that diversity in the sense of species richness and evenness of those species was greater on hard habitats, there was little change in the dominance of relative taxa between the two.
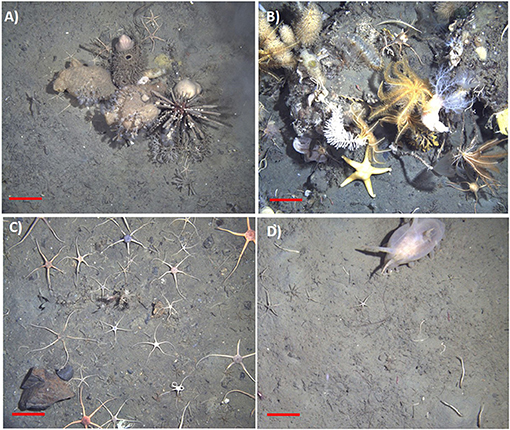
Figure 3. Examples of in-situ SUCS images of common benthic communities and taxa. (A) Biogenic habitat forming taxa including poriferan species and the pencil spine urchin cidaroid, (B) species associated with hard substrata including crinoid species, and suspension feeding dendrochirotid holothurians, cnidarians, and annelids, (C) ophiuroid dominated community (cf. Ophionotus victoriae), and (D) deposit feeding holothurian Protelpidia murrayi in a mud dominated area. Scale bars represent 10 cm.
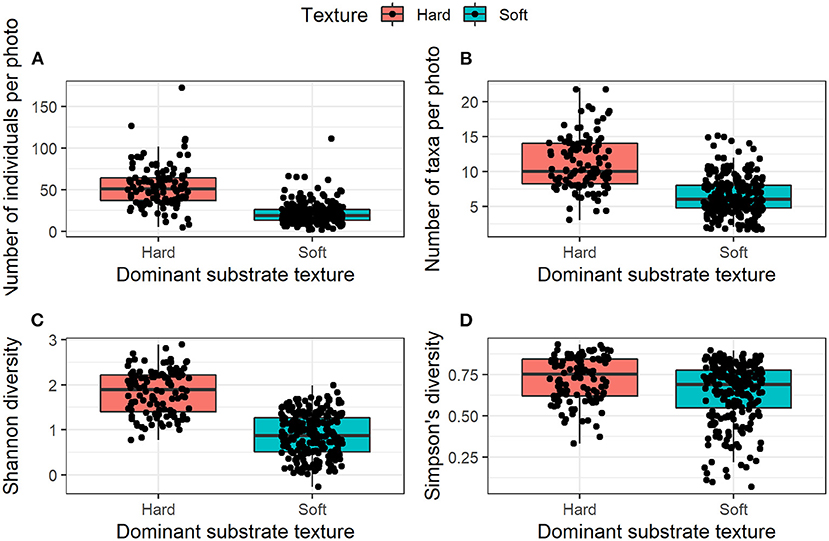
Figure 4. Mean number of individuals and taxa present per SUCS image and mean diversity indices per photo according to substrate texture. (A) Mean number of individuals per image, (B) Mean number of taxa per image, (C) Mean diversity (Shannon-Weiner Index), and (D) Mean diversity (Simpson's Index).
Region and substrate texture appeared to have a greater role in determining benthic community composition along CA1 than depth while CA2 appeared to be more related to depth (Figure 5). The first two axes of the CA explained 45% of the observed variation (CA1 = 36% and CA2 = 9%). The Andersson Island and Duse Bay communities show greater variation along CA1 compared to the other regions. The majority of the CA1 scores for Andersson Island and Duse Bay were negative. Cape Obelisk, PGC South, and PGC Mid all had positive CA1 scores except for only a few samples (Figure 5). Substrate texture also appeared to be important along CA1 as the hard substrate scores were all positive, apart from four images, while the soft substrate sites were distributed along the entire CA1 axis. The variability in CA2 scores increased with increasing CA1 scores. The majority of CA2 scores for Cape Obelisk were positive except for a few samples. This was the opposite for PGC South and PGC Mid. There appeared to be some relationship between CA2 scores and depth, albeit weak. The majority of samples taken at 200 and 850 m had negative CA2 scores, while those sampled at 400 and 800 m had mainly positive CA2 scores.
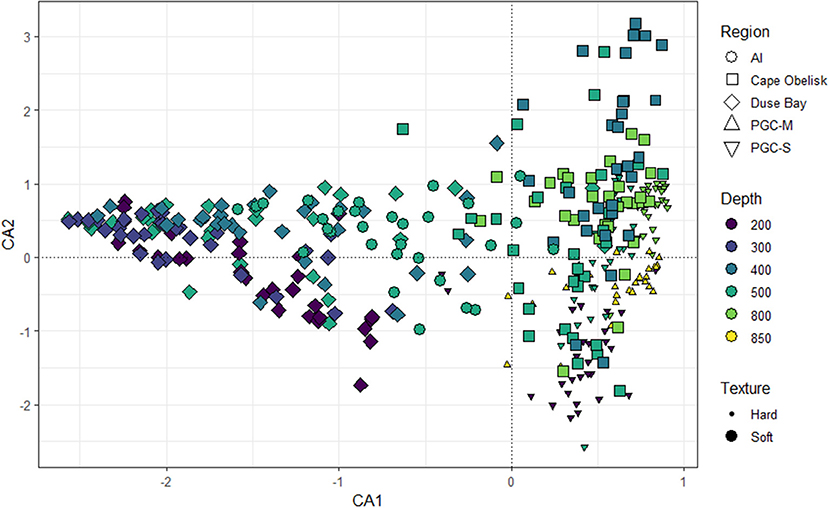
Figure 5. Correspondence Analysis (CA) of benthic community structure according to region, depth, and substrate texture. CA1 and CA2 axis together explain 45% of observed variation.
Substrate cover, slope angle, and depth together accounted for 52% of variation observed in the CCA which was related to benthic community composition of the SUCS images (Figure 6). High variance inflation factors (>10) were observed for slope gradient, cobbles, and bedrock. The CCA sites scores indicated that gravel, boulders, and bedrock and slope were all positively related to the benthos at PGC South whilst negatively related to Duse Bay and PGC Mid. Mud was an important determinant for Duse Bay and Cape Obelisk benthic communities which were further separated by depth (Figure 6A). The CCA species scores revealed that suspension feeding holothurians and crinoids were strongly associated with an increase in slope gradient and hard substrates such as cobbles, boulders and gravel, while suspension feeding annelids, anthozoans, bryozoans, porifera, hydrozoans, and ascidians were more closely associated with bedrock (Figure 6B). Deposit feeding annelids, holothurians, and ophiuroids were associated with mud. Abundance varied significantly for 11 of the 18 identified higher classifications that were tested (Table 4). All 11 saw their peak in abundance within the PGC South region. For Anthozoa, Ascidiacea, Bryozoa, Echinoidea, Hydroidilina, Porifera, and Scaphopoda this was at 200 m, although for the latter this may not be meaningful as Scaphopoda are infaunal so will rarely be identified using SUCS imagery. Crinoidea and Holothuridea were highest in abundance at 500 m, while Pycnogonida were highest in abundance at 800 m. Pycnogonida were also characterized by a very patchy abundance, reaching their highest abundance at an average of 23.04 ± 8.99 per photo, while at most sites this remained below five. Crinoidea and Echinoidea were also patchy in abundance, being among the few groups to not occur at every site.
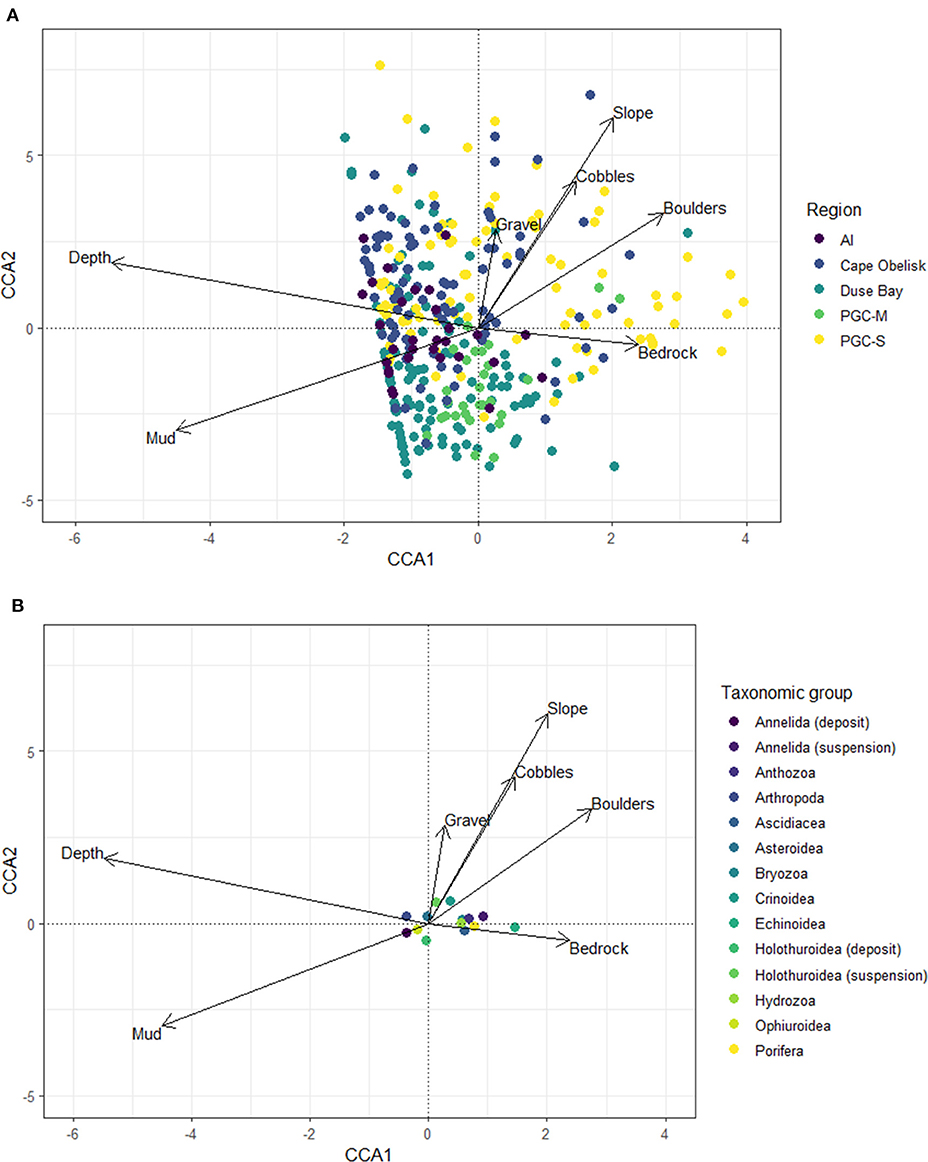
Figure 6. Canonical Correspondence Analysis (CCA) of community assemblage and environmental variables including substrate cover, depth, and seafloor slope gradient. Organisms are grouped into higher taxonomic classifications and functional groups were necessary. (A) Site scores. (B) Taxon scores.
The SEM investigating the effects of depth, gradient and substrate on CA1 and CA2 score was found to represent the data well (Fisher C = 4.49, p = 0.098, df = 9). There was a significant indirect pathway between slope gradient and CA1 score, mediated through its direct influence on substrate cover (Figure 7; Table 6). The relationship between gradient and hard substrate cover was positive while the relationship between gradient and soft substrate cover was negative. Hard substrate cover had a positive influence on CA1 score, while soft cover had a negative one. This suggests that the CA1 scores represent a gradient of soft to hard substrate that is influencing community structure, while depth does play a smaller yet still significant role. Contrary to what was expected, a significant direct association between seafloor gradient and CA2 score was found. This suggests seafloor gradient is influencing community structure via a different unmeasured variable. Depth had no association with abiotic substrate cover. Depth did have a positive direct influence on CA1 and a greater influence on CA2 but was negatively related to biogenic substrate cover. The cover of biogenic substrate had a direct negative influence on CA2. The CA2 scores likely represent a gradient of biogenic cover and depth. This suggests that some taxa are potentially physiologically depth limited, or an unmeasured variable is mediating this process. The SEM investigating the direct and indirect effects of slope gradient, depth, and substrate cover on VME biomass was a good fit for the data (Fisher C = 6.51, P = 0.072, df = 6). Seafloor gradient had a significant indirect effect on biomass, mediated through its influence on substrate cover (Figure 8; Table 6). Gradient had a negative relationship with soft substrate, while soft substrate had a negative relationship with biomass. The reverse was true of the relationship between gradient and hard substrate and between hard substrate and biomass. Cumulatively, seafloor gradient therefore has a strong, positive relationship with predicted biomass. Contrary to the predicted outcomes, depth had a direct, negative influence on biomass. This suggests that some VME taxa observed are either physiologically depth-limited or influenced by an unmeasured variable that is itself mediated by depth. It is also possible the full depth range within the channel has not been adequately sampled to properly identify depth trends.
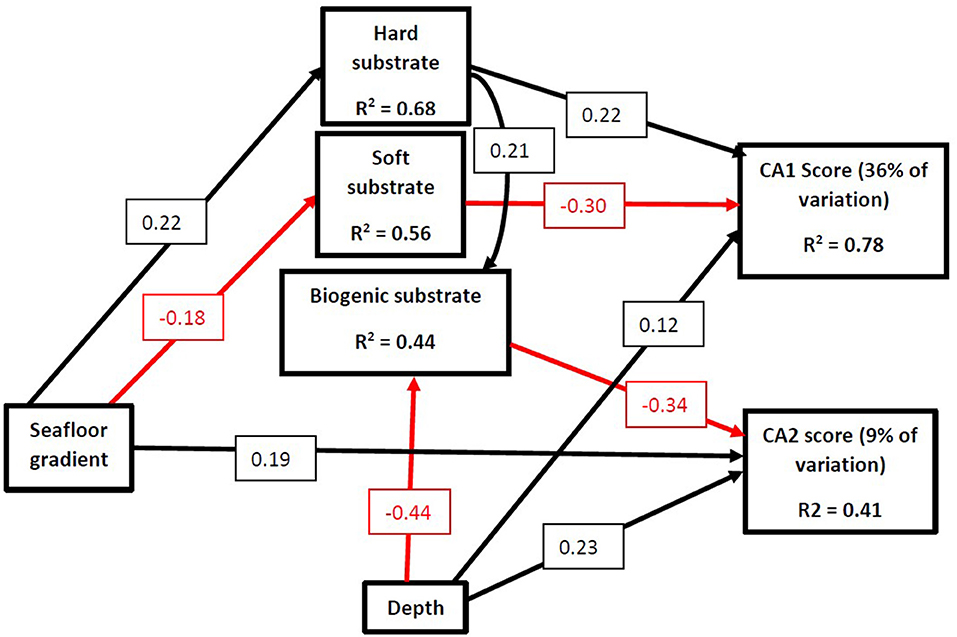
Figure 7. Structural equational model (SEM) exploring the relationships between seafloor gradient, depth, substrate cover, and community structure as measured by CA1 and CA2 axis scores. Arrows represent unidirectional relationships among variables, with black arrows denoting positive relationships and red arrows depicting negative ones. Only significant pathways (P ≤ 0.05) are displayed. The standardized regression coefficient is given in the associated box. R2s for component models are given in the boxes of the response variable. Model incorporates random effect of Region. Hard substrate represents the combined cover of gravel, cobbles, pebbles, boulders, and bedrock. Soft substrate represents the combined cover of sand and mud.
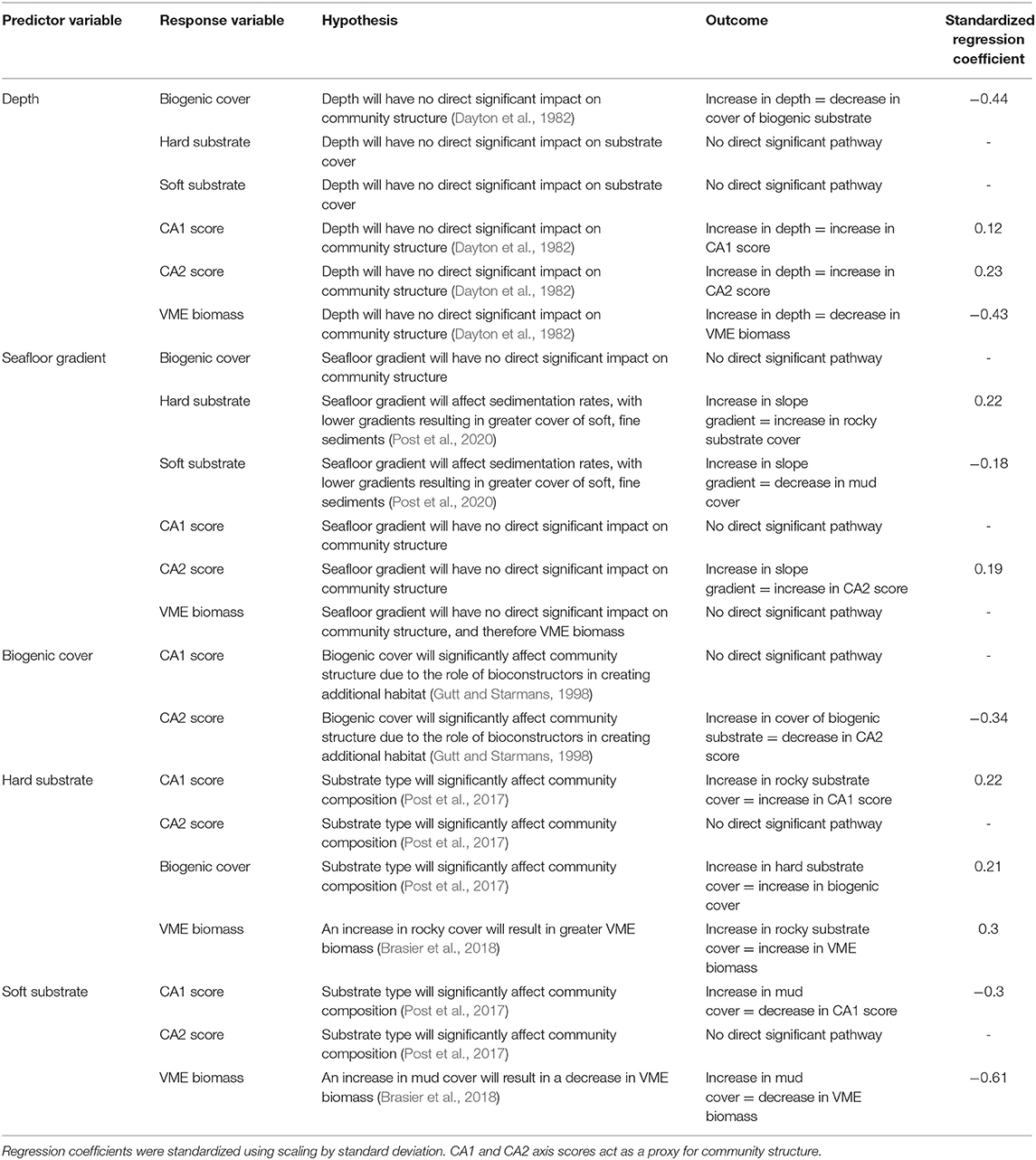
Table 6. Summary of all direct pathways investigated using structural equation models and the hypothesis underpinning the expected relationship between predictor and response variables.
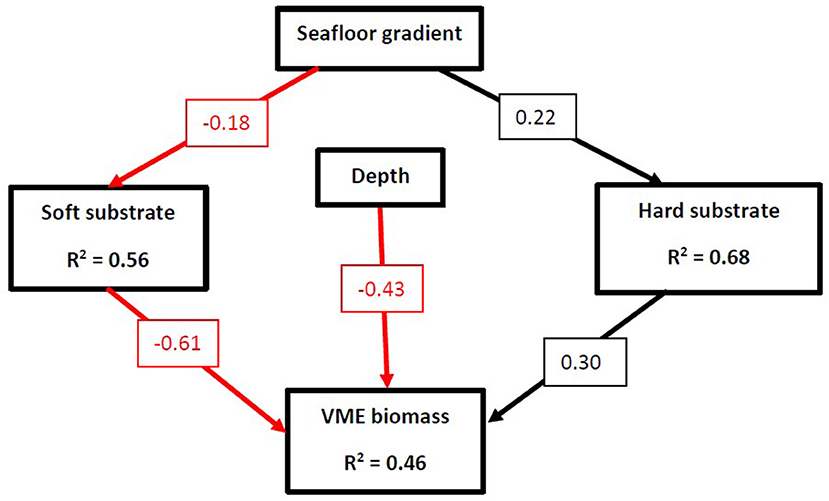
Figure 8. Structural equational model (SEM) exploring the relationships between slope angle, depth, substrate cover, and estimated VME biomass. Arrows represent unidirectional relationships among variables, with black arrows denoting positive relationships and red arrows depicting negative ones. Only significant pathways (P ≤ 0.05) are displayed. The standardized regression coefficient is given in the associated box. R2s for component models are given in the boxes of the response variable. Model incorporates random effect of Region. Hard substrate represents the combined cover of gravel, cobbles, pebbles, boulders, and bedrock. Soft substrate represents the combined cover of sand and mud.
Discussion
Our work found that benthic faunal composition was heavily influenced by the substrate type available, with greater abundances and diversity associated with hard, rocky substrate. This was often associated with the physiology and functional group of different taxa. Depth and seafloor gradient had direct and indirect effects on community structure, often mediated through their influence on habitat forming organisms and substrate type. High numbers of VME taxa were identified, and VME biomass was strongly associated with hard substrate and shallower depths.
Benthic Assemblages and Physical Variables
The influence of physical environmental variables such as substrate type, substrate texture, and depth have frequently been associated with the distribution and structure of benthic communities around the Antarctic continental shelf and slope. Substrate texture and type in particular have been found to play significant roles in structuring epifaunal communities in other regions around the Antarctic Peninsula, including the South Orkney Islands and King George Island (Quartino et al., 2001; Brasier et al., 2018). Similar results have been found in east Antarctica (Post et al., 2011) and in the Ross Sea, where substrate type and composition explained 66% of the variation observed in shallow water macrofaunal communities (Cummings et al., 2006). Coarse substrates are preferential for most filter or suspension feeders, as it often provides hard surface for attachment and an elevated position which enhances the capture success rate of these functional groups (Muschenheim, 1987). Conversely, finer, siltier sediments provide a food resource for both facultative and obligate deposit feeders (Gutt, 1990). This is reflected in the PGC, where deposit feeding holothurians and annelids were strongly associated with muddy sediments. Although, it is likely that the diversity and abundance of these taxonomic groups will be underestimated because the analysis is based on images of the benthic communities rather than a combination of infaunal and epifaunal sampling. Suspension feeders such as some cnidarians and crinoids were associated with rocky habitats. As in this present study, abundance, biomass, and taxonomic epifaunal diversity are often found to be greater in areas of coarse, hard substrates than in areas of finer sediments. This was the case in east Antarctica, where boulders and cobbles were associated with significant increases in faunal abundance (Post et al., 2017) and in deeper communities (c. 1000 m) in the Weddell Sea (Jones et al., 2007).
It has been suggested that differences in diversity could be related to the abundance of suspension feeding taxa that prefer hard substrates being highly diverse in Antarctic waters (Gutt and Starmans, 1998). The resolution of taxonomic data available from SUCS imagery prevents analysis of the exact number of epifaunal species present, but greater diversity amongst hard substrates of deep water corals has been found off the Antarctic Peninsula when identified to genus level (Roberts and Hirshfield, 2004). In the PGC, those areas dominated by harder substrates were also characterized by increased habitat heterogeneity. The higher diversity observed at these sites may therefore be a result of fauna characteristic of both coarse and fine sediment being able to occupy the same space, as more ecological niches and functional groups are provided for. The association of sessile invertebrates and hard substrates likely has a cumulative effect on the diversity of these assemblages. High diversity and abundance of taxa found on hard substrates is enhanced by the three-dimensional surface created by the organisms themselves, particularly Porifera, some anthozoans, and bryozoans, which form complex habitats for other invertebrates. This is reflected in the SEM which suggests biogenic cover played a significant role in determining overall community structure. Other studies have noted the positive correlations between overall abundance and diversity and the presence of ecosystem engineers such as large sponges, gorgonians, and bryozoans (Gutt and Shickan, 1998; Gutt and Starmans, 1998). It is also possible that limitations inherent in the SUCS imagery means biodiversity in soft sediment areas cannot be reliably estimated. Epifauna that periodically burrow and infauna will be underestimated or not observed using SUCS imagery. This may explain the observed underrepresentation of bivalves, hemichordates, echinoids, and holothurians in the SUCS data compared to the AGT data. Previous studies have noted higher than expected levels of diversity among infaunal communities in soft sediment areas, including in the South Shetland Islands and the Antarctic shelf in general (Gallardo, 1987; Lovell and Trego, 2003). It is possible that this may be the case in the PGC. However, this cannot be confirmed in the present study.
Community composition was influenced by depth and seafloor gradient in the PGC, albeit not as significantly as substrate type and often through indirect effects. Gutt and Starmans (1998) found that some variation in the benthos could be explained by a combination of these two factors. While several studies have highlighted the role of depth in determining benthic composition (Post et al., 2017; Neal et al., 2018), others suggest that the influence of depth is either non-existent or limited to indirectly effecting the benthos through its mediating impact on other physical variables (Brandt et al., 2007; Jones et al., 2007). After the mass-wasting of benthic communities caused during the Cenozoic glacial period, it is thought that the continental shelf was predominantly recolonised by deep-water organisms with wide bathymetric tolerances (Thatje et al., 2005). This legacy is still evident today, and as a result depth is typically regarded as less important in controlling species distributions than in many other areas (Dayton et al., 1982). Thus, any direct change in abundance and diversity with depth is likely related to a reduction in organic matter available to the benthos (Lampitt et al., 2001). The influence of seafloor gradient on the benthos has not been as thoroughly studied, although in this present study its influence was limited to its effect on substrate cover. Flatter gradients result in greater sedimentation accumulation and the dominance of fine sediments (Post et al., 2020), as evidenced in the present study through the high inflation factors between gradient and some hard substrates, which will in turn influence both the texture of the habitat and the accumulation of particulate matter available for deposit feeders.
The relative influence of physical environmental variables depends on the scale of investigation. Previous studies of the Antarctic shelf have highlighted the problems associated with using large-scale, regional patterns to predict benthic abundance and distribution. Variations in habitat and substrate on the local, often sub-meter, scale have significant impacts on overall diversity (Cummings et al., 2006; Post et al., 2017). Studies that consider variations in the small-scale also often result in stronger distinctions between assemblages than those that use larger geomorphic units (Douglass et al., 2014; Brasier et al., 2018). Gutt et al. (2012) argue that broad patterns in environmental variables can be used to explain variation at larger scales (>2 km), whereas fewer variables explain variation at finer scales. These variations are likely explained by non-measured variables as well as biological interactions and traits. The influence of small-scale heterogeneity in determining community composition, particularly in areas dominated by muddy sediments, is evident in this study. Dropstones in particular are a noted small-scale physical feature that act as important habitats and enhance diversity among sessile invertebrates in both the Antarctic and Arctic (Thrush et al., 2010). It has been suggested that dropstones act as important stepping stones for the dispersal and connectivity of communities that rely on hard substrates (Post et al., 2017), and given that most of the Antarctic shelf is dominated by muddy sediments (Smith et al., 2006), their potential importance for the overall biodiversity of a system cannot be underestimated. A higher level of connectivity between hard substrate-preferring taxa may also be reflected in the PGC data, evidenced by the high levels of similarity between the communities found at PGC South and Mid, especially when compared to the soft sites of Duse Bay and Cape Obelisk, which despite sharing similar sediment characteristics supported different communities. This suggests small scale heterogeneity is particularly important in muddy, soft sedimented habitats where local variation can greatly impact benthic abundance and diversity.
Changes in annual and seasonal sea ice cover must be considered when investigating benthic community structure. In the eastern Weddell Sea, benthic abundance, and biomass decreased by up to two thirds and composition shifted from suspension to deposit feeders as a result of sea ice increases between 1988 and 2014 (Pineda-Metz et al., 2020). In the present study, the communities found occupying all depths at Cape Obelisk were distinctly unique from the communities found elsewhere in the PGC. It is possible that the communities here are still experiencing the legacy of the Prince Gustav ice shelf, which permanently covered Cape Obelisk as recently as 1989 (Cooper, 1997). Fauna characteristic of a system that has undergone change from an oligotrophic sub-ice shelf ecosystem to a productive shelf ecosystem are often unique to these systems, and it has been suggested that some benthic communities can take 150–200 years to reach complete recolonization following exposure to the sea surface (Gutt et al., 2010). These fauna can vary from pioneer organisms such as demosponges and juvenile cnidaria to taxa more typical of advanced stages of recolonization such as sponges, compound ascidians, and unique bryozoans. While higher numbers of ascidians and porifera were found at Cape Obelisk compared to its muddy counterparts, resolution of the SUCS imagery and the lack of AGT data from this region prevents analysis down to species level.
The range and distribution patterns of different taxa are highly dependent on both life history and evolutionary history (Barnes and Griffiths, 2007; Convey et al., 2014). Some groups, such as pycnogonids, show a global hotspot of biodiversity within the Southern Ocean, and when examined on a regional scale have distinct local hotspots (Griffiths et al., 2011). This was observed at PGC South 800 m, where their abundance reached a significant peak. Polychaetes, similar to pycnogonids, are represented at higher than average levels in Antarctica (Barnes and Peck, 2008). At the South Shetlands Islands, polychaetes were found to compose up to 61% of the total macrobenthic abundance (Gutt and Starmans, 1998). Polychaetes were also among the predominating organisms at King George Island (Arnaud et al., 1986), and despite also being located around the Antarctic Peninsula, these patterns were not reflected in the PGC, where polychaete abundance remained relatively low throughout. However, this is reflective of the individuals observed on the seafloor and infaunal samples may provide a different perspective. Ophiuroids were the most dominant taxa found across all sites and among both substrate types. This has been observed around much of the Antarctic shelf. In east Antarctica, ophiuroids represent high proportions of overall observed taxa, sometimes up to 50% (Post et al., 2017), although the proportional abundance of ophiuroids is greater in this current study than in others that have occurred around the Peninsula (Grange and Smith, 2013; Brasier et al., 2018). This dominance is unsurprising, as ophiuroids, as well as other echinoderms such as echinoids, are generally considered ubiquitous in the Southern Ocean and can thrive across large depth ranges and on both hard and soft substrate (Thrush et al., 2006). Overall abundance per m2 was greater in the PGC than in many similar studies around the Antarctic shelf and continental slope (Table 7), including the Antarctic Peninsula shelf and fjords (Sumida et al., 2008; Grange and Smith, 2013). Abundance was however lower than in the Weddell Sea shelf (Gutt and Starmans, 1998). It is likely that the full extent of benthic distribution and diversity cannot be assessed completely. More AGTs would be necessary so that the sample size is meaningful enough to carry out more robust analysis on the catch data. The SUCS also has some inherent limitations. The SUCS is a downward facing camera, meaning it can only land on flat surfaces. This prevents certain regions such as steep slopes, boulders, and canyons from being studied due to their topography. These habitats are often considered diversity hotspots (Robert et al., 2015; Fernandez-Arcaya et al., 2017), meaning these potentially diverse regions are difficult to sample.
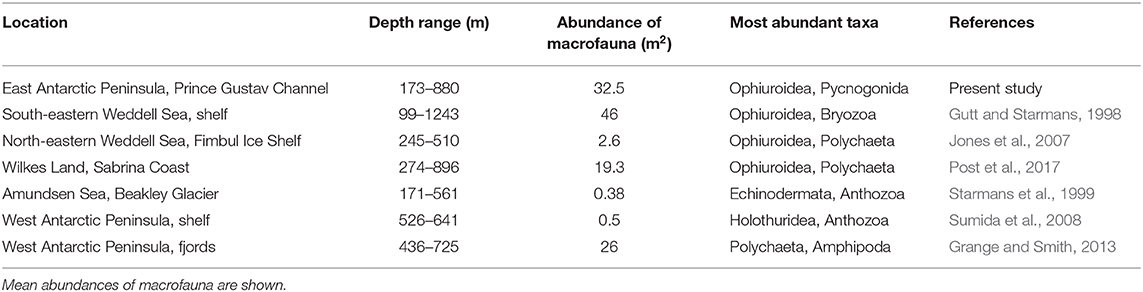
Table 7. Comparison of abundance and key taxa based on photographic studies at comparative depths on Antarctica's continental shelf.
VME Taxa
The relationship between benthic community composition and environmental characteristics is complex with many variables contributing to differences in community composition and the spatial structure of biodiversity (Convey et al., 2014). Given the current international effort to establish a representative system of MPAs in the Southern Ocean it is important to continue to investigate the relationship between both broad and local scale physical surrogates that could be used to infer high levels of biodiversity, VME locations, and potential MPA sites.
Abundances of VME taxa were greatest within the PGC South sites, and VME biomass was characterized by an increase association with rocky cover and a decrease with mud cover. This is unsurprising because of the high number of hard substrate-preferring suspension and filter feeders that are classified as VME taxa (CCAMLR, 2009b). VMEs have been found in lower abundances on soft sediment compared to hard elsewhere around the Antarctic Peninsula (Lockhart and Jones, 2008; Brasier et al., 2018). Depth had a negative influence on VME biomass, which is likely related to organic flux and food availability due to the functional nature of many VME taxa (Jansen et al., 2018). Slope gradient played an indirect role in influencing biomass, mediated through its effect on substrate cover. While the SEM presented in the present study may be useful in predicting biomass in specific regions, the overall impact of variables on VME taxa will likely be highly area specific (Parker and Bowden, 2010). More data are required on the relationship between seafloor gradient and VME taxa in order to check the validity of this model for other regions of the Antarctic shelf and continental slope.
VME Risk Area thresholds are projected to have been met in all sites with the exceptions of Andersson Island and Duse Bay 500 m. The projected wet weights over 1200 m2 in the current study may have implications for the development of future conservation measures across the Antarctic Peninsula as a whole and the PGC in particular. Several previous studies have provided evidence of VME thresholds being met in the northwestern Antarctic Peninsula region (Lockhart and Jones, 2008; Parker and Bowden, 2010), which have led to the notification and designation of new VME Risk Areas by CCAMLR. As in the present study, VME biomass is often driven by porifera, and in some cases the wet weight of porifera alone has been enough to exceed thresholds and make areas high risk (Brasier et al., 2018). This threshold may therefore be severely biased toward “heavy” taxa, namely porifera, such that communities of lighter taxa stand little to no chance of meeting these criteria and being adequately protected. This concern is reflected in the present study, as the two sites that do not meet this threshold are also characterized by disproportionately lower porifera biomass. It is possible that VME abundance or diversity, rather than biomass, may be a better way to define this threshold for certain taxa.
CCAMLR defined its VME thresholds on the basis of using actual longline haul data rather than imagery. In previous studies seafloor imagery has provided direct evidence of the presence of VME taxa where there was insufficient or no actual catch data (Jones and Lockhart, 2011; Lockhart and Hocevar, 2018). In the present study, various VME taxa such as bryozoans, gorgonians, porifera, and hydrozoans were underrepresented in the AGT sample. Similar results were found in Brasier et al. (2018), who found an AGT hauled lower biomass and abundance than predicted by SUCS imagery within the South Orkney region. This does suggest that some of the present study sites may not contain enough biomass to exceed the threshold on longline hauling alone. Trawls and longlines are highly destructive compared to image-based types of analysis, and often are not needed, especially when sampling in recently disturbed or vulnerable areas (Bowden, 2005). A switch from longline bycatch based to camera-based evidence, and instead focusing on species richness, diversity or abundance, may both reduce damage to the habitat and reduce bias toward organisms with greater biomass. Additionally, all sites within the proposed Weddell Sea MPA (Teschke et al., 2016), that is, all sites south of 64°S, exceeded the projected 10 kg of VME taxa per 1200 m2. This includes all SUCS deployments in PGC South and Cape Obelisk. This additional information on VME taxa in the region could be used to further support the case for a Weddell Sea MPA.
Conclusions
Benthic assemblages in the PGC were dominated across all regions by ophiuroids, regardless of depth or substrate texture. Abundance and diversity were greater on hard substrata, and the cover of rocky substrate played a significant role in increasing VME biomass. Overall average abundance of organisms across the PGC was greater than in many other studies on the Antarctic shelf, including other locations around the Antarctic Peninsula. The legacy of the Prince Gustav ice shelf may be still be reflected in the epibenthic community, although higher taxonomic resolution would be required to confirm this. Seafloor gradient and depth played significant roles in determining epibenthic community composition and VME biomass. Although more research and focused sampling of softer sediments is required to assess the infauna contribution to the regional diversity. It is likely that variables such as food availability, organic flux, and biological interactions are important in determining the structure of the benthic community, but these factors are beyond the scope of this present study. The PGC hosts large amounts of VME taxa, and further study of the region may be crucial in determining the future management strategy of not just the eastern Antarctic Peninsula region but potentially the wider Weddell Sea.
Data Availability Statement
The datasets presented in this study can be found in online repositories. The images used in the analysis have been deposited with the UK Polar Data Centre (Linse et al., 2020).
Ethics Statement
Ethical review and approval was not required for the animal study because ethical approval for this study was undertaken through the Preliminary Environmental Assessment by British Antarctic Survey. No experimentation or animal husbandry was planned, which required ethical approval. The study was designed to collect invertebrate macrofauna not vertebrate animals or cephalopod. All fish (n = 8) were killed following appropriate guidelines.
Author Contributions
PA, KL, and WR developed and designed the study. KL, MM, RW, SG, and WR collected the samples. PA, MM, KL, and HG identified habitats and the specimens to highest possible taxonomic level. HG provided environmental data and helped with the statistical analyses. SD provided bathymetry data and site maps. PA prepared the figures and tables, performed the statistical analyses, and drafted the original manuscript which was critically revised and improved by KL, WR, HG, SG, SD, RW, and MM. All authors contributed to the article and approved the submitted version.
Funding
PA and WR are funded by Newcastle University. KL, HG, SG, and RW are part of the British Antarctic Survey Polar Science for Planet Earth Programme funded by The Natural Environment Research Council (NERC) [NC-Science]. The RSS James Clark Ross expedition JR17003a and participation of KL, WR, RW, MM, and SG were funded by the NERC urgency grant NE/R012296/1. MM was also funded by Museums Victoria.
Conflict of Interest
The authors declare that the research was conducted in the absence of any commercial or financial relationships that could be construed as a potential conflict of interest.
Acknowledgments
We are grateful to the master and crew of RRS James Clark Ross, and the AME staff at BAS for tremendous logistic and shipboard support during the fieldwork in the Southern Ocean. We thank our colleagues on board of JR17003a for their company during hours of SUCS deployments. The fieldwork in the western Weddell Sea during JR17003a was undertaken under the permit No. 43/2017 issued by the Foreign and Commonwealth Office, London to section 3 of the Antarctic Act 1994.
References
Arnaud, P. M., Jazdzewski, K., Presler, P., and Sicinski, J. (1986). Preliminary survey of benthic invertebrates collected by Polish Antarctic Expeditions in Admiralty Bay (King George Island, South Shetland Islands, Antarctica). Pol. Polar Res. 7, 7–24.
Barnes, D. K. A., and Griffiths, H. J. (2007). Biodiversity and biogeography of southern temperate and polar bryozoans. Global Ecol. Biogeogr. 17, 84–99. doi: 10.1111/j.1466-8238.2007.00342.x
Barnes, D. K. A., and Peck, L. S. (2008). Vulnerability of Antarctic shelf biodiversity to predicted regional warming. Clim. Res. 37, 149–163. doi: 10.3354/cr00760
Bowden, D. A. (2005). Quantitative characterization of shallow marine benthic assemblages at Ryder Bay, Adelaide Island, Antarctica. Mar. Biol. 146, 1235–1249. doi: 10.1007/s00227-004-1526-0
Brandt, A., Gooday, A. J., Brandão, S. N., Brix, S., Brökeland, W., Cedhagen, T., et al. (2007). First insights into the biodiversity and biogeography of the Southern Ocean deep sea. Nature 447, 307–311. doi: 10.1038/nature05827
Brasier, M. J., Grant, S. M., Trathan, P. N., Allcock, L., Ashford, O., Blagbrough, H., et al. (2018). Benthic biodiversity in the South Orkney Islands Southern Shelf Marine Protected Area. Biodiversity 19, 5–19. doi: 10.1080/14888386.2018.1468821
CCAMLR (2009a). Conservation Measure 22-07. Interim Measure for Bottom Fishing Activities Subject to Conservation Measure 22-06 Encountering Potential Vulnerable Marine Ecosystems in the Convention Areas. Schedule of Conservation Measure in Force 2017/18. Hobart, TAS: CCAMLR.
Convey, P., Chown, S. L., Clarke, A., Barnes, D. K. A., Bokhorst, S., Cummings, V., et al. (2014). The spatial structure of Antarctic biodiversity. Ecol. Monogr. 84, 203–204. doi: 10.1890/12-2216.1
Cook, A. J., and Vaughan, D. G. (2010). Overview of areal changes of the ice shelves on the Antarctic Peninsula over the past 50 years. Cryosphere 4, 77–98. doi: 10.5194/tc-4-77-2010
Cooper, A. P. R. (1997). Historical observation of Prince Gustav ice shelf. Polar Rec. 33, 285–294. doi: 10.1017/S0032247400025389
Cummings, V., Thrush, S., Norkko, A., Andrew, N., Hewitt, J., Funnell, G., et al. (2006). Accounting for local scale variability in the benthos: implications for future assessments of latitudinal trends in the coastal Ross Sea. Antarct. Sci. 18, 633–644. doi: 10.1017/S0954102006000666
Dayton, P. K., Newman, W. A., and Oliver, J. (1982). The vertical zonation of the deep-sea Antarctic acorn barnacle, Bathylasma corolliforme (Hoek): experimental transplants from the shelf into shallow water. J. Biogeogr. 9, 95–109. doi: 10.2307/2844695
Douglass, L. L., Turner, J., Grantham, H. S., Kaiser, S., Constable, A., Nicoll, R., et al. (2014). A hierarchal classification of benthic biodiversity and assessment of protected areas in the Southern Ocean. PLoS ONE 7:e10551. doi: 10.1371/journal.pone.0100551
Dreutter, S., Dorschel, B., and Linse, K. (2020). Swath sonar bathymetry data of RRS JAMES CLARK ROSS during cruise JR17003a with links to multibeam raw data, EM122. PANAGAEA.
Fernandez-Arcaya, U., Ramirez-Llodra, E., Aguzzi, J., Allcock, A. L., Davies, J. S., Dissanayake, A., et al. (2017). Ecological role of submarine canyons and need for canyon conservation: a review. Front. Mar. Sci. 4:00005. doi: 10.3389/fmars.2017.00005
Gallardo, V. A. (1987). The sublittoral macrofaunal benthos of the Antarctic shelf. Environ. Int. 13, 71–81. doi: 10.1016/0160-4120(87)90045-6
Grange, L. J., and Smith, C. R. (2013). Megafaunal communities in rapidly warming fjords along the west Antarctic Peninsula: hotspots of abundance and beta diversity. PLoS ONE 8:e77917. doi: 10.1371/journal.pone.0077917
Griffiths, H. J. (2010). Antarctic marine biodiversity–what do we know about the distribution of life in the Southern Ocean? PLoS ONE 5:e11683. doi: 10.1371/journal.pone.0011683
Griffiths, H. J., Arango, C. P., Munilla, T., and Mcinnes, S. J. (2011). Biodiversity and biogeography of Southern Ocean pycnogonids. Ecography 34, 616–627. doi: 10.1111/j.1600-0587.2010.06612.x
Gutt, J. (1990). New Antarctic holothurians (Echinodermata)–II. Four species of the orders Aspidochirotida, Elasipodida, and Apodida. Zool. Scr. 19, 119–127. doi: 10.1111/j.1463-6409.1990.tb00244.x
Gutt, J., Barratt, I., Domack, E., d'Acoz, C. D., Dimmler, W., Gremare, A., et al. (2010). Biodiversity change after climate-induced ice-shelf collapse in the Antarctic. Deep-Sea Res. Pt. II. 58, 74–83. doi: 10.1016/j.dsr2.2010.05.024
Gutt, J., David, B., Isla, E., and Piepenburg, D. (2016). High environmental variability and steep biological gradients in the waters off the northern Antarctic Peninsula: Polarstern expedition PS81 (ANT-XXIX/3). Polar Biol. 39, 761–764. doi: 10.1007/s00300-016-1937-7
Gutt, J., Griffiths, H. J., and Jones, C. D. (2013). Circumpolar overview and spatial heterogeneity of Antarctic macrobenthic communities. Mar. Biodivers. 43, 481–487. doi: 10.1007/s12526-013-0152-9
Gutt, J., and Koltun, V. M. (1995). Sponges of the Lazarev and Weddell Sea, Antarctica: explanations for their patchy occurrence. Antarct. Sci. 19, 165–182. doi: 10.1017/S0954102007000247
Gutt, J., and Piepenburg, D. (2003). Scale-dependent impact on diversity of Antarctic benthos caused by groundings of icebergs. Mar. Ecol. Prog. Ser. 253, 77–83. doi: 10.3354/meps253077
Gutt, J., and Shickan, T. (1998). Epibiotic relationships in the Antarctic benthos. Antarct. Sci. 10, 398–405. doi: 10.1017/S0954102098000480
Gutt, J., Sirenko, B. I., Smirnov, I. S., and Arntz, W. E. (2004). How many macrozoobenthic species might inhabit the Antarctic shelf? Antarct. Sci. 16, 11–16. doi: 10.1017/S0954102004001750
Gutt, J., and Starmans, A. (1998). Structure and biodiversity of megabenthos in the Weddell and Lazarev Seas (Antarctica): ecological role of physical parameters and biological interactions. Polar Biol. 20, 229–247. doi: 10.1007/s003000050300
Gutt, J., Zurell, D., Bracegirdle, T. J., Cheung, W., Clark, M. S., Convey, P., et al. (2012). Correlative and dynamic species distribution modelling for ecological predictions in the Antarctic: a cross-disciplinary concept. Polar Res. 31:11091. doi: 10.3402/polar.v31i0.11091
Jansen, J., Hill, N. A., Dunstan, P. K., McKinlay, J., Sumner, M. D., Post, A. L., et al. (2018). Abundance and richness of key Antarctic seafloor fauna correlates with modelled food availability. Nat. Ecol. Evol. 2, 71–80. doi: 10.1038/s41559-017-0392-3
Jones, C. D., and Lockhart, S. J. (2011). Detecting vulnerable marine ecosystems in the Southern Ocean using research trawls and underwater imagery. Mar. Policy 35, 732–736. doi: 10.1016/j.marpol.2011.02.004
Jones, D. O. B., Bett, B. J., and Tyler, P. A. (2007). Depth-related changes to density, diversity, and structure of benthic megafaunal assemblages in the Fimbul ice shelf region, Weddell Sea, Antarctica. Polar Biol. 20, 1579–1592. doi: 10.1007/s00300-007-0319-6
Kaiser, S., Barnes, D. K. A., Sands, C. J., and Brandt, A. (2009). Biodiversity of an unknown Antarctic Sea: assessing isopod richness and abundance in the first benthic survey of the Amundsen continental shelf. Mar. Biodivers. 39. 27–43. doi: 10.1007/s12526-009-0004-9
Lampitt, R. S., Bett, B. J., Kiriakoulakis, K., Popova, E. E., Ragueneau, O., and Vangriesheim, A. (2001). Material supply to the abyssal seafloor in the northeast Atlantic. Prog. Oceanogr. 50, 27–63. doi: 10.1016/S0079-6611(01)00047-7
Lefcheck, J. S. (2016). piecewiseSEM: piecewise structural equation modelling in R for ecology, evolution, and systematics. Methods Ecol. Evol. 7, 573–579. doi: 10.1111/2041-210X.12512
Linse, K., Grant, S., Whittle, R., Reid, W., McKenzie, M., Federwisch, L., et al. (2020). Benthic seafloor images from Prince Gustav Channel and Duse Bay, Eastern Antarctic Peninsula, March 2018 (Version 1.0) [Data set]. UK Polar Data Centre, Natural Environment Research Council, UK Research and Innovation. doi: 10.5285/48DCEF16-6719-45E5-A335-3A97F099E451
Lockhart, S., and Hocevar, J. (2018). “Evidence of vulnerable marine ecosystems documented via submarine in the Antarctic Sound and Gerlache Strait (Subarea 48.1),” in CCAMLR WG-EMM-18/35 (Hobart, TAS).
Lockhart, S. J., and Jones, C. D. (2008). Biogeographic patterns of the benthic invertebrate megafauna on shelf areas within the Southern Ocean Atlantic sector. CCAMLR Sci. 15, 167–192.
Lovell, L. L., and Trego, K. D. (2003). The epibenthic megafaunal and benthic infaunal invertebrates of Port Foster, Deception Island (South Shetland Islands, Antarctica). Deep-Sea Res. Pt. II. 50, 1799–1819. doi: 10.1016/S0967-0645(03)00087-0
Muschenheim, D. K. (1987). The dynamics of near-bed seston flux and suspension-feeding benthos. J. Mar. Res. 45, 473–496. doi: 10.1357/002224087788401098
Neal, L., Linse, K., Brasier, M. J., Sherlock, E., and Glover, A. G. (2018). Comparative marine biodiversity and depth zonation in the Southern Ocean: evidence from a new large polychaete dataset from Scotia and Amundsen seas. Mar. Biodivers. 48, 581–601. doi: 10.1007/s12526-017-0735-y
Oksanen, J., Blanchet, F. G., Kindt, R., Legendre, P., O'Hara, R. B., Henry, M., et al. (2011). vegan: Community Ecology Package. R Package Version 1.17–10. Vienna: R Foundation for Statistical Computing.
Parker, S. J., and Bowden, D. A. (2010). Identifying taxonomic groups vulnerable to bottom longline fishing gear in the Ross Sea region. CCAMLR Sci. 17, 105–127.
Pineda-Metz, S. E. A., Gerdes, D., and Richter, C. (2020). Benthic fauna declined on a whitening Antarctic continental shelf. Nat. Commun. 11:2226. doi: 10.1038/s41467-020-16093-z
Post, A. L., Beaman, R. J., O'Brien, P. E., Eléaume, M., and Riddle, M. J. (2011). Community structure and benthic habitats across the George V Shelf, East Antarctica: trends through space and time. Deep-Sea Res. Pt. II. 58, 105–118. doi: 10.1016/j.dsr2.2010.05.020
Post, A. L., Lavoie, C., Domack, E. W., Leventer, A., Shevenell, A., and Fraser, A. D. (2017). Environmental drivers of benthic communities and habitat heterogeneity on an East Antarctic shelf. Antarct. Sci. 29, 17–32. doi: 10.1017/S0954102016000468
Post, A. L., Meuers, A. J. S., Fraser, A. D., Meiners, K. M., Ayers, J., Bindoff, N. L., et al. (2014). “Chapter 14. environmental setting.” in Biogeographic Atlas of the Southern Ocean. Cambridge: Scientific Committee on Antarctic Research, 46–64.
Post, A. L., O'Brien, P. E., Edwards, S., Carroll, A. G., Malakoff, K., Armand, L. K., et al. (2020). Upper slope processes and seafloor ecosystems on the Sabrina continental slope, East Antarctica. Mar. Geol. 422:e106091. doi: 10.1016/j.margeo.2019.106091
Quartino, M. L., Klöser, H., Schloss, I. R., and Wiencke, C. (2001). Biomass and associations of benthic marine macroalgae from the inner Potter Cove (King George Island, Antarctica) related to depth and substrate. Polar Biol. 24, 349–355. doi: 10.1007/s003000000218
Robert, K., Jones, D. O. B., Tyler, P. A., Van Rooij, D., and Huvenne, V. A. I. (2015). Finding the hotspots within a biodiversity hotspot: fine-scale biological predictions within a submarine canyon using high-resolution acoustic mapping techniques. Mar. Ecol. 36, 1256–1276. doi: 10.1111/maec.12228
Roberts, S., and Hirshfield, M. (2004). Deep-sea corals: out of sight, but no longer out of mind. Front. Ecol. Environ. 2, 123–130. doi: 10.1890/1540-9295(2004)002[0123:DCOOSB]2.0.CO;2
Smith, C. R., Mincks, S., and DeMaster, D. J. (2006). A synthesis of bentho-pelagic coupling on the Antarctic shelf: food banks, ecosystem inertia, and global climate change. Deep-Sea Res. Pt. II. 53, 875–894. doi: 10.1016/j.dsr2.2006.02.001
Starmans, A., Gutt, J., and Arntz, W. E. (1999). Mega-epibenthic communities in Arctic and Antarctic shelf areas. Mar. Biol. 135, 269–280. doi: 10.1007/s002270050624
Sumida, P. Y. G., Bernardino, A. F., Stedall, V. P., Glover, A. G., and Smith, C. R. (2008). Temporal changes in benthic megafaunal abundance and composition across the West Antarctic Peninsula shekf: results from video surveys. Deep-Sea Res. Pt. II. 55, 2465–2477. doi: 10.1016/j.dsr2.2008.06.006
Teschke, K., Beaver, D., Bester, M., Bombosch, A., Bornemann, H., Brandt, A., et al. (2016). Scientific Background Document in Support of the Development of a CCAMLR MPA in the Weddell Sea (Antarctica)–Version 2016–Part A: General Context of the Establishment of MPAs and Background Information on the Weddell Sea MPA Planning Area. WG-EMM-16/01, CCAMLR.
Thatje, S., Hillenbrand, C. D., and Larter, R. (2005). On the origin of Antarctic marine benthic community structure. Trends Ecol. Evol. 20, 534–540. doi: 10.1016/j.tree.2005.07.010
Thrush, S., Dayton, P., Cattaneo-Vietti, R., Chiantore, M., Cummings, V., Andrew, N., et al. (2006). Broad-scale factors influencing the biodiversity of coastal benthic communities of the Ross Sea. Deep-Sea Res. Pt. II. 53, 959–971. doi: 10.1016/j.dsr2.2006.02.006
Thrush, S. F., Hewitt, J. E., Cummings, V. J., Norkko, A., and Chiantore, M. (2010). β-diversity and species accumulation in Antarctic coastal benthos: influence of habitat, distance, and productivity on ecological connectivity. PLoS ONE 5:e11899. doi: 10.1371/journal.pone.0011899
Tucker, M. (1991). Sedimentary Petrology: An Introduction to the Origin of Sedimentary Rocks. Oxford: Blackwell Sciences Ltd.
Keywords: vulnerable marine ecosystem, habitat heterogeneity, benthic biodiversity, global climate change, ice shelf, marine protect area, Antarctic
Citation: Almond PM, Linse K, Dreutter S, Grant SM, Griffiths HJ, Whittle RJ, Mackenzie M and Reid WDK (2021) In-situ Image Analysis of Habitat Heterogeneity and Benthic Biodiversity in the Prince Gustav Channel, Eastern Antarctic Peninsula. Front. Mar. Sci. 8:614496. doi: 10.3389/fmars.2021.614496
Received: 06 October 2020; Accepted: 06 January 2021;
Published: 28 January 2021.
Edited by:
Wei-Jen Chen, National Taiwan University, TaiwanReviewed by:
Karine Olu, Institut Français de Recherche pour l'Exploitation de la Mer (IFREMER), FranceAnn Vanreusel, Ghent University, Belgium
Copyright © 2021 Almond, Linse, Dreutter, Grant, Griffiths, Whittle, Mackenzie and Reid. This is an open-access article distributed under the terms of the Creative Commons Attribution License (CC BY). The use, distribution or reproduction in other forums is permitted, provided the original author(s) and the copyright owner(s) are credited and that the original publication in this journal is cited, in accordance with accepted academic practice. No use, distribution or reproduction is permitted which does not comply with these terms.
*Correspondence: Peter M. Almond, cC5hbG1vbmRAbmV3Y2FzdGxlLmFjLnVr; William D. K. Reid, d2lsbGlhbS5yZWlkQG5ld2Nhc3RsZS5hYy51aw==